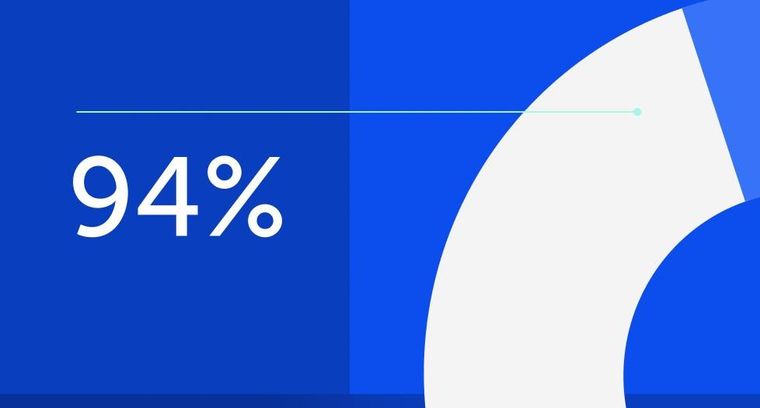
94% of researchers rate our articles as excellent or good
Learn more about the work of our research integrity team to safeguard the quality of each article we publish.
Find out more
PERSPECTIVE article
Front. Ecol. Evol., 06 September 2019
Sec. Chemical Ecology
Volume 7 - 2019 | https://doi.org/10.3389/fevo.2019.00332
This article is part of the Research TopicDiversity and Evolution of Animal Venoms: Neglected Targets, Ecological Interactions, Future PerspectivesView all 10 articles
Despite the fact that venom is an intrinsically ecological trait, the ecological perspective has been widely neglected in toxinological research. This neglect has hindered our understanding of the evolution of venom by causing us to ignore the interactions which shape this evolution, interactions that take place between venomous snakes and their prey and predators, as well as among conspecific venomous snakes within populations. In this opinion piece, we introduce and briefly discuss several ecologically oriented concepts that may be of interest to toxinologists, before reviewing a range of non-front-fanged snake taxa that have been neglected toxinologically, but which represent the majority of extant ecological diversity amongst snakes. We conclude by noting that the ecological perspective even has something to offer to clinical toxinology, in the wake of the World Health Organization reinstating snakebite envenoming to its list of Neglected Tropical Diseases.
Venom is a functional trait, used by one organism to subjugate or deter another (Jackson and Fry, 2016). Without this relationship between the venomous and the envenomed, it makes no sense to speak of “venom” – an organism may produce a plethora of potentially toxic compounds, but if they have not been selected for functional deployment in the subjugation or deterrence of other organisms, they are not venom. Thus, venom is an intrinsically relational trait. Ecology is the study of relationships amongst organisms and with their environment, therefore venom is “ecological” by definition. Despite this simple truth, the study of venom ecology—the usage and evolution of venom in context—has been a relatively neglected area in snake venom research and in toxinology more broadly. In the following opinion piece, we briefly discuss several ecologically-oriented concepts that have been neglected in venom research, but which we feel are worth considering within the field. Following this, we review some of the extant diversity of non-front-fanged venomous snake species which have received little to no attention in toxinological circles.
The importance of context for the evolution of venom begins at the molecular level. Proteins and the genes that encode them exist in their own interdependent ecological webs. Genes are located within structured genomes and their location within these networks has a profound impact on their evolution. For example, the arrangement of a genomic neighborhood affects the propensity that a given segment (containing one or more “genes”) within that region has for duplicating (Reams and Roth, 2015). Not only is this broadly relevant to the origin of novel functions via a number of pathways in which duplication plays a role, it is specifically relevant to the evolution of venom, as many important toxins are members of gene families which have expanded through duplication (Fry et al., 2009). Proteins themselves have been described as “fundamentally relational entities” (Guttinger, 2018), since they must interact with other molecules in order to effect their own functional roles—a protein in isolation is impotent. Exophysiological proteins, such as venom toxins, which interact with targets in the bodies of prey or predators (i.e., secondary organisms) vividly illustrate this point. However, it is no less the case for endophysiological molecules functioning within the ecosystem of other molecules that comprises the physiology of the primary organism. Furthermore, the ecology of molecules consists of more than molecular interactions and extends to the “environmental” features, such as pH and temperature, which make these interactions possible and influence their rate, potency etc.
Once the role of context in influencing the function of a protein is acknowledged, the importance of changes in context for the evolution of novel functions becomes clear. Again, the evolution of venom toxins, in particular their “recruitment” into venom arsenals, is instructive. In a recent study, Koludarov et al. (2019) reconstructed the history of the phospholipase A2g2 gene family, from which all viperid snake phospholipase A2 toxins originate. In tracking all duplication events that have occurred within the family since the most recent common ancestor of amniotes, the authors noted that duplication seemed to follow the origin of novel functions, rather than predate it as in Ohno's (1970) influential “neofunctionalization” model. In consideration of the recruitment of this family as a toxin in toxicoferan reptiles, it was inferred that this novel function arose when a shift in tissue-specific patterns of gene expression facilitated an association between this secretory protein and the dental glands of an early toxicoferan or ancestral snake. The association of this enzyme with an incipient delivery system was the first step toward exposing it to a radically novel context. Whilst the gene family had been ancestrally involved in innate immunity, fighting pathogens in an endophysiological context, its secretion in dental glands made possible its exophysiological deployment against other multicellular organisms. However, it was not until a delivery system capable of inoculating this potential toxin deeply into a prey organism's tissue evolved that the genuine functional novelty of a role within venom emerged in this gene family (Koludarov et al., 2019). Thus, it is only in viperid snakes that these proteins became a significant part of venom, a novel function precipitated by the exposure of the molecule to a novel context. Selection for the toxin function in turn enabled viper-specific expansions of the gene family, with associated neofunctionalizations.
The aforementioned role of secretory context in the origins of novel protein functions is highly analogous to the concepts of ecological opportunity and ecological release. These concepts have been central to discussions of adaptive radiation for at least 70 years (e.g., Simpson, 1949). According to such models, adaptive radiations occur following colonization of new environments, the origin of a novel functional trait/innovation, or escape from antagonistic/competitive species (Gavrilets and Vose, 2005; Yoder et al., 2010). Consideration of the origins of novel functions, however, reveals their similarity to the colonization of new environments—a change of context at either the protein or the organismal level results in opportunity for diversification. Furthermore, “escape from adaptive conflict” is a term used to describe a subfunctionalization process in which gene duplication facilitates the distribution of the multiple functions possessed by a “parent” gene amongst its duplicate “offspring” (Innan and Kondrashov, 2010). This segregation allows each gene to specialize for a particular function, a process often accompanied by segregation of the expression patterns of each gene product. Highlighting the close analogy between gene and organismal evolution, “escape and radiate coevolution” describes a process in which an organism evolves a novel trait which allows it to (literally) escape the adaptive constraints imposed upon it by an antagonistic species, opening a pathway to further diversification (see below for further discussion of coevolution).
“Evolvability” is a term that has been used to refer to a variety of phenomena influencing the propensity of a lineage to diversify and evolve novel functions. This has been described as the “evolutionary potential” of the lineage (Sterelny, 2007) and as “the ability of a biological system to produce phenotypic variation that is both heritable and adaptive” (Payne and Wagner, 2019). More specifically, evolvability is the result of unselected properties (e.g., mutation rate) a lineage possesses that constrain or facilitate that lineage's access to areas of “phenotype space” (i.e., the range of possible future phenotypes available to that lineage—Brown, 2014). This definition remains problematic, however, because the degree to which such properties remain unselected is controversial. Regardless of this point, venom genes, which appear to evolve rapidly (Chang and Duda, 2012; Casewell et al., 2013; Sunagar et al., 2013) are excellent candidates for research aimed at understanding the factors influencing evolvability.
Although a full treatment of the evolvability of venom is beyond the scope of this article, a few points are worth mentioning. Evolvability is increased in lineages that are “robust” enough to acquire variation neutrally (Wagner, 2012; Jackson et al., 2016). At the molecular level, this neutral variation is the substrate from which novelty emerges—unselected changes may result in the capricious discovery of functional novelty, upon which selection subsequently acts. This process highlights the close relation between “exaptation” (Gould and Vrba, 1982) and evolvability—the former describes an unselected property becoming a positively selected function (Jackson and Fry, 2016), whereas the latter describes the general propensity for this to occur within a lineage. The more neutral variation an evolving lineage can accumulate, the higher the chance of such a fortuitous occurrence and hence the more “evolvable” that lineage becomes.
Toxin genes have been independently recruited in diverse venomous taxa from a number of gene families that possess properties that facilitate the accumulation of neutral variation (Fry et al., 2009; Casewell et al., 2013). One such property is a high apparent rate of duplication—venom genes often originate from within multi-copy gene families and once a locus is recruited to a venom system further locus-specific expansion frequently takes place (Fry et al., 2009; Koludarov et al., 2019). The propensity for a locus to duplicate is believed to be an unselected consequence of its genomic context—the arrangement of genes surrounding it (Reams and Roth, 2015). Thus, it is not only the context in which a gene product is deployed that influences its functional evolution, but also the intragenomic context in which it is located. As with other factors influencing evolvability, it is controversial whether or not duplication propensity is always unselected, or rather might be positively selected in certain contexts. Evidence of increased duplication rates within sub-families of genes that have been recruited as venom toxins suggests that in some cases the origins of a novel function may lead to selection for increased duplication, or at least influence the preservation of duplicate genes (see Koludarov et al., 2019 for detailed discussion).
Direct study of the ecological relationships between venomous snakes and their prey, predators, and conspecifics (which may also be predators or prey!) presents many challenges. It is far easier to simply collect venom, analyse it in a laboratory setting, and then correlate the resultant data with previously accumulated knowledge of diet and behavior, than it is to perform integrated “eco-toxinological” studies. Correlating previously collected dietary data with new data on venom composition and activity is illuminating and may result in the generation of hypotheses that guide future research in venom evolution (e.g., Jackson et al., 2016; Zancolli et al., 2019). Adequately testing these hypotheses, however, often requires a more direct approach (e.g., prey-handling experiments; taxon-specific toxicity testing, etc.), which may be difficult to implement (although see Barlow et al., 2009 for a nice example of such work). The simple issue of practicality goes a long way toward explaining the neglect of ecology within toxinology. However, pragmatism should not prevent venom researchers from formulating research questions from an ecologically informed perspective. Given the intrinsic interdependence of venomous organisms and their prey, as well as the interdependence of both with the broader environment, ecology cannot fail to have a profound influence on the evolution of venom.
One area of research that is considerably limited by the challenges of conducting genuinely “ecological” venom research concerns cost-benefit analyses of venom production and deployment. Although an interesting literature exists on this subject (see Morgenstern and King, 2013; Evans et al., 2019), it typically concentrates on the cost of venom, whilst neglecting the benefit it confers. Venomous organisms may utilize various methods (e.g., venom metering; secretions with reduced protein content, etc.) to minimize the cost of venom, but effectively investigating whether or not venom is a “costly” trait, relative to other defensive and offensive strategies, requires an integrated approach. The metabolic cost of venom must be compared to that of other strategies, e.g., constriction in snakes, and the costs of each strategy offset against the energetic gains made accessible by their deployment. There are no free lunches in nature and it may be that venom is a particularly cost-effective strategy for acquiring larger amounts of energy in a single meal (i.e., subduing a larger prey item) than would otherwise be possible. Unfortunately, the kind of experiments required to comprehensively investigate these questions are complicated and costly (and may not provide the requisite career gains to offset these costs!).
The primary function of venom for venomous snakes is prey subjugation (Jackson and Fry, 2016). Although many studies have attempted to correlate venom composition with prey type, the nature of the interaction between predator and prey is influenced by far more than the species (or order) that each belongs to. Additional considerations may include the physiological states of both organisms at the time of the encounter, as well as their relative body sizes. Brief examples will serve to illustrate each of these points.
The physiological state of homeothermic endotherms (e.g., typical small mammals) may be relatively constant, whereas that of poikilothermic ectotherms is widely varying. Snakes that feed predominantly upon mammals, therefore, may have options unavailable to those that specialize in feeding upon reptiles. These options may include a high percentage of enzymatic toxins, which are rate-limited by temperature, in their venom (Jackson et al., 2016). Such toxins may be additionally favored for deployment against mammals because of the rapidity of their action against prey which are potentially dangerous and possess a capacity for sustained exertion which far exceeds that of a snake. The situation is considerably more complex for snakes feeding upon poikilothermic reptiles whose physiological state fluctuates widely according to time of day, level of activity, and seasonally (Pough et al., 2016). For a snake that hunts lizards during the day, when the lizards are themselves active and may have been basking or otherwise maintaining an elevated body temperature and metabolic rate, enzymatic toxins may still be an effective strategy. On the other hand, for a snake that feeds on the same species of lizard at night, when it is sheltering and its metabolic rate is depressed, another strategy, perhaps non-enzymatic neurotoxins coupled with constriction, may be preferable.
One of the obvious advantages of venom as a predatory strategy is the ability it confers to subdue larger prey than would be possible with purely physical means. For snakes, there may be trade-offs among prey size (snake), mobility, and subjugation strategy (e.g., venom vs. constriction). Regardless, even for a venomous snake, the relative size of a prey animal may exert considerable influence on the outcome of a predation attempt. Prior conceptions of venom as (definitionally) causing “rapid prey death” have long obscured the details of the “battle” that may take place when a venomous snake encounters a potential prey item. The view has often seemed to be that the primary challenge for the snake is to get close enough to strike successfully, after which the venom takes care of the rest. For a generation of researchers which honed its intuitions by studying rattlesnakes, this is entirely reasonable. As evolutionary toxinologists have diversified their research interests, however, the fact that rattlesnakes (along with mambas, taipans, and other venomous snakes employing a bite-and-release strategy) are exceptional cases, and “rapid prey death” (which is conceptually distinct from rapid incapacitation, but may be equivalent to it at the level of selection—Jackson and Fry, 2016) may be an unusual rather than typical consequence of envenoming, has become clear (Fry et al., 2012). In contrast, struggles between venomous snakes and some prey animals may take many minutes or even hours to resolve and there is no guarantee of success for the snake even after delivering its venom.
Observations of snakes feeding upon frogs, for example, vary from occasions in which the amphibians are consumed within seconds, apparently whilst still alive, to drawn out battles. The key variable here does not seem to be the potency of the snake's venom, or the sophistication of its venom delivery system, but rather the size of the frog relative to the size of the snake's head. One of the authors (TNWJ) has observed rear-fanged tree snakes from the genera Boiga and Dendrelaphis swallowing small frogs rapidly (almost too quickly to take photographs) and conversely observed front-fanged elapid snakes struggling to subdue larger frogs over periods exceeding 1 h. In a situation in which predator and prey are almost evenly matched physically, the “chemical edge” provided by venom may be a decisive factor. Indeed, this edge (though initially slight) is likely what first allowed the venomous function to be positively selected in ancestral snakes or even ancestral toxicoferan lizards (Fry et al., 2013; Jackson et al., 2017). Long before venom could rapidly subdue prey, it may have only slightly weakened it. This much is intuitively obvious, what may be less so is the fact that a snake possessing an “advanced high-pressure delivery system” and venom that could subdue a mouse in seconds may have to battle a frog for an hour and still not be guaranteed a meal. The interaction of prey type, size, and physiological state must therefore all be considered if we are to bring a genuinely ecological perspective to toxinological research.
As mentioned above, one of the factors traditionally associated with adaptive radiation is “escape” from antagonistic taxa. This form of adaptive radiation is often referred to as “escape and radiate coevolution” (Hui et al., 2015), and the evolution of chemical weaponry, both offensive and defensive, represents a prime example of this dynamic. Coevolutionary arms races are often invoked to account for diversity in venom composition and activity, as well as the dynamic molecular evolution of toxin genes (e.g., Casewell et al., 2013). On the other hand, interest in the evolution of resistance to venom in both the prey and predators of venomous organisms has also increased recently (after a long history of piecemeal research), highlighted by the publication of two excellent reviews (Holding et al., 2016 and Arbuckle et al., 2017) detailing the multiple molecular innovations that confer this resistance. The evolution of chemical weaponry and the reciprocal evolution of resistance may be one of the most important drivers of functional diversity at the molecular level. Thus, the fledgling field of snake venom eco-toxinology has the luxury of being able to draw upon insights from investigations of ecological phenomena as seemingly disparate as plant-herbivore coevolution, responsible for a huge variety of plant secondary metabolites (Fraenkel, 1959) and antimicrobial drug-resistance, which represents “an increasingly serious threat to global public health” (WHO, 2018).
Consideration of the interactions between venomous snakes and their prey/predators is instructive, but it should not obscure the fact that one of the primary drivers of evolution by natural selection is competition amongst varying phenotypes within populations—a snake's conspecifics are at least as influential on the evolution of its venom as its prey are. A recent study (Zancolli et al., 2019) of Mojave rattlesnakes (Crotalus scutulatus), a species that exhibits two distinct venom phenotypes, serves to illustrate this point, as well as the influence of other environmental factors. The study found no discernible difference in diet between Type A (neurotoxic) and Type B (haemotoxic) snakes. However, a strong association was found between venom type and climate, in which the neurotoxic type was found in regions with cooler winters and higher rainfall. Several possible explanations for this pattern are discussed in the paper, including climatic effects on prey availability, which may influence snake foraging strategies, in turn influencing exposure of the snakes to their own predators (a nice illustration of the interdependence of ecological factors). Insightfully, the authors point out that within widely distributed species, which occupy a diversity of environments across that distribution, there may be selection for locally optimal strategies leading to intraspecific diversity. Continuing this line of reasoning, we conjecture that these local optima could also result from the effect of climate on the taxon-specific effectiveness of specific venom compositions, and that the proximal driver of selection may be competition between the two strategies (as opposed to local prey availability, which would be a more distal pressure). Thus, whilst both strategies might be broadly successful, each possesses a slight competitive edge over the other in its “preferred” climate. Type B snakes rely on enzymatic metalloprotease (SVMP) toxins, which are likely rate-limited by temperature. The pre-synaptic neurotoxins favored in the Type A phenotype, on the other hand, are phospholipases which act non-enzymatically and are thus less affected by temperature. C. scutulatus are generalist predators and their diets include up to 30% reptiles alongside the more commonly consumed mammalian prey (Zancolli et al., 2019). As previously discussed, mammals, with their elevated metabolic rates, are often rapidly subdued by enzymatic toxins, but the effectiveness of these toxins against reptilian prey may vary according to the physiological state of the latter. In areas with milder winters, SVMPs may be a highly effective strategy for the rattlesnakes, but where temperature is more variable (a factor also influenced by precipitation rates) snakes with a rate-limited toxin arsenal maybe out competed by those with a slower-acting, but temperature invariant, neurotoxic strategy.
Heritable phenotypic variation within populations is a precondition of evolution by natural selection (Godfrey-Smith, 2007). Venom is a highly evolvable trait (see above), and it is thus unsurprising that we should see intraspecific variation in venom phenotypes. This variation is rarely likely to be as neat as that documented for C. scutulatus, however. Another recent study (Smiley-Walters et al., 2019) has demonstrated considerable intra-populational variation in the toxicity of venoms of individual pygmy rattlesnakes (Sistrurus miliarius) to lizards. This work is in its early stages, but it is already significant in adding another wrinkle to our investigations of the evolution and ecology of snake venoms. Variation was found to be considerably higher within the population than between populations and each of these variants may be considered an incipient “strategy,” though the majority of them will likely be transient. The authors described the variation as “functional,” however, in evolutionary terms it might well be epiphenomenal (unselected/neutral—see Jackson and Fry, 2016). Again, a large amount of unselected phenotypic variation is exactly what we should expect to see in a highly evolvable trait—such neutral variation is the substrate from which functional variation grows as effective strategies are hit upon by chance and give their bearers a slight competitive edge over other members of the population. As the authors comment, follow-up studies using additional prey species may show whether there are trade-offs associated with variation in toxicity toward lizards (Smiley-Walters et al., 2019) and thus whether the variation is truly functional.
The general neglect of an ecological perspective in toxinology has resulted in the neglect of the vast majority of ecological (and phenotypic) variants amongst extant snakes. As before, there are pragmatic reasons for this—venom research has naturally focused on the most common and most dangerous species of snake, and many venom researchers come from either clinical or biochemical backgrounds and may thus be unfamiliar with the cornucopia of research riches represented by snake diversity.
Snakes are the most species-rich squamate clade, numbering more than 3,700 extant species (Uetz et al., 2019). Our understanding of their higher-level relationships has recently progressed thanks to several molecular studies (reviewed in Miralles et al., 2018; Streicher and Ruane, 2018). They include ~500 species of paraphyletic and fossorial “Scolecophidia” (“worm snakes”), and ~3,200 species of Alethinophidia (“typical snakes”), almost 3,000 of them being Caenophidia (“advanced snakes”). Caenophidia includes three species of aglyphous file snakes (Acrochordidae), ~740 front-fanged venomous snakes including Elapidae, Viperidae, and Atractaspidinae (genera Atractaspis and Homoroselaps only), and several lineages formerly known as “colubrids” i.e., Caenophidia devoid of a front-fanged venom system. Although authors continue to debate the subfamilial/familial rank of some of these lineages, they mostly agree on their content, and we will here recognize the following eight families: Xenodermidae, Pareidae, Homalopsidae, Lamprophiidae, Pseudoxenodontidae, Dipsadidae, Natricidae, and Colubridae (Uetz et al., 2019).
Snakes are all carnivorous. The spectrum of their diets is huge, ranging from the eggs of social insects to large mammals. Immobilization is achieved by simply holding the prey firmly within the mouth, encircling the prey in body coils and applying pressure to cause asphyxiation or cardiovascular dysfunction (constriction), or injecting the prey with toxic venom (Lillywhite, 2014). Snake venoms and their associated delivery apparatuses are integrated systems with established functions of subduing prey, and deterring predators (Jackson et al., 2016). They are much more widespread among snakes than previously thought (Vidal, 2002). Moreover, snakes display an exceptional diversity of oral glands (including venom glands), the functions of which remain unknown in many cases (Jackson et al., 2017).
Venoms and toxins of front-fanged dangerous snakes have been the subject of the vast majority of toxinological studies, but snakes devoid of a front-fanged venom system are much more diverse ecologically and phylogenetically and may therefore harbor an untapped potential of new toxins or toxin families. As several studies have found a relationship between diet and venom composition (da Silva and Aird, 2001; Barlow et al., 2009; Jackson et al., 2016; Modahl et al., 2018; Healy et al., 2019), we will here focus on snake lineages with particular diets and/or lineages that have been particularly neglected from a toxinological point of view.
Although this assemblage is paraphyletic, all “scolecophidian” snakes feed primarily on insects, most notably social insects and their eggs (with a few exceptions such as the genus Acutotyphlops that feeds on earthworms). Phisalix (1922) first described temporomandibular glands that seem to be composed of serous secretory cells and include a duct. Two recent studies have brought surprising results. Using magnetic resonance imaging, Jackson et al. (2017) identified very large supralabial glands in a typhlopid while Martins et al. (2018) found that the largest glands in leptotyphlopids were infralabial ones. This is significant as leptotyphlopids ingest prey using a specialized mechanism named mandibular raking while typhlopids use an equally specialized maxillary mechanism (Kley and Brainerd, 1999; Kley, 2001). Further investigations of the function and composition of these well-developed serous glands are much needed.
Also called “mud snakes,” this family includes ~55 species, all Asian, most of which are found in estuarine, marine, or freshwater environments. Apart from nine species (genera Brachyorrhos, Calamophis, Karnsophis) that feed primarily on earthworms, they are rear-fanged snakes considered to be mildly venomous. These rear-fanged species feed mostly on aquatic vertebrates (fish and amphibians), but two of them (genera Gerarda, Fordonia) are specialized on crustaceans (crabs) that they grapple with, envenom and dismember before eating (Murphy and Voris, 2014).
The gland transcriptome and venom proteome of one species (Cerberus rhynchops) has been analyzed, resulting in the discovery of a new snake venom protein family named veficolins (OmPraba et al., 2010). Homalopsidae is therefore a promising group in terms of bioprospecting.
The subfamily Psammophiinae includes seven genera and 53 species that are all rear-fanged. Most species are active diurnal snakes and opportunistic predators feeding on lizards, frogs and mammals (Pough et al., 2016). With a few exceptions (Psammophis mossambicus, Fry et al., 2008; Brust et al., 2013), psammophiine venoms haven't been studied, although a new neurotoxin (rufoxin) was isolated from Ramphiophis oxyrhynchus by Lumsden et al. (2007).
The subfamily Pseudoxyrhophiinae is the main snake radiation in Madagascar and nearby Comoro Islands with 19 endemic genera and 84 species. The genera Alluaudina, Compsophis, Ithycyphus, Langaha, Leioheterodon, Madagascarophis, Micropisthodon, Parastenophis, Phisalixella are rear fanged and display of large variety of phenotypes and ecology (Ruane et al., 2015). With the exception of Leioheterodon madagascariensis (Fry et al., 2008), the composition of their venoms is unknown.
In addition to the well-studied case of some saw-scaled vipers (genus Echis) feeding on scorpions (Barlow et al., 2009), several non-front fanged caenophidian species are specialized for feeding upon potentially dangerous arthropod prey such as scorpions, centipedes and spiders. Moreover, they belong to different families: Lamprophiidae, Colubridae and Dipsadidae. To our knowledge, the venoms from these arthropod-eating species have not been studied. Among Lamprophiidae, the subfamily Atractaspidinae includes the rear-fanged genus Aparallactus (11 species) that feeds on centipedes (with the exception of Aparallactus modestus, a fangless species that feeds on earthworms; Portillo et al., 2018, 2019).
Among Colubridae, at least 13 genera including 95 species (Chionactis, Chilomeniscus, Conopsis, Ficimia, Geagras, Gyalopion, Pseudoficimia, Scolecophis, Sonora, Stenorrhina, Sympholis, Tantilla, Tantillita) feed on scorpions, centipedes and spiders. It is worth noting that almost all if not all of these genera belong to one tribe named Sonorini. Among Dipsadidae, at least one species, Philodryas agassizii, is known to feed upon spiders, which is first neutralizes with venom (Marques et al., 2006). In addition to their venoms, the possible mechanisms of immunity of these snakes to the bites of their dangerous prey remain to be investigated.
Snakes belonging to the Neotropical tribe Dipsadini (family Dipsadidae) mostly feed on gastropod molluscs (hence the common name of “snail-eating” snakes) and possess a hypertrophied protein-secreting infralabial gland (de Oliveira et al., 2008). The secretions of this gland are toxic to the snake's molluscan prey (Laporta-Ferreira and Salomaõ, 1991; Salomaõ and Laporta-Ferreira, 1994), although a recent study emphasizes a role in mucus control and transport (Zaher et al., 2014; see Jackson et al., 2017 for further discussion). The tribe includes ~80 species belonging to four genera (Dipsas, Plesiodipsas, Sibon, Tropidodipsas) but none of their venom systems have been investigated proteomically or transcriptomically. Of additional interest is their rear-fanged sister-group (genus Ninia, 11 species) that feeds on the same prey but without the specialized mandibular protein-secreting system.
At least 3 other dipsadid genera feed on molluscs: Contia (2 North American carphophiine species) has long needle-like teeth on its mandibles, probably an adaptation to gripping and eating slugs (Greene, 1997), while Calamodontophis and Tomodon (5 South American xenodontine species) possess specialized long needle-like teeth on their maxillaries (Bizerra et al., 2005).
Convergently, the Asian family Pareidae (20 species belonging to the genera Aplopeltura, Asthenodipsas, Pareas) has adopted a similar diet and associated mandibular specialization as the Dipsadini, but have been even less studied than their Neotropical counterparts.
Among the mostly African/Malagasy family Lamprophiidae, the genus Duberria (four species) feeds on molluscs only and the monotypic genus Micropisthodon is suspected to do the same due to its morphology, which resembles that displayed by Pareidae and Dipsadini (O'Shea, 2018). Finally the genus Storeria (five species, Natricidae) also includes mollusks in its diet (Rossman and Myer, 1971).
Given their potential danger or particular morphology, scalation and strength, this kind of prey requires subjugation by constriction and/or envenomation.
Among snakes considered to be “basal,” two unrelated lineages deserve particular interest: Aniliidae (Anilius scytale) and Cylindrophiidae (Cylindrophis, 14 species, and possibly Anomochilus, 3 species; Gower et al., 2005). Aniliidae feeds mostly on amphisbaenians and snakes while Cylindrophis commonly includes snakes in its diet in addition to “invertebrates” and other elongate vertebrates such as eels (Greene, 1997). Neither of these lineages is an effective constrictor and both of them possess large serous-secreting rictal glands, which may secrete 3-finger toxins (Fry et al., 2013). This system may therefore play a functional role in prey subjugation (Jackson et al., 2017).
Several non-front-fanged caenophidian species predominantly feed upon snakes/amphisbaenians. Among Dipsadidae, this includes the following rear-fanged genera: Boiruna, Clelia, Mussurana, Paraphimophis, and Pseudoboa (18 species belonging to the tribe Pseudoboini); Apostolepis, Elapomorphus and Phalotris (53 species, tribe Elapomorphini; Lema et al., 1983; Alencar et al., 2013; Gaiarsa et al., 2013), as well as Erythrolamprus (six species, tribe Xenodontini;Wallach et al., 2014; Marques et al., 2016; Sánchez et al., 2019). The venom composition of Phalotris mertensi has been studied appears to be distinct from that of other non-front-fanged species as it includes a unique snake venom acid lipase (svLIPA) (Campos et al., 2016; Junqueira-de-Azevedo et al., 2016).
Among Lamprophiidae, the following rear-fanged genera (belonging to the tribe Atractaspidinae) feed mostly on snakes and fossorial squamates: Amblyodipsas, Brachyophis, Chilorhinophis, Hypoptophis, Macrelaps, Polemon, and Xenocalamus (32 species; Portillo et al., 2018, 2019). Given its peculiar dentition (enlarged front and back maxillary teeth), use of venom and inclusion of skinks in its diet, the “mock viper” (genus Psammodynastes, 2 species, family Lamprophiidae) also deserves special interest (Jackson and Fritts, 1996). Another poorly studied genus is Micrelaps (four species, family Lamprophiidae), which mostly feed on snakes and has enlarged venom glands (Greene, 1997).
Situating venom in its ecological, and therefore evolutionary, context may have considerable impact for toxinological research. This extends far beyond the few concepts and taxa we have discussed and reviewed here and has implications even for research in clinical toxinology. Snakebite envenoming was recently reinstated to the World Health Organization's list of Neglected Tropical Diseases (WHO, 2017), in recognition of the fact that it results in well over 100,000 deaths, and 400,000 permanent disablements worldwide annually (Gutiérrez et al., 2017). Whilst there is much important research to be done in that space concerning the improvement of antivenom products and their distribution to those most in need, the ecological perspective should not be neglected. Snakebite envenoming is one of the most impactful forms of human-animal conflict in the modern world and such conflict occurs as the result of an interaction between two organisms in an environment—in this case between a human and a snake.
As with antimicrobial resistance, the fact that humans are involved should not prevent us from considering these interactions “ecological.” Each organism employs a range of strategies in meeting the challenges presented by its environment. For humans, these strategies include technological responses such as the use of antibiotics and antivenom. These strategies are different by degree, but not in kind, from strategies employed by other organisms, which may either produce their own antimicrobial or anti-toxin molecules or sequester them from other species (much as we “sequestered” penicillin and continue to sequester horse antibodies). These philosophical points aside, rigorous investigation of the ecology of snakebite—the circumstances in which bites from venomous snakes to humans occur—is perhaps the most neglected of all the areas of research we have discussed in this paper. It is also potentially the most impactful. As snake lovers will attest, the majority of snake bites to humans are defensive, but not all are. Regardless, all are “ecological,” and thus gaining a deeper understanding of the nature of the interactions which lead to these occasionally catastrophic incidents may help us decrease their number. For snakebite envenoming, as in so many other cases, prevention is far better than cure, and prevention requires an understanding of the ecology of both venomous snakes and the humans with whom they share their environment.
In this brief article we have highlighted a number of ways in which context, and therefore ecology, is relevant to the study of snake venom and its evolution. We have traversed a considerable amount of conceptual distance, from molecular evolution to clinical toxinology. For this reason, our treatment of each area has been unfortunately abbreviated—each of these topics either has been, or should be, discussed elsewhere in more detail. Nonetheless, we feel that attempting to unite these diverse fields and phenomena within an ecological perspective is a worthwhile exercise. Though this perspective has been somewhat neglected, the ecology of venom is a research agenda which continues to gather steam (see e.g., Diz and Calvete, 2016; papers in this issue; and a forthcoming special edition of the journal Toxins). Another exciting development in ecological research is the fledgling field of “ecological genomics” (Shafer et al., 2016) – the introduction of these methods into venom research may contribute to rapidly advancing the agenda we have advocated in this piece. Our modest hope for this article is that it piques the interest of our fellow toxinologists and illustrates some of the future research directions that derive from viewing the venomous world through an ecological lens.
All authors listed have made a substantial, direct and intellectual contribution to the work, and approved it for publication.
This work was supported by MNHN.
The authors declare that the research was conducted in the absence of any commercial or financial relationships that could be construed as a potential conflict of interest.
Alencar, L. R. V., Gaiarsa, M. P., and Martins, M. (2013). The evolution of diet and microhabitat use in pseudoboine snakes. S. Am. J. Herpetol. 8, 60–66. doi: 10.2994/SAJH-D-13-00005.1
Arbuckle, K., Rodriguez de la Vega, R. C., and Casewell, N. R. (2017). Coevolution takes the sting out of it: evolutionary biology and mechanisms of toxin resistance in animals. Toxicon 140, 118–131. doi: 10.1016/j.toxicon.2017.10.026
Barlow, A., Pook, C. E., Harrison, R. A., and Wüster, W. (2009). Coevolution of diet and prey-specific venom activity supports the role of selection in snake venom evolution. Proc. Biol. Sci. 276, 2443–2449. doi: 10.1098/rspb.2009.0048
Bizerra, A., Marques, O. A. V., and Sazima, I. (2005). Reproduction and feeding of the colubrid snake Tomodon dorsatus from south-eastern Brazil. Amphib. Rept. 26, 33–38. doi: 10.1163/1568538053693350
Brown, R. L. (2014). What evolvability really is. Brit. J. Philos. Sci. 65, 549–572. doi: 10.1093/bjps/axt014
Brust, A., Sunagar, K., Undheim, E. A., Vetter, I., Yang, D. C., Casewell, N. R., et al. (2013). Differential evolution and neofunctionalization of snake venom metalloprotease domains. Mol. Cell. Proteomics 12, 651–663. doi: 10.1074/mcp.M112.023135
Campos, P. F., Andrade-Silva, D., Zelanis, A., Paes Leme, A. F., Rocha, M. M., Menezes, M. C., et al. (2016). Trends in the evolution of snake toxins underscored by an integrative omics approach to profile the venom of the colubrid Phalotris mertensi. Genome Biol. Evol. 8, 2266–2287. doi: 10.1093/gbe/evw149
Casewell, N. R., Wüster, W. W., Vonk, F. J., Harrison, R. A., and Fry, B. G. (2013). Complex cocktails: the evolutionary novelty of venoms. Trends Ecol. Evol. 28, 219–229. doi: 10.1016/j.tree.2012.10.020
Chang, D., and Duda, T. F. (2012). Extensive and continuous duplication facilitates rapid evolution and diversification of gene families. Mol. Biol. Evol. 29, 2019–2029. doi: 10.1093/molbev/mss068
da Silva, N. J., and Aird, S. D. (2001). Prey specificity, comparative lethality and compositional differences of coral snake venoms. Comp. Biochem. Physiol. C Toxicol. Pharmacol. 128, 425–456. doi: 10.1016/S1532-0456(00)00215-5
de Oliveira, L., Jared, C., da Costa Prudente, A. L., Zaher, H., and Antoniazzi, M. M. (2008). Oral glands in dipsadine “goo-eater” snakes: morphology and histochemistry of the infralabial glands in Atractus reticulatus, Dipsas indica, and Sibynomorphus mikanii. Toxicon 51, 898–913. doi: 10.1016/j.toxicon.2007.12.021
Diz, A. P., and Calvete, J. J. (2016). Ecological proteomics: is the field ripe for integrating proteomics into evolutionary ecology research? J. Proteomics 135, 1–3. doi: 10.1016/j.jprot.2016.01.020
Evans, E. R. J., Northfield, T. D., Daly, N. L., and Wilson, D. T. (2019). Venom costs and optimization in scorpions. Front. Ecol. Evol. 7:196. doi: 10.3389/fevo.2019.00196
Fraenkel, G. S. (1959). The raison d'être of secondary plant substances; these odd chemicals arose as a means of protecting plants from insects and now guide insects to food. Science 129, 1466–1470. doi: 10.1126/science.129.3361.1466
Fry, B. G., Casewell, N. R., Wüster, W., Vidal, N., Young, B., and Jackson, T. N. (2012). The structural and functional diversification of the Toxicofera reptile venom system. Toxicon 60, 434–448. doi: 10.1016/j.toxicon.2012.02.013
Fry, B. G., Roelants, K., Champagne, D. E., Scheib, H., Tyndall, J. D. A., King, G. F., et al. (2009). The toxicogenomic multiverse: convergent recruitment of proteins into animal venoms. Annu. Rev. Genom. Hum. Genet. 10, 483–511. doi: 10.1146/annurev.genom.9.081307.164356
Fry, B. G., Scheib, H., van der Weerd, L., Young, B., McNaughtan, J., Ryan Ramjan, S. F., et al. (2008). Evolution of an arsenal: structural and functional diversification of the venom system in the advanced snakes. Mol. Cell. Proteomics 7, 215–246. doi: 10.1074/mcp.M700094-MCP200
Fry, B. G., Undheim, E. A. B., Ali, S., Debono, J., Scheib, H., Ruder, T., et al. (2013). Squeezers and leaf-cutters: differential diversification and degeneration of the venom system in toxicoferan reptiles. Mol. Cell. Proteomics 12, 1881–1899. doi: 10.1074/mcp.M112.023143
Gaiarsa, M. P., Alencar, L. R. V., and Martins, M. (2013). Natural history of the Pseudoboini snakes. Pap. Avulsos Zool. 53, 261–283 doi: 10.1590/S0031-10492013001900001
Gavrilets, S., and Vose, A. (2005). Dynamic patterns of adaptive radiation. Proc. Natl. Acad. Sci. U.S.A. 102, 18040–18045. doi: 10.1073/pnas.0506330102
Godfrey-Smith, P. (2007). Conditions for evolution by natural selection. J. Philos. 104, 489–516. doi: 10.5840/jphil2007104103
Gould, S., and Vrba, E. (1982). Exaptation-a missing term in the science of form. Paleobiology 8, 4–15. doi: 10.1017/S0094837300004310
Gower, D. J., Vidal, N., Spinks, J. N., and McCarthy, C. J. (2005). First inclusion of Anomochilidae in molecular phylogenetics of the major lineages of snakes. J. Zool. Syst. Evol. Res. 43, 315–320. doi: 10.1111/j.1439-0469.2005.00315.x
Greene, H. W. (1997). Snakes: the Evolution of Mystery in Nature. Berkeley: University of California Press.
Gutiérrez, J. M., Calvete, J. J., Habib, A. G., Harrison, R. A., Williams, D. J., and Warrell, D. A. (2017). Snakebite envenoming. Nat. Rev. Dis. Primers 3:17063. doi: 10.1038/nrdp.2017.63
Guttinger, S. (2018). “A process ontology for macromolecular biology,” in Everything Flows: Towards a Processual Philosophy of Biology, eds D. J. Nicholson and J. Dupré (Oxford University Press). doi: 10.1093/oso/9780198779636.001.0001
Healy, K., Carbone, C., and Jackson, A. L. (2019). Snake venom potency and yield are associated with prey-evolution, predator metabolism and habitat structure. Ecol. Lett. 22, 527–537. doi: 10.1111/ele.13216
Holding, M. L., Drabeck, D. H., Jansa, S. A., and Gibbs, H. L. (2016). Venom resistance as a model for understanding the molecular basis of complex coevolutionary adaptations. Integr. Comp. Biol. 56, 1032–1043. doi: 10.1093/icb/icw082
Hui, C., Minoarivelo, H. O., Nuwagaba, S., and Ramanantoanina, A. (2015). “Adaptive diversification in coevolutionary systems,” in Evolutionary Biology: Biodiversification From Genotype to Phenotype, ed P. Pontarotti (Cham: Springer), 167–186.
Innan, H., and Kondrashov, F. (2010). The evolution of gene duplications: classifying and distinguishing between models. Nat. Rev. Genet. 11, 97–108. doi: 10.1038/nrg2689
Jackson, K., and Fritts, T. H. (1996). Observations of a grooved anterior fang in Psammodynastes pulverulentus: does the mock viper resemble a protoelapid? J. Herpetol. 30, 128–131. doi: 10.2307/1564727
Jackson, T. N., and Fry, B. G. (2016). A tricky trait: applying the fruits of the “function debate” in the philosophy of biology to the “venom debate” in the science of toxinology. Toxins 8:263. doi: 10.3390/toxins8090263
Jackson, T. N., Koludarov, I., Ali, S. A., Dobson, J., Zdenek, C. N., Dashevsky, D., et al. (2016). Rapid radiations and the race to redundancy: an investigation of the evolution of Australian elapid snake venoms. Toxins 8:309. doi: 10.3390/toxins8110309
Jackson, T. N. W., Young, Y., Underwood, G., McCarthy, C. J., Kochva, E., Vidal, N., et al. (2017). Endless forms most beautiful: the evolution of ophidian oral glands, including the venom system, and the use of appropriate terminology for homologous structures. Zoomorphology 136, 107–130. doi: 10.1007/s00435-016-0332-9
Junqueira-de-Azevedo, I. L., Campos, P. F., Ching, A. T., and Mackessy, S. P. (2016). Colubrid venom composition: an –omics perspective. Toxins 8:E230. doi: 10.3390/toxins8080230
Kley, N., and Brainerd, E. (1999). Feeding by mandibular raking in a snake. Nature 402, 369–370. doi: 10.1038/46460
Kley, N. J. (2001). Prey transport mechanisms in blindsnakes and the evolution of unilateral feeding systems in snakes. Amer. Zool. 41, 1321–1337. doi: 10.1093/icb/41.6.1321
Koludarov, I., Jackson, T. N. W., Pozzi, A., and Mikheyev, A. S. (2019). Family Saga: Reconstructing the Evolutionary History of a Functionally Diverse Gene Family Reveals Complexity at the Genetic Origins of Novelty. bioRxiv preprint first posted online
Laporta-Ferreira, L. I., and Salomaõ, D. G. M. (1991). Morphology, physiology and toxicology of the oral glands of a tropical cochleophagous snake, Sibynomorphus neuwiedi (Colubridae-Dipsadinae). Zool. Anz. 227, 198–208
Lema, T., Azevedo, A. C., and Lemitão, M. M. (1983). Contribuição ao conhecimento da amimenção e do modo alimentar de Serpentes do Brasil. Comun. Mus. Ci. PUCRS 26, 41–121.
Lillywhite, H. W. (2014). How Snakes Work. Structure, Function, and Behavior of the World's Snakes. New York, NY: Oxford University Press.
Lumsden, N. G., Banerjee, Y., Kini, R. M., Kuruppu, S., and Hodgson, W. C. (2007). Isolation and characterization of rufoxin, a novel protein exhibiting neurotoxicity from venom of the psammophiine, Rhamphiophis oxyrhynchus (Rufous beaked snake). Neuropharmacology 52, 1065–1070. doi: 10.1016/j.neuropharm.2006.11.002
Marques, O. A. V., Sawaya, R. J., Stender-Oliveira, F., and Franca, F. G. R. (2006). Ecology of the colubrid snake Pseudablabes agassizii in Southeastern South America. Herpetol. J. 16, 37–45.
Marques, R., Mebert, K., Fonseca, É., Rödder, D., Solé, M., and Tinôco, M. S. (2016). Composition and natural history notes of the coastal snake assemblage from Northern Bahia, Brazil. ZooKeys 611, 93–142. doi: 10.3897/zookeys.611.9529
Martins, A., Passos, P., and Pinto, R. (2018). Unveiling diversity under the skin: comparative morphology study of the cephalic glands in threadsnakes (Serpentes: Leptotyphlopidae: Epictinae). Zoomorphology 137, 433–443. doi: 10.1007/s00435-018-0409-8
Miralles, A., Marin, J., Markus, D., Herrel, A., Hedges, S. B., and Vidal, N. (2018). Molecular evidence for the paraphyly of Scolecophidia and its evolutionary implications. J. Evol. Biol. 31, 1782–1793. doi: 10.1111/jeb.13373.
Modahl, C. M., Mrinalini Frietze, S., and Mackessy, S.P. (2018). Adaptive evolution of distinct prey-specific toxin genes in rear-fanged snake venom. Proc. R. Soc. B. 285:20181003. doi: 10.1098/rspb.2018.1003
Morgenstern, D., and King, G. F. (2013). The venom optimization hypothesis revisited. Toxicon 63, 120–128. doi: 10.1016/j.toxicon.2012.11.022
Murphy, J. C., and Voris, H. K. (2014). A checklist and key to the homalopsid snakes (Reptilia, Squamata, Serpentes), with the description of new genera. Fieldiana Life Earth Sci. 8, 1–43. doi: 10.3158/2158-5520-14.8.1
OmPraba, G., Chapeaurouge, A., Doley, R., Devi, K. R., Padmanaban, P., Venkatraman, C., et al. (2010). Identification of a novel family of snake venom proteins veficolins from Cerberus rynchops using a venom gland transcriptomics and proteomics approach. J. Proteome Res. 9, 1882–1893. doi: 10.1021/pr901044x
O'Shea, M. (2018). The Book of Snakes. A Life-Size Guide to Six Hundred Species From Around the World. London: Ivy Press.
Payne, J. L., and Wagner, A. (2019). The causes of evolvability and their evolution. Nat. Rev. Genet. 20, 24–38. doi: 10.1038/s41576-018-0069-z
Portillo, F., Branch, W. R., Conradie, W., Rödel, M. O., Penner, J., Barej, M. F., et al. (2018). Phylogeny and biogeography of the African burrowing snake subfamily Aparallactinae (Squamata: Lamprophiidae). Mol. Phylogenet. Evol. 127, 288–303. doi: 10.1016/j.ympev.2018.03.019
Portillo, F., Stanley, E. L., Branch, W. R., Conradie, W., Rödel, M. O., Penner, J., et al. (2019). Evolutionary history of burrowing asps (Lamprophiidae: Atractaspidinae) with emphasis on fang evolution and prey selection. PLoS One 14:e0214889. doi: 10.1371/journal.pone.0214889
Pough, F. H., Andrews, R. M., Crump, M. L., Savitzky, A. H., Wells, K. D., and Brandley, M. C. (2016). Herpetology, 4th Edn. Sunderland, MA: Sinauer.
Reams, A. B., and Roth, J. R. (2015). Mechanisms of gene duplication and amplification. Cold Spring Harb. Perspect. Biol. 7:a016592. doi: 10.1101/cshperspect.a016592
Rossman, D. A., and Myer, P. A. (1971). Behavioral and morphological adaptations for snail extraction in the North American brown snakes (genus Storeria). J. Herpetol. 24, 434–438. doi: 10.2307/1565069
Ruane, S., Raxworthy, C. J., Lemmon, A. R., Lemmon, E. M., and Burbrink, F. T. (2015). Comparing species tree estimation with large anchored phylogenomic and small Sanger-sequenced molecular datasets: an empirical study on Malagasy pseudoxyrhophiine snakes. BMC Evol. Biol. 15:221. doi: 10.1186/s12862-015-0503-1
Salomaõ, M., and Laporta-Ferreira, I. L. (1994). The role of secretions from the supralabial, infralabial, and duvernoy's glands of the slug-eating snake Sibynomorphus mikani (Colubridae: Dipsadinae) in the immobilization of molluscan prey. J. Herpetol. 28, 369–371. doi: 10.2307/1564537
Sánchez, M. N., Teibler, G. P., Sciani, J. M., Casafús, M. G., Maruñak, S. L., Mackessy, S. P., et al. (2019). Unveiling toxicological aspects of venom from the Aesculapian false coral snake Erythrolamprus aesculapii. Toxicon 164, 71–81. doi: 10.1016/j.toxicon.2019.04.007
Shafer, A. B. A., Northrup, J. M., Wikelski, M., Wittemyer, G., and Wolf, J. B. W. (2016). Forecasting ecological genomics: high-tech animal instrumentation meets high-throughput sequencing. PLoS Biol 14:e1002350. doi: 10.1371/journal.pbio.1002350
Smiley-Walters, S. A., Farrell, T. M., and Gibbs, H. L. (2019). High levels of functional divergence in toxicity towards prey among the venoms of individual pigmy rattlesnakes. Biol. Lett.15:20180876. doi: 10.1098/rsbl.2018.0876
Sterelny, K. (2007). “What is Evolvability?,” in Philosophy of Biology, eds M. Matthen, and C. Stephens (Amsterdam: Elsevier, 177–92.
Streicher, J. W., and Ruane, S. (2018). “Phylogenomics of snakes,” in eLS (Chichester: John Wiley & Sons Ltd.), 1–8. doi: 10.1002/9780470015902.a0027476
Sunagar, K., Jackson, T. N. W., Undheim, E. A. B., Ali, S. A., Antunes, A., and Fry, B. G. (2013). Three-fingered RAVERs: rapid accumulation of variations in exposed residues of snake venom toxins. Toxins (Basel) 5, 2172–2208. doi: 10.3390/toxins5112172
Uetz, P., Freed, P., and Hošek, J. (2019). The Reptile Database. http://www.reptile-database.org (accessed August 1, 2019).
Vidal, N. (2002). Colubroid systematics: evidence for an early appearance of the venom apparatus followed by extensive evolutionary tinkering. J. Toxicol. Toxin Rev. 21, 27–47. doi: 10.1081/TXR-120004740
Wagner, A. (2012). The role of robustness in phenotypic adaptation and innovation. Proc. R. Soc. B. 279, 1249–1258. doi: 10.1098/rspb.2011.2293
Wallach, V., Williams, K. L., and Boundy, J. (2014). Snakes of the World. A Catalogue of Living and Extinct Species. New York, NY: CRC Press.
WHO (2017). Neglected Tropical Diseases. Available online at: https://www.who.int/neglected_diseases/diseases/en/ (accessed August 1, 2019).
WHO (2018). Antimicrobial Resistance. Available online at: https://www.who.int/en/news-room/fact-sheets/detail/antimicrobial-resistance (accessed August 1, 2019).
Yoder, J. B., Clancey, E., Des Roches, S., Eastman, J. M., Gentry, L., Godsoe, W., et al. (2010). Ecological opportunity and the origin of adaptive radiations. J. Evol. Biol. 23, 1581–1596. doi: 10.1111/j.1420-9101.2010.02029.x
Zaher, H., de Oliveira, L., Grazziotin, F. G., Campagner, M., Jared, C., Antoniazzi, M. M., et al. (2014). Consuming viscous prey: a novel protein-secreting delivery system in neotropical snail-eatingsnakes. BMC Evol. Biol. 14:58. doi: 10.1186/1471-2148-14-58
Keywords: reptile, snake, evolution, ecology, toxin
Citation: Jackson TNW, Jouanne H and Vidal N (2019) Snake Venom in Context: Neglected Clades and Concepts. Front. Ecol. Evol. 7:332. doi: 10.3389/fevo.2019.00332
Received: 30 April 2019; Accepted: 21 August 2019;
Published: 06 September 2019.
Edited by:
Maria Vittoria Modica, Stazione Zoologica Anton Dohrn, ItalyReviewed by:
Juan J. Calvete, Spanish National Research Council (CSIC), SpainCopyright © 2019 Jackson, Jouanne and Vidal. This is an open-access article distributed under the terms of the Creative Commons Attribution License (CC BY). The use, distribution or reproduction in other forums is permitted, provided the original author(s) and the copyright owner(s) are credited and that the original publication in this journal is cited, in accordance with accepted academic practice. No use, distribution or reproduction is permitted which does not comply with these terms.
*Correspondence: Timothy N. W. Jackson, dGltb3RoeS5qYWNrc29uQHVuaW1lbGIuZWR1LmF1; Nicolas Vidal, bmljb2xhcy52aWRhbEBtbmhuLmZy
†These authors have contributed equally to this work
Disclaimer: All claims expressed in this article are solely those of the authors and do not necessarily represent those of their affiliated organizations, or those of the publisher, the editors and the reviewers. Any product that may be evaluated in this article or claim that may be made by its manufacturer is not guaranteed or endorsed by the publisher.
Research integrity at Frontiers
Learn more about the work of our research integrity team to safeguard the quality of each article we publish.