- 1Department of Entomology, College of Agriculture and Natural Resources, Michigan State University, East Lansing, MI, United States
- 2Department of Osteopathic Medical Specialties, Michigan State University, East Lansing, MI, United States
- 3Ecology, Evolutionary Biology and Behavior Program, Michigan State University, East Lansing, MI, United States
- 4Department of Biological Sciences, College of Science, University of Notre Dame, Notre Dame, IN, United States
Resource subsidies affect nutrient cycling, species interactions, and food webs in ways that influence ecosystem structure and function, but their effects depend on the history, magnitude, and recurrence frequency of the subsidies. In aquatic ecosystems, plant detritus has been considered the predominant form of such subsidies; however, while considered less abundant in many ecosystems, carrion represents subsidies with relatively rapid turnover and highly concentrated nutrient and energy release that can have strong and lasting effects on ecosystems. Carrion subsidies can be both autochthonous or allochthonous, and come in the form of natural senescence or disease-related non-consumptive mortality, phenology-based programed death (e.g., salmon spawning and death), or stochastic and episodic events (e.g., mass fish die-offs). All aquatic ecosystems have some level of non-consumptive mortality that provides a background level of carcasses to aquatic ecosystems, while others have a natural history of carrion resource subsidies (e.g., natural salmon-bearing streams), and some have only recently been exposed to phenology-based carrion subsidies (e.g., anthropogenic salmon introductions around the world). Many aquatic ecosystems experience episodic subsidies in the form of unexpected mass mortalities (e.g., eutrophication-, disease-, or climate-related mass die-offs) or have seasonally dependent pulses, like that of marine or lake snow in the form of zooplankton carcasses. The responses of ecosystems to these different histories and frequencies of carrion subsidies have often been independently investigated, with little effort to compare and bridge research boundaries in the broader context of resource subsidies. In this review, we provide a synthesis of how pulsed carrion nutrient and energy subsidies have widespread and lasting impacts on many aquatic ecosystems. We do this with a synthesis of literature from freshwater and marine ecosystems along three themes of how carrion is produced and decomposes: autochthonous and allochthonous necromass; phenology-based mortality; and stochastic and episodic mass mortality subsidies. Studies of charismatic megafauna carrion (e.g., whales) have described significant impacts in deep ocean systems, but much less is understood for other groups of animals. Quantifying the energy, nutrient, and foodweb effects of carrion is needed for more species and among habitats to more fully understand how ubiquitous forms of necromass contribute to aquatic ecosystem structure and function.
Introduction to Necromass Resource Subsidies in Aquatic Ecosystems
Background
Decaying organic matter, or necromass, comes in the form of both plant and animal biomass, recently differentiated as autotrophically (e.g., leaf litter) and heterotrophically (e.g., carrion) derived biomass, respectively, and is recycled back into ecosystems by the necrobiome community (Benbow et al., 2019). While both forms of necromass are considered important to ecosystems, plant detritus processing has historically dominated research as the main energy pathway in aquatic ecosystems (Vannote et al., 1980; Webster and Benfield, 1986; Getz, 2011; Boyero et al., 2016), especially for lotic systems lacking anadromous fish populations (Moore et al., 2004; Gessner et al., 2010; Benbow et al., 2019). Until recently (McDowell et al., 2017; Subalusky et al., 2017; DuBose et al., 2019; Wenger et al., 2019), aquatic studies of animal necromass (carrion) have largely focused on fish carcass decomposition (Richey et al., 1975; Garman, 1992; Schindler, 1992), or lake/marine snow represented by zooplankton carcasses in the water column of lentic ecosystems (Alldredge and Silver, 1988; Grossart and Simon, 1998; Giering et al., 2014). Some research has addressed the long-term decomposition of whale (and other vertebrate) falls in the oceanic abyss (Allison et al., 1991; Bennett et al., 1994; Smith and Baco, 2003; Kemp et al., 2006; Higgs et al., 2014). Much of past research on carrion in aquatic ecosystems has focused on species that are large in size, have anthropogenic importance (e.g., salmon runs), or elicit public intrigue (e.g., whale falls); however, a large portion of aquatic ecosystem necromass comes in the form of smaller organisms (e.g., phytoplankton, zooplankton, and invertebrates) with fast generation times and high turnover rates that substantially contribute to ecosystem production (Waters, 1977; Benke, 1998; Huryn and Wallace, 2000; Landry and Calbet, 2004; Patrick et al., 2019). While we acknowledge the importance of phototrophically derived necromass in aquatic ecosystems and the associated trophic relationships (Little and Altermatt, 2018), we direct interested readers to the many extensive reviews and empirical research of such resources ranging from phytoplankton, seaweed, and macrophytes to large wood debris (Lindeman, 1942; Mann, 1969; Wallace et al., 1999; Moore et al., 2004; Entrekin et al., 2009; Tank et al., 2010).
For this review, we focus on heterotrophically derived necromass (from bacteria to whales) with emphasis on animal carrion, and also acknowledge that dung, frass, and other forms of animal tissue (e.g., gametes) contribute to the larger resource pool (Subalusky et al., 2015; Dutton et al., 2018; Subalusky and Post, 2018; Benbow et al., 2019). For an informative argument for the importance of egested heterotrophically derived forms of particulate organic matter to energy budgets of pelagic zones of lake ecosystems we direct readers to Wetzel (1995).
In general, the importance of carrion has been qualitatively considered relatively greater in large lakes and oceans (Alldredge and Silver, 1988; Smith and Baco, 2003; Tang et al., 2014) compared to shallow wetlands and marshes (Brinson et al., 1981; Duggins et al., 1989). However, Subalusky and Post (2018) discuss how recipient ecosystem qualities (e.g., productivity or trophic structure) are important for understanding cross-system effects of carrion. In watersheds, carrion has been largely studied as pulsed resource subsidies like fish die-offs (Parmenter and Lamarra, 1991) or as part of programed phenologically driven semelparous death like salmon life cycles (Moore et al., 2004; Benbow et al., 2019). Regardless of origin, most organic matter ultimately decomposes and is recycled in ecosystems (Wetzel, 1995; Moore et al., 2004; Benbow et al., 2019), providing an intimate connection between ecosystem structure and function through interacting necrobiome species responsible for the breakdown (physical destruction into smaller and smaller units) and decomposition (biochemical alteration and conversion) of necromass (Lindeman, 1942; Putman, 1983). This process of decomposition, or turnover rate, is also known to be much faster for carrion compared to plant litter biomass, and is more important to ecosystems than once considered (Barton et al., 2019).
Precedent From Terrestrial Ecosystems
Most studies of carrion ecology have been conducted in terrestrial ecosystems. Here we provide examples from terrestrial habitats to show the potential of carrion to ecosystems, which can potentially be transferred to aquatic conditions. In terrestrial systems, carrion has often been thought to contribute marginally to ecosystem energetics (Swift et al., 1979; Barton et al., 2019). However, Barton et al. (2019) argue that because of the high turnover rate of carrion, carcasses have historically gone understudied since they are quickly recycled back into the ecosystem and are hidden from observation; thus, they have been presumed to have a negligible contribution to energy and nutrient flow in ecosystems. This view is plausible because it has been historically difficult to quantify natural rates of carrion decomposition in ecosystems, and so the relative production of energy from carrion compared to the production from an equivalent amount of plant necromass may be disproportionate and underestimated (Barton et al., 2019). As one example (see review by Scott, 1998), burying beetles have been shown to remove and conceal 91% of exposed small (21–210 g) mammal carcasses by burial within an average of 1.4 days (Trumbo, 1992). In the same study, 22.7% of the exposed carcasses were eaten or removed by vertebrate scavengers. This example demonstrates how quickly small carrion can be removed from scientists’ observation.
Decomposing carcasses contribute hot spots of nutrient release (Carter et al., 2007; Benbow et al., 2019) that in terrestrial systems can have direct and indirect long-term (e.g., months to years) effects on soil conditions (Bornemissza, 1957; Strickland and Wickings, 2015), plant communities (Towne, 2000; Wardle et al., 2004), and both invertebrate and vertebrate scavengers (Bump et al., 2009; Beasley et al., 2012; Barton et al., 2013; Benbow et al., 2015). The spatial extent of such effects in ecosystems has not been well documented, but could be potentially important over decades (Bump et al., 2009). As an example of this potential, Hawlena et al. (2012) found that fear of spider predation by living grasshoppers resulted in carcasses with significantly higher carbon to nitrogen ratios than non-stressed specimens, and the resulting change in carcass quality, even when the biomass was about 140 times lower than the plant litter biomass, affected below ground community function and subsequent leaf litter decomposition on carcass sites in an old prairie ecosystem. Thus, even small amounts of carrion biomass have significant effects on measurable ecosystem processes, such as leaf litter decomposition and soil function, suggesting that if scaled by population density, mortality rates, and turnover in a landscape carcasses may significantly impact ecosystem functions in ways yet to be examined. If scaled in this manner, carrion will likely be shown to have collective ecosystem level effects like those recognized for ungulate dung and urine deposition in prairies (Norman and Green, 1958; Seastedt et al., 1991) or hippopotamus dung for some African rivers (Subalusky et al., 2015).
The Need to Scale From Carcass to Collective Effects
The hypothesis that individual carcass effects can have large spatial and long temporal scale effects has been supported by work in Isle Royale National Park, Michigan (Bump et al., 2009). In this 50-year study of the effects of over 3,600 wolf-killed moose carcasses on landscape heterogeneity and ecosystem function, Bump et al. (2009) reported that soil nutrients (e.g., 100–600% higher inorganic nitrogen at carcass compared to control sites), microbial biomass, microbial community composition, and surrounding plant leaf nitrogen (e.g., leaf nitrogen was 25–47% greater at carcass sites) was significantly elevated for at least 2–3 years at carcass deposition locations. In case studies of whale falls, Smith and Baco (2003) estimate that the sediment beneath whale carcasses receive a pulse of organic matter that is equal to almost 2000 years of background material over decomposition. Furthermore, in observations on large elasmobranch (i.e., whale shark and mobulid rays) carcass falls, Higgs et al. (2014) estimated that such carcasses represent on average about 4% of the normal particulate organic carbon flux to the seafloor in the bounding area of their occurrence. The authors suggest that the deep-sea scavenger communities benefit most from these significant energetic subsidies.
The same considerations for the underappreciated role of carrion in terrestrial ecosystems or the deep sea abyss is true for other aquatic ecosystems, where studies have documented localized carcass effects in salmon-bearing streams, whale falls, and other fauna of the deep ocean benthos (Smith and Baco, 2003; Kemp et al., 2006; Anderson and Bell, 2014; Higgs et al., 2014), including prehistoric carrion (Reisdorf et al., 2012; Danise et al., 2014); fish and waterfowl carcasses of salt marshes (Parmenter and Lamarra, 1991); and additional carcass effects likely in most aquatic habitats.
Indeed, vertebrate carrion placed in deep (300–3000 m) marine habitats initiates a succession of both invertebrate and vertebrate scavengers that take advantage of this punctuated, heterotrophically derived subsidy (Kemp et al., 2006; Anderson and Bell, 2016). What is less understood is how individual carcasses scale with mortality at population and community levels of biological organization. New studies are needed to better use population demographics and mortality estimates for calculating ecosystem level carrion production from species that do not have phenology-based or episodic mass mortalities. In Figure 1 of Barton et al. (2019) aquatic carrion biomass ranged from 102 kg/km2 for copepods up to 107 kg/km2 for bivalves, with different species of fish ranging from about 103 to 106 kg/km2. Among the examples they provided, terrestrial vertebrate biomass was at least two orders of magnitude lower than aquatic species at 103 kg/km2. While this example is not comprehensive, it does suggest that the effect of heterotrophically derived necromass in ecosystems is likely much greater than historically considered. To better incorporate the contributions of carrion in aquatic ecosystem energy and nutrient budgets, additional studies are needed that directly quantify carcass necromass and turnover across biomes. Such broadly available data on non-consumptive heterotrophically derived necromass in ecosystems would advance theory in consumer-resource and food web ecology (Getz, 2011).
In this paper we support the arguments of Subalusky and Post (2018) and Barton et al. (2019) that carrion resources are important subsidies in most ecosystems. We do this with a synthesis of literature from freshwater and marine ecosystems along three themes of how carrion is produced and decomposes through the following: autochthonous and allochthonous necromass decomposition; phenology-based mortality; and stochastic and episodic mass mortality subsidies. We provide examples and case studies that collectively show that carrion, along a size continuum that spans multiple orders of magnitude, likely plays an underappreciated role in ecosystem energetics and nutrient dynamics. Furthermore, we posit that death links living resource pools of multiple and interacting populations of species through pathways of non-consumptive mortality that result in a dead resource pool represented by carcasses that are quickly decomposed and recycled back into the living resource pool through detritivores and omnivores (Figure 1). In aquatic systems, such carrion represents autochthonous or allochthonous energy and nutrient subsidies that affect foodwebs and ecosystem function in complex and understudied ways.
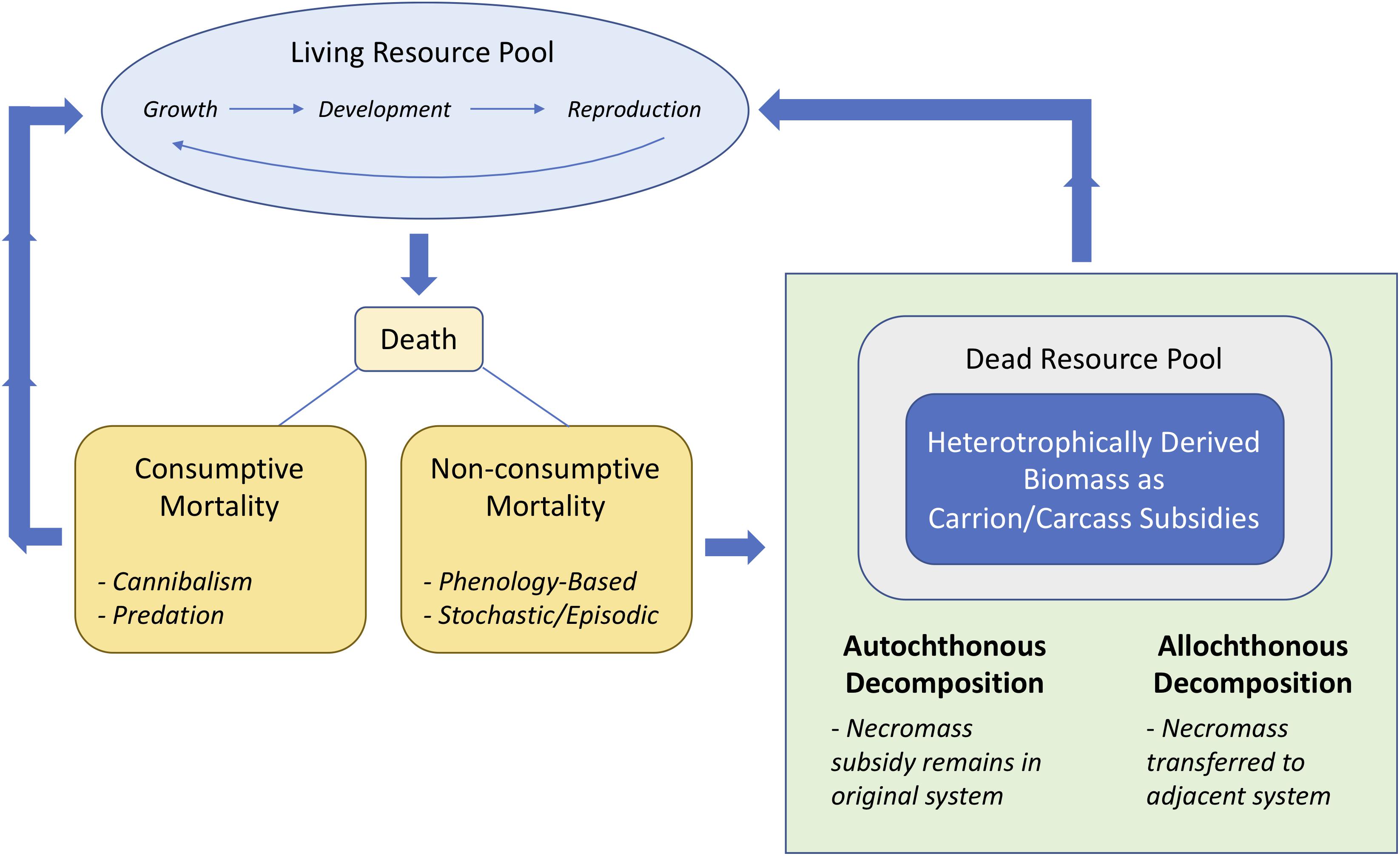
Figure 1. Conceptual diagram linking living resource pools to dead resource pools through the death and decomposition of heterotrophically derived biomass in the form of carrion subsidies. Each species is part of a living resource pool that encompasses growth, development, and reproduction; however, death of a proportion of the living individuals leads to the scavenging or decomposition of the once living biomass (or necromass) that ultimately becomes incorporated into the living resource pool through food web interactions. Death can come in the form of consumptive mortality (i.e., cannibalism or predation) where the nutrients and energy of dead organisms goes back to the living resource pool. Death can also come in the form of non-consumptive mortality, as part of natural senescence of the life cycle that can be through phenology-based (e.g., salmon life cycle) or stochastic/episodic (e.g., mass mortality of bivalve populations) processes. The resulting decomposition of these carrion subsidies can be autochthonous or allochthonous, depending on the life history and range of the once living species, affecting energy and nutrient flow within and among ecosystems.
Autochthonous and Allochthonous Carrion Necromass
Similar to phototrophically derived detritus, carrion necromass in aquatic ecosystems can come in the form of autochthonous and allochthonous sources. Carrion necromass of aquatic ecosystems can be generated from within or outside of a system in the form of autochthonous and allochthonous resources, respectively (Figure 1). As part of the autochthonous resource pool, carcasses resulting from natural senescence, physiological intolerance, or disease-related death of aquatic heterotrophs enter the detrital pool based on rates of mortality, disease, partial predation, and generation times related to natural senescence. These resources represent necromass from all organisms that live in aquatic habitats, from bacteria to blue whales (Minshall et al., 1991; Fenoglio et al., 2005; Subalusky and Post, 2018).
For any specific taxon, the degree of natural senescence, physiological intolerance (e.g., temperature, physical and chemical thresholds), or disease-related non-consumptive death contribute to the within-system detrital pool and associated energy and nutrient dynamics. For instance, non-consumptive mortality is a natural part of the population dynamics of any species, but the overall magnitude is rarely quantified, except for studies related to aquaculture production of commercially important taxa (Rowe et al., 1989; Karunasagar et al., 1994; Chen et al., 1995; Lorenzen, 1996). Furthermore, the mere presence of predators is known to have negative effects on life history traits and fitness of many species (Preisser et al., 2005). Since many organisms are prey for a variety of predators, this collective effect of non-consumptive predation threats can lead to facilitated senescence within many animal populations, although this form of non-consumptive mortality has not been broadly studied. In general, non-consumptive predator effects have been documented to be as strong or stronger than direct predation effects for many species, and the non-consumptive predator presence effect is generally stronger in aquatic than terrestrial ecosystems (Preisser et al., 2005).
Non-consumptive effects can come in the form of increased costs of defensive strategies that include lower mating success, increased vulnerability to other predators, energetic investments related to finding resources or defensive structures (e.g., morphology or biochemical), or through reduced survivorship (Kotler et al., 1993; Preisser et al., 2005; Sheriff et al., 2009). For instance, McCauley et al. (2011) reported significant non-consumptive predator induced mortality and failed metamorphosis to adults in dragonfly larvae exposed to predatory fish and other dragonfly predators. Depending on predator density, survivorship was 1.2–4.3 higher under no-predator mesocosm conditions. The mechanisms responsible for such non-consumptive predator induced mortality are not clear, but have been speculated to be related to increased susceptibility to disease and energetic costs of foraging related to induced stress (Ramirez and Snyder, 2009; Hawlena and Schmitz, 2010). This example demonstrates that just the presence of a predator leads to greater mortality, supporting the hypothesis that there are degrees of non-consumptive mortality that occur in natural environments; however, the mechanisms and sources of non-consumptive mortality are difficult to identity and quantify. Such non-consumptive mortality may result in increased heterotrophically derived necromass contributions to ecosystem organic matter budgets through decomposition (Figure 1).
The non-consumptive component of population mortality has not been studied in any detail for most aquatic organisms, and is often presumed negligible in ecosystem level budgets or calculations of secondary production where predator consumption rates have often been assumed to lead to 100% prey mortality (Hynes, 1970; Waters, 1977; Wetzel, 1995). This absolute consumption rate is not often the case in natural systems, with many individuals of a population avoiding predation and succumbing to other means (e.g., disease or starvation) of mortality (Wetzel, 1995). Thus, the availability of carcasses to aquatic ecosystems is likely larger than previously assumed, especially if the effects of individual carcasses are scaled by their collective density and rates of availability in the environment as discussed by Barton et al. (2019).
The effect of individual carcasses on ecosystem structure and function has been shown to be variable (Minshall et al., 1991; Fenoglio et al., 2005; Barton et al., 2019; Benbow et al., 2019); however, individual carcass effects have not been scaled to account for population level mortality over space and time in a way that would reveal the collective pool of heterotrophically derived necromass for ecosystems based on natural, non-consumptive rates of mortality (Barton et al., 2019). A significant challenge to addressing this need lies with differentiating natural senescence, starvation, climate, or disease-related mortality from predation or consumption rates for a specific population. Quantifying non-consumptive mortality is inherently difficult, but could potentially be done by evaluating life tables (Deevey, 1947) of organisms with and without predators, much like that for humans (Haldane, 1953). Additional studies are needed to devise ways to account for non-consumptive mortality, much like that of recent examples that quantified seal (Quaggiotto et al., 2018) and wildebeest (Subalusky et al., 2017) mortality on aquatic ecosystems. However, there have been surprisingly few studies on non-consumptive mortality of micro- and macroinvertebrates; those taxa that can often have fast generation times and high mortality.
Potential ways to quantify non-consumptive mortality of macroinvertebrates can come from life history and secondary production studies in the absence of predators in natural ecosystems or from more artificial conditions, such as those conducted for ecotoxicology studies and aquaculture. Some estimates of macroinvertebrate secondary production are available from fish-bearing and fishless lakes (Arnott and Vanni, 1993; Northington et al., 2010), but the body size distributions are not often reported to allow for estimates of non-consumptive mortality and how that may mediate secondary production. It is often assumed in studies of secondary production that population loss can all be attributed to predation, but this is likely not the case. Data from control groups (e.g., no treatment with a contaminant) used in ecotoxicology studies may also provide data on non-consumptive mortality, but often these experiments are done under artificial conditions associated with laboratory or field mesocosms, where densities and abiotic conditions may not represent natural conditions (Rand et al., 1995; Boudou, 2018). These artificial conditions are similar to aquaculture systems and associated research (Huet et al., 1986). The degree to which non-consumptive mortality in aquaculture conditions differs from natural ecosystems is not well understood. Another potential mechanism for determining non-consumptive mortality in aquatic populations, and employed in fisheries management, is to derive mortality–weight relationships in populations in different natural ecosystems compared to conditions with eliminated or significantly reduced predation pressure (Lorenzen, 1996, 2000). For instance, Lorenzen (1996) reported allometric scaling of fish mortality to non-predatory mortality by modeling mortality–weight relationships of fish populations from natural ecosystems compared to ponds/cages and tanks with no predation pressure. In these conditions, mortality is attributed to diseases, water quality problems, or winter starvation (Huet et al., 1986). The derived weight exponents of mortality were consistently negative for populations in ponds/cages/tanks compared to natural ecosystems, suggesting non-predatory mortality is more weight (i.e., surrogate for age) dependent than is predatory mortality.
Additional information on carrion impacts on aquatic ecosystems can be gathered from mass mortality studies of autochthonous heterotrophs (see the section “Stochastic and Episodic Mass Mortality and Decomposition”) and those related to programed phenology-based death of allochthonous taxa. As part of allochthonous resources, carcasses ultimately come from outside of the system and may include anadromous (e.g., salmon, sturgeon) or catadromous (e.g., eels) vertebrates, crustaceans, and molluscs (e.g., amphidromous shrimp and snails) that spend a portion of their life cycle growing and developing in other habitats or ecosystems (e.g., ocean or mangroves), but complete their life cycle in the freshwater environment (McDowall, 1988; Cederholm et al., 1989; Thuesen et al., 2011; Weaver et al., 2018). These resource subsidies can be made available through natural senescence, physiological intolerance, starvation, or disease-related, non-consumptive death (e.g., amphidromous herring) or through programed phenology-based mortality (e.g., post-spawning salmon). The effects of allochthonous sources of necromass have been studied in considerable detail, especially for salmon, both within its native range (Cederholm et al., 1989; Schindler, 1992; Chaloner et al., 2002; Janetski et al., 2009), but also where it has been introduced to naïve watersheds (Richey et al., 1975; Schuldt and Hershey, 1995; O’Toole et al., 2006).
Parasitized terrestrial insects can also represent allochthonous inputs in aquatic systems, entering streams from the riparian canopy due to modified behavior associated with their parasites (Schmidt-Rhaesa, 2001; Thomas et al., 2002). In one well-documented example, the horsehair worm (Nematomorpha) infects the camel cricket (Orthoptera: Rhaphidophoroidae) in Japanese watersheds. Once infected, the riparian crickets either slowly enter or jump into the streams, upon which the parasite leaves the body immediately or soon after the cricket is dead. These terrestrial subsidies have been shown to lower predation of resident aquatic invertebrates (Sato et al., 2008, 2011a,b, 2012). While not as well studied, other parasite-infected terrestrial insects (e.g., praying mantis) can also enter water with immediate horsehair release (Schmidt-Rhaesa, 2001). These examples suggest that parasitized insect subsidies enter the detrital pool upon death if not consumed, and may also offer a predatory release of other species that could potentially lead to additional non-consumptive mortality of those species in stream ecosystems.
Phenology-Based Mortality and Decomposition
A variety of aquatic organisms use phenology-based cues, such as temperature and day length, to control important behaviors including migration, hatching, and spawning. While these behaviors play critical roles in the fitness of the organisms themselves, they also affect other taxa throughout the food web. For example, the movement of anadromous fish can transport large amounts of nutrients and biomass upstream through their spawning activities and phenology-based mortality (Cederholm et al., 1999; Schindler et al., 2003; Wipfli et al., 2003), which are then used by other consumers (Bilby et al., 1996; Chaloner et al., 2002; Baxter et al., 2005; Hocking and Reynolds, 2011). Although mass mortality events of semelparous fish (e.g., salmon) following spawning are a dramatic introduction of necromass into freshwater systems, the release of eggs (which commonly exhibit high rates of mortality) and excrement by both iteroparous and semelparous fish comprise an important, though less studied, component of the available necromass, in some cases exceeding the nutrient inputs from carcasses (Tiegs et al., 2011; Childress and McIntyre, 2015). These inputs represent a major linkage of marine and freshwater systems as many anadromous fish derive most of their mass (>95%) from marine-based sources before migrating into freshwater systems via migration and subsequent semelparous death (Mathisen et al., 1988; Cederholm et al., 1999; Lamberti et al., 2010).
In contrast to stochastic mass mortality events, phenology-based events provide a regular input of nutrients into aquatic systems, with many organisms altering their life histories to coincide with these predictable influxes of resources (Hocking and Reynolds, 2011; Lisi and Schindler, 2011; Deacy et al., 2017). Predators, such as mink (Mustela vison) or brown bears (Ursus arctos), alter their behavior and timing of reproduction to coincide with the availability of salmon runs (Ben-David, 1997). These scavengers use salmon as a major nutritional source, in some locations obtaining >90% of their carbon and nitrogen from these fish (Willson and Halupka, 1995; Hilderbrand et al., 1996, 1999). The landscape can also affect how salmon carcass resource subsidies impact terrestrial and estuarine ecosystems, with differences reported in how wolves and bears transport carcasses to riparian forests and meadows depending on stream size and location within the watershed (Harding et al., 2019). A range of other mammals, birds, and insects use the carcasses of anadromous fish as resources that can affect the decomposition dynamics in streams (Cederholm et al., 1989; Zhang et al., 2003) and alter their behavior to better use and consume these resources (Moore and Schindler, 2010). In addition to direct consumption by eukaryotes, carcasses in aquatic systems can have strong interactions with microbial communities (Wipfli et al., 1998; Pechal and Benbow, 2016; Pechal et al., 2019). Although the impact of decomposing carcasses is highly dependent on biotic and abiotic factors (e.g., stream physical structure, riparian conditions, organisms present, etc.) the input of nutrients from carcasses stimulates microbial activity and primary production (Mitchell and Lamberti, 2005), leading to additional effects on higher trophic levels (Wipfli et al., 1998; Cederholm et al., 1999; Gende et al., 2002).
Salmon carcasses can also act as a resource subsidy to estuaries of salmon-bearing streams (Cederholm et al., 1999; Gende et al., 2004; Cak et al., 2008), with linkages to macroalgae through copepods (Fujiwara and Highsmith, 1997), marine invertebrates including echinoderms and crustaceans (Reimchen, 1994), and vertebrate taxa, such as coyotes and wolves (Gende et al., 2004). The availability of these resources to estuary systems can be mediated by complex interactions among trophic groups and habitat conditions. While the feeding activity of gray wolves (Canis lupus) and bears (Ursus spp.) can transfer carcasses from stream reaches to riparian habitats (Gende et al., 2004), where they become available to other scavengers, how many and which species of salmon they transfer depends on both species-specific interactions and landscape structure (e.g., riparian habitat and length of spawning reach) (Harding et al., 2019). Besides their well-documented effects during spawning seasons, inputs of necromass can have residual effects across seasons, with spawning salmon biomass in the autumn predictive of bird density and diversity in estuaries the following summer (Field and Reynolds, 2011). Findings such as these illustrate the important and complex roles necromass and phenology-based mortality play in aquatic ecosystems.
Although few aquatic insects have evolved to feed directly on carrion, the influx of nutrients from salmon carcasses, and resulting increases in primary production, can increase aquatic insect density by 8–25 times in artificial and natural streams where carcasses are present (Wipfli et al., 1998; Fenoglio et al., 2014). Isotopic studies have shown that salmon-derived carbon and nitrogen is incorporated into both primary producers and invertebrate feeding groups that consume microbes (e.g., filterers and grazers) (Bilby et al., 1996; Johnston et al., 1997; Guyette et al., 2014). While salmon remains the best studied example of phenology-based mortality (Schindler et al., 2003), they are by no means the only group of aquatic animals with programed mortality that leads to cascading effects in aquatic ecosystems. In addition to bony fish and invertebrates (see below), carcasses of other aquatic organisms, such as the sea lamprey (Petromyzon marinus), represent important nutrient sources, connect marine and freshwater systems, and can stimulate primary productivity (Weaver et al., 2018). While catadromous organisms which migrate to the ocean to spawn, [e.g., eels (Anguilla spp.)], also link marine and freshwater systems; how their behavior impacts marine ecosystems remains largely unknown. However, their spawning and subsequent death likely introduces considerable nutrients into otherwise oligotrophic environments where spawning occurs (e.g., Sargasso Sea).
Stochastic and Episodic Mass Mortality and Decomposition
Vertebrate Carrion Mass Mortalities
One of the most striking examples of vertebrate mortality altering aquatic ecosystem comes in the form of episodic mass death, and the resulting carcasses that undergo decomposition, due to stochastic factors not easily predicted in nature (Fey et al., 2015). Mass fish kills are one of the most visible forms of this kind of mass mortality, where tens of thousands of fish may die within a short (e.g., hours to days) period of time, causing mass decomposition in the water and on banks of aquatic ecosystems (Ochumba, 1990; Thronson and Quigg, 2008). Fey et al. (2015) reported fish mass mortality events made up about 56% of all mass mortality events reported in scientific literature since the 1940s (N = 727). Episodic mass mortalities can be the result of stochastic changes in physical–chemical conditions (Cooper, 1993), toxic algal blooms (Hallegraeff, 1993), disease (Grizzle and Brunner, 2003), pollutants (Cooper, 1993), and other unknown factors. In many instances these conditions are the result of eutrophication over many years (Vollenweider, 1970; Harper, 1992; Nixon, 1995), or through punctuated high inputs of nutrients, like in the case of hippopotamus urine and feces (Subalusky et al., 2015) or mass drownings of wildebeest (Subalusky et al., 2017; Dutton et al., 2018). There are also other forms of vertebrate mass mortality that occur in aquatic ecosystems (Fey et al., 2015). For instance, annual mass drownings of wildebeest (Subalusky et al., 2017), aquatic reptiles (Rachowicz et al., 2006) and mammals that succumb to disease (Osterhaus et al., 1997; Kennedy, 1998) have both short- and long-term effects on ecosystem function. Fey et al. (2015) also provide an excellent quantitative assessment of mass mortality events worldwide, showing an increase in their occurrence for mammals, birds, amphibians, reptiles, fish, and marine invertebrates since 1940.
Invertebrate Carrion Mass Mortalities
Much like cicada emergences that have been quantified to have significant impacts on terrestrial food webs and ecosystems (Yang, 2004), mayflies (Ephemeroptera), midges (Gratton et al., 2008), salmonflies (Plecoptera) (Walters et al., 2018; Wesner et al., 2019), and other aquatic insects (Baxter et al., 2005) emerge in masses as adults to mate and die, with their carcasses falling back to the aquatic habitat or into the adjacent riparian zones and inland landscapes (Gergs et al., 2014). When these mass emergences cross habitat or ecosystem boundaries (e.g., from a stream or lake onto the shoreline), such cross-ecosystem resource subsidies (Polis et al., 1997) can represent significant nutrient and energy pulses (Polis, 1994; Polis and Hurd, 1996). For example, Wesner et al. (2019) reported that for several stream sites with massive emergences of salmonflies (Pteronarcys californica), the resulting insect carrion deposition on the adjacent shore over only a single week was equal to or greater than annual atmospheric nitrogen and phosphorus deposition and the annual secondary production of all terrestrial insects from that watershed. Such contributions to the detrital pools of adjacent ecosystems have not been well studied, especially compared to the living emerged insects that are consumed by predators. Indeed, more studies are needed to better quantify the contributions of necromass originating from aquatic ecosystems and acting as resource subsidies to the decomposition budgets of adjacent ecosystems.
Mass mortalities of aquatic invertebrates, beyond what was discussed above with parasite-mediated terrestrial insect drownings, also affect in-stream and riparian communities and ecosystem properties. While not as well documented as fish subsidies, invertebrate mass mortalities have significant and sometimes long lasting effects on aquatic ecosystems since many of the invertebrate species, like mussels, have important functional roles (Vaughn, 2018), but also can contribute mass subsidies of highly recalcitrant structures, such as shells of molluscs (McDowell et al., 2017; DuBose et al., 2019). Beyond the pulsed effects of nutrient release related to rapid soft tissue decomposition of bivalves, Wenger et al. (2019) estimated that mussel shells from mortality events may have once provided about 1% of total phosphorus load in streams and rivers of the southeastern United States.
McDowell and Sausa (2019) reviewed the effects of mass mortality effects of the invasive bivalves Corbicula sp., the zebra mussel Dreissena polymorpha, the golden mussel Limnoperna fortunei, and the Chinese pond mussel Sinanodonta woodiana. Low and high water temperatures and water levels were the leading causes of mass mortality of both invasive and native species. There were short-term (i.e., over days) nutrient pulse releases associated with en masse soft tissue decomposition and longer-term microhabitat effects in the form of remaining shells both as part of the benthic substrata but also on stream and river banks. For instance, a mass mortality event of about 100 million C. fluminea contributed an estimated 751 kg of carbon, 180 kg of nitrogen, and 45 kg of phosphorus to a stream over the course of several days (McDowell et al., 2017). The dead and dying bivalve carcasses may also serve as food resources for local scavengers like fish, invertebrates, and birds (Mouthon and Daufresne, 2006; McDowell and Sausa, 2019). Mass mortality of C. fluminea left on stream banks attract a wide diversity of terrestrial invertebrates (Novais et al., 2015) and below ground nutrients (Novais et al., 2017), suggesting that bivalves washed onto banks during floods can become pulsed resources subsidies for adjacent habitats.
Mass mortality events also occur throughout the world in marine and estuarine habitats, and not surprisingly changing climate patterns and warming temperatures are increasing their magnitude and frequency (Coma et al., 2009). While not always independent of increases in water temperature, diseases also contribute to marine invertebrate mass mortality (Harvell et al., 1999). Not matter what the cause, marine invertebrate mass mortality has significant effects on coral reef and intertidal ecosystems by affecting both hard and soft corals, benthic burrowers and filterers with cascading effects on upper trophic level consumers (Knowlton, 2004). These massive death events are often extensive. For instance, in 2016, one of the three pan-tropical mass coral bleaching events occurred in the Great Barrier Reef of Australia as a result of a significant marine heat wave, where 90% of the surveyed reefs suffered mortality (Hughes et al., 2017). The ecosystem consequences of this event included significant community restructuring, functional changes, and widespread declines in consumers resulting directly from the water temperature increases, but also in relation to coral loss (Stuart-Smith et al., 2018). Increased frequency and magnitudes of such global weather events will have widespread ecological impacts on all ecosystems, with mass mortalities contributing to many of the most negative effects of a changing climate.
Summary
Death and decomposition occur in all ecosystems, but the extent and magnitude of the resulting necromass varies in space and time. Some forms of necromass come from within the system, as part of the life cycles and life histories of the resident organisms (e.g., planktonic snow in a lake or ocean or insects in a stream) or during mass deaths resulting from changing and intolerable habitat conditions (e.g., fish kills and coral reef bleaching). Other forms of necromass subsidies come from outside of the system, sometimes as migratory fish (e.g., salmon) or parasitized terrestrial insects that have been behaviorally hijacked to enter aquatic habitats where they drown. In all cases, the once living biomass becomes an often significant and functionally important component of the detrital pool. This component of heterotrophically derived necromass has historically been difficult to quantify due to the rapid turnover of such labile resources, and has arguably been overlooked or underappreciated in many ecosystem level energy and nutrient budgets. Recent work has provided conceptual models and methods for improving the ability to identify, quantify, and better study how carrion resource subsidies affect aquatic ecosystems, ranging from small, headwater streams to saltmarshes and mangroves, to the deep oceanic abyss and enormous stretches of coastline habitats around the world. Furthermore, while studies of megafauna carrion (e.g., whales and whale sharks) have demonstrated significant impacts to deep ocean habitats, much less is understood for other groups of animals. Quantifying the energy, nutrient, and foodweb effects of autochthonous and allochthonous carrion resulting from non-consumptive morality, phenology-based mortality and stochastic and episodic mortality events will allow broader assessments of all necromass contributions in aquatic ecosystems. With advances in geographical (e.g., drones and satellite imagery), genomic (e.g., next-generation sequencing), and other forms of technology (e.g., cell phone cameras and associated software) it will become increasingly feasible to quantify baseline levels of carrion to more fully evaluate how this resource subsidy affects ecosystem energy and nutrient dynamics.
Author Contributions
MB, JR, and GL performed the literature search, and writing and editing of this review.
Funding
MB was supported by the College of Agriculture and Natural Resources and College of Osteopathic Medicine of Michigan State University. GL was supported by the Department of Biological Sciences and the Environmental Change Initiative at the University of Notre Dame.
Conflict of Interest
The authors declare that the research was conducted in the absence of any commercial or financial relationships that could be construed as a potential conflict of interest.
Acknowledgments
We would like to thank P. Barton, J. Tomberlin, H. Jordan, and A. Tarone for thoughtful discussions that have led to this manuscript, and J. Pechal also for reviewing an earlier version. This manuscript was the ultimate product of a special session at the 2018 Annual Meeting of the Society for Freshwater Science, hosted in Detroit, MI (https://freshwater-science.org/annual-meeting) by MB and GL, where many other scientists inspired our writing through their presentations and manuscript contributions to this special issue.
References
Alldredge, A. L., and Silver, M. W. (1988). Characteristics, dynamics and significance of marine snow. Prog. Oceanogr. 20, 41–82. doi: 10.1016/0079-6611(88)90053-5
Allison, P. A., Smith, C. R., Kukert, H., Deming, J. W., and Bennett, B. A. (1991). Deep-water taphonomy of vertebrate carcasses: a whale skeleton in the bathyal Santa Catalina Basin. Paleobiology 17, 78–89. doi: 10.1017/s0094837300010368
Anderson, G. S., and Bell, L. S. (2014). Deep coastal marine taphonomy: investigation into carcass decomposition in the Saanich Inlet, British Columbia using a baited camera. PLoS One 9:e110710. doi: 10.1371/journal.pone.0110710
Anderson, G. S., and Bell, L. S. (2016). Impact of marine submergence and season on faunal colonization and decomposition of pig carcasses in the Salish Sea. PLoS One 11:e0149107. doi: 10.1371/journal.pone.0149107
Arnott, S. E., and Vanni, M. J. (1993). Zooplankton assemblages in fishless bog lakes: influence of biotic and abiotic factors. Ecology 74, 2361–2380. doi: 10.2307/1939588
Barton, P. S., Cunningham, S. A., Lindenmayer, D. B., and Manning, A. D. (2013). The role of carrion in maintaining biodiversity and ecological processes in terrestrial ecosystems. Oecologia 171, 761–772. doi: 10.1007/s00442-012-2460-3
Barton, P. S., Evans, M. J., Foster, C. N., Pechal, J. L., Bump, J. K., Quaggiotto, M. M., et al. (2019). Towards quantifying carrion biomass in ecosystems. Trends Evol. 34, 950–961. doi: 10.1016/j.tree.2019.06.001
Baxter, C. V., Fausch, K. D., and Saunders, C. W. (2005). Tangled webs: reciprocal flows of invertebrate prey link streams and riparian zones. Freshw. Biol. 50, 201–220. doi: 10.1111/j.1365-2427.2004.01328.x
Beasley, J. C., Olson, Z., and DeVault, T. (2012). Carrion cycling in food webs: comparisons among terrestrial and marine ecosystems. Oikos 121, 1021–1026. doi: 10.1111/j.1600-0706.2012.20353.x
Benbow, E. M., Tomberlin, J. K., and Tarone, A. M. (2015). Carrion Ecology, Evolution, and Their Applications. Boca Raton, FL: CRC Press.
Benbow, M. E., Barton, P. S., Ulyshen, M. D., Beasley, J. C., DeVault, T. L., Strickland, M. S., et al. (2019). Necrobiome framework for bridging decomposition ecology of autotrophically and heterotrophically derived organic matter. Ecol. Monogr. 89:e01331. doi: 10.1002/ecm.1331
Ben-David, M. (1997). Timing of reproduction in wild mink: the influence of spawning Pacific salmon. Can. J. Zool. 75, 376–382. doi: 10.1139/z97-047
Benke, A. C. (1998). Production dynamics of riverine chironomids: extremely high biomass turnover rates of primary consumers. Ecology 79, 899–910. doi: 10.1890/0012-9658(1998)079%5B0899:pdorce%5D2.0.co;2
Bennett, B. A., Smith, C. R., Glaser, B., and Maybaum, H. L. (1994). Faunal community structure of a chemoautotrophic assemblage on whale bones in the deep northeast Pacific Ocean. Mar. Ecol. Prog. Ser. 108, 205–223. doi: 10.3354/meps108205
Bilby, R. E., Fransen, B. R., and Bisson, P. A. (1996). Incorporation of nitrogen and carbon from spawning coho salmon into the trophic system of small streams: evidence from stable isotopes. Can. J. Fish. Aquat. Sci. 53, 164–173. doi: 10.1139/f95-159
Bornemissza, G. F. (1957). An analysis of arthropod succession in carrion and the effect of its decomposition on the soil fauna. Austr. J. Zool. 5, 1–12.
Boudou, A. (2018). Aquatic Ecotoxicology: Volume 1: Fundamental Concepts and Methodologies. Boca Raton, FL: CRC Press.
Boyero, L., Pearson, R. G., Hui, C., Gessner, M. O., Pérez, J., Alexandrou, M. A., et al. (2016). Biotic and abiotic variables influencing plant litter breakdown in streams: a global study. Proc. R. Soc. B Biol. Sci. U.S.A. 283:20152664. doi: 10.1098/rspb.2015.2664
Brinson, M. M., Lugo, A. E., and Brown, S. (1981). Primary productivity, decomposition and consumer activity in freshwater wetlands. Ann. Rev. Ecol. Syst. 12, 123–161. doi: 10.1146/annurev.es.12.110181.001011
Bump, J. K., Peterson, R. O., and Vucetich, J. A. (2009). Wolves modulate soil nutrient heterogeneity and foliar nitrogen by configuring the distribution of ungulate carcasses. Ecology 90, 3159–3167. doi: 10.1890/09-0292.1
Cak, A. D., Chaloner, D. T., and Lamberti, G. A. (2008). Effects of spawning salmon on dissolved nutrients and epilithon in coupled stream-estuary systems of southeastern Alaska. Aquat. Sci. 70, 169–178. doi: 10.1007/s00027-008-8090-5
Carter, D. O., Yellowlees, D., and Tibbett, M. (2007). Cadaver decomposition in terrestrial ecosystems. Naturwissenschaften 94, 12–24. doi: 10.1007/s00114-006-0159-1
Cederholm, C. J., Houston, D. B., Cole, D. L., and Scarlett, W. J. (1989). Fate of coho salmon (Oncorhynchus kisutch) carcasses in spawning streams. Can. J. Fish. Aquat. Sci. 46, 1347–1355. doi: 10.1139/f89-173
Cederholm, C. J., Kunze, M. D., Murota, T., and Sibatani, A. (1999). Pacific salmon carcasses: essential contributions of nutrients and energy for aquatic and terrestrial ecosystems. Fisheries 24, 6–15. doi: 10.1577/1548-8446(1999)024<0006:psc>2.0.co;2
Chaloner, D. T., Wipfli, M. S., and Caouette, J. P. (2002). Mass loss and macroinvertebrate colonization of Pacific salmon carcassess in southeastern Alaskan streams. Freshw. Biol. 47, 263–274.
Chen, S., Wu, J., and Malone, R. F. (1995). Effects of temperature on mean molt interval, molting and mortality of red swamp crawfish (Procambarus clarkii). Aquaculture 131, 205–217. doi: 10.1016/0044-8486(94)00327-k
Childress, E. S., and McIntyre, P. B. (2015). Multiple nutrient subsidy pathways from a spawning migration of iteroparous fish. Freshw. Biol. 60, 490–499. doi: 10.1111/fwb.12494
Coma, R., Ribes, M., Serrano, E., Jiménez, E., Salat, J., and Pascual, J. (2009). Global warming-enhanced stratification and mass mortality events in the Mediterranean. Proc. Natl. Acad. Sci. U.S.A. 106, 6176–6181. doi: 10.1073/pnas.0805801106
Cooper, C. (1993). Biological effects of agriculturally derived surface water pollutants on aquatic systems–a review. J. Environ. Q. 22, 402–408. doi: 10.2134/jeq1993.00472425002200030003x
Danise, S., Twitchett, R. J., and Matts, K. (2014). Ecological succession of a Jurassic shallow-water ichthyosaur fall. Nat. Commun. 5:4789. doi: 10.1038/ncomms5789
Deacy, W. W., Armstrong, J. B., Leacock, W. B., Robbins, C. T., Gustine, D. D., Ward, E. J., et al. (2017). Phenological synchronization disrupts trophic interactions between Kodiak brown bears and salmon. Proc. Natl. Acad. Sci. U.S.A. 114, 10432–10437. doi: 10.1073/pnas.1705248114
Deevey, E. S. Jr. (1947). Life tables for natural populations of animals. Q. Rev. Biol. 22, 283–314. doi: 10.1086/395888
DuBose, T. P., Atkinson, C. L., Vaughn, C. C., and Golladay, S. W. (2019). Drought-induced, punctuated loss of freshwater mussels alters ecosystem function across temporal scales. Front. Ecol. Evol. 7:274. doi: 10.3389/fevo.2019.00274
Duggins, D., Simenstad, C., and Estes, J. (1989). Magnification of secondary production by kelp detritus in coastal marine ecosystems. Science 245, 170–173. doi: 10.1126/science.245.4914.170
Dutton, C. L., Subalusky, A. L., Hamilton, S. K., Rosi, E. J., and Post, D. M. (2018). Organic matter loading by hippopotami causes subsidy overload resulting in downstream hypoxia and fish kills. Nat. Commun. 9:1951. doi: 10.1038/s41467-018-04391-6
Entrekin, S. A., Tank, J. L., Rosi-Marshall, E. J., Hoellein, T. J., and Lamberti, G. A. (2009). Response of secondary production by macroinvertebrates to large wood addition in three Michigan streams. Freshw. Biol. 54, 1741–1758. doi: 10.1111/j.1365-2427.2009.02223.x
Fenoglio, S., Bo, T., Agosta, P., and Cucco, M. (2005). Mass loss and macroinvertebrate colonisation of fish carcasses in riffles and pools of a NW Italian stream. Hydrobiologia 532, 111–122. doi: 10.1007/s10750-004-9451-2
Fenoglio, S., Merritt, R. W., and Cummins, K. W. (2014). Why do no specialized necrophagous species exist among aquatic insects? Freshw. Sci. 33, 711–715. doi: 10.1086/677038
Fey, S. B., Siepielski, A. M., Nusslé, S., Cervantes-Yoshida, K., Hwan, J. L., Huber, E. R., et al. (2015). Recent shifts in the occurrence, cause, and magnitude of animal mass mortality events. Proc. Natl. Acad. Sci. U.S.A. 112, 1083–1088. doi: 10.1073/pnas.1414894112
Field, R. D., and Reynolds, J. D. (2011). Sea to sky: impacts of residual salmon-derived nutrients on estuarine breeding bird communities. Proc. R. Soc. B Biol. Sci. 278, 3081–3088. doi: 10.1098/rspb.2010.2731
Fujiwara, M., and Highsmith, R. C. (1997). Harpacticoid copepods: potential link between inbound adult salmon and outbound juvenile salmon. Mar. Ecol. Prog. Ser. 158, 205–216. doi: 10.3354/meps158205
Garman, G. C. (1992). Fate and potential significance of postspawning anadromous fish carcasses in an Atlantic coastal river. Trans. Am. Fish. Soc. 121, 390–394. doi: 10.1577/1548-8659(1992)121<0390:fapsop>2.3.co;2
Gende, S. M., Edwards, R. T., Willson, M. F., and Wipfli, M. S. (2002). Pacific salmon in aquatic and terrestrial ecosystems. Bioscience 52, 917–928.
Gende, S. M., Quinn, T. P., Willson, M. F., Heintz, R., and Scott, T. M. (2004). Magnitude and fate of salmon-derived nutrients and energy in a coastal stream ecosystem. J. Freshw. Ecol. 19, 149–160. doi: 10.1080/02705060.2004.9664522
Gergs, R., Koester, M., Schulz, R. S., and Schulz, R. (2014). Potential alteration of cross-ecosystem resource subsidies by an invasive aquatic macroinvertebrate: implications for the terrestrial food web. Freshw. Biol. 59, 2645–2655. doi: 10.1111/fwb.12463
Gessner, M. O., Swan, C. M., Dang, C. K., McKie, B. G., Bardgett, R. D., Wall, D. H., et al. (2010). Diversity meets decomposition. Trends Ecol. Evol. 25, 372–380. doi: 10.1016/j.tree.2010.01.010
Getz, W. M. (2011). Biomass transformation webs provide a unified approach to consumer–resource modelling. Ecol. Lett. 14, 113–124. doi: 10.1111/j.1461-0248.2010.01566.x
Giering, S. L., Sanders, R., Lampitt, R. S., Anderson, T. R., Tamburini, C., Boutrif, M., et al. (2014). Reconciliation of the carbon budget in the ocean’s twilight zone. Nature 507:480. doi: 10.1038/nature13123
Gratton, C., Donaldson, J., and Vander Zanden, M. J. (2008). Ecosystem linkages between lakes and the surrounding terrestrial landscape in northeast Iceland. Ecosystems 11, 764–774. doi: 10.1007/s10021-008-9158-8
Grizzle, J. M., and Brunner, C. J. (2003). Review of largemouth bass virus. Fisheries 28, 10–14. doi: 10.1577/1548-8446(2003)28%5B10:rolbv%5D2.0.co;2
Grossart, H.-P., and Simon, M. (1998). Bacterial colonization and microbial decomposition of limnetic organic aggregates (lake snow). Aquat. Microb. Ecol. 15, 127–140. doi: 10.3354/ame015127
Guyette, M. Q., Loftin, C. S., Zydlewski, J., and Cunjak, R. (2014). Carcass analogues provide marine subsidies for macroinvertebrates and juvenile Atlantic salmon in temperate oligotrophic streams. Freshw. Biol. 59, 392–406. doi: 10.1111/fwb.12272
Haldane, J. (1953). Some animal life tables. J. Instit. Actuar. 79, 83–89. doi: 10.1017/s0020268100053907
Hallegraeff, G. M. (1993). A review of harmful algal blooms and their apparent global increase. Phycologia 32, 79–99. doi: 10.1186/1476-069X-7-S2-S4
Harding, J., Harding, J. N., Field, R. D., Pendray, J. E., Swain, N. R., Wagner, M. A., et al. (2019). Landscape structure and species interactions drive the distribution of salmon carcasses in coastal watersheds. Front. Ecol. Evol. 7:192. doi: 10.3389/fevo.2019.00192
Harper, D. (1992). What is Eutrophication? in Eutrophication of Freshwaters. Boston, MA: Springer, 1–28.
Harvell, C. D., Kim, K., Burkholder, J. M., Colwell, R. R., Epstein, P. R., Grimes, D. J., et al. (1999). Emerging marine diseases–climate links and anthropogenic factors. Science 285:1505. doi: 10.1126/science.285.5433.1505
Hawlena, D., and Schmitz, O. J. (2010). Physiological stress as a fundamental mechanism linking predation to ecosystem functioning. Am. Natur. 176, 537–556. doi: 10.1086/656495
Hawlena, D., Strickland, M. S., Bradford, M. A., and Schmitz, O. J. (2012). Fear of predation slows plant-litter decomposition. Science 336, 1434–1438. doi: 10.1126/science.1220097
Higgs, N. D., Gates, A. R., and Jones, D. O. (2014). Fish food in the deep sea: revisiting the role of large food-falls. PLoS One 9:e96016. doi: 10.1371/journal.pone.0096016
Hilderbrand, G. V., Farley, S. D., Robbins, C. T., Hanley, T. A., Titus, K., and Servheen, C. (1996). Use of stable isotopes to determine diets of living and extinct bears. Can. J. Zool. 74, 2080–2088. doi: 10.1139/z96-236
Hilderbrand, G. V., Hanley, T. A., Robbins, C. T., and Schwartz, C. C. (1999). Role of brown bears (Ursa arctos) in the flow of marine nitrogen into a terrestrial ecosystem. Oecologia 121:546. doi: 10.1007/s004420050961
Hocking, M. D., and Reynolds, J. D. (2011). Impacts of salmon on riparian plant diversity. Science 331, 1609–1612. doi: 10.1126/science.1201079
Huet, M., Timmermans, J., and Kahn, H. (1986). Textbook of Fish Culture: Breeding and Cultivation of Fish. London: Fishing News Books.
Hughes, T. P., Kerry, J. T., Álvarez-Noriega, M., Álvarez-Romero, J. G., Anderson, K. D., Baird, A. H., et al. (2017). Global warming and recurrent mass bleaching of corals. Nature 543, 373. doi: 10.1038/nature21707
Huryn, A. D., and Wallace, J. B. (2000). Life history and production of stream insects. Ann. Rev. Entomol. 45, 83–110. doi: 10.1146/annurev.ento.45.1.83
Janetski, D. J., Chaloner, D. T., Tiegs, S. D., and Lamberti, G. A. (2009). Pacific salmon effects on stream ecosystems: a quantitative synthesis. Oecologia 159, 583–595. doi: 10.1007/s00442-008-1249-x
Johnston, N., MacDonald, J., Hall, K., and Tschaplinski, P. (1997). A Preliminary Study of the Role of Sockeye Salmon (Oncorhynchus nerka) Carcasses as Carbon and Nitrogen Sources for Benthic insects and Fishes in the “Early Stuart” stock Spawning Streams, 1050 km from the Ocean. Fisheries Project Report RD55. Victoria, B.C: British Columbia Ministry of Environment.
Karunasagar, I., Pai, R., Malathi, G., and Karunasagar, I. (1994). Mass mortality of Penaeus monodon larvae due to antibiotic-resistant Vibrio harveyi infection. Aquaculture 128, 203–209. doi: 10.1016/0044-8486(94)90309-3
Kemp, K. M., Jamieson, A. J., Bagley, P. M., McGrath, H., Bailey, D. M., Collins, M. A., et al. (2006). Consumption of large bathyal food fall, a six month study in the NE Atlantic. Mar. Ecol. Prog. Ser. 310, 65–76. doi: 10.3354/meps310065
Kennedy, S. (1998). Morbillivirus infections in aquatic mammals. J. Compar. Pathol. 119, 201–225. doi: 10.1016/s0021-9975(98)80045-5
Knowlton, N. (2004). Multiple “stable” states and the conservation of marine ecosystems. Prog. Oceanogr. 60, 387–396. doi: 10.1016/j.pocean.2004.02.011
Kotler, B. P., Brown, J. S., Slotow, R. H., Goodfriend, W. L., and Strauss, M. (1993). The influence of snakes on the foraging behavior of gerbils. Oikos 67, 309–316.
Lamberti, G. A., Chaloner, D. T., and Hershey, A. E. (2010). Linkages among aquatic ecosystems. J. N. Am. Benthol. Soc. 29, 245–263. doi: 10.1899/08-166.1
Landry, M. R., and Calbet, A. (2004). Microzooplankton production in the oceans. ICES J. Mar. Sci. 61, 501–507. doi: 10.1016/j.icesjms.2004.03.011
Lindeman, R. L. (1942). The trophic-dynamic aspect of ecology. Ecology 23, 399–417. doi: 10.2307/1930126
Lisi, P., and Schindler, D. E. (2011). Spatial variation in timing of marine subsidies influences riparian phenology through a plant-pollinator mutualism. Ecosphere 2, 1–14.
Little, C. J., and Altermatt, F. (2018). Species turnover and invasion of dominant freshwater invertebrates alter biodiversity–ecosystem-function relationship. Ecol. Monogr. 88, 461–480. doi: 10.1002/ecm.1299
Lorenzen, K. (1996). The relationship between body weight and natural mortality in juvenile and adult fish: a comparison of natural ecosystems and aquaculture. J. Fish Biol. 49, 627–642. doi: 10.1111/j.1095-8649.1996.tb00060.x
Lorenzen, K. (2000). Allometry of natural mortality as a basis for assessing optimal release size in fish-stocking programmes. Can. J. Fish. Aquat. Sci. 57, 2374–2381. doi: 10.1139/f00-215
Mathisen, O. A., Parker, P. L., Goering, J. J., Kline, T., Poe, P. H., and Scalan, R. S. (1988). Recycling of marine elements transported into freshwater systems by anadromous salmon: with 4 figures and 3 tables in the text. Verhandlungen 23, 2249–2258. doi: 10.1080/03680770.1987.11899884
McCauley, S. J., Rowe, L., and Fortin, M.-J. (2011). The deadly effects of “nonlethal” predators. Ecology 92, 2043–2048. doi: 10.1890/11-0455.1
McDowall, R. M. (1988). Diadromy in Fishes: Migrations Between Freshwater and Marine Environments. London: Croom Helm.
McDowell, W., McDowell, W., and Byers, J. (2017). Mass mortality of a dominant invasive species in response to an extreme climate event: implications for ecosystem function. Limnol. Oceanogr. 62, 177–188. doi: 10.1002/lno.10384
McDowell, W. G., and Sausa, R. (2019). Mass mortality events of invasive freshwater bivalves: our current understanding and future directions for research. Front. Ecol. Evol. 62, 177–188. doi: 10.3389/fevo.2019.00331
Minshall, G. W., Hitchcock, E., and Barnes, J. R. (1991). Decomposition of rainbow trout (Oncorhynchus mykiss) carcasses in a forest stream ecosystem inhabited only by nonanadromous fish populations. Can. J. Fish. Aquat. Sci. 48, 191–195. doi: 10.1139/f91-026
Mitchell, N. L., and Lamberti, G. A. (2005). Responses in dissolved nutrients and epilithon abundance to spawning salmon in southeast Alaska streams. Limnol. Oceanogr. 50, 217–227. doi: 10.4319/lo.2005.50.1.0217
Moore, J. C., Berlow, E. L., Coleman, D. C., de Ruiter, P. C., Dong, Q., Hastings, A., et al. (2004). Detritus, trophic dynamics and biodiversity. Ecol. Lett. 7, 584–600. doi: 10.1111/j.1461-0248.2004.00606.x
Moore, J. W., and Schindler, D. E. (2010). Spawning salmon and the phenology of emergence in stream insects. Proc. R. Soc. B Biol. Sci. 277, 1695–1703. doi: 10.1098/rspb.2009.2342
Mouthon, J., and Daufresne, M. (2006). Effects of the 2003 heatwave and climatic warming on mollusc communities of the Saône: a large lowland river and of its two main tributaries (France). Glob. Change Biol. 12, 441–449. doi: 10.1111/j.1365-2486.2006.01095.x
Nixon, S. W. (1995). Coastal marine eutrophication: a definition, social causes, and future concerns. Ophelia 41, 199–219. doi: 10.1080/00785236.1995.10422044
Norman, M., and Green, J. (1958). The local influence of cattle dung and urine upon the yield and botanical composition of permanent pasture. Grass Forage Sci. 13, 39–45. doi: 10.1111/j.1365-2494.1958.tb00108.x
Northington, R. M., Keyse, M. D., Beaty, S. R., Whalen, S. C., Sokol, E. R., and Hershey, A. E. (2010). Benthic secondary production in eight oligotrophic arctic Alaskan lakes. J. N. Am. Benthol. Soc. 29, 465–479. doi: 10.1899/09-026.1
Novais, A., Batista, D., Cássio, F., Pascoal, C., and Sousa, R. (2017). Effects of invasive clam (Corbicula fluminea) die-offs on the structure and functioning of freshwater ecosystems. Freshw. Biol. 62, 1908–1916.
Novais, A., Souza, A. T., Ilarri, M., Pascoal, C., and Sousa, R. (2015). From water to land: how an invasive clam may function as a resource pulse to terrestrial invertebrates. Sci. Total Environ. 538, 664–671. doi: 10.1016/j.scitotenv.2015.08.106
Ochumba, P. B. (1990). Massive fish kills within the Nyanza Gulf of lake Victoria, Kenya. Hydrobiologia 208, 93–99. doi: 10.1007/bf00008448
Osterhaus, A., Groen, J., Niesters, H., van de Bildt, M., Martina, B., Vedder, L., et al. (1997). Morbillivirus in monk seal mass mortality. Nature 388:838. doi: 10.1038/42163
O’Toole, S., Metcalfe, C., Craine, I., and Gross, M. (2006). Release of persistent organic contaminants from carcasses of Lake ontario chinook salmon (Oncorhynchus tshawytscha). Environ. Pollut. 140, 102–113. doi: 10.1016/j.envpol.2005.06.019
Parmenter, R. R., and Lamarra, V. A. (1991). Nutrient cycling in a freshwater marsh: the decomposition of fish and waterfowl carrion. Limnol. Oceanogr. 36, 976–987. doi: 10.4319/lo.1991.36.5.0976
Patrick, C. J., McGarvey, D. J., Larson, J. H., Cross, W. F., Allen, D. C., Benke, A. C., et al. (2019). Precipitation and temperature drive continental-scale patterns in stream invertebrate production. Sci. Adv. 5:eaav2348. doi: 10.1126/sciadv.aav2348
Pechal, J. L., and Benbow, M. E. (2016). Microbial ecology of the salmon necrobiome: evidence salmon carrion decomposition influences aquatic and terrestrial insect microbiomes. Environ. Microbiol. 18, 1511–1522. doi: 10.1111/1462-2920.13187
Pechal, J. L., Crippen, T. L., Cammack, J. A., Tomberlin, J. K., and Benbow, M. E. (2019). Microbial communities of salmon resource subsidies and associated necrophagous consumers during decomposition: potential of cross-ecosystem microbial dispersal. Food Webs 19:e00114. doi: 10.1016/j.fooweb.2019.e00114
Polis, G. A. (1994). Food webs, trophic cascades and community structure. Austr. J. Ecol. 19, 121–136. doi: 10.1111/j.1442-9993.1994.tb00475.x
Polis, G. A., Anderson, W. B., and Holt, R. D. (1997). Toward an integration of landscape and food web ecology: the dynamics of spatially subsidized food webs. Ann. Rev. Ecol. Syst. 28, 289–316. doi: 10.1146/annurev.ecolsys.28.1.289
Polis, G. A., and Hurd, S. D. (1996). Linking marine and terrestrial food webs: allochthonous input from the ocean supports high secondary productivity on small islands and coastal land communities. Am. Natur. 147, 396–423. doi: 10.1086/285858
Preisser, E. L., Bolnick, D. I., and Benard, M. F. (2005). Scared to death? The effects of intimidation and consumption in predator–prey interactions. Ecology 86, 501–509. doi: 10.1890/04-0719
Quaggiotto, M.-M., Barton, P. S., Morris, C. D., Moss, S. E. W., Pomeroy, P. P., McCafferty, D. J., et al. (2018). Seal carrion is a predictable resource for coastal ecosystems. Acta Oecol. 88, 41–51. doi: 10.1016/j.actao.2018.02.010
Rachowicz, L. J., Knapp, R. A., Morgan, J. A., Stice, M. J., Vredenburg, V. T., Parker, J. M., et al. (2006). Emerging infectious disease as a proximate cause of amphibian mass mortality. Ecology 87, 1671–1683. doi: 10.1890/0012-9658(2006)87%5B1671:eidaap%5D2.0.co;2
Ramirez, R. A., and Snyder, W. E. (2009). Scared sick? Predator–pathogen facilitation enhances exploitation of a shared resource. Ecology 90, 2832–2839. doi: 10.1890/08-1941.1
Rand, G. M., Wells, P. G., and McCarty, L. S. (1995). “Introduction to aquatic toxicology,” in Fundamentals of Aquatic Toxicology, 2nd Edn, ed. G. M. Rand, (Washington, D.C: Taylor & Francis), 3–67.
Reimchen, T. E. (1994). Further Studies of Black Bear and Chum Salmon in Stream and Estuarine Habitats at Bag Harbour, Gwaii Haanas. Queen Charlotte City, B.C: Canadian Parks Service.
Reisdorf, A. G., Bux, R., Wyler, D., Benecke, M., Klug, C., Maisch, M. W., et al. (2012). Float, explode or sink: postmortem fate of lung-breathing marine vertebrates. Palaeobiodivers. Palaeoenviron. 92, 67–81. doi: 10.1007/s12549-011-0067-z
Richey, J. E., Perkins, A. M., and Goldman, C. R. (1975). Effects of kokanee salmon (Oncorhynchus nerka) decomposition on the ecology of a subalpine stream. J. Fish. Board Canada 32:817. doi: 10.1139/f75-109
Rowe, L., Berrill, M., Hollett, L., and Hall, R. J. (1989). The effects of short-term laboratory pH depressions on molting, mortality and major ion concentrations in the mayflies Stenonema femoratum and Leptophlebia cupida. Hydrobiologia 184, 89–97. doi: 10.1007/bf00014305
Sato, T., Arizono, M., Sone, R., and Harada, Y. (2008). Parasite-mediated allochthonous input: do hairworms enhance subsidized predation of stream salmonids on crickets? Can. J. Zool. 86, 231–235. doi: 10.1139/z07-135
Sato, T., Egusa, T., Fukushima, K., Oda, T., Ohte, N., Tokuchi, N., et al. (2012). Nematomorph parasites indirectly alter the food web and ecosystem function of streams through behavioural manipulation of their cricket hosts. Ecol. Lett. 15, 786–793. doi: 10.1111/j.1461-0248.2012.01798.x
Sato, T., Watanabe, K., Kanaiwa, M., Niizuma, Y., Harada, Y., and Lafferty, K. D. (2011a). Nematomorph parasites drive energy flow through a riparian ecosystem. Ecology 92, 201–207. doi: 10.1890/09-1565.1
Sato, T., Watanabe, K., Tokuchi, N., Kamauchi, H., Harada, Y., and Lafferty, K. D. (2011b). A nematomorph parasite explains variation in terrestrial subsidies to trout streams in Japan. Oikos 120, 1595–1599. doi: 10.1111/j.1600-0706.2011.19121.x
Schindler, D. E. (1992). Nutrient regeneration by sockeye salmon (Oncorhynchus nerka) fry and subsequent effects on zooplankton and phytoplankton. Can. J. Fish. Aquat. Sci. 49:2498. doi: 10.1139/f92-276
Schindler, D. E., Scheuerell, M. D., Moore, J. W., Gende, S. M., Francis, T. B., and Palen, W. J. (2003). Pacific salmon and the ecology of coastal ecosystems. Front. Ecol. Environ. 1, 31–37. doi: 10.1890/1540-92952003001[0031:PSATEO]2.0.CO;2
Schmidt-Rhaesa, A. (2001). The life cycle of horsehair worms (Nematomorpha). Acta Parasitol. 46, 151–158.
Schuldt, J. A., and Hershey, A. E. (1995). Effect of salmon carcass decomposition on Lake superior tributary streams. J. N. Am. Benthol. Soc. 14, 259–268. doi: 10.2307/1467778
Scott, M. P. (1998). The ecology and behavior of burying beetles. Ann. Rev. Entomol. 43, 595–618. doi: 10.1146/annurev.ento.43.1.595
Seastedt, T., Briggs, J., and Gibson, D. (1991). Controls of nitrogen limitation in tallgrass prairie. Oecologia 87, 72–79. doi: 10.1007/BF00323782
Sheriff, M. J., Krebs, C. J., and Boonstra, R. (2009). The sensitive hare: sublethal effects of predator stress on reproduction in snowshoe hares. J. Anim. Ecol. 78, 1249–1258. doi: 10.1111/j.1365-2656.2009.01552.x
Smith, C. R., and Baco, A. R. (2003). Ecology of whale falls at the deep-sea floor. Oceanogr. Mar. Biol. 41, 311–354.
Strickland, M. S., and Wickings, K. (2015). “Carrion effects on belowground communities and consequences for soil processes,” in Carrion Ecology, Evolution, and Their Applications, eds E. M. Benbow, J. K. Tomberlin, and A. M. Tarone, (Milton Park: Taylor & Francis), 93–106. doi: 10.1201/b18819-7
Stuart-Smith, R. D., Brown, C. J., Ceccarelli, D. M., and Edgar, G. J. (2018). Ecosystem restructuring along the Great Barrier Reef following mass coral bleaching. Nature 560:92. doi: 10.1038/s41586-018-0359-9
Subalusky, A. L., Dutton, C. L., Rosi, E. J., and Post, D. M. (2017). Annual mass drownings of the Serengeti wildebeest migration influence nutrient cycling and storage in the Mara River. Proc. Natl. Acad. Sci. U.S.A. 114, 7647–7652. doi: 10.1073/pnas.1614778114
Subalusky, A. L., Dutton, C. L., Rosi-Marshall, E. J., and Post, D. M. (2015). The hippopotamus conveyor belt: vectors of carbon and nutrients from terrestrial grasslands to aquatic systems in sub–Saharan Africa. Freshw. Biol. 60, 512–525. doi: 10.1111/fwb.12474
Subalusky, A. L., and Post, D. M. (2018). Context dependency of animal resource subsidies. Biol. Rev. 94, 517–538. doi: 10.1111/brv.12465
Swift, M. J., Heal, O. W., and Anderson, J. M. (1979). Decomposition in Terrestrial Ecosystems. Studies in Ecology. Oxford, UK: Blackwell Scientific.
Tang, K. W., Gladyshev, M. I., Dubovskaya, O. P., Kirillin, G., and Grossart, H.-P. (2014). Zooplankton carcasses and non-predatory mortality in freshwater and inland sea environments. J. Plankton Res. 36, 597–612. doi: 10.1093/plankt/fbu014
Tank, J. L., Rosi-Marshall, E. J., Griffiths, N. A., Entrekin, S. A., and Stephen, M. L. (2010). A review of allochthonous organic matter dynamics and metabolism in streams. J. N. Am. Benthol. Soc. 29, 118–146. doi: 10.1899/08-170.1
Thomas, F., Schmidt-Rhaesa, A., Martin, G., Manu, C., Durand, P., and Renaud, F. (2002). Do hairworms (Nematomorpha) manipulate the water seeking behaviour of their terrestrial hosts? J. Evol. Biol. 15, 356–361. doi: 10.1046/j.1420-9101.2002.00410.x
Thronson, A., and Quigg, A. (2008). Fifty-five years of fish kills in coastal Texas. Estuar. Coast. 31, 802–813. doi: 10.1007/s12237-008-9056-5
Thuesen, P. A., Ebner, B. C., Larson, H., Keith, P., Silcock, R. M., Prince, J., et al. (2011). Amphidromy links a newly documented fish community of continental Australian streams, to oceanic islands of the West Pacific. PLoS One 6:e26685. doi: 10.1371/journal.pone.0026685
Tiegs, S. D., Levi, P. S., Rüegg, J., Chaloner, D. T., Tank, J. L., and Lamberti, G. A. (2011). Ecological effects of live salmon exceed those of carcasses during an annual spawning migration. Ecosystems 14, 598–614. doi: 10.1007/s10021-011-9431-0
Towne, E. G. (2000). Prairie vegetation and soil nutrient responses to ungulate carcasses. Oecologia 122, 232–239. doi: 10.1007/PL00008851
Trumbo, S. T. (1992). Monogamy to communal breeding: exploitation of a broad resource base by burying beetles (Nicrophorus). Ecol. Entomol. 17, 289–298. doi: 10.1111/j.1365-2311.1992.tb01060.x
Vannote, R. L., Minshall, G. W., Cummins, K. W., Sedell, J. R., and Cushing, C. E. (1980). River continuum concept. Can. J. Fish. Aquat. Sci. 37, 130–137.
Vaughn, C. C. (2018). Ecosystem services provided by freshwater mussels. Hydrobiologia 810, 15–27. doi: 10.1007/s10750-017-3139-x
Vollenweider, R. A. (1970). Scientific Fundamentals of the Eutrophication of Lakes and Flowing Waters, With Particular Reference to Nitrogen and Phosphorus as Factors in Eutrophication. Paris: OECD.
Wallace, J. B., Eggert, S., Meyer, J. L., and Webster, J. (1999). Effects of resource limitation on a detrital-based ecosystem. Ecol. Monogr. 69, 409–442. doi: 10.1890/0012-9615(1999)069%5B0409:eorloa%5D2.0.co;2
Walters, D., Wesner, J. S., Zuellig, R. E., Kowalski, D. A., and Kondratieff, M. C. (2018). Holy flux: spatial and temporal variation in massive pulses of emerging insect biomass from western US rivers. Ecology 99, 238–240. doi: 10.1002/ecy.2023
Wardle, D. A., Bardgett, R. D., Klironomos, J. N., Setälä, H., van der Putten, W. H., and Wall, D. H. (2004). Ecological linkages between aboveground and belowground biota. Science 304, 1629–1633. doi: 10.1126/science.1094875
Waters, T. F. (1977). Secondary production in inland waters. Adv. Ecol. Res. 10, 91–164. doi: 10.1016/s0065-2504(08)60235-4
Weaver, D., Coghlan, S. Jr., Greig, H., Klemmer, A., Perkins, L., and Zydlewski, J. (2018). Subsidies from anadromous sea lamprey (Petromyzon marinus) carcasses function as a reciprocal nutrient exchange between marine and freshwaters. River Res. Appl. 34, 824–833. doi: 10.1002/rra.3291
Webster, J., and Benfield, E. (1986). Vascular plant breakdown in freshwater ecosystems. Ann. Rev. Ecol. Syst. 17, 567–594. doi: 10.1146/annurev.es.17.110186.003031
Wenger, S. J., Subalusky, A. L., and Freeman, M. C. (2019). The missing dead: the lost role of animal remains in nutrient cycling in North American rivers. Food Webs 18:e00106. doi: 10.1016/j.fooweb.2018.e00106
Wesner, J. S., Walters, D. M., and Zuellig, R. E. (2019). Pulsed salmonfly emergence and its potential contribution to terrestrial detrital pools. Food Webs 18:e00105. doi: 10.1016/j.fooweb.2018.e00105
Wetzel, R. G. (1995). Death, detritus, and energy flow in aquatic ecosystems. Freshw. Biol. 33, 83–89. doi: 10.1111/j.1365-2427.1995.tb00388.x
Willson, M. F., and Halupka, K. C. (1995). Anadromous fish as keystone species in vertebrate communities. Conserv. Biol. 9, 489–497. doi: 10.1046/j.1523-1739.1995.09030489.x
Wipfli, M. S., Hudson, J., and Caouette, J. (1998). Influence of salmon carcasses on stream productivity: response of biofilm and benthic macroinvertebrates in southeastern Alaska, USA. Can. J. Fish. Aquat. Sci. 55, 1503–1511. doi: 10.1139/f98-031
Wipfli, M. S., Hudson, J. P., Caouette, J. P., and Chaloner, D. T. (2003). Marine subsidies in freshwater ecosystems: salmon carcasses increase the growth rates of stream-resident salmonids. Trans. Am. Fish. Soc. 132, 371–381. doi: 10.1577/1548-8659(2003)132<0371:msifes>2.0.co;2
Yang, L. H. (2004). Periodical cicadas as resource pulses in North American forests. Science 306, 1565–1567. doi: 10.1126/science.1103114
Keywords: decomposition, microbial, ecosystem metabolism, community assembly, necrobiome, carrion, mass mortality, forensics
Citation: Benbow ME, Receveur JP and Lamberti GA (2020) Death and Decomposition in Aquatic Ecosystems. Front. Ecol. Evol. 8:17. doi: 10.3389/fevo.2020.00017
Received: 29 August 2019; Accepted: 21 January 2020;
Published: 07 February 2020.
Edited by:
Alexei B. Ryabov, University of Oldenburg, GermanyReviewed by:
Ralf Schulz, University of Koblenz Landau, GermanyZhang Yixin, Soochow University, China
Copyright © 2020 Benbow, Receveur and Lamberti. This is an open-access article distributed under the terms of the Creative Commons Attribution License (CC BY). The use, distribution or reproduction in other forums is permitted, provided the original author(s) and the copyright owner(s) are credited and that the original publication in this journal is cited, in accordance with accepted academic practice. No use, distribution or reproduction is permitted which does not comply with these terms.
*Correspondence: M. Eric Benbow, YmVuYm93QG1zdS5lZHU=
†ORCID: M. Eric Benbow, orcid.org/0000-0003-2630-0282