Corrigendum: The Internal, External and Extended Microbiomes of Hominins
- 1Department of Applied Ecology, North Carolina State University, Raleigh, NC, United States
- 2Centre for Evolutionary Hologenomics, The GLOBE Institute, University of Copenhagen, Copenhagen, Denmark
- 3Department of Anthropology, Northwestern University, Evanston, IL, United States
- 4Department of Biological Sciences, University of Notre Dame, Notre Dame, IN, United States
- 5Max Planck Institute for Evolutionary Anthropology, Leipzig, Germany
- 6Department of Anthropology, University of Nevada, Las Vegas, Las Vegas, NV, United States
The social structure of primates has recently been shown to influence the composition of their microbiomes. What is less clear is how primate microbiomes might in turn influence their social behavior, either in general or with particular reference to hominins. Here we use a comparative approach to understand how microbiomes of hominins have, or might have, changed since the last common ancestor (LCA) of chimpanzees and humans, roughly six million years ago. We focus on microbiomes associated with social evolution, namely those hosted or influenced by stomachs, intestines, armpits, and food fermentation. In doing so, we highlight the potential influence of microbiomes in hominin evolution while also offering a series of hypotheses and questions with regard to evolution of human stomach acidity, the factors structuring gut microbiomes, the functional consequences of changes in armpit ecology, and whether Homo erectus was engaged in fermentation. We conclude by briefly considering the possibility that hominin social behavior was influenced by prosocial microbes whose fitness was favored by social interactions among individual hominins.
Introduction
As part of an article collection on the drivers of sociality we were asked to consider the influence of hominin microbiomes on the evolution of hominin social behavior. As a starting point, we consider how large-scale physical, social, and behavioral changes that occurred during human evolution have (or might have) affected our interactions with microbes. We focus especially on the last six million years or so, starting from when we last shared a common ancestor with chimpanzees (Pan troglodytes) and bonobos (Pan paniscus), our last common ancestor (LCA), and before the industrial revolution (at which point many changes in human lifestyle appear to have begun to precipitate rapid changes in microbiomes). We use the word “hominins” to include all of the species after the split from the LCA, fossil species more closely related to human ancestors than chimpanzees or bonobos, and our own species, Homo sapiens. We use the word “hominids” to describe the broader lineage that includes the common ancestor of all great apes along with hominins.
Reconstructing the microbiomes of ancient hominins will ultimately rely on two main sources of data: (i) ancient microbial DNA from humans and non-human primates (Compton et al., 2013; Weyrich et al., 2017), and (ii) comparisons of modern genes, phenotypes and microbiota among humans, great apes, and other non-human primates, mammals and birds. Here we leverage the second of these sources to explore the complex interplay between human societies and behavior, microbiomes, and evolution. We consider four features of hominin bodies and lifestyles that have changed in the time since that LCA in ways that might both influence the microbiome and influence the effects of the microbiome on human social behavior. We begin with the stomach.
The Stomach
The stomach plays two key roles in mammals. One of those roles is in the degradation of protein (and, in some cases, chitin). This role has received disproportionate research attention (and is the focus, for example, in medical texts). The other role is as a kind of ecological filter, allowing some species into the intestines but not others. Like a bouncer at the door to the intestines’ microbial party, the stomach (acting as a filter) can be more or less restrictive. When very acidic, the stomach prevents most microbes ingested in food (apart from the most acid-tolerant) from arriving intact in the intestines. When more neutral, it allows most microbes through to the intestines alive. In primates that exclusively ingest fruits and leaves, the cost of allowing food borne microbes into the intestines is modest. Indeed, such microbes, in as much as they have already begun to degrade the food on which they are found, may be especially likely to aid in the breakdown of that food. This is particularly true in foregut fermenters (in which the fermentation chamber is the first chamber of the gut and serves as a gastrointestinal analog of the brewer’s tank; Figure 1). However, in omnivorous primates that include raw meat in their diets, the potential to ingest food borne pathogens is relatively great and hence the stomach might be expected to be more acidic (Ragir et al., 2000). In general, across mammals and birds, these patterns bear out. The more carnivorous an animal it is, the more likely its stomach is to be more acidic. But as can be seen in Figure 1, humans appear to be an outlier even within this schema. Humans have stomachs with a mean pH of 1.5 (Beasley et al., 2015). The extremely acidic stomachs of humans are unlike those of any other primate so far sampled, and find their closest analogs not in other primates but, instead, in the stomachs of vultures (which are similarly acidic) and potentially hyenas. No studies have documented the pH of hyena stomachs, but digestive anecdotes from hyena researchers (Christine Drea, pers. comm.) suggests hyenas have stomachs that are similar in acidity to vultures and humans. Vultures and hyenas have a good reason to have very acidic stomachs. They employ their stomachs as defenses against the bountiful food borne pathogens they ingest daily. Amazingly, however, while some vulture species, such as the white-backed vulture (Gyps africanus, pH of 1.2) have stomachs that are more acidic than those of humans, others actually have stomachs that are less acidic than humans. In the primate story, the stomachs of humans are unusual.
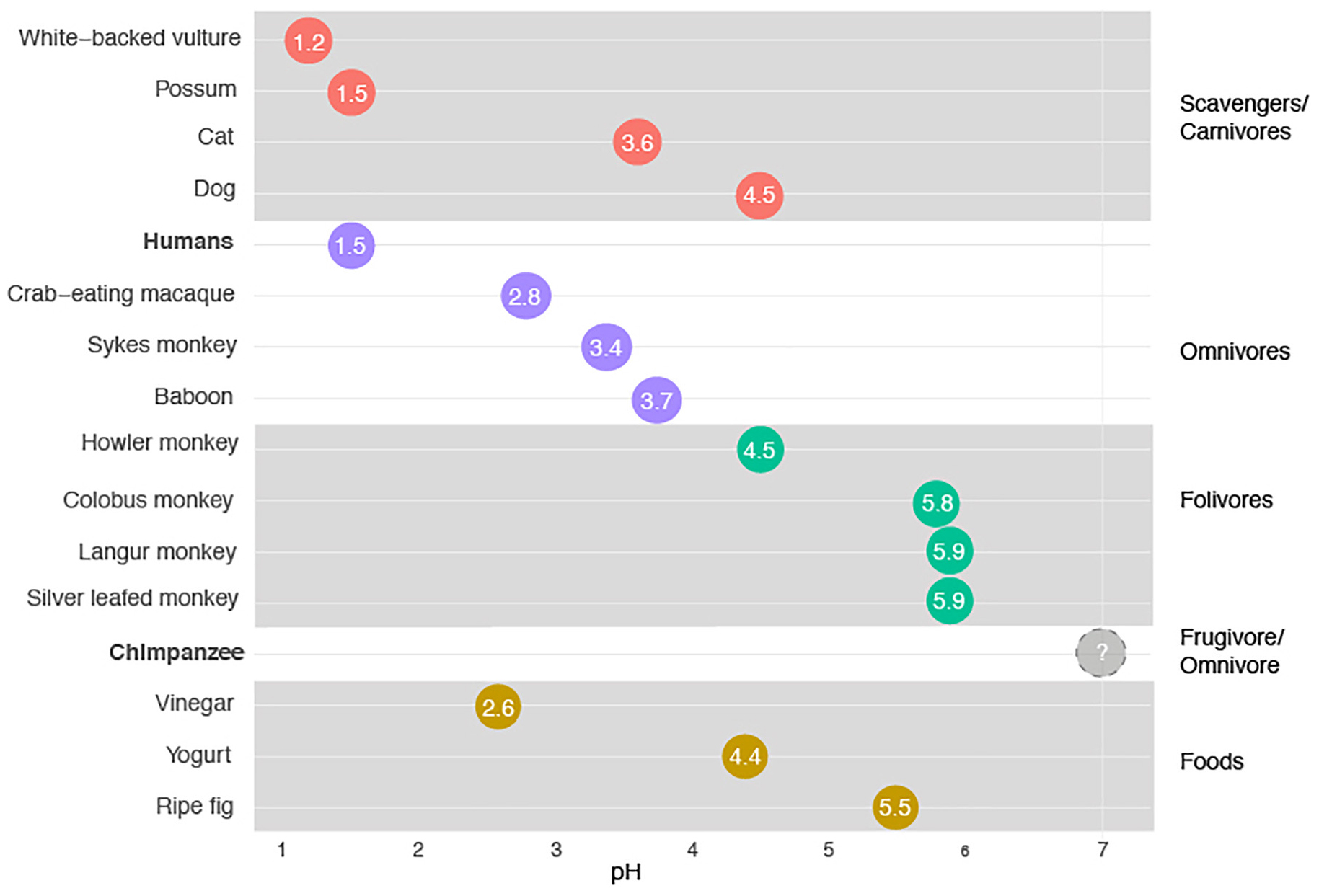
Figure 1. Stomach pH as a function of animal species. A pH of 7 is neutral. Data for chimpanzees come from a single laboratory study of a single individual chimpanzee. A sampling of acidic foods eaten by modern humans is included for reference.
The acidity of the human stomach poses two mysteries. The first relates to the timing of the origin of the acidity. The stomachs of chimpanzees and bonobos have been poorly studied, which is remarkable given the long history of the use of chimpanzees as laboratory animals. It is possible that the stomachs of chimpanzees and bonobos are like those of humans, very acidic (which might suggest that such acidity evolved in one of our common ancestors). That the stomachs of chimpanzees are (or can be) at least somewhat acidic is suggested by the observation that the bacteria species Helicobacter pylori more readily establishes in the stomachs of chimpanzees in the laboratory after they have been given antacids (Hazell et al., 1992), as is also the case in humans. If the stomachs of chimpanzees are as acidic as those of humans, one needs to explain why our common ancestors evolved acidic stomachs prior to six million years ago. On the other hand, the stomachs of chimpanzees might also be closer to neutral in pH, as is the case for other fruit eating primates. The truth is we don’t know enough to distinguish these possibilities yet. Two anecdotes, however, are intriguing. The first is the study of the stomach pH of a single captive chimpanzee. That chimpanzee is reported to have had a stomach pH that was approximately neutral (Brodie and Marshall, 1963). Several strong caveats exist, of course. The captive animals in the study were fed a processed primate mash, supplemented with vitamins, and lived in a captive environment completely dissimilar to the wild. Yet, despite these caveats the observation is of interest. The second anecdote relates to reports of dissections of recently dead, captive chimpanzees that note “yeast overgrowth,” in their stomachs (Migaki et al., 1982). Very few yeast species are able to grow in hyper-acidic environments. As a result, the observation is reconcilable with the idea that the stomachs of chimpanzees are not as acidic as those of humans.
We hypothesize that the stomachs of chimpanzees are likely somewhat acidic, but less so than those of humans. We also propose that the extreme acidity of human stomachs evolved after our split with the LCA with chimpanzees. If this is the case, it raises the question of what factors favored such acidity. One possible explanation is scavenging prey items abandoned by carnivores and/or the consumption of prey items too big to eat all at once. Chimpanzees in all habitats where they are found in the wild eat meat (Moore et al., 2017), as do bonobos (Wakefield et al., 2019), leading many to think that the LCA did as well. Sometimes the meat chimpanzees consumed Is scavenged (Nakamura et al., 2019) but relatively rarely (compared to other foods in their diet). More often the meat is eaten fresh from kills, though chimpanzees exhibit great variability between communities in success, technique, and seasonality of hunting behavior (Moore et al., 2017; Figure 2). Given that several chimpanzee communities target mammalian prey, and may do so using tools (Pruetz and Bertolani, 2007; Nakamura and Itoh, 2008), it is likely that species of Australopithecus, Homo habilis or Homo erectus also targeted and consumed meat, but also that how much meat they consumed, how fresh the meat was and how much was excess varied. While there is broad consensus among paleoanthropologists and evolutionary anthropologists that meat-eating played a role in the evolution of Homo, the relative importance of hunted and scavenged meat is contested. At least some of the meat that early hominins were eating was carrion (Pante et al., 2018). Some bones, for example, from the time during which H. erectus was extant, show evidence both of cut marks by stone tools and, in a layer beneath the cuts from those tools, tooth marks from hyenas (Blumenschine, 1995). The obvious inference is that such bones were scavenged by our ancestors after being killed by another mammal (maybe hyena, maybe something else). Any hominins that scavenged for prey before the advent of fire may have avoided food borne pathogens if their stomachs were acidic. As a result, it is possible that the acidity of the hominin stomach may have played a role in human foraging behavior and diet. That said, we note that the question of how much hominins scavenged, and how central it was to social evolution, is the subject of intense debate (Dominguez-Rodrigo and Pickering, 2017). An alternate (but not mutually exclusive) hypothesis is that acidic stomachs became advantageous once our ancestors began to hunt large prey. This might be expected if the meat from such a prey items was often more than could be eaten in a sitting such that meat was eaten later (after it had begun to rot) even though it had not been scavenged.
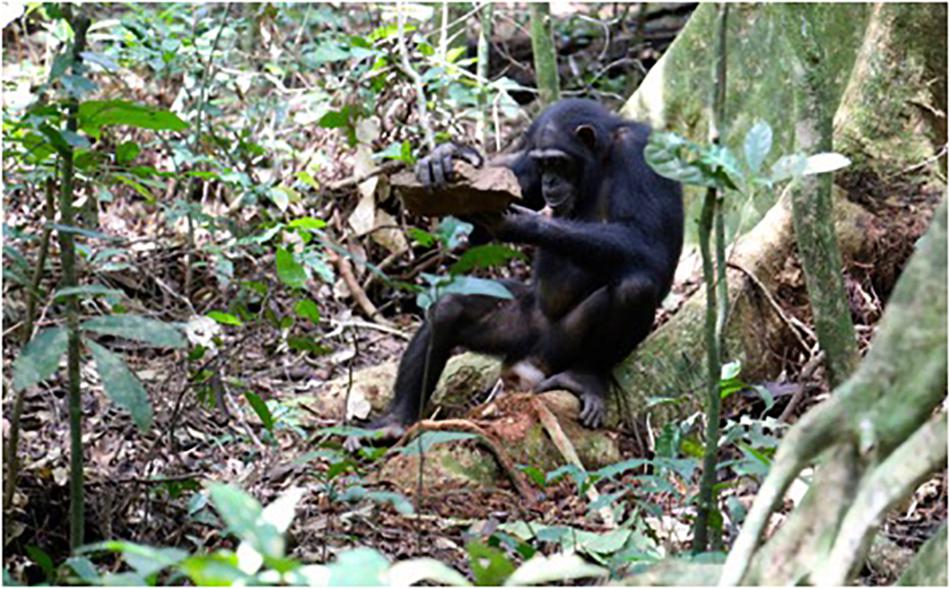
Figure 2. Chimpanzee pounding a nut with a stone hammer. Photo by Liran Samuni as part of the Tai Chimpanzee Project.
The Intestines
At some point in the last six million years, in addition to the potential changes in stomach acidity, the guts of our ancestors changed in other ways. The large intestine became shorter relative to the small intestine, while total intestine length also declined relative to body size. That this shift and shortening happened is suggested based on comparisons between the guts of chimpanzees, bonobos, and humans as well as the relatively smaller rib cage (and hence space available for the intestines) in the genus Homo compared to earlier hominin species (Aiello and Wheeler, 1995). However, it is worth noting that even within humans that the length of the large intestine varies even among individuals with similar genetic backgrounds. In one study of one hundred individuals, the shortest small intestine observed in any individual was half the length of the longest small intestine. Similarly, the ratio of small intestine to large intestine varied from 2.6 to 4.5. Given that gut morphology differs within populations of modern humans, it is possible (indeed likely) that variation among modern human populations is even greater (Underhill, 1955). To date no studies have considered such variation. The mean ratio of the small to large intestine length for chimpanzees is 1.0 (such that the chimpanzee large intestine is equal in length, on average) to the small intestine (Chivers and Hladik, 1984). But undoubtedly this value varies among chimpanzees as well, such that it is not inconceivable that some human populations and some chimpanzee populations actually have far more similar gut morphologies than tends to be assumed.
The shortening in the relative size of the human large intestine, whatever its consistency and magnitude, raises two questions: why the shortening occurred and what its consequences might have been for digestive physiology and the gut microbiome. In general there seems to be an emerging consensus that the use of tools, especially stick and stone kitchen tools of various sorts, to obtain and process foods made our ancestors less reliant on the fermentation that occurs in the large intestine. Cooking is likely one of the tools that our ancestors had at their disposal. Recent work has shown that cooking plant food reshapes the gut microbial environment (Carmody et al., 2019), suggesting that the use of fire, despite mixed evidence for its impact on starch digestibility (Schnorr et al., 2016), may have made nutrients in some types of food more available and also eased the chewing necessary to break down food (Wrangham, 2009). Fire may have also made it possible to smoke hives and therefore easier to harvest large quantities of honey with its easy to digest calories (which do not necessarily require gut microbes; Marlowe et al., 2014). In addition, fishing techniques and tools might have made fish and shellfish protein available which, even raw, is very easy to digest. Pounding tools, such as those employed by chimpanzees, would have made roots and tubers also easier to digest (Crittenden, 2016). Similar tools are used by many small-scale societies around the world, including contemporary subsistence foragers (Benito-Calvo et al., 2018) as well as by chimpanzees (and hence likely our LCA; Figure 2). All of this is to say that as our ancestors invented more kitchen implements they would have been able to pre-digest and pre-process some of their foods, allowing them to rely less on microbes in their guts to break down recalcitrant components of their diets, such as cellulose. They could get by with smaller guts and invest their bodily energy elsewhere, for example in big brains (an idea called the expensive tissue hypothesis; Aiello and Wheeler, 1995).
The shorter average large intestine length of species of Homo compared to those of their ancestors would have had at least two potential consequences for microbiomes. The shorter larger intestine would have sustained a smaller biomass of microbes relative to their body mass (simply because of the reduction in volume). In addition, the retention time of foods in the gut may have been reduced (Ragir et al., 2000). Some features of microbiomes, however, seem likely to have been similar between hominins and our LCA with chimpanzees despite changes in gross intestinal morphology. For example, the taxonomic classes of bacteria found in the guts of both chimpanzees and humans (from urban and rural settings) tend to overlap. What is more, the same families and genera of bacteria tend to occur in similar proportions (Moeller et al., 2012). This overlap is hypothesized to pre-date the human-chimpanzee split (and hence to be characteristic of our LCA). Furthermore, humans in small-scale, non-industrialized populations host a handful of microbial taxa that appear to be genetically equivalent to those in great apes at the level of operational taxanomic units (OTUs) or strains (Amato et al., 2019b). The same humans also share a range of bacterial metabolic pathways with other extant apes, including those involved in vitamin and amino acid synthesis. These results suggest that despite the reduction in length of the human intestines, enough physiological similarities remain between humans and apes such that the composition and function of their microbiomes is similar.
Nevertheless, despite these similarities, it is important to point out that the gut microbiomes of modern humans diverge in important ways from those of extant apes. These differences do not, however, appear to relate to gross morphological features of the gut but instead to diet. The gut microbiomes of humans, while similar to those of modern chimpanzees, appear to be even more similar to those of cercopithecine monkeys, such as baboons (genus Papio; Amato et al., 2019b; Figure 3). Differences in gut microbiome composition are greater between humans and apes (PERMANOVA F1,55 = 14.4, r2 = 0.21, p < 0.01) than between humans and cercopithecines (PERMANOVA F1,57 = 10.0, r2 = 0.15, p < 0.01). Differences in gut microbiome functional potential are similar between humans and apes (PERMANOVA F1,35 = 5.4, r2 = 0.16, p < 0.01) and humans and cercopithecines (PERMANOVA F1,35 = 7.4, r2 = 0.18, p < 0.01). While humans are genetically far more similar to chimpanzees than to baboons, baboons are more similar in diet (and habitat use) to ancestral Homo species than are chimpanzees. Baboons eat diets that are highly omnivorous and relatively high in starch content. Since the gut microbiome plays an important role in processing host dietary compounds, particularly resistant carbohydrates (and in some cases, specifically fibrous plant foods, see Schnorr et al., 2014) it is likely that the same microbial lineages and metabolic pathways nutritionally benefited both our hominin ancestors and extant cercopithecines. Given that the human shift toward habitats and diets like those of modern baboons are often linked to tool use, cooking, and ultimately, reductions in human intestinal length, it seems reasonable to suggest that this suite of changes altered the human gut microbiome. The result appears to be a “characteristic” human microbiome composed of both “ape” and “cercopithecine” traits.
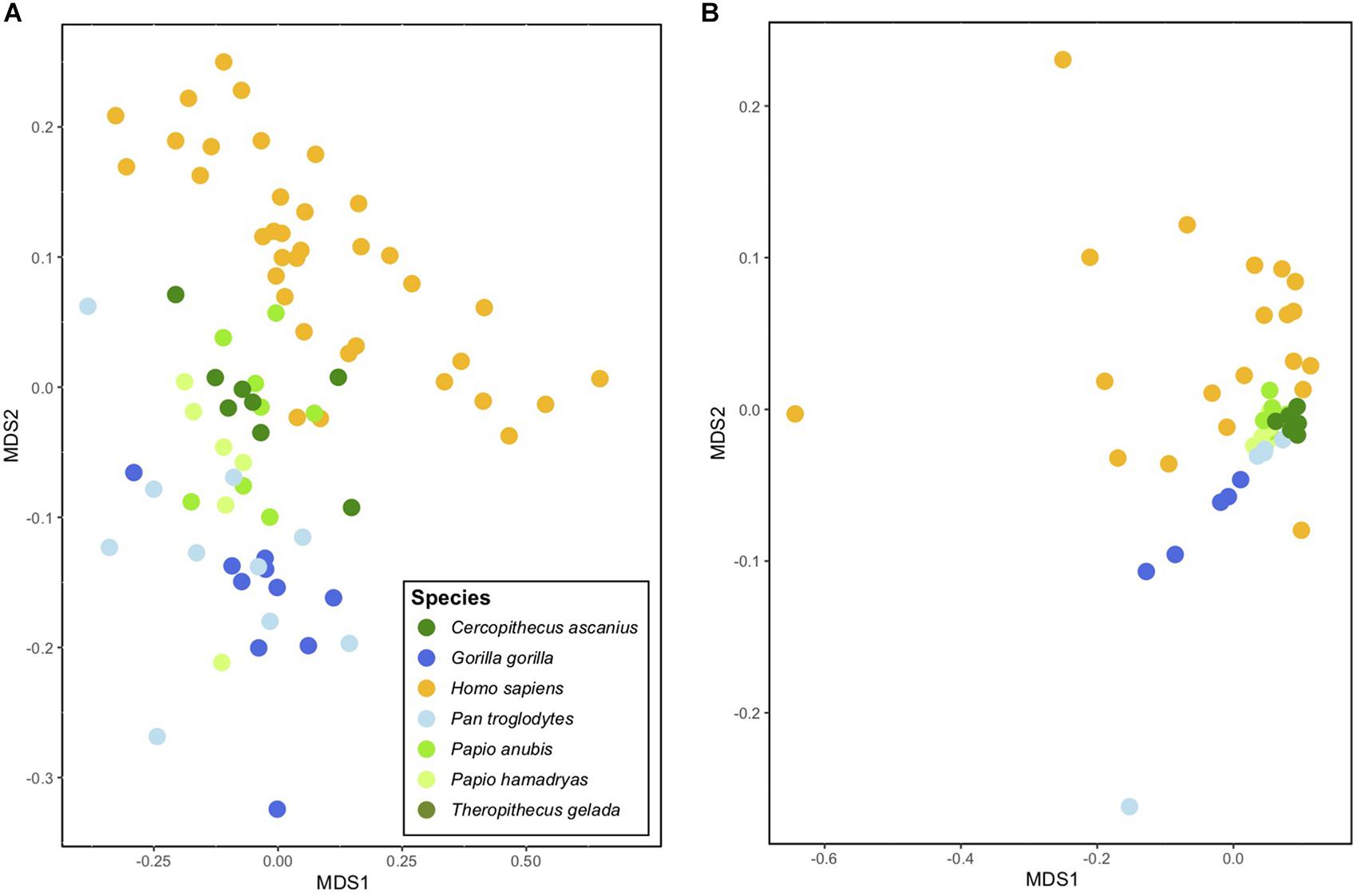
Figure 3. Ordination plot indicating similarities in the gut microbiomes of select primate species based on (A) taxonomic composition and (B) functional potential (data from Amato et al., 2019b). Each point represents the gut microbiome of a single individual, and clustering of points indicates similarity. Note that redtail monkeys (Cercopithecus ascanius) and baboons (Papio anubis and P. hamadryas) generally cluster closer to humans than do chimpanzees (Pan troglodytes) and gorillas (Gorilla gorilla) in both plots. Note also the relatively higher variation among individual humans (even with the small sample size included here) in functional bacterial genes (scatter of yellow points at right).
Beyond these “characteristic” human microbiome traits, however, substantial variation exists in the composition and function of the human microbiome. Whether or not this variation is associated with variation in gut length and more generally morphology is unknown, but at broad scales it correlates strongly with geography and lifestyle (Yatsunenko et al., 2012; Obregon-Tito et al., 2015; Gomez et al., 2019). This pattern suggests that the human gut microbiome has the potential to play a role in local adaptation. If local populations of H. erectus tended (as with modern baboons or chimpanzees) to be more likely to share microbes with each other than with geographically isolated populations, they might also be more likely to share microbes able to digest or detoxify the foods they were eating in a local region. Or perhaps they shared microbial taxa that increased resistance to endemic infectious diseases. Either way social life (and sharing microbes within social groups) might facilitate digestive plasticity in response to new conditions. More to the point, the social sharing of microbes, might have led to local microbial adaptations to environments, even without changes to host genomes. It has been recently shown is that in some modern human populations, but also other mammals, microbes can contribute to ecological niche differentiation and expansion. For instance, gut microbes enable woodrats to consume a diet high in tannins, allowing them to gain food resources inaccessible to mammals that do not have this gut microbial adaptation (Kohl et al., 2016). In modern humans, such microbial local adaptation appears to allow, for example, populations that consume a diet rich in seaweed to extract normally inaccessible complex carbohydrates (Hehemann et al., 2010). Fewer studies have examined microbiome adaptations to local infectious disease profiles, but one can imagine similar dynamics. And whatever these effects they will ultimately be (and have been) strongly influenced by the social behavior of hominins.
‘We hypothesize that microbially facilitated local adaptations were critical to the human evolutionary trajectory. A defining feature of the first humans (be they H. habilis or H. erectus) was the extent to which they moved, which happened in two ways. First, early members of the genus Homo roamed the African landscape bipedally. In doing so, they confronted more food choices than had their ancestors. An abundance of carbon isotope data show a variability in diet including plants such as grasses and sedges, as well as the animals who consume these plants (Sponheimer and Dufour, 2009). Further, the manufacture of stone tools and their uses for targeting both plant foods as well as terrestrial and aquatic animals is well documented (Braun et al., 2010; Lemorini et al., 2014), suggesting that our early ancestors enjoyed a diet far more diverse than our great ape counterparts. Second, H. erectus, a very successful hominin by all accounts, moved into new geographic areas. H. erectus would eventually arrive as far north as Spain and as far east as China. In doing so, H. erectus used a diversity of approaches to eat: in different places, different foods, and utilizing a variety of different tools, both as reflection of what was available in those environments but, by analogy to modern chimpanzees (Figure 4), probably also due to differences in culinary culture. It was also likely exposed to novel disease landscapes. A plastic microbiome that could shift rapidly both within and across individuals and populations could have facilitated dietary diversity by contributing key metabolic pathways to maximize nutritional output from a range of foods and may have also increased the ability of H. erectus to endure new diseases (Amato et al., 2019a). While we cannot assess H. erectus microbiomes directly, modern human microbiomes exhibit more inter-individual variation compared to closely related non-human primates (Schnorr et al., 2016; Amato et al., 2019b).
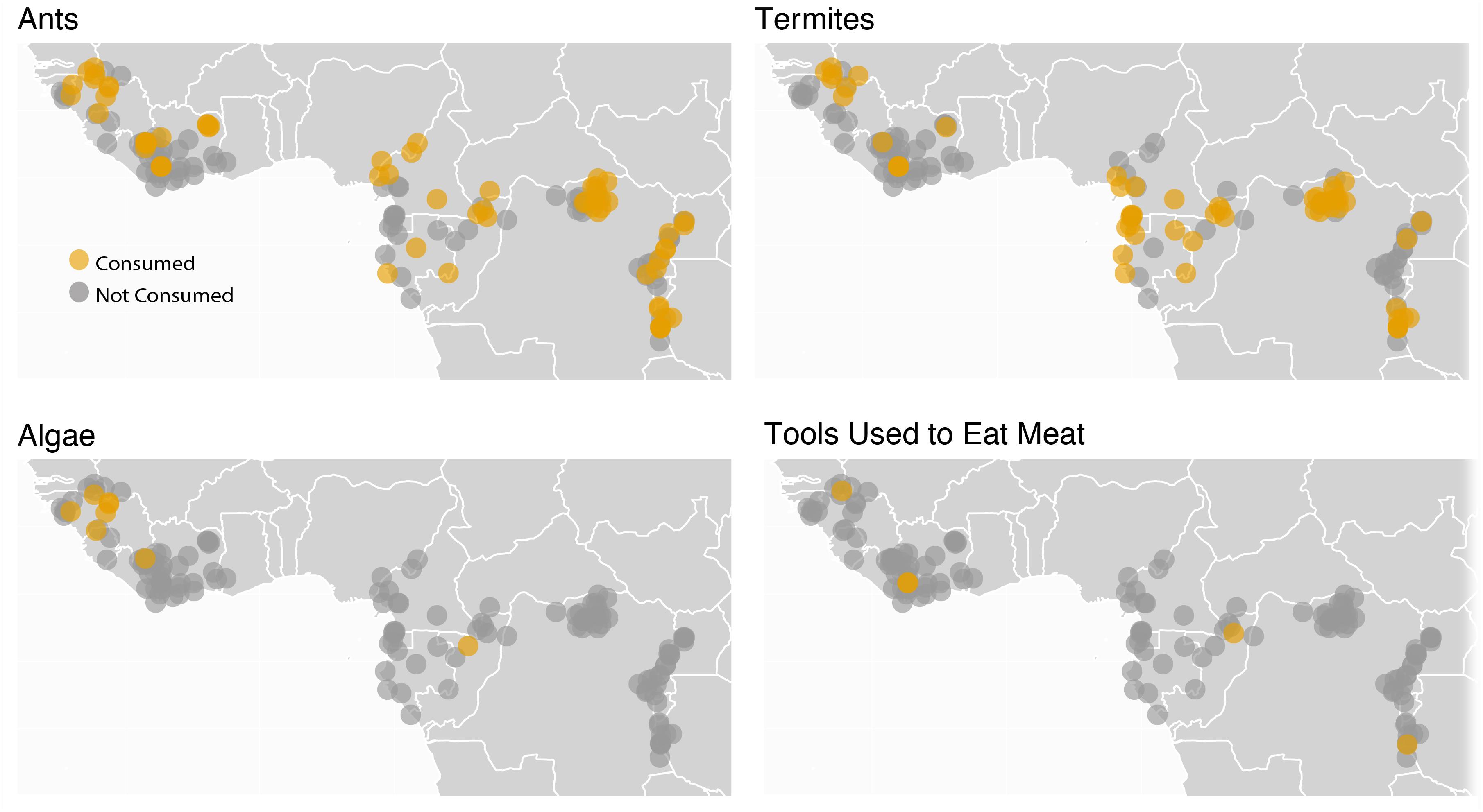
Figure 4. The variation in diets among H. erectus populations is likely to have exceeded that of any hominin that lived previously, both because of the diversity of tools used by H. erectus and because of its large geographic range. However, this is not to say other primates do not also vary in their diets geographically. The map above shows sites at which chimpanzees have and have not been observed feeding on ants, termites, algae, and meat using tools (data from Kühl et al., 2019), one measure of chimpanzee dietary diversity. It is of note that the animal species chimpanzees use tools to eat differ among communities even in cases in which the environment does not differ, due to chimpanzee culinary cultures. For example, the chimpanzees at Gombe in Tanzania use tools to eat driver ants (Dorylus spp.) and acrobat ants (Crematogaster spp.), but the chimpanzees at nearby Mahale in Tanzania (Nishida and Hiraiwa, 1982), where both driver ants and acrobat ants are present, use tools to feed on carpenter ants (Camponotus spp.).
It is reasonable to imagine that this microbiome diversity is tied directly to the vast dietary and pathogen exposure diversity represented by humans globally, which began to emerge with H. erectus. Additional research is necessary to explore this idea further. Ancient DNA studies may allow some insights. Some insight might also be garnered from the study of modern chimpanzee populations, especially given that the tool use and culinary cultures of chimpanzees differ greatly among communities (Figure 4). We can predict that if microbiome composition was associated with dietary differences among H. erectus populations that the same should also be true among extant chimpanzee populations. This has yet to be tested, but is testable. Assuming local microbial adaptations facilitated human dietary niche expansion and subsequent human success in a range of environments around the world, human social structures likely played an important role in establishing and maintaining geographically specialized microbiomes.
Skin
Human bodies have several kinds of “sweat” glands. One kind, eccrine sweat glands, is associated with evaporative cooling. However, humans also have a second important kind of “sweat” glands, apocrine glands. In some non-humans, such as camels (genus Camelus), the primary function of apocrine glands is to produce sweat and to function in the way that eccrine glands function in humans (Folk and Semken, 1991). But in humans and apes apocrine glands appear to play other roles. They are located primarily in armpits (and to a much lesser extent around the genitals and anus), where they collectively form what have been termed axillary organs (Ellis and Montagna, 1962). In extant hominids (humans and living apes) the apocrine glands produce a white, milky substance that feeds slow-growing bacteria species living in the glands themselves and on the surface of the skin. It is these bacteria that are responsible for the main body odors associated both with the armpits and the genitals (Shelley et al., 1953).
It is thought that the primary function of apocrine glands in primates is to help convey chemical signals among individuals within a species. Aroma wicks up the hair associated with the apocrine glands (which in gorillas, chimpanzees and humans has a different morphology than does ordinary body hair; Weiss, 2009) and travels to the noses of conspecifics, much as occurs (whether one likes it or not) between one human and another in, say, a crowded elevator. The key question is just what the aromas produced by apocrine glands convey. Of course, they might convey different types of information depending on species and context. In lemurs, aromas from apocrine glands can signal individual identity (“It is me!”; Scordato et al., 2007), as well as relatedness (“I am not your brother.”; Charpentier et al., 2008). In Western lowland gorillas (Gorilla gorilla gorilla), the same seems possible, in as much as humans are able to distinguish the aroma of individual gorillas (Hepper and Wells, 2010), and one imagines that gorillas are better at distinguishing among gorillas than are humans. Because apocrine glands produce their secretion in response to stressful situations and arousal, the aromas produced by the bacteria in these glands might also signal fear, arousal or stress. Finally, the products of the glands include both proteins and fats and where nutrients are scarce must be relatively expensive to the host (Zeng et al., 1992). Some aromas might thus be reliable signals that an individual is sufficiently well-fed to produce apocrine substances, and hence also healthy. Conversely, unusual apocrine aromas might reliably signal infection or poor nutrition. In this framing, armpit aromas might be one hominid equivalent of a peacock tail (as has been suggested to be the case for similar glands in some lemur species; Walker-Bolton and Parga, 2017). Realistically, however, the social role of these organs, while likely to have been important in our ancestors, has been poorly considered. We know both that human armpit odors and microbes tend to be different than those of chimpanzees and gorillas, but also very variable among humans (Council et al., 2016; Figure 5). This variation is intriguing in light of the discovery of the influence of the ABCC11 gene on the apocrine glands.
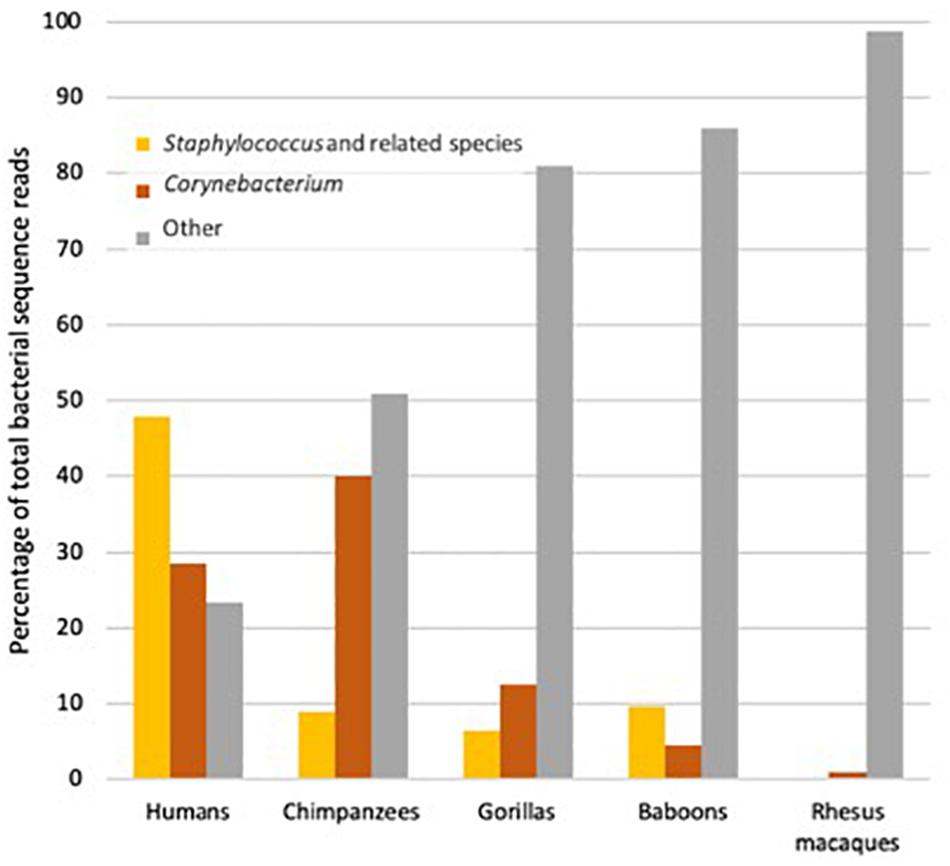
Figure 5. The proportion of bacterial reads in samples of armpits of humans (Homo sapiens), chimpanzees (Pan troglodytes), gorillas (Gorilla gorilla) and two monkey species, Hamadryas baboons (Papio hamadryas), and Rhesus macaques (Macaca mulatta) that were from species of slow-growing, odor-producing Corynebacterium, weedy fast-growing species of Staphylococcus and relatives or other taxa (Data from Council et al., 2016).
One variant of the ABCC11 gene, with a single nucleotide substitution, is much more common in some human populations than in others, particularly east Asian populations but also in some populations from cold habitats (Ohashi et al., 2010). This version of the gene is noticeable for two reasons. In the homozygous form it produces dry earwax (ear wax is also produced by an apocrine gland, albeit a highly modified one). In addition, that same variant leads to apocrine glands in the armpit that produce very little secretion. As a result, individuals with this variant of the ABCC11 gene have microbes that are very different from those with the ancestral variant. This single nucleotide substitution explains much of the variation in skin microbe compositions among individual humans (with additional variation explained by whether or not individuals use antiperspirant; Urban et al., 2016). The effect of ABCC11 is seen not just in the armpit but also more generally (Coyle, 2018). What is most remarkable is that this single nucleotide substitution, which arose roughly forty thousand years ago appears to have been under extraordinarily strong selection in temperate Asia, for reasons that remain enigmatic but could relate to the ways in which changes in human social systems impacted the value of the odors being produced by armpit microbes to human survival (Ohashi et al., 2010).
If we are to target for study bacterial taxa on the skin that might have been influenced by or influence hominin social behavior for further study, bacteria of the genus Corynebacterium are of interest, but so too are those of the genus Staphylococcus. Species of Staphyloccoccus have long been thought to be the medically normal beneficial skin bacteria of humans, bacteria able to help defend the skin against pathogens and perform other functions. Attempts were even (successfully) made to innoculate the skin of newborn babies with particular strains of Staphylococcus bacteria so as to ward off pathogens (see history reviewed in Dunn, 2018). However, the study of the skin bacteria of other primates suggests that the dominance of Staphylococcus bacteria on human skin is unusual (Figure 3). This raises the question of when Staphylococcus bacteria began to dominate human skin microbiomes. One intriguing observation was made in a recent study by Ashley Ross and colleagues of skin bacteria across mammals. The study found that Staphylococcus was a minor player in the skin microbiota of most mammals. However, there were exceptions. Staphylococcus was the most common genus of bacteria on the skin of some wild sheep (Ammotragus lervia), goats, cows (all of which are domesticated animals or relatives of domesticated animals) and humans. This observation raises the possibility that skin Staphylococcus spread among humans and domesticated animals during cohabitation and, in doing so, changed the dominant skin microbial taxa. One can imagine a scenario in which the dominance of this bacteria across both humans and domesticates then also facilitated domestication in as much as it would increase the similarity of the aroma of domestic animals to humans and vice versa. As cohabitation with domesticated animals became more common, and human communities benefited from these associations, this may have led to the further spread of Staphylococcus dominated skin communities. On the other hand, it may be revealed that the skin microbes of zoo animals are unusual and record recent sharings of taxa and, in doing so, obscure ancient ones.
The Extended Microbiome
Recent research has highlighted the role of food processing in human evolution and the evolution of human sociality (Henrich, 2017). Food processing has a potentially large impact in as much as it reduces the calories needed for digestion, and the amount of chewing necessary for a given food item (Zink and Lieberman, 2016). In addition, food processing is thought to lead to an increased probability of the use of key sites on the landscape as home bases. The types of food processing that have received the most attention to date have been those that chimpanzees undertake, such as the pounding of nuts and seeds (Boesch and Boesch, 1983), the use of sticks to access tubers, insects, and even animal prey, and roots (Bogart and Pruetz, 2008). Additional types of processing that our ancestors may have engaged in included the use of fire to cook food, the use of fire and smoke to calm bees (Crittenden, 2011), and in doing so allow the extraction of large quantities of honey and the use of new kinds of tools to cut into, dismember and divide meat, whether from animals that have been killed or those that have been scavenged. Some of these forms of food processing involve microbes to a degree, but typically as supporting characters. For example, one of the advantages of cooking is that it kills off potential pathogens in meat. Another is that it makes nutrients in tubers and roots more available to microbes in the large intestine (and easier for them to metabolize). But at some point microbes began to play a more central role, once humans began to actively control fermentation.
The use of technologies to control rot allowed humans to begin to favor microbes with traits that were desirable. Those traits might include aromas, flavors, acids or alcohol (as well as nutritional properties that these attributes might portend). Simultaneously, fermentation allowed other microbes to be disfavored, thanks to the allelopathic effects of alcohol, acids and other products of fermentation. Finally, fermentation could enrich certain vitamins in foods and begin the process of processing (ultimately, digesting) food such that more nutrients would be available (Speth, 2017).
The timing of the first controlled fermentation by humans is unknown. It is possible that H. erectus fermented foods. Some fermentations require vessels, but not all. Food can be fermented in animal stomachs (Frink and Giordano, 2015). In addition, food can be fermented by submerging it in slow-moving streams or by burying it underground. Many carnivore species ferment food. In hot regions, Hyenas appear to store (and to some extent ferment) food items by putting them in water (Selvaggio, 1998). In cold regions, foxes and other carnivores store and ferment foods by burying them (Vander Wall and Smith, 1987). All of this is to say that neither technical nor intellectual barriers would have prevented H. erectus or their relatives from fermenting at least some kinds of foods. Recently Speth has suggested that Neanderthals may have fermented meat and nothing in his argument precludes far earlier uses of fermentation (Speth, 2017). In as much as fermentation requires very little in terms of persistent tools, it is difficult to know what archeological evidence would support (or refute) the idea that H. erectus or later hominins fermented foods, or to estimate the timing of the first fermented foods. The evidence that does exist relates to two genetically encoded human traits, those associated with sour taste receptors and those associated with the enzyme alcohol dehydrogenase and its function.
In nearly all primates that have been studied to date, even slightly acidic foods are perceived as sour and aversive. In general, it is thought that sour taste receptors evolved in mammals so as to lead them away from foods (be they fruit or meat) that had begun to rot due to the presence of lactic acid bacteria or acetic acid bacteria or fruits that were unripe and hence might contain plant defensive compounds. In studies to date, there appear to be only two or three primate species that respond differently to sour foods. Night monkeys (genus Aotus), which forage in the dark and so must smell foods as much as they see them, are able to detect acidic substances and perceive them as sour. Additionally, unlike most other non-human primates, unless these foods are highly acidic, night monkeys perceive them as pleasant (Glaser and Hobi, 1985). The other clear exception is humans. Adult humans, like night monkeys, perceive slightly acidic foods as pleasant and can learn to enjoy even very acidic foods; Liem and De Graaf, 2004; Breslin, 2013. Therefore, at the moment, our picture of sour taste preference is one in which two lineages, that of night monkeys and that of our own species, evolved a preference for sour foods. This portrait of the past is obviously heavily contingent on how poorly studied sour taste has been in primate species. It is possible the preference for sour tastes is more common than is so far appreciated. For example, Toshisada Nishida found that a relatively high proportion of the fruits ingested by the Mahale chimpanzees tasted sour to him (Nishida et al., 2000), such that it seems plausible that the Mahale chimpanzees enjoy such fruits (whether they be sour due to unripeness or rot). While it is possible that the chimpanzees have learned to enjoy sour fruits rather than innately enjoy them, it seems less likely. Assuming that both chimpanzees and humans innately prefer sour foods, it is reasonable to hypothesize that the preference occurred in our common ancestor. Unfortunately, the workings of and genes associated with sour taste receptors have not yet been well characterized (although see Montell, 2018). Regardless, once our ancestors evolved a preference for sour foods it would have been much easier to learn to control fermentation in as much as one of the key products of fermentations (acids) tasted pleasant.
A second evolutionary change that certainly influenced the ways in which our ancestors fermented foods is the evolution of alcohol dehydrogenase. Alcohol dehydrogenase facilitates the first key step in the breakdown of alcohol, yielding toxic acetaldehyde that must be further degraded. While the other genes in this pathway have yet to be explored, humans and apes possess a variant of the alcohol dehydrogenase gene that is forty times more efficient than that of almost all other primates (Carrigan et al., 2015). Given that ethanol is a necessary byproduct of the fermentation process, changes in human alcohol dehydrogenase are often linked to human consumption of fermented foods, particularly fermented beverages. Recent reconstructions, however, suggest that this gene evolved roughly ten million years ago, in line with what might be expected if it evolved when early apes began to spend more time on the ground and began to encounter and consume fermented fallen fruit that was more ethanol-rich than ripe fruit picked directly from trees (Carrigan et al., 2015). Some modern chimpanzee communities (like modern humans) enjoy a tipple, and even make tools with which to access alcohol (Hockings et al., 2015). The same may well have also been true of our common ancestors.
Regardless of when fermented food use first emerged in the human lineage though, the fermentation process ultimately allowed our ancestors to begin to store food (and also to stay in one place for more time). It would have facilitated the persistence of larger groups of individuals living together. In addition, it set up a potential feedback. Individuals who fermented foods very often relied upon bodily microbes to do so. Sometimes they were microbes found on the bodies of insects, as is the case with brewer’s yeast (Madden et al., 2018). In other cases, they were microbes associated with human or other mammal bodies. Modern examples of the latter include the use of salivary microbial communities to initiate the fermenting process in chicha production in Peru, and similar fermented beverages around the world (Freire et al., 2016), the use of skin microbes to produce bodily aromas in some semi-soft cheeses (Pham et al., 2017), the use of body associated Lactobacillus species in sourdough breads (Gänzle and Ripari, 2016) or the use of the ancestrally mouth-associated bacteria species, Streptococcus thermophilus, in the production of yogurt (Goh et al., 2011). Once they began using body and other microbes to ferment foods, our ancestors extended their genomes and ultimately their phenotypes in much the way that beavers do in building a dam that yields a pond (Carthey et al., 2018). More specifically, by co-opting body microbes, they extended their guts, allowing digestion to begin to happen where food was fermented. Furthermore, when those food items were and are consumed, they can re-inoculate consumers, becoming even more common within the communities of individuals that rely upon them. For individuals who ate together, this would have been a mechanism through which microbes and microbial genes within groups became more similar than between groups. As a result, the complex dynamics of social networks interacting with microbes would intensify in these contexts.
Prosocial Microbes
Ultimately, what we are left with in regard to the potential influence of microbes on the evolution of hominins is a sketch. It is a rough sketch, subject to revision. And it is a sketch based on what we can observe today. We conclude by considering, even more speculatively, what might be. More specifically, we consider the possibility that some of the microbes associated with hominin microbiomes (be they those of stomachs, intestines, skin, the extended microbiome, or other microbiomes such as the breast milk microbiome or the vaginal microbiome, which differs greatly among primate species; Miller et al., 2016) might have directly favored particular kinds of social behavior and, in as much, account for two of the major social transitions in hominins: the transition to larger more sedentary populations and the transition to urban living.
As we have already noted, human control over microbial populations might have facilitated such transitions (e.g., by allowing food storage and turning pathogenic water into non-pathogenic booze). In turn, could microbes have controlled human behavior? Recently, a number of microbes have been shown to control the social behavior of their hosts in ways that increase microbial fitness. The eukaryote, Salpingoeca rosetta, for instance, can lead a solitary or multicellular lifestyle. The transition between these two lifestyles is mediated by lipids produced by a Algoriphagus machipongonensis, its bacterial commensal. In other words, the products of the microbiome of this eukaryote determine whether or not it is social (Woznica et al., 2016). Or consider leaf cutter ants such as the species Acromyrmex echinatior. Leaf cutter ants, like most social insects, recognize each other on the basis of their cuticular hydrocarbons. Those cuticular hydrocarbons are produced, in part, by bacteria on the exoskeletons of the ants (perhaps a phenomenon not so very different than apes recognizing each other on the basis of their armpit odors). If the ants are treated with antibiotics, their nestmates attack them (Teseo et al., 2019). In considering the evolution of hominins, these examples raise the question: is it possible that at critical junctures in hominin social evolution that some microbes were favored by social interactions and evolved in association with hominin populations? If such microbes lived persistently on or with their host (and so were disadvantaged by the death of the host) and spread human to human through social interactions, they might increase their fitness if they caused their hosts to behave more socially, live in larger groups and interact more frequently. It is now well-documented that the malaria parasite can influence its hosts so as to make transmission more likely (by making hosts more attractive to vector mosquitoes; De Moraes et al., 2014). It doesn’t seem much more outlandish to imagine a microbe (be it a species of bacteria, fungus, protist or even virus) that would make its own spread more likely by making humans more social. In concluding with this example, we pose the question to the field of how we might even look for such a microbe. One might argue that the spread of yeast strains that produce more alcohol represents a relatively recent example of such a scenario (in which alcohol producing yeasts lead us to addiction, drunken social interactions and the desire for more products of such alcohol producing yeasts). But we can’t yet preclude far more ancient influences of microbes on the ways in which we interact.
Data Availability Statement
All data are from previously published papers (Yatsunenko et al., 2012; Beasley et al., 2015; Clemente et al., 2015; Obregon-Tito et al., 2015; Smits et al., 2017; McDonald et al., 2018; Amato et al., 2019c; Kühl et al., 2019) and are available through NCBI as Bioproject PRJNA281417, https://science.sciencemag.org/content/suppl/2019/03/06/science.aau4532.DC1 and from the EBI with the following Project IDs: ERP104379 (Amato et al., 2018), ERP008799 (Clemente et al., 2016), ERP008694 (Clemente et al., 2016), ERP014589 (Obregon-Tito et al., 2016), PRJEB3079 (Yatsunenko et al., 2016), ERP109605 (Smits et al., 2018), and ERP012803 (McDonald et al., 2016). They are also publicly available on Qiita (Gonzalez et al., 2018) with the following Qiita IDs: 11212, 10052, 1448, 850, 11358, and 10317.
Author Contributions
All authors listed have made a substantial, direct and intellectual contribution to the work, and approved it for publication.
Funding
RD and LN were supported while writing this manuscript by funds from the Max Planck Institute for Evolutionary Anthropology in Leipzig, Germany and a sabbatical fellowship from the German Centre for Integrative Biodiversity Research (iDiv). KA was supported as a fellow of the CIFAR “Humans and the Microbiome” program.
Conflict of Interest
The authors declare that the research was conducted in the absence of any commercial or financial relationships that could be construed as a potential conflict of interest.
Acknowledgments
We are thankful to Christine Drea for insights about the food storage behaviors of hyenas. Special thanks are also due to the organizers of the Wenner-Gren Symposium #160 “Cultures of Fermentation.” Discussions at the symposium greatly improved this manuscript.
References
Aiello, L. C., and Wheeler, P. (1995). The expensive-tissue hypothesis: the brain and the digestive system in human and primate evolution. Curr. Anthropol. 36, 199–221. doi: 10.1086/204350
Amato, K. R., Jeyakumar, T., Poinar, H., and Gros, P. (2019a). Shifting climates, foods, and diseases: the human microbiome through evolution. Bioessays 41:1900034. doi: 10.1002/bies.201900034
Amato, K. R., Mallott, E. K., McDonald, D., Dominy, N. J., Goldberg, T., Lambert, J. E., et al. (2019b). Convergence of human and old world monkey gut microbiomes demonstrates the importance of human ecology over phylogeny. Genome Biol. 20:201. doi: 10.1186/s13059-019-1807-z
Amato, K. R., Sanders, J., Song, S. J., Nute, M., Metcalf, J. L., Thompson, L. R., et al. (2018). Evolutionary trends in host physiology outweigh dietary niche in structuring primate gut microbiomes. EBI European Nucleotide Archive Available at: https://www.ebi.ac.uk/ena/data/view/PRJEB22679
Amato, K. R., Sanders, J., Song, S. J., Nute, M., Metcalf, J. L., Thompson, L. R., et al. (2019c). Evolutionary trends in host physiology outweigh dietary niche in structuring primate gut microbiomes. ISME J. 13, 576–587. doi: 10.1038/s41396-018-0175-0
Beasley, D. E., Koltz, A. M., Lambert, J. E., Fierer, N., and Dunn, R. R. (2015). The evolution of stomach acidity and its relevance to the human microbiome. PLoS One 10:e0134116. doi: 10.1371/journal.pone.0134116
Benito-Calvo, A., Crittenden, A. N., Livengood, S. V., Sánchez-Romero, L., Martínez-Fernández, A., and de la Torre, I. (2018). 3D 360 surface morphometric analysis of pounding stone tools used by Hadza foragers of Tanzania: a new methodological approach for studying percussive stone artefacts. J. Archaeol. Sci. Rep. 20, 611–621. doi: 10.1016/j.jasrep.2018.06.003
Blumenschine, R. J. (1995). Percussion marks, tooth marks, and experimental determinations of the timing of hominid and carnivore access to long bones at FLK Zinjanthropus, Olduvai Gorge, Tanzania. J. Hum. Evol. 29, 21–51. doi: 10.1006/jhev.1995.1046
Boesch, C., and Boesch, H. (1983). Optimisation of nut-cracking with natural hammers by wild chimpanzees. Behavioure 83, 265–286. doi: 10.1163/156853983x00192
Bogart, S. L., and Pruetz, J. D. (2008). Ecological context of savanna chimpanzee (Pan troglodytes verus) termite fishing at Fongoli, Senegal. Am. J. Primatol. Off. J. Am. Soc. Primatol. 70, 605–612. doi: 10.1002/ajp.20530
Braun, D. R., Harris, J. W. K., Levin, N. E., McCoy, J. T., Herries, A. I. R., Bamford, M. K., et al. (2010). Early hominin diet included diverse terrestrial and aquatic animals 1.95 Ma in East Turkana, Kenya. Proc. Natl. Acad. Sci. U.S.A. 107, 10002–10007. doi: 10.1073/pnas.1002181107
Breslin, P. A. S. (2013). An evolutionary perspective on food and human taste. Curr. Biol. 23, R409–R418.
Brodie, D. A., and Marshall, R. W. (1963). Gastric content of fasted primates: a survey. Science 141, 174–175. doi: 10.1126/science.141.3576.174
Carmody, R. N., Bisanz, J. E., Bowen, B. P., Maurice, C. F., Lyalina, S., and Louie, K. B. (2019). Cooking shapes the structure and function of the gut microbiome. Nat. Microbiol. 4, 2052–2063.
Carrigan, M. A., Uryasev, O., Frye, C. B., Eckman, B. L., Myers, C. R., and Hurley, T. D. (2015). Hominids adapted to metabolize ethanol long before human-directed fermentation. Proc. Natl. Acad. Sci. U.S.A. 112, 458–463. doi: 10.1073/pnas.1404167111
Carthey, A. J., Gillings, M. R., and Blumstein, D. T. (2018). The extended genotype: microbially mediated olfactory communication. Trends Ecol. Evol. 33, 885–894. doi: 10.1016/j.tree.2018.08.010
Charpentier, M. J., Boulet, M., and Drea, C. M. (2008). Smelling right: the scent of male lemurs advertises genetic quality and relatedness. Mol. Ecol. 17, 3225–3233. doi: 10.1111/j.1365-294X.2008.03831.x
Chivers, D. J., and Hladik, C. M. (1984). “Diet and gut morphology in primates,” in Food acquisition and processing in primates, eds D. J. Chivers, B. A. Wood, and A. Bilsborough, (Boston, MA: Springer), 213–230. doi: 10.1007/978-1-4757-5244-1_9
Clemente, J. C., Pehrsson, E. C., Blaser, M. J., Sandhu, K., Gao, Z., Wang, B., et al. (2015). The microbiome of uncontacted Amerindians. Sci Adv. 1:e1500183. doi: 10.1126/sciadv.1500183
Clemente, J. C., Pehrsson, E. C., Blaser, M. J., Sandhu, K., Gao, Z., Wang, B., et al. (2016). The microbiome of uncontacted Amerindians. EBI European Nucleotide Archive. Available at: https://www.ebi.ac.uk/ena/data/view/PRJEB7825
Compton, A. A., Malik, H. S., and Emerman, M. (2013). Host gene evolution traces the evolutionary history of ancient primate lentiviruses. Philos. Transact. R. Soc. B Biol. Sci. 368:20120496. doi: 10.1098/rstb.2012.0496
Council, S. E., Savage, A. M., Urban, J. M., Ehlers, M. E., Skene, J. P., Platt, M. L., et al. (2016). Diversity and evolution of the primate skin microbiome. Proc. R. Soc. B Biol. Sci. 283:20152586. doi: 10.1098/rspb.2015.2586
Coyle, K. P. (2018). Recent Evolution of Host Genetic Control of Microbiota in Cichlid Fishes and Humans. Doctoral dissertation, North Carolina State University, Raleigh, NC.
Crittenden, A. N. (2011). The importance of honey consumption in human evolution. Food Foodways 19, 257–273. doi: 10.1080/07409710.2011.630618
Crittenden, A. N. (2016). “Ethnobotany in evolutionary perspective: wild plants in diet composition and daily use among Hadza hunter-gatherers,” in Plants in the Hominin and Pre-agrarian Human Worlds, eds K. Hardy, and L. Kubiak-Martens, (Oxford: Oxbow Books).
De Moraes, C. M., Stanczyk, N. M., Betz, H. S., Pulido, H., Sim, D. G., Read, A. F., et al. (2014). Malaria-induced changes in host odors enhance mosquito attraction. Proc. Natl. Acad. Sci. U.S.A. 111, 11079–11084. doi: 10.1073/pnas.1405617111
Dominguez-Rodrigo, M., and Pickering, T. R. (2017). The meat of the matter: an evolutionary perspective on human carnivory. Azania Archaeol. Res. Afr. 52, 4–32. doi: 10.1080/0067270x.2016.1252066
Dunn, R. R. (2018). Never Home Alone: From Microbes to Millipedes, Camel Crickets, and Honeybees, the Natural History of Where we Live. New York, NY: Basic Books.
Ellis, R. A., and Montagna, W. (1962). The skin of primates. VI. The skin of the gorilla (Gorilla gorilla). Am. J. Phys. Anthropol. 20, 79–93. doi: 10.1002/ajpa.1330200210
Folk, G. E., and Semken, A. (1991). The evolution of sweat glands. Int. J. Biometeorol. 35, 180–186. doi: 10.1007/bf01049065
Freire, A. L., Zapata, S., Mosquera, J., Mejia, M. L., and Trueba, G. (2016). Bacteria associated with human saliva are major microbial components of Ecuadorian indigenous beers (chicha). PeerJ 4:e1962. doi: 10.7717/peerj.1962
Frink, L. and Giordano, C. (2015). Women and subsistence food technology: the Arctic seal poke storage system. Food Foodways 23, 251–272. doi: 10.1080/07409710.2015.1099906
Gänzle, M., and Ripari, V. (2016). Composition and function of sourdough microbiota: from ecological theory to bread quality. Int. J. Food Microbiol. 239, 19–25. doi: 10.1016/j.ijfoodmicro.2016.05.004
Glaser, D., and Hobi, G. (1985). Taste responses in primates to citric and acetic acid. Int. J. Primatol. 6, 395–398. doi: 10.1007/bf02736385
Goh, Y.J., Goin, C., O’Flaherty, S., Altermann, E. and Hutkins, R. (2011). Specialized adaptation of a lactic acid bacterium to the milk environment: the comparative genomics of Streptococcus thermophilus LMD-9. Microb. Cell Fact. 10(Suppl. 1):S22.
Gomez, A., Sharma, A. K., Mallott, E. K., Petrzelkova, K. J., Robinson, C. A., Yeoman, C. J., et al. (2019). Plasticity in the human gut microbiome defies evolutionary constraints. mSphere 4:e00271-19. doi: 10.1128/mSphere.00271-19
Gonzalez, A., Navas-Molina, J. A., Kosciolek, T., McDonald, D., Vazquez-Baeza, Y., Ackermann, G., et al. (2018). Qiita: rapid, web-enabled microbiome meta-analysis. Nat. Methods 15, 796–798. doi: 10.1038/s41592-018-0141-9
Hazell, S. L., Eichberg, J. W., Lee, D. R., Alpert, L., Evans, D. G., Evans, D. J., et al. (1992). Selection of the chimpanzee over the baboon as a model for Helicobacter pylori infection. Gastroenterology 103, 848–854. doi: 10.1016/0016-5085(92)90016-r
Hehemann, J. H., Correc, G., Barbeyron, T., Helbert, W., Czjzek, M., and Michel, G. (2010). Transfer of carbohydrate-active enzymes from marine bacteria to Japanese gut microbiota. Nature 464, 908–912. doi: 10.1038/nature08937
Henrich, J. (2017). The Secret of Our Success: How Culture Is Driving Human Evolution, Domesticating Our Species, and Making Us Smarter. Princeton, NJ: Princeton University Press.
Hepper, P. G., and Wells, D. L. (2010). Individually identifiable body odors are produced by the gorilla and discriminated by humans. Chem. Senses 35, 263–268. doi: 10.1093/chemse/bjq015
Hockings, K. J., Bryson-Morrison, N., Carvalho, S., Fujisawa, M., Humle, T., McGrew, W. C., et al. (2015). Tools to tipple: ethanol ingestion by wild chimpanzees using leaf-sponges. R. Soc. Open Sci. 2:150150. doi: 10.1098/rsos.150150
Kohl, K. D., Stengel, A., and Dearing, M. D. (2016). Inoculation of tannin-degrading bacteria into novel hosts increases performance on tannin-rich diets. Environ. Microbiol. 18, 1720–1729. doi: 10.1111/1462-2920.12841
Kühl, H. S., Boesch, C., Kulik, L., Haas, F., Arandjelovic, M., Dieguez, P., et al. (2019). Human impact erodes chimpanzee behavioral diversity. Science 363, 1453–1455. doi: 10.1126/science.aau4532
Lemorini, C., Plummer, T. W., Braun, D. R., Crittenden, A. N., Ditchfield, P. W., Bishop, L. C., et al. (2014). Old stones’ song: use-wear experiments and analysis of the Oldowan quartz and quartzite assemblage from Kanjera South (Kenya). J. Hum. Evol. 72, 10–25. doi: 10.1016/j.jhevol.2014.03.002
Liem, D. G., and De Graaf, C. (2004). Sweet and sour preferences in young children and adults: role of repeated exposure. Physiol. Behav. 83, 421–429. doi: 10.1016/j.physbeh.2004.08.028
Madden, A. A., Epps, M. J., Fukami, T., Irwin, R. E., Sheppard, J., Sorger, D. M., et al. (2018). The ecology of insect–yeast relationships and its relevance to human industry. Proc. R. Soc. B Biol. Sci. 285:20172733. doi: 10.1098/rspb.2017.2733
Marlowe, F. W., Berbesque, J. C., Wood, B., Crittenden, A., Porter, C., and Mabulla, A. (2014). Honey, Hadza, hunter-gatherers, and human evolution. J. Hum. Evol. 71, 119–128. doi: 10.1016/j.jhevol.2014.03.006
McDonald, D., Hyde, E., Debelius, J. W., Morton, J. T., Gonzalez, A., Ackermann, G., et al. (2016). American Gut: an open platform for citizen science microbiome research. EBI European Nucleotide Archive. Available at: https://www.ebi.ac.uk/ena/data/view/PRJEB11419
McDonald, D., Hyde, E., Debelius, J. W., Morton, J. T., Gonzalez, A., Ackermann, G., et al. (2018). American Gut: an open platform for citizen science microbiome research. mSystems 3:e00031-18. doi: 10.1128/mSystems.00031-18
Migaki, G., Schmidt, R. E., Toft, J. D., and Kaufmann, A. F. (1982). Mycotic infections of the alimentary tract of nonhuman primates: a review. Vet. Pathol. Suppl. 19, 93–103. doi: 10.1177/030098588201907s07
Miller, E. A., Beasley, D. E., Dunn, R. R., and Archie, E. A. (2016). Lactobacilli dominance and vaginal pH: why is the human vaginal microbiome unique? Front. Microbiol. 7:1936. doi: 10.3389/fmicb.2016.01936
Moeller, A. H., Degnan, P. H., Pusey, A. E., Wilson, M. L., Hahn, B. H., and Ochman, H. (2012). Chimpanzees and humans harbour compositionally similar gut enterotypes. Nat. Commun. 3:1179. doi: 10.1038/ncomms2159
Montell, C. (2018). pHirst sour taste channels pHound? Science 359, 991–992. doi: 10.1126/science.aas9772
Moore, J., Black, J., Hernandez-Aguilar, R. A., Idani, G., Piel, A., and Stewart, F. (2017). Chimpanzee vertebrate consumption: Savanna and forest chimpanzees compared. J. Hum. Evol. 112, 30–40. doi: 10.1016/j.jhevol.2017.09.004
Nakamura, M., Hosaka, K., Itoh, N., Matsumoto, T., Matsusaka, T., Nakazawa, N., et al. (2019). Wild chimpanzees deprived a leopard of its kill: implications for the origin of hominin confrontational scavenging. J. Hum. Evol. 131, 129–138. doi: 10.1016/j.jhevol.2019.03.011
Nakamura, M., and Itoh, N. (2008). Hunting with tools by Mahale chimpanzees. Pan Afr. News 15, 3–6. doi: 10.5134/143489
Nishida, T., and Hiraiwa, M. (1982). Natural history of a tool-using behavior by wild chimpanzees in feeding upon wood-boring ants. J. Hum. Evol. 11, 73–99. doi: 10.1016/s0047-2484(82)80033-x
Nishida, T., Ohigashi, H., and Koshimizu, K. (2000). Tastes of chimpanzee plant foods. Curr. Anthropol. 41, 431–438. doi: 10.1086/300149
Obregon-Tito, A. J., Tito, R. Y., Metcalf, J., Sankaranarayanan, K., Clemente, J. C., Ursell, L. K., et al. (2015). Subsistence strategies in traditional societies distinguish gut microbiomes. Nat. Commun. 6:6505. doi: 10.1038/ncomms7505
Obregon-Tito, A. J., Tito, R. Y., Metcalf, J., Sankaranarayanan, K., Clemente, J. C., Ursell, L. K., et al. (2016). Subsistence strategies in traditional societies distinguish gut microbiomes. EBI European Nucleotide Archive. Available at: https://www.ebi.ac.uk/ena/data/view/PRJEB13051
Ohashi, J., Naka, I., and Tsuchiya, N. (2010). The impact of natural selection on an ABCC11 SNP determining earwax type. Mol. Biol. Evol. 28, 849–857. doi: 10.1093/molbev/msq264
Pante, M. C., Njau, J. K., Hensley-Marschand, B., Keevil, T. L., Martín-Ramos, C., Franco Peters, R., et al. (2018). The carnivorous feeding behavior of early Homo at HWK EE, Bed II, Olduvai Gorge, Tanzania. J. Hum. Evol. 120, 215–235. doi: 10.1016/j.jhevol.2017.06.005
Pham, N. P., Layec, S., Dugat-Bony, E., Vidal, M., Irlinger, F., and Monnet, C. (2017). Comparative genomic analysis of Brevibacterium strains: insights into key genetic determinants involved in adaptation to the cheese habitat. BMC Genomics 18:955. doi: 10.1186/s12864-017-4322-1
Pruetz, J. D., and Bertolani, P. (2007). Savanna chimpanzees, Pan troglodytes verus, hunt with tools. Curr. Biol. 17, 412–417. doi: 10.1016/j.cub.2006.12.042
Ragir, S., Rosenberg, M., and Tierno, P. (2000). Gut morphology and the avoidance of carrion among chimpanzees, baboons, and early hominids. J. Anthropol. Res. 56, 477–512. doi: 10.1086/jar.56.4.3630928
Schnorr, S. L., Candela, M., Rampelli, S., Centanni, M., Consolandi, C., Basaglia, G., et al. (2014). Gut microbiome of the Hadza hunter-gatherers. Nat. Commun. 5:3654. doi: 10.1038/ncomms4654
Schnorr, S. L., Crittenden, A. N., and Henry, A. G. (2016). Impact of brief roasting on starch gelatinization in whole foods and implications for plant food nutritional ecology in human evolution. Ethnoarchaeology 8, 30–56. doi: 10.1080/19442890.2016.1150629
Scordato, E. S., Dubay, G., and Drea, C. M. (2007). Chemical composition of scent marks in the ringtailed lemur (Lemur catta): glandular differences, seasonal variation, and individual signatures. Chem. Senses 32, 493–504. doi: 10.1093/chemse/bjm018
Selvaggio, M. M. (1998). The archaeological implications of water-cached hyena kills. Curr. Anthropol. 39, 380–383. doi: 10.1086/204750
Shelley, W. B., Hurley, H. J., and Nichols, A. C. (1953). Axillary odor: experimental study of the role of bacteria, apocrine sweat, and deodorants. Ama Arch. Dermatol. Syphilol. 68, 430–446.
Smits, S. A., Leach, J., Sonnenburg, E. D., Gonzalez, C. G., Lichtman, J. S., Reid, G., et al. (2017). Seasonal cycling in the gut microbiome of the Hadza hunter-gatherers of Tanzania1. Science 357, 802–806. doi: 10.1126/science.aan4834
Smits, S. A., Leach, J., Sonnenburg, E. D., Gonzalez, C. G., Lichtman, J. S., Reid, G., et al. (2018). Seasonal cycling in the gut microbiome of the Hadza hunter-gatherers of Tanzania1. EBI European Nucleotide Archive. Available at: https://www.ebi.ac.uk/ena/data/view/PRJEB27517
Speth, J. D. (2017). Putrid meat and fish in the Eurasian middle and upper Paleolithic: are we missing a key part of Neanderthal and modern human diet? PaleoAnthropology 2017, 44–72.
Sponheimer, M., and Dufour, D. L. (2009). “Increased dietary breadth in early hominin evolution: revisiting arguments and evidence with a focus on biogeochemical contributions,” in The Evolution of Hominin Diets, eds J. J. Hublin, and M. P. Richards, (Dordrecht: Springer), 229–240. doi: 10.1007/978-1-4020-9699-0_18
Teseo, S., van Zweden, J. S., Pontieri, L., Kooij, P. W., Sørensen, S. J., Wenseleers, T., et al. (2019). The scent of symbiosis: gut bacteria may affect social interactions in leaf-cutting ants. Anim. Behav. 150, 239–254. doi: 10.1016/j.anbehav.2018.12.017
Underhill, B. M. L. (1955). Intestinal length in man. Br. Med. J. 2, 1243–1246. doi: 10.1136/bmj.2.4950.1243
Urban, J., Fergus, D. J., Savage, A. M., Ehlers, M., Menninger, H. L., Dunn, R. R., et al. (2016). The effect of habitual and experimental antiperspirant and deodorant product use on the armpit microbiome. PeerJ 4:e1605. doi: 10.7717/peerj.1605
Vander Wall, S. B., and Smith, K. G. (1987). “Cache-protecting behavior of food-hoarding animals,” in Foraging Behavior, eds A. C. Kamil, J. R. Krebs, and H. R. Pulliam, (Boston, MA: Springer), 611–644. doi: 10.1007/978-1-4613-1839-2_22
Wakefield, M. L., Hickmott, A. J., Brand, C. M., Takaoka, I. Y., Meador, L. M., Waller, M. T., et al. (2019). New observations of meat eating and sharing in wild bonobos (Pan paniscus) at Iyema, Lomako Forest Reserve, Democratic Republic of the Congo. Folia Primatol. 90, 179–189. doi: 10.1159/000496026
Walker-Bolton, A. D., and Parga, J. A. (2017). Stink flirting” in ring-tailed lemurs (Lemur catta): male olfactory displays to females as honest, costly signals. Am. J. Primatol. 79:e22724. doi: 10.1002/ajp.22724
Weyrich, L. S., Duchene, S., Soubrier, J., Arriola, L., Llamas, B., Breen, J., et al. (2017). Neanderthal behaviour, diet, and disease inferred from ancient DNA in dental calculus. Nature 544, 357–361. doi: 10.1038/nature21674
Woznica, A., Cantley, A. M., Beemelmanns, C., Freinkman, E., Clardy, J., and King, N. (2016). Bacterial lipids activate, synergize, and inhibit a developmental switch in choanoflagellates. Proc. Natl. Acad. Sci. U.S.A. 113, 7894–7899. doi: 10.1073/pnas.1605015113
Yatsunenko, T., Rey, F. E., Manary, M. J., Trehan, I., Dominguez-Bello, M. G., Contreras, M., et al. (2012). Human gut microbiome viewed across age and geography. Nature 486, 222–227. doi: 10.1038/nature11053
Yatsunenko, T., Rey, F. E., Manary, M. J., Trehan, I., Dominguez-Bello, M. G., Contreras, M., et al. (2016). Human gut microbiome viewed across age and geography. EBI European Nucleotide Archive. Available at: https://www.ebi.ac.uk/ena/data/view/PRJEB3079
Zeng, X. N., Leyden, J. J., Brand, J. G., Spielman, A. I., McGinley, K. J., and Preti, G. (1992). An investigation of human apocrine gland secretion for axillary odor precursors. J. Chem. Ecol. 18, 1039–1055. doi: 10.1007/BF00980061
Keywords: fermentation, primates, prosocial microbes, feces, food, armpits, alcohol
Citation: Dunn RR, Amato KR, Archie EA, Arandjelovic M, Crittenden AN and Nichols LM (2020) The Internal, External and Extended Microbiomes of Hominins. Front. Ecol. Evol. 8:25. doi: 10.3389/fevo.2020.00025
Received: 01 November 2019; Accepted: 28 January 2020;
Published: 19 February 2020.
Edited by:
Peter H. W. Biedermann, Julius Maximilian University of Würzburg, GermanyReviewed by:
Dan Vanderpool, Indiana University Bloomington, United StatesChristina Warinner, Harvard University, United States
Copyright © 2020 Dunn, Amato, Archie, Arandjelovic, Crittenden and Nichols. This is an open-access article distributed under the terms of the Creative Commons Attribution License (CC BY). The use, distribution or reproduction in other forums is permitted, provided the original author(s) and the copyright owner(s) are credited and that the original publication in this journal is cited, in accordance with accepted academic practice. No use, distribution or reproduction is permitted which does not comply with these terms.
*Correspondence: Robert R. Dunn, rrdunn@ncsu.edu