- Department of Marine and Environmental Sciences, Northeastern University, Boston, MA, United States
As an ecologically dominant taxon, termites appear to be resilient to environmental stressors. However, swarming alates (winged-individuals) encounter a myriad of environmental pressures that drastically reduce the probability of colony foundation. Dispersing alates face high rates of predation, desiccation, nitrogen limitation, and risks of infection, among others. We propose that alates benefit from mate assistance and biparental care to overcome some of these challenges. We assessed whether the bacteria, Serratia marcescens (an ecologically relevant, gram-negative, facultative termite pathogen), negatively affected the growth of newly founded termite colonies. Additionally, we revealed the significance of the king's presence in improving successful establishment of incipient colonies. Virgin queens of the dampwood termite, Zootermopsis angusticollis, were subjected to one of four treatments: naïve (untreated), or injections with either sterile saline, heat-killed S. marcescens, or a sublethal does of live S. marcescens. Each queen was then paired with a naïve, virgin king. The incipient colonies underwent censuses every 4 days for 80 days. We estimated survival rates and compared the onset of oviposition and hatching, overall egg production and larval hatching success, all as a function of queen treatment and the presence of a mate. We identified factors that, under pathogenic stress, influenced these fitness-related milestones. Queen infection significantly reduced the number of successfully established colonies. Moreover, both the presence of a king and his mass significantly influenced the queen's survival, her onset of oviposition, overall egg production, and hatching success. We conclude that termite colonies incur significant fitness costs after a queen suffers an acute infection and that the presence of a king (and his stored resources) may help mitigate the negative effects of a queen's infection. Pathogenic pressures, combined with the significant role of kings in colony success, appear to reinforce two-parent colony foundation, mate assistance, biparental care, and ultimately the overlap of generations, all of which have been considered pre-adaptations for eusociality. By studying the fitness consequences of pathogenic stress during the ontogeny of a termite colony, we can infer some of the conditions and pressures under which termite sociality likely emerged.
Introduction
Termites, and other eusocial insects (social Hymenoptera), have long garnered the interest of evolutionary biologists (e.g., Boomsma and Gawne, 2018, and references therein). In spite of the convergent social organization across all eusocial insects, termites represent an especially interesting taxon, differing from their social Hymenopteran counterparts in striking ways. Termites are hemimetabolous, diploid insects, their worker and/or soldier castes are typically composed of both males and females, they feed on a nitrogen-limited cellulose-based diet, and their colonies are mostly established by a monogamous reproductive pair that exhibits biparental care (Krishna and Wesner, 1970; Wilson, 1971; Shellman-Reeve, 1990, 1997a; Rosengaus and Traniello, 1991; Bignell et al., 2011). Unfortunately, studies on their evolutionary trajectory toward eusociality are hampered, in part, by the fact that this taxon lacks graded levels of sociality (Thorne, 1997; Korb and Throne, 2017). All termites are considered eusocial, whether facultatively (i.e., the worker and soldier castes retain the potential for reproduction) or obligatorily (workers and soldiers are sterile; Boomsma, 2013; Boomsma and Gawne, 2018). Hence, contrary to the common approach used in bees and wasps which exhibit a full spectrum of sociality (Hunt and Toth, 2017; Wcislo and Fewell, 2017), comparative approaches across levels of sociality within the single termite clade are not possible. Instead, we can make inferences about the origins and maintenance of their eusociality by using a combination of molecular phylogenetics, and ecological, physiological, nutritional, and behavioral comparisons with its related sister taxa, the subsocial wood roach, Cryptocercus (e.g., Wheeler, 1904; Shellman-Reeve, 1990; Nalepa, 1991, 2010, 2011; Inward et al., 2007; Klass et al., 2008; Todaka et al., 2010; Bourguignon et al., 2014, 2017; Tai et al., 2015; Korb and Heinze, 2016, Maekawa et al., 2008; Nalepa and Arellano, 2016; Harrison et al., 2018).
Here we use an alternative approach that may prove helpful in elucidating the selective pressures and intermediate steps that culminated in the evolution of termite eusociality. Studying the current selective pressures faced by incipient colonies in basal termite species allows us to make inferences about the transitions in social complexity based on proposed evolutionary scenarios (Figure 1). We therefore examined multiple fitness parameters of newly founded colonies while under pathogenic stress. We identified factors that constrained or promoted the successful establishment of a colony. These conditions may have been similar to the conditions and pressures under which termite sociality originated ~150 million years ago (Thorne, 1997; Bourguignon et al., 2014; Harrison et al., 2018).
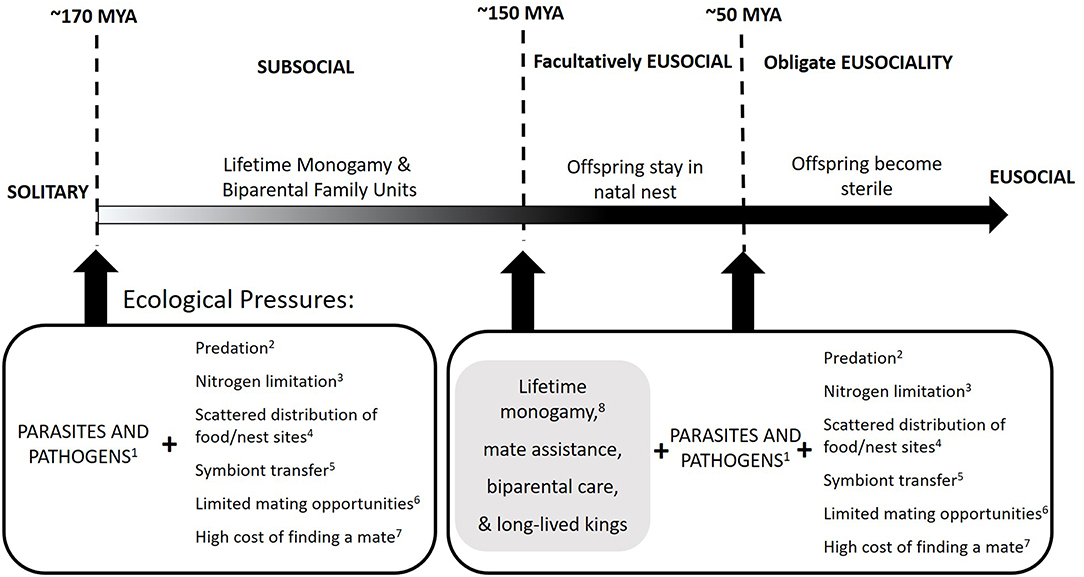
Figure 1. Proposed scenario of the evolution of termite sociality. Based on basic termite biology, previous empirical studies and theoretical frameworks, we propose an evolutionary scenario in which the transitions between levels of social complexity occurred along a continuum, spanning ~120 million years (MYA). Throughout this continuum, multiple, and sustained ecological pressures (including pathogenic stress) likely promoted the transition from a solitary to a subsocial lifestyle, which in the putative termite ancestor, likely consisted of monogamy, longer life expectancies of parents, mate assistance, and biparental care. These subsocial traits served as preadaptations (in gray box) which, together with continued strong ecological pressures, might have selected for non-dispersing progeny who retained reproductive potential (facultative eusociality; Boomsma, 2009, 2013; Boomsma and Gawne, 2018) by around ~150 MYA (Bourguignon et al., 2014). By ~50 MYA (Bourguignon et al., 2014, 2017), the evolution of sterility in the offspring took place (obligatory eusociality; Boomsma, 2009, 2013; Boomsma and Gawne, 2018). Given the risks posed by multiple environmental stressors, the fitness benefits accrued by progeny while rearing siblings likely outweighed any benefits from nest dispersal and personal reproduction. The dashed lines represent the timing of these major transitions in social complexity (Bourguignon et al., 2014, 2017; Harrison et al., 2018). Possible environmental factors influencing sociality throughout this continuum: 1Cruse (1998), Schmid-Hempel (1998), Rosengaus et al. (2000, 2003, 2011), Tunaz and Stanley (2009), Chouvenc et al. (2011) and Wilson-Rich et al. (2007). 2Delighne et al. (1981), Dial and Vaughan (1987), Lepage (1991), Matsuura and Nishida (2002) and Sheppe (1970). 3Nalepa (1991, 2010, 2015), Hunt and Nalepa (1994), Higashi et al. (2000) and Shellman-Reeve (2013). 4Hamilton (1978), Korb and Heinze (2008) and Nutting (1969). 5Nalepa (1991, 2015). 6Hamilton (1978) and Nutting (1969). 7Hamilton (1978) and Nutting (1969). 8Boomsma (2009, 2013).
As termite colonies develop, they progress through graded levels of sociality. First, winged reproductives swarm away from their natal colony without displaying any apparent social cohesion (~solitary stage). After attracting a mate, the de-winged alates establish a monogamous pair that engages in frequent social interactions (Nutting, 1969; Shellman-Reeve, 1990, 1999; Rosengaus and Traniello, 1991; Brent et al., 2007). Such monogamy often correlates with biparental care and mate assistance in termites (Shellman-Reeve, 1997a,b; Klug, 2018). These three traits are considered prerequisites for the evolution of termite eusociality, and eusocial evolution more broadly (Boomsma, 2009, 2013). Subsequently, the royal pair enters a subsocial phase, where the king and queen provide biparental care, functioning as a family unit while exploiting their nitrogen-poor wooden resources (Nalepa and Jones, 1991; Rosengaus and Traniello, 1991, 1993a). These family units then proceed toward more complex levels of sociality. The formerly altricial larvae start contributing labor in the colony (nest expansion, hygiene, royal, and brood care; Rosengaus and Traniello, 1993a; Brent and Traniello, 2001; Chouvenc and Su, 2017) while remaining within their natal nest (i.e., non-dispersing brood) and forgoing their own reproduction (either temporarily or permanently; Thorne, 1997; Boomsma, 2009, 2013; Boomsma and Gawne, 2018). It is at this developmental stage, that a termite colony attains the required traits of any eusocial species: reproductive division of labor, overlap of generations and cooperative brood care; as defined by Wilson (1971). By focusing on the incipient stages of colony foundation in the basal termite, Zootermopsis angusticollis, we identified some of the selective pressures affecting colony establishment. We also quantified their fitness-related milestones and made inferences about how mate assistance and biparental care help overcome the myriad stressors encountered by the founding pair.
In spite their reputation for hardiness, termite colonies have extremely low probabilities of becoming established, even under ideal laboratory conditions (Rosengaus and Traniello, 1993b; Fei and Henderson, 2003; Calleri et al., 2006; Hartke and Rosengaus, 2013; Cole et al., 2018). In nature, swarming alates fall prey to diverse aerial and terrestrial predators (Sheppe, 1970; Delighne et al., 1981; Dial and Vaughan, 1987; Lepage, 1991; Matsuura and Nishida, 2002). The few that survive have to quickly locate a mate, shed their wings, and search for an adequate nesting site before desiccation takes a toll (Nutting, 1969). The search for a nest involves scurrying above ground, under the leaf-litter and/or subterraneously, environments known for their high microbial, potentially pathogenic, loads (Cruse, 1998; Schmid-Hempel, 1998; Rosengaus et al., 2003, 2011; Tunaz and Stanley, 2009; Chouvenc et al., 2011). Once the nesting site is located, the future king and queen sequester themselves within the copularium where they likely encounter additional bacterial, fungal, viral pathogens as well as entomopathogenic nematodes (Schmid-Hempel, 1998; Rosengaus et al., 2000, 2003, 2011; Wilson-Rich et al., 2007).
Beyond the above-mentioned environmental challenges, the royal pair has to cope with intrinsic factors that further reduce their probability of colony establishment (Cole et al., 2018). These include their own genetic background, behavioral incompatibility with their mate, nutritional stress due to their cellulose-based diets, and limited stored resources (Cowling and Merrill, 1966; Nalepa, 1988; Hunt and Nalepa, 1994; Higashi et al., 2000; Bauerfeind and Fischer, 2005; Shellman-Reeve, 2013; Cole et al., 2018; Nottingham et al., 2018). The latter two are particularly important, as restricted energy must be allocated to several competing demands (Cole et al., 2018). These include nest construction, nest sanitation (via the deposition of antimicrobial compounds on their nesting substrate), courtship, copulation, gametogenesis, parental care (at least until the first altricial larvae become independent), and the generation of costly immune responses if exposed to pathogens (Armitage et al., 2003; Schwenke et al., 2016; Brace et al., 2017).
Recently, while investigating the short-term pathogen-induced fitness costs at the colony level, Cole et al. (2018) identified several extrinsic and intrinsic factors that affect the successful establishment of a termite colony within the first 30 days post-establishment. Here, through a series of new experiments, we expand on these results and assessed the longer-term, colony-wide fitness costs associated with queen's bacterial infection. We asked whether the initial pathogen-induced fitness costs are temporary or sustained for up to 80 days post-pairing. We compared queen and king survival, onset of oviposition, overall egg production, onset of hatching as well as hatching success during colony foundation, all as a function of queen bacterial exposure. By framing our results around basic termite biology, previous empirical and theoretical studies (Wilson, 1971, 1975; Hamilton, 1978; Nalepa, 1991, 2010, 2011; Thorne, 1997; Higashi et al., 2000; Korb, 2008a; Boomsma, 2009, 2013; Korb and Heinze, 2016; Nalepa and Arellano, 2016; Boomsma and Gawne, 2018), we reveal some of the underlying factors and dynamics fostering termite biparental care, mate assistance, and king longevity—all putative pre-adaptations for sociality while under a scenario of pathogenic stress.
Methods
Collection, Maintenance, and Establishment of Incipient Colonies
Male and female Z. angusticollis alates were retrieved from 10 different mature stock colonies, all collected from the Redwood East Bay Regional Parks, Oakland California. These colonies were transported to our USDA approved containment room (Northeastern University, Boston, MA; USDA Permit P526P-17-03814) and maintained at 25°C. Upon molting, the alates were removed, sexed, de-winged, weighed, queens were experimentally treated (see below) and then, paired inside a Petri dish (60 × 15 mm; lined with moist Whatman # 1 filter paper) with a naïve king (n = 271 pairs in total). We only used heavily pigmented alates who were physiologically and motivationally ready to mate (Cole et al., 2018). To each incipient colony, we added approximately ~2.5 mg of white birch (Betula) to provide a nesting/feeding substrate in which to build their initial mating chamber (copularium; Nutting, 1969). The incipient colonies were stacked inside covered plastic boxes lined with wet paper towel to keep high humidity. The colonies were watered and additional wood chips added as needed. To ensure robust sample sizes of surviving incipient colonies across all treatments (see below), we purposefully established a surplus of incipient colonies headed by queens infected with a sublethal dose of live Serratia marcescens (see below). Our original bias explains the unbalanced sample sizes across treatments. Based on the non-synchronized swarming under our laboratory conditions, we establish incipient colonies headed by either, kings and queens collected from the same (nestmates, n = 178) or different parental colonies (non-nestmates, n = 93).
Experimental Design and Colony Census
We quantified the colony-wide fitness consequences of a queen's bacterial infection by establishing colonies headed by naïve queen + naïve king: n = 76; Burnes-Tracey Saline (BTS) -injected queen + naïve king: n = 49; 107 CFU/mL heat-killed S. marcescens (HK-Sm) queen + naïve king: n = 50; 2 × 105 CFU/mL sublethal dose of live S. marcescens (live-Sm) queen + naïve king: n = 96 (bacterial culturing protocol in Supplementary Methods 1). S. marcescens is a common Gram-negative soil bacterium known to naturally infect termites upon entering the hemocoel, either through the gut lining or via cuticular wounding (Chouvenc et al., 2011; Mirabito and Rosengaus, 2016). Previous work has shown that more than 50% of individuals survive a dose 1 μl injection of 2 × 105 CFU/mL of live S. marcescens, and hence it is considered sublethal (Cole et al., 2018). Such a sub-lethal dose allows us to study the effects of pathogenic stress in incipient colonies during the production of the first brood while maintaining a reasonable sample size. Periodic census were performed every 4 days for 80 days in which we recorded queen and king survival, number of days elapsed till the onset of oviposition, total number of eggs at 80 days (a measure of overall fecundity), onset of hatching and hatching success.
Microinjections
To mimic the natural infectious process, and to administer precise known pathogen loads, we cultured (Supplementary Methods 1) and injected 1 μL suspensions of S. marcescens suspended in sterile BTS or sterile BTS alone. All injections were performed as described previously in Cole et al. (2018). Briefly, after 10–20 min of cold immobilization, the queens were injected with a picospritzer III (Parker Hannifin) and a pulled borosilicate capillary tube with a 2 μm diameter at its point. Queens were allowed to recover for 1 h prior to pairing with their naïve mates.
Statistical Analysis
All statistics were run using IBM SPSS version 24.
Survival of Queens and Kings
We first ran separate Cox proportional hazards regression models (henceforth, Cox model) for each sex across the entire 80-day census period (Supplemental Tables 1, 2). These models included queen treatment and whether the mate was present or not (i.e., mate death), as extrinsic covariates. Mate death in these (and subsequent) models allowed us to estimate fitness-related benefits attained from mate assistance and biparental care. Queen mass, king mass, and “nestmate vs. non-nestmate pairs” were also included as intrinsic (inherent to the individual) covariates. All survival curves across 80 days post-pairing showed a clear inflection point at ~day 20 post-pairing (Supplemental Figure 1). Given these inflection points, the survival data violated the assumptions of proportional hazards (Kleinbaum and Klein, 2012). For this reason, we ran two additional separate Cox models for each sex, one targeting days 0–20 and the other focusing on days 21–80 post-pairing. Both models included the same extrinsic and intrinsic variables described for the 80–day analysis. Where appropriate, post-hoc pairwise comparisons were performed using a Bonferroni correction, setting a more conservative threshold p-value of 0.008 (Rice, 1989).
Fitness Consequences of Queen's Treatment
Likelihood and onset of oviposition
We first ran a single Cox model for the entire 0–80 day period to estimate the likelihood of oviposition. The model included all established incipient colonies—those in which the parents survived even if they yielded no eggs as well as those in which one or both of the parents died after oviposition. The following extrinsic covariates were included: queen treatment, mate death and whether or not they were paired with a nestmate or non-nestmate. The intrinsic covariates included queen mass and king mass. From these Cox models, we can draw information on both the onset (time course of egg-laying on the x-axis) as well as the likelihood (proportion of egg-laying colonies on the y-axis) of oviposition. We also ran an identical second Cox model, in which, only colonies that had produced at least one egg were included. Together, both models provide a more complete picture of the effects that each intrinsic and extrinsic variable has on the production of the first brood of eggs.
Total egg and larvae counts by day 80
To assess the effect of queen treatment on the overall number of eggs and larvae produced by day 80, regardless of the viability of those eggs, we ran two mixed effect models. The first model was generated for the total number of eggs produced within each colony, and the second on total number of larvae produced by day 80, both as a function of queen treatment. These models were identical except in the dependent variable, and included all of the originally established colonies. The fixed variables included queen treatment, nestmate vs. non-nestmate pairs, and mate death. Queen and king mass, and onset of oviposition were included as continuous covariates. Accounting for onset of oviposition was important as a later onset of oviposition was bound to result in lower overall egg count due to the fixed endpoint of the 80-day experiment. The models also included the following interactions: queen treatment × queen mass, queen treatment × king mass, queen treatment × king death, and queen treatment × queen death.
Likelihood and onset of hatching
Two Cox models were generated, one to estimate the likelihood of hatching and the second to determine the onset of hatching. The former model included all colonies regardless of queen and king survival, oviposition, and larval hatching status. The second model included only those colonies that had at least one larvae by day 80. Both models encompassed the entire 80-day period, and included both extrinsic categorical covariates (queen treatment, mate death, nestmate vs. non-nestmate pairs) and intrinsic continuous covariates (queen and king mass, onset of oviposition).
Hatching success
We defined hatching success as the percent of oviposited eggs that actually yielded larvae. Given that hatching success is constrained by the number of eggs present in the incipient colony, this analysis only included colonies that produced eggs regardless of whether king and/or queen died or not. This GLMM included queen treatment, nestmate vs. non-nestmate pairs, mate death, as fixed factors; queen mass, king mass and onset of oviposition as continuous covariates and the following interactions: queen treatment × queen mass, queen treatment × king mass, queen treatment × king death, and queen treatment × queen death.
Proportion of intact colonies 80 days post-pairing
We assumed that hatched larvae had the potential for maturing into functional workers past the 80-day experimental period. Thus, to estimate the probability that an incipient termite colony, while under pathogenic pressures, reached the initial colony growth phase (Cole et al., 2018), we defined “intact colonies” as those with both a surviving king, queen and at least one hatched larvae. To test whether the number of intact incipient colonies was disproportionately lower when queens experienced pathogenic stress, we compared the absolute number of intact vs. non-intact incipient colonies as a function of queen treatment with a 2 × 4 chi-square test.
Results
Queen Survival
Queen survival between days 0–20 was significantly influenced by both queen treatment (Wald = 19.1, df = 3, p < 0.001; Figure 2A) and the king's survival (Wald = 12.5, df = 1, p < 0.001; Figure 2B). Live-Sm injected queens were 2.9 times more susceptible than naïve queens (Figure 2A) and after controlling for the effect of all other variables in the model, including queen treatment, queens without a mate were 1.9 times more likely to die than queens with a living partner were (Figure 2B). No other factor influenced queen survival (Supplemental Table 3).
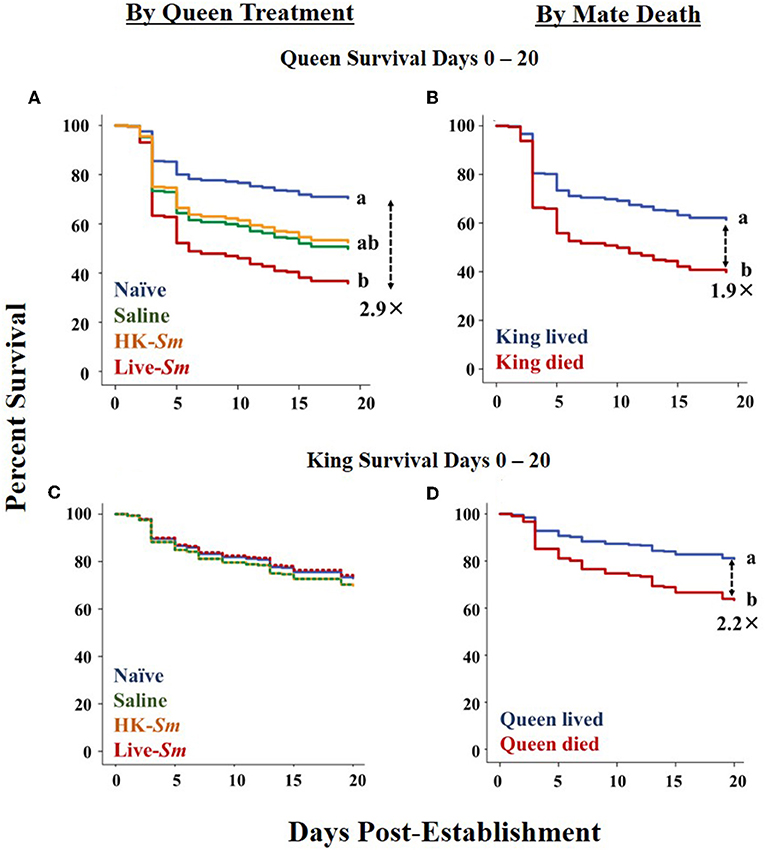
Figure 2. Survival curves across the first 20 days post-establishment (Cox model). (A) Queen survival as a function of queen treatment. (B) Queen survival as a function of king death. (C) King survival as a function of queen treatment. The survival curves for kings mated to saline queens overlaps with those mated to HK-Sm queens. Similarly, there is overlap between the curves of kings mated to saline and live-Sm queens. (D) King survival as a function of the death of his queen. Dashed arrows with corresponding numbers indicate hazard ratios of death between the significant pairwise comparisons.
In contrast to the 0–20 day period, queen survival between days 21–80 post-pairing was not influenced by her treatment (Wald = 5.3, df = 3, p = 0.2; Figure 3A). Instead, her survival was significantly influenced by whether she was accompanied by her mate or not (Wald = 20.9, df = 1, p < 0.001, Figure 3B) and his mass (Wald = 3.7, df = 1, p = 0.05). After controlling for the effects of all other variables in the model, queens without a living partner were 10.6 times more likely to die than their paired counterparts (Wald = 20.9, df = 1, p < 0.001; Figure 3B; Supplemental Table 4).
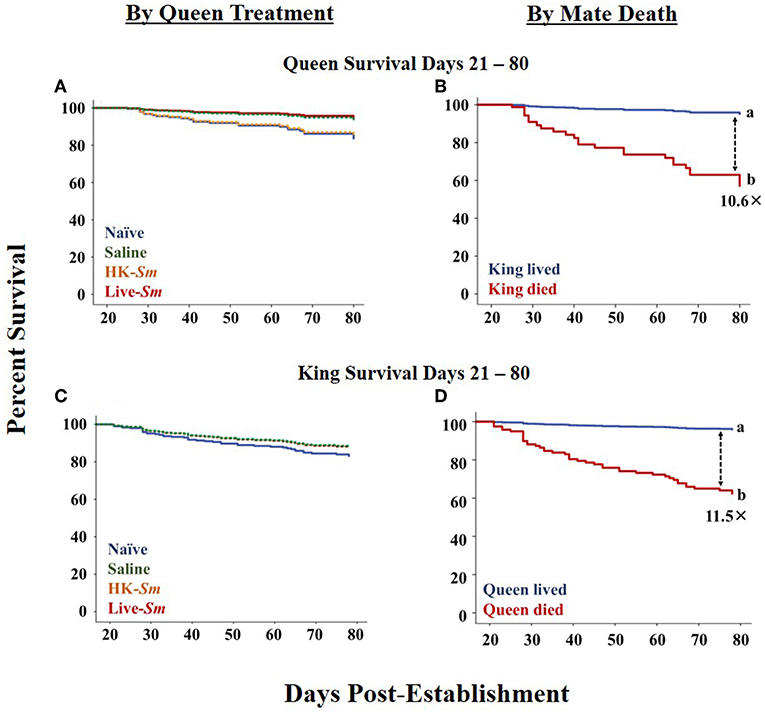
Figure 3. Survival curves across days 21–80 post-establishment (Cox model). (A) Queen survival as a function of queen treatment. The survival curves for live-Sm and saline, and the naive and HK-Sm, treatments overlap. (B) Queen survival as a function of king death. (C) King survival as a function of queen treatment. The survival curves for kings mated to saline, HK-Sm and live-Sm queens overlap, and resemble a single line. (D) King survival as a function of queen death. Dashed arrows with corresponding numbers indicate hazard ratios of death between significant pairwise comparisons. Note that the magnitude of the hazard ratios of death when an individual's mate dies (B and D) are an order of magnitude higher on days 21–80 post-establishment than on days 0–20 (Figures 2B,D).
King Survival
Between days 0–20 post-pairing, survival of the naïve kings was significantly influenced only by the survival of his queen (Wald = 8.7, df = 1, p = 0.003; Figures 2C,D). Kings who lost their queen were 2.1 times more likely to die than kings accompanied by a living partner (Figure 2D). No other variable, including queen treatment (Wald = 0.8, df = 3, p = 0.9), influenced king survival at this early stage of colony life (Supplemental Table 5).
King survival between days 21–80 post-pairing was also not influenced by queen treatment (Wald = 0.9, df = 3, p = 0.8; Figure 3C; Supplemental Table 6). His survival however, was significantly dependent on the queen's survival (Wald = 20.9, df = 1, p < 0.001; Figure 3D), with kings whose queens died being 11.5 times more likely to die than those with surviving queens. Interestingly, between days 21–80, whether or not a king was paired with a nestmate or non-nestmate queen was also a significant factor influencing his survival (Wald = 4.8, df = 1, p = 0.03, Supplemental Table 6).
Likelihood and Onset of Oviposition
Queen treatment was a marginally significant predictor of her likelihood to oviposit (Wald Statistic = 7.3, df = 3, p = 0.06, Figure 4, Supplemental Table 7). In pairwise comparisons and after adjusting the p-value with a Bonferroni correction, the live-Sm treatment had a tendency for lower likelihood of oviposition relative to the other three treatments. Compared to naïve queens, live-Sm queens were 1.8 times less likely to oviposit (Wald = 5.9, df = 1, p = 0.015). Live-Sm queens were also 1.7 and 1.9 times less likely to oviposit than saline and HK-Sm queens (Wald = 3.4, df = 1, p = 0.065; Wald = 5.0, df = 1, p = 0.025, respectively; Figure 4A). Both queen death and king mass were significant and independent predictors of likelihood of oviposition (Wald = 83.8, df = 1, p < 0.001, Figure 4B; Wald = 14.2, df = 1, p < 0.001, respectively). Colonies whose queens died within the 80-day period were 9.7 times less likely to produce at least one egg (Figure 4B). King mass was also a significant predictor of the onset of oviposition (Wald = 17.4, df = 1, p < 0.001). No other factors were a significant predictor of either the likelihood or the onset of oviposition (Supplemental Tables 7, 8; Supplemental Figure 2).
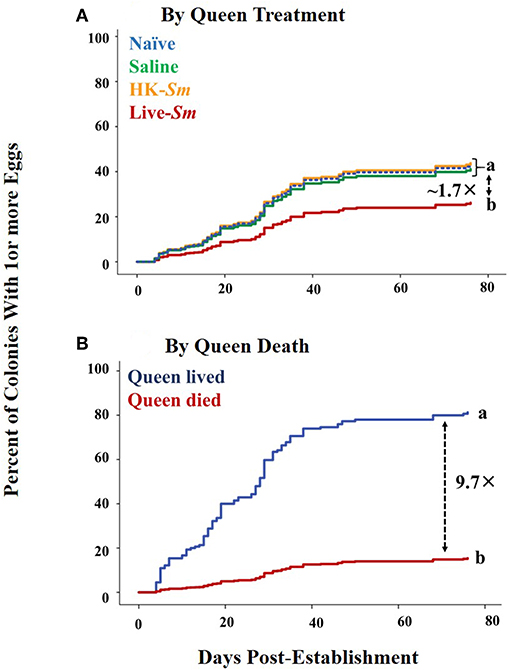
Figure 4. Likelihood of oviposition across the first 80 days post-establishment (Cox models). (A) Likelihood of oviposition as a function of queen treatment. Of the established 271 incipient colonies, only 120 (44.3%) oviposited at least one egg within the first 80 days post-pairing (n = 45 colonies headed by naïve queens + naïve kings; n = 22 by saline queens + naïve kings; n = 26 by HK-Sm queens + naïve kings; n = 27 by live-Sm queens + naïve kings). (B) Likelihood of oviposition as a function of queen death. Differing letters denote statistical significance. Dashed arrow with corresponding number indicate how many times greater the likelihood of oviposition is between significant pairwise comparisons.
Overall Egg Count
Onset of oviposition was predictive of overall egg count (F = 123.0, df = 1, 247, p < 0.001). After accounting for the effects of onset of oviposition, queen and king mass had both significant main effects (F = 8.6, df = 1, 247, p = 0.004, Supplemental Table 10; F = 5.2, 1, 247, p = 0.02, Supplemental Table 11, respectively), and significant interactions with queen treatment (F = 5.1, df = 3, 247, p = 0.002; F = 4.4, 3, 247, p = 0.005, respectively). When queens were injected with saline, their mass positively correlated with egg count, producing 0.34 ± 0.0.08 SE eggs/mg. Given that queen mass ranged from 24.2 to 82.2 mg, the heaviest queens produced ~19 more eggs on average than the lighter queens. Likewise, when queens were naïve, king mass positively correlated with egg count: for every mg of his mass, his queens produced 0.35 ± 0.03 SE more eggs. King mass also positively correlated with egg count when queens received the HK-Sm treatment (0.31 ± 0.19 SE eggs/mg). King mass did not appear to impact egg production when queens were infected with live S. marcescens (Supplemental Table 11). No other variable in the model was significant (Supplemental Table 10).
Likelihood and Onset of Hatching
After controlling for the effect of onset of oviposition (Wald = 35.5, df = 1, p < 0.001), queen treatment was not a significant predictor of larval hatching (Wald = 1.8, df = 3, p = 0.6; Supplemental Figure 3; Supplemental Table 12). The death of the king, however, was a significant an independent predictor of how likely eggs were to hatch by the end of the 80-day period (Wald = 9.1, df = 1, p = 0.003). If the king lived, a colony was 3.3 times more likely to produce larvae than their counterparts with no kings. The onset of hatching was similar for colonies with and without surviving kings (Supplemental Table 13). The death of the queen also predicted if a colony produced larvae (Wald = 3.8, df = 1, p = 0.05). Colonies with surviving queens were 2.6 times more likely to produce larvae. As with the kings, queen death did not impact the onset of hatching, only its likelihood. No other variables significantly predicted likelihood of hatching or the onset of hatching (Supplemental Figure 4; Supplemental Tables 12, 13).
Hatching Success
After controlling for onset of oviposition (F = 8.8, df = 1, 97, p = 0.004), the presence or absence of the king was a significant and independent predictor of the percentage of eggs that hatched. On average, colonies with surviving kings hatched three times the proportion of eggs compared to those in which kings died (Figure 5). Queen treatment and queen death had no impact on percent hatching (F = 0.1, df = 3, 97, p = 0.9; F = 0.5, df = 1, 97, p = 0.5, respectively). No other factor was significant (Supplemental Table 14).
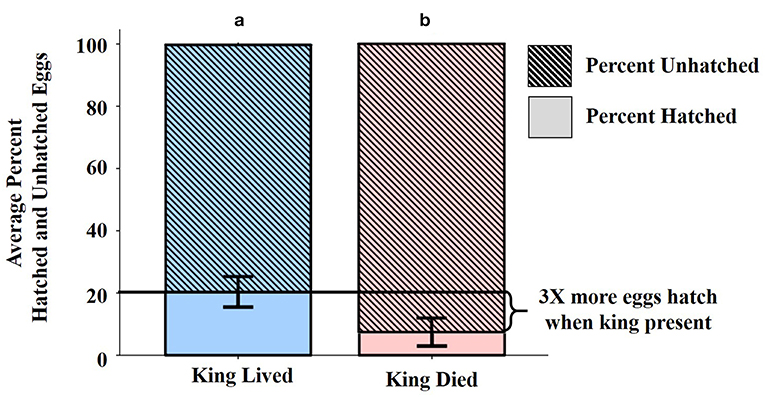
Figure 5. Average percent hatched and unhatched eggs as a function of king death. When kings lived, an average of 20% of a colony's eggs hatched compared to an average of only 7% when kings died. Differing letters denote statistical significance. Error bars represent ± 2 SD around the average.
Total Number of Larvae
After controlling for the significant effect of onset of oviposition (F = 37.4, df = 1, 246, p < 0.001), neither queen treatment nor king death were significant predictors of total larval count (F = 0.6, df = 3, 246, p = 0.6; F = 1.9, df = 1, 246, p = 0.2). The interaction “queen treatment × queen mass” approached significance (F = 1.9, df = 3, 246, p = 0.06; Supplemental Table 15). No other factor was significant (Supplemental Table 15).
Proportion of Intact Colonies by Day 80 Post-establishment
Only 14 and 14.6% of the originally established colonies headed by HK-Sm and live-Sm injected queens were scored as intact colonies relative to 20 to 25% of the colonies founded by naïve and saline-injected queens, respectively. These differences, however, were not statistically significant (χ2 = 3.9, df = 3, p = 0.3).
Discussion
To understand the drivers and dynamics that may have led to the evolution of eusociality as a viable strategy in termites, we can use present-day incipient colonies to identify current selection pressures and extrapolate how pathogens may have promoted their social evolution. Moreover, because newly established colonies transition during their ontogeny from solitary → subsocial → eusocial (whether facultatively or obligatorily; Boomsma and Gawne, 2018), we can tease apart the role and significance of each of these selection pressures against the backdrop of increased social complexity.
There are multiple factors influencing insect social evolution (Figure 1, and references therein). Here we focus on pathogenic pressures, which are particularly stringent in termites (Rosengaus and Traniello, 1993b; Schmid-Hempel, 1998; Rosengaus et al., 2000, 2003, 2011; Calleri et al., 2006). In the present study, we explored how colony foundation under pathogenic stress results in a bottleneck that is comprised of the two “fitness checkpoints” (Supplemental Figure 5). Our analyses also indicate that mate assistance mitigates some of the pathogen-induced fitness-related costs. Given that kings play such a significant role in present-day colonies, we propose that a king's presence and participation in tasks associated with colony establishment were crucial during the origins and maintenance of termite sociality. Below, we first introduce the amended checkpoint model of colony foundation, followed by a description of the role of pathogens during each checkpoint. We then discuss how mate assistance and biparental care may help mitigate fitness costs during these stages. Finally, we place our findings within the broader context of current theory in the evolution of insect sociality.
The “Checkpoints” Model of Colony Foundation
Cole et al. (2018) identified factors that influenced the successful establishment of a colony during the first 30-days. Based on these data, a colony passes through two “all-or-nothing” fitness checkpoints: initial survival (checkpoint 1) and oviposition (checkpoint 2). By expanding our census to encompass 80 days of colony life (Supplemental Figure 5), which now includes the subsocial phase, and using a new set of alates from different natal nests from those used by Cole et al. (2018), we not only confirm and replicate our initial observations, but also refine this model.
Based on the current data, checkpoint 1, characterized by a steep decline in the survival of reproductives, extends through the first 20 days post-establishment rather than the first 10 days as reported previously (Cole et al., 2018; Figures 2, 3). Checkpoint 2, which encompasses the onset of oviposition, now extends to day 40 (Figure 4). Irrespective of bacterial infection of one or both members of the royal pair, these two checkpoints result in a significant bottleneck with a massive loss of colonies (present study; Cole et al., 2018). These results, in combination with previous work (Rosengaus and Traniello, 1993b; Shellman-Reeve, 1997a; Hartke and Rosengaus, 2013; Chouvenc et al., 2014; Cole et al., 2018), supports the assertion that the incipient stage of colony foundation in termites is a vulnerable time, influenced by multiple intrinsic and extrinsic factors, including pathogens. Once oviposition begins, the colony enters the “initial growth phase” (Cole et al., 2018), characterized by the presence of eggs and the first dependent hatchlings (Supplemental Figure 5). We did not follow colonies past this phase, yet, we assume that once the hatchlings become independent, functional workers (~3rd instar; Rosengaus and Traniello, 1993b; Crosland et al., 1998), the colony reaches the ergonomic growth phase (Oster and Wilson, 1978).
Pathogenic Stress Negatively Influences the First Two Checkpoints
Checkpoint 1: Survival
Our initial 80-day model of survival showed a clear inflection point at day ~20 (Supplemental Figure 1), suggesting that different selection pressures influence the survival of reproductives at different times during colony ontogeny. Separating our survival analyses within each sex into the periods before and after day 20 post-establishment, we identified relatively short-term effects of pathogenic stress on the survival of treated queens (Figure 2A). There was no evidence that pathogenic stress affected queen survival from days 21–80 (Figure 3A), or that queens infected their kings at any time throughout the census period (Figures 2C, 3C). Likewise, Cole et al. (2018) found that direct exposure of kings to live Serratia also reduced their survival. These two studies strongly suggest that direct pathogenic exposure reduces survival and thus, decreases the number of colonies that pass through the first checkpoint (Supplemental Figure 5).
Checkpoint 2: Oviposition
We compared both the likelihood and onset of oviposition as a function of queen treatment. Live-Sm queens were the least likely to produce eggs, with only 26.1% of queens laying at least one egg compared to the ~42% of any of the other three treatments (Figure 4A). The likelihood of oviposition by live-Sm injected queens was the lowest relative to all other three treatments, hinting at possible physiological and fitness-related costs associated with bacterial exposure throughout checkpoint 2. When focusing only on those colonies that produced at least one egg, the majority began oviposition between days 15–35 (Supplemental Figure 2). Only 4% of these colonies initiated oviposition after day 40 (Supplemental Figure 2). In agreement with Cole et al. (2018), the present data indicate that queen treatment did not significantly influence the onset of egg laying even after expanding the colony census by an additional 50 days from Cole et al. (2018; Supplemental Figure 2). This lends support to the idea that the timing of oviposition is a fixed trait, impervious to pathogenic stress.
Initial Growth Phase
Although queen treatment was not a significant predictor of hatching success, only ~14% of colonies exposed to S. marcescens (live or heat-killed) were intact, having two surviving parents and larvae by day 80, compared to the 25 and 20% of intact naïve and saline colonies, respectively. These proportions did not differ significantly from each other, however, we suspect this is due to the low sample size of intact colonies after the bottleneck of checkpoints 1 and 2. The lack of statistical significance do not rule out the possibility of longer-term, cascading effects of pathogenic stress beyond colony foundation.
Our present data, together with that of Cole et al. (2018), demonstrate that S. marcescens has relatively short-term effects, reducing the survival of the royal pair during checkpoint 1 and the likelihood of oviposition during checkpoint 2 (Supplemental Figure 5). Bacterial exposure did not affect the onset of oviposition, nor egg quality (Cole et al., 2018). We argue that any strategy that ameliorates the fitness costs during these checkpoints would provide a significant fitness advantage and therefore, should be favored by natural selection. The current data provide evidence that the presence of the king (i.e., his mate assistance and biparental care) help realize this advantage.
Mate Assistance Increases Colony Success
Checkpoint 1: Mate Presence Enhanced Survival
In agreement with previous work (Shellman-Reeve, 1997a; Rosengaus et al., 2000), both queens and kings benefited from the presence of a living mate throughout the first 80 days of colony life (Figures 2, 3). Interestingly, the magnitude of these benefits differed when comparing days 0–20 vs. 21–80. Within the first 20 days post-establishment, queens without a mate were almost twice as likely to die, but, during days 21–80, these queens were 10.6 times more likely to die. Kings without a mate exhibited similar patterns, showing 2.2 and 11.5 times higher likelihood of death, relative to accompanied kings on days 0–20 and 21–80, respectively. Thus, in terms of survival, the persistence of a royal pair helps to shield the new colony against the many stressors of colony foundation, including pathogenic stress.
Mechanisms underlying these advantages could include mutual grooming to remove cuticular pathogens (Cruse, 1998; Rosengaus et al., 2000), proctodeal exchanges resulting in the transfer of protein-rich secretions (Shellman-Reeve, 1990) and/or the exchange immune-factors via trophallaxis (e.g., Traniello et al., 2002; Hamilton et al., 2011; but see Mirabito and Rosengaus, 2016), all of which can mitigate the threat of pathogens. Evolutionarily, such co-dependency between queens and kings could have reinforced the reproductive caste's longer-lifespans and two-parent colony foundation, presumed steppingstones in the evolution of eusociality (Boomsma, 2009; Davies and Gardner, 2018; Klug, 2018).
Checkpoint 2: Kings Enhance Oviposition and Overall Egg Production
Surviving queens mated to heavier kings (i.e., assumed to have greater energetic/metabolic resources) were more likely to oviposit and initiated oviposition sooner than queens mated to lighter kings (Supplemental Table 8). With respect to the overall number of eggs laid by day 80, naïve, saline-, and HK-Sm-treated queens mated with well-resourced kings produced a greater number of eggs than those mated to lighter kings (Supplemental Tables 9, 11). Interestingly, this effect was absent in live-Sm-injected queens, suggesting that the contributions made by a king are insufficient to boost the number of eggs produced by an infected queen. Thus, total egg production appeared to suffer due to pathogenic stress during the first 80-days post-establishment.
The fact that king mass consistently influenced the queen's reproductive output (current study; Cole et al., 2018; Chouvenc, 2019), suggests that well-resourced kings provide superior mate assistance, which in turn, increases colony fitness. Such assistance could be in the form of transfer of nutritious secretions via proctodeal trophallaxis (Shellman-Reeve, 1990), aid in housekeeping tasks such as nest construction and sanitation (Rosengaus and Traniello, 1991), and the promotion of ovarian maturation (Shellman-Reeve, 1999; Brent and Traniello, 2001; Brent et al., 2005). Behavioral observation are needed to pinpoint which of these forms of assistance play a role, if any.
The Initial Growth Phase: Kings Enhance Hatching Success
The likelihood of a colony producing larvae, and the proportion of hatched eggs, were most influenced by the presence of a surviving king (Supplemental Figure 3, Supplemental Tables 12, 14). On average, by day 80 post-establishment, colonies with surviving kings had three times the hatching success of those without kings. Hence, the king's presence, but not the queen's, significantly enhances hatching success. These data are in agreement with Shellman-Reeve (1990) who suggested that kings specialize in brood care, although Rosengaus and Traniello (1991) found no sex-based bias in brood care.
In spite the significant role that kings have on colony fitness between days 21–80, we were surprised that ~80% of eggs from colonies with a living king never hatched (Figure 5), indicating that colonies still face many stressors. There are at least three possible explanations for the low hatching success recorded in all colonies. We observed some eggs across all treatments that appeared to be infected with various bacterial or fungal pathogens, or were desiccated and misshapen (Supplemental Figure 6). There is also a high probability that kings and queens consumed some of the eggs. Cannibalism in termites is common (Sun et al., 2018) and has been considered to be a mechanism by which termites cope with nitrogen limitation (e.g., Hunt and Nalepa, 1994). By consuming eggs, the royal pair can recoup some of the nitrogen contained within those eggs. Additionally, culling a proportion of their eggs would reduce the number of dependent larvae needing future attention (Nalepa, 1988, 2010). By tailoring the number of larvae in a context-dependent fashion, the royal pair could optimize the energy allotted to all of the demands of colony foundation. Examples of culling are seen in a variety of taxa (e.g., Mehlis et al., 2009; Miller and Zink, 2012; Takata et al., 2013).
Monogamy, Mate Assistance, and Biparental Care: Preadaptations in the Evolution of Termite Eusociality
The hostile environment faced by dispersing alates may have promoted the evolution of monogamy, mate assistance, king longevity and biparental care in the termite ancestor (Hamilton, 1978; Nalepa, 1991, 2011; Hunt and Nalepa, 1994; Shellman-Reeve, 1997a,b; Thorne, 1997; Korb, 2008a; Korb and Heinze, 2016). We argue that, in addition to the many ecological pressures faced by new kings and queens (see Figure 1 and references therein), pathogenic pressures also selected for these same life-history traits that ultimately, served as pre-adaptations for the evolution of termite sociality.
In the present study, we used the “subsocial” phase of colony development in a basal termite species that is consistent with the proposed ancestral life style of one-piece nesting (Abe, 1987; Nalepa, 1988, 2010, 2011; Thorne, 1997; Inward et al., 2007; Klass et al., 2008; Korb, 2008a,b; Korb and Heinze, 2008; Bourguignon et al., 2014; Nalepa and Arellano, 2016; Harrison et al., 2018) to speculate on the possible role of pathogens in termite evolution. Our data show that in the face of disease, the presence of kings enhance colony fitness in several important ways (Figure 6; Supplemental Figure 5). The enhanced queen survival (Figures 2, 3) and enhanced hatching success (Figure 5) due to the king's presence may explain, in part, the longer lifespan of termite kings relative to that of drones of the social Hymenoptera, who die shortly after mating (Wilson, 1971). In the latter, any potential contributions a drone makes toward his queen or progeny can only occur during copulation. In termites, his continued contributions appear to have a sustained impact on colony fitness. If these results accurately reflect the circumstances under which termites evolved, then it is reasonable to hypothesize that long-lived ancestral males who provided mate assistance for longer periods would have reaped enhanced fitness benefits, reinforcing a monogamous mating strategy. This in turn, would have set the stage for prolonged family life.
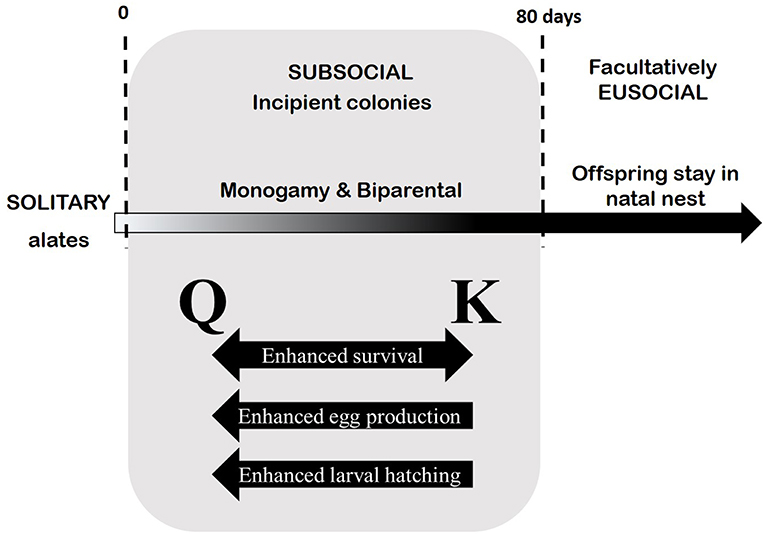
Figure 6. Ontogeny of present-day Z. angusticollis colonies informed by our current data. Gray box summarizes the results of our experiment manipulations, which inform our understanding of the dynamics between the queens and kings during the subsocial phase of colony life (~ first 80 days post-establishment). Specifically, the continued presence of the king and/or his stored resources (i.e., mass) result in enhanced fitness, as measured by queen survival, egg production and larval hatching success.
Boomsma (2009), for example, suggested that under conditions of lifetime monogamy by the diploid parental generation, genetic gains would have been equivalent whether progeny opted for personal reproduction or for becoming helpers at the nest. Workers would have still had on average, half of their genetic makeup represented. However, Korb and Schneider (2007) found that average relatedness in colonies of Cryptotermes secundus did not predict whether termite workers remained in the nest or developed into dispersing adults. Korb (2008a,b) and Korb and Heinze (2016) suggest that the harsh environment faced by dispersing individuals selects for progeny to remain in the nest. Such false-workers in one-piece nesting species reap direct fitness by inheriting the nest and the reproductive position. Although no consensus exists yet, it is likely that both the equivalent fitness gains derived from monogamy in the diploid king and queens, together with the harsh ecological pressures faced by dispersing alates, played significant roles in the origins of termite eusociality. Additional benefits of colony life could have further promoted social cohesion of early termites, including improved nitrogen recycling (Potrikus and Breznak, 1981; Machida et al., 2001), the reproductive specialization of queens (Oster and Wilson, 1978), and notably, colony-wide social immunity (Traniello et al., 2002; Cremer et al., 2018). We argue that pathogens, along with other environmental stressors, could have posed significant selection pressures that reinforced monogamy, king longevity, mate assistance, and brood care. The data we present here support the maintenance of two-parent colony foundation in termites which may have promoted monogamy thereby paving the way for inclusive fitness benefits for non-reproducing termites as predicted by Boomsma (2009, 2013). Our data also support the costs associated with dispersal, as alates that attempt to found new colonies face a reduced risk of survival, even in the absence of predators. Such costs of dispersal, in combination with the probability of becoming a reproducing individual likely promoted staying in the natal nest (Korb, 2008a; Korb and Heinze, 2016).
Conclusion
Dispersing termite alates face a hostile environment, including pathogens. Even under ideal conditions, few incipient colonies pass through the bottleneck (checkpoints 1 and 2). Yet, the presence of pathogens further exacerbates colony failure. An acute sublethal bacterial infection reduces alate survival. For those that survive, pathogenic stress has cascading consequences on several fitness-related parameters. Our results indicate that mate assistance and biparental care can mitigate some of the negative effects of infection. By identifying some of the factors that currently influence colony establishment, we can infer some of the conditions and pressures faced by the termite ancestor that may have led to the origins and maintenance of termite eusociality.
Data Availability Statement
The datasets generated for this study are available on request to the corresponding author.
Author Contributions
EC and RR collected termite colonies in the field, set up incipient colonies in the lab and prepared the manuscript. EC treated queens and performed periodic census and ran statistical analyses. RR financially supported collection trips.
Funding
Funding was provided by the Bill and Ruth Nutting award (2013) awarded by the International Union for the Study of Social Insects, North American Section.
Conflict of Interest
The authors declare that the research was conducted in the absence of any commercial or financial relationships that could be construed as a potential conflict of interest.
Acknowledgments
We thank the administrators of the East Bay Regional Park District in Oakland, California. We thank Drs. Iulian Ilieş and Tarik Gouhier for guidance in statistical analysis. We also appreciate the helpful discussions with Dr. Judith Korb. We thank the many undergraduate researchers and work studies who assisted in data collection under our supervision, especially Sahana Simonetti, Akira Watanabe and Matthew Everett. Finally, we thank the reviewers whose comments and suggestions improved our work.
Supplementary Material
The Supplementary Material for this article can be found online at: https://www.frontiersin.org/articles/10.3389/fevo.2019.00473/full#supplementary-material
References
Abe, T. (1987). “Evolution of life types in termites,” in Evolution and Coadaptation in Biotic Communities, eds S. Kawano, J. H. Connell, and T. Hidaka (Tokyo: University of Tokyo press, 125–148.
Armitage, S. A., Thompson, J. J., Rolff, J., and Siva-Jothy, M. T. (2003). Examining costs of induced and constitutive immune investment in Tenebrio molitor. J. Evol. Biol. 16, 1038–1044. doi: 10.1046/j.1420-9101.2003.00551.x
Bauerfeind, S. S., and Fischer, K. (2005). Effects of adult-derived carbohydrates, amino acids and micronutrients on female reproduction in a fruit-feeding butterfly. J. Insect Physiol. 51, 545–554. doi: 10.1016/j.jinsphys.2005.02.002
Bignell, D. E., Roisin, Y., and Lo, N., (eds.). (2011). Biology of Termites: A Modern Synthesis. Biology of Termites: A Modern Synthesis, 1st Edn. Dordrecht: Springer. doi: 10.1007/978-90-481-3977-4
Boomsma, J. J. (2009). Lifetime monogamy and the evolution of eusociality. Philos. Trans. R. Soc. B Biol. Sci. 364, 3191–3207. doi: 10.1098/rstb.2009.0101
Boomsma, J. J. (2013). Beyond promiscuity: mate-choice commitments in social breeding. Philos. Trans. R. Soc. B Biol. Sci. 368, 1–21. doi: 10.1098/rstb.2012.0050
Boomsma, J. J., and Gawne, R. (2018). Superorganismality and caste differentiation as points of no return: how the major evolutionary transitions were lost in translation. Biol. Rev. 93, 28–54. doi: 10.1111/brv.12330
Bourguignon, T., Lo, N., Cameron, S. L., Šobotník, J., Hayashi, Y., Shigenobu, S., et al. (2014). The evolutionary history of termites as inferred from 66 mitochondrial genomes. Mol. Biol. Evol. 32, 406–421. doi: 10.1093/molbev/msu308
Bourguignon, T., Lo, N., Šobotník, J., Ho, S. Y., Iqbal, N., Coissac, E., et al. (2017). Mitochondrial phylogenomics resolves the global spread of higher termites, ecosystem engineers of the tropics. Mol. Biol. Evol. 34, 589–597. doi: 10.1093/molbev/msw253
Brace, A. J., Lajeunesse, M. J., Ardia, D. R., Hawley, D. M., Adelman, J. S., Buchanan, K. L., et al. (2017). Costs of immune responses are related to host body size and lifespan. J. Exp. Zool. Part A Ecol. Integr. Physiol. 327, 254–261. doi: 10.1002/jez.2084
Brent, C. S., Schal, C., and Vargo, E. L. (2005). Endocrine changes in maturing primary queens of Zootermopsis angusticollis. J. Insect Physiol. 51, 1200–1209. doi: 10.1016/j.jinsphys.2005.06.009
Brent, C. S., Schal, C., and Vargo, E. L. (2007). Endocrine effects of social stimuli on maturing queens of the dampwood termite Zootermopsis angusticollis. Physiol. Entomol. 32, 26–33. doi: 10.1111/j.1365-3032.2006.00536.x
Brent, C. S., and Traniello, J. F. A. (2001). Social influence of larvae on ovarian maturation in primary and secondary reproductives of the dampwood termite Zootermopsis angusticollis. Physiol. Entomol. 26, 78–85. doi: 10.1046/j.1365-3032.2001.00221.x
Calleri, D. V., Rosengaus, R. B., and Traniello, J. F. A. (2006). Disease and colony establishment in the dampwood termite Zootermopsis angusticollis: survival and fitness consequences of infection in primary reproductives. Insectes Soc. 53, 204–211. doi: 10.1007/s00040-005-0859-0
Chouvenc, T. (2019). The relative importance of queen and king initial weights in termite colony foundation success. Insectes Soc. 66, 177–184. doi: 10.1007/s00040-019-00690-3
Chouvenc, T., Basille, M., Li, H. F., and Su, N. Y. (2014). Developmental instability in incipient colonies of social insects. PloS One 9:e113949. doi: 10.1371/journal.pone.0113949
Chouvenc, T., and Su, N. Y. (2017). Irreversible transfer of brood care duties and insights into the burden of caregiving in incipient subterranean termite colonies. Ecol. Entomol. 42, 777–784. doi: 10.1111/een.12443
Chouvenc, T., Su, N. Y., and Grace, J. K. (2011). Fifty years of attempted biological control of termites–analysis of a failure. Biol. Control 59, 69–82. doi: 10.1016/j.biocontrol.2011.06.015
Cole, E. L., Ilieş, I., and Rosengaus, R. B. (2018). Competing physiological demands during incipient colony foundation in a social insect: consequences of pathogenic stress. Front. Ecol. Evol. 6:103. doi: 10.3389/fevo.2018.00103
Cowling, E. B., and Merrill, W. (1966). Nitrogen in wood and its role in wood deterioration. Can. J. Bot. 44, 1539–1554. doi: 10.1139/b66-167
Cremer, S., Pull, C. D., and Fürst, M. A. (2018). Social immunity: emergence and evolution of colony-level disease protection. Annu. Rev. Entomol. 63, 105–123. doi: 10.1146/annurev-ento-020117-043110
Crosland, M. W. J., Ren, S. X., and Traniello, J. F. A. (1998). Division of labour among workers in the termite, Reticulitermes fukienensis, (Isoptera: Rhinotermitidae). Ethology 104, 57–67. doi: 10.1111/j.1439-0310.1998.tb00029.x
Cruse, A. (1998). Termite defenses against microbial pathogens (Ph.D. dissertation). Macquarie University, Sydney, NSW, Australia.
Davies, N. G., and Gardner, A. (2018). Monogamy promotes altruistic sterility in insect societies. R. Soc. Open Sci. 5, 1–18. doi: 10.1098/rsos.172190
Delighne, J., Quennedey, A. C., and Blum, M. C. (1981). “The enemies and defence mechanisms of termites,” in Social Insects, Vol. 2, ed H. R. Hermann (London: Academic Press), 1–76. doi: 10.1016/B978-0-12-342202-6.50008-3
Dial, K. P., and Vaughan, T. A. (1987). Opportunistic predation on alate termites in kenya. Biotropica 19, 185–187. doi: 10.2307/2388744
Fei, H. X., and Henderson, G. (2003). Comparative study of incipient colony development in the formosan subterranean termite, Coptotermes formosanus Shiraki (Isoptera, Rhinotermitidae). Insectes Soc. 50, 226–233. doi: 10.1007/s00040-003-0666-4
Hamilton, C., Lejeune, B. T., and Rosengaus, R. B. (2011). Trophallaxis and prophylaxis : social immunity in the carpenter ant Camponotus pennsylvanicus. Biol. Lett. 7 89–92. doi: 10.1098/rsbl.2010.0466
Hamilton, W. D. (1978). “Evolution and diversity under bark,” in Diversity of Insect Fauna, eds L. A. Mound and N. Waloff (Oxford: Blackwell Publishing, 154–77.
Harrison, M. C., Jongepier, E., Robertson, H. M., Arning, N., Bitard-Feildel, T., Chao, H., et al. (2018). Hemimetabolous genomes reveal molecular basis of termite eusociality. Nat. Ecol. Evol. 2, 557–566. doi: 10.1038/s41559-017-0459-1
Hartke, T. R., and Rosengaus, R. B. (2013). Costs of pleometrosis in a polygamous termite. Proc. R. Soc. B Biol. Sci. 280:20122563. doi: 10.1098/rspb.2012.2563
Higashi, M., Abe, T., and Burns, T. P. (2000). Carbon-nitrogen balance and termite ecology. Proc. R. Soc. B Biol. Sci. 249, 303–308. doi: 10.1098/rspb.1992.0119
Hunt, J. H., and Nalepa, C. A., (eds.). (1994). Nourishment and Evolution in Insect Societies. Boulder, CO: Westview Press, Inc.
Hunt, J. H., and Toth, A. (2017). “Sociality in wasps,” in Comparative Social Evolution, eds D. R. Rubenstein and P. Abbot (Cambridge: Cambridge University Press, 124–153.
Inward, D., Beccaloni, G., and Eggleton, P. (2007). Death of an order: a comprehensive molecular phylogenetic study confirms that termites are eusocial cockroaches. Biol. Lett. 3, 331–335. doi: 10.1098/rsbl.2007.0102
Klass, K. D., Nalepa, C., and Lo, N. (2008). Wood-feeding cockroaches as models for termite evolution (Insecta: Dictyoptera): Cryptocercus vs. Parasphaeria boleiriana. Mol. Phylogenet. Evol. 46, 809–817. doi: 10.1016/j.ympev.2007.11.028
Kleinbaum, D. G., and Klein, M. (2012). “The cox proportional hazards model and its characteristics,” in Survival Analysis: Statistics for Biology and Health (New York, NY: Springer), 97–159. doi: 10.1007/978-1-4419-6646-9_3
Klug, H. (2018). Why monogamy? a review of potential ultimate drivers. Front. Ecol. Evol. 6:30. doi: 10.3389/fevo.2018.00030
Korb, J. (2008a). “Termites: an alternative road to eusociality and the importance of group benefits in social insects,” in Organization of Insect Societies: From Genomes to Sociocomplexity, eds J. Gadau and J. Fewell (Cambridge, MA: Harvard University Press, 126–145.
Korb, J. (2008b). Termites, hemimetabolous diploid white ants? Front. Zool. 5:15. doi: 10.1186/1742-9994-5-15
Korb, J., and Heinze, J. (2008). “The ecology of social life: a synthesis,” in Ecology of Social Evolution, eds J. Korb and J. Heinze (Heidelberg: Springer), 245–260. doi: 10.1007/978-3-540-75957-7
Korb, J., and Heinze, J. (2016). Major hurdles for the evolution of sociality. Annu. Rev. Entomol. 61, 297–316. doi: 10.1146/annurev-ento-010715-023711
Korb, J., and Schneider, K. (2007). Does kin structure explain the occurrence of workers in a lower termite? Evol. Ecol. 21, 817–828. doi: 10.1007/s10682-006-9153-5
Korb, J., and Throne, B. (2017). “Sociality in Termites,” in Comparative Social Evolution, eds D. R. Rubenstein and P. Abbot (Cambridge: Cambridge University Press, 124–153.
Krishna, K., and Wesner, F. M., (eds.). (1970). Biology of Termites, 2nd Edn. New York, NY: Academic Press, 37–72.
Lepage, M. (1991). Predation on the termite Macrotermes michaelseni reproductives and post-settlement survival in the field (Isoptera, Macrotermitinae). Sociobiology 18, 153–166.
Machida, M., Kitade, O., Miura, T., and Matsumoto, T. (2001). Nitrogen recycling through proctodeal trophallaxis in the japanese damp-wood termite Hodotermopsis japonica (Isopterea, Termopsidae). Insectes Soc. 48, 52–56. doi: 10.1007/PL00001745
Maekawa, K., Matsumoto, T., and Nalepa, C. A. (2008). Social biology of the wood-feeding cockroach genus Salganea (Dictyoptera, Blaberidae, Panesthiinae): did ovoviviparity prevent the evolution of eusociality in the lineage? Insectes Soc. 55, 107–114. doi: 10.1007/s00040-008-0997-2
Matsuura, K., and Nishida, T. (2002). Homosexual tandem running as selfish herd in Reticulitermes speratus: novel antipredatory behavior in termites. J. Theor. Biol. 214, 63–70. doi: 10.1006/jtbi.2001.2447
Mehlis, M., Bakker, T. C., and Frommen, J. G. (2009). Nutritional benefits of filial cannibalism in three-spined sticklebacks (Gasterosteus aculeatus). Naturwissenschaften 96, 399–403. doi: 10.1007/s00114-008-0485-6
Miller, J. S., and Zink, A. G. (2012). Parental care trade-offs and the role of filial cannibalism in the maritime earwig, Anisolabis maritima. Anim. Behav. 83, 1387–1394. doi: 10.1016/j.anbehav.2012.03.006
Mirabito, D., and Rosengaus, R. B. (2016). A double-edged sword? The cost of proctodeal trophallaxis in termites. Insectes Soc. 63, 135–142. doi: 10.1007/s00040-015-0448-9
Nalepa, C. A. (1988). Cost of parental care in the woodroach Cryptocercus punctulatus Scudder (Dictyoptera: Cryptocercidae). Behav. Ecol. Sociobiol. 23, 135–140. doi: 10.1007/BF00300348
Nalepa, C. A. (1991). Ancestral transfer of symbionts between cockroaches and termites: an unlikely scenario. Proc. R. Soc. B Biol. Sci. 246, 185–189. doi: 10.1098/rspb.1991.0143
Nalepa, C. A. (2010). Altricial development in subsocial cockroach ancestors: foundation for the evolution of phenotypic plasticity in termites. Evol. Dev. 12, 95–105. doi: 10.1111/j.1525-142X.2009.00394.x
Nalepa, C. A. (2011). Body size and termite evolution. Evol. Biol. 38, 243–257. doi: 10.1007/s11692-011-9121-z
Nalepa, C. A. (2015). Origin of termite eusociality: trophallaxis integrates the social, nutritional, and microbial environments. Ecol. Entomol. 40, 323–335. doi: 10.1111/een.12197
Nalepa, C. A., and Arellano, C. (2016). Parental social environment alters development of nutritionally independent nymphs in Cryptocercus punctulatus (Dictyoptera: Cryptocercidae). Behav. Ecol. Sociobiol. 70, 881–887. doi: 10.1007/s00265-016-2110-6
Nalepa, C. A., and Jones, S. C. (1991). Monogamy in termites. Biol. Rev. 66, 83–97. doi: 10.1111/j.1469-185X.1991.tb01136.x
Nottingham, A. T., Hicks, L. C., Ccahuana, A. J., Salinas, N., Bååth, E., and Meir, P. (2018). Nutrient limitations to bacterial and fungal growth during cellulose decomposition in tropical forest soils. Biol. Fert. Soils 54, 219–228. doi: 10.1007/s00374-017-1247-4
Nutting, W. L. (1969). Flight and colony foundation. Biol. Termites 1, 233–282. doi: 10.1016/B978-0-12-395529-6.50012-X
Oster, G. F., and Wilson, E. O. (1978). Caste and Ecology in the Social Insects. Princeton, NJ: Princeton University Press.
Potrikus, C. J., and Breznak, J. A. (1981). Gut bacteria recycle uric acid nitrogen in termites: a strategy for nutrient conservation. Proc. Natl. Acad. Sci. U.S.A. 78, 4601–4605. doi: 10.1073/pnas.78.7.4601
Rice, W. R. (1989). Analyzing tables of statistical tests. Evolution 43, 223–225. doi: 10.1111/j.1558-5646.1989.tb04220.x
Rosengaus, R. B., Moustakas, J. E., Calleri, D. V., and Traniello, J. F. (2003). Nesting ecology and cuticular microbial loads in dampwood (Zootermopsis angusticollis) and drywood termites (Incisitermes minor, I. Schwarzi, Cryptotermes Cavifrons). J. Insect Sci. 3, 1–6. doi: 10.1673/031.003.3101
Rosengaus, R. B., and Traniello, J. F. (1991). Biparental care in incipient colonies of the dampwood termite Zootermopsis angusticollis Hagen (Isoptera: Termopsidae). J. Insect Behav. 4, 633–647. doi: 10.1007/BF01048075
Rosengaus, R. B., and Traniello, J. F. (1993a). Temporal polyethism in incipient colonies of the primitive termite Zootermopsis angusticollis: a single multiage caste. J. Insect Behav. 6, 237–252. doi: 10.1007/BF01051507
Rosengaus, R. B., and Traniello, J. F. (1993b). Disease risk as a cost of outbreeding in the termite Zootermopsis angusticollis. Proc. Natl. Acad. Sci. U.S.A. 90, 6641–6645. doi: 10.1073/pnas.90.14.6641
Rosengaus, R. B., Traniello, J. F. A., and Bulmer, M. S. (2011). “Ecology, behavior, and evolution of disease resistance in termites,” in Biology of Termites: A Modern Synthesis, eds D. E. Bignell, R. Yves, and N. Lo (New York, NY: Springer), 165–191. doi: 10.1007/978-90-481-3977-4_7
Rosengaus, R. B., Traniello, J. F. A., Lefebvre, M. L., and Carlock, D. M. (2000). The social transmission of disease between adult male and female reproductives of the dampwood termite Zootermopsis angusticollis. Ethol. Ecol. Evol. 12, 419–433. doi: 10.1080/08927014.2000.9522796
Schwenke, R. A., Lazzaro, B. P., and Wolfner, M. F. (2016). Reproduction-immunity trade-offs in insects. Annu. Rev. Entomol. 61, 239–256. doi: 10.1146/annurev-ento-010715-023924
Shellman-Reeve, J. S. (1990). Dynamics of biparental care in the dampwood termite, Zootermopsis nevadensis (Hagen): response to nitrogen availability. Behav. Ecol. Sociobiol. 26, 389–397. doi: 10.1007/BF00170895
Shellman-Reeve, J. S. (1997a). Advantages of biparental care in the wood-dwelling termite, Zootermopsis nevadensis. Anim. Behav. 54, 163–170. doi: 10.1006/anbe.1996.0412
Shellman-Reeve, J. S. (1997b). “The spectrum of eusociality in termites,” in The Evolution of Social Behavior in Insects and Arachnids, eds J. C. Choe and B. J. Crespi (Cambridge: Cambridge University Press), 52–93. doi: 10.1017/CBO9780511721953.005
Shellman-Reeve, J. S. (1999). Courting strategies and conflicts in a monogamous, biparental termite. Proc. R. Soc. B Biol. Sci. 266, 137–144. doi: 10.1098/rspb.1999.0613
Shellman-Reeve, J. S. (2013). Limited nutrients in a dampwood termite : nest preference, competition and cooperative nest defence. J. Anim. Ecol. 63, 921–932. doi: 10.2307/5269
Sheppe, W. (1970). Invertebrate predation on termites of the African savanna. Insectes Soc. 17, 205–218. doi: 10.1007/BF02226194
Sun, Q., Haynes, K. F., and Zhou, X. (2018). Managing the risks and rewards of death in eusocial insects. Philos. Trans. R. Soc. B Biol. Sci. 373, 1–12. doi: 10.1098/rstb.2017.0258
Tai, V., James, E. R., Nalepa, C. A., Scheffrahn, R. H., Perlman, S. J., and Keeling, P. J. (2015). The role of host phylogeny varies in shaping microbial diversity in the hindguts of lower termites. Appl. Environ. Microbiol. 81, 1059–1070. doi: 10.1128/AEM.02945-14
Takata, M., Koyama, S., Satoh, T., and Fugo, H. (2013). Asynchronous hatching and brood reduction by filial cannibalism in the burying beetle Nicrophorus quadripunctatus. J. Ethol. 31, 249–254. doi: 10.1007/s10164-013-0373-1
Thorne, B. L. (1997). Evolution of eusociality in termites. Annu. Rev. Ecol. Syst. 28, 27–54. doi: 10.1146/annurev.ecolsys.28.1.27
Todaka, N., Inoue, T., Saita, K., Ohkuma, M., Nalepa, C. A., Lenz, M., et al. (2010). Phylogenetic analysis of cellulolytic enzyme genes from representative lineages of termites and a related cockroach. PLoS ONE 5:e8636. doi: 10.1371/journal.pone.0008636
Traniello, J. F., Rosengaus, R. B., and Savoie, K. (2002). The development of immunity in a social insect: evidence for the group facilitation of disease resistance. Proc. Natl. Acad. Sci. U.S.A. 99, 6838–6842. doi: 10.1073/pnas.102176599
Tunaz, H., and Stanley, D. (2009). An immunological axis of biocontrol: infections in field-trapped insects. Naturwissenschaften 96, 1115–1119. doi: 10.1007/s00114-009-0572-3
Wcislo, W., and Fewell, J. H. (2017). “Sociality in Bees,” in Comparative Social Evolution, eds D. R. Rubenstein and P. Abbot (Cambridge: Cambridge University Press), 50–83. doi: 10.1017/9781107338319.004
Wilson, E. O. (1971). The Insect Societies. Cambridge: Cambridge Belknap Press of Cambridge University Press; The Belknap Press of Harvard University Press.
Wilson, E. O. (1975). Sociobiology: The New synthesis. Oxford: Belknap Press of Harvard University Press.
Keywords: social evolution, social insect, mate assistance, pathogens, colony foundation, fitness, biparental care, isoptera
Citation: Cole EL and Rosengaus RB (2019) Pathogenic Dynamics During Colony Ontogeny Reinforce Potential Drivers of Termite Eusociality: Mate Assistance and Biparental Care. Front. Ecol. Evol. 7:473. doi: 10.3389/fevo.2019.00473
Received: 06 August 2019; Accepted: 21 November 2019;
Published: 05 December 2019.
Edited by:
Joël Meunier, UMR7261 Institut de Recherche sur la Biologie de l'insecte (IRBI), FranceReviewed by:
Michael Poulsen, University of Copenhagen, DenmarkMaximilian Körner, University of Bayreuth, Germany
Copyright © 2019 Cole and Rosengaus. This is an open-access article distributed under the terms of the Creative Commons Attribution License (CC BY). The use, distribution or reproduction in other forums is permitted, provided the original author(s) and the copyright owner(s) are credited and that the original publication in this journal is cited, in accordance with accepted academic practice. No use, distribution or reproduction is permitted which does not comply with these terms.
*Correspondence: Rebeca B. Rosengaus, ci5yb3NlbmdhdXNAbm9ydGhlYXN0ZXJuLmVkdQ==