- Department of Biology, Advanced Facility for Avian Research, University of Western Ontario, London, ON, Canada
Many birds undertake long migrations when they are only a few months of age. Although they are typically of adult body size, their performance, and survival are often poor compared to adults. This differential performance could be due to lack of experience, selection against poor-performing cohort members, or inherent constraints of continuing physiological and morphological maturation of juveniles. Limited evidence suggests that digestive and muscle physiology of juveniles during their first migration may differ from that of adults. We compared body composition, metabolic rate, and digestive physiology between juvenile and adult passerines during fall migration. First, we measured fat and lean masses by quantitative magnetic resonance, and organ and muscle masses of salvaged carcasses of fall migrants from four passerine species. In general, juveniles had more lean mass and heavier digestive organs (especially liver) than adults in hermit thrushes (Catharus guttatus), Swainson's thrushes (Catharus ustulatus), ovenbirds (Seiurus aurocapilla), and white-throated sparrows (Zonotrichia albicollis). Principal components analysis of all organs and muscles revealed that juveniles for three of four species had overall larger digestive components and smaller flight muscles than adults. We then used open-flow respirometry to measure basal metabolic rates (BMRs) of juvenile and adult Swainson's thrushes and white-throated sparrows captured in fall at a migratory stopover site. Controlling for a significant effect of body mass, juveniles had 6% higher BMRs than adults in both species. We then conducted total collection mass balance feeding trials with fall migratory Swainson's thrushes and white-throated sparrows. Juvenile thrushes had greater metabolizable energy intake than adults, which was achieved through higher food intake rather than greater utilization efficiency. Age classes of white-throated sparrows did not differ in these measures of digestive performance, although juveniles had greater food intake capacity at low lean body masses. We propose that age-related differences in foraging ecology, diet composition, and energy requirements may be responsible for larger digestive organs of juvenile migrants. Larger guts may allow juveniles to consume more food or a more dilute diet, but may contribute to higher BMRs.
Introduction
Piersma and van Gils (2011) described the morphology and physiology of animal bodies as expressions of their ecologies. Animal bodies are not static; changes during development transform phenotype and performance capacity, and some animals maintain an ability to reversibly alter phenotype even when fully mature to cope with changes in workload associated with environmental variation or life history stages (Secor and Diamond, 1995; Piersma and Lindström, 1997; Piersma and Drent, 2003). During migration, animals such as birds face greatly elevated physiological demands and may experience a variety of environmental conditions as they move between breeding and wintering areas. Migratory birds express a migratory phenotype, which includes hyperphagia, fattening, changes in muscle and organ sizes, increased liver fatty acid synthesis, and increased muscle aerobic and fatty acid oxidation capacity (Guglielmo, 2018). Individuals that do not achieve an appropriate migratory phenotype may be at risk of failure or mortality because they lack sufficient functional capacity, and in particular, this, along with potential behavioral deficits in navigation or foraging, could explain the poor migratory ability of juvenile birds.
Migrating between breeding and distant wintering areas is both energetically expensive (Wikelski et al., 2003) and dangerous (Sillett and Holmes, 2002) for birds. For most migratory passerines, migration consists of multiple flights interspersed with stopover periods where birds rest and refuel to prepare for subsequent migratory flights. Migrant birds spend more time (Dolnik and Blyumental, 1967; Fransson, 1986) and energy (Wikelski et al., 2003), at these stopover locations than during actual flight. Thus, the amount of time and energy spent at stopover sites can limit overall migration speed (Alerstam and Lindström, 1990).
Altricial migratory songbirds develop quickly from a helpless nestling with functionally immature muscles and poor motor and thermoregulatory abilities (Starck and Ricklefs, 1998) to a migratory phenotype capable of traveling thousands of kilometers. During their first migration, juvenile birds are often the same physical size and mass as adults, although they can sometimes be distinguished by plumage markings, amount of feather wear, or evidence of incompletely pneumatized skulls (Pyle, 1997). Juveniles generally tend to underperform adults during migration. For example, juveniles migrate slower (Ellegren, 1990; Fransson, 1995; Susanna et al., 2008), and may spend more time at stopovers (Veiga, 1986; Ellegren, 1991; Morris et al., 1996; Yong et al., 1998; Rguibi-Idrissi et al., 2003; Mills et al., 2011) than adults. These observations suggest that juveniles refuel differently from adults during fall migration. Indeed, for some species adults often arrive at stopover locations in better body condition, while juveniles may arrive lighter and with less fat (Woodrey, 2000; Jones et al., 2002), but these differences are not always observed (Kennedy, 2012). Before leaving breeding areas on fall migration, juveniles forage less efficiently than adults (Weathers and Sullivan, 1991; Heise and Moore, 2003; Vanderhoff and Eason, 2007, 2008), but may improve prior to departure (Heise and Moore, 2003; Wheelwright and Templeton, 2003). If juveniles remain less skilled foragers during migration, they may be able to compensate by increasing foraging time to achieve similar overall energy intake to adults. Several studies found refueling differences between juvenile and adult passerines during stopover on fall migration (Veiga, 1986; Morris et al., 1996; Woodrey and Moore, 1997; Yong et al., 1998; Jones et al., 2002; Leist, 2007; Arizaga et al., 2008; but for different findings see Heise and Moore, 2003; Seewagen et al., 2013).
Further evidence suggests juveniles are physiologically different from adults during fall migration. For example, juvenile western sandpipers (Calidris mauri) had lower concentrations of heart-type fatty acid binding protein in flight muscle and lower activities of several digestive enzymes than adults (Guglielmo et al., 2002; Stein et al., 2005). Activities of several aerobic and glycolytic enzymes in pectoralis muscle are much lower in juvenile than in adult barnacle geese (Branta leucopsis), but rapidly increase prior to fall migration departure (Bishop et al., 1995). Juvenile migrants had heavier livers and digestive tracts than adults in several studies (Graber and Graber, 1962; Hume and Biebach, 1996; Guglielmo and Williams, 2003; Stein et al., 2005). Non-migratory house sparrows (Passer domesticus) of similar age to migrant juveniles also had larger digestive tracts and livers than adults (Chappell et al., 1999).
Generally, larger organ size indicates a greater functional capability for that organ (Hammond and Diamond, 1992; McWilliams et al., 1999; Secor and Diamond, 2000). Larger alimentary tracts and livers of juveniles may indicate greater capacity for digestion of food, absorption of nutrients, and post-absorptive processing of nutrients (Klasing, 1998). Additionally, larger alimentary tracts could increase storage capacity for ingested food, and thereby facilitate higher levels of food intake (Dykstra and Karasov, 1992; Hammond and Diamond, 1992; McWilliams et al., 1999; Starck, 1999; Starck and Rahmaan, 2003). Furthermore, bigger intestines might provide greater surface area and more capability to absorb nutrients from ingested food (Klasing, 1998). If digesta moves more rapidly through larger and presumably longer digestive tracts, then digestive efficiency could remain equivalent to adult-sized digestive tracts, with comparable or shorter digesta retention times, while enabling higher rates of food intake. However, if digesta flow rates are similar through larger and longer digestive tracts, overall time contents are retained within the gut should be longer as well, which should enhance digestive efficiency, but not permit notably increased food intake (Penry and Jumars, 1986; Martinez del Rio and Karasov, 1990; Karasov and Martinez del Rio, 2007).
Little consideration has been given to the possibility that differential energy expenditure contributes to differences between age classes in stopover duration. In non-migratory house sparrows (Passer domesticus), Chappell et al. (1999) found that 4-month-old juveniles had higher basal metabolic rates (BMR) than adults. Juvenile non-migratory yellow-eyed juncos (Junco phaeonotus) had higher daily energy expenditures, but not higher BMR than breeding adults (Weathers and Sullivan, 1989). In several shorebird species, Lindström (1997) found juveniles had higher BMR during migration compared to after migration. If juveniles migrating in fall have physiological attributes that increase BMR, such as expensive digestive organs (Martin and Fuhrman, 1955; Piersma et al., 1999), then juveniles may be at an energy disadvantage compared to adults at stopovers. Juveniles might require more foraging time to consume enough food to meet elevated maintenance costs, rebuild tissues and accumulate sufficient energy stores to resume migration. To our knowledge, no one has yet tested for BMR differences between age classes of migrant songbirds at stopover.
Our objective was to investigate whether adult and juvenile birds differ in body composition (particularly digestive system size), BMR, and digestive performance during fall migration. We conducted three related studies. First we obtained carcasses of birds killed accidentally from collisions during fall migration through the city of Toronto, Ontario, Canada. We measured body composition of juveniles and adults of four passerine migrants (white-throated sparrow, Zonotrichia albicollis; hermit thrush, Catharus guttatus; Swainson's thrush, Catharus ustulatus; and ovenbird, Seiurus aurocapilla) to test the hypothesis that juvenile birds generally have enlarged digestive systems. All species differed in at least one aspect of primary diet (i.e., frugivorous, insectivorous, or granivorous) or migration distance (i.e., short or long; Van Horn and Donovan, 1994; Jones and Donovan, 1996; Mack and Yong, 2000; Falls and Kopachena, 2010). Second, we measured BMRs of adult and juvenile white-throated sparrows and Swainson's thrushes captured during fall migration at a stopover site. We hypothesized that due to continued physiological maturation and potentially larger digestive systems, that juveniles would have greater BMRs than adults. Third, we conducted total collection mass balance feeding trials to measure food intake, diet utilization efficiencies, and total assimilated energy of juvenile and adult Swainson's thrushes and white-throated sparrows captured and kept in short-term captivity at the same stopover site. Measuring these parameters of digestive physiology allowed us to determine whether and how juvenile migratory birds benefit from larger digestive organs. We hypothesized that juveniles would assimilate more total energy and have either higher food intake or digestive efficiency than adults, but not both.
Methods
All animal procedures complied with guidelines of the Canadian Council on Animal Care and were approved by the University of Western Ontario Animal Use Sub-committee (Protocol # 2010-020). Live birds were captured under a scientific collection permit from the Canadian Wildlife Service (CA-0255) and carcasses were obtained under a Canadian Wildlife Service salvage permit (SA 0208).
Body Composition Analysis of Window Strike Carcasses
Carcasses of hermit thrushes (Catharus guttatus), Swainson's thrushes (Catharus ustulatus), ovenbirds (Seiurus aurocapilla), and white-throated sparrows (Zonotrichia albicollis) were collected by volunteer participants of the Fatal Light Awareness Program (FLAP; www.flap.org) who routinely search sidewalks of downtown Toronto during spring (mid-March to early June) and fall (early August to mid-November) migration seasons for birds injured or killed by collisions with buildings or other structures. Volunteers conduct searches during mornings and record species, location, and collection date of each bird. Bird carcasses for our study were salvaged between 24 August and 11 November during four fall migration seasons (2008–2011), with 95% found during September and October of each year. During our study 88 to 94% of the birds were salvaged during mornings, which followed the nocturnal flights or morning feeding when birds likely perished, so carcasses were in a good condition for analysis. Carcasses were stored in freezers (−20°C) at the Royal Ontario Museum, Toronto, Ontario, Canada until they were transported on ice to the Advanced Facility for Avian Research, London, Ontario, Canada and stored in a freezer (−30°C) until dissection.
We thawed carcasses overnight in a refrigerator, brought them to room temperature, and measured fat and wet lean mass using a quantitative magnetic resonance (QMR) body composition analyzer (Echo-MRI-B, Echo Medical Systems, Houston, TX, USA; Guglielmo et al., 2011). QMR of thawed carcasses was previously validated against chemical analysis using bats (McGuire and Guglielmo, 2010), which showed relative accuracies (±3% for wet lean mass and ±11.6% for fat mass) very close to those for live birds (Guglielmo et al., 2011). Seventeen birds prepared for dissection showed obvious signs of decomposition and were discarded. We determined age class by the degree of skull pneumatization (Miller, 1946; Pyle, 1997). Since the sample of carcasses consisted of many more juveniles than adults, in later years we first removed and thawed heads of carcasses for careful examination of the skull so we could preferentially identify adults for dissection and prevent the sample from becoming excessively skewed toward juveniles. We measured unflattened wing length to the nearest mm using a wing ruler and measured tarsus, keel length, and bill (nares to tip) to the nearest 0.01 mm using digital calipers (Pyle, 1997). We dissected and placed proventriculus, gizzard, small intestine, large intestine, pancreas, liver, kidneys, heart, and flight muscle (pectoralis and supracoracoideus) into pre-weighed aluminum dishes. Organ fat was trimmed and placed with the remaining carcass. Digestive tract organs (proventriculus, gizzard, small intestine, and large intestine) were washed in 0.9% NaCl, pressed on paper towel to remove digesta, and re-washed in saline before being placed on weighing tins. We could not reliably locate gonads in their immature or regressed conditions, so therefore we did not determine sex. Remaining carcass components (including excess fat and feathers) were also placed in a weighing tin. We dried samples at 60°C and weighed to the nearest 0.001 g on a digital balance (Sartorius CP 423S) until mass was constant for 2-days. Total gastrointestinal tract dry mass was calculated by summing dry masses of proventriculus, gizzard, small intestine, and large intestine. Total dry mass was calculated by summing dry masses of all body components (carcass, organs, and muscle).
Basal Metabolic Rates
Swainson's thrushes and white-throated sparrows were captured using mist nets at Long Point Bird Observatory (LPBO), Long Point, Ontario, Canada (latitude: 42° 34' 58” N; longitude: 80° 23' 52” W), between 11 September and 31 October, 2010. Age class was assigned according to plumage and feather molt characteristics and degree of skull pneumatization (Pyle, 1997). Each day, up to four Swainson's thrushes or white-throated sparrows in good condition (signs of visible fat stores and no injuries or signs of molt) were individually held in cages (66 cm × 46 cm × 50 cm) within animal quarters of a specially equipped mobile laboratory. Tarsus length and unflattened wing length were measured as described above. The animal room was temperature controlled (mean temperature = 19.2 ± 0.3°C) and the birds were exposed to a natural light cycle for the day they were captured. Birds had ad libitum access to food (live mealworms and millet seed for sparrows and live mealworms for thrushes) and water until we removed food 2 h before sunset.
At approximately sunset on the day of capture, up to four fasted birds were weighed to the nearest 0.001 g on an electronic balance (Acculab Vicon-123), and placed into air and light-tight stainless steel canisters with opaque plastic lids (12 cm diameter × 13 cm height, 1.5 L). All canisters were equipped with a perch and attached to an open flow respirometry system. Within a temperature cabinet (PTC-1, Sable Systems), canister temperatures were maintained at 30°C by a Peltier effect device controller (Pelt 5, Sable Systems), which is within the thermoneutral zone of both species (Yarbrough, 1971; Holmes and Sawyer, 1975). While a colder temperature would more closely approximate natural conditions experienced by birds during fall migration, 30°C was sufficiently above lower critical temperature to avoid potential confounding effects due to age differences in insulation. Furthermore, 30°C allowed both species to be measured simultaneously. Drierite™ (W.A. Hammond Drierite Company, Zenia, USA) removed water from outside air before it was pumped through the chambers at flow rates between 380 and 420 mL min−1, which was measured continuously upstream of each chamber by a mass flow meter (Flowbar-8, Sable Systems). Over the entire night (11–13 h), dried excurrent and baseline air were sub-sampled at 10 min intervals using a multiplexer which led to an infrared CO2 analyzer (CA-2A, Sable Systems) and a fuel cell O2 analyzer (FC-1B, Sable Systems), to measure carbon dioxide and oxygen, respectively. Between the CO2 and O2 analyzers, Drierite™ and soda lime (EMD chemicals, Cincinnati, USA) scrubbed water and carbon dioxide, respectively, from excurrent air. Both gas analyzers were calibrated with a certified span gas (20.94% O2, 1.000% CO2, balance N2; Praxair, London, ON, Canada) at the beginning of the field season. Analyzers were checked daily for gas concentration readings of dried atmospheric air (dried CO2-free air for O2 analyzer). Variation of CO2 analyzer readings of dried atmospheric air was < ±0.003% throughout the field season. The O2 analyzer was re-spanned using dried CO2-free atmospheric air as a reference when readings of dried atmospheric air differed by more than 0.02% from expected. Flow rates, and CO2 and O2 concentrations were recorded with Expedata software (Version 1.1.15, Sable Systems). Before releasing birds in the morning, we sampled 70–140 μL of blood by puncturing the brachial vein of the wing with a 26-gauge needle and collecting blood in heparinized microhematocrit tubes (Fisher Scientific, Pittsburgh, USA). Whole blood was stored 1.5 mL microcentrifuge tubes in a freezer (−20°C) for molecular sexing.
To calculate metabolic rate, we used LabAnalyst software (Warthog Systems) to select the lowest 3–5 min of carbon dioxide production with coefficients of variation less than two percent. We excluded the first 2 min of each sampling interval to account for transition from the previous channel. To ensure birds were post-absorptive, we only included sampling intervals recorded more than 3 h after sunset, and therefore when birds had fasted for at least 5 h. Using immediately preceding baseline intervals and lag-corrected fractional O2 and CO2 concentrations, we calculated V°O2, V°CO2 using equations 10.1 and 10.7 in Lighton (2008). Following the recommendation of Lighton (2008), we calculated basal metabolic rates (BMR) by multiplying V°O2 by an oxyjoule equivalent [16 + 5.164 (RQ)].
Following McCabe (2006), we amplified CHD-W and CHD-Z genes directly from whole blood (Bercovich et al., 1999) to identify sex (Griffiths et al., 1998). Briefly, final polymerase chain reaction (PCR) volumes were 25 μL and consisted of 5 μL of a 2% whole blood suspension (Tomasulo et al., 2002), 10 mM Tris-HCl (pH 8.3), 50 mM KCl, 2.5 mM MgCl2, 200 μM of each dNTP, and 1 μM of P2 and P8 primers described by Griffiths et al. (1998), and 1 U Taq™ DNA polymerase (Takara, Inc.). Using an Eppendorf Mastercycler thermocylcer, PCR conditions were as follows: (a) three cycles of 94°C for 3 min then 48°C for 3 min, (b) 94°C for 4 min, (c) addition of 1 U Taq, (d) 35 cycles of 94°C for 45 s, 48°C for 45 s, then 72°C for 45 s, (e) 72°C for 5 min. We separated PCR products on 3% agarose gels stained with ethidium bromide (0.5 μg/mL) for 60–90 min at 8.5 V/cm, and then observed resulting bands under UV light. One band indicated males, whereas two bands indicated females.
Digestive Performance
We obtained migrating adult and juvenile Swainson's thrushes and white-throated sparrows at the Old Cut station of the LPBO between 12 September and 28 October, 2011. We used individuals that had been assigned fat scores by LPBO personnel of 1 or higher on a 0 to 7 scale (Dunn, 2003), and that weighed at least 27 g for thrushes and 22 g for sparrows. Birds were weighed to the nearest 0.001 g on a digital balance (Acculab Vicon-123) and body fat and wet lean mass was measured by QMR. We placed birds in individual cages (66 cm × 46 cm × 50 cm) within an animal room in the research trailer at the field site. Temperature was controlled (20°C) and the birds were exposed to a natural light cycle that tracked local sunrise and sunset throughout the season.
We provided birds with ad libitum water and a species-specific diet. Food and water cups were elevated to reduce spillage and mixing with excreta during mass balance feeding trials (below). Swainson's thrushes ate a frugivore-based synthetic banana-mash style diet cut into ~5 mm3 cubes. This diet was modified from Denslow et al. (1987) and each batch consisted of: one L water, 680 g fully ripe bananas, 37 g wheat germ (Kretschmer, Quaker Oats Co.), 25 g casein (high nitrogen, ultrapure, 12845, Affymetrix, Inc.), 22 g agar (ultrapure, bacteriological, type A, 10906, Affymetrix, Inc.), 7 g vegetable oil (soybean oil, Crisco, The J.M. Smucker Co.), 3.5 g Briggs salt mixture (902834, MP biomedicals, LLC.), and 1.5 g vitamin mix (AIN vitamin mixture 76, 905454, MP biomedicals, LLC.). To encourage Swainson's thrushes to eat the diet, we used a sufficient quantity of red and blue food color (McCormick, Canada) to visually change the diet to a deep-purple color (Boyle, 2009). To further entice Swainson's thrushes to sample the synthetic diet, we provided several thawed, frozen-blueberries on top of the synthetic diet for the first day birds stayed in the animal room. We fed white-throated sparrows hulled sunflower seeds (200805, Wild Birds Unlimited, Inc.) in order to provide a seed diet that would not produce large quantities of husks.
Birds ate their respective diets for 2-days prior to the total collection mass balance feeding trials to allow birds time to recognize and consume the diet and to pass remnants of food consumed prior to capture. To keep birds as close to their wild condition as possible, we minimized time in captivity prior to feeding trials to limit changes in digestive tract morphology related to captivity and captive diet (Miller, 1975; Levey and Karasov, 1989; Moore and Battley, 2006). Provided that birds ate at least some captive diet, minimal changes in digestive tract morphology likely occurred, given that Pierce and McWilliams (2004) reported similar masses of digestive components among white-throated sparrows fed either ad libitum or restricted diets. We released birds that did not eat the diet or fell below a critical mass (Swainson's thrush < 25 g, 21 released of 41 captured; white-throated sparrow < 21 g, 13 released of 33 captured).
Cages for 2-day total collection mass balance feeding trials were the same as those used to house birds upon arrival at the animal housing room. The smooth-walled collection cages included a smooth half-wall frontal barrier, and a galvanized steel mesh (1.9 cm2) floor, raised (1.9 cm) above a dropping pan lined with a clear plastic sheet. The plastic lining facilitated collections of excreta and uneaten food, which were separated and collected daily, and then frozen (−20°C) for later analysis. Collections of the diet, excreta, and uneaten food were later dried in a convection oven at 70°C to constant mass (0.001 g, Sartorius CP 4235). Afterward, dried samples were crushed into powder using a mortar and pestle.
We measured energy content of excreta and food using a Philipson microbomb calorimeter (Gentry Instruments) with benzoic acid standards. Total nitrogen content of excreta and food sub-samples was measured using flow injection analysis at the University of Wisconsin-Madison Soil and Plant Analysis Lab (Verona, WI, USA). Samples were run in duplicate for both analyses with coefficients of variation <4% for energy content and <5% for total nitrogen content.
We calculated the apparent assimilable mass coefficient (AMC*) and apparent metabolizable energy coefficient (MEC*), as follows:
where Qi and Qe are quantities of dry food intake and excreta production, respectively, and GEi and GEe are gross energy contents of dry food and excreta, respectively (Karasov, 1990; Guglielmo and Karasov, 1993). We also corrected these apparent utilization efficiencies for nitrogen balance using the following equations:
where Ni and Ne are proportion nitrogen content of dry food and excreta, respectively (Guglielmo et al., 1996). Dry nitrogen intake for the second day of the feeding trial was calculated from:
Energy deposition during day 2 of the feeding trial was calculated as the sum of Δ QMR wet lean mass × energy density of protein and Δ QMR fat mass x energy density of fat, where the energy densities of protein and fat are 5.3 kJ g−1 wet mass and 39.6 kJ g−1 dry matter, respectively (Jenni and Jenni-Eiermann, 1999). We calculated metabolizable energy intake (MEI) following Guglielmo et al. (1996) as:
Statistical Analysis
Body Composition of Window Strike Carcasses
For each species we used the first principal component of a PCA analysis of tarsus, keel and bill measurements as a size metric (SizePC1). Eigenvalues for SizePC1 always exceeded 1.0. We excluded wing length since adults of many passerine and near-passerine species may have longer wing lengths than juveniles (Alatalo et al., 1984; Francis and Wood, 1989; Pyle, 1997). We found low loadings (<0.10) for bill length in PC1 for hermit thrush, Swainson's thrush, and ovenbird. Therefore, we recalculated SizePC1 for these three species with only tarsus and keel.
Separately for each species, we tested for age class differences in morphometric length measurements (wing, tarsus, keel, and bill) and SizePC1 using Student's t-tests. We then performed analyses of covariance (ANCOVA) on each body composition component (QMR fat and lean masses, dry organ and muscle masses, gastrointestinal tract dry mass and total dry mass) using age class as the factor and SizePC1 as a covariate. We excluded pancreas from analysis as it was usually in a deteriorated state. Other studies suggest that the pancreas quickly deteriorates after death (Shimizu et al., 1990). Using PCA, we condensed organ and muscle dry mass variables into fewer principal components (OrganPC's) so that we could examine overall relationships among body components and test in a more general way the patterns revealed by univariate analyses. We selected the first two OrganPC axes as dependent variables and tested for differences between age classes while controlling for SizePC1 using multivariate analysis of covariance (MANCOVA).
All mean and least squares mean (LSM) values given are ± SE unless otherwise noted. We performed analyses using IBM SPSS statistics version 20.0.0. We used one-tailed tests where we had reason a priori to predict differences between age classes (i.e., larger juvenile dry masses of proventriculus, gizzard, small intestine, large intestine, liver, and total gastrointestinal tract, as well as larger adult wing lengths). Tests of all other differences, including those involving OrganPC's, used two-tailed tests with α = 0.05. All P-values are unadjusted for multiple comparisons, because we expected associations among some body components, such as among organs of the digestive tract. In doing so, we accepted a higher probability of type I error (false positives) in order to avoid increasing the likelihood of type II error (false negatives).
Basal Metabolic Rate
For each species we used ANCOVA to test for the effects of age class and sex on body mass, while including tarsus length as a covariate. We used ANCOVA to test for effects of age class and sex on BMR, while including body mass and minimum daily temperature of the testing day (from Environment Canada's National Climate Data and Information Archive for Long Point, Ontario station; http://climate.weatheroffice.gc.ca/climateData/canada_e.html) as covariates. Minimum daily temperature was used as a covariate because variation in previous exposure to temperature may influence BMR (Williams and Tieleman, 2000). Additionally, we used ANCOVA to test for effects of age class on overnight mass loss, while controlling for initial mass and total time spent in respirometry chambers. We tested all two-way interactions and used backward selection to remove non-significant (P > 0.05) terms from analysis. We visually confirmed ANCOVA assumptions for normality and homogeneity of variance/covariance. Body masses and basal metabolic rates were log10 transformed to account for allometric scaling for all statistical tests associated with BMR. However, untransformed BMR values were used to calculate least-squares means. Unless otherwise stated, values reported are mean ± SE. A Grubb's test was used to check for outliers (Dunn and Clark, 1987). Differences were considered significant at α = 0.05 for two-tailed tests. PASW Statistics (v. 18.0.0) was used to perform statistical tests.
Digestive Performance
To ensure that initial body composition of birds retained for feeding trials was not a bias for inclusion, we used ANOVA's to separately test species for differences in body mass, QMR wet lean mass, and QMR fat mass, between birds that were selected for and excluded from feeding trials, while including age class as a factor. Among birds that participated in feeding trials, we tested for differences in arrival date, body mass, QMR wet lean mass, and QMR fat mass at capture between adults and juveniles using t-tests. We tested for age class differences in measurements generated from feeding trials (mass at start of trials, nitrogen balance, dry mass food intake, dry mass excretion, dry mass utilization, energy intake, energy excretion, MEI, 2-day mass change, AMC*, MEC*, , and ) using t-tests. We used ANCOVA to test for age class differences in dry food intake while controlling for QMR wet lean mass, 2-day mass change while controlling for dry mass intake, energy deposition while controlling for MEI, and separately for QMR wet lean mass deposition while controlling for dry nitrogen intake and nitrogen balance. We performed all statistical tests using IBM SPSS (version 20.0.00) and considered differences significant when P < 0.05. Since we expected associations among measures of digestive function, all P-values were unadjusted for multiple comparisons.
Results
Body Composition Analysis
We dissected carcasses of 71 white-throated sparrows, 25 hermit thrushes, 37 Swainson's thrushes, and 44 ovenbirds. There were no age class related differences in wing, tarsus, keel, or bill length for any species (Table S1). Tarsus, keel, and bill generally produced a single principal component axis representing body size (Table S2) and SizePC1 did not differ between adults and juveniles of any species (Table S1).
We controlled for body size using SizePC1, regardless of whether the covariate contributed significantly to the ANCOVA. While SizePC1 typically was not a significant covariate when testing for differences in body composition, QMR wet lean mass [F(1, 68) = 13.92, P < 0.001], total dry mass [F(1, 68) = 8.83, P = 0.004], and dry heart mass [F(1, 68) = 7.93, P = 0.006] of white-throated sparrows, and dry flight muscle of white-throated sparrows [F(1, 68) = 37.26, P < 0.001] and Swainson's thrushes [F(1, 34) = 24.21, P < 0.001] increased with SizePC1.
Controlling for SizePC1, there were no differences in total dry mass between adults and juveniles for any species (Figure 1A). However, juveniles had 8–9% greater size-corrected wet lean mass measured by QMR than adults in white-throated sparrows [F(1, 68) = 13.18, P = 0.001] and hermit thrushes [F(1, 22) = 10.64, P = 0.004], and there was a tendency for juveniles to have higher wet lean mass than adults among Swainson's thrushes [F(1, 34) = 3.45, P = 0.07, Figure 1B]. No significant age differences in QMR fat mass were detected (Figure 1C).
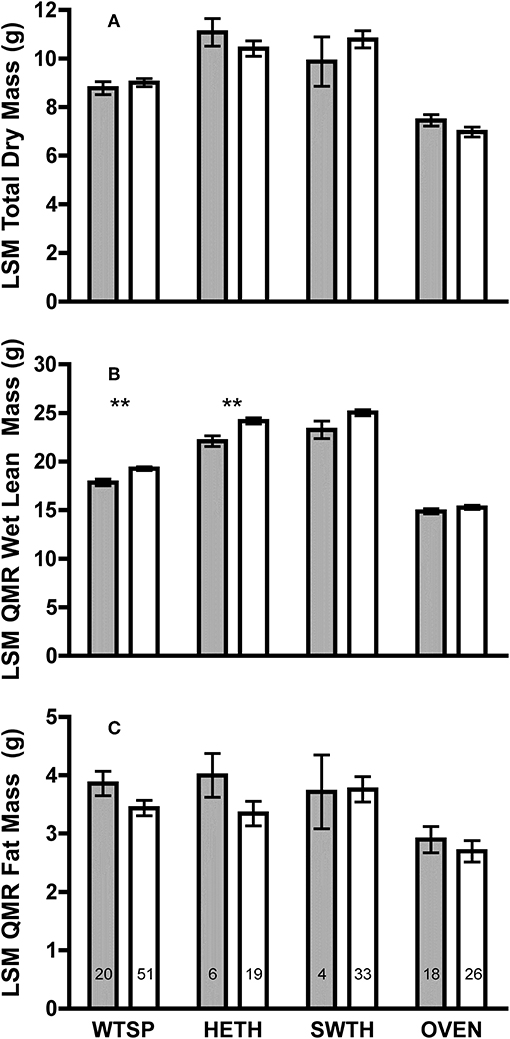
Figure 1. Least square means (±SE) (A) total dry mass; (B) QMR wet lean; and (C) QMR fat masses from ANCOVA using SizePC1 as a covariate of adult (gray bars) and juvenile (white bars) white-throated sparrow (WTSP), hermit thrush (HETH), Swainson's thrush (SWTH), and ovenbird (OVEN). SizePC1 is a principal component containing tarsus and keel measurements for hermit thrush, Swainson's thrush, and ovenbird, and tarsus, keel, and bill measurements for white-throated sparrow. Numbers within bars indicate sample sizes for each group. Asterisks denote differences between juveniles and adults at P < 0.01 (**).
Juveniles had larger size-corrected livers (4 species), proventriculi (3 species), gizzards (3 species), small intestines (2 species), and large intestines (1 species) than adults (Figure 2, Table 1). Additionally, size-corrected hearts were larger in juveniles from two species. Total gastrointestinal tract dry masses (size-corrected) of juveniles were heavier than those of adults among three of four species examined (Table 2).
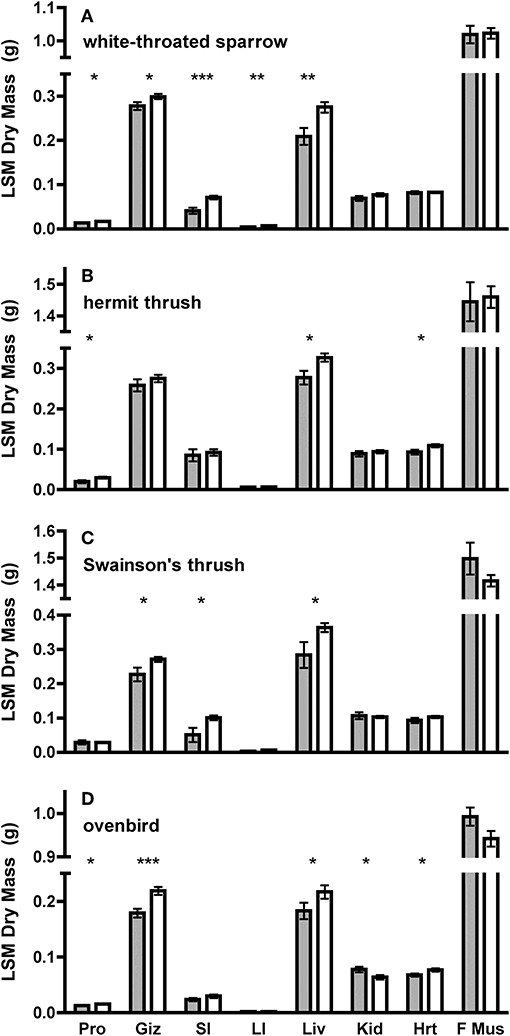
Figure 2. Least square means (±SE) dry masses of organs from ANCOVA using SizePC1 as a covariate of adult and juvenile (A) white-throated sparrow, (B) hermit thrush, (C) Swainson's thrush, and (D) ovenbird. SizePC1 is a principal component containing tarsus and keel measurements for (B) hermit thrush, (C) Swainson's thrush, and (D) ovenbird, and tarsus, keel, and bill measurements for (A) white-throated sparrow. Gray bars represent adults and white bars represent juveniles. Organs are: Pro, proventriculus; Giz, gizzard; SI, small intestine; LI, large intestine; Liv liver; Kid, kidney; Hrt, heart; and F Mus, flight muscle (pectoralis and supracoracoideus). Asterisks denote differences between juveniles and adults at P < 0.05 (*), P < 0.01 (**), or P < 0.01 (***). Comparisons of Pro, Giz, SI, LI, and Liv used one-tailed tests predicting larger dry masses among juveniles, whereas comparisons of Kid, Hrt, and F Mus used two-tailed tests.
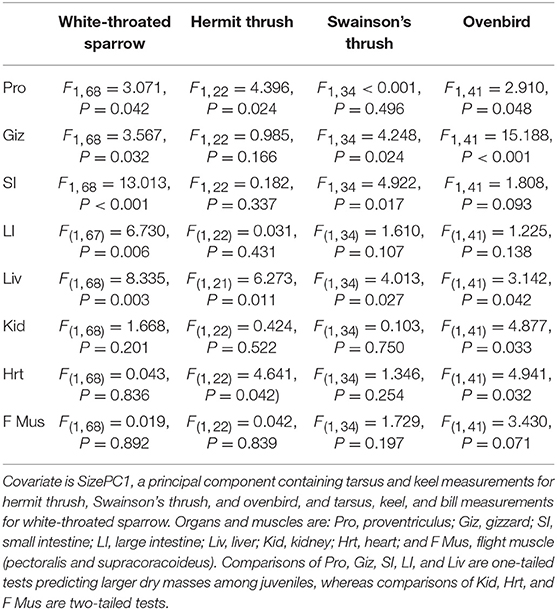
Table 1. Results of ANCOVA comparing dry organ masses of adult and juvenile migrants from four species.
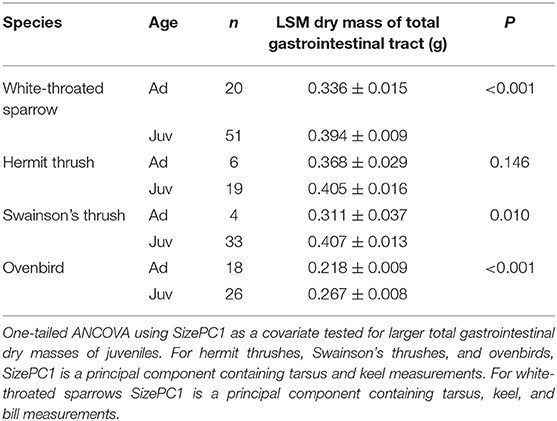
Table 2. Least square means (±SE) dry masses of total gastrointestinal tracts from adults (Ad) and juveniles (Juv) of four migrant bird species collected during fall migration (2008–2011).
The first two principal components generated by PCA explained 48% or more of the variation in organ dry masses for each species (Table S3). In general, OrganPC1 was characterized by positive loadings of nearly all organs. Positive OrganPC2 loadings characterized larger masses of hearts and flight muscles and smaller digestive organs, except for ovenbirds where OrganPC2 represented heavier hearts and lighter flight muscles. Controlling for SizePC1, age classes differed for the combination of OrganPC1 and OrganPC2 in white-throated sparrows [Wilks' λ = 0.83, partial ε2 = 0.17; F(2, 66) = 6.95, P = 0.002], Swainson's thrushes [Wilks' λ = 0.82, partial ε2 = 0.18; F(2, 33) = 3.67, P = 0.04], and ovenbirds [Wilks' λ = 0.73, partial ε2 = 0.27; F(2, 40) = 7.48, P = 0.002], but not in hermit thrushes [Wilks' λ = 0.82, partial ε2 = 0.19; F(2, 20) = 2.28, P = 0.13; Figure 3]. Compared with adults, OrganPC1 scores of juvenile white-throated sparrows [F(1, 67) = 10.50, P = 0.002], Swainson's thrushes [F(1, 34) = 7.55, P = 0.01], and ovenbirds [F(1, 41) = 8.94, P = 0.005] were higher. OrganPC2 scores of juvenile white-throated sparrows approached being significantly lower than adults [F(1, 67) = 3.84, P = 0.054], and OrganPC2 scores of juvenile ovenbirds were very close to being significantly higher than adults [F(1, 41) = 3.954, P = 0.053]. The directions of principal component loadings imply that overall, juvenile white-throated sparrows, Swainson's thrushes, and ovenbirds had heavier digestive organs and hearts, but lighter flight muscles than adults.
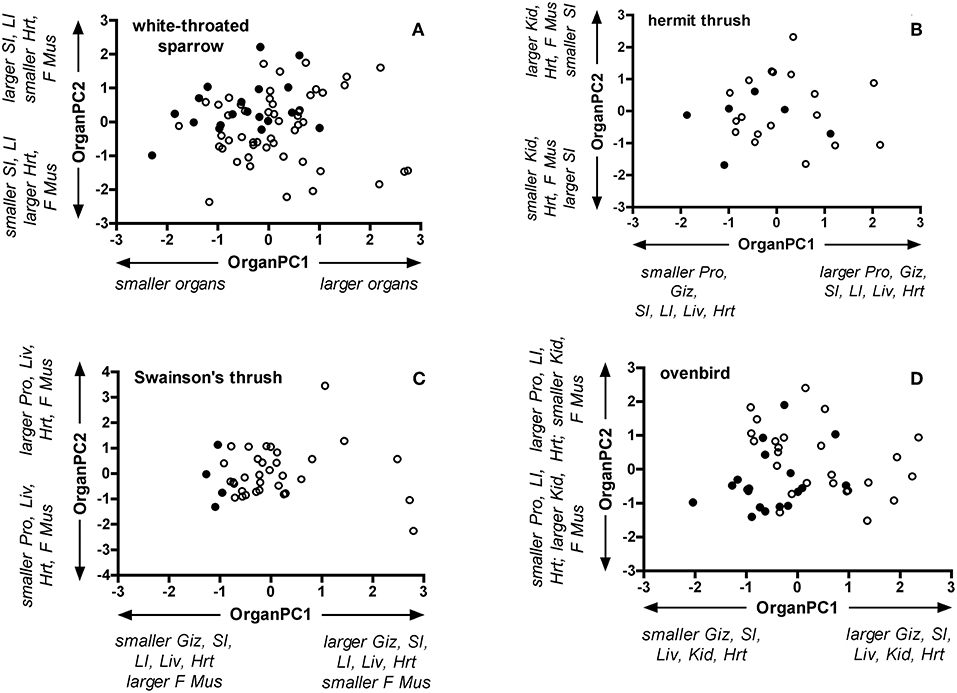
Figure 3. Bi-plots of OrganPC1 and Organ PC2 for adult and juvenile (A) white-throated sparrows, (B) hermit thrushes, (C) Swainson's thrushes, and (D) ovenbirds. Filled circles represent adults and open circles represent juveniles. OrganPC1 and OrganPC2 are the first and second principal components of separate PCA for each species that included dry masses of the proventriculus, gizzard, small intestine, large intestine, liver, kidney, heart, and flight muscle (pectoralis and supracoracoideus).
Basal Metabolic Rates
Morphometric measurements and associated statistical analyses of birds used for BMR measurements are presented in Table S4. Generally, juvenile male Swainson's thrushes were heavier than adults and juvenile females, and male white-throated sparrows were heavier than females, regardless of age class.
One adult Swainson's thrush and one juvenile white-throated sparrow did not satisfy the BMR selection criteria of at least 3 min of CO2 production with a coefficient of variation below two percent, and were excluded. Furthermore, we detected one outlier BMR value for one adult Swainson's thrush [T(51) = 3.32, P < 0.05], so it was removed. In ANCOVA of the effects of age class, sex, minimum daily temperature, and body mass on BMR of both species, all interaction terms were not significant. For Swainson's thrushes, minimum daily temperature [F(1, 44) = 0.85, P = 0.36] and sex [F(1, 46) = 2.62, P = 0.11] did not influence BMR, and were sequentially removed from the ANCOVA model. In Swainson's thrushes, after controlling for body mass [F(1, 47) = 8.19, P = 0.006], juveniles had higher BMR than adults [least squares means untransformed BMR: juvenile = 0.38 ± 0.005 W; adult = 0.36 ± 0.007 W; F(1, 47) = 6.72, P = 0.013, Figure 4A]. For white-throated sparrows, sex [F(1, 51) = 1.22, P = 0.27] and minimum daily temperature [F(1, 52) = 1.69, P = 0.20] were removed from the ANCOVA model. After controlling for body mass [F(1, 53) = 105.90, P < 0.001] juvenile white-throated sparrows had higher BMR than adults [least squares means untransfomed BMR: juvenile = 0.40 ± 0.004 W; adult = 0.38 ± 0.005 W; F(1, 53) = 12.45, P = 0.001, Figure 4B].
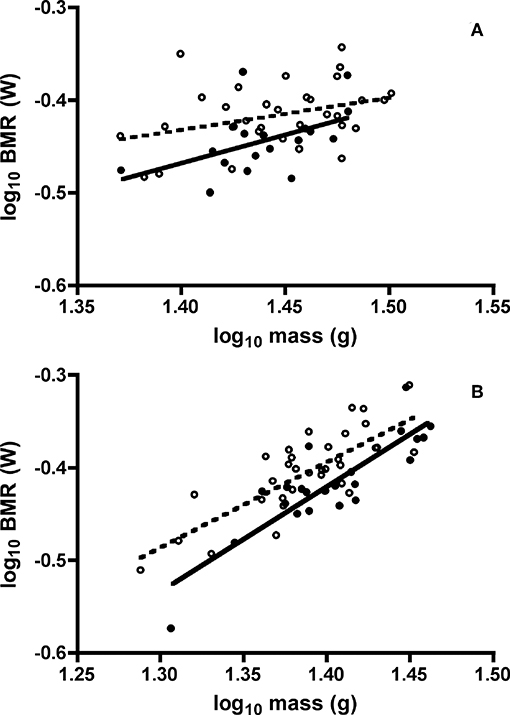
Figure 4. Effect of mass on basal metabolic rates (BMR) of juvenile and adult (A) Swainson's thrushes and (B) white-throated sparrows captured at Long Point, ON, Canada, during fall 2010 migratory stopover. Unfilled circles and dashed line represent juveniles: thrushes log10 BMR = −0.919 (±0.244) + 0.348 (±0.168) * log10 mass, r2 = 0.121; sparrows log10 BMR = −1.686 (±0.179) + 0.923 (±0.129) * log10 mass, r2 = 0.610. Filled circles and solid line represent adults: thrushes log10 BMR = −1.333 (±0.416) + 0.618 (±0.289) * log10 mass, r2 = 0.234; sparrows log10 BMR = −2.009 (±0.214) + 1.135 (±0.153) * log10 mass, r2 = 0.744.
In Swainson's thrushes, when controlling for initial mass [F(1, 46) = 4.06, P = 0.050] and total overnight time [F(1, 46) = 6.69, P = 0.013], there were no age class related differences in overnight mass loss [F(1, 46) = 0.07, P = 0.80]. Similarly, for white-throated sparrows, when controlling for initial mass [F(1, 52) = 6.27, P = 0.015] and total overnight time [F(1, 52) = 0.96, P = 0.33], there were no age class related differences in mass lost overnight [F(1, 52) = 0.26, P = 0.62].
Digestive Performance
Within each species, body mass and composition at capture did not differ between age classes or between birds that were selected for or excluded from feeding trials (Table S5). Twenty Swainson's thrushes (7 adults and 13 juveniles) and twenty white-throated sparrows (10 adults and 10 juveniles) completed 2-day total collection mass balance feeding trials. In these birds, adults and juveniles had similar arrival dates [thrushes: t(18) = −1.69, P = 0.11; sparrows: t(18) = 0.76, P = 0.46], body masses [thrushes: t(18) = 1.47, P = 0.16; sparrows: t(18) = −0.92, P = 0.37], QMR wet lean masses [thrushes: t(14) = −0.50, P = 0.63; sparrows: t(18) = −1.16, P = 0.26], and QMR fat masses [thrushes: t(14) = 1.30, P = 0.22; sparrows: t(18) = −0.16, P = 0.87] at capture (Table 3).
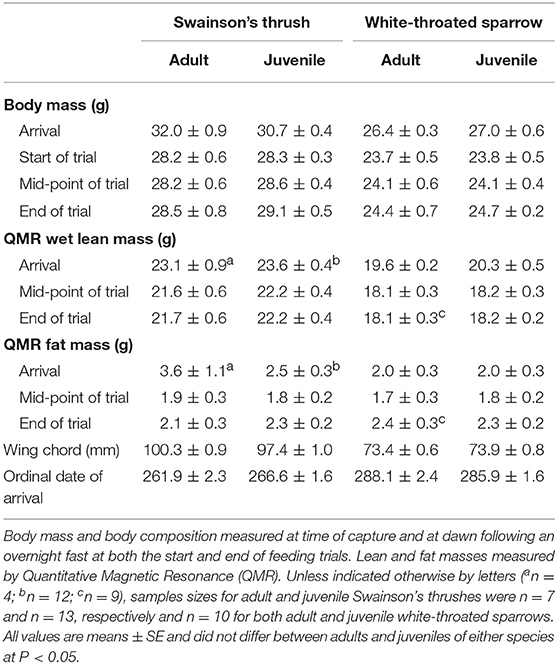
Table 3. Body mass, body composition, wing chord, and arrival date for migrating adult and juvenile Swainson's thrushes and white-throated sparrows that completed 2-day total collection mass balance feeding trials, which began 2-days after birds were captured on stopover at Long Point, Ontario, fall 2011.
At the start of a feeding trial, body masses of juveniles and adults were similar for both Swainson's thrushes [t(18) = −0.12, P = 0.91] and white-throated sparrows [t(18) = −0.044, P = 0.97; Table 3]. All Swainson's thrushes and white-throated sparrows were in positive nitrogen balance during the 2-day feeding trial. Nitrogen balance did not differ between adults and juveniles [thrushes: t(18) = −1.12, P = 0.28; sparrows: t(18) = −0.55, P = 0.59; Table 4], and nitrogen balance increased with dry food intake for both species (Figure 5). At the end of the feeding trial, body mass [thrushes: t(18) = −0.58, P = 0.57; sparrows: t(18) = −0.42, P = 0.68], QMR wet lean mass [thrushes: t(18) = −0.73, P = 0.47; sparrows: t(17) = −0.18, P = 0.86], and QMR fat mass [thrushes: t(18) = −0.45, P = 0.66; sparrows: t(17) = 0.12, P = 0.91] were similar for juveniles and adults of both species (Table 3).
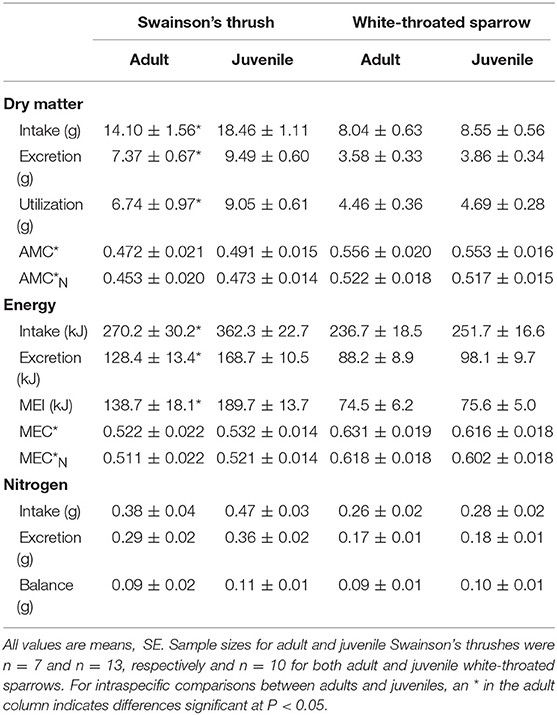
Table 4. Measures of digestive efficiency and nitrogen balance from a 2-day total collection mass balance feeding trial for migrating adult and juvenile Swainson's thrushes and white-throated sparrows captured on stopover at Long Point, Ontario, fall 2011.
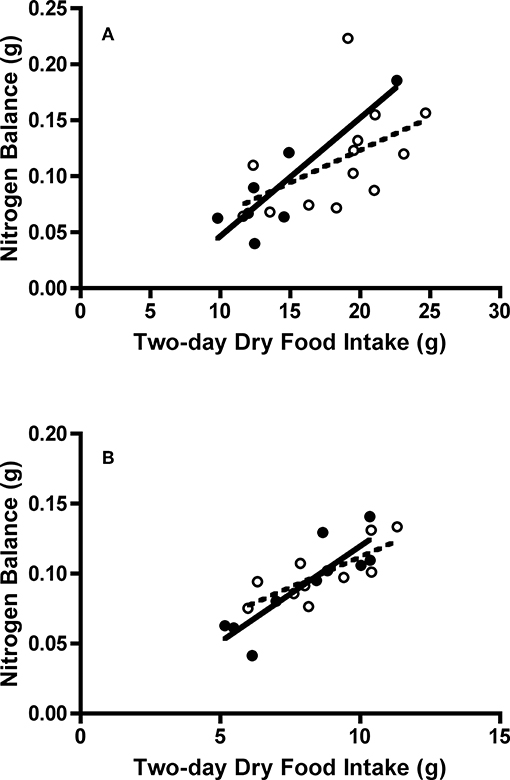
Figure 5. Effect of dry food intake on nitrogen balance of adult and juvenile (A) Swainson's thrushes and (B) white-throated sparrows during a 2-day total collection mass balance feeding trial. All birds captured at Long Point, ON, Canada during fall 2011 migration stopover. Filled circles and solid lines represent adults: thrushes nitrogen balance = −0.0588 (±0.0369) + 0.01055 (±0.0025) * dry food intake, r2 = 0.78; sparrows nitrogen balance = −0.0173 (±0.0233) + 0.0137 (±0.0028) * dry food intake, r2 = 0.75. Unfilled circles and dashed lines represent juveniles: thrushes nitrogen balance = 0.0075 (±0.0556) + 0.0058 (±0.0029) * dry food intake, r2 = 0.26; sparrows nitrogen balance = 0.0251 (±0.0221) + 0.0087 (±0.0025) * dry food intake, r2 = 0.60.
Juvenile Swainson's thrushes consumed more food than adults [t(18) = −2.30, P = 0.033; Table 4]. When controlling for QMR wet lean mass during the feeding trial [F(1, 17) = 10.36, P = 0.005], juveniles still consumed more food (LSM dry food intake = 18.11 ± 0.91 g) than adults [LSM dry food intake = 14.75 ± 1.25 g; F(1, 17) = 4.61, P = 0.046, Figure 6A]. Juvenile thrushes also had greater dry matter excretion [t(18) = −2.21, P = 0.04], dry matter utilization [t(18) = −2.11, P = 0.049], energy intake [t(18) = −2.42, P = 0.026], energy excretion [t(18) = −2.32, P = 0.032], and metabolizable energy intake [t(18) = −2.22, P = 0.039] than adults (Table 4). Adult and juvenile thrushes had similar utilization efficiencies as measured by AMC* [t(18) = −0.78, P = 0.45], [t(18) = −0.82, P = 0.43], MEC* [t(18) = −0.38, P = 0.71], and [t(18) = −0.40, P = 0.70; Table 4].
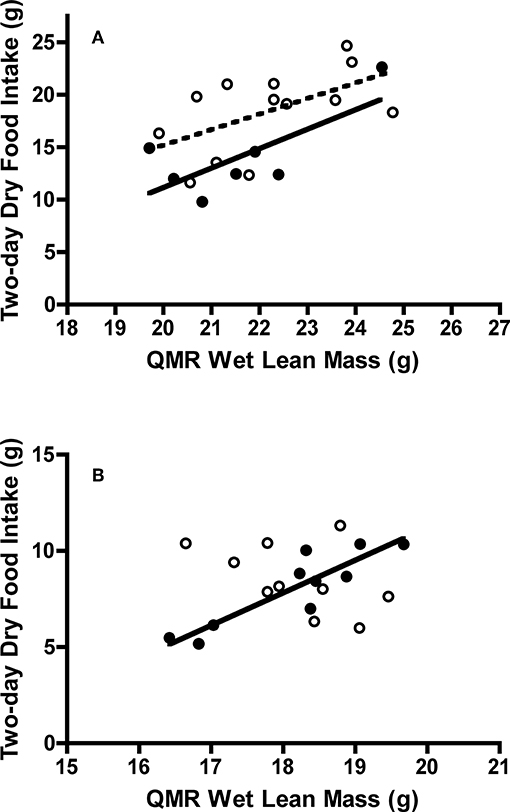
Figure 6. Effect of QMR wet lean mass on dry food intake of adult and juvenile (A) Swainson's thrushes and (B) white-throated sparrows during a 2-day total collection mass balance feeding trial. All birds captured at Long Point, ON, Canada during fall 2011 migration stopover. Filled circles and solid lines represent adults: thrushes dry food intake = −25.95 (±17.09) + 1.855 (±0.790) * QMR wet lean mass, r2 = 0.53; sparrows dry food intake = −22.74 (±5.39) + 1.698 (±0.297) * QMR wet lean mass, r2 = 0.80. Unfilled circles and dashed lines represent juveniles: thrushes dry food intake = −14.54 (±14.95) + 1.486 (±0.672) * QMR wet lean mass, r2 = 0.31; sparrows dry food intake slope did not differ from zero [F(1, 8) = 2.323, P = 0.166].
There was no difference in 2-day body mass change between adult (0.31 ± 0.32 g) and juvenile (0.77 ± 0.27 g) Swainson's thrushes during feeding trials [t(18) = −1.08, P = 0.30]. However, when controlling for dry food intake [F(1, 17) = 135.21, P < 0.001], adult thrushes (LSM body mass change = 0.92 ± 0.13 g) gained more mass than juveniles [LSM body mass change = 0.44 ± 0.09 g; F(1, 17) = 7.86, P = 0.012; Figure 7A]. While 2-day body mass change increased with nitrogen balance [F(1, 17) = 13.29, P = 0.002], no difference existed between age classes [F(1, 17) = 0.18, P = 0.68]. Energy deposited (as fat mass plus wet lean mass) increased with metabolizable energy intake [MEI; F(1, 17) = 36.48, P < 0.001], and was similar for adults and juveniles [F(1, 17) = 0.53, P = 0.48; Figure 8A]. There was no relationship between dry nitrogen intake and deposition of QMR wet lean mass [F(1, 17) = 0.073, P = 0.79], or between nitrogen balance and deposition of QMR wet lean mass [F(1, 17) = 0.24, P = 0.63], with no effect of age class on either [F(1, 17) = 0.18, P = 0.68; F(1, 17) = 0.043, P = 0.84].
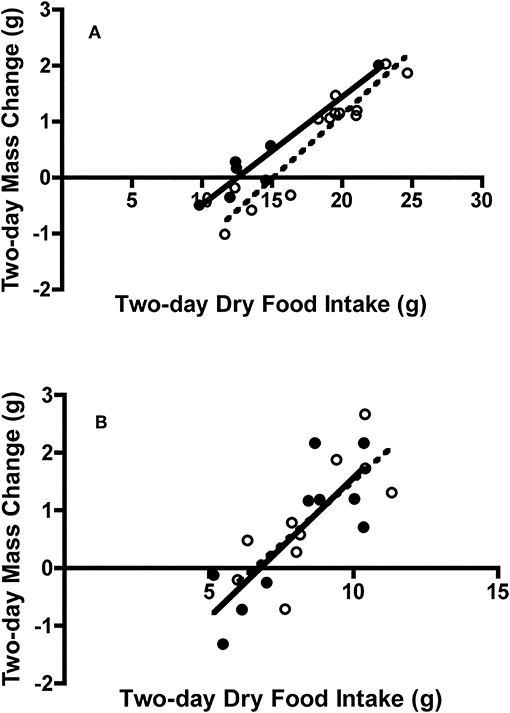
Figure 7. Effect of dry food intake on mass change of adult and juvenile (A) Swainson's thrushes and (B) white-throated sparrows during a 2-day total collection mass balance feeding trial. All birds captured at Long Point, ON, Canada during fall 2011 migration stopover. Filled circles and solid lines represent adults: thrushes mass change = −2.41 (±0.41) + 0.193 (±0.028) * dry food intake, r2 = 0.90; sparrows mass change = −3.28 (±1.02) + 0.485 (±0.124) * dry food intake, r2 = 0.66. Unfilled circles and dashed lines represent juveniles: thrushes mass change = −3.43 (±0.46) + 0.227 (±0.024) * dry food intake, r2 = 0.90; sparrows mass change = −2.89 (±1.14) + 0.440 (±0.131) * dry food intake, r2 = 0.59.
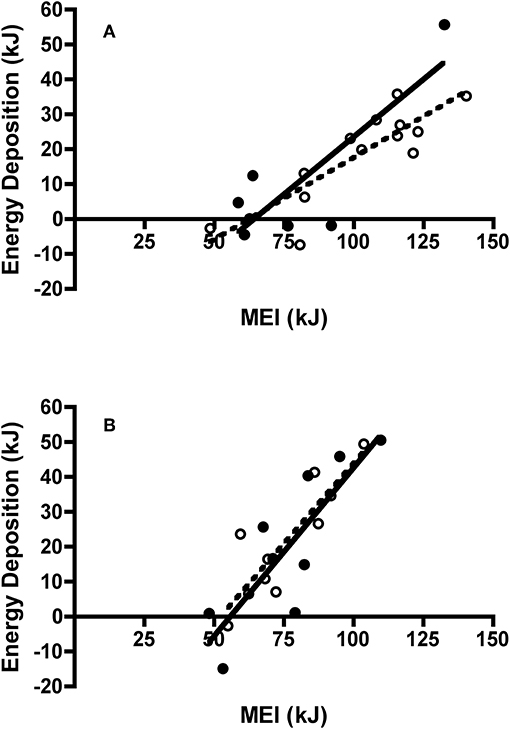
Figure 8. Effect of metabolizable energy intake (MEI) on energy deposition of adult and juvenile (A) Swainson's thrushes and (B) white-throated sparrows during the last day of a 2-day total collection mass balance feeding trial. All birds captured at Long Point, ON, Canada during fall 2011 migration stopover. Filled circles and solid lines represent adults: thrushes energy deposition = −42.15 (±16.40) + 0.658 (±0.200) * MEI, r2 = 0.68; sparrows energy deposition = −53.91 (±19.24) + 0.965 (±0.244) * MEI, r2 = 0.69. Unfilled circles and dashed lines represent juveniles: thrushes energy deposition = −29.36 (±9.23) + 0.471 (±0.088) * MEI, r2 = 0.72; sparrows energy deposition = −48.01 (±14.16) + 0.919 (±0.184) * MEI, r2 = 0.76.
Adult and juvenile white-throated sparrows consumed similar amounts of food [t(18) = −0.60, P = 0.56; Table 4]. Controlling for QMR wet lean mass during the feeding trial [F(1, 16) = 1.05, P = 0.32] revealed an interaction between age class and QMR wet lean mass [F(1, 16) = 15.60, P = 0.001], which complicated analysis. Adults increased food intake with lean mass, whereas juveniles had high food intake regardless of lean mass [F(1, 16) = 15.87, P = 0.001; Figure 6B]. Dry matter excretion [t(18) = −0.59, P = 0.56], dry matter utilization [t(18) = −0.50, P = 0.63], energy intake [t(18) = −0.60, P = 0.56], energy excretion [t(18) = −0.75, P = 0.46], and metabolizable energy intake [t(18) = −0.14, P = 0.89] were similar for adults and juvenile sparrows (Table 4). Adult and juvenile sparrows had similar AMC* [t(18) = 0.14, P = 0.89], [t(18) = 0.20, P = 0.85], MEC* [t(18) = 0.59, P = 0.56], and [t(18) = 0.63, P = 0.54; Table 4].
There was no difference in 2-day body mass change between adult (0.62 ± 0.37 g) and juvenile sparrows (0.88 ± 0.32 g) during feeding trials [t(18) = −0.53, P = 0.61]. Controlling for food intake [F(1, 17) = 28.43, P < 0.001] did not change this finding, with adults (LSM body mass change = 0.74 ± 0.22 g) and juveniles (LSM body mass change = 0.76 ± 0.22 g) gaining similar amounts of mass [F(1, 17) = 0.006, P = 0.94; Figure 7B]. Similarly, while 2-day body mass change increased with nitrogen balance [F(1, 17) = 22.30, P < 0.001], there was no difference between age classes [F(1, 17) = 0.026, P = 0.87]. Energy deposited (as fat mass and wet lean mass) increased with metabolizable energy intake [MEI; F(1, 16) = 40.36, P < 0.001], and was similar for adult and juvenile sparrows [F(1, 16) = 0.23, P = 0.64; Figure 8B]. There were no relationships between either dry nitrogen intake and deposition of QMR wet lean mass [F(1, 16) = 0.15, P = 0.71], or nitrogen balance and deposition of QMR wet lean mass [F(1, 16) = 0.16, P = 0.69], and no effects of age class [F(1, 16) = 1.74, P = 0.21; F(1, 16) = 1.69, P = 0.21].
Discussion
Our findings that juvenile birds have larger digestive organs, higher metabolic rates, and higher rates of food intake support the hypothesis that juvenile birds making their first migratory journey are physiologically different from adults in ways that influence their performance and success. It is often conjectured that young birds have poor survival during migration because they lack foraging, navigation, and predator-avoidance skills, which require cognitive development through experience and learning to improve. However, evidence is accumulating that physiological maturation of flight muscles, skeletal components, the immune system, and the digestive system may continue during migration, potentially affecting metabolic rates, energy budgets, diet selection, and ultimately migration behavior. The apparently sub-optimal physiological characteristics of juveniles may be viewed as constraints derived from the requirement to migrate before development is fully complete. However, some of their physiological characteristics are undoubtedly adaptive responses by juveniles to maximize fitness given the added challenges they face. For example, enlarged guts may allow juveniles to exploit different diets, consume more food when it is available, or employ a different digestive strategy than adults. Here we show that enlarged guts may come at the cost of increased energy expenditure. If bodies express ecology as put forward by Piersma and van Gils (2011), then differences in body composition suggest that juveniles and adults have different ecologies during migration. Moreover, if “migration takes guts” (McWilliams and Karasov, 2005), then it appears to take extra guts for juvenile birds. The larger digestive organs and higher BMRs of juveniles imply age-related disparities in foraging ecology and energy budgets. More studies of adult and juvenile birds during migratory stopovers are needed to reveal how foraging ecology and energetics of these age classes differ.
Body Composition
Our analysis of carcasses from building collisions showed that migrating juvenile songbirds were the same structural size and total dry mass as adults, which is consistent with most passerines attaining adult structural size before or shortly after fledging (Alatalo and Lundberg, 1986; Richner, 1989; Kaiser and Lindell, 2007; Verspoor et al., 2007). In contrast to some previous reports (Alatalo et al., 1984; Francis and Wood, 1989; Pyle, 1997), adults had similar wing lengths as juveniles. Juveniles from all species examined had larger livers and at least one larger component of the gastrointestinal tract than adults. These larger digestive organs appeared to contribute to greater wet lean masses of juveniles of the two short-distance migrant species (white-throated sparrows and hermit thrushes). On the other hand, while juvenile Swainson's thrushes and ovenbirds had heavier digestive organs than adults, QMR wet lean mass did not differ between age classes. This may be because adults of both of these long-distance migrant species tended to have larger flight muscles than juveniles.
Previous studies have reported similar results to ours. Hume and Biebach (1996) found migrating juvenile garden warblers (Sylvia borin) had larger dry digestive tract mass than adults. Similarly, juvenile Western sandpipers had larger digestive organs, such as small intestine and liver, than adults during fall migration (Guglielmo and Williams, 2003; Stein et al., 2005). In an analysis of organ masses of nocturnal passerine migrants killed at a television tower during fall migration, Graber and Graber (1962) reported that in general, juveniles appeared to have larger livers and smaller pectoral muscles than adults. We found similar results for the four passerine species examined, suggesting that heavier livers and digestive tract organs in juveniles during fall migration is a widespread pattern among passerine species. Larger digestive organs of juvenile migrants may be a remnant of their developmental past. That is, juvenile migrants may still be undergoing maturation or remodeling processes during fall migration, having not yet reached a fully adult condition. As nestlings, digestive organs account for a higher proportion of overall body mass than they do in adults, but the proportion declines as nestlings grow and develop (Bech and Østnes, 1999; Vézina et al., 2009). Alternatively, larger guts in juveniles may have an adaptive function to match the refueling conditions that juveniles face at stopovers, such as lower foraging success due to competition with adults or poor prey capture skills (favoring digestive efficiency maximization), a bulky less digestible diet such as fruits, or greater energy expenditure (for maintenance, thermoregulation or activity).
Basal Metabolic Rates
Our measurements of BMR for adult Swainson's thrushes (0.013 W g−1) and adult white-throated sparrows (0.015 W g−1) were very similar to BMRs previously measured resting metabolic rates (RMR) of adult Swainson's thrushes (0.017 W g-1 equivalent) during summer (Holmes and Sawyer, 1975), and BMR of adult white-throated sparrows (0.014 W g−1 equivalent) during winter (Yarbrough, 1971). Unlike our study, Holmes and Sawyer (1975) measured RMR during the active phase and during summer, which likely explains their slightly greater values. In our study BMRs of juvenile white-throated sparrows and Swainson's thrushes were consistently about 6% greater than adults. The species differ in diets, migration distance, and evolutionary lineages (Mack and Yong, 2000; Falls and Kopachena, 2010), suggesting that higher BMR in juveniles may be widespread amongst migrant passerines. Previous studies have found BMR or daily energy expenditure (DEE) to be higher for non-migratory juvenile songbirds during a timeframe comparable to pre-migration for fall migrants (Weathers and Sullivan, 1989; Chappell et al., 1999).
There are many possible physiological mechanisms for higher BMR in juveniles, including differences in organ sizes, rates of protein turnover, and continuing maturation. Lean mass, and digestive organs in particular, are energetically expensive to maintain (Martin and Fuhrman, 1955; Piersma et al., 1999), and larger lean mass can contribute to higher basal metabolic rates (Daan et al., 1990; Piersma et al., 1996; Hammond and Diamond, 1997; Williams and Tieleman, 2000; Battley et al., 2001a). Metabolic rates of digestive organs are among the highest of all organs (Krebs, 1950). For example, in European starlings (Sturnus vulgaris), in vitro basal oxygen uptake of liver was 2.66 ml O2 g−1 h−1 compared with 0.66 ml O2 g−1 h−1 for pectoralis muscle (Scott and Evans, 1992). One reason organs are expensive is that they have high rates of carbon turnover (Bauchinger and McWilliams, 2009), which likely reflect high rates of protein turnover (Carleton and Martínez del Rio, 2005; Bauchinger et al., 2010). Both protein turnover (Waterlow, 1980; Hawkins, 1991) and carbon turnover (Tieszen et al., 1983) rates are linked to metabolic rates. In particular, liver accounts for higher rates of fractional protein synthesis (Murphy and Taruscio, 1995), nitrogen incorporation rates (Muñoz-Garcia et al., 2012), and oxygen consumption due to protein synthesis (Rolfe and Brown, 1997) compared to skeletal muscle. High energy costs of organs mean they can have disproportionate effects on BMR; liver mass alone (Bech and Østnes, 1999), or together with intestines, kidney, and heart or breast muscle can explain at least half of the variation in BMR (Konarzewski and Diamond, 1995; Chappell et al., 1999). However, in an interspecific study of birds, Daan et al. (1990) instead found kidney and heart together explained 50% of variation in BMR. Burness et al. (1998) only found larger kidneys, but not liver, intestine, or heart, to be related to higher BMR among breeding tree swallows (Tachycineta bicolor).
Tissues of juvenile birds may continue to mature during migration, adding energy costs. The skeleton is incompletely formed as shown from the second layer of bone that grows in the skull of passerines during fall migration (Hamel et al., 1983; Wiley and Piper, 1992), and perhaps other bones continue to grow and mature. Similarly the immune system may be maturing in juvenile migrants, as indicated by their large and active bursa of Fabricius (Warner and Szenberg, 1964; Glick, 2000; Ratcliffe, 2006). However, adult and juvenile passerines had similar levels of constitutive (Owen and Moore, 2006; Palacios et al., 2009; Girard et al., 2011) and induced (Palacios et al., 2009) immune function prior to or during fall migration. Juvenile migrants may have higher rates of total body protein turnover than adults. It is well-documented that young rats (Rattus norvegicus) have higher protein turnover rates than adults (Yousef and Johnson, 1970; Millward and Garlick, 1972). However, Hobson and Clark (1992) found no age difference in carbon turnover rates of Japanese quail (Coturnix japonica).
Regardless of the specific mechanism(s) involved, juvenile migrants will have greater inherent energy expenditure at stopover than adults due to higher BMR. Even if daytime refueling rates are similar between age classes (Woodrey and Moore, 1997; Yong et al., 1998; Jones et al., 2002; Leist, 2007; Seewagen et al., 2013), higher BMR in juveniles will have an effect during nighttime roosting at ambient temperatures both above and below (if heat produced by BMR does not replace costs of thermogenesis) the lower critical temperature. Although we did not find a difference in the overnight loss of mass between adults and juveniles, higher nocturnal energy expenditure (due to higher BMR) could cause juveniles to have lower total daily refueling rates, and consequently longer stopover durations than adults. Higher BMR has been associated with higher field metabolic rate (FMR; Nagy, 1987; Daan et al., 1990; Koteja, 1991), but the full extent of this relationship is not clear (Ricklefs et al., 1996; Meerlo et al., 1997; Nagy, 2005). If this is the case, then juveniles will also have higher FMR than adults. Juveniles could also have greater thermoregulatory costs attributable to plumage of lower insulative quality, higher activity costs due to more frequent antagonistic interactions (Weathers and Sullivan, 1989), or greater protein requirements due to higher rates of tissue remodeling (Fisher, 1972; Bairlein, 1987). Any or all of these factors could further increase food requirements and reduce refueling performance. Additional measurements of these factors in migrating birds is needed. However, at the least, our results show that juveniles may endure costs imposed by higher BMR, which could make fall migration even more challenging for these first time migrants.
Digestive Performance
Digestive physiology of juvenile Swainson's thrushes clearly differed from that of adults, with juveniles using higher food intake to assimilate more energy. Conversely, we found no age class related differences in digestive physiology among white-throated sparrows. Larger thrushes consumed more food, but at the same lean mass, juveniles consumed more food than adults. Presumably, juveniles achieved higher food intake due to larger digestive organs, which we observed in carcasses of conspecifics sampled in Toronto about 170 km northeast of Long Point. However, juveniles did not have higher digestive efficiency, which agrees with predictions of van Gils et al. (2008). Juvenile thrushes ingested more energy and appeared to pursue a more rate maximizing digestive strategy relative to adults by using their larger guts to accommodate extra food while maintaining a similar digestive efficiency. Swainson's thrushes in our study had lower MEC* (0.53) compared to other passerines consuming natural fruit diets (0.64; Karasov, 1990). Compared to thrushes from our study, American robins (Turdus migratorius) had similar MEC* values consuming fruit (0.55), but not when consuming a comparable banana mash diet (0.77; Levey and Karasov, 1989). Juvenile thrushes converted food into body stores less efficiently than adults, suggesting that they expend more energy than adults either for maintenance (BMR, see above), thermoregulation or activity. Although we did not measure activity levels, it is possible that juveniles were more active in cages than adults.
Juvenile and adult white-throated sparrows consumed comparable amounts of food. However, juveniles consumed a similar amount of food regardless of lean mass, whereas among adults, heavier sparrows consumed more food. This suggests that juveniles may maintain a high digestive capacity under all conditions, whereas food intake in adults may be limited by low digestive capacity. The presumed larger digestive organs of juveniles may have permitted them to consume more food at low lean body masses. Juveniles did not appear to use their larger digestive organs to pursue a more efficiency-maximizing digestive strategy as juveniles and adults had similar digestive efficiencies. White-throated sparrows that consumed sunflower seeds in our experiment had lower MEC* values (0.62) than several passerines that consumed either cultivated (0.80) or wild (0.75) seeds (Karasov, 1990). Both juvenile and adult white-throated sparrows converted food into body stores with similar efficiencies. Thus, the age-related difference in BMR we measured did not translate into difference in how efficiently juveniles and adults converted food into body mass. However, as we did not monitor activity levels of birds while in captivity, it might be possible that adults were more active compared to juveniles. Among recently captured blackcaps (Sylvia atricapilla), adults displayed greater nocturnal restlessness than juveniles (Berthold, 1996), but Ketterson and Nolan (1985) noted no difference in nightly zugunrhue activity among adult and juvenile dark-eyed juncos (Junco hyemalis).
Why might juvenile Swainson's thrushes show increased food intake relative to adults, whereas juvenile white-throated sparrows did not? During fall migration, Swainson's thrushes typically consume a predominantly fruit-based diet, supplemented with insects (Jones and Donovan, 1996; Mack and Yong, 2000; Parrish, 2000), whereas white-throated sparrows tend to consume a more varied diet that includes a combination of mostly seeds, fruits, and some insects (Falls and Kopachena, 2010). Generally, birds consuming fruits have lower digestive efficiencies than when consuming insects or seeds (Castro et al., 1989; Karasov, 1990). Additionally, due to the lower energy density and protein content of fruits relative to insects and seeds (Johnson et al., 1985; Moermond and Denslow, 1985; Karasov, 1990), frugivorous species need to consume greater quantities of food to satisfy daily energy and protein requirements (Aamidor et al., 2011). Accordingly, in our experiment, Swainson's thrushes consumed a synthetic fruit-based diet that was about 85% water, whereas sparrows were fed shelled sunflower seeds that were about 5% water. Over the 2-day feeding trials a typical 28.2 g adult thrush consumed 94.0 ± 10.4 g wet food, whereas a 23.7 g adult white-throated sparrow ate 8.46 ± 0.66 g wet food. Perhaps bulk of the synthetic fruit-based diet pushed digestive systems of thrushes nearer to the limits of food intake capacity, while digestive systems of sparrows might have had sufficient capacity to readily accommodate the energy-dense diet of sunflower seeds. Furthermore, the sunflower seeds were shelled, which require less handling time and have more metabolizable energy than intact, wild equivalents (Karasov, 1990). Consequently, juvenile sparrows may have met their energy and protein requirements from consuming a smaller volume of food than they otherwise would in a natural setting with a more varied diet. Along with many other passerines migrating during fall, white-throated sparrows include fruits as part of their diet (Parrish, 1997, 2000). During fall migration stopover on Block Island, Rhode Island, 92% of fecal samples from white-throated sparrows contained fruit (Parrish, 1997). Larger digestive organs of juvenile white-throated sparrows suggest they may be capable of accommodating the additional bulk from a diet that consists of more fruit. We propose that juvenile white-throated sparrows may rely more heavily on fruit to meet dietary needs at stopover than do adults, and under those conditions they may show similar patterns to thrushes.
Whereas, diets of passerines during migration are understood in general terms, whether and how diet compositions of juveniles and adults differ is not. Wheelwright (1986) found higher proportions of fruits in stomachs of juvenile than of adult American robins (Turdus migratorius), but it was not clear if the samples were taken during migration. Future studies of diet compositions of juvenile and adult passerines at migratory stopover sites may reveal how diets of juveniles and adults compare, and whether diets of juveniles consist of relatively more fruit. Use of DNA barcoding to identify species origins of diet remains within fecal samples may facilitate analysis (Hebert et al., 2003; CBOL Plant Working Group, 2009). Isotopic analysis of breath or tissues of migrants may also provide information on dietary differences (Hobson, 2011). Clearly, foraging ecologies of juvenile and adult migrant passerines during stopover require further study.
Conclusion
Larger digestive organs of juvenile migrants can both result from and enable higher food intake, while larger organs can contribute to higher maintenance metabolic rates. The collective findings of this study imply that disparities in foraging ecology, physiology, or both prompt juveniles to consume greater quantities of food, which subsequently causes hypertrophy of digestive organs. Specifically, the diets of juvenile songbirds could be more dilute, consisting of relatively more fruit in comparison to adults. Indeed, Fruit is voluminous, high in water, and has lower energy densities and protein contents compared to insects and seeds (Moermond and Denslow, 1985; Karasov, 1990). Juveniles that consume relatively more fruit may consequently consume larger volumes to meet protein and energy requirements, which may cause juveniles to enlarge their digestive organs in response. However, the fact that livers were consistently larger in juveniles of all species suggests that the story may not just be about diet quality, and that more total energy and nutrients may need to be processed by juveniles following digestion and absorption (Starck, 1999; Starck and Rahmaan, 2003; Battley and Piersma, 2005). Thus, an alternative explanation is that high cellular activities and protein turnover rates associated with the development and maturation of the skeletal, immune, muscular, and other systems of juveniles could contribute to higher metabolic rates. To meet higher demands for energy juveniles would need to consume more food, which could cause hypertrophy of digestive organs. In this manner, it would be possible for physiology to influence ecology, as foraging of juveniles in the wild would likely be affected. Longer-term captive feeding studies could differentiate between ecology and physiology as drivers of large digestive systems in juvenile migrants.
Data Availability Statement
The data used in the analyses are available at Guglielmo, Christopher; McCabe, Brendan (2019), Migration takes extra guts for juvenile songbirds: energetics and digestive physiology during the first journey, Dryad, Dataset, https://doi.org/10.5061/dryad.p5hqbzkkh.
Ethics Statement
The animal study was reviewed and approved by Animal Care Committee, University of Western Ontario.
Author Contributions
BM participated in experimental design, conducted experiments and laboratory analyses, collected data, analyzed data, and co-wrote the manuscript. CG participated in experimental design, obtained animal care protocols and permits, assisted with experiments, laboratory analyses and data analysis, and co-wrote the manuscript.
Funding
Funding for this study provided by grants to CG from the Natural Sciences and Engineering Research Council of Canada (05245-2015 RGPIN), the Canada Foundation for Innovation (11743), an Ontario Early Researcher Award, and the Ontario Research Fund.
Conflict of Interest
The authors declare that the research was conducted in the absence of any commercial or financial relationships that could be construed as a potential conflict of interest.
Acknowledgments
We thank Michael Mesure and Paloma Plant of the Fatal Light Awareness Program and Mark Peck of the Royal Ontario Museum for coordinating transfer of frozen carcasses previously salvaged by volunteers. We thank the volunteers and staff of the Long Point Bird Observatory for assistance with field studies and Mike Burrell for his expertise on aging birds. We thank Alexander Gerson and Liam McGuire for advice on respirometry and statistics. We are very grateful to Lisa Kennedy for help with the feeding trials. We extend a special thanks to all volunteers (Brieann Adair, Dillon Chung, Lisa Cohen, Patrick David, Melissa Giamou, Alexander Macmillan, Angela Paric, Anna Pauer, Sara Rea, and Dustin Yen) that helped perform dissections.
Supplementary Material
The Supplementary Material for this article can be found online at: https://www.frontiersin.org/articles/10.3389/fevo.2019.00381/full#supplementary-material
References
Aamidor, S. E., Bauchinger, U., Mizrahy, O., McWilliams, S. R., and Pinshow, B. (2011). During stopover, migrating blackcaps adjust behavior and intake of food depending on the content of protein in their diets. Integr. Comp. Biol. 51, 385–393. doi: 10.1093/icb/icr054
Alatalo, R. V., Gustafsson, L., and Lundberg, A. (1984). Why do young passerine birds have shorter wings than older birds? IBIS 126, 410–415. doi: 10.1111/j.1474-919X.1984.tb00264.x
Alatalo, R. V., and Lundberg, A. (1986). Heritability and selection on tarsus length in the pied flycatcher (Ficedula hypoleuca). Evolution 40, 574–583. doi: 10.1111/j.1558-5646.1986.tb00508.x
Alerstam, T., and Lindström, Å. (1990). “Optimal bird migration: the relative importance of time, energy and safety,” in Bird Migration: Physiology and Ecophysiology, ed E. Gwinner (Berlin; Heidelberg: Springer-Verlag, 331–351.
Arizaga, J., Barba, E., and Belda, E. J. (2008). Fuel management and stopover duration of blackcaps Sylvia atricapilla stopping over in northern Spain during autumn migration period. Bird Study 55, 124–134. doi: 10.1080/00063650809461513
Bairlein, F. (1987). Nutritional requirements for maintenance of body weight and fat deposition in the long-distance migratory garden warbler, Sylvia borin (Boddaert). Comp. Biochem. Physiol. 86A, 337–347. doi: 10.1016/0300-9629(87)90340-9
Battley, P. F., Dekinga, A., Dietz, M. W., Piersma, T., Tang, S., and Hulsman, K. (2001). Basal metabolic rate declines during long-distance migratory flight in great knots. Condor 103, 838–845. doi: 10.1093/condor/103.4.838
Battley, P. F., and Piersma, T. (2005). “Adaptive interplay between feeding ecology and features of the digestive tract in birds,” in Physiological and Ecological Adaptations to Feeding in Vertebrates, eds J. M. Starck and T. Wang (Enfield, NH: Science Publishers, 201–228.
Bauchinger, U., Keil, J., McKinney, R. A., Starck, J. M., and McWilliams, S. R. (2010). Exposure to cold but not exercise increases carbon turnover rates in specific tissues of a passerine. J. Exp. Biol. 213, 526–534. doi: 10.1242/jeb.037408
Bauchinger, U., and McWilliams, S. (2009). Carbon turnover in tissues of a passerine bird: allometry, isotopic clocks, and phenotypic flexibility in organ size. Physiol. Biochem. Zool. 82, 787–797. doi: 10.1086/605548
Bech, C., and Østnes, J. E. (1999). Influence of body composition on the metabolic rate of nestling European shags (Phalacrocorax aristotelis). J. Comp. Physiol. B 169, 263–270. doi: 10.1007/s003600050220
Bercovich, D., Plotsky, Y., and Gruenbaum, Y. (1999). Improved protocol for using avian red blood cells as substrates for the polymerase chain reaction. Biotechniques 26, 1080–1082. doi: 10.2144/99266bm16
Bishop, C. M., Butler, P. J., Egginton, S., El Haj, A. J., and Gabrielsen, G. W. (1995). Development of metabolic enzyme activity in locomotor and cardiac muscles of the migratory barnacle goose. Am. J. Physiol. 269, R64–R72. doi: 10.1152/ajpregu.1995.269.1.R64
Boyle, W. A. (2009). How to keep tropical montane frugivorous birds in captivity. Ornitol. Neotrop. 20, 265–273.
Burness, G. P., Ydenberg, R. C., and Hochachka, P. W. (1998). Interindividual variability in body composition and resting oxygen consumption rate in breeding tree swallows, Tachycineta bicolor. Physiol. Ecol. 71, 247–256. doi: 10.1086/515917
Carleton, S. A., and Martínez del Rio, C. (2005). The effect of cold-induced increased metabolic rate on the rate of 13C and 15N incorporation in house sparrows (Passer domesticus). Oecologia 144, 226–232. doi: 10.1007/s00442-005-0066-8
Castro, G., Stoyan, N., and Myers, J. P. (1989). Assimilation efficiency in birds: a function of taxon or food type? Comp. Biochem. Physiol. 92A, 271–278. doi: 10.1016/0300-9629(89)90563-X.
CBOL Plant Working Group (2009). A DNA barcode for land plants. Proc. Natl. Acad. Sci. U.S.A. 106, 12794–12797. doi: 10.1073/pnas.0905845106
Chappell, M. A., Bech, C., and Buttemer, W. A. (1999). The relationship of central and peripheral organ masses to aerobic performance variation in house sparrows. J. Exp. Biol. 202, 2269–2279.
Daan, S., Masman, D., and Groenewold, A. (1990). Avian basal metabolic rates: their association with body composition and energy expenditure in nature. Am. J. Physiol. 259, R333–R340. doi: 10.1152/ajpregu.1990.259.2.R333
Denslow, J. S., Levey, D. J., Moermond, T. C., and Wentworth, B. C. (1987). A synthetic diet for fruit-eating birds. Wilson Bull. 99, 131–135.
Dolnik, V. R., and Blyumental, T. I. (1967). Autumnal premigratory and migratory periods in the chaffinch Fringilla coelebs and some other temperate zone passerine birds. Condor 69, 435–468. doi: 10.2307/1366146
Dunn, O. J., and Clark, V. A. (1987). Applied Statistics: Analysis of Variance and Regression, 2nd Edn. New York, NY: John Wiley.
Dykstra, C. R., and Karasov, W. H. (1992). Changes in gut structure and function of house wrens (Troglodytes aedon) in response to increased energy demands. Physiol. Zool. 65, 422–442. doi: 10.1086/physzool.65.2.30158261
Ellegren, H. (1990). Autumn migration speed in Scandinavian bluethroats Luscinia s. svecica. Ringing Migr. 11, 121–131. doi: 10.1080/03078698.1990.9673974
Ellegren, H. (1991). Stopover ecology of autumn migrating bluethroats Luscinia s. svecica in relation to age and sex. Ornis Scand. 22, 340–348. doi: 10.2307/3676506
Falls, J. B., and Kopachena, J. G. (2010). “White-throated sparrow (Zonotrichia albicollis),” in The Birds of North America Online, ed A. Poole (Ithaca, NY: Cornell Lab of Ornithology). Retrieved from the Birds of North America Online: http://bna.birds.cornell.edu/bna/species/128
Fisher, H. (1972). “The nutrition of birds,” in Avian Biology, Vol. 2, eds D. S. Farner, J. R. King, and K. C. Parkes (New York, NY:Academic Press), 431–469.
Francis, C. M., and Wood, D. S. (1989). Effects of age and wear on wing length of wood-warblers. J. Field Ornithol. 60, 495–503.
Fransson, T. (1986). The migration and wintering area of Nordic spotted flycatcher Muscicapa striata. Vår Fågelvärld 45, 4–18.
Fransson, T. (1995). Timing and speed of migration in North and West European populations of Sylvia warblers. J. Avian Biol. 26, 39–48. doi: 10.2307/3677211
Girard, J., Goldberg, T. L., and Hamer, G. L. (2011). Field investigation of innate immunity in passerine birds in suburban Chicago, Illinois, USA. J. Wildl. Dis. 47, 603–611. doi: 10.7589/0090-3558-47.3.603
Glick, B. (2000). “Immunophysiology,” in Sturkie's Avian Physiology, 5th Edn. ed G. C. Whittow (New York, NY: Academic Press), 657–670.
Graber, R. R., and Graber, J. W. (1962). Weight characteristics of birds killed in nocturnal migration. Wilson Bull. 74, 74–88.
Griffiths, R., Double, M. C., Orr, K., and Dawson, R. J. G. (1998). Mol. Ecol. 7, 1071–1075. doi: 10.1046/j.1365-294x.1998.00389.x
Guglielmo, C. G. (2018). Obese super athletes: fat-fueled migration in birds and bats. J. Exp. Biol. 221 (Suppl. 1):jeb165753. doi: 10.1242/jeb.165753
Guglielmo, C. G., Haunerland, N. H., Hochachka, P. W., and Williams, T. D. (2002). Seasonal dynamics of flight muscle fatty acid binding protein and catabolic enzymes in a migratory shorebird. Am. J. Physiol. Regul. Integr. Comp. Physiol. 282, R1405–R1413. doi: 10.1152/ajpregu.00267.2001
Guglielmo, C. G., and Karasov, W. H. (1993). Endogenous mass and energy losses in ruffed grouse. Auk 110, 386–390.
Guglielmo, C. G., Karasov, W. H., and Jakubas, W. J. (1996). Nutritional costs of a plant secondary metabolite explain selective foraging by ruffed grouse. Ecology 77, 1103–1115. doi: 10.2307/2265579
Guglielmo, C. G., McGuire, L. P., Gerson, A. R., and Seewagen, C. L. (2011). Simple, rapid, and non-invasive measurement of fat, lean, and total water masses of live birds using quantitative magnetic resonance. J. Ornithol. 152, S75–S85. doi: 10.1007/s10336-011-0724-z
Guglielmo, C. G., and Williams, T. D. (2003). Phenotypic flexibility of body composition in relation to migratory state, age, and sex in the western sandpiper (Calidris mauri). Physiol. Biochem. Zool. 76, 84–98. doi: 10.1086/367942
Hamel, P. B., Beacham, J. L., and Ross, A. E. (1983). A laboratory study of cranial pneumatization in indigo buntings. J. Field Ornithol. 54, 58–66.
Hammond, K. A., and Diamond, J. (1992). An experimental test for a ceiling on sustained metabolic rate in lactating mice. Physiol. Zool. 65, 952–977. doi: 10.1086/physzool.65.5.30158552
Hammond, K. A., and Diamond, J. (1997). Maximal sustained energy budgets in humans and animals. Nature 386, 457–462. doi: 10.1038/386457a0
Hawkins, A. J. S. (1991). Protein turnover: a functional appraisal. Funct. Ecol. 5, 222–223. doi: 10.2307/2389260
Hebert, P. D., Cyminska, A., Ball, S. L., and deWaard, J. R. (2003). Biological identifications through DNA barcodes. Proc. Biol. Sci. 270, 313–321. doi: 10.1098/rspb.2002.2218
Heise, C. D., and Moore, F. R. (2003). Age-related differences in foraging efficiency, molt, and fat deposition of gray catbirds prior to autumn migration. Condor 105, 496–504. doi: 10.1093/condor/105.3.496
Hobson, K. A. (2011). Isotopic ornithology: a perspective. J. Ornithol. 152, S49–S66. doi: 10.1007/s10336-011-0653-x
Hobson, K. A., and Clark, R. G. (1992). Assessing avian diets using stable isotopes I: turnover of 13C in tissues. Condor 94, 181–188. doi: 10.2307/1368807
Holmes, R. T., and Sawyer, R. H. (1975). Oxygen consumption in relation to ambient temperature in five species of forest-dwelling thrushes (Hylocichla and Catharus). Comp. Biochem. Physiol. 50A, 527–531. doi: 10.1016/0300-9629(75)90310-2
Hume, I. D., and Biebach, H. (1996). Digestive tract function in the long-distance migratory garden warbler, Sylvia borin. J. Comp. Physiol. B 166, 388–395. doi: 10.1007/BF02336922
Jenni, L., and Jenni-Eiermann, S. (1999). “Fat and protein utilisation during migratory flight,” in Proceedings of the International Ornithological Congress, Vol. 22, 1437–1449.
Johnson, R. A., Willson, M. F., Thompson, J. N., and Bertin, R. I. (1985). Nutritional values of wild fruits and consumption by migrant frugivorous birds. Ecology 66, 819–827. doi: 10.2307/1940543
Jones, J., Francis, C. M., Drew, M., Fuller, S., and Ng, M. W. S. (2002). Age-related differences in body mass and rates of mass gain of passerines during autumn migratory stopover. Condor 104, 49–58. doi: 10.1093/condor/104.1.49
Jones, P. W., and Donovan, T. M. (1996). “Hermit thrush (Catharus guttatus),” in The Birds of North American Online, ed A. Poole (Ithaca: Cornell Lab of Ornithology). Retrieved from the Birds of North America Online: http://bna.birds.cornell.edu/bna/species/261
Kaiser, S. A., and Lindell, C. A. (2007). Effects of distance to edge and edge type on nestling growth and nest survival in the wood thrush. Condor 109, 288–303. doi: 10.1093/condor/109.2.288
Karasov, W. H. (1990). Digestion in birds: chemical and physiological determinants and ecological implications. Stud. Avian Biol. 13, 391–415.
Karasov, W. H., and Martinez del Rio, C. (2007). Physiological Ecology: How Animals Process Energy, Nutrients and Toxins. Princeton: Princeton University Press.
Kennedy, L. V. (2012). Dynamics of fat and lean mass in refueling migrant passerines measured using quantitative magnetic resonance (thesis). University of Western Ontario, London, ON, Canada.
Ketterson, E. D., and Nolan, V. Jr. (1985). Intraspecific variation in avian migration: evolutionary and regulatory aspects. Contrib. Mar. Sci. 27, 553–579.
Konarzewski, M., and Diamond, J. (1995). Evolution of basal metabolic rate and organ masses in laboratory mice. Evolution 49, 1239–1248. doi: 10.1111/j.1558-5646.1995.tb04450.x
Koteja, P. (1991). On the relation between basal and field metabolic rates in birds and mammals. Funct. Ecol. 5, 56–64. doi: 10.2307/2389555
Krebs, H. A. (1950). Body size and tissue respiration. Biochim. Biophys. Acta 4, 249–269. doi: 10.1016/0006-3002(50)90032-1
Leist, A. J. (2007). The importance of fruit to swainson's thrushes, catharus ustulatus, during fall migration: a field test of plasma metabolite analysis (Thesis). Humboldt State University, Arcata, CA, United States.
Levey, D. J., and Karasov, W. H. (1989). Responses of temperate birds switched to fruit or insect diets. Auk 106, 675–686. doi: 10.2307/4087757
Lighton, J. R. B. (2008). Measuring Metabolic Rates: A Manual for Scientists. New York, NY: Oxford University Press.
Lindström, Å. (1997). Basal metabolic rates of migrating waders in the Eurasian arctic. J. Avian Biol. 28, 87–92. doi: 10.2307/3677098
Mack, D. E., and Yong, W. (2000). “Swainson's thrush (Catharus ustulatus),” in The Birds of North America Online, ed A. Poole (Ithaca: Cornell Lab of Ornithology). Retrieved from the Birds of North America Online: http://bna.birds.cornell.edu/bna/species/540
Martin, A. W., and Fuhrman, F. A. (1955). The relationship between summated tissue respiration and metabolic rate in the mouse and dog. Physiol. Zool. 28, 18–34. doi: 10.1086/physzool.28.1.30152176
Martinez del Rio, C., and Karasov, W. H. (1990). Digestion strategies in nectar- and fruit-eating birds and the sugar composition of plant rewards. Am. Nat. 136, 618–637. doi: 10.1086/285119
McCabe, B. J. (2006). Fat stores, plumage morphs, and sex of migrant white-throated sparrows (Thesis). State University of New York College at Brockport, Brockport, NY, United States.
McGuire, L. P., and Guglielmo, C. G. (2010). Quantitative magnetic resonance: a rapid, noninvasive body composition analysis technique for live and salvaged bats. J. Mammal. 91, 1375–1380. doi: 10.1644/10-MAMM-A-051.1
McWilliams, S. R., Caviedes-Vidal, E., and Karasov, W. H. (1999). Digestive adjustments in cedar waxwings to high feeding rate. J. Exp. Zool. 283, 394–407.
McWilliams, S. R., and Karasov, W. H. (2005). “Migration takes guts: digestive physiology of migratory birds and its ecological significance,” in Birds of Two Worlds, eds P. P. Marra and R. Greenberg (Washington, DC: Smithsonian Institution Press, 67–78.
Meerlo, P., Bolle, L., Visser, G. H., Masman, D., and Daan, S. (1997). Basal metabolic rate in relation to body composition and daily energy expenditure in the field vole, Microtus agrestis. Physiol. Zool. 70, 362–369. doi: 10.1086/639616
Miller, A. H. (1946). A method of determining the age of live passerine birds. Bird Banding 17, 33–35. doi: 10.2307/4509901
Miller, M. R. (1975). Gut morphology of mallards in relation to diet quality. J. Wildl. Manage. 39, 168–173. doi: 10.2307/3800481
Mills, A. M., Thurber, B. G., Mackenzie, S. A., and Taylor, P. D. (2011). Passerines use nocturnal flights for landscape-scale movements during migration stopover. Condor 113, 597–607. doi: 10.1525/cond.2011.100186
Millward, D. J., and Garlick, P. J. (1972). The pattern of protein turnover in the whole animal and the effect of dietary variations. Proc. Nutr. Soc. 31, 257–263. doi: 10.1079/PNS19720049
Moermond, T. C., and Denslow, J. S. (1985). Neotropical avian frugivores: patterns of behavior, morphology, and nutrition, with consequences for fruit selection. Ornithol. Monogr. 36, 865–897. doi: 10.2307/40168322
Moore, S. J., and Battley, P. F. (2006). Differences in the digestive organ morphology of captive and wild brown teal Anas chlorotis and implications for releases. Bird Conserv. Int. 16, 253–264. doi: 10.1017/S0959270906000396
Morris, S. R., Holmes, D. W., and Richmond, M. E. (1996). A ten-year study of the stopover patterns of migratory passerines during fall migration on Appledore Island, Maine. Condor 98, 395–409. doi: 10.2307/1369157
Muñoz-Garcia, A., Aamidor, S. E., McCue, M. D., McWilliams, S. R., and Pinshow, B. (2012). Allocation of endogenous and dietary protein in the reconstitution of the gastrointestinal tract in migratory blackcaps at stopover sites. J. Exp. Biol. 215, 1069–1075. doi: 10.1242/jeb.062547
Murphy, M. E., and Taruscio, T. G. (1995). Sparrows increase their rates of tissue and whole-body protein synthesis during the annual molt. Comp. Biochem. Physiol. 111A, 385–396. doi: 10.1016/0300-9629(95)00039-A
Nagy, K. A. (1987). Field metabolic rate and food requirement scaling in mammals and birds. Ecol. Monogr. 57, 111–128. doi: 10.2307/1942620
Nagy, K. A. (2005). Field metabolic rate and body size. J. Exp. Biol. 208, 1621–1625. doi: 10.1242/jeb.01553
Owen, J. C., and Moore, F. R. (2006). Seasonal differences in immunological condition of three species of thrushes. Condor 108, 389–398. doi: 10.1093/condor/108.2.389
Palacios, M. G., Cunnick, J. E., Vleck, D., and Vleck, C. M. (2009). Ontogeny of innate and adaptive immune defense components in free-living tree swallows, Tachycineta bicolor. Dev. Comp. Immunol. 33, 456–463. doi: 10.1016/j.dci.2008.09.006
Parrish, J. D. (1997). Patterns of frugivory and energetic condition in nearctic landbirds during autumn migration. Condor 99, 681–697. doi: 10.2307/1370480
Parrish, J. D. (2000). Behavioral, energetic, and conservation implications of foraging plasticity during migration. Stud Avian Biol. 20, 53–70.
Penry, D. L., and Jumars, P. A. (1986). Chemical reactor analysis and optimal digestion. Bioscience 36, 310–315.
Pierce, B. J., and McWilliams, S. R. (2004). Diet quality and food limitation affect the dynamics of body composition and digestive organs in a migratory songbird (Zonotrichia albicollis). Physiol. Biochem. Zool. 77, 471–483. doi: 10.1086/383503
Piersma, T., Bruinzeel, L., Drent, R., Kersten, M., Van der Meer, J., and Wiersma, P. (1996). Variability in basal metabolic rate of a long-distance migrant shorebird (red knot, Calidris canutus) reflects shifts in organ sizes. Physiol. Zool. 68, 191–217. doi: 10.1086/physzool.69.1.30164207
Piersma, T., and Drent, J. (2003). Phenotypic flexibility and the evolution of organismal design. Trends Ecol. Evol. 18, 228–233. doi: 10.1016/S0169-5347(03)00036-3
Piersma, T., Gudmundsson, G. A., and Lilliendahl, K. (1999). Rapid changes in the size of different functional organ and muscle groups during refueling in a long-distance migrating shorebird. Physiol. Biochem. Zool. 72, 405–415. doi: 10.1086/316680
Piersma, T., and Lindström, A. (1997). Rapid reversible changes in organ size as a component of adaptive behaviour. Trends Ecol. Evol. 12, 134–138. doi: 10.1016/S0169-5347(97)01003-3
Piersma, T., and van Gils, J. A. (2011). The Flexible Phenotype. New York, NY: Oxford University Press.
Ratcliffe, M. J. (2006). Antibodies, immunoglobulin genes and the bursa of Fabricius in chicken B cell development. Dev. Comp. Immunol. 30, 101–118. doi: 10.1016/j.dci.2005.06.018
Rguibi-Idrissi, H., Julliard, R., and Bairlein, F. (2003). Variation in the stopover duration of reed warblers Acrocephalus scirpaceus in Morocco: effects of season, age and site. IBIS 145, 650–656. doi: 10.1046/j.1474-919X.2003.00208.x
Richner, H. (1989). Habitat-specific growth and fitness in carrion crows (Corvus corone corone). J. Anim. Ecol. 58, 427–440. doi: 10.2307/4840
Ricklefs, R. E., Konarzewski, M., and Daan, S. (1996). The relationship between basal metabolic rate and daily energy expenditure in birds and mammals. Am. Nat. 147, 1047–1071. doi: 10.1086/285892
Rolfe, D. F. S., and Brown, G. C. (1997). Cellular energy utilization and molecular origin of standard metabolic rate in mammals. Physiol. Rev. 77, 731–758. doi: 10.1152/physrev.1997.77.3.731
Scott, I., and Evans, P. R. (1992). The metabolic output of avian (Sturnus vulgaris, Calidris alpina) adipose tissue liver and skeletal muscle: implication for BMR/body mass relationships. Comp. Biochem. Physiol. 103A, 329–332. doi: 10.1016/0300-9629(92)90589-I
Secor, S. M., and Diamond, J. (1995). Adaptive responses to feeding in Burmese pythons: pay before pumping. J. Exp. Biol. 198, 1313–1325.
Secor, S. M., and Diamond, J. M. (2000). Evolution of regulatory responses to feeding in snakes. Physiol. Biochem. Zool. 73, 123–141. doi: 10.1086/316734
Seewagen, C. L., Guglielmo, C. G., and Morbey, Y. E. (2013). Stopover refueling rate underlies protandry and seasonal variation in migration timing of songbirds. Behav. Ecol. 24, 634–642. doi: 10.1093/beheco/ars225
Shimizu, M., Hayashi, T., Saitoh, Y., Ohta, K., and Itoh, H. (1990). Postmortem autolysis in the pancreas: multivariate statistical study. The influence of clinicopathological conditions. Pancreas 5, 91–94. doi: 10.1097/00006676-199001000-00013
Sillett, T. S., and Holmes, R. T. (2002). Variation in survivorship of a migratory songbird throughout its annual cycle. J. Anim. Ecol. 71, 296–308. doi: 10.1046/j.1365-2656.2002.00599.x
Starck, J. M. (1999). Phenotypic flexibility of the avian gizzard: rapid, reversible and repeated changes of organ size in response to changes in dietary fibre content. J. Exp. Biol. 202, 3171–3179.
Starck, J. M., and Rahmaan, G. H. (2003). Phenotypic flexibility of structure and function of the digestive system of Japanese quail. J. Exp. Biol. 206, 1887–1897. doi: 10.1242/jeb.00372
Starck, J. M., and Ricklefs, R. E. (1998). “Patterns of development: the altricial-precocial spectrum,” in Avian Growth and Development: Evolution Within the Altricial-precocial Spectrum, eds J. M. Starck and R. E. Ricklefs (New York, NY: Oxford University Press, 3–30.
Stein, R. W., Place, A. R., Lacourse, T., Guglielmo, C. G., and Williams, T. D. (2005). Digestive organ sizes and enzyme activities of refueling western sandpipers (Calidris mauri): contrasting effects of season and age. Physiol. Biochem. Zool. 78, 434–446. doi: 10.1086/430038
Susanna, K., Hall-Karlsson, S., and Fransson, T. (2008). How far do birds fly during one migratory stage? Ringing Migr. 24, 95–100. doi: 10.1080/03078698.2008.9674381
Tieszen, L. L., Boutton, T. W., Tesdahl, K. G., and Slade, N. A. (1983). Fractionation and turnover of stable carbon isotopes in animal tissues: implications for δ13C analysis of diet. Oecologia 57, 32–37. doi: 10.1007/BF00379558
Tomasulo, A. M., Del Lama, S. N., and Rocha, C. D. (2002). Molecular method of sexingwaterbirds without DNA extraction. Waterbirds 25, 245–248.
van Gils, J. A., Beekman, J. H., Coehoorn, P., Corporaal, E., Dekkers, T., Klaassen, M., et al. (2008). Longer guts and higher food quality increase energy intake in migratory swans. J. Anim. Ecol. 77, 1234–1241. doi: 10.1111/j.1365-2656.2008.01452.x
Van Horn, M. A., and Donovan, T. M. (1994). “Ovenbird (Seiurus aurocapilla),” in The Birds of North America Online, ed A. Poole (Ithaca: Cornell Lab of Ornithology). Retrieved from: http://bna.birds.cornell.edu/bna/species/088
Vanderhoff, E. N., and Eason, P. K. (2007). Disparity between adult and juvenile American robins Turdus migratorius foraging for ground invertebrates and cherry fruits. Ethology 113, 1212–1218. doi: 10.1111/j.1439-0310.2007.01434.x
Vanderhoff, E. N., and Eason, P. K. (2008). Comparisons between juvenile and adult American robins foraging for mulberry fruit. Wilson J. Ornithol. 120, 209–213. doi: 10.1676/07-036.1
Veiga, J. P. (1986). Settlement and fat accumulation by migrant pied flycatchers in Spain. Ringing Migr. 7, 85–98. doi: 10.1080/03078698.1986.9673885
Verspoor, J. J., Love, O. P., Rowland, E., Chin, E. H., and Williams, T. D. (2007). Sex-specific development of avian flight performance under experimentally altered rearing conditions. Behav. Ecol. 18, 967–973. doi: 10.1093/beheco/arm089
Vézina, F., Love, O. P., Lessard, M., and Williams, T. D. (2009). Shifts in metabolic demands in growing altricial nestlings illustrate context-specific relationships between basal metabolic rate and body composition. Physiol. Biochem. Zool. 82, 248–257. doi: 10.1086/597548
Warner, N. L., and Szenberg, A. (1964). The immunological function of the bursa of Fabricius in the chicken. Annu. Rev. Microbiol. 18, 253–268. doi: 10.1146/annurev.mi.18.100164.001345
Weathers, W. W., and Sullivan, K. A. (1989). Juvenile foraging proficiency, parental effort, and avian reproductive success. Ecol. Monogr. 59, 223–246. doi: 10.2307/1942600
Weathers, W. W., and Sullivan, K. A. (1991). Foraging efficiency of parent juncos and their young. Condor 93, 346–353. doi: 10.2307/1368950
Wheelwright, N. T. (1986). The diet of American robins: an analysis of U.S. biological survey records. Auk 103, 710–725.
Wheelwright, N. T., and Templeton, J. T. (2003). Development of foraging skills and the transition to independence in juvenile savannah sparrows. Condor 105, 279–287. doi: 10.1093/condor/105.2.279
Wikelski, M., Tarlow, E. M., Rain, A., Diehl, R. H., Larkin, R. P., and Visser, G. H. (2003). Costs of migration in free-living songbirds. Nature 423:704. doi: 10.1038/423704a
Wiley, R. H., and Piper, W. H. (1992). Timing of cranial pneumatization in white-throated sparrows. Condor 94, 336–343. doi: 10.2307/1369206
Williams, J. B., and Tieleman, B. I. (2000). Flexibility in basal metabolic rate and evaporative water loss among hoopoe larks exposed to different environmental temperatures. J. Exp. Biol. 203, 3153–3159.
Woodrey, M. S. (2000). Age-dependent aspects of stopover biology of passerine migrants. Stud. Avian Biol. 20, 43–52.
Woodrey, M. S., and Moore, F. R. (1997). Differences in the stopover of fall landbird migrants on the coast of Alabama. Auk 114, 695–707. doi: 10.2307/4089289
Yarbrough, C. G. (1971). The influence of distribution and ecology on the thermoregulation of small birds. Comp. Biochem. Physiol. 39A, 235–266. doi: 10.1016/0300-9629(71)90082-X
Yong, W., Finch, D. M., Moore, F. R., and Kelly, J. F. (1998). Stopover ecology and habitat use of migratory Wilson's warblers. Auk 115, 829–842. doi: 10.2307/4089502
Keywords: age, metabolic rate, body composition, digestive efficiency, migration, stopover
Citation: McCabe BJ and Guglielmo CG (2019) Migration Takes Extra Guts for Juvenile Songbirds: Energetics and Digestive Physiology During the First Journey. Front. Ecol. Evol. 7:381. doi: 10.3389/fevo.2019.00381
Received: 29 May 2019; Accepted: 23 September 2019;
Published: 16 October 2019.
Edited by:
Nathan R. Senner, University of South Carolina, United StatesReviewed by:
Scott McWilliams, University of Rhode Island, United StatesMaria Stager, University of Montana, United States
Copyright © 2019 McCabe and Guglielmo. This is an open-access article distributed under the terms of the Creative Commons Attribution License (CC BY). The use, distribution or reproduction in other forums is permitted, provided the original author(s) and the copyright owner(s) are credited and that the original publication in this journal is cited, in accordance with accepted academic practice. No use, distribution or reproduction is permitted which does not comply with these terms.
*Correspondence: Christopher G. Guglielmo, Y2d1Z2xpZTImI3gwMDA0MDt1d28uY2E=