- 1Escuela de Ciencias Biológicas, Pontificia Universidad Católica del Ecuador, Quito, Ecuador
- 2Center for Molecular Medicine, Umeå University, Umeå, Sweden
- 3Oceans Graduate School, University of Western Australia, Crawley, WA, Australia
- 4Oceans Institute, University of Western Australia, Crawley, WA, Australia
- 5School of Biological Sciences, University of Western Australia, Perth, WA, Australia
- 6Center for Ophthalmology and Visual Science, Lions Eye Institute, University of Western Australia, Perth, WA, Australia
Reptiles are a highly diverse class that consists of snakes, geckos, iguanid lizards, and chameleons among others. Given their unique phylogenetic position in relation to both birds and mammals, reptiles are interesting animal models with which to decipher the evolution of vertebrate photopigments (opsin protein plus a light-sensitive retinal chromophore) and their contribution to vision. Reptiles possess different types of retinae that are defined primarily by variations in photoreceptor morphology, which range from pure-cone to rod-dominated retinae with many species possessing duplex (rods and cones) retinae. In most cases, the type of retina is thought to reflect both the lifestyle and the behavior of the animal, which can vary between diurnal, nocturnal, or crepuscular behavioral activities. Reptiles, and in particular geckos and snakes, have been used as prime examples for the “transmutation” hypothesis proposed by Walls in the 1930s-1940s, which postulates that some reptilian species have migrated from diurnality to nocturnality, before subsequently returning to diurnal activities once again. This theory further states that these behavioral changes are reflected in subsequent changes in photoreceptor morphology and function from cones to rods, with a return to cone-like photoreceptors once again. Modern sequencing techniques have further investigated the “transmutation” hypothesis by using molecular biology to study the phototransduction cascades of rod- and cone-like photoreceptors in the reptilian retina. This review will discuss what is currently known about the evolution of opsin-based photopigments in reptiles, relating habitat to photoreceptor morphology, as well as opsin and phototransduction cascade gene expression.
Introduction
Reptiles belong to a diverse clade of amniotes, the Sauropsida that can be further separated into two subclasses, namely Lepidosauria and Archelosauria. The subclass Lepidosauria encompasses all squamate reptiles (Squamata: snakes and lizards), as well as the only living species of the order Rhynchocephalia (Sphenodon punctatus). Conversely, the subclass Archelosauria contains two extant major lineages, Testudines (turtles) and Archosauria (birds and crocodilians; Figure 1). Squamate reptiles represent by far the most numerous order of extant reptiles with over 10,000 species worldwide (Uetz et al., 2019). Squamates encompass about 95% of all known living sauropsids, and their visual systems have been studied more than any other order. As more information is available from these organisms, the visual system and specifically the visual photopigments have been studied in detail. This review will discuss what is known about the visual photopigments of squamate reptiles, but will also include some available information on testudine and crocodilian vision.
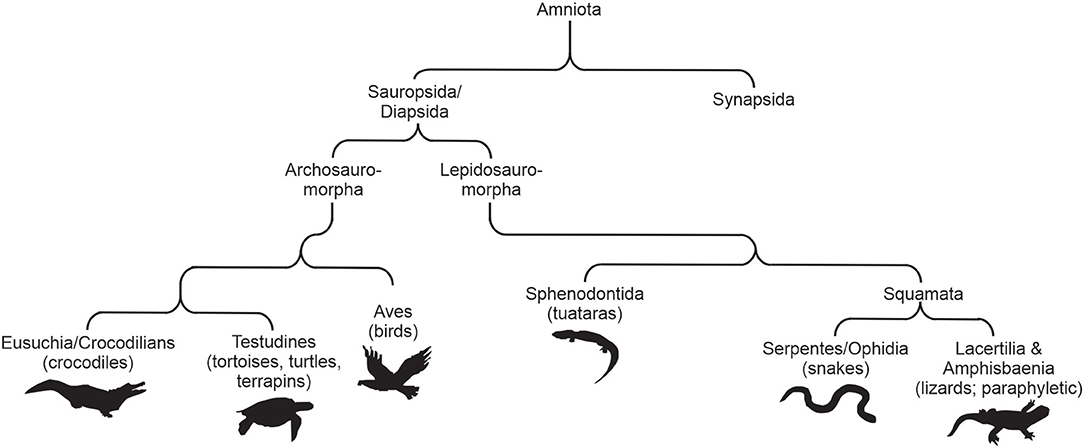
Figure 1. A basic summary cladogram showing the relationships of the amniotes. Modified from Davies et al. (2012).
In reptiles, as in all vertebrates, the general duplex retina consists of rods and cones that contain visual photopigments composed of a protein (opsin) covalently linked to a light-sensitive chromophore (Davies et al., 2012; Figure 2). The most widely utilized retinal chromophore is one derived from the aldehyde of vitamin A1 (11-cis-retinal) forming the rhodopsins, but can also be a product of the aldehyde of vitamin A2 (11-cis-3,4-didehydroretinal) that comprise the porphyropsins (Rando, 1996; Yokoyama, 2000; Menon et al., 2001; Bowmaker, 2008; Davies et al., 2012). Opsins are members of a superfamily of G-protein coupled receptors (GPCR) that permit photopic (bright light and color) and scotopic (dim-light) vision mostly within the visible light spectrum, and in many cases they can also detect ultraviolet (UV) wavelengths (Yokoyama, 2000; Bowmaker, 2008; Davies et al., 2012; Figure 2). Visual opsins are generally characterized by their gene and amino acid sequences, as well as their spectral sensitivities (often dictated by evolutionary conserved tuning sites, Figure 2).
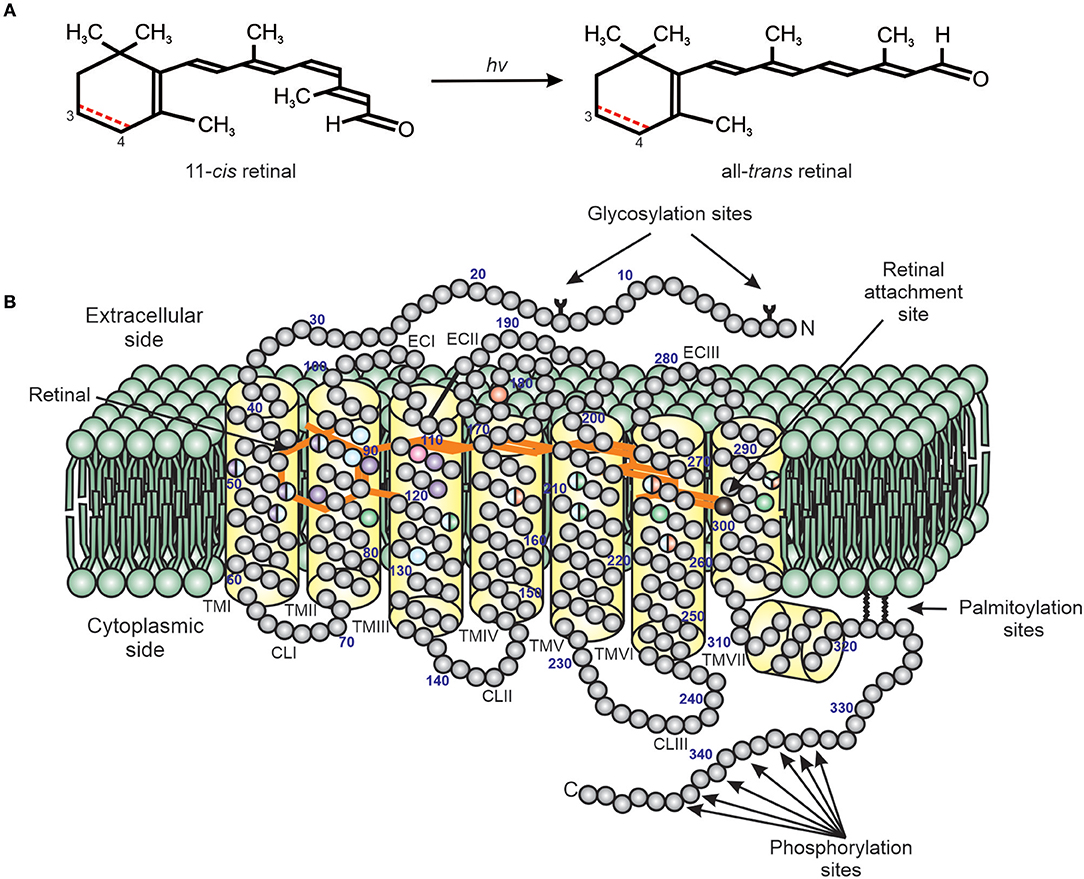
Figure 2. A diagram of the structure of a typical vertebrate photopigment (Modified from Davies et al., 2012). (A) The initial step in phototransduction consists of photon (hv) absorption by 11-cis retinal, which photoconverts to all-trans retinal. Vertebrate photopigments are broadly divided into rhodopsins that utilize a vitamin A1-derived chromophore (black line) or porphyropsins that contain a vitamin A2-derived chromophore (3,4-didehydroretinal). In the latter case, the presence of a double (C=C) bond between C3 and C4 is shown as a dotted red line. (B) Side view of photopigment (opsin and retinal chromophore), showing the presence of seven transmembrane domains (yellow), archetypal of the GPCR superfamily, and their arrangement around the retinal chromophore (orange) (modified from Davies et al., 2012). The retinal attachment site (Lys296, black) and counterion (Glu113, pink) to the Schiff base are shown. Opsin residues that cluster either around the Schiff base or ionone ring of the retinal chromophore are colored to highlight the amino acids involved in the spectral tuning of LWS (red), SWS1 (violet), SWS2 (blue), and RH2/RH1 (green) photopigments. Residues important for stabilizing the tertiary structure [e.g., disulphide bridge, amino-terminal (N) glycosylation sites] and the activation/deactivation of photopigments [e.g., carboxyl-terminal (C) phosphorylation sites], as well as membrane anchorage (e.g., palmitoylation sites), are also shown. TM, transmembrane; CL, cytoplasmic loop; EC, extracellular loop. The numbering is based on the bovine rod opsin (RH1) sequence.
Vertebrate visual opsin genes, including those of reptiles, are mainly classified into five classes, namely long-wavelength-sensitive (LWS) opsin, short-wavelength-sensitive 1 (SWS1) opsin, short-wavelength-sensitive 2 (SWS2) opsin, rhodopsin-like 2 (RH2) opsin, and rod (RH1) opsin (Yokoyama, 2000; Bowmaker, 2008; Davies et al., 2012; Figure 3). Spectrally, the opsins encoded by these genes form A1-based photopigments that show peak sensitivity to different ranges of wavelengths, for example 355–445 nm (SWS1), 400–470 nm (SWS2), 480–530 nm (RH2), 500–570 nm (LWS), and the rod opsin that is sensitive to ~500 nm (Yokoyama, 2000; Bowmaker, 2008; Davies et al., 2012).
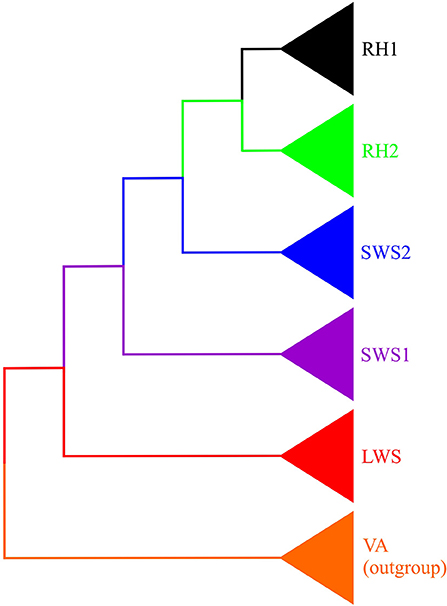
Figure 3. Phylogeny of opsin-based photopigment genes. Vertebrate ancient (VA) opsin (orange) was used as an outgroup. The diagram depicts the relationships among four cone photopigments: long-wavelength-sensitive (LWS) opsin (red), short-wavelength-sensitive 1 (SWS1) opsin (purple), short-wavelength-sensitive 2 (SWS2) opsin (blue) and rhodopsin-like 2 (RH2) opsin (green), as well as the rod (RH1) opsin (black).
Opsins are found within the membranes of photoreceptor outer segments and respond to light by changing their structural conformation. This subsequently activates a visual hyperpolarizing phototransduction cascade (Miller, 1981; Wensel, 2008; Shichida and Matsuyama, 2009; Yau and Hardie, 2009; Fain et al., 2010; Lamb, 2013) that finally reaches the visual cortex, thus transmitting external visual information to the brain (Van Hazel et al., 2006). More specifically, the first step in visual stimulation is photon capture by the photopigment, which causes the retinal chromophore to photoisomerize from its 11-cis form to its all-trans form (Wald, 1968a,b; Figure 1). Subsequent to photoisomerization, the photopigment transforms into metarhodopsin-II (Meta-II), the active form of the GPCR, which activates the G-protein transducin (encoded by the GNAT gene, GNAT1 in rods and GNAT2 in cones; Miller, 1981; Wensel, 2008; Shichida and Matsuyama, 2009; Yau and Hardie, 2009; Fain et al., 2010; Lamb, 2013). Activated transducin converts GDP into and binds GTP, with the G alpha subunit of the trimeric enzyme dissociating from the beta and gamma subunit complex. Subsequently, the alpha subunit activates phosphodiesterase 6 (PDE6) and inhibits its activity. PDE hydrolyzes cyclic GMP (cGMP), which as a result increases the GMP intracellular concentration, thus lowering the cGMP concentration and causing cGMP-gated sodium channels to close. This causes photoreceptor hyperpolarization (activation); photoreceptors are in fact the only type of neurons activated when hyperpolarized (Arshavsky et al., 2002).
Although the reptilian visual system, and in particular their visual photopigments, is a topic that has been reviewed relatively recently by Simões and Gower (2017), many studies have been published since that publication, primarily investigating the visual system of snakes (Bhattacharyya et al., 2017; Hauzman et al., 2017; Katti et al., 2018; Schott et al., 2018; Bittencourt et al., 2019; Gower et al., 2019; among others); thus shedding more light on the visual system and the activity patterns of these animals. In addition, this review forms part of a special Research (Themed) Topic (“Multiplicity of physiological systems that detect light or are regulated by photic information”).
Sauria (Lizards)
It should be noted that lizards are not grouped together as a single clade, but represent many paraphyletic groups, that are often discussed as a singular class for easy of communication; we have also chosen to discuss them as a group within this review. Many studies have shown that lizards, independent of their daily activity pattern (e.g., diurnality or nocturnality), possess retinae that vary in their photoreceptor complements from all-cone to being predominantly rod-based. Lizards are thought to have evolved from an ancestral vertebrate that is believed to have possessed the following opsin genes: four cone opsins (LWS, SWS1, SWS2, and RH2) and one rod opsin (RH1; Collin et al., 2003; Davies et al., 2007, 2012).
The most intensively studied genus is perhaps Anolis and in particular the American “chameleon” Anolis carolinensis (Provencio et al., 1992; Kawamura and Yokoyama, 1996, 1997, 1998). A. carolinensis is a diurnal lizard with the ability to detect light of a wide range of wavelengths from the ultraviolet to far red (Provencio et al., 1992; Kawamura and Yokoyama, 1996). Kawamura and Yokoyama (1996) stated that A. carolinensis has a “unique visual system,” because this species possesses a pure-cone retina and uses 11-cis-3,4-dehydroretinal (vitamin A2-based) as a chromophore (Provencio et al., 1992; Kawamura and Yokoyama, 1996). Typically, visual photopigments that utilize vitamin A2 as a chromophore absorb at longer wavelengths (Whitmore and Bowmaker, 1989) and, therefore, red-shift the spectral sensitivity of the visual system of a particular animal.
The pure-cone retina of A. carolinensis is composed of three distinct types of cones: double cones, large single cones and small single cones (Walls, 1934, 1942). An early study (Provencio et al., 1992) used microspectrophotometry (MSP) to show that these photoreceptors contain three distinct visual photopigments with different peak sensitivities, namely SWS (λmax = 365 nm), MWS (λmax = 503 nm), and LWS (λmax = 625 nm). This study, however, did not sequence the respective opsin genes. Foster et al. (1993) identified a rhodopsin-like photopigment in the pineal gland of A. carolinensis through immunocytochemical analysis, although previous studies failed to detect rod opsin or rod photoreceptors in this pure-cone retina (Yu and Fager, 1982; Fowlkes et al., 1984; Walter et al., 1986; Foster et al., 1993).
More detailed studies (Kawamura and Yokoyama, 1993, 1994, 1995, 1996, 1997) characterized the visual system of A. carolinensis and sequenced all five visual opsin genes (LWS, SWS1, SWS2, RH2, and RH1). Kawamura and Yokoyama (1998) expressed the respective opsins in vitro by regeneration and reconstitution with 11-cis-retinal, resulting in photopigments maximally sensitive to the following λmax values, 358, 437, 491, 495, 560 nm (visual photopigments) and in addition 482 nm (P-opsin or pineal opsin). Interestingly, these authors discovered the presence of the rod (RH1) opsin gene being expressed in an all-cone retina. Based on these results, A. carolinensis was one of the first lizards shown to be sensitive to UV, even though Fleishman et al. (1993) had mentioned previously that some closely related anoline species from Puerto Rico had UV vision.
It is now well-known that UV-sensitive photoreceptors are present in a variety of vertebrates (Avery et al., 1983; Hárosi and Hashimoto, 1983; Chen et al., 1984; Arnold and Neumeyer, 1987; Jacobs et al., 1991; Müller et al., 2009; Veilleux and Cummings, 2012). However, attempts in anolines to correlate visual photoreceptor sensitivity to bodily coloration (e.g., the color of their dewlap), even for UV reflectance have thus far not shown a positive correlation (Loew et al., 2002; Yewers et al., 2015). This is surprising as the color of dewlap in lizards is widely known to be used for different display purposes (Hover, 1985; Thompson and Moore, 1991a,b; Steffen and Guyer, 2014). Although no correlation has been identified between the dewlap color exhibited by anoles and their visual spectral sensitivity (Loew et al., 2002), the study was conducted in Caribbean anoles. Islands have been traditionally considered as spaces where colonizing species are more likely to prosper and diversify, as they face fewer predators/competitive species and generally do not compete for resources such as food and water (Simpson, 1953; Schluter, 2000); a prime example being the case of Darwin's finches in the Galapagos Islands, which exhibit remarkably variable morphologies (Burns et al., 2002). Conversely, anoline lizards have diversified extensively in central and northern regions of South America, and in fact more Anolis spp. exist on the mainland (197) than on the Caribbean islands (154) (Nicholson et al., 2005). Pinto et al. (2008) in an extensive study, which included a total of 92 species and examined various morphological aspects, showed that mainland and Caribbean anoles diversified morphologically with about the same rates. When anoline diversity was studied specifically taking in consideration dewlap coloration in this group, Caribbean anoles exhibited only 10% of all the possible color and pattern combinations, and most species fell within the yellow/orange color range. Thus, it is possible that in other species of anoles (e.g., on the mainland), photoreceptor sensitivity might be associated with the colors that they exhibit in their bodies.
Beside anoles, the visual systems of different gecko species, both nocturnal and diurnal, have been investigated extensively. Most of the gecko species described (72%) are active at night, making them the only primarily nocturnal major lizard clade (Gamble et al., 2015). It is known that nocturnal geckos have evolved several sensory systems to adapt to darkness, such as special auditory senses (Bergevin, 2011), reduced color vision, a multifocal optical system and vision with particularly high sensitivity (Röll, 2000). Adaptation of their acute vision in low light conditions is demonstrated by their large eyes, pupils capable of an extreme degree of constriction and dilation, retinae without foveae and rod-like cone photoreceptors, among others (Underwood, 1951; Röll, 2000). By contrast, the visual system of diurnal geckos has evolved to accommodate their diurnal activities and exhibit characteristics such as smaller round pupils, cone-like photoreceptors, smaller eyes, and UV-filtering lenses (a characteristic of many diurnal animals; Walls, 1931; Cooper and Robson, 1969a,b; Norren, 1972; Yolton et al., 1974; Chou and Cullen, 1984; Petry and Hárosi, 1990; Dillon et al., 1999; Jacobs et al., 2003; Kessel et al., 2010; Gaillard et al., 2011), among others (Underwood, 1970; Röll, 2001a; Thomas et al., 2006; Werner and Seifan, 2006; Schmitz and Higham, 2018).
It was initially thought that the nocturnal Tokay gecko (Gekko gekko) possessed two visual pigments named P521 and P467, according to their respective λmax values (Crescitelli et al., 1977; Kojima et al., 1992). These photopigments, although present in a rod-only retina (at least morphologically), were shown to have basic isoelectric points, a characteristic of cone, but not rod opsins, providing perhaps one of the first biochemical pieces of evidence supporting Walls' transmutation hypothesis that rods and cones are not fixed entities but can evolve to some degree one into the other (see Walls, 1942). Gecko photopigments are very similar to chicken cone opsins; specifically, iodopsin (a red sensitive cone pigment in the chicken retina) and green opsin, respectively, another indicator of the possible presence of cone-like photopigments in a supposed rod-only retina. Subsequently, three visual pigment genes were cloned from the Tokay gecko (G. gekko), namely LWS, SWS1, and RH2 (Kojima et al., 1992; Loew, 1994). In a more recent study, Liu et al. (2015) sequenced these same opsins from the retina of another nocturnal gecko, G. japonicus that, presumably, also has cones. During this study, rod (RH1) opsin and SWS2 cone opsin genes were also identified, but present only as non-functional pseudogenes. These results support the hypothesis that the modern gecko ancestor was a diurnal lizard lacking rod opsin (Röll, 2000) and that SWS2 photopigments were subsequently lost as an adaptation to primarily nocturnal activities (Yokoyama and Blow, 2001). However, it should be noted that, although mostly nocturnal, many species of the family Gekkonidae possess cone-like photopigments and UV-sensitive photoreceptors (Loew, 1994; Loew et al., 1996; Yokoyama and Blow, 2001), a photoreceptor class that is sometimes lost in nocturnal vertebrates (Carvalho et al., 2006, 2017).
The retina of another nocturnal gecko (Teratoscincus scincus) was examined using immunohistochemistry (Szél et al., 1986). Specifically, an antibody that detected medium- and long-wavelength opsins labeled almost all cones (single and the principal member of double cones), leaving the accessory member of double cones unstained. In turn, the accessory member was labeled by an antibody raised against bovine rod opsin (Szél and Röhlich, 1985). Electron microscopy performed within this study provided evidence that although the outer segments of these photoreceptors were superficially cone like, they had a rather cylindrical shape and separated disks that are both characteristics of the rod ultrastructure (Szél et al., 1986). This is in accordance with aforementioned findings showing that cone photopigments occur within the retina of nocturnal geckos (Loew, 1994; Loew et al., 1996; Yokoyama and Blow, 2001).
Interestingly, when the retina of the diurnal gecko Gonatodes albogularis was examined, a photopigment complement similar to the nocturnal geckos was observed, although the retina was comprised exclusively of cones (as far as morphology was concerned). The retina contained single cones, and two types of double cones. The single cone, one of the two types of double cones, as well as the principal member of the other type of double cone, contained a photopigment with a λmax value around 542 nm, whereas the accessory member of the second type of double cone contained photopigments with λmax values around 475 nm or around 362 nm (Ellingson et al., 1995). Although the λmax values of the photopigments was somewhat shifted, the general tendency of two photopigments in the visible spectrum and one within the UV range was similar to the nocturnal G. gekko (Loew, 1994; Ellingson et al., 1995).
Another genus of lizards whose visual system has been studied are dragon lizards (genus Ctenophorus). Like many other diurnal lizards, Ctenophorus sp. were initially thought to possess a pure-cone retina, without the presence of any rods or rod-elements, such as rod opsin (Walls, 1942; Underwood, 1970; Peterson, 1992; Röll, 2001a,b). When the retina of C. ornatus was examined, it was shown to comprise double and single cones that varied in size depending on their retinal location (Barbour et al., 2002). MSP in C. ornatus revealed the presence of three visual photopigments, namely an SWS photopigment (λmax 440 nm), a MWS photopigment (λmax 493 nm), and a LWS photopigment (λmax 571 nm). These photopigments are all sensitive within the visible range and no UV sensitivity was detected (Barbour et al., 2002). A more recent study that focussed on two lineages of the lizard C. decresii and compared their photopigments corroborated the results of Barbour et al. (2002), showing that their retinae were also pure-cone in nature, with photoreceptor sensitivities similar to those previously detected in C. ornatus, specifically, an SWS photopigment with a λmax value at 436 nm, a MWS photopigment with a λmax value at 495 nm and a LWS photopigment with a λmax value at 569 nm. This study, however, identified four cone opsin genes, as well as rod opsin gene expressed in the retina of C. decreii (Yewers et al., 2015). However, the authors did not offer an explanation for the discrepancy between the opsin genes expressed and the spectral sensitivities of the photoreceptors measured by MSP. Presumably, the authors may have missed a UV-sensitive cone with a λmax value around 360 nm (which is common with MSP if the number of SWS1-expressing cones are few: Davies et al., 2009), with the other cones expressing SWS2 (436 nm), RH2 (495 nm), and LWS (569 nm), respectively. In addition, it is possible that this MSP study did not distinguish between photoreceptors expressing RH2 and RH1 (around 500 nm) given their near identical spectral peaks. These results are another example where retinae from various diurnal lizards, which although morphologically do not contain rods, express rod opsin, detected either by using immunohistochemistry or by cloning the rod (RH1) opsin gene (Ellingson et al., 1995; Kawamura and Yokoyama, 1997, 1998; Bennis et al., 2005; New et al., 2012). Together, these studies support the “transmutation” hypothesis; however, investigation of the phototransduction cascade genes is required to provide conclusive evidence for the “transmutation” hypothesis given that rods and cones utilize different isoforms of similar, but functionally different, cascade proteins (Peng et al., 1992; Downes and Gautam, 1999; Nordström et al., 2004).
Ophidia (Snakes)
In comparison to lizards, far less is known about the visual systems of snakes, although in the last few years a plethora of studies have been published (Davies et al., 2009; Hauzman et al., 2014, 2017; Simões et al., 2015, 2016a,b; Schott et al., 2016; Bhattacharyya et al., 2017; Katti et al., 2018). Snakes in general terms can be separated into Scolecophidia (blindsnakes and wormsnakes), Henophidia (boas, pythons and reatives), and Caenophidia (all other snakes), and in many ways these three groups have distinct visual systems. No snake possesses more than three of the possible five opsin classes expressed in reptiles, specifically LWS, SWS1, and RH1 (Davies et al., 2009; Hauzman et al., 2014, 2017; Simões et al., 2015, 2016a,b; Schott et al., 2016; Bhattacharyya et al., 2017; Katti et al., 2018; Bittencourt et al., 2019; Gower et al., 2019). The first snake visual opsin genes to be sequenced were by Davies et al. (2009) in the henophidian royal python (Python regius) and sunbeam snake (Xenopeltis unicolor). Most snakes possess UV-sensitive (360 nm) and LWS (around 550 nm) cones, suggesting they are dichromatic (Sillman et al., 1999, 2001; Davies et al., 2009; Katti et al., 2018; Bittencourt et al., 2019; Gower et al., 2019). However, with little overlap in the spectra of these photopigments, it would be predicted that snakes are generally colorblind due to photoreceptor opponency (Michael, 1966; Hemmi et al., 2002). Nonetheless, snakes also possess an RH1-expressing photoreceptor, which under mesopic conditions (where rods and cones are active) may provide a green MWS channel (around 500 nm) that might provide “conditional trichromacy” and a form of rudimentary color vision (Davies et al., 2009). The ancestral loss of two opsin genes (namely SWS2 and RH2) has been interpreted as evidence of dim-light (possibly fossorial) origins of snakes (Davies et al., 2009, 2012; Gerkema et al., 2013), consistent also with the vastly different ocular structures of snakes in comparison to other squamates (Walls, 1940; Bellairs and Underwood, 1951; Underwood, 1970; Caprette et al., 2004).
Scolecophidia are fossorial snakes with greatly reduced eyes, typically permanently covered with scales. Their visual systems have not been studied in detail, but Simões et al. (2015) found that scolecophidians they sampled possessed only rod (RH1) opsin in their retinae whereas the two cone opsins normally present in other snakes (i.e., LWS and SWS1) were absent.
Retinal photoreceptor morphology of non-scolecophidians is quite variable. Henophidians are non-venomous, crepuscular/nocturnal and/or burrowing snakes (Conant and Collins, 1998). They are mostly nocturnal and have rod-dominated retinas (90% rods, 10% cones; Sillman et al., 1999, 2001). Sillman et al. (1999, 2001) investigated the retinae of two large predators, namely a python (P. regius) and a boa (Boa constrictor imperator), and showed that the retina of these mostly nocturnal species, consists of mostly rods but with some cones and probably the ability to visualize colors. They possess two morphologically distinct cones, one with a short and thick outer segment that was found more frequently, and a rarer type with more elongated outer segments (Sillman et al., 1999, 2001). When the photoreceptor spectral sensitivities were examined in vivo and in vitro in P. regius and B. constrictor imperator they were almost identical; the rods were sensitive to wavelengths at 494/495 nm (presumably expressing RH1), the larger abundant cones to wavelengths at 551/549 nm (presumably expressing LWS), and the smaller and rarer cones to wavelengths at 360/357 nm (presumably expressing SWS1), indicating that these snakes, in spite of their preference for a nocturnal lifestyle, possessed rod photopigments and two functional cone photopigments, one of which could detect UV light (Sillman et al., 1999, 2001; Davies et al., 2009). Davies et al. (2009) revealed that P. regius and another henophidian, X. unicolor possessed three visual opsins, rod opsin as well as two cone opsins. Microspectrophotometry and in vitro regeneration revealed that their spectral sensitivities were 497, 361, and 550 nm, respectively, which were similar to previous studies identifying another mostly nocturnal snake with a UV-sensitive cone (Sillman et al., 1999, 2001; Davies et al., 2009).
Caenophidia comprises most living snakes, including both diurnal and nocturnal species with diverse ecologies and retinae. Diurnal caenophidians generally have pure-cone retinae (Hauzman et al., 2014). Early studies revealed that the retinae of different species of the genus Thamnophis (e.g., T. sirtalis and T. marcianus) had three types of cones and lacked rod photoreceptors (Wong, 1989; Jacobs et al., 1992), and Sillman et al. (1997) verified that T. sirtalis has a pure-cone retina, which comprised of four morphologically different types of cones, namely double cones with a large principal and much smaller accessory member (45.5%), large single cones (40%) and two subtypes of small single cones (14.5%). The large single cones and the larger segment of the double cones expressed a photopigment with a maximal absorbance peak in the dark at 554 nm, which reacted with an antibody that detects MWS/LWS photopigments in vertebrates (i.e., opsin proteins encoded by the LWS gene). By contrast, the small cones contained visual photopigments with λmax values either around 482 (presumably a blue-shifted rod-like RH1 photopigment) or around 360 nm (presumably a UV-sensitive SWS1 photopigment), indicating that these are two different populations of photoreceptors. This result was further corroborated by the identification of two subsets of small cones with different immunoreactivities (Sillman et al., 1997).
Hauzman et al. (2014) investigated the retina of the colurbids Philodryas olfersii and P. patagoniensis which both have four morphologically distinct types of cones, namely double cones, large single cones, small single cones and very small single cones, with over 80% of the photoreceptor population in both species being comprised of the first two subgroups. Electron microscopy failed to detect any presence of morphological rods in these animals. This study also used immunohistochemistry to demonstrate the presence of LWS photopigments in the double and large single cones and SWS1 photopigments in a subpopulation of the small single cones (Hauzman et al., 2014). The lack of labeling in some small single cones and in the very small single cones indicated the presence of a different visual photopigment in addition to LWS and SWS1 cones in the retinae of these caenophidians; however, immunoreactivity to RH1 photopigments was not tested.
The presence of RH1 expression, which encodes the visual opsin that was not detected by immunohistochemistry in the study by Hauzman et al. (2014), in cone-only colubrids (e.g., Pseustes poecilonotus and Atractus flammigerus), was confirmed by Simões et al. (2015). Simões et al. (2016b) sequenced visual opsin genes from caenophidians with retinae that varied from being all-cone (with putative transmutated rods) to “all-rod” (with putative transmutated cones) and various stages in between (retinae with both rods and cones and those with photoreceptors that were morphologically intermediate; Simões et al., 2016b). Three opsin genes (LWS, SWS1, and RH1) were detected in all species examined, supporting the transmutation hypothesis proposed by Walls for caenophidians (Walls, 1942).
Schott et al. (2016) examined T. proximus (Western ribbon snake) in further detail. Electron microscopy revealed the presence of four morphologically distinct cone types as observed in previous studies of T. sirtalis (the common garter snake), but that a subset of the small cones possessed an ultrastructure that resembled rods. Specifically, these cells exhibited inner and outer segment widths that were similar, as well as outer segment discs that were completely enclosed by plasma membrane (Schott et al., 2016). This study also cloned the opsin genes expressed in the retina of T. proximus, revealing the presence of LWS and SWS1 cone opsins, as well as RH1 (Schott et al., 2016). In terms of spectral sensitivity, opsin-based photopigments in T. proximus were similar to homologous photopigments found in other Thamnophis sp. examined thus far (Sillman et al., 1997). Schott et al. (2016) showed using MPS that the RH1 opsin was not only present in a subset of cones, but that it was actually functional (Schott et al., 2016). Given the presence of a functional rod opsin in an “all-cone” retina, this study was one of the first to provide functional evidence of potential transmutation in colubrid snakes, thus further corroborating Walls' transmutation hypothesis (Walls, 1942).
Recent studies on snake vision have further focused on the visual systems of caenophidians and more specifically colubrid snakes (Bhattacharyya et al., 2017; Hauzman et al., 2017). Hauzman et al. (2017) demonstrated that nocturnal and diurnal colubrids exhibited differences in their retinal photoreceptors. Nocturnal snakes had canonical duplex retinae with both rod and cone photoreceptors, with two types of cones (expressing LWS or SWS1 opsin genes). Conversely, diurnal species possessed pure-cone retinae, in which one of the cone types was a transmuted rod, as shown by immunoreactivity with antibodies raised against rod opsin (Hauzman et al., 2017). As far as the influence of daily activity patterns on the opsin genes was concerned, these effects seem to be more pronounced for both the RH1 and SWS1 opsin genes. Hauzman et al. (2017) showed that there was a higher evolutionary pressure to maintain short-wavelength sensitivity specifically in nocturnal snakes, whereas there was stronger pressure to maintaining rod opsin in diurnal animals. Furthermore, both of these evolutionary adaptations are probably present to maximize color vision during the day in both diurnal and nocturnal animals.
Bhattacharyya et al. (2017) investigated the all-cone retina of the colubrid pine snake (Pituophis melanoleucus) and detected the presence of a cone-like rod opsin in some of their cones. The spectral peak of absorbance of this rod (RH1) opsin photopigment was significantly blue-shifted to ~480 nm, similar to the RH1 photopigment identified in T. proximus by Schott et al. (2016). However, the spectral tuning mechanisms to account for the shift to shorter wavelengths were not investigated. Specifically, the spectral peak of RH1 photopigments in these snake species were deemed to be closer to the spectral sensitivity of some RH2 photopigments, an opsin that is lost in all snakes investigated so far (Hauzman et al., 2014, 2017; Simões et al., 2015, 2016a,b; Schott et al., 2016; Bhattacharyya et al., 2017; Katti et al., 2018). As such, it might be hypothesized that RH1 in these species has been spectrally tuned to functionally overlap the loss of RH2. Indeed, given the diurnality of these animals, replacing the lost RH2 photopigment could be functionally advantageous. That is to say that a rod opsin encoded by RH1 that can act similarly to an RH2 cone opsin photopigment may permit a higher level of visual sensitivity with mutual spectral overlaps with both LWS and SWS1 photopigments. Thus, these snakes exhibit the potential for trichromatic vision, which would clearly be advantageous for a diurnal animal. The results of these studies open the possibility of the existence of trichromacy in other diurnal colubrids, where functional rod opsin photopigments are present in transmuted cones as a third opsin that could be utilized under photopic conditions (Bhattacharyya et al., 2017; Hauzman et al., 2017). However, trichromacy in these species has yet to be confirmed with electrophysiological or behavioral experiments.
The visual system of some hydrophid elapids has also been examined in detail. Hart et al. (2012) used light microscopy and electron microscopy to investigate the visual system of two species of sea snakes, Lapemis curtus and Acalyptophis peronii, both having three types of single cones, one type of double cone and no discernible rods. MSP was used to identify three visual photopigments with spectral peaks at 428–430 nm (short-wavelength-sensitive), 496 nm (medium-wavelength-sensitive), and 555–559 nm (long-wavelength-sensitive), presumably via photoreceptors expressing SWS1, RH1, and LWS, respectively. These genes were sequenced later by Simões et al. (2016b), confirming the presence of these three opsins in hydrophid snakes, and confirming that the medium-sensitive single “cones” detected by Hart et al. (2012) are transmuted rods.
The visual system of viperid caenophidians has been investigated in detail in a few recent studies (Simões et al., 2016a; Katti et al., 2018; Bittencourt et al., 2019; Gower et al., 2019). Simões et al. (2016a) obtained partial sequences of visual opsin genes expressed in three African vipers (Echis ocellatus, Causus rhombeatus, and Bitis nasicornis), Katti et al. (2018) and Bittencourt et al. (2019) generated homologous sequences from South American vipers (Bothrops atrox, Bothrops jararaca, and Crotalus durissus terrificus), and Gower et al. (2019) sampled vipers from different continents including some of those previously studied (i.e., Azemiops kharini, Bothriechis schlegelii, Trimeresurus trigonocephalus, Vipera berus, Agkistrodon contortrix, Bitis arietans, Cerastes cerastes, Crotalus durissus, Echis coloratus, and Crotalus atrox). All of these investigations revealed that vipers follow the same pattern of opsin expression as observed in other snakes by expressing LWS, SWS1, and RH1 in the retina.
Regarding photopigment spectral sensitivities, all the predicted and experimentally demonstrated (by MSP) values for LWS-expressing cones were close to 553–555 nm (Simões et al., 2016a; Katti et al., 2018; Bittencourt et al., 2019; Gower et al., 2019). Interestingly, Gower et al. (2019) measured a spectral peak near 554 nm for the LWS photopigment for one viperine and one crotaline (A. contortrix, B. arietans), whereas this photopigment was blue-shifted to 541 nm for a different crotaline, C. atrox; however the tuning mechanisms were not investigated in detail. The predicted and measured spectral maxima for the RH1 photopigments were close to 495–500 nm, depending principally on one amino acid substitution, where aspartic acid was changed to asparagine at position 83 of RH1, a site that is often changed in the spectral tuning of RH1 photopigments (Yokoyama, 2000; Bowmaker, 2008; Davies et al., 2012). This change was also present in all African vipers sampled by Simões et al. (2016a), as well as in some vipers investigated by Gower et al. (2019). Conversely, SWS1 photopigments were predicted to be exclusively UV-sensitive (Katti et al., 2018; Bittencourt et al., 2019; Gower et al., 2019); however, when maximal sensitivity values of SWS clones were measured (Gower et al., 2019), only two vipers fell within the UV range (B. arietans, C. atrox). Surprisingly, the SWS cone of one crotaline (A. contortrix) was sensitive within the visible range; more specifically its maximal absorbance was 416 nm (Gower et al., 2019), with the spectral tuning mechanism remaining to be clarified. Given these photopigment spectral sensitivities, and in particular the non-blue shifted rod photopigment, it may be concluded that the photopigments of most vipers are more similar to henophidians than to more diurnal caenophidians, as well as other nocturnal vertebrates (Davies et al., 2009; Veilleux and Cummings, 2012; Joesch and Meister, 2016).
In addition to spectral sensitivities, two of these studies (Bittencourt et al., 2019; Gower et al., 2019) investigated photoreceptor morphology and immunolabeled different photoreceptors. All vipers examined possessed rod-dominated retinae, with the presence of three different types of cones, namely small single cones, large single cones and double cones. All single cones presented specific immunoreactivities, i.e., small single cones were immunoreactive to antibodies raised against SWS1 and large single cones were immunoreactive to antibodies detecting LWS. However, a discrepancy was observed between the two studies regarding the double cones. Gower et al. (2019) detected two types of double cones with regards to their immunoreactivity: one type contained LWS photopigments in both the principal and accessory members and a different double cone (not as common) contained SWS1 photopigments in both members. By contrast, in the study by Bittencourt et al. (2019), only the first type of double cones was detected (immunoreactive to LWS photopigments). According to Gower et al. (2019) the SWS1-expressing double cones were rarer than those expressing the LWS opsin gene, something that could have contributed to their non-detection by Bittencourt et al. (2019). Nonetheless, these two studies employed different antibodies for opsin detection, which might be another factor that could contribute to this variation.
Thus far, most of the molecular genetic studies concerning the snake visual system have dealt primarily with visual opsins. However, within the last few years, and especially with the development of next-generation sequencing (NGS) techniques that produce a vast amount of data, a few snake genomes and transcriptomes have been sequenced. Together, these have permitted a broader investigation of both visual and non-visual opsins, as well as phototransduction genes (Castoe et al., 2013; Vonk et al., 2013; Emerling, 2017a; Perry et al., 2018; Schott et al., 2018). Castoe et al. (2013) investigated opsins in the genome of the python P. molurus bivittatus and the king cobra Ophiophagus hannah [previously sequenced by Vonk et al. (2013)], Emerling (2017a) investigated opsins using bioinformatics in P. bivittatus and seven colubroids (Crotalus mitchellii, Crotalus horridus, Protobothrops mucrosquamatus, Vipera berus, Ophiophagus hannah, Thamnophis sirtalis, and Pantherophis guttatus), whereas Perry et al. (2018) investigated opsin expression in the retina of T. sirtalis. These animals maintained the visual opsin complement observed so far in all snakes, excluding the blindsnakes, with the presence of LWS, SWS1, and RH1 opsin genes and absence of SWS2 and RH2 opsin genes. However, these datasets were also able to provide additional information concerning non-visual opsins present in the snake genome and expressed in their retinae. Typically, all the snakes examined have melanopsin (specifically the Xenopus-like melanopsin (OPN4X) form), peropsin, vertebrate ancient (VA) opsin, encephalopsin/OPN3, neuropsin 1, neuropsin 4, neuropsin 5, among others, but lack mammalian-like melanopsin (OPN4M), teleost multiple tissue (TMT) opsins 1-3 (TMT1, 2, and 3), parapinopsin, parietopsin, and others (Castoe et al., 2013; Emerling, 2017a; Perry et al., 2018). The fact that the presence or absence of these genes is consistent across relatively distant snake families may be an indication that most snakes (or perhaps all reptiles—see further details in the crocodilian section below) might exhibit the same opsin repertoire; however more studies are necessary to confirm this conjecture, especially to generalize for reptiles. It should also be noted that most of these studies examined snake genomes. Thus, it would be of interest to examine multiple transcriptomes derived from multiple snake tissues (especially the retina) to assess if these genes are actually expressed and, therefore, could be functional in the appropriate tissues under investigation. Nevertheless, simply the presence of these non-visual opsins in snakes reveals that these animals most likely have both visual (image-forming) and non-visual (for non-image-forming tasks, such as the maintenance of circadian rhythms) functions (Castoe et al., 2013; Davies et al., 2014; Hankins et al., 2014; Emerling, 2017a; Perry et al., 2018).
Schott et al. (2018) used transcriptomic analyses to investigate evolutionary selection pressures on various phototransduction genes from seven colubrids. Once again, these analyses confirmed the loss of the two opsin genes (SWS2 and RH2) in caenophidians observed by other groups (Hauzman et al., 2014, 2017; Simões et al., 2015, 2016a,b; Schott et al., 2016; Bhattacharyya et al., 2017; Katti et al., 2018; Bittencourt et al., 2019; Gower et al., 2019); however, this study also showed a loss of the rod opsin-specific kinase GRK1, selectively in Caenophidia, as well as significant and long-term shifts in most of the cone-specific phototransduction genes examined (Schott et al., 2018). Given these results it is possible that visual phototransduction genes expressed in the caenophidian retina have experienced positive selection throughout evolution, but this selection was limited to cone-specific genes, probably as an adaptation of these all-rod nocturnal animals so that they could function in dimly lit environments. These adaptations may be indicative of many evolutionary transitions from diurnality to nocturnality (or cone to rod changes) and fewer transitions (if any) from nocturnality to diurnality (or rod to cone changes); however many more extensive studies are required to resolve this matter (Schott et al., 2018), especially considering that fewer changes may be expected in rod-specific genes simply because rods already tend to function close to their biophysical limits (Gozem et al., 2012).
Non-Squamate Reptiles
Although the squamate reptiles (lizards and snakes) encompass about 95% of all known reptile species, the class reptilia includes other orders such as testudines, crocodilians, and rhynocephalians (which only contains the tuatara). While squamate vision has been extensively studied, the visual system of other reptiles, such as crocodilians and testudines, has also been investigated and is reviewed briefly here.
Eusuchia (Crocodilians)
The crocodilian visual system has been studied to some degree, but not nearly to the level of detail of the squamates, an outcome that may be related to the difference in the number of extant species that are easily attainable for study (10,221 squamates, 24 crocodilians, Uetz et al., 2019). Thus far, information regarding the visual photopigments of five species of crocodilians has been collected (Dartnall and Lythgoe, 1965; Govardovskii et al., 1988; Sillman et al., 1991; Nagloo et al., 2016).
The retina of Alligator mississippiensis is perhaps the best-studied among crocodilians; it is a duplex retina that contains rods and single and double cones. Although rods dominate the retina, cones can reach 28% (Laurens and Detwiler, 1921; Walls, 1942; Sillman et al., 1991). Sillman et al. (1991) identified five different visual photopigments using MSP. Specifically, they detected a single rod opsin photopigment with a maximal absorbance at 501 nm and four cone opsins: single cones expressing visual photopigments with λmax values either around 444 nm (perhaps a violet-sensitive SWS1 or an SWS2 opsin) or around 535 nm (perhaps an RH2 opsin), and double cones where two photopigments were present, one with a λmax at 566 nm (presumably an LWS opsin) within the principal member and one with a λmax at 503 nm within the accessory member, which may be a greatly red-shifted SWS2 opsin or a rod opsin expressed in a cone, similar to that observed in some pure-cone snakes. Further studies are required to assign the opsin subclass to each cone subtype.
A brief description of extracted visual photopigments of the Nile crocodile Crocodylus niloticus by Dartnall and Lythgoe (1965) showed that these animals possess at least two visual photopigments, one maximally sensitive at 507 nm and another at 527 nm. This study did not examine the visual system in more detail, so the underlying opsins were unclear.
The visual system of the spectacled caiman (Caiman crocodilus), a species that can inhabit both saltwater and freshwater environments, has also been studied. Govardovskii et al. (1988) revealed that this crocodilian possessed two types of single cones sensitive within the blue (430 nm) and red (535 nm) ranges, as well as a double cone (with peak spectral sensitivities at 535 nm for the principal and 506 nm for the accessory member) and a rod with a peak absorbance at 506 nm. Once again the opsins expressed in these photoreceptors require elucidation.
Most recently Nagloo et al. (2016) described and compared the visual systems of two Australian crocodiles, Crocodylus porosus (a saltwater crocodile) and C. johnstoni (a freshwater crocodile). The retinae of both species possessed three different types of single cones (deemed to be distinct photoreceptors based on their size and spectral sensitivity), as well as a double cone and a rod. Spectral sensitivities of these were similar to other species previously studied. Rods were sensitive to green wavelengths (503/510 nm), and the three single cones were sensitive to violet (424/426 nm), green (502/510 nm), and red (546/554 nm) wavelengths, respectively for the salt- and freshwater species. The spectral sensitivity of both members of the double cone was identical to the red single cone (i.e., 546/554 nm). All visual photopigments presented with higher λmax values for the freshwater crocodile in comparison with the saltwater crocodile, something that was expected given that freshwater is more abundant in longer wavelengths (Jerlov, 1976; Kirk, 1980; Chen et al., 2010). It is possible that spectral tuning by amino acid substitutions accounted for the red-shift in the photopigments of the freshwater crocodile, but it is more likely that these photopigments are utilizing a vitamin A2-based chromophore (i.e., they are porphyropsins or a mixture of porphyropsins and rhodopsins) as is common in freshwater species, such as many bony fishes (Bridges and Yoshikami, 1970a,b; Hart et al., 2008; Toyama et al., 2008). The aforementioned photopigment sensitivities provide the potential for the existence of color vision in these animals and the differences in spectral sensitivities between the two species clearly demonstrate the plasticity of the visual system, which has the ability to adapt over time to specific visual tasks (e.g., adaptation to specific habitats).
The photoreceptor sensitivities of all crocodilians studied so far are relatively similar, with some species having greater ranges than others. Probably the most detailed study within this group is also the more recent one and, therefore, care must be given when interpreting previous results as methods have generally become more sophisticated over the years. For example, it would be of interest to reexamine some of these previously studied species (e.g., the Nile crocodile investigated by Dartnall and Lythgoe, 1965). It is also important to note that all of the crocodilian species studied so far, with the exception of C. posorus, are freshwater or predominantly freshwater species. In the study of Australian crocodilians, their photoreceptor spectral sensitivity was compared between two different habitats and it was evident that although the sensitivities were relatively similar, the freshwater species almost invariably exhibited greater λmax values for each type of photoreceptor, probably as an adaptation to different aquatic environments.
Another aspect worth noting is that the visual systems of crocodilians are not sensitive to UV illumination. This could be simply an effect of insufficient sampling from a variety of species, but it is most likely an adaptation to their aquatic habitats. Crocodilians are mostly nocturnal and spend much of their time ambushing their prey by being mostly covered by water and only revealing their eyes and snout. Although they exhibit various social behaviors, such as inflated posturing and snout lifting during courtship and mating (Garrick and Lang, 1977), these behaviors can be grossly detected, without the need for particular visual system adaptations. Additionally, unlike lizards which do possess photoreceptors which can detect UV light, no evidence indicates that crocodiles reflect UV wavelengths on their bodies, a mechanism that lizards frequently use to demonstrate dominance or sexual prowess to predators and potential mates, respectively (Fleishman et al., 2011).
Recently Emerling (2017b) used available crocodilian genomes (Wan et al., 2013; Green et al., 2014) to investigate the presence of opsin genes in Alligator mississippiensis, Alligator sinensis, Crocodylus porosus, and Gavialis gangeticus. All four species retained two functional cone opsin genes (LWS and SWS2), whereas all lacked SWS1. The RH2 gene was present albeit fragmentary, thus indicating that this opsin gene had probably recently been converted to a pseudogene in the eusuchian ancestor (Emerling, 2017b). RH1, conversely, was intact in all species with the exception of A. sinensis where RH1 presented with a deletion predicted to not alter its function and is possibly a rather recent genetic event. Given the rarity of RH1 deletion across various reptiles and other vertebrates (Yokoyama, 2000; Bowmaker, 2008; Davies et al., 2012; Niemiller et al., 2013; Liu et al., 2015; Simões et al., 2016b) it would be of great interest to further investigate this finding and determine the functional consequences of this deletion (Emerling, 2017b).
Emerling (2017b) investigated non-visual as well as visual opsin genes in these crocodilians and found that mammal-like melanopsin (OPN4M), neuropsin-like 2, parapinopsin, parietopsin, and pinopsin were either not present or inactivated in all crocodilians examined, similar to the snake species examined in previous studies (Castoe et al., 2013; Emerling, 2017a; Perry et al., 2018). Unlike squamate reptiles, crocodilians have lost the parietal eye (where at least some of these opsins such as parietopsin and parapinopsin are expressed) and this may have contributed to the eventual loss of some of the aforementioned opsins (Emerling, 2017b).
Testudines (Turtles)
The visual system of turtles has also been studied and in fact most of these studies were conducted in the 1980s (Ohtsuka, 1985a,b; Gaur et al., 1988; Ohtsuka and Kawamata, 1990). Indeed, some of the turtle photoreceptor photopigments were among the first to be examined by MSP (Liebman and Granda, 1971; Liebman, 1972; Lipetz and MacNichol, 1982, 1983, 1990; Lipetz, 1985). Also, the turtle retina was extensively used to study the neural mechanisms of chromatic processing (Wheeler and Naka, 1977; Ventura et al., 2001).
In addition to a rod photoreceptor with a spectral peak at 520 nm [identified in Pseudemys scripta elegans by Baylor and Hodgkin (1973)], three cone photopigments were initially identified by Liebman and Granda (1971), Baylor and Hodgkin (1973), and Ohtsuka (1978, 1985a,b) in the retinae of Chelonia mydas, Geoclemys reevesii, and Pseudemys scripta elegans, which probably corresponded to the expression of the SWS2 (460 nm), RH2 (540–550 nm), and LWS (620–630 nm) opsin genes given the spectral maxima observed. Subsequently, Loew and Govardovskii (2001), again using MSP, identified a photoreceptor with a different sensitivity (around 372 nm) in the retina of T. scripta elegans, indicating the presence of an additional type of photoreceptor that was sensitive to short-wavelengths and most definitely contained SWS1 photopigments.
The turtle retina, specifically the retinae of T. reevesii and T. scripta elegans, were examined using intracellular recordings in which the cells were filled with Lucifer Yellow. Both retinae contained different types of rod and cone photoreceptors (Ohtsuka, 1985a,b), namely single rods, as well as blue-, green-, and red-sensitive cones that could be morphologically distinguished by the presence of differentially colored oil droplets. The four types of single cones contained red, pale green, orange, or clear oil droplets and the double cones contained a yellow oil droplet within the principal member, but did not contain an oil droplet in the accessory member (Ohtsuka, 1985a,b). The double cones, as well as the single cones containing a red or a pale green oil droplet were red-sensitive (620 nm), whereas the single cones containing an orange or clear oil droplet were green- and blue-sensitive, with spectral maxima at 540 and 460 nm, respectively (Ohtsuka, 1985a,b). UV cones were presented as photoreceptors with transparent non-fluorescent oil droplets (Kolb and Jones, 1987; Goede and Kolb, 1994).
Further analyses of the turtle retina by immunohistochemistry using an antibody raised against bovine rod opsin showed differential immunoreactivities of the turtle photoreceptors (Gaur et al., 1988; Ohtsuka and Kawamata, 1990). Interestingly, this antibody labeled rod and some cone outer segments in different turtle retinas (i.e., Geoclemys reevesii, Trachemys scripta and P. scripta elegans). Specifically, rods in addition to some red-sensitive cones were intensely labeled, green- and blue-sensitive cones were labeled less intensely, and double cones and red-sensitive cones containing a pale green oil droplet were not labeled at all (Ohtsuka and Kawamata, 1990). This result indicated a certain degree of antibody cross-reactivity with different opsin classes (i.e., rods, as well as red-, green-, and blue-sensitive cones), which suggests that the epitope that the antibody recognized was fairly conserved across the different opsins subclasses present in the turtle retinae being examined. Nonetheless, these results indicated the presence of two types of red-sensitive cones, one that was immunoreactive to this antibody and another that is not (Ohtsuka and Kawamata, 1990).
Until 1997, there were no studies that demonstrated the presence of a UV-sensitive photoreceptor (S-cone) in the turtle retina besides some pioneering studies using behavioral training techniques that suggested this photoreceptor was present in the turtle retina (Arnold and Neumeyer, 1987; Ventura et al., 2001). Ventura et al. (2001) was finally able to stimulate the S-cone photoreceptor, which provided conclusive evidence that turtles express functional UV-sensitive photopigments in their retinae, thus providing them with the potential for tetrachromatic vision.
Most recently, Emerling (2017b) investigated the opsin genes from four turtles (Chrysemys picta, Chelonia mydas, Pelodiscus sinensis, and Apalone spinifera), using genomes available as previously discussed above (Wang et al., 2013; Badenhorst et al., 2015). All four species possess at least LWS and RH2 cone opsin genes, as well as a rod (RH1) opsin gene (Emerling, 2017b). Conversely, C. picta and C. mydas possess all five opsin classes believed to have been present in the ancestral vertebrate (e.g., the Agnathans, Collin et al., 2003; Davies et al., 2007). The remaining two species (A. spinifera and P. sinensis) were shown to have lost the SWS1 opsin gene, whereas P. sinensis did not appear to have a functional SWS2 photopigment, as the respective SWS2 gene was rather unusual and may be a pseudogene (Emerling, 2017b). Emerling (2017b) also investigated non-visual opsins in the testudines and showed that parapinopsin and parietopsin were either not present or inactivated in all four testudines examined, possibly because of the loss of the parietal eye in this order (Emerling, 2017b).
The Transmutation Hypothesis
Walls presented his transmutation hypothesis in the 1940s to explain the rod-like morphology of some rods present in reptiles (Walls, 1942) based on evidence from snakes and nocturnal lizards. This proposed that early tetrapods began life by exhibiting nocturnal behaviors, then shifted their activity patterns to being more diurnal in nature. This shift probably occurred so that these species could improve their metabolic activities and in general their well-being by taking advantage of the sun. Due to their exposure to bright light, these animals eventually lost their rod functions. However, because of complex predator-prey relationships, some of these animals returned to a nocturnal lifestyle to increase their chances of survival. These species had to adapt to their new nocturnal environment and as a result their cones transmutated to photoreceptor cells with rod-like characteristics, such as increased sensitivity to detect low light levels (Walls, 1942).
After more than 70 years of research in this field, it has been shown that retinae of some lizards (geckos; Walls, 1940; Kojima et al., 1992; Taniguchi et al., 1999; Yokoyama and Blow, 2001) and many caenophidian snakes fit this hypothesis well (Simões et al., 2015, 2016b; Schott et al., 2016; Bhattacharyya et al., 2017; Hauzman et al., 2017). Although the examination of geckos within the context of the transmutation hypothesis were conducted many years ago (Kojima et al., 1992; Taniguchi et al., 1999; Yokoyama and Blow, 2001), within the last 5 years various studies have been published looking at the snake visual system with regards to Walls' hypothesis (Simões et al., 2015, 2016b; Schott et al., 2016; Bhattacharyya et al., 2017; Hauzman et al., 2017). All of these studies have presented strong evidence that at least the colubroids support the transmutation hypothesis, as shown by the presence of photoreceptors with intermediate morphologies as well as opsins expressed in photoreceptors in a non-canonical manner (Simões et al., 2015, 2016b; Schott et al., 2016; Bhattacharyya et al., 2017; Hauzman et al., 2017).
The study of snake vision in relation to the transmutation hypothesis has advanced significantly in the last few years, and thorough studies have specifically been designed to address the accuracy of the transmutation hypothesis (Schott et al., 2016). However, these snake-based studies have focused exclusively on the colubroids. Admittedly, Colubroidea is the largest superfamily of snakes, consisting of >2,500 extant species and encompassing animals with highly variable retinal morphology and daily activity patterns (i.e., diurnality vs. nocturnality; Pyron and Wiens, 2011; Schott et al., 2016; Hauzman et al., 2017). However, more work is needed to advance the field and provide conclusive evidence that confirms the transmutation hypothesis in non-colubrid squamates and non-squamate reptiles in general.
Concluding Remarks
This review summarizes the existing bibliography regarding certain aspects of the the visual system of extant reptiles, such as photopigment complement and photoreceptor morphology (where available) for each species examined. Lizards generally express five visual photopigments, as were present in the ancestral vertebrate, whereas snakes have lost two visual photopigment classes presumably due to their dim-light (possibly fossorial) origins. Despite being neglected for sometime, snakes in particular have been the focus of numerous recent studies, especially with regards to Walls' transmutation hypothesis. This conjecture states that animals began exhibiting nocturnal behaviors, then changed to a diurnal daily activity lifestyle before subsequently returning to nocturnality, and that to accommodate these necessities/behaviors, the photoreceptors exhibit cellular plasticity where one photoreceptor can evolve into the other if necessary. First formulated in the 1940s, geckos were the first animals that were thought to fit this hypothesis.
The review also makes reference to the visual system of other (non-squamate) reptiles, such as the crocodilians and the testudines. Much less is known about their visual systems, but as with snakes, further studies have emerged during the past few years. Crocodilians possess three visual opsin classes, albeit not the same as retained in snakes, whereas some turtles maintain all five ancestral vertebrate visual photopigments (four cone types and a single rod type) providing them with the potential for tetrachromacy.
Finally, although the main focus of this review is the study of visual photopigments and their adaptation to different habitats, some work on non-visual photopigments is also summarized. This work refers to predominantly non-squamate reptiles that lack non-visual opsin classes such as parapinopsin, parietopsin, among others, which may possibly be the result of the loss of the parietal eye.
As far as their photoreceptor morphology is concerned, reptiles possess retinae that can vary from being all-cone to one that is rod-dominated. This makes it clear that the visual system exhibits notable evolutionary plasticity and an ability to adapt to the particular needs of a species, such as habitats or daily activity patterns.
Although this is a topic that has been reviewed relatively recently (Simões and Gower, 2017), many scientific articles have been published within the past 2 years, especially regarding the visual system of snakes and other non-squamate reptiles. Therefore, this more up-to-date review is provided to synthesize these latest studies within a wider context of reptilian visual photobiology.
Author Contributions
CK and WD have written the manuscript. MS-S and NC-R have helped summarizing some of the articles reviewed in this manuscript.
Funding
This work was supported by a fellowship awarded to CK by Proyecto Prometeo of the Secretaría de Educación Superior, Ciencia, Tecnología e Innovación del Ecuador (SENESCYT), research grants from Pontificia Universidad Católica del Ecuador (L13359 and N13433) awarded to CK, as well as the Australian Research Council (ARC) via a Future Fellowship (FT110100176) and a Discovery Project grant (DP140102117) awarded to WD.
Conflict of Interest
The authors declare that the research was conducted in the absence of any commercial or financial relationships that could be construed as a potential conflict of interest.
References
Arnold, K., and Neumeyer, C. (1987). Wavelength discrimination in the turtle Pseudemys scripta elegans. Vision Res. 27, 1501–1511. doi: 10.1016/0042-6989(87)90159-3
Arshavsky, V. Y., Lamb, T. D., and Pugh, E. N. (2002). G proteins and phototransduction. Annu. Rev. Physiol. 64, 153–187. doi: 10.1146/annurev.physiol.64.082701.102229
Avery, J. A., Bowmaker, J. K., Djamgoz, M. E. A., and Downing, J. E. G. (1983). Ultra-violet sensitive receptors in a freshwater fish. J. Physiol. 334:23. doi: 10.1016/0042-6989(87)90124-6
Badenhorst, D., Hillier, L. D. W., Literman, R., Montiel, E. E., Radhakrishnan, S., Shen, Y., et al. (2015). Physical mapping and refinement of the painted turtle genome (Chrysemys, picta) inform amniote genome evolution and challenge turtle-bird chromosomal conservation. Genome Biol. Evol. 7, 2038–2050. doi: 10.1093/gbe/evv119
Barbour, H. R., Archer, M. A., Hart, N. S., Thomas, N., Dunlop, S. A., Beazley, L. D., et al. (2002). Retinal characteristics of the ornate dragon lizard, Ctenophorus ornatus. J. Comp. Neurol. 450, 334–344. doi: 10.1002/cne.10308
Baylor, D. A., and Hodgkin, A. L. (1973). Detection and resolution of visual stimuli by turtle photoreceptors. J. Physiol. 234, 163–198. doi: 10.1113/jphysiol.1973.sp010340
Bellairs, A. D., and Underwood, G. (1951). The origin of snakes. Biol. Rev. Camb. Philos. Soc. 26, 193–237. doi: 10.1111/j.1469-185X.1951.tb00646.x
Bennis, M., Molday, R. S., Versaux-Botteri, C., Repérant, J., Jeanny, J. C., and McDevitt, D. S. (2005). Rhodopsin-like immunoreactivity in the “all cone” retina of the chameleon (Chameleo chameleo). Exp. Eye Res. 80, 623–627. doi: 10.1016/j.exer.2004.11.004
Bergevin, C. (2011). Comparison of otoacoustic emissions within gecko subfamilies: morphological implications for auditory function in lizards. J. Assoc. Res. Otolaryngol. 12, 203–217. doi: 10.1007/s10162-010-0253-0
Bhattacharyya, N., Darren, B., Schott, R. K., Tropepe, V., and Chang, B. S. W. (2017). Cone-like rhodopsin expressed in the all-cone retina of the colubrid pine snake as a potential adaptation to diurnality. J. Exp. Biol. 220, 2418–2425. doi: 10.1242/jeb.156430
Bittencourt, G. B., Hauzman, E., Bonci, D. M. O., and Ventura, D. F. (2019). Photoreceptors morphology and genetics of the visual pigments of Bothrops jararaca and Crotalus durissus terrificus (Serpentes, Viperidae). Vision Res. 158, 72–77. doi: 10.1016/j.visres.2019.02.006
Bowmaker, J. K. (2008). Evolution of vertebrate visual pigments. Vision Res. 48, 2022–2041. doi: 10.1016/j.visres.2008.03.025
Bridges, C. D., and Yoshikami, S. (1970a). The rhodopsin-porphyropsin system in freshwater fishes−2. Turnover and interconversion of visual pigment prosthetic groups in light and darkness: role of the pigment epithelium. Vision Res. 10, 1333–1345. doi: 10.1016/0042-6989(70)90085-4
Bridges, C. D., and Yoshikami, S. (1970b). The rhodopsin-porphyropsin system in freshwater fishes. 1. Effects of age and photic environment. Vision Res. 10, 1315–1332. doi: 10.1016/0042-6989(70)90084-2
Burns, K. J., Hackett, S. J., and Klein, N. K. (2002). Phylogenetic relationships and morphological diversity in Darwin's finches and their relatives. Evolution 56, 1240–1252. doi: 10.1111/j.0014-3820.2002.tb01435.x
Caprette, C. L., Lee, M. S. Y., Shine, R., Mokany, A., and Downhower, J. F. (2004). The origin of snakes (Serpentes) as seen through eye anatomy. Biol. J. Linn. Soc. 81, 469–482. doi: 10.1111/j.1095-8312.2003.00305.x
Carvalho, L. D. S., Cowing, J. A., Wilkie, S. E., Bowmaker, J. K., and Hunt, D. M. (2006). Shortwave visual sensitivity in tree and flying squirrels reflects changes in lifestyle. Curr. Biol. 16. R81–R83. doi: 10.1016/j.cub.2006.01.045
Carvalho, L. S., Pessoa, D., Mountford, J. K., Davies, W. I., and Hunt, D. M. (2017). The genetic and evolutionary drives behind primate color vision. Front. Ecol. Evol. 5:34. doi: 10.3389/fevo.2017.00034
Castoe, T. A., Jason De Koning, A. P., Hall, K. T., Card, D. C., Schield, D. R., Fujita, M. K., et al. (2013). The Burmese python genome reveals the molecular basis for extreme adaptation in snakes. Proc. Natl. Acad. Sci. U.S.A. 110, 20645–20650. doi: 10.1073/pnas.1314475110
Chen, D. M., Collins, J. S., and Goldsmith, T. H. (1984). The ultraviolet receptor of bird retinas. Science 225, 337–340. doi: 10.1126/science.6740315
Chen, M., Price, R. M., Yamashita, Y., and Jaffé, R. (2010). Comparative study of dissolved organic matter from groundwater and surface water in the Florida coastal Everglades using multi-dimensional spectrofluorometry combined with multivariate statistics. Appl. Geochem. 25, 872–880. doi: 10.1016/j.apgeochem.2010.03.005
Chou, B. R., and Cullen, A. P. (1984). Spectral transmittance of the ocular media of the thirteen-lined ground squirrel (Spermophilus tridecemlineatus). Can. J. Zool. 62, 825–830. doi: 10.1139/z84-120
Collin, S. P., Knight, M. A., Davies, W. L., Potter, I. C., Hunt, D. M., and Trezise, A. E. O. (2003). Ancient colour vision: multiple opsin genes in the ancestral vertebrates. Curr. Biol. 13:R864–R865. doi: 10.1016/j.cub.2003.10.044
Conant, R., and Collins, J. T. (1998). A Field Guide to Reptiles & Amphibians: Eastern and Central North America. New York, NY: Houghton Mifflin Harcourt.
Cooper, G. F., and Robson, J. G. (1969a). The yellow colour of the lens of man and other primates. J. Physiol. 203, 411–417. doi: 10.1113/jphysiol.1969.sp008871
Cooper, G. F., and Robson, J. G. (1969b). The yellow colour of the lens of the grey squirrel (Sciurus carolinensis leucotis). J. Physiol. 203, 403–410. doi: 10.1113/jphysiol.1969.sp008870
Crescitelli, F., Dartnall, H. J. A., and Loew, E. R. (1977). The gecko visual pigments: a microspectrophotometric study. J. Physiol. 268, 559–573. doi: 10.1113/jphysiol.1977.sp011872
Dartnall, H. J. A., and Lythgoe, J. N. (1965). The spectral clustering of visual pigments. Vision Res. 5, 81–100. doi: 10.1016/0042-6989(65)90057-X
Davies, W. I., Collin, S. P., and Hunt, D. M. (2012). Molecular ecology and adaptation of visual photopigments in craniates. Mol. Ecol. 21, 3121–3158. doi: 10.1111/j.1365-294X.2012.05617.x
Davies, W. I., Foster, R. G., and Hankins, M. W. (2014). The Evolution and Function of Melanopsin in Craniates. Boston, MA: Springer.
Davies, W. L., Cowing, J. A., Bowmaker, J. K., Carvalho, L. S., Gower, D. J., and Hunt, D. M. (2009). Shedding light on serpent sight: the visual pigments of henophidian snakes. J. Neurosci. 29, 7519–7525. doi: 10.1523/JNEUROSCI.0517-09.2009
Davies, W. L., Cowing, J. A., Carvalho, L. S., Potter, I. C., Trezise, A. E., Hunt, D. M., et al. (2007). Functional characterization, tuning, and regulation of visual pigment gene expression in an anadromous lamprey. FASEB J. 21, 2713–2724. doi: 10.1096/fj.06-8057com
Dillon, J., Zheng, L. E. I., Merriam, J. C., and Gaillard, E. R. (1999). The optical properties of the anterior segment of the eye: implications for cortical cataract. Exp. Eye Res. 68, 785–795. doi: 10.1006/exer.1999.0687
Downes, G. B., and Gautam, N. (1999). The G protein subunit gene families. Genomics 62, 544–552. doi: 10.1006/geno.1999.5992
Ellingson, J. M., Fleishman, L. J., and Loew, E. R. (1995). Visual pigments and spectral sensitivity of the diurnal gecko Gonatodes albogularis. J. Comp. Physiol. A Sens. Neural Behav. Physiol. 177, 559–567. doi: 10.1007/BF00207185
Emerling, C. A. (2017a). Genomic regression of claw keratin, taste receptor and light-associated genes inform biology and evolutionary origins of snakes. Mol. Phylogenet. Evol. 115, 40–49. doi: 10.1016/j.ympev.2017.07.014
Emerling, C. A. (2017b). Archelosaurian colour vision, parietal eye loss and the crocodilian nocturnal bottleneck. Mol. Biol. Evol. 34 666–676. doi: 10.1093/molbev/msw265
Fain, G. L., Hardie, R., and Laughlin, S. B. (2010). Phototransduction and the evolution of photoreceptors. Curr. Biol. 20, R114–R124. doi: 10.1016/j.cub.2009.12.006
Fleishman, L. J., Loew, E. R., and Leal, M. (1993). Ultraviolet vision in lizards. Nature 365, 397. doi: 10.1038/365397a0
Fleishman, L. J., Loew, E. R., and Whiting, M. J. (2011). High sensitivity to short wavelengths in a lizard and implications for understanding the evolution of visual systems in lizards. Proc. R. Soc. B Biol. Sci. 278, 2891–2899. doi: 10.1098/rspb.2011.0118
Foster, R. G., Garcia-Fernandez, J. M., Provencio, I., and DeGrip, W. J. (1993). Opsin localization and chromophore retinoids identified within the basal brain of the lizard Anolis carolinensis. J. Comp. Physiol. A 172, 33–45. doi: 10.1007/bf00214713
Fowlkes, D. H., Karwoski, C. J., and Proenza, L. M. (1984). Endogenous circadian rhythm in electroretinogram of free-moving lizards. Invest. Ophthalmol. Visual Sci. 25, 121–124.
Gaillard, E. R., Merriam, J., Zheng, L., and Dillon, J. (2011). Transmission of light to the young primate retina: possible implications for the formation of lipofuscin. Photochem. Photobiol. 87, 18–21. doi: 10.1111/j.1751-1097.2010.00837.x
Gamble, T., Greenbaum, E., Jackman, T. R., and Bauer, A. M. (2015). Into the light: diurnality has evolved multiple times in geckos. Biol. J. Linn. Soc. 115, 896–910. doi: 10.1111/bij.12536
Garrick, L. D., and Lang, J. W. (1977). Social signals and behaviors of adult alligators and crocodiles. Am. Zool. 17, 225–239. doi: 10.1093/icb/17.1.225
Gaur, V. P., Eldred, W., and Sarthy, P. V. (1988). Distribution of Müller cells in the turtle retina: an immunocytochemical study. J. Neurocytol. 17, 683–692. doi: 10.1007/bf01260995
Gerkema, M. P., Davies, W. I., Foster, R. G., Menaker, M., and Hut, R. A. (2013). The nocturnal bottleneck and the evolution of activity patterns in mammals. Proc. R. Soc. B Biol. Sci. 280:20130508. doi: 10.1098/rspb.2013.0508
Goede, P., and Kolb, H. (1994). Identification of the synaptic pedicles belonging to the different spectral types of photoreceptor in the turtle retina. Vision Res. 34, 2801–2811. doi: 10.1016/0042-6989(94)90049-3
Govardovskii, V. I., Chkheidze, N. I., and Zueva, L. V. (1988). Morphofunctional investigation of the retina in the crocodilian caiman Caiman crocodilus. Sens. Syst. 1, 19–25.
Gower, D. J., Sampaio, F. L., Peichl, L., Wagner, H. J., Loew, E. R., McLamb, W., et al. (2019). Evolution of the eyes of vipers with and without infrared-sensing pit organs. Biol. J. Linn. Soc. 126, 796–823. doi: 10.1093/biolinnean/blz003
Gozem, S., Schapiro, I., Ferre, N., and Olivucci, M. (2012). The molecular mechanism of thermal noise in rod photoreceptors. Science 137, 1225–1228. doi: 10.1126/science.1220461
Green, R. E., Braun, E. L., Armstrong, J., Earl, D., Nguyen, N., Hickey, G., et al. (2014). Three crocodilian genomes reveal ancestral patterns of evolution among archosaurs. Science 346, 1335. doi: 10.1126/science.1254449
Hankins, M. W., Davies, W. I., and Foster, R. G. (2014). The Evolution of Non-visual Photopigments in the Central Nervous System of Vertebrates. Boston, MA: Springer.
Hárosi, F. I., and Hashimoto, Y. (1983). Ultraviolet visual pigment in a vertebrate: a tetrachromatic cone system in the dace. Science 222, 1021–1023. doi: 10.1126/science.6648514
Hart, N. S., Bailes, H. J., Vorobyev, M., Marshall, N. J., and Collin, S. P. (2008). Visual ecology of the Australian lungfish (Neoceratodus forsteri). BMC Ecol. 8:21. doi: 10.1186/1472-6785-8-21
Hart, N. S., Coimbra, J. P., Collin, S. P., and Westhoff, G. (2012). Photoreceptor types, visual pigments, and topographic specializations in the retinas of hydrophiid sea snakes. J. Comp. Neurol. 520, 1246–1261. doi: 10.1002/cne.22784
Hauzman, E., Bonci, D. M. O., Grotzner, S. R., Mela, M., Liber, A. M. P., Martins, S. L., et al. (2014). Comparative study of photoreceptor and retinal ganglion cell topography and spatial resolving power in dipsadidae snakes. Brain Behav. Evol. 84, 197–213. doi: 10.1159/000365275
Hauzman, E., Bonci, D. M. O., Suárez-Villota, E. Y., Neitz, M., and Ventura, D. F. (2017). Daily activity patterns influence retinal morphology, signatures of selection, and spectral tuning of opsin genes in colubrid snakes. BMC Evol. Biol. 17:249. doi: 10.1186/s12862-017-1110-0
Hemmi, J. M., James, A., and Taylor, W. R. (2002). Color opponent retinal ganglion cells in the tammar wallaby retina. J. Vision 2, 3. doi: 10.1167/2.9.3
Hover, E. L. (1985). Differences in aggressive behavior between two throat color morphs in a lizard, Urosaurus ornatus. Copeia 1985, 933–940. doi: 10.2307/1445244
Jacobs, G. H., Calderone, J. B., Fenwick, J. A., Krogh, K., and Williams, G. A. (2003). Visual adaptations in a diurnal rodent, Octodon degus. J. Comp. Physiol. A 189, 347–361. doi: 10.1007/s00359-003-0408-0
Jacobs, G. H., Fenwick, J. A., Crognale, M. A., and Deegan, J. F. (1992). The all-cone retina of the garter snake: spectral mechanisms and photopigment. J. Comp. Physiol. A 170, 701–707. doi: 10.1007/BF00198980
Jacobs, G. H., Neitz, J., and Deegan, J. F. I. I. (1991). Retinal receptors in rodents maximally sensitive to ultraviolet light. Nature 353, 655–656. doi: 10.1038/353655a0
Joesch, M., and Meister, M. (2016). A neuronal circuit for colour vision based on rod-cone opponency. Nature 532, 236–239. doi: 10.1038/nature17158
Katti, C., Stacey-Solis, M., Coronel-Rojas, N., and Davies, W. (2018). Opsin-based photopigments expressed in the retina of a South American pit viper, Bothrops atrox (Viperidae). Vis. Neurosci. 35:E027. doi: 10.1017/S0952523818000056
Kawamura, S., and Yokoyama, S. (1993). Molecular characterization of the red visual pigment gene of the American chameleon (Anolis carolinensis). FEBS Lett. 323, 247–251. doi: 10.1016/0014-5793(93)81350-9
Kawamura, S., and Yokoyama, S. (1994). Cloning of the rhodopsin-encoding gene from the rod-less lizard Anolis carolinensis. Gene 149, 267–270. doi: 10.1016/0378-1119(94)90159-7
Kawamura, S., and Yokoyama, S. (1995). Paralogous origin of the rhodopsin-like opsin genes in lizards. J. Mol. Evol. 40, 494–600. doi: 10.1007/BF00160506
Kawamura, S., and Yokoyama, S. (1996). Phylogenetic relationships among short wavelength-sensitive opsins of American chameleon (Anolis carolinensis) and other vertebrates. Vision Res. 36, 2797–2804. doi: 10.1016/0042-6989(96)00034-X
Kawamura, S., and Yokoyama, S. (1997). Expression of visual and nonvisual opsins in American chameleon. Vision Res. 37, 1867–1871. doi: 10.1016/S0042-6989(96)00309-4
Kawamura, S., and Yokoyama, S. (1998). Functional characterization of visual and nonvisual pigments of American chameleon (Anolis carolinensis). Vision Res. 38, 37–44. doi: 10.1016/S0042-6989(97)00160-0
Kessel, L., Lundeman, J. H., Herbst, K., Andersen, T. V., and Larsen, M. (2010). Age-related changes in the transmission properties of the human lens and their relevance to circadian entrainment. J. Cataract Refract. Surg. 36, 308–312. doi: 10.1016/j.jcrs.2009.08.035
Kirk, J. T. O. (1980). Spectral adsorption properties of natural waters: contribution of the soluble and particulate fractions to light absorption in some inland waters of south-eastern Australia. Mar. Freshw. Res. 31, 287–296. doi: 10.1071/MF9800287
Kojima, D., Okano, T., Fukada, Y., Shichida, Y., Yoshizawa, T., and Ebrey, T. G. (1992). Cone visual pigments are present in gecko rod cells. Proc. Natl. Acad. Sci.U.S.A. 89, 6841–6845. doi: 10.1073/pnas.89.15.6841
Kolb, H., and Jones, J. (1987). The distinction by light and electron microscopy of two types of cone containing colorless oil droplets in the retina of the turtle. Vision Res. 27, 1445–1458. doi: 10.1016/0042-6989(87)90154-4
Lamb, T. D. (2013). Evolution of phototransduction, vertebrate photoreceptors and retina. Prog. Retin. Eye Res. 36, 52–119. doi: 10.1016/j.preteyeres.2013.06.001
Laurens, H., and Detwiler, S. R. (1921). Studies on the retina. J. Exp. Zool. 32:207. doi: 10.1002/jez.1400320204
Liebman, P. A. (1972). “Microspectrophotometry of photoreceptors,” in Photochemistry of Vision (Handbook of Sensory Physiology), ed H. J. A. Dartnall (Berlin: Springer).
Liebman, P. A., and Granda, A. M. (1971). Microspectrophotometric measurements of visual pigments in 2 species of turtle, Pseudemys scripta and Chelonia mydas. Vision Res. 11, 105–114. doi: 10.1016/0042-6989(71)90227-6
Lipetz, L. E. (1985). “Some neuronal circuits of the turtle retina,” in The Visual System, eds A. Fein and J. S. Levine (New York, NY: Alan R. Liss, Inc.), 107–132.
Lipetz, L. E., and MacNichol, E. F. Jr. (1982). Photoreceptors of freshwater turtles: cell types and visual pigments. Biol. Bull. 163, 396.
Lipetz, L. E., and MacNichol, E. F. Jr. (1983). Visual pigments of two freshwater turtles. Biophys. J. 41, 26a.
Lipetz, L. E., and MacNichol, E. F. Jr. (1990). Visual pigments, screening pigments, and spectral sensitivities of turtle photoreceptors. Invest. Ophthalmology 31, 259.
Liu, Y., Zhou, Q., Wang, Y., Luo, L., Yang, J., Yang, L., et al. (2015). Gekko japonicus genome reveals evolution of adhesive toe pads and tail regeneration. Nat. Commun. 6:10033. doi: 10.1038/ncomms10033
Loew, E. (1994). A third, ultraviolet-sensitive, visual pigment in the Tokay gecko (Gekko gekko). Vision Res. 34, 1427–1431. doi: 10.1016/0042-6989(94)90143-0
Loew, E. R., Fleishman, L. J., Foster, R. G., and Provencio, I. (2002). Visual pigments and oil droplets in diurnal lizards: a comparative study of Caribbean anoles. J. Exp. Biol. 205, 927–938.
Loew, E. R., and Govardovskii, V. I. (2001). Photoreceptors and visual pigments in the red-eared turtle, Trachemys scripta elegans. Vis. Neurosci. 18, 753–757. doi: 10.1017/S0952523801185081
Loew, E. R., Govardovskii, V. I., Rohlich, P., and Szel, A. (1996). Microspectrophotometric and immunocytochemical identification of ultraviolet photoreceptors in geckos. Vis. Neurosc. 13, 247–256. doi: 10.1017/S0952523800007483
Menon, S. T., Han, M., and Sakmar, T. P. (2001). Rhodopsin: structural basis of molecular physiology. Physiol. Rev. 81, 1659–1688. doi: 10.1152/physrev.2001.81.4.1659
Michael, C. R. (1966). Receptive fields of opponent color units in the optic nerve of the ground squirrel. Science 152, 1095–1097. doi: 10.1126/science.152.3725.1095
Miller, W. H. (1981). “Ca2+and cGMP,” in Current Topics in Membranes and Transport, eds F. Bronner and A. Kleinzellar (New York, NY: Academic Press, 441–445.
Müller, B., Glösmann, M., Peichl, L., Knop, G. C., Hagemann, C., and Ammermüller, J. (2009). Bat eyes have ultraviolet-sensitive cone photoreceptors. PLoS ONE 4:e6390. doi: 10.1371/journal.pone.0006390
Nagloo, N., Collin, S. P., Hemmi, J. M., and Hart, N. S. (2016). Spatial resolving power and spectral sensitivity of the saltwater crocodile, Crocodylus porosus, and the freshwater crocodile, Crocodylus johnstoni. J. Exp. Biol. 219, 1394–1404. doi: 10.1242/jeb.135673
New, S. T., Hemmi, J. M., Kerr, G. D., and Bull, C. M. (2012). Ocular anatomy and retinal photoreceptors in a skink, the sleepy lizard (Tiliqua rugosa). Anat. Rec. 295, 1727–1735. doi: 10.1002/ar.22546
Nicholson, K. E., Glor, R. E., Kolbe, J. J., Larson, A., Hedges, S. B., and Losos, J. B. (2005). Mainland colonization by island lizards. J. Biogeogr. 32, 929–938. doi: 10.1111/j.1365-2699.2004.01222.x
Niemiller, M. L., Fitzpatrick, B. M., Shah, P., Schmitz, L., and Near, T. J. (2013). Evidence for repeated loss of selective constraint in rhodopsin of amblyopsid cavefishes (Teleostei: Amblyopsidae). Evolution 67, 732–748. doi: 10.1111/j.1558-5646.2012.01822.x
Nordström, K., Larsson, T. A., and Larhammar, D. (2004). Extensive duplications of phototransduction genes in early vertebrate evolution correlate with block (chromosome) duplications. Genomics 83, 852–872. doi: 10.1016/j.ygeno.2003.11.008
Ohtsuka, T. (1978). Combination of oil droplets with different types of photoreceptor in a freshwater turtle, Geoclemys reevesii. Sens. Process. 2, 321–325.
Ohtsuka, T. (1985a). Spectral sensitivities of seven morphological types of photoreceptors in the retina of the turtle, Geoclemys reevesii. J. Comp. Neurol. 237, 145–154. doi: 10.1002/cne.902370202
Ohtsuka, T. (1985b). Relation of spectral types of oil droplets in cones of turtle retina. Science 229, 874–877. doi: 10.1126/science.4023716
Ohtsuka, T., and Kawamata, K. (1990). Monoclonal antibody labels both rod and cone outer segments of turtle photoreceptors. Exp. Eye Res. 50, 483–486. doi: 10.1016/0014-4835(90)90036-T
Peng, Y. W., Robishaw, J. D., Levine, M. A., and Yau, K. W. (1992). Retinal rods and cones have distinct G protein beta and gamma subunits. Proc. Natl. Acad. Sci. U.S.A. 89, 10882–10886. doi: 10.1073/pnas.89.22.10882
Perry, B. W., Card, D. C., McGlothlin, J. W., Pasquesi, G. I. M., Adams, R. H., Schield, D. R., et al. (2018). Molecular adaptations for sensing and securing prey and insight into amniote genome diversity from the garter snake genome. Genome Biol. Evol. 10, 2110–2129. doi: 10.1093/gbe/evy157
Peterson, E. H. (1992). “Retinal structure,” in Sensorimotor Integration. Biology of the Reptilia, eds C. Gans and P. S. Ulinski (Chicago, IL: University of Chicago Press), 1–135.
Petry, H. M., and Hárosi, F. I. (1990). Visual pigments of the tree shrew (Tupaia belangeri) and greater galago (Galago crassicaudatus): a microspectrophotometric investigation. Vision Res. 30, 839–851. doi: 10.1016/0042-6989(90)90053-N
Pinto, G., Mahler, D. L., Harmon, L. J., and Losos, J. B. (2008). Testing the island effect in adaptive radiation: rates and patterns of morphological diversification in Caribbean and mainland Anolis lizards. Proc. R. Soc. B Biol. Sci. 275, 2749–2757. doi: 10.1098/rspb.2008.0686
Provencio, I., Loew, E. R., and Foster, R. G. (1992). Vitamin-A2-based visual pigments in fully terrestrial vertebrates. Vision Res. 32, 2201–2208. doi: 10.1016/0042-6989(92)90084-V
Pyron, A., and Wiens, J. J. (2011). A large-scale phylogeny of Amphibia including over 2800 species, and a revised classification of extant frogs, salamanders, and caecilians. Mol. Phylogenet. Evol. 61, 543–583. doi: 10.1016/j.ympev.2011.06.012
Rando, R. R. (1996). Polyenes and vision. Chem. Biol. 3, 255–262. doi: 10.1016/S1074-5521(96)90105-2
Röll, B. (2000). Gecko vision—Visual cells, evolution, and ecological constraints. J. Neurocytol. 29, 471–484. doi: 10.1023/A:1007293511912
Röll, B. (2001a). Gecko vision—retinal organization, foveae and implications for binocular vision. Vision Res. 41, 2043–2056. doi: 10.1016/S0042-6989(01)00093-1
Röll, B. (2001b). Retina of Bouton's skink (Reptilia, Scincidae): visual cells, fovea, and ecological constraints. J. Comp. Neurol. 436, 487–496. doi: 10.1002/cne.1082
Schluter, D. (2000). “The ecology of adaptive radiation”, in Oxford Series in Ecology and Evolution (Oxford, UK: Oxford University Press), 288. doi: 10.2307/3558417
Schmitz, L., and Higham, T. E. (2018). Non-uniform evolutionary response of gecko eye size to changes in diel activity patterns. Biol. Lett. 14:20180064. doi: 10.1098/rsbl.2018.0064
Schott, R. K., Müller, J., Yang, C. G. Y., Bhattacharyya, N., Chan, N., Xu, M., et al. (2016). Evolutionary transformation of rod photoreceptors in the all-cone retina of a diurnal garter snake. Proc. Natl. Acad. Sci. U.S.A. 113, 356–361. doi: 10.1073/pnas.1513284113
Schott, R. K., Van Nynatten, A., Card, D. C., Castoe, T. A., and Chang, B. S. W. (2018). Shifts in selective pressures on snake phototransduction genes associated with photoreceptor transmutation and dim-light ancestry. Mol. Biol. Evol. 35, 1376–1389. doi: 10.1093/molbev/msy025
Shichida, Y., and Matsuyama, T. (2009). Evolution of opsins and phototransduction. Philos. Trans. R. Soc. B Biol. Sci. 364, 2881–2895. doi: 10.1098/rstb.2009.0051
Sillman, A. J., Carver, J., and Loew, E. (1999). The photoreceptors and visual pigments in the retina of a boid snake, the ball python (Python regius). J. Exp. Biol. 202, 1931–1938.
Sillman, A. J., Govardovskii, V. I., Rohlich, P., Southard, J. A., and Loew, E. R. (1997). The photoreceptors and visual pigments of the garter snake (Thamnophis sirtalis): a microspectrophotometric, scanning electron microscopic and immunocytochemical study. J. Comp. Physiol. A, 181, 89–101. doi: 10.1007/s003590050096
Sillman, A. J., Johnson, J. L., and Loew, E. R. (2001). Retinal photoreceptors and visual pigments in Boa constrictor imperator. J. Exp. Zool. 290, 359–365. doi: 10.1002/jez.1076
Sillman, A. J., Ronan, S. J., and Loew, E. R. (1991). Histology and microspectrophotometry of the photoreceptors of a crocodilian, Alligator mississippiensis. Proc. R. Soc. Lond. Ser. B Biol. Sci. 243, 93–98. doi: 10.1098/rspb.1991.0016
Simões, B., Sampaio, F. L., Douglas, R. H., Kodandaramaiah, U., Casewell, N. R., Harrison, R. A., et al. (2016a). Visual pigments, ocular filters and the evolution of snake vision. Mol. Biol. Evol. 33, 2483–2495. doi: 10.1093/molbev/msw148
Simões, B., Sampaio, F. L., Jared, C., Antoniazzi, M. M., Loew, E. R., Bowmaker, J. K., et al. (2015). Visual system evolution and the nature of the ancestral snake. J. Evol. Biol. 28, 1309–1320. doi: 10.1111/jeb.12663
Simões, B., Sampaio, F. L., Loew, E. R., Sanders, K. L., Fisher, R. N., Hart, N. S., et al. (2016b). Multiple rod–cone and cone–rod photoreceptor transmutations in snakes: evidence from visual opsin gene expression. Proc. R. Soc. B Biol. Sci. 283:20152624. doi: 10.1098/rspb.2015.2624
Simões, B. F., and Gower, D. J. (2017). “Visual pigment evolution in reptiles,” in eLS (Chichester: John Wiley & Sons Ltd.). doi: 10.1002/9780470015902.a0026519
Simpson, G. G. (1953). “Mutation,” in The Major Features of Evolution (New York, NY: Columbia University Press), 310–312.
Steffen, J. E., and Guyer, C. C. (2014). Display behaviour and dewlap colour as predictors of contest success in brown anoles. Biol. J. Linn. Soc. 111, 646–655. doi: 10.1111/bij.12229
Szél, Á., and Röhlich, P. (1985). Localization of visual pigment antigens to photoreceptor cells with different oil droplets in the chicken retina. Ada Biol. Hung. 36, 319–324.
Szél, Á., Röhlich, P., and Govardovskii, V. (1986). Immunocytochemical discrimination of visual pigments in the retinal photoreceptors of the nocturnal gecko Teratoscincus scincus. Exp. Eye Res. 43, 895–904. doi: 10.1016/0014-4835(86)90068-0
Taniguchi, Y., Hisatomi, O., Yoshida, Y., and Tokunaga, F. (1999). Evolution of visual pigments in geckos. FEBS Lett. 44, 536–540. doi: 10.1016/S0014-5793(99)00089-7
Thomas, R., Szekely, T., Powell, R., and Cuthill, I. (2006). Eye size, foraging methods and the timing of foraging in shorebirds. Funct. Ecol. 20, 157–165. doi: 10.1111/j.1365-2435.2006.01073.x
Thompson, C. W., and Moore, M. C. (1991a). Syntopic occurence of multiple dewlap color morphs in male tree lizards, Urosaurus ornatus. Copeia 1991, 493–503. doi: 10.2307/1446597
Thompson, C. W., and Moore, M. C. (1991b). Throat colour reliably signals status in male tree lizards, Urosaurus ornatus. Anim. Behav. 42, 745–753. doi: 10.1016/S0003-3472(05)80120-4
Toyama, M., Hironaka, M., Yamahama, Y., Horiguchi, H., Tsukada, O., Uto, N., et al. (2008). Presence of rhodopsin and porphyropsin in the eyes of 164 fishes, representing marine, diadromous, coastal and freshwater species—a qualitative and comparative study. Photochem. Photobiol. 84, 996–1002. doi: 10.1111/j.1751-1097.2008.00344.x
Uetz, P., Freed, P., and Hošek, J. (eds.). (2019). The Reptile Database. Available online at: http://www.reptile-database.org (accessed May, 2019).
Underwood, G. (1970). “The eye,” in Biology of the Reptilia, ed C. Gans and T. S. Parsons (London: Academic Press, 1–97.
Van Hazel, I., Santini, F., Müller, J., and Chang, B. S. W. (2006). Short-wavelength sensitive opsin (SWS1) as a new marker for vertebrate phylogenetics. BMC Evol. Biol. 6:97. doi: 10.1186/1471-2148-6-97
Veilleux, C. C., and Cummings, M. E. (2012). Nocturnal light environments and species ecology: implications for nocturnal color vision in forests. J. Exp. Biol. 215, 4085–4096. doi: 10.1242/jeb.071415
Ventura, D. F., Zana, Y., De Souza, J. M., and Devoe, R. D. (2001). Ultraviolet colour opponency in the turtle retina. J. Exp. Biol. 204, 2527–2534.
Vonk, F. J., Casewell, N. R., Henkel, C. V., Heimberg, A. M., Jansen, H. J., Mccleary, R. J. R., et al. (2013). The king cobra genome reveals dynamic gene evolution and adaptation in the snake venom system. Proc. Natl. Acad. Sci. U.S.A. 110, 20651–20656. doi: 10.1073/pnas.1314702110
Wald, G. (1968a). Molecular basis of visual excitation. Science 162, 230–239. doi: 10.1126/science.162.3850.230
Wald, G. (1968b). The molecular basis of visual excitation. Nature 219, 800–807. doi: 10.1038/219800a0
Walls, G. L. (1931). The occurrence of colored lenses in the eyes of snakes and squirrels, and their probable significance. Copeia 1931, 125–127.
Walls, G. L. (1934). The reptilian retina. I. A new concept of visual cell evolution. Am. J. Ophthalmol. 17, 892–915.
Walls, G. L. (1940). Ophthalmological implications for the early history of the snakes. Copeia 1940, 1–8. doi: 10.2307/1439015
Walls, G. L. (1942). The Vertebrate Eye and Its Adaptive Radiation. New York, NY: Hafner Publishing Co, 1–302.
Walter, A. E., Shuster, T. A., and Farber, D. B. (1986). Light-induced phosphorylation of proteins from the all-cone retina of the lizard, Anolis carolinensis. Invest. Ophthalmol. Vis. Sci. 27, 1609–1614.
Wan, Q. H., Pan, S. D. K., Hu, L., Zhu, Y., Xu, P. D. W., Xia, J. D. Q., et al. (2013). Genome analysis and signature discovery for diving and sensory properties of the endangered Chinese alligator. Cell Res. 23, 1091–1105. doi: 10.1038/cr.2013.104
Wang, Z., Pascual-Anaya, J., Zadissa, A., Li, W., Niimura, Y., Huang, Z., et al. (2013). The draft genomes of soft-shell turtle and green sea turtle yield insights into the development and evolution of the turtle-specific body plan. Nat. Genet. 45, 701–706. doi: 10.1038/ng.2615
Wensel, T. G. (2008). Signal transducing membrane complexes of photoreceptor outer segments. Vision Res. 48, 2052–2061. doi: 10.1016/j.visres.2008.03.010
Werner, Y. L., and Seifan, T. (2006). Eye size in geckos: asymmetry, allometry, sexual dimorphism, and behavioral correlates. J. Morphol. 267, 1486–1500. doi: 10.1002/jmor.10499
Wheeler, T. G., and Naka, K. I. (1977). The modes of chromatic interactions in the retina. Vision Res. 17, 1015–1018. doi: 10.1016/0042-6989(77)90004-9
Whitmore, A. V., and Bowmaker, J. K. (1989). Seasonal variation in cone sensitivity and short-wave absorbing visual pigments in the rudd Scardinius erythrophthalmus. J. Comp. Physiol. A 166, 103–115. doi: 10.1007/BF00190215
Wong, R. O. (1989). Morphology and distribution of neurons in the retina of the American garter snake Thamnophis sirtalis. J. Comp. Neurol. 283, 587–601.
Yau, K. W., and Hardie, R. C. (2009). Phototransduction Motifs and Variations. Cell. 139, 246–264. doi: 10.1016/j.cell.2009.09.029
Yewers, M. S., McLean, C. A., Moussalli, A., Stuart-Fox, D., Bennett, A. T. D., and Knott, B. (2015). Spectral sensitivity of cone photoreceptors and opsin expression in two colour-divergent lineages of the lizard Ctenophorus decresii. J. Exp. Biol. 218(Pt 10), 1556–1563. doi: 10.1242/jeb.131854
Yokoyama, S. (2000). Molecular evolution of vertebrate visual pigments. Prog. Retin. Eye Res. 19, 385–419. doi: 10.1016/S1350-9462(00)00002-1
Yokoyama, S., and Blow, N. S. (2001). Molecular evolution of the cone visual pigments in the pure rod-retina of the nocturnal gecko, Gekko gekko. Gene 276, 117–125. doi: 10.1016/S0378-1119(01)00643-6
Yolton, R. L., Yolton, D. P., Renz, J., and Jacobs, G. H. (1974). Preretinal absorbance in sciurid eyes. J. Mammal. 55, 14–20.
Keywords: opsin, reptiles, rods, cones, color vision, photopigment
Citation: Katti C, Stacey-Solis M, Coronel-Rojas NA and Davies WIL (2019) The Diversity and Adaptive Evolution of Visual Photopigments in Reptiles. Front. Ecol. Evol. 7:352. doi: 10.3389/fevo.2019.00352
Received: 31 January 2019; Accepted: 04 September 2019;
Published: 19 September 2019.
Edited by:
Renoult P. Julien, UMR5175 Centre d'Ecologie Fonctionnelle et Evolutive (CEFE), FranceReviewed by:
Daniel Osorio, University of Sussex, United KingdomDavid Gower, The Natural History Museum, London, United Kingdom
Copyright © 2019 Katti, Stacey-Solis, Coronel-Rojas and Davies. This is an open-access article distributed under the terms of the Creative Commons Attribution License (CC BY). The use, distribution or reproduction in other forums is permitted, provided the original author(s) and the copyright owner(s) are credited and that the original publication in this journal is cited, in accordance with accepted academic practice. No use, distribution or reproduction is permitted which does not comply with these terms.
*Correspondence: Christiana Katti, Y2thdHRpQGdtYWlsLmNvbQ==