- 1U.S. Forest Service, Rocky Mountain Research Station, Provo, UT, United States
- 2Department of Biology, Brigham Young University, Provo, UT, United States
- 3Monte Lafayette Bean Life Science Museum, Brigham Young University, Provo, UT, United States
- 4School of Life Sciences, University of Nevada, Las Vegas, NV, United States
- 5Department of Ecology and Evolutionary Biology, University of Connecticut, Storrs, CT, United States
- 6Department of Microbiology and Plant Pathology, Institute for Integrative Genome Biology, University of California, Riverside, Riverside, CA, United States
- 7Department of Plant and Wildlife Sciences, Brigham Young University, Provo, UT, United States
Biological soil crusts (BSCs) consist of a diverse and highly integrated community of organisms that effectively colonize and collectively stabilize soil surfaces. BSCs vary in terms of soil chemistry and texture as well as the environmental parameters that combine to support unique combinations of organisms—including cyanobacteria dominated, lichen-dominated, and bryophyte-dominated crusts. The list of organismal groups that make up BSC communities in various and unique combinations include—free living, lichenized, and mycorrhizal fungi, chemoheterotrophic bacteria, cyanobacteria, diazotrophic bacteria and archaea, eukaryotic algae, and bryophytes. The various BSC organismal groups demonstrate several common characteristics including—desiccation and extreme temperature tolerance, production of various soil binding chemistries, a near exclusive dependency on asexual reproduction, a pattern of aerial dispersal over impressive distances, and a universal vulnerability to a wide range of human-related perturbations. With this publication, we provide literature-based insights as to how each organismal group contributes to the formation and maintenance of the structural and functional attributes of BSCs, how they reproduce, and how they are dispersed. We also emphasize the importance of effective application of molecular and microenvironment sampling and assessment tools in order to provide cogent and essential answers that will allow scientists and land managers to better understand and manage the biodiversity and functional relationships of soil crust communities.
Biological soil crusts (BSCs) consist of various combinations of living organisms that colonize, organize, and stabilize soil surfaces against the erosive forces of wind and water. Many BSC organisms are photoautotrophic, fixing, and accumulating organic carbon (Green and Proctor, 2016) while other organismal groups fix and distribute organic nitrogen. Groups of organisms known to contribute to the formation BSC communities include cyano-, chemoheterotrophic, and diazotrophic bacteria, free-living, lichenized, and mycorrhizal fungi, terrestrial algae (including diatoms), and bryophytes (Belnap et al., 2001; Weber et al., 2016). To be recognized as a contributor to the formation and maintenance of BSC communities, an organism must be involved in the consolidation and stabilization of soil particles and aggregates, resulting in the formation of an intact BSC community. In this review of BSC organisms, we evaluate each organismal group independently, documenting how they contribute to the formation of BSCs, and how they reproduce and disperse.
Bacteria
Prokaryotic bacteria commonly found in BSC communities include cyanobacteria, chemoheterotrophic bacteria, and free-living diazotrophic (nitrogen fixing) bacteria.
Cyanobacteria
Cyanobacteria are photoautotrophic prokaryotes (Whitton and Potts, 2000). Traditionally, cyanobacteria were classified with the eukaryotic “algae” based on the presence of chlorophyll a and the production of molecular oxygen. However, given the absence of membrane bound subcellular structures (e.g., nuclei, mitochondria, etc.) and the occurrence of prokaryotic type ribosomes, the cyanobacteria are now classified as bacteria. Cyanobacteria also produce a unique cell wall chemistry containing peptidoglycans rather than cellulose. Thallus-types range from unicellular to multicellular filaments, sheets, or globular thalli (Schirrmeister et al., 2011; Herrero et al., 2016). Numerous genera of BSC cyanobacteria have been reported—spanning five orders, Chroococcales, Nostocales, Oscillatoriales, Pleurocapsales, and Synechococcales. Cyanobacteria are recognized as one of the most important and abundant photoautotrophs in many arid land BSC communities (Colesie et al., 2016)—occurring at or just below the soil surface (Hu et al., 2003). Through photosynthesis, cyanobacteria contribute significant fixed carbon to the BSC community. Many species, including many of the filamentous and globular forms, also produce heterocysts, specialized nitrogen fixing structures (Fay, 1992; Bergman et al., 1997; Kumar et al., 2010). Nitrogen fixing cyanobacteria, both as free living and symbiotic forms, also contribute organic nitrogen to the general soil crust community (Bergman et al., 1997). Filamentous species such as Microcoleus are capable of binding together into rope-like structures that allows them to colonize physically unstable sedimentary environments (Garcia-Pichel and Wojciechowski, 2009). Some filamentous species also secrete exopolysaccharides that effectively aggregate soil particles and thus contribute to BSC structure and stability (Rossi and De Philippis, 2015). Terrestrial cyanobacteria are poikilohydric—tolerating severe desiccation as well as high levels of UV light (Karsten and Holzinger, 2014). The UV filtering capacity of terrestrial cyanobacteria is related to the presence of light screening compounds found in their cells and sheath material, often resulting in the characteristic dark color typical of many cyanobacteria-dominated BSC communities (Scherer et al., 1988; Rosentreter et al., 2007; Rastogi and Incharoensakdi, 2014). During winter months, the authors have anecdotally observed that the dark surface of BSC communities in the Great Basin tends to absorb winter sunlight, causing snow and ice to melt providing the BSC community with liquid water that in turn potentially supports brief but important periods of metabolic activity.
Reproduction in the cyanobacteria is strictly asexual (Mur et al., 1999) and may be accomplished by budding (Waterbury and Stanier, 1977), non-specialized thallus fragments (Meeks and Elhai, 2002), binary fission (Kunkel, 1984), or the formation of specialized asexual structures (Somon, 1977) including akinetes (Kaplan-Levy et al., 2010). Hormogonia—specialized thallus fragments commonly produced by some filamentous cyanobacteria (Campbell and Meeks, 1989; Meeks and Elhai, 2002) also function as effective asexual propagules. Cyanobacteria act as pioneer species while also commonly occurring in more mature BSC communities as both free living and symbiotic taxa.
Cyanobacteria have been found in BSC communities on all continents, and upwards of 50 taxa have been identified from regional samples of BSC communities (Rippin et al., 2018). Many terrestrial cyanobacteria have also been collected from the atmosphere (Sharma and Singh, 2010; Genitsaris et al., 2011) and are among the most numerous airborne microorganisms reported from aerial samples (Sharma and Singh, 2010; Després et al., 2012). In terrestrial environments, filamentous cyanobacteria are able to glide along thin layers of water coating soil particles when the soil is moist (Castenholz, 1982; Hoiczyk, 2000). However, in terrestrial environments, local dispersal distances are extremely limited (cm scale) compared to dispersal through the air (km or intercontinental scale). In Antarctica, cyanobacterial communities in close proximity to each other had a low degree of similarity with each other, indicating the probability of longer-distance aerial transport (Namsaraev et al., 2010).
Chemoheterotrophic Bacteria and Free-Living Diazotrophic Bacteria
Chemoheterotrophic bacteria and free-living diazotrophic bacteria commonly integrate and closely interact with other BSC organismal groups. They are, however, one of the least studied and understood constituents of BSCs. These bacteria reside in a nutrient-rich zone where cyanobacteria and other biocrust constituents create a “cyanosphere” (Couradeau et al., 2019) with relatively enhanced organic carbon, nitrogen, and water availability. Large numbers of soil heterotrophic bacteria are positively correlated with BSC stability (Andrade et al., 1998; Makhalanyane et al., 2015; Nunes da Rocha et al., 2015). Many heterotrophic bacteria are filamentous and/or produce exopolysaccharides (Crania et al., 2019), characteristics that contribute to the formation and stabilization of BSCs. The occurrence of these BSC bacteria are ultimately a product of multiple environmental filters (e.g., climate, soil type, and disturbance regimes Eldridge and Delgado-Baquerizo, 2019. Each crust type (cyanobacteria dominated, lichen-dominated, and bryophyte-dominated) exerts control over the bacterial phyla and families present (Maier et al., 2018). To begin to identify the higher-taxonomical convergence among BSC bacteria, other than cyanobacteria, we examined the patterns of abundant bacterial families in the three crust types (Table 1). Of the 24 dominant heterotrophic bacterial orders/families found to occur in BSC communities over eleven studies, nine were cosmopolitan taxa [i.e., present in all three biocrust types, Acidobacteriaceae (Acidobacteria), Rubrobacteraceae (Actinobacteria), Shingomonadaceae (Alphaproteobacteria), Bradyrhizobiaceae (Alphaproteobacteria), Rhodobacterales (Alphaproteobacteria) Chitinophagaceae (Bacteroidetes), Cytophagaceae (Bacteroidetes), Oxalobacteraceae (Betaproteobacteria), Chthoniobacteraceae (Verrucomicrobia)], while five were unique to either cyanobacteria-dominated [i.e., Solirubrobacterales (Actinobacteria), Burkholderiales (Betaproteobacteria), Trueperaceae (Deinicoccus-Thermus) or lichen-dominated crusts [i.e., Armatiomonadaceae (Armatimonadetes), Cystobacteraceae (Deltaproteobacteria)]. The Alphaproteobacteria contained the greatest number of families at five, followed by the Actinobacteria that housed four families or orders. The most commonly found taxon in cyanobacteria-dominated crusts was the Rubrobacteraceae (Actinobacteria), and Shingomonadaceae (Alphaproteobacteria) in lichen- and moss-dominated crusts. These patterns may serve as a starting point for a conversation to find novel community patterns among biocrust copiotrophs. In all BSCs, regardless of the type, heterotrophic bacteria exert significant influence over soil biogeochemistry and structure by regulating carbon and nitrogen cycling (Schimel and Schaeffer, 2012; Nelson et al., 2016). In addition, the production of exopolymeric compounds by many heterotrophic bacteria contributes to soil aggregation that has a direct influence on water infiltration and soil structure (Costa et al., 2018). The presence of free-living diazotrophs (N-fixing bacteria and archaea) within the cyanosphere (Couradeau et al., 2019) adds essential available nitrogen to predominantly nitrogen-limited desert soils. In Microcoleus vaginatus-dominated crusts, Couradeau et al. (2019) identified multiple heterotrophic diazotrophs associated with several taxa from the Alphaproteobacteria, Betaproteobacteria, and Gammaproteobacteria groups. Furthermore, in developing cyanobacteria-dominated BSCs several of the same diazotrophs were identified by Pepe-Ranney et al. (2016) using 15N-DNA SIP methods.
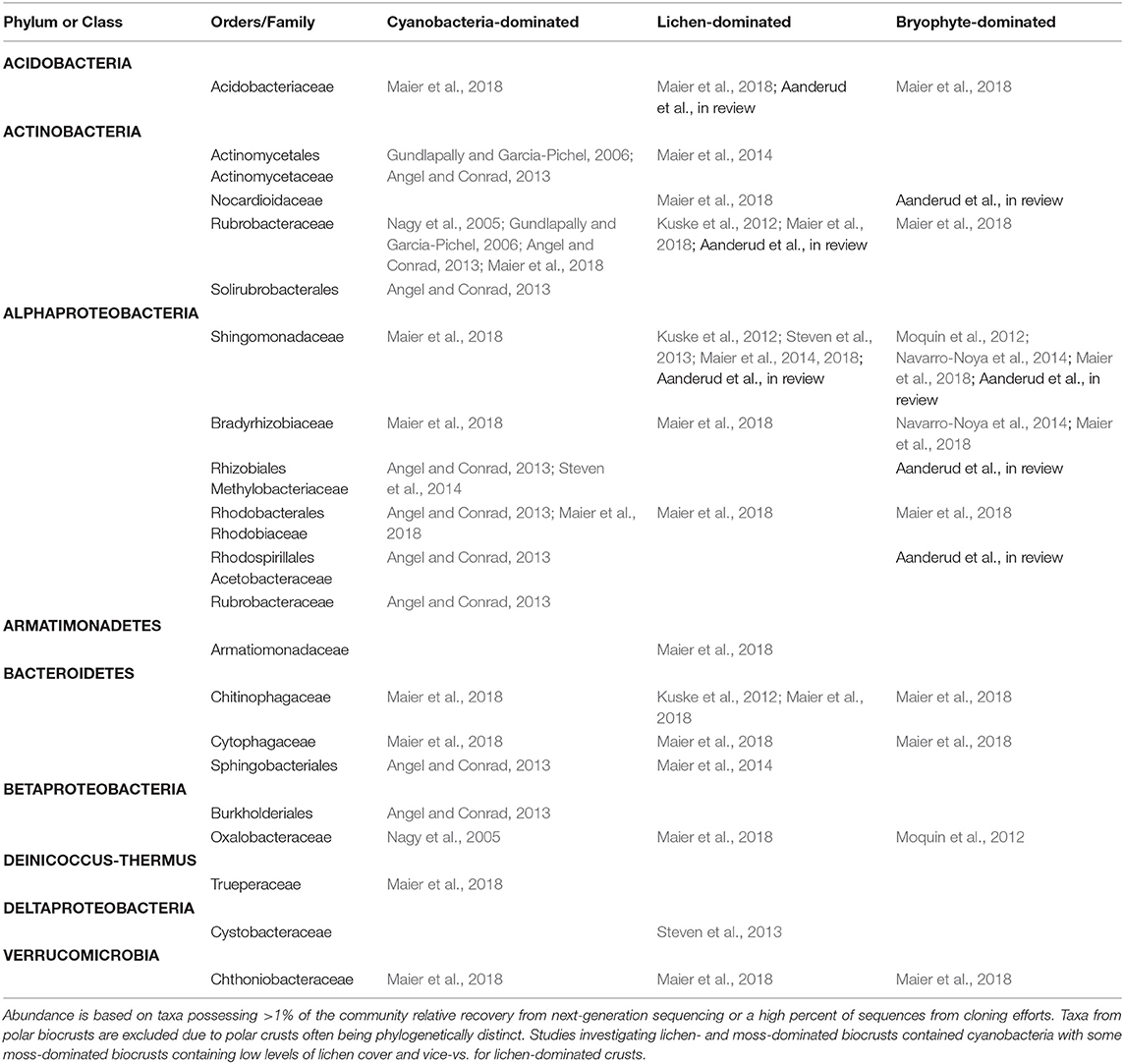
Table 1. Abundant chemoheterotrophic bacterial families/orders in cyanobacteria-dominated, lichen-dominated, and/or bryophyte-dominated biocrusts based on eleven studies.
Biological soil crust bacteria, like other soil bacteria, reproduce rapidly by binary fission when conditions are favorable. Otherwise, they enter a reversible state of reduced metabolic activity or dormancy. Dormancy establishes reservoirs of inactive individuals that resume metabolic and reproductive activity when appropriate environmental conditions return (Lennon and Jones, 2011; Joergensen and Wichern, 2018). Potentially, as much as 90% of the BSC microbial community is inactive with more than 50% of all bacterial taxa dormant during periods of stress (Alvarez et al., 1998; Lennon and Jones, 2011; Aanderud et al., 2015). Invariably, the single most limiting resource contributing to the onset of dormancy in the BSC community is soil moisture. For example, using H218O RNA-stable isotope probes in a cyanobacteria-dominated BSC community in Israel's Negev Desert, the rewetting of dry crusts caused bacteria to grow, resulting in the development of a distinctly different bacterial community with the previously dominant Actinobacteria population significantly reduced (Angel and Conrad, 2013). BSCs host a diverse bacterial community whose members grow, interact, and alter community composition depending on environmental conditions and activities that may impact BSC structure and health.
The primary dispersal mechanism for heterotrophic bacteria and diazotrophs within the BSC community is bioaerosols. Although BSCs generally stabilize desert soils against wind erosion, dust entrainment is the primary pathway for the movement of BSC bacteria from arid and semi-arid BSC communities. The various biological components of BSCs differ in their contributions and role in reducing soil erosion (Belnap and Gardner, 1993; Mazor et al., 1996; Bowker et al., 2008; Tisdall et al., 2012). Notwithstanding all the erosion benefits provided by BSCs, deserts remain a primary source of dust globally with desert winds aerosolizing several billion tons of soil-derived dust each year (Kellogg and Griffin, 2006). Local dispersal of bacteria occurs through the soil matrix but is extremely limited (at a cm scale) compared to dispersal through the air (intercontinental, at a km scale) (Choudoir et al., 2018). Dust from deserts may travel across continents providing an opportunity for BSC organismal groups to disperse across long distances. For example, dusty snow on Mont Blanc in the alps on the French and Italian border contained soil bacteria deposited by four Saharan desert dust storms over a 3-year period (Chuvochina et al., 2011). Similarly, dust from deserts in Chad were deposited in the Cape Verde Islands with many of the dust-borne bacterial families identical to families commonly reported for BSC communities (Favet et al., 2013). Dust contains an immense diversity of bacteria (Choudoir et al., 2018; Dastrup et al., 2018) and retains much of the diversity profile typical of soil surfaces (Boose et al., 2016; Weil et al., 2017; Dastrup et al., 2018). Dust also acts as a source of wind-borne propagules with dust particles settling in response to gravity or acting as ice nucleators enhancing snowfall (Christner et al., 2008). Dust entrainment from deserts is also enhanced by anthropogenic disturbance of soil surfaces (Belnap and Gillette, 1998). Disturbed BSC communities have been shown to serve as a source of inoculants (Warren et al., 2019), further enhancing dispersal bridges between crust communities or into open habitats—suitable for biocrust development.
Fungi
Three categories of fungi are reported for BSC communities including free-living fungi, lichenized fungi, and mycorrhizal fungi. All three groups of terrestrial fungi play important ecological roles in the establishment and maintenance of BSC communities.
Free-Living Fungi
Free-living fungi are eukaryotic heterotrophs that acquire energy and matter from other organisms (living or dead). They produce a wide range of enzymes that degrade chitin, keratin, cellulose, and lignin (Alexopoulos et al., 1996; Kendrick, 2017), substrates that are not readily degraded by other organisms. Fungi have the unique ability to digest/consume organic substrates while also releasing compounds that are often appropriated by other organisms. Moreover, fungi are also able to survive extreme environments such as temperature extremes, desiccation, pH extremes, etc. (Kendrick, 2017). Fungi in general are recognized for their role as decomposers, and their ability to withstand environmental extremes. They are now often included in the suite of microorganisms that comprise BSCs, primarily because of their contributions to the process of consolidating and stabilizing soil particles and aggregates (Caesar-TonThat and Cochran, 2001; States et al., 2001). Free-living fungi are found in greater abundance in well-developed BSCs than uncrusted neighboring soils (Maier et al., 2016). Although free-living fungi have been recognized as an important component of the BSC micro-community, research on free-living fungal communities in biocrusts has been limited and generally descriptive in nature (Bates and Garcia-Pichel, 2009; Bates et al., 2012; Steven et al., 2015). Ascomycota represents the predominant fungal phylum in BSCs (Maier et al., 2016). However, research has shown that the composition of BSC fungal communities differs based on soil types and geographical location (Reininger et al., 2015). In general, fungi produce vegetative filaments (hyphae) which bind soil particles together and help to consolidate the surface of BSC communities (Tisdall, 1991; Degens et al., 1996). More specifically, many of the major BSC free-living fungi in the Ascomycota are classified in the Dothideomycetes class of the Pleosporales order. Members of this group of fungi are called dark-septate endophytic fungi, due to the dark pigments commonly found in their cell walls. These pigments are usually melanin and mycosporine-like amino acids which provide protection in environments of severe desiccation and high levels of UV light (Oren and Gunde-Cimerman, 2007; Gostinčar et al., 2009). There are two types of melanin produced by melanized fungi: 1,8-DHN melanin and L-Dopa melanin (Henson et al., 1999). Dark-septate fungi have also been suggested as contributors to nutrient links between desert vascular plants and the BSC community (Maier et al., 2016). As a result, free-living fungi have been proposed as an important component in the fungal loop hypothesis that suggests that nutrient exchange and the vascular plant-BSC connection may be mediated by fungi (Collins et al., 2008; Green et al., 2008).
While almost all free-living fungi are capable of sexual reproduction, sex is costly (Aanen and Hoekstra, 2007), and most fungi generally reproduce using a variety of asexual mechanisms (Nieuwenhuis and James, 2016; Ojeda-López et al., 2018). Asexual reproduction may be accomplished by fission or budding of somatic cells, fragmentation of mycelial strands or hyphae, or production of asexual spores. All such processes result in haploid individuals. Because sexual reproduction is possible yet infrequent, outcrossing is, likewise, rare to infrequent (Nieuwenhuis and James, 2016; Ojeda-López et al., 2018). Indeed, sexual reproduction is infrequent in many fungi, yet self-fertility is relatively common (Lee et al., 2010). In BSCs, free-living fungi are generally classified based on their asexual reproductive structures and confirmed more specifically by limited observations of sexual fruiting bodies (Maier et al., 2016). BSCs are dominated by Dothideomycetes fungi (Steven et al., 2015) which are most often found in their anamorph (asexual) state and are recognized as filamentous molds when cultured in the laboratory. They reproduce asexually by producing non-motile conidia type spores that can be dispersed by wind or insects. Black yeasts are a second common morphotype encountered when culturing fungi from BSCs and encompasses two distinct fungal evolutionary lineages, Dothideomycetes and Chaetothyriales (Eurotiomycetes). The black yeasts are characterized by thick, melanized cell walls, and exopolysaccharide production that support a variety of extremophile properties that allow them to endure high UV radiation and extreme desiccation, while also contributing to the consolidation of soil particles. The black yeasts have only been documented to reproduce asexually through the production of conidia type spores and unicellular budding. Some species of black yeasts produce fission or budding divisions when grown in submerged environments while others are capable of producing conidiophores when growing in low moisture environments (Seyedmousavi et al., 2014).
Some free-living fungi produce specialized structures that forcibly eject thousands of spores into the air (Roper et al., 2010; https://www.anbg.gov.au/fungi/dispersal.html). Ascomycota are the dominant group of fungi in BSCs, and are also the dominant fungi found in aerobiota (Frölich-Nowoisky et al., 2016). Generally, fungal species, which rely on windblown dispersal, produce aerodynamically shaped spores that can be transported thousands of kilometers through the atmosphere (Golan and Pringle, 2017). Abundant and diverse numbers of fungal spores have been collected from the atmosphere above Antarctica (Marshall, 1997), Australia (Mitakakis and Guest, 2001), Brazil (Womack et al., 2015), Chile (Ibañez et al., 2001), China (Fang et al., 2005), India (Priyamvada et al., 2017), Iran (Shams-Ghahfarokhi et al., 2014), Italy (Sandrone, 2014), Kuwait (Halwagy, 1994), Mexico (Rosas et al., 1990), Nigeria (Ezike et al., 2016), Poland (Stȩpalska and Wolek, 2005), Spain (Sabariego et al., 2007), and the United States (Dupont et al., 1967), among others. The quantity and diversity of airborne fungal spores is seasonally variable (Reineria Diaz et al., 1998; Kasprzyk and Worek, 2006). Their function is dispersal followed by growth and development of vegetative structures when essential resources and ideal environmental conditions are available (Kendrick, 2017). Due to the arbitrary nature of wind dispersal, spores may not encounter conditions suitable for germination and viability. To increase their chances of reproductive success, many fungi produce and release an “over-abundance” of spores throughout the year to ensure that a sustainable number encounter a suitable environment with essential resources to accommodate germination and establishment (Kendrick, 2017). The regional production of spores is dependent on the availability of water and local spore trapping show a cyclical pattern dependent on moisture patterns. Global dispersal of fungal spores is facilitated by dust-borne transport that in turn supports proliferation processes (Shinn et al., 2003).
Lichenized Fungi
Lichenized fungi include more than 19,000 species (Lücking et al., 2016, 2017). Lichens are composite systems involving complicated symbiotic interactions between heterotrophic fungi primarily of the phylum Ascomycota or less frequently of the phylum Basidiomycota, and a green alga and/or a cyanobacterium (Brodo et al., 2001). The fungus, or mycobiont, provides structure and a favorable living environment in the form of a thallus or vegetative plant-like body undifferentiated into true stems, roots, and leaves, and lacking a vascular system. The green alga and/or cyanobacterium photobionts provide a carbon-based food source for themselves and the mycobiont through photosynthesis. In those cases where the photobiont is a cyanobacterium, it also fixes atmospheric nitrogen. Lichen scientific names are based strictly on the fungal symbiont, which can cause some confusion when trying to determine if a BSC fungus is lichenized or not—unless an intact lichen thallus is observed or collected and identified by a trained lichenologist. Lichens are broadly classified based on their thallus morphology as being crustose, squamulose, foliose, or fruticose. Crustose lichens are crust-like. They are slow-growing and adhere tightly to the substrate or, in some cases, are physically integrated into the surface of the substrate (Armstrong and Bradwell, 2010). Squamulose lichens are composed of tightly clustered or overlapping scale-like structures (squamules) with no or only a poorly developed lower surface. However, squamules of some squamulose species form rhizoidal hyphae on their lower surface that effectively anchor the squamules to the soil surface. Squamulose lichens constitute one of the most common growth forms associated with BSCs (St. Clair et al., 1993). Foliose lichens are leaf-like, with distinctive upper and lower surfaces and are generally loosely attached to the substrate by root-like structures called rhizines (Brodo et al., 2001). Fruticose lichens are highly branched and are either upright and shrubby or pendant with a single point of attachment (Baron, 1999). BSC lichen dominated communities tend to be more common in undisturbed locations with well-developed vertical BSC structure (Rosentreter et al., 2007). Some BSC lichens, mostly foliose forms referred to as “vagrant lichens” (e.g., the lichen genera Xanthoparmelia and Rhizoplaca), occur unattached on the soil surface (Rosentreter, 1993). Generally, some of the more common BSC lichen genera include Psora, Placidium, Xanthocarpia, Lecanora, Circinaria, and Acarospora. Lichens have a broad distribution, occurring on a variety of substrates and occupying habitats ranging from the humid tropics to hyper-arid zones, and from the frigid tundra to hot deserts. When dry, they suspend all metabolic activity and are able to tolerate extremely high temperatures for extended periods of time. Lichens occur on a variety of substrates, including soil, rocks, monuments, statues, roofs, walls, fences, bark, and decorticated wood (St. Clair and Seaward, 2004). Lichens contribute to the weathering of stone substrates by producing weak organic acids (Chen et al., 2000; Souza-Egipsy et al., 2004; Leger and Forister, 2009). Lichens are desiccation-tolerant allowing them to inhabit very harsh environments and experience drought conditions for extended periods (Nash et al., 1990; Kranner et al., 2008).
Some lichens reproduce asexually using unspecialized thallus fragments (Armstrong, 2017; Almeida Pereira et al., 2018) or through the formation of small-specialized asexual structures containing cells of both the mycobiont and the photobiont. These specialized asexual structures include soredia, isidia, and lobules (Ott et al., 1993). Soredia are small, spherical bundles of algal cells wrapped in a mesh of fungal hyphae. Soredia frequently occur as powdery patches (soralia), which erupt through the upper surface (cortex) of some lichens. Isidia are specialized thallus fragments of various shapes and configurations attached to the upper surfaces of lichens. Like soredia, isidia contain both algal cells and fungal hyphae; however, unlike soredia the surfaces of isidia are encased in a layer of cortical cells. Lobules are small, flattened, variously shaped structures that generally occur along the edges of the thallus of some lichens. These specialized asexual regenerative structures are easily detached from the thallus surface by wind, rain, or other physical disturbance (Blackwell et al., 1996) and may subsequently be dispersed to a new suitable substrate where they become attached and form a new lichen thallus. Lichens commonly reproduce sexually as well; however, sexual reproduction in lichens is limited to the fungal partner, which produces sexual spores called ascospores within fruiting bodies called ascocarps. The photobiont (alga or cyanobacterium) is also capable of sexual reproduction, but, unlike the fungus, not while engaged in the lichen symbiosis. The primary limitation to sexual reproduction in lichens is the obstacle of the germinating ascospore encountering a viable photobiont partner in the process of attempting to reconstitute a new lichen thallus (Bowler and Rundel, 1975). However, the fact that many broadly distributed lichen species reproduce only sexually through the production of ascospores suggests that the likelihood of an ascospore germinating and surviving long enough to encounter an appropriate photobiont happens with impressive frequency. Though little is known about the dispersal of lichen gametes, the gametes of the lichen-forming fungus, Lobaria pulmonaria, are known to have an aerial dispersal range of several hundred meters to kilometers from mature individuals. On the other hand, clonal propagules (i.e., soredia and isidia) disperse over a distance of tens of meters while spores may be dispersed for several kilometers (Ronnås et al., 2017). However, joint dispersal of the mycobiont and photobiont does not necessarily imply that the two will be rejoined (Wornik and Grube, 2010). Indeed, they may switch symbiotic partners (Ertz et al., 2018).
Lichen thalli, are readily dispersed into adjacent and expanded habitats as both sexual and asexual propagules. Dispersal of either non-specialized or specialized thallus fragments over significant distances is accomplished by wind, water, or as attached to animal feathers, fur, or feet (Bailey, 1966). Researchers in northeastern Germany, a region not known for high winds, found that the dispersal distance of lichen fragments was negligible, and was measured in centimeters (Heinken, 1999). In contrast, areas where high surface winds are more common, lichen thallus fragments, detached reproductive structures (soredia, isidia, lobules), and spores can be lifted into the atmosphere and transported very long distances (Després et al., 2012). For example, lichen soredia have been collected at high altitudes in southwest Spain (Tormo et al., 2001) and Antarctica (Marshall, 1996). Airborne asexual lichen fragments have been collected from rooftops throughout the continental United States (Tripp et al., 2016). On an ocean voyage from the Polish Antarctic Station on King George Island by way of South Shetland Island to Gdynia, Poland, scientists intended to collect pollen grains daily on blotter paper. In addition to pollen, the scientists commonly found fungal spores, lichen thallus fragments, isidia, and soredia in abundance in all samples, indicating widespread distribution of airborne lichen propagules (Harmata and Olech, 1991). The abundance and distribution of fungal spores vary by species and climate (Marshall, 1997). The lichen genus Ramalina is found on the main island of New Zealand, as well as the outlying islands of New Zealand (Bannister and Blanchon, 2003), suggesting long-distance dispersal. The foliose lichen genus Xanthoparmelia, purportedly originated in South Africa, but is now present in South and North America as well as Australia (Amo de Paz et al., 2012).
Mycorrhizal Fungi
Mycorrhizal fungi are obligate plant root symbionts that provide their host plants with increased water and nutrient absorption capacity while the plant provides the fungus with carbohydrates from photosynthesis (Berruti et al., 2015). The roots of more than 80% of terrestrial plant species are obligatorily associated with mycorrizal fungi (Pirozynski, 1981; Smith and Read, 2008). In a mycorrhizal association, the fungus colonizes the host plant's roots, either intracellularly as in endomycorrhizal fungi or extracellularly as in ectomycorrhizal fungi. In endomycorrhizal fungi, the fungal hyphae penetrate the cortical layer of cells below the epidermis of the plant root. Some endomycorrhizal fungi form an intercellular multi-branched hyphal structure with slightly swollen chambers to store nutrients. Such a structure resembles a tree. Hence, such endomycorrhizal fungi are often referred to as vesicular mycorrhizae or vesicular-arbuscular mycorrhizae, or simply VM or VAM for short. All vesicular or vesicular-arbuscular mycorrizae are formed by members of the Glomeromycota phylum of the kingdom fungi (Kehri et al., 2018). There is a trend toward host-specificity among mycorrhizal fungi (Torrecillas et al., 2012) such that mycorrhizal fugal diversity can be used as an index of plant biodiversity in similar ecosystems (van der Heijden et al., 1998). Soils with vesicular arbuscular mycorrhizal fungi have significantly more stable aggregates than soils without (Andrade et al., 1995). The mycelia of mycorrhizal fungi can extend several centimeters beyond the plant roots, forming a mycelial mesh that binds soil particles (Miller and Jastrow, 1992; Soka and Ritchie, 2014). The mycelia also produce a glue-like glycoprotein, glomalin, that functions as an adhesive that binds soil particles together thus reducing soil erosion (Gianinazzi et al., 2010; Singh, 2012; Gomathy et al., 2018; Prasad et al., 2018), while enhancing the structural integrity of the BSC community (Chaudhary et al., 2009).
Sexual reproduction in Glomeromycota is unknown (Pawlowska, 2005). Hence, reliance on asexual modes of reproduction is essential. Typically, mycorrhizal fungi reproduce by asexual spores (Camargo-Ricalde, 2002; Marleau et al., 2011) or by hyphal fragments or plant roots containing mycorrhizal hyphae (Berruti et al., 2014).
Dispersal of mycorrhizal fungi is facilitated by the activity of burrowing insects, rodents as well as birds that disperse spores or hyphal fragments for short distances or expose them to the erosive forces of water and wind (Camargo-Ricalde, 2002) that can move them much farther. Recent research has documented large numbers of airborne mycorrhizal spores in a variety of different biomes and ecoregions in North America where they were previously unknown (Egan et al., 2014). Spores were most frequent in the air above more arid ecosystems, suggesting that they are more likely dispersed aerially from those systems. Efforts to detect and identify airborne mycorrhizal propagules is often difficult and tends to under-report some fungal taxa. The use of molecular approaches such as DNA metabarcoding, however, can uncover fungal diversity missed by traditional morphological techniques including microscopy (Pashley et al., 2012; Chen et al., 2018), thus providing a more comprehensive estimate of fungal diversity in aerial samples (Banchi et al., 2018).
Terrestrial Algae
Terrestrial eukaryotic algae of BSC communities include green algae, diatoms, xanthophytes, and eustigmatophytes. Like cyanobacteria, eukaryotic algae are photoautotrophic, using energy from sunlight to synthesize energy-rich organic compounds. Unlike cyanobacteria, they possess well-defined, membrane-bound, subcellular structures (e.g., nuclei, mitochondria, etc.) and may reproduce sexually. Although eukaryotic algae are usually aquatic, many species inhabit the soil, and participate in the formation of BSCs. Here we focus specifically on two groups of eukaryotic algae that are known to contribute to the structure of BSCs, namely, the green algae and diatoms.
Green Algae
Green algae of BSCs include free living and lichenized species, and most are unicellular, colonial, or filamentous in morphology. Several classes of green algae (Chlorophyceae, Trebouxiophyceae, Ulvophyceae, Klebsormidiophyceae, and Zygnematophyceae) including dozens of genera are found in BSC communities. In many cases, species of BSC green algae are closely related to aquatic sister species found in freshwater habitats, suggesting that the evolutionary transition to BSC habitats has happened many times in the green algae (Lewis and Lewis, 2005; Fučíková et al., 2014). As a group, green algal cell walls are highly diverse and include cellulose as well as other polysaccharides, and glycoproteins (Domozych and Domozych, 2014). Under certain conditions, filamentous green algae (e.g., Klebsormidium) may achieve high biomass and contribute to the consolidation of soil particles in BSCs due to their sticky polysaccharide-containing cell sheaths and exudates (Hoppert et al., 2004; Warren, 2014; Büdel et al., 2016). Other green algae may have lower biomass, but they also may produce sheath material that directly adheres to cyanobacteria, fungi, and other green algae (Hoppert et al., 2004). Hu et al. (2003) characterized the vertical profile of algae in a BSC “horizon,” intricately mapping the physical locations of green algae and other species. Green algae typically are distributed just below the surface of desert crusts, but they are known to form thick mats at the soil surface in other types of BSC communities (Hu et al., 2003; Hoppert et al., 2004). Some green algal taxa also live symbiotically as lichen photobionts and are typically distributed just below the surface of lichen thalli, their cells protected by fungal sunscreens (Nguyen et al., 2013).
Among BSC green algae, propagules include zygotes, asexual spores, or filament fragments. In nearly all cases, the green algae of BSC are small, unicellular, with dominant haploid vegetative stages. They can reproduce sexually, or asexually by fragmentation of filaments or simple cell division, by autospores (non-motile cells formed inside the mother cell wall), and through zoospores (flagellated cells). In aquatic relatives of BSC green algae, the diploid stage (zygote) is considered the stage that persists under conditions not supporting active growth (e.g., lack of water, freezing temperatures). For example, in temperate regions, the zygotes of pond algae persist in sediments over winter, and in the spring they undergo meiosis directly to form haploid vegetative cells. Likewise, if zygotes were formed by BSC green algae, they also could potentially serve as resistant aerial propagules. Motile cells have been documented in BSC species (Flechtner et al., 2013) but it is not clear if these represent gametes or zoospores because these stages often are morphologically similar. Sexual reproduction is difficult to demonstrate in unicellular terrestrial green algae unless the fusion of motile gametes is observed directly or zygotes are observed. Sex is difficult to induce even in the laboratory, and generally is not tested in cultured algae. It is also impossible to detect directly in field-collected material. That said, sexual reproduction is thought to be possible in these species, even if rare. Support for this is indirect, coming from surveys of genomic and transcriptomic data for meiosis genes in diverse eukaryotes, which are present in many trebouxiophycean genera, including terrestrial taxa (Fučíková et al., 2015). However, compared to algae living in aquatic habitats the opportunities for sexual reproduction in BSC taxa is greatly constrained because green algae require liquid water for their swimming gametes and because green algae in BSCs often are not present in high densities.
Green algae of BSCs do not necessarily need specialized spores or zygotes to disperse. Reproductive propagules of unicellular and multicellular eukaryotic algae are mostly dispersed aerially (Brown et al., 1964; Broady and Smith, 1994; Tesson et al., 2016). Genitsaris et al. (2011) documented 353 morphological taxa that have been reported in the literature related to aerobiology, with more reported regularly. The fact that new, cryptic species are being discovered and described (Cardon et al., 2008) suggests that additional species will be added to the list of aerobionts. Many algae occur in both the Arctic and Antarctic polar regions demonstrating a significant capacity for global long-distance dispersal (Jungblut et al., 2012). In addition to their occurrence in BSCs, airborne algae may also be deposited and survive on a variety of other substrates, including tree bark (Kharkongor and Ramanujam, 2014), glaciers and snow fields (Takeuchi, 2001; Kvíderova, 2012), polar fellfields (Marshall and Chalmers, 1997), and exterior building walls (Nakajima et al., 2015). In fact, the ability of vegetative cells to survive without water can be immediate. Hydrated, actively growing cells from diverse genera of green algae have been shown to survive rapid desiccation (Gray et al., 2007; Cardon et al., 2008) indicating the presence of mechanisms that prepare even actively growing cells for desiccation. In other species, the ability to survive is a function of the age of vegetative cells. For example, compared to young cells of the alpine taxon Zygnema that are tolerant to exposure to high UV-B stress (Holzinger et al., 2018) and Herburger et al. (2015) demonstrated that older vegetative cells (akinetes) are more tolerant to desiccation. Such stages likely are important to the ability of some species to survive long-range dispersal. To be part of the aerial flora, and in order to successfully colonize BSC habitats after dispersal, algae need to produce structures that can be lifted and moved in the air, and they need traits that enhance survival under drying conditions (Sharma and Singh, 2010). These algae exhibit the hallmarks of poikilohydry, by equilibrating to ambient humidity and having rapid responses upon water gain [reviewed in Green and Proctor (2016)]. Green algae, like cyanobacteria, and other photosynthetic species must also be able to survive in high light and possibly high UV habitats (Karsten and Holzinger, 2014), allowing them to survive in harsh, high elevation terrestrial habitats and during transport, deposition, and settlement while airborne (Tesson et al., 2016). Protective compounds including carotenoids, mycosporine-like amino acids, and a variety of phenolics are known in green algae (Kitzing et al., 2014; Holzinger et al., 2018). Diverse airborne green (and other) algae were noted by early researchers including van Overeem (1937) and Brown et al. (1964), with a recent review by Tesson et al. (2016). Given that BSC green algae are so diverse, and that different reproductive stages can survive dispersal, it is reasonable to assume that the underlying mechanisms that allow these species to live in habitats supporting BSCs may also vary (Holzinger and Karsten, 2013). While airborne, microalgae are tolerant to freezing temperatures and desiccation and are important ice nucleators contributing to the formation of ice and snow (Tesson and Šanti-Temkiv, 2018).
Diatoms
Diatoms, the second group of eukaryotic algae included in this section are commonly found in BSC communities (Ettl and Gärtner, 1995; Büdel et al., 2016). Diatoms are species diverse and best known from aquatic habitats, often being in high abundance in both marine and freshwater communities. However, several groups of diatoms include terrestrial species known to occur in damp soils or ephemeral aquatic habitats, as well as more arid habitats. BSC diatoms are phylogenetically diverse but are dominated by pennate species from at least four taxonomic orders. On a broad geographical scale, diatoms occur in diverse BSC habitats on all continents (Sharma et al., 2007; Souffreau et al., 2013a; Büdel et al., 2016), and many of the same species have been found on different continents, indicating that diatoms can be transported great distances (Souffreau et al., 2013a). More locally, diatom diversity in BSCs can be high, with upwards of 50 species reported (Borchhardt et al., 2017). Unlike green algae, whose walls are carbon-based, diatom cell walls are made of silicon dioxide. Often pennate diatoms, typical of many BSC species, have a slit-like channel (raphe) along the length of the cell that produces carbon-based mucilaginous exudates that accommodate motility (Edgar and Pickett-Heaps, 1982). These mucilaginous exudates also likely contribute to soil aggregation and stabilization in BSC habitats. Motile diatoms exhibit vertical movement in BSCs, in response to light and water availability (Hu et al., 2003). A significant diversity of diatom vegetative cells isolated from damp soils have been shown to be tolerant of wide temperature fluctuations (both heating and freezing), but they were not tolerant of desiccation. On the other hand, resting stages of terrestrial diatoms were resistant to periods without water (Souffreau et al., 2010, 2013b). This ability would allow for aerial transport, and a number of viable diatoms have been detected in airborne samples (Brown et al., 1964; Sharma et al., 2007). However, the precise mechanisms used by diatoms to tolerate periods of desiccation are not well-known.
Diatom vegetative cells including other cell types relevant to dispersal and perennation (e.g., auxospores) are diploid (2n). Asexual reproduction in diatoms involves mitotic divisions of vegetative cells (Mann, 1993). Initiation of sexual reproduction is directly related to the size of vegetative cells and is typically triggered when vegetative cell size has been reduced through repeated mitotic cell divisions, to a minimum size—usually smaller than half of the normal size for most species (Edlund and Stoermer, 1997). This overall reduction in cell size, through repeated mitotic divisions, is due to the fact that as vegetative cells divide the overlapping cell halves (valves or frustules) separate with both the top (larger) and bottom (smaller) halves regenerating a new bottom (smaller) half. The specific processes associated with sexual reproduction differs between the two main groups of diatoms—centric and pennate forms. However, the outcome is similar for both groups—production of a diploid structure—the auxospore that eventually restores the vegetative cell to the normal size and form (Kaczmarska et al., 2001). With subsequent mitotic cell divisions again reducing the size of future generations of vegetative cells to a point that sexual reproduction is triggered with meiosis producing gametes, by either oogamy or isogamy depending on the species. Gametes subsequently fuse to form the next generation of diploid auxospores (Mann, 1993).
Aquatic diatoms are generally dispersed by water currents. However, terrestrial diatoms (e.g., vegetative cells and auxospores) are typically dispersed through the atmosphere under very dry conditions (Sharma and Singh, 2010; Aguilera et al., 2018). Research has shown that diatoms can reach the atmosphere in various ways including volcanic eruptions (Pike, 2013; Van Eaton et al., 2013) and dust and sand storms (Griffin et al., 2002). Airborne diatom propagules have been collected by various methods from a variety of locations (Holzapfel, 1978; Vanormelingen et al., 2008; Pearce et al., 2016; Tesson et al., 2016).
Bryophytes
BRYOPHYTES are small spore-producing plants with a dominant and normally persistent gametophyte and include mosses, liverworts, and hornworts. Although without true xylem, many species of mosses are capable of effectively conducting water both endohydrically (in specialized water conducting tissues containing xylem precursors) and ectohydrically by quickly wicking up liquid water along external cell surfaces, often in a matter of seconds from the substrate into the shoot apices (Glime, 2017). Bryophytes, similar to lichens, are poikilohydric, whereby their tissues are capable of exchanging water vapor from ambient air and equilibrating their tissue water content with the water potential of the surrounding air (Glime, 2017). Most bryophytes differ from lichens in being unable to fully activate metabolism using just water vapor, although imbibing water vapor while yet desiccated acts to mitigate desiccation damage to tissues (Pardow and Lakatos, 2013; Slate et al., 2018). Nevertheless, carbon balance can be positive and recovery complete in some mosses without the addition of liquid water (Lange, 1969; Lakatos, 2011). Between the three groups, bryophytes comprise some 20,000 species (Shaw et al., 2011). Although there is a common misconception that bryophytes occur only in damp, shady environments, some species occur in hot, dry environments, or dry polar environments (McCleary, 1959; Scott, 1982). With the exception of the liverwort genus Cryptothallus, virtually all bryophytes contain chlorophyll and are photosynthetic. Cryptothallus has no chlorophyll and gets its photosynthates indirectly from a host tree via a symbiotic relationship with a saprophytic fungus of the Basidiomycota (Wickett and Goffinet, 2008). Most bryophytes are capable of surviving desiccation during rainless periods (Proctor et al., 2007) and desiccation tolerance even extends to antheridia and male gametes (Shortlidge et al., 2012; Stark et al., 2016b), embryonic sporophytes, and asexual propagules (Brinda et al., 2016; Stark et al., 2016a). Upon addition of liquid water, shoots become metabolically active (Harten and Eickmeier, 1987) and require about 24 h to recover in most instances (Coe et al., 2014). Bryophytes are able to anchor themselves to the ground or to other suitable substrates with slender root-like rhizoids that occur on the underside of the thallus, bases of shoots, or when protonemata become subterranean (Odu, 1978; Jang et al., 2011; Glime, 2017).
Bryophytes may reproduce sexually and asexually and these modes may co-occur in time. Although dependent upon liquid water for gamete transfer, in deserts selection has likely favored monoecious over dioecious (monoicy over dioicy) reproduction (Wyatt, 1982). A significant number of dioecious BSC mosses exist, and some of the more common species may (e.g., Bryum argenteum) or may not (e.g., Syntrichia caninervis, S. ruralis) produce aboveground specialized asexual propagules. Such asexual propagules may be cryptic and only produced during periods of abundant moisture, as in the protonemal gemmae of S. caninervis, Funaria hygrometrica, and B. argenteum (Glime, 2017). One of the most successful and widespread BSC species, B. argenteum, is capable of producing not only abundant spore capsules when both sexes are present, but also two kinds of gemmae (bulbils and protonemal gemmae). While the spores of B. argenteum are dispersed by air currents, their gemmae float and are likely instrumental in short distance dispersal by water. In addition to spores and propagules, probably all BSC bryophyte species commonly disperse by fragmentation of the plant body, the cells of which are totipotent (Glime, 2017). In their sexual life cycle bryophytes produce multicellular haploid individuals (gametophytes) which produce gametes through mitosis (Glime, 2017). Male and female gametes then fuse to form diploid individuals (sporophytes) which remain attached to the gametophytes and produce haploid spores by meiosis that in turn divide by mitosis to produce the multicellular haploid gametophyte generation—thus completing the bryophyte life cycle (Glime, 2017). Sexual reproduction in many bryophytes is uncommon in dry environments due to the paucity of sexual spores or the rarity of male plants (Bowker et al., 2000; Stark et al., 2000; Horsley et al., 2011). Asexual reproduction in bryophytes is far more prevalent than sexual reproduction (During and van Tooren, 1987; Hugonnot and Celle, 2012) and generally regarded as the primary avenue of species dispersal (Mishler, 1988). Genetic diversity of at least some BSC bryophyte species can remain high regardless of whether reproduction is sexual or asexual (Paasch et al., 2015). Asexual reproduction is more common in mosses (Pohjamo and Laaka-Lindberg, 2003) whereas sexual reproduction is more common in liverworts (Peñaloza-Bojacá et al., 2018).
Some bryophyte species are capable of producing millions of meiospores from individual sporophyte capsules or from a single m2 (Longton, 1997; Australian National Botanic Gardens, 2016). However, the release of aerial propagules and particles can vary significantly over the period of a year based on weather conditions (Barbé et al., 2017), including wind speed and turbulence (Johansson et al., 2014). Aerial dispersal of bryophyte propagules is common (Flø and Hågvar, 2013) and long-distance dispersal rates may exceed shorter-distance dispersal (Lönnell et al., 2012). Long-distance aerial dispersal of diaspores (spores, gemmae, and/or leaf fragments) have all been reported (Laaka-Lindberg et al., 2003). Trans-oceanic and intercontinental dispersal is common (van Zanten, 1978; Shaw et al., 2011) and contributes to the intercontinental and bipolar distribution of species (Piñeiro et al., 2012; Lewis et al., 2014; Biersma et al., 2017). Such long-distance dispersal is now regarded as explanatory for the relative rarity of narrow endemics among bryophytes and other spore-bearing organisms (Shaw and Goffinet, 2000) when compared to seed plants. Gene flow through occasional long-distance dispersal events apparently prevents the evolution of, for example, sister species on different continents. The apparent lack of connectivity of populations is due in large part to the lack of environmental uniformity. Although propagules may be widely dispersed, not all end up in a climate or on a substrate conducive to their survival. Desert soils of the world are often dominated by a relatively few, widely distributed species of bryophytes. These are characterized by strongly developed traits of desiccation tolerance, whereby a constitutive or strongly inducible ecological strategy of desiccation tolerance has evolved (e.g., the genera Syntrichia, Tortula, Bryum, Crossidium, Aloina, Pterygoneurum). Interestingly, an inducible strategy of tolerating desiccation characterizes some of the common arid land mosses in the latter three genera. Such strategies enable the gametophyte shoots of mosses to tolerate rapid or slow rates of drying to the very low equilibrating relative humidity typical of desert habitats. Even so, the life phases of bryophytes exhibit a range of desiccation tolerance, with protonema and juvenile structures normally less resistant to desiccation (Greenwood et al., in review). In Janice Glime's E-book chapter on diaspore dispersal in bryophytes (2017), a number of studies based on several species and lines of reasoning support the idea of long-distance transport of diaspores that in turn lends credibility to the Baas-Becking hypothesis that “everything is everywhere and the environment selects.” Briefly, bryophyte species disperse farther than vascular plants, bryophyte species are generally more widely distributed than those of vascular plants, and the large numbers of disjunct bryophyte taxa between distant regions align with long-distance dispersal as the most supported process underlying these facts. The Baas-Becking hypothesis has been demonstrated for at least two bryophyte species, Bryum argenteum and Plagiobryum zierii, and also for the Sphagnum flora on an archipelago in the Baltic Sea. In addition, her review determined that spore size differences (small vs. large) does not inhibit dispersal, and that trans-continental spore dispersal is occurring. The Sphagnum spore rain is significant and thermal updraft and wind are significant factors controlling the dispersal of spores. Both updrafts and smoke from fires also likely facilitate spore dispersal. In the species Discelium nudum, evidence suggests that the majority of spores “escape the parent colony to travel greater distances” on the order of at least kilometers. Such evidence is consistent with the life history of many bryophyte species. Further, van Zanten (1976, 1978) and van Zanten and Pocs (1981) document spore survival under conditions typical of high altitude atmospheric air currents (i.e., desiccation, wetting, and freezing) and provide evidence that bryophyte spores can enter the jet stream and travel great distances, adding to other studies indicating that spores may be recovered in rainwater. Multiple genetic studies support a long distance dispersal hypothesis. Bryophyte spores are capable of surviving in the desiccated state for decades. Comparisons of spore desiccation tolerance and frost resistance between transoceanic and endemic species support long distance dispersal patterns, and the spore wall chemical composition suggests high survival capability befitting long distance transport.
Summary And Discussion
Biological soil crust communities include a variety of organisms that occupy the surface layer of the soil and consolidate soil particles and aggregates into a distinguishable stable crust resistant to the erosive forces of wind and water. The most common and abundant BSC organismal groups include—cyano-, heterotrophic, and diazotrophic bacteria, free-living, lichenized, and mycorrhizal fungi, eukaryotic algae including diatoms, and bryophytes. Historically, BSCs have been most noticeable and researched in arid and semiarid environments where the vascular plant community tends to be dominated by smaller shrubs with open inter-shrub spaces that offer minimal competition for direct sunlight and provide for more equitable distribution of essential resources. BSCs are becoming more recognized in humid environments, including the humid tropics; particularly, where disturbance has temporarily removed vascular plants that normally compete for sunlight and other essential resources at soil surfaces.
Herein, we provide a thorough discussion of the various modes of reproduction and dispersal employed by the core BSC organismal groups. Modes of reproduction vary between the organismal groups but generally include both sexual and asexual alternatives, although asexual options (e.g., fission, budding, fragmentation, etc.) are far more prevalent among BSC organisms. Following successful reproduction, sexual, and/or asexual propagules must be dispersed to new, suitable environments in order to avoid competition with established local BSC communities. Almost universally, BSCs are dispersed aerially, often for very long distances, sometimes intercontinentally and interhemispherically (Muñoz et al., 2004; Sharma and Singh, 2010; Smith et al., 2013, 2018; Herbold et al., 2014; Maki et al., 2019; Warren et al., 2019).
In spite of the extensive, research concerning arid BSC communities over the last 50 years there are still areas of research that need additional work. Specifically, some of the organismal groups are poorly known—particularly in terms of species diversity and ecological roles. For example, very little is known about the diversity and ecological roles of both the free-living and mycorrhizal fungi associated with BSCs. More work is also needed in terms of better understanding the community and ecosystem dynamics of BSCs. This paper provides important background information about the occurrence, reproduction, and dispersal of the various core organismal groups that contribute to the development and maintenance of BSC communities. However, effective application of modern molecular and microenvironment sampling tools will be pivotal in answering key questions about the diversity and functional attributes and relationships within BSC communities.
Author Contributions
SW and LLS initiated, organized, and directed the endeavor. They jointly composed the section on cyanobacteria. SW composed the section on mycorrhizal fungi. LLS composed the section on lichenized fungi. LRS composed the section on bryophytes. LL composed the section on terrestrial algae. JS, NP, and TK composed the section on free-living fungi. ZA composed the section chemoheterotrophic bacteria.
Funding
LRS contributed to publication fees for this article via the UNLV University Libraries Open Article Fund. Funding for publication was contributed by LLS via the Monte L. Bean Museum, by grant number DEB-1036448 from the National Science Foundation to LL, and by USDA-National Institute of Food and Agriculture Hatch project CA-R-PPA-5062-H to JS. NP was supported by a Royal Thai government fellowship.
Conflict of Interest
The authors declare that the research was conducted in the absence of any commercial or financial relationships that could be construed as a potential conflict of interest.
References
Aanderud, Z. T., Jones, S. E., Fierer, N., and Lennon, J. T. (2015). Resuscitation of the rare biosphere contributes to pulses of ecosystem activity. Front. Microbiol. 6:24. doi: 10.3389/fmicb.2015.00024
Aanen, D., and Hoekstra, R. (2007). “Why sex is good: on fungi and beyond,” in Sex in Fungi, eds J. Heitman, J. Kronstad, J. Taylor, and L. Casselton (Washington, DC: ASM Press), 527–534.
Aguilera, Á., de Diego-Castilla, G., Osuna, S., Bardera, R., Mendi, S. S., Blanco, Y., et al. (2018). “Microbial ecology in the atmosphere: the last extreme environment,” in Extremophilic Microbes and Metabolites Diversity Bioprospecting and Biotechnological Applications, eds A. Najjari, A. Cherif, H. Sghaier, and H. I. Ouzari (IntechOpen Online Open Access Books). doi: 10.5772/intechopen.81650
Almeida Pereira, T., de Oliveira Passos, P., Alves dos Santos, L., Lücking, R., and da Silva Cäceres, M. E. (2018). Going extinct before being discovered? New lichen fungi form from a small fragment of the vanishing Atlantic rainforest in Brazil. Biota Neotropica 18:e20170445. doi: 10.1590/1676-0611-bn-2017-0445
Alvarez, C. R., Alvarez, R., Grigera, S., and Lavado, R. S. (1998). Associations between organic matter fractions and the active soil microbial biomass. Soil Biol. Biochem. 30, 767–773. doi: 10.1016/S0038-0717(97)00168-5
Amo de Paz, G., Cubas, P., Crespo, A., Elix, J. A., and Lumbsch, T. (2012). Transoceanic dispersal and subsequent diversification on separate continents shaped diversity of the Xanthoparmelia pulla group (Ascomycota). PLoS ONE 7:e39683. doi: 10.1371/journal.pone.0039683
Andrade, A., Azcón, B., and Bethlenfalvay, G. (1995). A rhizobacterium modifies plant and soil responses to the mycorrhizal fungus Glomus mosseae. Appl. Soil Ecol. 2, 195–202. doi: 10.1016/0929-1393(95)00054-O
Andrade, G., Mihara, K. L., Linderman, R. G., and Bethlenfalvay, G. J. (1998). Soil aggregation status and rhizobacteria in the mycorrhizosphere. Plant Soil 202, 89–96. doi: 10.1023/A:1004301423150
Angel, R., and Conrad, R. (2013). Elucidating the microbial resuscitation cascade in biological soil crusts following a simulated rain event. Environ. Microbiol. 15, 2799–2815. doi: 10.1111/1462-2920.12140
Armstrong, R., and Bradwell, T. (2010). Growth of crustose lichens: a review. Geogr. Ann. 92, 3–17. doi: 10.1111/j.1468-0459.2010.00374.x
Armstrong, R. A. (2017). A study of fragmentation rates in lichen populations on rock surfaces using the Kaplan-Meir estimator and Cox regression. Ann. Botanici Fennici 54, 169–178. doi: 10.5735/085.054.0326
Australian National Botanic Gardens (2016). Australian Bryophytes, Reproduction & Dispersal, Sexual vs. Vegetative. Available online at: https://www.anbg.gov.au/bryophyte/sexual-vegetative.html
Bailey, R. H. (1966). Studies on the dispersal of lichen soredia. J. Linn. Soc. Bot. 59, 479–490. doi: 10.1111/j.1095-8339.1966.tb00074.x
Banchi, E., Ametrano, C. G., Stanković, D., Verardo, P., Moretti, O., Gabrielli, F., et al. (2018). DNA metabarcoding uncovers fungal diversity of mixed airborne samples in Italy. PLoS ONE 13:094489. doi: 10.1371/journal.pone.0194489
Bannister, J. M., and Blanchon, D. J. (2003). The lichen genus Ranalina Ach. (Ramalinaceae) on the outlying islands of the New Zealand geographic area. Lichenologist 35, 137–146. doi: 10.1016/S0024-2829(03)00017-3
Barbé, M., Fenton, N. J., Caners, R., and Bergeron, Y. (2017). Interannual variation in bryophyte dispersal: linking bryophyte phenophases and weather conditions. Botany 95, 1151–1169. doi: 10.1139/cjb-2017-0054
Bates, S. T., and Garcia-Pichel, F. (2009). A culture-independent study of free-living fungi in biological soil crusts of the Colorado Plateau: their diversity and relative contribution to microbial biomass. Environ. Microbiol. 11, 56–67. doi: 10.1111/j.1462-2920.2008.01738.x
Bates, S. T., Nash, T. H. III., and Garcia-Pichel, F. (2012). Patterns of diversity for fungal assemblages of biological soil crusts from the southwestern United States. Mycologia 104, 353–361. doi: 10.3852/11-232
Belnap, J., Büdel, B., and Lange, O. L. (2001). “Biological soil crusts: characteristics and distribution,” in Biological Soil Crusts: Structure, Function, and Management, eds J. Belnap and O. L. Lange (Heidelberg: Lange Springer-Verlag), 3–30. doi: 10.1007/978-3-642-56475-8_1
Belnap, J., and Gardner, J. S. (1993). Soil microstructure in soils of the Colorado Plateau: the role of the cyanobacterium Microcoleus vaginatus. Great Basin Natural. 53, 40–47.
Belnap, J., and Gillette, D. A. (1998). Vulnerability of desert biological soil crusts to wind erosion: the influences of crust development, soil texture, and disturbance. J. Arid Environ. 39, 133–142. doi: 10.1006/jare.1998.0388
Bergman, B., Gallon, J. R., Rai, A. N., and Stal, L. J. (1997). N2 fixation by non-heterocystous cyanobacteria. FEMS Microbiol. Rev. 19, 139–185. doi: 10.1016/S0168-6445(96)00028-9
Berruti, A., Borriello, R., Orgiazzi, A., Barbera, A. C., Lumini, E., and Bianciotto, V. (2014). “Chapter 8: Arbuscular mycorrhizal fungi and their value for ecosystem management,” in Biodiversity - the Dynamic Balance of the Planet, ed O. Grillo (Intech Online Open Access Books), 159–191. doi: 10.5772/58231
Berruti, A., Lumini, E., Balestrini, R., and Biancotto, C. (2015). Arbuscular mycorrhizal fungi as natural biofertilizers: let's benefit from past successes. Front. Microbiol. 6:1559. doi: 10.3389/fmicb.2015.01559
Biersma, E. M., Jackson, J. A., Hyvönen, J., Koskinen, S., Linse, K., Griffiths, H., et al. (2017). Global biogeographic patterns of bipolar moss species. R. Soc. Open Sci. 4:170147. doi: 10.1098/rsos.170147
Blackwell, M., Alexopoulos, C. J., and Mims, C. W. (1996). Introductory Mycology. New York, NY: Wiley.
Boose, Y., Welti, A., Atkinson, J., Ramelli, F., Danielczok, A., Bingemer, H. G., et al. (2016). Heterogeneous ice nucleation on dust particles sourced from nine deserts worldwide - Part 1: Immersion freezing. Atmos. Chem. Phys. 16, 15075–15095. doi: 10.5194/acp-16-15075-2016
Borchhardt, N., Schiefelbein, U., Abarca, N., Boy, J., Mikhailyuk, T., Sipman, H. J. M., et al. (2017). Diversity of algae and lichens in biological soil crusts of Ardley and King George Islands, Antarctica. Antarct. Sci. 29, 229–237. doi: 10.1017/S0954102016000638
Bowker, M. A., Belnap, J., Chaudhary, V. B., and Johnson, N. C. (2008). Revisiting classic water erosion models in drylands: the strong impact of biological soil crusts. Soil Biol. Biochem. 40, 2309–2316. doi: 10.1016/j.soilbio.2008.05.008
Bowker, M. A., Stark, L. R., McLetchie, D. N., and Mishler, B. D. (2000). Sex expression, skewed sex ratios, and microhabitat distribution in the dioecious desert moss Syntrichia caninervis (Pottiaceae). Am. J. Bot. 87, 517–526. doi: 10.2307/2656595
Bowler, P. A., and Rundel, P. W. (1975). Reproductive strategies in lichens. Bot. J. Linn. Soc. 59, 470–480. doi: 10.1111/j.1095-8339.1975.tb01653.x
Brinda, J. C., Toren, D. R., and Shevock, J. R. (2016). Campylostelium (Ptychomitriaceae) in the southwestern United States: Campylostelium laegerae sp. nov. and C. pitardii new to the Americas. Madroño 63, 353–358. doi: 10.3120/0024-9637-63.4.353
Broady, P. A., and Smith, R. A. (1994). A preliminary investigation of the diversity, survivability and dispersal of algae introduced into Antarctica by human activity. Proc. Natl. Inst. Polar Res. 7, 185–197.
Brodo, I. M., Sharnoff, S. D., and Sharnoff, S. (2001). Lichens of North America. New Haven, CT: Yale University Press.
Brown, R. M., Larson, D. A., and Bold, H. C. (1964). Airborne algae: their abundance and heterogeneity. Science 143, 583–585. doi: 10.1126/science.143.3606.583
Büdel, B., Dulić, T., Darienko, T., Rubalka, M., and Friedl, T. (2016). “Cyanobacteria and algae of biological soil crusts,” in Biological Soil Crusts: An Organizing Principle in Drylands, Ecological Studies 226, eds B. Weber, B. Büdel, and J. Belnap (Cham: Springer), 55–80.
Caesar-TonThat, T. C., and Cochran, V. L. (2001). “Role of a saprophytic Basidiomycete soil fungus in aggregate stabilization. Sustaining the global farm,” in 10th International Soil Conservation Organization Meeting, eds D. E. Stott, R. H. Rohtar, and G. C. Steinhardt. 24–29 May 1999 and the USDA-ARS National Soil Erosion Research Laboratory Pages, 575–579.
Camargo-Ricalde, S. L. (2002). Dispersal, distribution and establishment of arbuscular mycorrhizal fungi: a review. Boletín de la Soc. Bot. México 71, 33–44. doi: 10.17129/botsci.1661
Campbell, E. L., and Meeks, J. C. (1989). Characteristics of hormogonia formation by symbiotic Nostoc spp. in response to the presence of Anthoceros punctatus or its extracellular products. Appl. Environ. Microbiol. 35, 125–131.
Cardon, Z. G., Gray, D. W., and Lewis, L. A. (2008). The green algal underground: evolutionary secrets of desert cells. BioScience 58, 114–122. doi: 10.1641/B580206
Castenholz, R. W. (1982). “The movements of cyanobacteria,” in The Biology of Cyanobacteria, eds N. G. Carr and B. A. Whitton (London: Blackwell), 413–439.
Chaudhary, V. B., Bowker, M. A., O'Dell, T. E., Grace, J. B., Redman, A. E., Rillig, M. C., et al. (2009). Untangling the biological contributions to soil stability in semiarid shrublands. Ecol. Appl. 19, 110–122. doi: 10.1890/07-2076.1
Chen, J., Blume, H.-P., and Beyer, L. (2000). Weathering of rocks induced by lichen colonization – a review. Catena 39, 121–146. doi: 10.1016/S0341-8162(99)00085-5
Chen, W., Humbleton, S., Seifert, K. A., Carrise, O., Diarra, M. S., Peters, R. D., et al. (2018). Assessing performance of spore samplers in monitoring Aeromycobiota and fungal plant pathogen in Canada. Appl. Environ. Microbiol. 84, e02601–e02617. doi: 10.1128/AEM.02601-17
Choudoir, M. J., Barberan, A., Menninger, H. L., Dunn, R. R., and Fierer, N. (2018). Variation in range size and dispersal capabilities of microbial taxa. Ecology 99, 322–334. doi: 10.1002/ecy.2094
Christner, B. C., Morris, C. E., Foreman, C. M., Cai, R. M., and Sands, D. C. (2008). Ubiquity of biological ice nucleators in snowfall. Science 319, 1214–1214. doi: 10.1126/science.1149757
Chuvochina, M. S., Alekhina, I. A., Normand, P., Petit, J. R., and Bulat, S. A. (2011). Three events of Saharan dust deposition on the Mont Blanc glacier associated with different snow-colonizing bacterial phylotypes. Microbiology 80, 125–131. doi: 10.1134/S0026261711010061
Coe, K. K., Sparks, J. P., and Belnap, J. (2014). “Chapter 16: Physiological ecology of dryland biocrust mosses,” in Photosynthesis in Bryophytes and Early Land Plants, eds D. T. Hanson and S. K. Rice (Dordrecht: Springer), 291–308. doi: 10.1007/978-94-007-6988-5_16
Colesie, C., Felde, V. J. M. L., and Büdel, B. (2016). Composition and macrostructure of biological soil crusts. Biol. Soil Crusts 226, 159–172. doi: 10.1007/978-3-319-30214-0_9
Collins, S. L., Sinsabaugh, R. L., Crenshaw, C., Green, L., Porras-Alfaro, A., Stursova, M., et al. (2008). Pulse dynamics and microbial processes in aridland ecosystems. J. Ecol. 96, 413–420. doi: 10.1111/j.1365-2745.2008.01362.x
Costa, O. Y. A., Raaijmakers, J. M., and Kuramae, E. E. (2018). Microbial extracellular polymeric substances: ecological function and impact on soil aggregation. Front. Microbiol. 9:1636. doi: 10.3389/fmicb.2018.01636
Couradeau, E., Giraldo-Silva, A., De Martini, F., and Garcia-Pichel, F. (2019). Spatial segregation of the biological soil crust microbiome around its foundational cyanobacterium, Microcoleus vaginatus, and the formation of a nitrogen-fixing cyanosphere. Microbiome 7:55a. doi: 10.1186/s40168-019-0661-2
Crania, B. J., Vestergaard, G., Kubli, K., Köhne, J. M., Fischer, T., Albert, A., et al. (2019). Biological soil crusts from different soil substrates harbor distinct bacterial groups with the potential to produce exopolysaccharides and lipopolysaccharides. Microbial. Ecol. doi: 10.1007/s00248-019-01415-6. [Epub ahead of print].
Dastrup, D. B., Carling, G. T., Collins, S. A., Nelson, S. T., Fernandez, D. P., Tingey, D. G., et al. (2018). Aeolian dust chemistry and bacterial communities in snow are unique to airshed locations across northern Utah, USA. Atmos. Environ. 193, 251–261. doi: 10.1016/j.atmosenv.2018.09.016
Degens, B. P., Sparling, G. P., and Abbott, L. K. (1996). Increasing the length of hyphae in a sandy soil increases the amount of water-stable aggregates. Appl. Soil Ecol. 3, 149–159. doi: 10.1016/0929-1393(95)00074-7
Després, V. R., Huffman, J. A., Burrows, S. M., Hoose, C., Safatov, A. S., Buryak, G., et al. (2012). Primary biological aerosol particles in the atmosphere: a review. Tellus B 64:e15598. doi: 10.3402/tellusb.v64i0.15598
Domozych, D. S., and Domozych, C. E. (2014). Multicellularity in green algae: upsizing in a walled complex. Front. Plant Sci. 5:e649. doi: 10.3389/fpls.2014.00649
Dupont, E. M., Field, R. C., Leathers, C. E., and Northey, W. T. (1967). A survey of the airborne fungi in the Albuquerque, New Mexico, metropolitan area. J. Allergy 39, 238–244. doi: 10.1016/0021-8707(67)90017-2
During, H. J., and van Tooren, B. F. (1987). Recent developments in bryophyte population ecology. Tree 2, 89–93. doi: 10.1016/0169-5347(87)90164-9
Edgar, L. A., and Pickett-Heaps, J. D. (1982). Utrastructural localization of polysaccharides in the motile diatom Navicula cuspidata. Protoplasma 113, 10–22. doi: 10.1007/BF01283035
Edlund, M. B., and Stoermer, E. F. (1997). Ecological, evolutionary, and systematic significance of diatom life histories. J. Phycol. 33, 897–918. doi: 10.1111/j.0022-3646.1997.00897.x
Egan, C., Li, D. W., and Klironomos, J. (2014). Detection of arbuscular mycorrhizal fungal spores in the air across different biomes and ecoregions. Fungal Ecol. 12, 26–31. doi: 10.1016/j.funeco.2014.06.004
Eldridge, D. J., and Delgado-Baquerizo, M. (2019). The influence of climatic legacies on the distribution of dryland biocrust communities. Glob. Change Biol. 25, 327–336. doi: 10.1111/gcb.14506
Ertz, D., Guzow-Krzeminska, B., Thor, G., Łubek, A., and Kukwa, M. (2018). Photobiont switching causes changes in the reproduction strategy and phenotypic dimorphism in the Arthoniomycetes. Sci. Rep. 8:e4952. doi: 10.1038/s41598-018-23219-3
Ettl, H., and Gärtner, G. (1995). Syllabus der Boden-, Luft- und Flechtenalgen. Stuttgart: Gustav Fischer Verlag.
Ezike, D. N., Nnamani, C. V., Ogundipe, O. T., and Adekanmbi, O. H. (2016). Airborne pollen and fungal spores in Garki, Abuja (North-Central Nigeria). Aerobiologia 32, 697–707. doi: 10.1007/s10453-016-9443-5
Fang, Z., Ouyang, Z., Hu, L., Wang, L., Zheng, H., and Lin, X. (2005). Culturable airborne fungi in outdoor environments in Beijing, China. Sci. Total Environ. 350, 47–58. doi: 10.1016/j.scitotenv.2005.01.032
Favet, J., Lapanje, A., Giongo, A., Kennedy, S., Aung, Y. Y., Cattaneo, A., et al. (2013). Microbial hitchhikers on intercontinental crust: catching a lift in Chad. Int. Soc. Microb. Ecol. 7, 850–867. doi: 10.1038/ismej.2012.152
Fay, P. (1992). Oxygen relations of nitrogen fixation in cyanobacteria. Microbiol. Rev. 56, 340–373.
Flechtner, V. R., Pietrasiak, N., and Lewis, L. A. (2013). Newly revealed diversity of eukaryotic algae from wilderness areas of Joshua Tree National Park (JTNP). Monogr. Western North Am. Natural. 6, 43–63. doi: 10.3398/042.006.0103
Flø, D., and Hågvar, S. (2013). Aerial dispersal of invertebrates and mosses close to a receding alpine glacier in southern Norway. Arctic Antarct. Alpine Res. 45, 481–490. doi: 10.1657/1938-4246-45.4.481
Frölich-Nowoisky, J., Kampf, C. J., Weber, B., Huffman, J. A., Pöhlker, C., Andreae, M. O., et al. (2016). Bioaerosols in the earth system: climate, health, and ecosystem interactions. Atmos. Res. 12. 346–376. doi: 10.1016/j.atmosres.2016.07.018
Fučíková, K., Lewis, P. O., and Lewis, L. A. (2014). Widespread desert affiliation of trebouxiophycean green algae (Trebouxiophyceae, Chlorophyta) including discovery of three new desert genera. Phycol. Res. 62, 294–305. doi: 10.1111/pre.12062
Fučíková, K., PaŽoutová, M., and Rindi, F. (2015). Meiotic genes and sexual reproduction in the green algal class Trebouxiophyceae (Chlorophyta). J. Phycol. 51, 419–430. doi: 10.1111/jpy.12293
Garcia-Pichel, F., and Wojciechowski, M. F. (2009). The evolution of a capacity to build supra-cellular ropes enable filamentous cyanobacteria to colonize highly erodible structures. PLoS ONE 4:e7801. doi: 10.1371/journal.pone.0007801
Genitsaris, S., Moustaka-Gouni, M., and Kormas, K. A. (2011). Airborne microeukaryote colonists in experimental water containers: diversity, succession, life histories and established food webs. Aquat. Microb. Ecol. 62, 139–152. doi: 10.3354/ame01463
Gianinazzi, S., Gollotte, A., Binet, M.-N., van Tuinen, D., Redecker, D., and Wipf, D. (2010). Agroecology: the key role of arbuscular mycorrhizas in ecosystem services. Mycorrhiza 20, 519–530. doi: 10.1007/s00572-010-0333-3
Glime, J. M. (2017). Bryophyte Ecology. Available online at: http://digitalcommons.mtu.edu/bryophyte-ecology
Golan, J. J., and Pringle, A. (2017). Long-distance dispersal of fungi. Microbiol. Spectr. 5:FUNK-0047-2016. doi: 10.1128/microbiolspec.FUNK-0047-2016
Gomathy, M., Sabarinathan, K. G., Sivasankari Devi, T., and Pandiyarajan, P. (2018). Arbuscular mycorrhizal fungi and glomalin - super glue. Int. J. Curr. Microbiol. Appl. Sci. 7, 2853–2857. doi: 10.20546/ijcmas.2018.707.334
Gostinčar, C., Grube, M., De Hoog, S., Zalar, P., and Gunde-Cimerman, N. (2009). Extremotolerance in fungi: evolution on the edge. FEMS Microbiol. Ecol. 71, 2–11. doi: 10.1111/j.1574-6941.2009.00794.x
Gray, D. W., Lewis, L. A., and Cardon, Z. G. (2007). Photosynthetic recovery following desiccation of desert green algae (Chlorophyta) and their aquatic relatives. Plant Cell Environ. 30, 1240–1255. doi: 10.1111/j.1365-3040.2007.01704.x
Green, L. E., Porras-Alfaro, A., and Sinsabaugh, R. L. (2008). Translocation of nitrogen and carbon integrates biotic crust and grass production in desert grassland. J. Ecol. 96, 1076–1085. doi: 10.1111/j.1365-2745.2008.01388.x
Green, T. G. A., and Proctor, M. C. F. (2016). “Physiology of photosynthetic organisms within biological soil crusts: their adaptation, flexibility, and plasticity,” in Biological Soil Crusts: An Organizing Principle in Drylands, eds B. Weber, B. Büdel and J. Belnap (Cham: Springer), 347–381. doi: 10.1007/978-3-319-30214-0_18
Griffin, D. W., Kellogg, C. A., Garrison, V. H., and Shinn, E. A. (2002). The global transport of dust: an intercontinental river of dust, microorganisms and toxic chemicals flows through the Earth's atmosphere. Am. Sci. 90, 228–235. doi: 10.1511/2002.3.228
Gundlapally, S. R., and Garcia-Pichel, F. (2006). The community and phylogenetic diversity of biological soil crusts in the Colorado Plateau studied by molecular fingerprinting and intensive cultivation. Microb. Ecol. 52, 345–357. doi: 10.1007/s00248-006-9011-6
Halwagy, M. H. (1994). Fungal airspora of Kuwait City, Kuwait, 1975–1987. Grana 33, 320–345. doi: 10.1080/00173139409429022
Harmata, K., and Olech, M. (1991). Transect for aerobiological studies from Antarctica to Poland. Grana 30, 458–463. doi: 10.1080/00173139109432009
Harten, J. B., and Eickmeier, W. G. (1987). Comparative desiccation tolerance of three desert Pteridophytes: response to long-term desiccation. Am. Midland Natural. 118, 337–347. doi: 10.2307/2425790
Heinken, T. (1999). Dispersal patterns of terricolous lichens by thallus fragments. Lichenologist 34, 603–612. doi: 10.1006/lich.1999.0219
Henson, J. M., Butler, M. J., and Day, A. (1999). The dark side of the mycelium: melanins of phytopathogenic fungi. Annu. Rev. Phytopathol. 37, 447–471. doi: 10.1146/annurev.phyto.37.1.447
Herbold, C. W., Lee, C. K., McDonald, I. R., and Cary, S. C. (2014). Evidence of global-scale aeolian dispersal and endemism in isolated geothermal microbial communities of Antarctica. Nat. Commun. 5:3875. doi: 10.1038/ncomms4875
Herburger, K., Lewis, L. A., and Holzinger, A. (2015). Photosynthetic efficiency, desiccation tolerance and ultrastructure in two phylogenetically distinct strains of alpine Zygnema sp. (Zygnematophyceae, Streptophyta): role of pre-akinete formation. Protoplasma 252, 571–589. doi: 10.1007/s00709-014-0703-3
Herrero, A., Stavans, J., and Flores, E. (2016). The multicellular nature of filamentous heterocyst-forming cyanobacteria. FEMS Microbiol. Rev. 40, 831–854. doi: 10.1093/femsre/fuw029
Hoiczyk, E. (2000). Gliding motility in cyanobacteria: observations and possible explanations. Arch. Microbiol. 174, 11–17. doi: 10.1007/s002030000187
Holzapfel, E. P. (1978). Transoceanic airplane sampling for organisms and particles. Pac. Insects 18, 169–189.
Holzinger, A., Albert, A., Aigner, S., Uhl, J., Schmitt-Kopplin, P., Trumhová, K., et al. (2018). Artic, Antarctic, and temperate green algae Zygnema spp. under UV-B stress: vegetative cells perform better than pre-akinetes. Protoplasma 255, 1239–1252. doi: 10.1007/s00709-018-1225-1
Holzinger, A., and Karsten, U. (2013). Desiccation stress and tolerance in green algae: consequences for ultrastructure, physiological, and molecular mechanisms. Front. Plant Sci. 4:327. doi: 10.3389/fpls.2013.00327
Hoppert, M., Reimer, R., Kemmling, A., Schröder, A., Günzl, B., and Heinken, T. (2004). Structure and reactivity of a biological soil crust from a xeric sandy soil in central Europe. Geomicrobiol. J. 21, 183–191. doi: 10.1080/01490450490275433
Horsley, K., Stark, L. R., and McLetchie, D. N. (2011). Does the silver moss Bryum argenteum exhibit sex-specific patterns in vegetative growth rate, asexual fitness or prezygotic reproductive investment? Ann. Bot. 107, 897–907. doi: 10.1093/aob/mcr027
Hu, C., Zhang, D., Huang, Z., and Liu, Y. (2003). The vertical microdistribution of cyanobacteria and algae within desert crusts and the development of the algal crusts. Plant and Soil 257, 97–111. doi: 10.1023/A:1026253307432
Hugonnot, V., and Celle, J. (2012). Asexual reproduction by leaf fragmentation in Mnium stellare Hedw. J. Bryol. 34, 67–70. doi: 10.1179/1743282011Y.0000000036
Ibañez, V., Rojas, G., and Roure, J. (2001). Airborne fungi monitoring in Santiago, Chile. Aerobiologia 17, 137–142. doi: 10.1023/A:1010833101583
Jang, G., Yi, K., Nuno, D., Pires, N. D., Menand, B., and Dolan, L. (2011). RSL genes are sufficient for rhizoid system development in early diverging land plants. Development 138, 2273–2281. doi: 10.1242/dev.060582
Joergensen, R. G., and Wichern, F. (2018). Alive and kicking: why dormant soil microorganisms matter. Soil Biol. Biochem. 116, 419–430. doi: 10.1016/j.soilbio.2017.10.022
Johansson, V., Lönnell, N., Sundberg, S., and Hylander, K. (2014). Release thresholds for moss spores: the importance of turbulence and sporophyte length. J. Ecol. 102, 721–729. doi: 10.1111/1365-2745.12245
Jungblut, A. D., Vincent, W. F., and Lovejoy, C. (2012). Eukaryotes in arctic and antarctic cyanobacterial mats. FEMS Microb. Ecol. 82, 416–428. doi: 10.1111/j.1574-6941.2012.01418.x
Kaczmarska, L. J. M., Ehrman and Bates, S. S. (2001). “A review of auxospore structure, ontogeny, and diatom phylogeny,” Sixteenth International Diatom Symposium, ed A. Economou-Amilli (Athens: Aegean Islands proceedings), 601.
Kaplan-Levy, R. N., Hadas, O., Summers, M. L., Rücker, J., and Sukenik, A. (2010). “Akinetes: dormant cells of cyanobacteria,” in Dormancy and Resistance in Harsh Environments. Topics in Current Genetics, Vol. 21, eds E. Lubzens, J. Cerda, and M. Clark (Berlin: Springer), 5–27. doi: 10.1007/978-3-642-12422-8_2
Karsten, U., and Holzinger, A. (2014). Green algae in alpine biological soil crust communities: acclimation strategies against ultraviolet radiation and dehydration. Biodiver. Conserv. 23, 1845–1858. doi: 10.1007/s10531-014-0653-2
Kasprzyk, I., and Worek, M. (2006). Airborne fungal spores in urban and rural environments in Poland. Aerobiologia 22, 169–176. doi: 10.1007/s10453-006-9029-8
Kehri, H. K., Akhtar, O., Zoomi, I., and Pandey, D. (2018). Arbuscular mycorrhizal fungi: taxonomy and its systematics. Int. J. Life Sci. Res. 6, 58–71.
Kellogg, C. A., and Griffin, D. W. (2006). Aerobiology and the global transport of desert dust. Trends Ecol. Evol. 21, 638–644. doi: 10.1016/j.tree.2006.07.004
Kharkongor, D., and Ramanujam, P. (2014). Diversity and species composition of subaerial algal communities in forested areas of Meghalaya, India. Int. J. Biodiversity 2014:e456202. doi: 10.1155/2014/456202
Kitzing, C., Pröschold, T., and Karsten, U. (2014). UV-induced effects on growth, photosynthetic performance and sunscreen contents in different populations of the green alga Klebsormidium fluitans (Streptophyta) from alpine soil crusts. Microb. Ecol. 67, 327–340. doi: 10.1007/s00248-013-0317-x
Kranner, I., Beckett, R., Hochman, A., and Nash, T. H. III. (2008). Desiccation-tolerance in lichens: a review. Bryologist 111, 576–593. doi: 10.1639/0007-2745-111.4.576
Kumar, K., Mella-Herrera, R. A., and Golden, J. W. (2010). Cyanobacterial heterocysts. Cold Springs Harbor Perspectives Biol. 2:a000315. doi: 10.1101/cshperspect.a000315
Kunkel, D. D. (1984). Cell division in baeocyte producing cyanobacteria. Protoplasma 123, 104–115. doi: 10.1007/BF01283581
Kuske, C. R., Yeager, C. M., Johnson, S., Ticknor, L. O., and Belnap, J. (2012). Response and resilience of soil biocrust bacterial communities to chronic physical disturbance in arid shrublands. ISME J. 6, 886–897. doi: 10.1038/ismej.2011.153
Kvíderova, J. (2012). Research on cryogenic communities in Svalbard: the snow algae in temporary snowfields in Petuniabukta, Central Svalbard. Czech Polar Rep. 2, 8–19. doi: 10.5817/CPR2012-1-2
Laaka-Lindberg, S., Korpelainen, H., and Pohjamo, M. (2003). Dispersal of asexual propagules in bryophytes. J. Hattori Bot. Lab. 93, 319–330.
Lakatos, M. (2011). “Lichens and bryophytes: habitats and species,” in Plant Desiccation Tolerance, Ecological Studies 215, eds U. Lüttge, E. Beck, and D. Bartels (Berlin: Springer-Verlag), 65–87. doi: 10.1007/978-3-642-19106-0_5
Lange, O. L. (1969). CO2-Gaswechsel von Mossen nach Wasserdampfaufnahme aus dem Luftraum. Planta 89, 90–94. doi: 10.1007/BF00386500
Lee, S. C., Ni, M., Li, W., Shertz, C., and Heitman, J. (2010). The evolution of sex: a perspective from the fungal kingdom. Microbiol. Molecul. Biol. Rev. 74, 298–340. doi: 10.1128/MMBR.00005-10
Leger, E. A., and Forister, M. J. (2009). Colonization, abundance, and geographic range size of gravestone lichens. Basic Appl. Ecol. 10, 279–287. doi: 10.1016/j.baae.2008.04.001
Lennon, J. T., and Jones, S. E. (2011). Microbial seed banks: the ecological and evolutionary implications of dormancy. Nat. Rev. Microbiol. 9, 119–130. doi: 10.1038/nrmicro2504
Lewis, L. A., and Lewis, P. O. (2005). Unearthing the molecular phylodiversity of desert soil green algae (Chlorophyta). Syst. Biol. 54, 936–947. doi: 10.1080/10635150500354852
Lewis, L. R., Rozzi, R., and Goffinet, B. (2014). Direct long-distance dispersal shapes a New World amphitropical disjunction in the dispersal-limited dung moss Tetraplodon (Bryopsida: Splachnaceae). J. Biogeogr. 41, 2385–2395. doi: 10.1111/jbi.12385
Longton, R. E. (ed.). (1997). Advances in Bryology, Vol. 6 in Population Studies. Berlin: J. Cramer.
Lönnell, N., Hylander, K., Johnsson, B. G., and Sundberg, S. (2012). The fate of the missing spores – patterns of realized dispersal beyond the closest vicinity of a sporulating moss. PLoS ONE 7:e41987. doi: 10.1371/journal.pone.0041987
Lücking, R., Hodkinson, B. P., and Leavitt, S. D. (2016). The 2016 classification of lichenized fungi in the Ascomycota and Basidiomycota – Approaching one thousand genera. Bryologist 119, 361–416. doi: 10.1639/0007-2745-119.4.361
Lücking, R., Hodkinson, B. P., and Leavitt, S. D. (2017). Corrections and amendments to the 2016 classification of lichenized fungi in the Ascomycota and Basidiomycota. Bryologist 120, 58–69. doi: 10.1639/0007-2745-120.1.058
Maier, S., Muggia, L., Kuske, C. R., and Grube, M. (2016). “Bacteria and non-lichenized fungi within biological soil crusts,” in Biological Soil Crusts: An Organizing Principle in Drylands, eds B. Weber, B. Büdel, and J. Belnap (Chaml: Springer), 81–100. doi: 10.1007/978-3-319-30214-0_5
Maier, S., Schmidt, T. S. B., Zheng, L. J., Peer, T., Wagner, V., and Grube, M. (2014). Analyses of dryland biological soil crusts highlight lichens as an important regulator of microbial communities. Biodiver. Conserv. 23, 1735–1755. doi: 10.1007/s10531-014-0719-1
Maier, S., Tamm, A., Wu, D. M., Caesar, J., Grube, M., and Weber, B. (2018). Photoautotrophic organisms control microbial abundance, diversity, and physiology in different types of biological soil crusts. Int. Soc. Microb. Ecol. J. 12, 1032–1046. doi: 10.1038/s41396-018-0062-8
Makhalanyane, T. P., Valverde, A., Gunnigle, E., Frossard, A., Ramond, J. B., and Cowan, D. A. (2015). Microbial ecology of hot desert edaphic systems. FEMS Microbiol. Rev. 39, 203–221. doi: 10.1093/femsre/fuu011
Maki, T., Lee, K. C., Kawai, K., Onishi, K., Hong, C. S., Kurosaki, Y., et al. (2019). Aeolian dispersal of bacteria associated with desert dust and anthropogenic particles over continental and oceanic surfaces. J. Geophys. Res. 124, 5579–5588. doi: 10.1029/2018JD029597
Mann, D. G. (1993). “Patterns of sexual reproduction in diatoms,” in Twelfth International Diatom Symposium. Developments in Hydrobiology, ed H Van Dam (Dordrecht: Springer), 11–20. doi: 10.1007/978-94-017-3622-0_2
Marleau, J., Dalpé, Y., St-Arnaud, M., and Hijri, M. (2011). Spore development and nuclear inheritance in arbuscular mycorrhizal fungi. BMC Evol. Biol. 1:51. doi: 10.1186/1471-2148-11-97
Marshall, W. A. (1996). Aerial dispersal of lichen soredia in the maritime Antarctica. N. Phytol. 134, 523–530. doi: 10.1111/j.1469-8137.1996.tb04370.x
Marshall, W. A. (1997). Seasonality in Antarctic airborne fungal spores. Appl. Environ. Microbiol. 63, 2240–2245.
Marshall, W. A., and Chalmers, M. O. (1997). Airborne dispersal of Antarctic terrestrial algae and cyanobacteria. Ecography 20, 585–593. doi: 10.1111/j.1600-0587.1997.tb00427.x
Mazor, G., Kidron, G. J., Vonshak, A., and Abeliovich, A. (1996). The role of cyanobacterial exopolysaccharides in structuring microbial crusts. FEMS Microbiol. Ecol. 21, 121–130. doi: 10.1111/j.1574-6941.1996.tb00339.x
McCleary, J. A. (1959). The bryophytes of a desert region in Arizona. Bryologist 62, 58–62. doi: 10.1639/0007-2745(1959)62[58:TBOADR]2.0.CO;2
Meeks, J. C., and Elhai, J. (2002). Regulation of cellular differentiation in filamentous cyanobacteria in free- living and plant-associated symbiotic growth states. Microbiol. Molecul. Biol. Rev. 66, 94–121. doi: 10.1128/MMBR.66.1.94-121.2002
Miller, R. M., and Jastrow, J. D. (1992). The Role of Mycorrhizal Fungi in Soil Conservation. Madison, WI: American Society of Agronomy Special Publication No. 54
Mishler, B. D. (1988). “Reproductive ecology of bryophytes,” in Plant Reproductive Ecology: Patterns and Strategies. eds J. Lovett Doust and L. Lovett (New York, NY: Doust Oxford University Press), 285–306.
Mitakakis, T. Z., and Guest, D. I. (2001). A fungal spore calendar for the atmosphere of Melbourne, Australia, for the year 1993. Aerobiologia 17, 171–176. doi: 10.1023/A:1011028412526
Moquin, S. A., Garcia, J. R., Brantley, S. L., Takacs-Vesbach, C. D., and Shepherd, U. L. (2012). Bacterial diversity of bryophyte-dominant biological soil crusts and associated mites. J. Arid Environ. 87, 110–117. doi: 10.1016/j.jaridenv.2012.05.004
Muñoz, J., Felicísimo, Á. M., Cabezas, F., Burgaz, A. R., and Martinez, I. (2004). Wind as a long-distance dispersal vehicle in the southern hemisphere. Science 304, 1144–1147. doi: 10.1126/science.1095210
Mur, L. R., Skulberg, O. M., and Utkilen, H. (1999). “Chapter 2: Cyanobacteria in the environment,” in Toxic Cyanobacteria in Water: A Guide to Their Public Health Consequences, Monitoring and Management, eds I. Chorus and J. Bartram (Geneva: World Health Organization).
Nagy, M. L., Perez, A., and Garcia-Pichel, F. (2005). The prokaryotic diversity of biological soil crusts in the Sonoran Desert (Organ Pipe Cactus National Monument, AZ). Fems Microbiol. Ecol. 54, 233–245. doi: 10.1016/j.femsec.2005.03.011
Nakajima, M., Hokoi, S., Ogura, D., and IBA, C. (2015). Relationship between environmental conditions and algal growth on exterior walls of the Ninna-ji Temple, Kyoto. Energy Proc. 78, 1329–1334. doi: 10.1016/j.egypro.2015.11.149
Namsaraev, Z., Mano, M. -J., Fernandez, R., and Wilmotte, A. (2010). Biogeography of terrestrial cyanobacteria from Antarctic ice-free areas. Ann. Glaciol. 51, 171–177. doi: 10.3189/172756411795931930
Nash, T. H. III., Reiner, A., Demmig-Adams, B., Killian, E., Kaiser, W., and Lange, O. L. (1990). The effect of atmospheric desiccation and osmotic water stress on photosynthesis and dark respiration of lichens. N. Phytol. 116, 269–276. doi: 10.1111/j.1469-8137.1990.tb04714.x
Navarro-Noya, Y. E., Jimenez-Aguilar, A., Valenzuela-Encinas, C., Alcantara-Hernandez, R. J., Ruiz-Valdiviezo, V. M., Ponce-Mendoza, A., et al. (2014). Bacterial communities in soil under moss and lichen-moss crusts. Geomicrobiol. J. 31, 152–160. doi: 10.1080/01490451.2013.820236
Nelson, M. B., Martiny, A. C., and Martiny, J. B. H. (2016). Global biogeography of microbial nitrogen-cycling traits in soil. Proc. Natl. Acad. Sci. U.S.A. 113, 8033–8040. doi: 10.1073/pnas.1601070113
Nguyen, K. H., Chollet-Krugler, M., Gouault, N., and Tomasi, S. (2013). UV-protectant metabolites from lichens and their symbiotic partners. Nat. Product Rep. 30, 1490–1508. doi: 10.1039/c3np70064j
Nieuwenhuis, B. P. S., and James, T. Y. (2016). The frequency of sex in fungi. Philos. Transac. R. Soc. B 371:20150540. doi: 10.1098/rstb.2015.0540
Nunes da Rocha, U., Cadillo-Quiroz, H., Karaoz, U., Rajeev, L., Klitgord, N., Dunn, S., et al. (2015). Isolation of a significant fraction of non-phototroph diversity from a desert biological soil crust. Front. Microbiol. 6:277. doi: 10.3389/fmicb.2015.00277
Odu, E. A. (1978). The adaptive importance of moss rhizoids for attachment to the substratum. J. Bryol. 10, 163–181. doi: 10.1179/jbr.1978.10.2.163
Ojeda-López, M., Chen, W., Eagle, C. E., Gutiérrez, G., Jia, W. L., Swilaiman, S. S., et al. (2018). Evolution of asexual and sexual reproduction in the aspergilli. Stud. Mycol. 91, 37–59. doi: 10.1016/j.simyco.2018.10.002
Oren, A., and Gunde-Cimerman, N. (2007). Mycosporines and mycosporine-like amino acids: UV protectants or multipurpose secondary metabolites? FEMS Microbiol. Lett. 269, 1–10. doi: 10.1111/j.1574-6968.2007.00650.x
Ott, S., Treiber, K., and Jahns, H. M. (1993). The development of regenerative thallus structures in lichens. Botan. J. Linn. Soc. 113, 61–76. doi: 10.1111/j.1095-8339.1993.tb00329.x
Paasch, A. E., Mishler, B. D., Nosratinia, S. L. R., and Fishler, K. M. (2015). Decoupling of sexual reproduction and genetic diversity in the female-biased Mojave Desert moss Syntrichia caninervis (Pottiaceae). Int. J. Plant Sci. 176, 751–761. doi: 10.1086/682708
Pardow, A., and Lakatos, M. (2013). Desiccation tolerance and global change; implications for tropical bryophytes in lowland forests. Biotropica 45, 27–36. doi: 10.1111/j.1744-7429.2012.00884.x
Pashley, C. H., Fairs, A., Free, R. C., and Wardlaw, A. J. (2012). DNA analysis of outdoor air reveals a high degree of fungal diversity, temporal variability, and genera not seen by spore morphology. Fungal Biol. 116, 214–224. doi: 10.1016/j.funbio.2011.11.004
Pawlowska, T. E. (2005). Genetic processes in arbuscular mycorrhizal fungi. FEMS Microbiol. Lett. 251, 185–192. doi: 10.1016/j.femsle.2005.08.007
Pearce, D. A., Alekhina, I. A., Terauds, A., Wilmotte, A., Quesada, A., Edwards, A., Dommergue, A., et al. (2016). Aerobiology over Antarctica – a new initiative for atmospheric ecology. Front. Microbiol. 7:16. doi: 10.3389/fmicb.2016.00016
Peñaloza-Bojacá, G. F., Axevedo de Oliveira, B., Teixeira Araújo, C. A., Bubantz Fantecelle, L., and Maciel Silva, A. S. (2018). Bryophyte reproduction on ironstone outcrops: delicate plants in harsh environments. Flora 38, 155–161. doi: 10.1016/j.flora.2017.02.017
Pepe-Ranney, C., Koechli, C., Potrafka, R., Andam, C., Eggleston, E., Garcia-Pichel, F., et al. (2016). Non-cyanobacterial diazotrophs mediate dinitrogen fixation in biological soil crusts during early crust formation. Int. Soc. Microb. Ecol. J. 10, 287–298. doi: 10.1038/ismej.2015.106
Piñeiro, R., Popp, M., Hassel, K., Listl, D., Westergaard, K. B., Flatberg, K. I., et al. (2012). Circumarctic dispersal and long-distance colonization of South America: the moss genus Conclidium. J. Biogeogr. 39, 2041–2051. doi: 10.1111/j.1365-2699.2012.02765.x
Pirozynski, K. A. (1981). Interactions between fungi and plants through the ages. Can. J. Bot. 59, 1824–1825. doi: 10.1139/b81-243
Pohjamo, M., and Laaka-Lindberg, S. (2003). Reproductive modes in the epixylic hepatic Anastrophyllum hellenarium. Perspect. Plant Ecol. Evol. Systemat. 6, 159–168. doi: 10.1078/1433-8319-00074
Prasad, M., Chaudhary, M., Ramakrishnan, S., and Mahawer, S. K. (2018). Glomalin: a miracle protein for soil sustainability. Indian Farmer 5, 1092–1100.
Priyamvada, H., Akila, M., Singh, R. K., Ravikrishna, R., Verma, R. S., Philip, L., et al. (2017). Terrestrial macrofungal diversity from the tropical dry evergreen biome of southern India and its potential role in aerobiology. PLoS ONE 12:e016333. doi: 10.1371/journal.pone.0169333
Proctor, M. C. F., Oliver, M. J., Wood, A. J., Alpert, P., Stark, L. R., Cleavitt, N. L., et al. (2007). Desiccation-tolerance in bryophytes: a review. Bryologist 110, 595–621. doi: 10.1639/0007-2745(2007)110[595:DIBAR]2.0.CO;2
Rastogi, R. P., and Incharoensakdi, A. (2014). Characterization of UV-screening compounds, mycosporine-like amino acids, and scytonemin n the cyanobacterium Lyngbya sp. CU2555. FEMS Microbiol. Ecol. 87, 244–256. doi: 10.1111/1574-6941.12220
Reineria Diaz, M., Iglesias, I., and Jato, V. (1998). Seasonal variation of airborne fungal spore concentrations in a vineyard of North-West Spain. Aerobiologia 14, 221–227. doi: 10.1007/BF02694210
Reininger, V., Martinez-Garcia, L. B., Sanderson, L., and Antunes, P. M. (2015). Composition of fungal communities differs based on soil types and geographical location. AoB Plants 7:plv110. doi: 10.1093/aobpla/plv110
Rippin, M., Bourchhardt, N., Williams, L., Colesie, C., Jung, P., Büdel, B., et al. (2018). Genus richness of microalgae and cyanobacteria in biological soil crusts from Svalbard and Livingston Island: morphological versus molecular approaches. Polar Biol. 41, 909–923. doi: 10.1007/s00300-018-2252-2
Ronnås, C., Werth, S., Ovaskainen, O., Várkonyi, G., Scheidegger, C., and Snäll, T. (2017). Discovery of long-distance dispersal in a lichen-forming ascomycete. N. Phytol. 216, 216–226. doi: 10.1111/nph.14714
Roper, M., Seminara, A., Bandi, M. M., Cobb, A., Dillard, H. R., and Pringle, A. (2010). Dispersal of fungal spores on a cooperatively generated wind. Proc. Natl. Acad. Sci. 107, 17474–17479. doi: 10.1073/pnas.1003577107
Rosas, I., Escamilla, B., Calderon, C., and Mosiño, P. (1990). The daily variations of airborne fungal spores in Mexico City. Aerobiologia 6:153. doi: 10.1007/BF02539108
Rosentreter, R. (1993). Vagrant lichens in North America. Bryologist 96, 333–338. doi: 10.2307/3243861
Rosentreter, R., Bowker, M., and Belnap, J. (2007). A Field Guide to Biological Soil Crusts of Western U.S. Drylands. U.S. Government Printing Office, Denver, Colorado.
Rossi, F., and De Philippis, R. (2015). Role of cyanobacterial exopolysaccharides in phototrophic biofilms and in complex microbial mats. Life 5, 1218–1238. doi: 10.3390/life5021218
Sabariego, S., Díez, A., and Guttiérrez, M. (2007). Monitoring of airborne fungi in Madrid (Spain). Acta Botan. Croat. 66, 117–126. Available online at: https://hrcak.srce.hr/index.php?show=clanak&id_clanak=17179
Sandrone, S. (2014). Indagini lichenologiche e aerobiologiche in ambiente aperto in Valle d'Acosta. Revue Valdôtaine d'Histoire Naturelle 68, 17–23.
Scherer, S., Chen, T. W., and Böger, P. (1988). A new UV-A/B protecting pigment in the terrestrial cyanobacterium Nostoc commune. Plant Physiol. 88, 1055–1057. doi: 10.1104/pp.88.4.1055
Schimel, J. P., and Schaeffer, S. M. (2012). Microbial control over carbon cycling in soil. Front. Microbiol. 3:11. doi: 10.3389/fmicb.2012.00348
Schirrmeister, B. E., Antonelli, A., and Bagheri, H. C. (2011). The origin of multicellularity in cyanobacteria. BMC Evol. Biol. 11:45. doi: 10.1186/1471-2148-11-45
Scott, G. A. M. (1982). “Desert bryophytes,” in Bryophyte Ecology, ed A. J. E. Smith (Dordrecht: Springer), 105-122. doi: 10.1007/978-94-009-5891-3_4
Seyedmousavi, S., Netea, M. G., Mouton, J. W., Melchers, W. J., Verweij, P. E., and de Hoog, G. S. (2014). Black yeasts and their filamentous relatives: principles of pathogenesis and host defense. Clin. Microbiol. Rev. 27, 527–542. doi: 10.1128/CMR.00093-13
Shams-Ghahfarokhi, M., Aghaei-Gharehbolagh, S., Aslani, N., and Razzaghi-Abyaneh, M. (2014). Investigation on distribution of airborne fungi in outdoor environment in Tehran, Iran. J. Environ. Health Sci. Eng. 12:54. doi: 10.1186/2052-336X-12-54
Sharma, N. K., Rai, A. K., Singh, S., and Brown, R. M. Jr. (2007). Airborne algae: their present status and relevance. J. Phycol. 43, 615–627. doi: 10.1111/j.1529-8817.2007.00373.x
Sharma, N. K., and Singh, S. (2010). Differential aerosolization of algal and cyanobacterial particles in the atmosphere. Ind. J. Microbiol. 50, 468–473. doi: 10.1007/s12088-011-0146-x
Shaw, A. J., and Goffinet, B. (eds.). (2000). Bryophyte Biology. Cambridge: Cambridge University Press.
Shaw, A. J., Szövényl, P., and Shaw, B. (2011). Bryophyte diversity and evolution: windows into the early evolution of land plants. Am. J. Bot. 98, 352–369. doi: 10.3732/ajb.1000316
Shinn, E. A., Griffin, D. W., and Seba, D. B. (2003). Atmospheric transport of mold spores in clouds of desert dust. Arch. Environ. Health 58, 498–504. Available online at: https://pdfs.semanticscholar.org/a540/8ad168e1e95727a504ef7bb4792e5500c728.pdf
Shortlidge, E. E., Rosenstiel, T. N., and Eppley, S. M. (2012). Tolerance to environmental desiccation in moss sperm. N. Phytol. 194, 741–750. doi: 10.1111/j.1469-8137.2012.04106.x
Singh, P. K. (2012). Role of glomalin related soil protein produced by arbuscular mycorrhizal fungi: a review. Agricul. Sci. Res. J. 2, 119–125.
Slate, M. L., Stark, L. R., Greenwood, J. L., Clark, T. A., and Brinda, J. C. (2018). The role of prehydration in rescuing shoots of mosses damaged by extreme desiccation events: Syntrichia norvegica (Pottiaceae). Bryologist 121, 193–204. doi: 10.1639/0007-2745-121.2.193
Smith, D. J., Ravichandar, J. D., Jain, S., Griffin, D. W., Yu, H., Tan, Q., et al. (2018). Airborne bacteria in Earth's lower stratosphere resemble taxa detected in the troposphere: results from a new NASA aircraft bioaerosol collector (ABC). Front. Microbiol. 9:1752. doi: 10.3389/fmicb.2018.01752
Smith, D. J., Timonen, H. J., Jaffe, D. A., Griffin, D. W., Birmele, M. N., Perry, K. D., et al. (2013). Intercontinental dispersal of bacteria and archaea by transpacific winds. Appl. Environ. Microbiol. 39, 1134–1139. doi: 10.1128/AEM.03029-12
Soka, G., and Ritchie, M. (2014). Arbuscular mycorrhizal symbiosis and ecosystem processes: prospects for future research in tropical soils. Open J. Ecol. 4, 11–22. doi: 10.4236/oje.2014.41002
Somon, R. D. (1977). Sporulation in the filamentous cyanobacterium Anabaena cylindrica. The course of spore formulation. Arch. Microbiol. 111, 283–288. doi: 10.1007/BF00549367
Souffreau, C., Vanormelingen, P., Sabbe, K., and Vyverman, V. (2013a). Tolerance of resting cells of freshwater and terrestrial benthic diatoms to experimental desiccation and freezing is habitat-dependent. Phycologia 52, 246–255. doi: 10.2216/12-087.1
Souffreau, C., Vanormelingen, P., van de Vijver, B., Sheva, T., Verleyen, E., Sabbe, K., et al. (2013b). Molecular evidence for distinct Antarctic lineages in the cosmopolitan terrestrial diatoms Pinnularia borealis and Hantzschia amphioxys. Protist 164, 101–115. doi: 10.1016/j.protis.2012.04.001
Souffreau, C., Vanormelingen, P., Verleyen, E., Sabbe, K., and Vyverman, V. (2010). Tolerance of benthic diatoms from temperature aquatic and terrestrial habitats to experimental desiccation and temperature stress. Phycologia 49, 309–324. doi: 10.2216/09-30.1
Souza-Egipsy, V., Wierzchos, J., Sancho, C., Belmonte, A., and Ascaso, C. (2004). Role of biological soil crust cover in bioweathering and protection of sandstones in a semi-arid landscape (Torrollones de Gabarda, Huesca, Spain). Earth Surface Proc. Landforms 29, 1651–1661. doi: 10.1002/esp.1118
Stark, L. R., Brinda, J. C., and Greenwood, J. L. (2016a). Propagula and shoots of Syntrichia pagorum (Pottiaceae) exhibit different ecological strategies of desiccation tolerance. Bryologist 119, 181–192. doi: 10.1639/0007-2745-119.2.181
Stark, L. R., McLetchie, D. N., Greenwood, J. L., and Eppley, S. M. (2016b). Moss antheridia are desiccation tolerant: rehydration dynamics influence sperm release in Bryum argenteum. Am. J. Bot. 103, 856–864. doi: 10.3732/ajb.1600026
Stark, L. R., Mishler, B. D., and McLetchie, D. M. (2000). The cost of realized sexual reproduction: assessing patterns of reproductive allocation and sporophyte abortion in a desert moss. Am. J/ Bot. 7, 1599–1608. doi: 10.2307/2656736
States, J. S., Christensen, M., and Kinter, C. L. (2001). “Soil fungi as components of biological soil crusts,” in Biological Soil Crusts: Structure, Function, and Management, eds J. Belnap and O. L. Lange (Berlin: Springer-Verlag), 155–166. doi: 10.1007/978-3-642-56475-8_13
St. Clair, L. L., Johansen, J. R., and Rushforth, S. R. (1993). Lichens of soil crust communities in the Intermountain area of the western United States. Great Basin Naturalist 53, 5–12.
St. Clair, L. L., and Seaward, M. R. D. (eds.). (2004). Bio-Deterioration of Stone Surfaces. Dordrecht: Kluwer Academic Press.
Stȩpalska, D., and Wolek, J. (2005). Variation in fungal spore concentrations of selected taxa associated to weather conditions in Cracow, Poland, in 1997. Aerobiologia 21, 43–52. doi: 10.1007/s10453-004-5877-2
Steven, B., Gallegos-Graves, L., Belnap, J., and Kuske, C. R. (2013). Dryland soil microbial communities display spatial biogeographic patterns associated with soil depth and soil parent material. FEMS Microbiol. Ecol. 86, 101–113. doi: 10.1111/1574-6941.12143
Steven, B., Gallegos-Graves, L. V., Yeager, C., Belnap, J., and Kuske, C. R. (2014). Common and distinguishing features of the bacterial and fungal communities in biological soil crusts and shrub root zone soils. Soil Biol. Biochem. 69, 302–312. doi: 10.1016/j.soilbio.2013.11.008
Steven, B., Hesse, C., Gallegos-Graves, L. V., Belnap, J., and Kuske, C. R. (2015). “Chapter E: Fungal diversity in biological soil crusts of the Colorado Plateau,” in Proceedings of the 12th Biennial Conference of Research on the Colorado Plateau, U.S. Geological Survey Scientific Investigations Report 2015-5180, ed B. E. Ralston (Reston, VA), 41–47. doi: 10.3133/sir20155180E
Takeuchi, N. (2001). Structure, formation, and darkening process of albedo-reducing material (Cryoconite) on a Himalayan glacier: a granular algal mat growing on the glacier. Arct. Antarct. Alpine Res. 33, 115–122. doi: 10.1080/15230430.2001.12003413
Tesson, S. V. M., and Šanti-Temkiv, T. (2018). Ice nucleation activity and aeolian dispersal success in airborne and aquatic microalgae. Front. Microbiol. 9:2681. doi: 10.3389/fmicb.2018.02681
Tesson, S. V. M., Skjøth, C. A., Šanti-Temkiv, T., and Löndahl, J. (2016). Airborne microalgae: insights, opportunities, and challenges. Appl. Environ. Microbiol. 82, 1978–1991. doi: 10.1128/AEM.03333-15
Tisdall, J. M. (1991). Fungal hyphae and structural stability of soil. Austral. J. Soil Res.29, 729–743. doi: 10.1071/SR9910729
Tisdall, J. M., Nelson, S. E., Wilkinson, K. G., Smith, S. E., and McKenzie, B. M. (2012). Stabilization of soil against wind erosion by six saprotrophic fungi. Soil Biol. Biochem. 50, 134–141. doi: 10.1016/j.soilbio.2012.02.035
Tormo, R., Recio, D., Silva, I., and Muñoz, A. F. (2001). A quantitative investigation of airborne algae and lichen soredia obtained from pollen traps in south-west Spain. Eur. J. Phycol. 36, 385–390. doi: 10.1080/09670260110001735538
Torrecillas, E., Alguacil, M. M., and Roldán, A. (2012). Host preferences of arbuscular mycorrhizal fungi colonizing annual herbaceous plant species in semiarid Mediterranean prairies. Appl. Environ. Microbiol. 78, 6180–6186. doi: 10.1128/AEM.01287-12
Tripp, E. A., Lendemer, J. C., Barberán, A., Dunn, R. R., and Fierer, N. (2016). Biodiversity gradients in obligate symbiotic organisms: exploring the diversity and traits of lichen propagules across the United States. J. Biogeogr. 43, 1667–1678. doi: 10.1111/jbi.12746
van der Heijden, M. G. A., Klironomos, J. N., Ursic, M., Moutoglis, P., Streitwolf-Engel, R., Boller, T., et al. (1998). Mycorrhizal fungal diversity determines plant biodiversity, ecosystem variability and productivity. Nature 396, 69–72. doi: 10.1038/23932
Van Eaton, A. E., Harper, M. A., and Wilson, C. J. N. (2013). High-flying diatoms: widespread dispersal of microorganisms in an explosive volcanic eruption. Geology 41, 1187–1190. doi: 10.1130/G34829.1
van Overeem, M. A. (1937). On green organisms occurring in the lower troposphere. Recueil des Trav. Botaniques Neerlandais 34, 388–442.
van Zanten, B. O. (1976). Preliminary report on germination experiments designed to estimate the survival chances of moss spores during aerial trans-oceanic long-range dispersal in the southern hemisphere, with particular reference to New Zealand. J. Hattori Bot. Lab. 41, 133–140.
van Zanten, B. O. (1978). Experimental studies on trans-oceanic long-range dispersal of moss spores in the southern hemisphere. J. Hattori Bot. Lab. 44, 455–482.
van Zanten, B. O., and Pocs, T. (1981). Distribution and dispersal of bryophytes. Adv. Bryol. 1, 479–562.
Vanormelingen, P., Verleyen, E., and Vyverman, W. (2008). The diversity and distribution of diatoms: from cosmopolitanism to narrow endemism. Biodiver. Conserv. 17, 393–405. doi: 10.1007/s10531-007-9257-4
Warren, S. D. (2014). “Role of biological soil crusts in desert hydrology and geomorphology: implications for military training operations,” in Military Geosciences in the Twenty-First Century, Vol. 22, eds R. S. Harmon, S. E. Baker, and E. V. McDonald (Boulder, CO: Geological Society of America). doi: 10.1130/2014.4122(16)
Warren, S. D., St. Clair, L. L., and Leavitt, S. D. (2019). Aerobiology and passive restoration of biological soil crusts. Aerobiologia 35, 45–56. doi: 10.1007/s10453-018-9539-1
Waterbury, J., and Stanier, R. (1977). Two unicellular cyanobacteria which reproduce by budding. Arch. Microbiol. 115, 249–257. doi: 10.1007/BF00446449
Weber, B., Büdel, B., and Belnap, J. (eds.). (2016). Biological Soil Crusts: An Organizing Principle in Drylands. Ecological Studies 226. Cham: Springer.
Weil, T., De Filippo, C., Albanese, D., Donati, C., Pindo, M., Pavarini, L., et al. (2017). Legal immigrants: invasion of alien microbial communities during winter occurring desert dust storms. Microbiome 5:32. doi: 10.1186/s40168-017-0249-7
Whitton, B. A., and Potts, M. (eds.). (2000). The Ecology of Cyanobacteria: Their Diversity in Time and Space. New York, NY: Kluwer Academic Publishers.
Wickett, N. J., and Goffinet, B. (2008). Origin and relationships of the myco-heterotrophic liverwort Cryptothallus mirabilis Malmb. (Metzgeriales, Marchantiophyta). Bot. J. Linn. Soc. 56, 1–12. doi: 10.1111/j.1095-8339.2007.00743.x
Womack, A. M., Artaxo, P. E., Ishida, F. Y., Mueller, R. C., Saleska, S. R., Wiedemann, K. T., et al. (2015). Characterization of active and total fungal communities in the atmosphere over the Amazon rainforest. Biogeosciences 12:6337–6349. doi: 10.5194/bg-12-6337-2015
Wornik, S., and Grube, M. (2010). Joint dispersal does not imply maintenance of partnerships in lichen symbioses. Microb. Ecol. 59, 150–157. doi: 10.1007/s00248-009-9584-y
Keywords: biological soil crusts (BSCs), bacteria, fungi, terrestrial algae, bryophytes, reproduction, aerial dispersal
Citation: Warren SD, St. Clair LL, Stark LR, Lewis LA, Pombubpa N, Kurbessoian T, Stajich JE and Aanderud ZT (2019) Reproduction and Dispersal of Biological Soil Crust Organisms. Front. Ecol. Evol. 7:344. doi: 10.3389/fevo.2019.00344
Received: 27 June 2019; Accepted: 28 August 2019;
Published: 04 October 2019.
Edited by:
Oana Moldovan, Emil Racovita Institute of Speleology, RomaniaReviewed by:
Laura Concostrina-Zubiri, Rey Juan Carlos University, SpainJosef Elster, University of South Bohemia, Czechia
Copyright © 2019 Warren, St. Clair, Stark, Lewis, Pombubpa, Kurbessoian, Stajich and Aanderud. This is an open-access article distributed under the terms of the Creative Commons Attribution License (CC BY). The use, distribution or reproduction in other forums is permitted, provided the original author(s) and the copyright owner(s) are credited and that the original publication in this journal is cited, in accordance with accepted academic practice. No use, distribution or reproduction is permitted which does not comply with these terms.
*Correspondence: Steven D. Warren, U3RldmUuV2FycmVuQHVzZGEuZ292