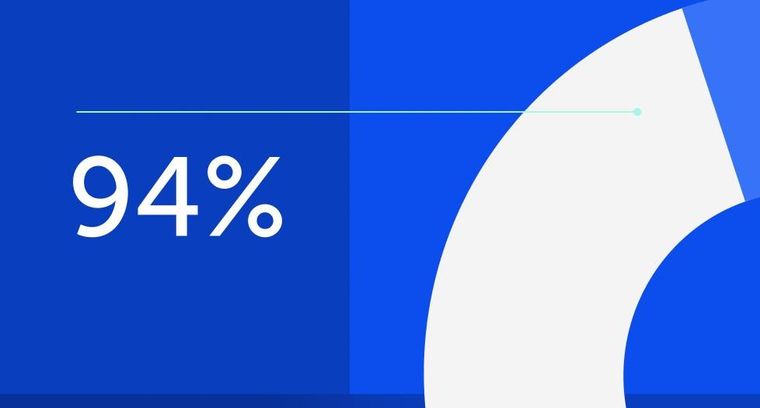
94% of researchers rate our articles as excellent or good
Learn more about the work of our research integrity team to safeguard the quality of each article we publish.
Find out more
ORIGINAL RESEARCH article
Front. Ecol. Evol., 28 August 2019
Sec. Population, Community, and Ecosystem Dynamics
Volume 7 - 2019 | https://doi.org/10.3389/fevo.2019.00314
This article is part of the Research TopicArthropod Interactions and Responses to Disturbance in a Changing WorldView all 20 articles
The effects of climate change on solitary bee species, the most diverse and abundant group of wild pollinators, remain poorly understood, limiting our ability to forecast consequences for bee-plant interactions and pollination services. Life history traits, such as overwintering life stage, sex, and body size may influence solitary bee responses to climate change by mediating the effects of temperature on physiological processes spanning fall, winter, and spring. Yet, most studies assessing the effects of temperature on solitary bees have focused on managed species and have isolated the effects of winter temperature. Here, we reared male and female individuals representing eight cavity-nesting solitary bee species that overwinter either as adults (i.e., Osmia spp.) or prepupae (i.e., Megachile spp.). Eight rearing treatments were used, in which we manipulated fall and spring temperature, fall duration, and the timing of spring onset. We measured pre-emergence mortality, pre-emergence weight loss, emergence timing, and post-emergence lifespan. We found that Osmia spp. responded primarily to the timing of spring onset, whereas Megachile spp. responded primarily to spring temperature. Early-spring onset increased both pre-emergence mortality and pre-emergence weight loss and reduced post-emergence lifespan in Osmia spp. In addition, treatments caused unequal shifts in the timing of emergence between male and female Osmia spp. By contrast, warmer spring temperature decreased weight loss, and increased lifespan in Megachile spp. These findings suggest that Osmia spp. may be more vulnerable to negative fitness consequences of climate change compared to Megachile spp., and that climate change may have implications for population-level sex-ratios and mating success in species of Osmia. This work helps build a mechanistic understanding of how life histories may mediate solitary bee responses to climate change, and how these responses may impact solitary bee fitness and plant-bee interactions.
Temperature strongly influences most biological and ecological processes in plants and insects (Deutsch et al., 2008; Lambers et al., 2008), including processes, such as survival and phenology, that mediate plant-insect interactions. Thus, there is concern that climate change will affect plant-insect interactions (Tylianakis et al., 2008), and in turn, disrupt essential ecosystem services, like pollination (Hegland et al., 2009; Burkle et al., 2013; Vanbergen et al., 2013; Potts et al., 2016). Central to forecasting the consequences of climate-change for plant-pollinator interactions is a better understanding of the mechanisms, and underlying species traits, that mediate response patterns among plant and pollinator species (Cariveau and Winfree, 2015; Rafferty, 2017). While linking plant traits, such as flowering season and frost tolerance, with plant responses to climate change (Fitter and Fitter, 2002; Sherry et al., 2007; Cook et al., 2012; Gezon et al., 2016; Pardee et al., 2018) has improved our understanding of the consequences of climate change for plant species and plant-pollinator interactions, similar progress with pollinator species is lacking (Ogilvie and Forrest, 2017). In particular, few studies have examined the role of species traits in mediating solitary bee responses to climate change, the most diverse and abundant group of wild pollinators (but see Fründ et al., 2013).
Understanding how solitary bee species are responding to climate change is difficult in part because, unlike plants, there is little long-term data on phenology and species abundances to link with changes in climate (Ogilvie and Forrest, 2017). While studies incorporating temperature manipulations in the field impart realism (e.g., Forrest and Thomson, 2011; CaraDonna et al., 2018), controlled laboratory studies are better equipped to elucidate response mechanisms. However, solitary bee responses to temperature have only been investigated in a limited number of managed species (e.g., Bosch and Kemp, 2003, 2004; Sgolastra et al., 2010, 2011; O'Neill et al., 2014; Schenk et al., 2018a), and have primarily isolated responses to a single component of climate change (e.g., extended fall duration, winter temperature, or spring temperature, Bosch and Kemp, 2000, 2003; Sgolastra et al., 2011; Fründ et al., 2013; O'Neill et al., 2014; Schenk et al., 2018a; but see Bosch and Kemp, 2004). While such studies have provided an important foundation for understanding how temperature influences biological processes in solitary bees, bee life cycles can span several seasons or years (Forrest et al., 2019), and climate change is occurring year-round (Sparks and Menzel, 2002; Alexander et al., 2006; Burrows et al., 2011). In addition, the paucity of studies on non-managed species hinders our ability to generalize response patterns across species based on shared traits. Therefore, to gain a more accurate picture of how climate change is impacting solitary bees, and to avoid misleading conclusions based on temperature manipulations isolated within a single season, assessing responses to temperature across multiple seasons and life stages is required (Kingsolver et al., 2011).
For solitary bees, responses to climate change will likely be driven in part by the influence of temperature on biological processes occurring prior to emergence. For example, warmer temperatures can accelerate development (Radmacher and Strohm, 2010; O'Neill et al., 2014), reduce stored-energy reserves (Bosch and Kemp, 2003, 2004; O'Neill et al., 2011, 2014; Sgolastra et al., 2011; Schenk et al., 2018a), increase mortality prior to emergence (Bosch and Kemp, 2003; O'Neill et al., 2011), advance emergence (Forrest and Thomson, 2011), and reduce post-emergence lifespan (Bosch and Kemp, 2003, 2004; Bosch et al., 2010). In addition, elevated seasonal temperatures and shorter winters associated with climate change (Sparks and Menzel, 2002; Menzel et al., 2006) may shorten periods of cold exposure, which can disrupt diapause development (Hodek and Hodkova, 1988; Sgolastra et al., 2010) and cause increased pre-emergence energy consumption, increased winter mortality, and delayed emergence (Bosch and Kemp, 2003, 2004; Sgolastra et al., 2010). In turn, such effects on bee species could alter seasonal activity periods in bees (Memmott et al., 2007) and affect reproductive output (Bosch and Kemp, 2004; Bosch and Vicens, 2006), which could alter the composition and abundance of bee species in a community and influence bee-plant interactions.
Understanding solitary bee responses to climate change will ultimately depend on interplay among multiple biological mechanisms acting during different developmental stages. In insects and other organisms, the outcomes of this interplay can be complex (Post et al., 2008; Kingsolver et al., 2011), even counterintuitive (Cook et al., 2012), with responses depending on physiological processes associated with life-history traits (e.g., Diamond et al., 2011; Buckley and Kingsolver, 2012; Cook et al., 2012; Sgolastra et al., 2016; Schenk et al., 2018a). First, solitary bee species in temperate zones overwinter either as adults or as prepupae (Stephen et al., 1969). In both cases, bees overwinter on a fixed energetic budget and do not consume food until after they emerge. Overwintering life histories determine the seasonal timing of transitions between life stages, and may influence how physiological processes are impacted by temperature and season length. For example, adult-wintering species may have a higher metabolic demand during fall while in the nest (i.e., prior to overwintering) compared to prepupal-wintering species that overwinter in an immature life stage (Waters and Harrison, 2012). If so, fitness in adult-wintering species may be more sensitive to warmer, extended falls compared to prepupal-wintering species (Bosch et al., 2010). Further, cold exposure during winter can influence post-emergence fitness in adult-wintering species by affecting adult diapause development and termination (Sgolastra et al., 2010). By contrast, prepupal-wintering species develop into adults during spring and early summer and emerge in mid-summer. Therefore, prepupal-wintering species may be more sensitive to spring temperatures and less sensitive to fall and winter temperatures (Kemp and Bosch, 2000, 2001; Kemp et al., 2004; O'Neill et al., 2011). Second, body size and sex may influence the effects of temperature on pre-emergence energy consumption in solitary bees due to differences in the ratio of lipids to body mass entering winter (O'Neill et al., 2014). Size differences among species, and between male and female bees within species, may therefore mediate responses to temperature, particularly in adult-wintering solitary bee species during fall and winter.
Here, we used a combination of field and laboratory techniques that allowed us to investigate the influence of temperature and season length on mortality, stored-energy consumption, and the timing of emergence across multiple species of wild, cavity-nesting solitary bees that overwinter either as adults or prepupae. We investigated (1) how seasonal temperature interacts with the duration and timing of fall, winter, and spring to influence biological responses in cavity-nesting solitary bees, and (2) how overwintering life histories, body size, and sex mediate these responses. We reared eight cavity-nesting solitary bee species that overwinter either as adults or prepupae within one of eight treatment combinations (Figure 1). Treatments manipulated fall and spring temperature, the duration of fall, and the start of spring. We quantified the number of bees that died prior to emergence (i.e., pre-emergence mortality), weight loss during treatments (i.e., pre-emergence weight loss), the number of days it took bees to emerge after the start of the spring treatment (i.e., days to emergence), and lifespan after emergence without feeding (i.e., post-emergence lifespan).
Figure 1. Description and timeline of experimental treatments. Treatments are 4 factorial combinations of fall duration (“short” or “long”) and date of spring onset (“early” or “late”), nested within 2 temperature regimes that manipulated fall and spring temperature while holding winter temperature constant (i.e., “cool” regime: 6/4/18°C or “warm” regime: 9/4/21°C, fall/winter/spring). Fall and spring treatment combinations resulted in three winter durations (60, 90, 120 days), with the length of winter, as well as the start and end date of winter, determined by fall and spring treatment combinations.
Based on differences in overwintering life histories, as well as physiological responses to temperature observed in managed solitary bee species and other insects, we tested the following hypotheses. For adult-wintering species, we expected that elevated temperature, and extended fall duration would increase weight loss and pre-emergence mortality, and decrease both the number of days to emergence and post-emergence lifespan (Bosch and Kemp, 2004; Figure 2). We expected that these patterns for adult-wintering species would depend on sex and body size (Figure 2). For prepupal-wintering species, we hypothesized that elevated spring temperature would reduce the number of days to emergence, but we did not expect temperature during fall or the duration of fall to influence the timing of emergence (Kemp and Bosch, 2000; O'Neill et al., 2011; Figure 2). As a result of decreased days to emergence, and, in turn, less time spent in non-feeding life-stages, we predicted that elevated temperature would reduce pre-emergence weight loss and increase post-emergence lifespan in prepupal-wintering species (O'Neill et al., 2011; Figure 2). Because the prepupal life stage may be less sensitive to temperature (Pitts-Singer and James, 2009), we did not expect pre-emergence mortality in prepupal-wintering species to vary in this study, and we did not anticipate body size to mediate the effects of temperature. Finally, due to the potential influence of cold exposure during winter on adult diapause termination, we expected a shorter winter duration to increase both the number of days to emergence and pre-emergence weight loss in adult-wintering species, but not prepupal-wintering species (Bosch and Kemp, 2003; Sgolastra et al., 2010; but see Forrest et al., 2019; Figure 2).
Figure 2. Conceptual figure showing response variables (boxes on right side of each panel) and independent variables (boxes on left side of each panel) included in our hypotheses for Osmia spp. (i.e., adult-wintering species) and Megachile spp. (i.e., prepupal-wintering species). Solid directional arrows linking treatments and responses signify expectations of treatment effects. Arrows between explanatory variables show expected interactions, with arrow type (i.e., directional or double arrow) denoting the expected direction of the interaction (e.g., for adult-wintering bees, body size, and sex are expected to influence the effect of temperature and fall duration, but not vice versa, whereas fall duration, and temperature are expected to influence each other). Dashed lines represent expected correlations among response variables that we assessed with Piecewise SEM.
We studied cavity-nesting solitary bees from the genus Osmia (mason bees) and the genus Megachile (leafcutter bees) (Hymenoptera: Megachilidae). In total, we studied eight species with at least 8 emerged male and 8 emerged female individuals per species. Six of the species overwinter as adults: Osmia californica Cresson, Osmia coloradensis Cresson, Osmia lignaria Say, Osmia montana Cresson, Osmia tersula Cockerell, and Osmia vallicola Cockerell, and two species overwinter as prepupae: Megachile lapponica Thomson and Megachile relativa Cresson (see Table S1 for sample sizes). Species included in the study range in size from ~6–20 mm long and are widespread and abundant pollinators of both wild plants and crops, making this a relevant suite of species to address the objectives of this study. In addition, as cavity-nesting species that nest above ground in existing cavities, these species are expected to be more susceptible to changing seasonal temperatures compared to species that nest below ground, due to the lack of insolating effects that nesting below the soil surface provides (Forrest and Thomson, 2011; Sgolastra et al., 2011).
The solitary bees examined in this study were collected by placing trap-nests at six locations near Bozeman, Montana starting in March and April 2013 (see Table S2 for descriptions of collection sites). Environmental conditions, not local genotypes, predicted adult emergence dates in several montane cavity-nesting bee species (Forrest and Thomson, 2011). Thus, we assumed that individuals of the same species collected at different locations in our study would not have pre-adapted sensitivities to local temperatures. After retrieving nests during September of 2013, we determined overwintering life-stage (i.e., adult or prepupae) by inspecting cocoons with a dissecting microscope. We assumed that each individual had developed to their overwintering life stage by this time, and that provisions had been consumed (Stephen and Torchio, 1961; Torchio, 1989). To avoid the disruption caused by opening cocoons, we used nesting materials, and cocoon sizes to approximate species; species identities were later confirmed after emergence.
To investigate how seasonal temperatures, as well as the duration and timing of seasons influenced responses in solitary bees, we reared individual bees inside gelatin capsules using 4.5 × 4.5 meter Conviron temperature-controlled chambers. Temperature treatments consisted of two regimes that manipulated fall and spring temperature, while exposing bees to the same winter temperature (“cool” regime: 6/4/18°C, “warm” regime: 9/4/21°C, fall/winter/spring; Figure 1). Temperature manipulations were based on seasonal average temperatures near Bozeman, MT (NOAA, https://www.ncdc.noaa.gov/) and were designed to simulate a projected 3°C increase in seasonal average temperatures caused by a doubling of atmospheric CO2 (IPCC, 2014). All treatments received the same winter temperature to isolate the effects of fall and spring temperature on physiological processes occurring during non-wintering periods of the solitary bee life cycle. Within each temperature regime, we used four factorial treatment combinations consisting of either a “short” or “long” fall duration and an “early” or “late” date of spring onset (Figure 1). We manipulated the duration of fall by moving bees between chambers set to fall and winter temperatures after 30 (short-fall duration) or 60 days (long-fall duration). Spring onset was manipulated by moving bees between chambers set to winter and spring temperatures on either 26 March (early-spring onset) or 25 April (late-spring onset). Fall duration and spring onset treatment combinations resulted in winter durations of either 60, 90, or 120 days. The start and end dates for each 90 day winter period depended on fall and spring treatment combinations, allowing an assessment of how fall duration and the date of spring onset interacted with winter duration. Historically (1971–2000), mean air temperatures near Bozeman, MT fall below 0°C from December through February (NOAA, https://www.ncdc.noaa.gov/). Using these data to roughly approximate the start and end dates of winter, we manipulated short-fall duration treatments to end during December and long-fall duration treatments to extend into January. For the spring treatment, we manipulated early-spring onset to begin during March (i.e., the first month of mean temperature above 0°C) and late-spring onset treatments to extend into April. We quantified bee weight prior to the start of the study by weighing each cocoon inside a labeled capsule (i.e., cocoon containing the bee plus all nesting materials and feces) to the nearest 0.1 mg using a Mettler Toledo NewClassic MF electronic balance. We distributed individuals from the same mother (i.e., cocoons from the same nesting tube), species, and nesting location equally across treatments. In total, we assigned 1,326 individual cocoons to treatments.
After moving bees into spring treatments, emergence was monitored twice each day (0800 and 1800) and the date on which bees emerged was recorded. We then calculated “days to emergence” as the number of days it took for a bee to emerge following the start of the spring treatment (early- vs. late-spring onset treatments differed by 30 days, but days to emergence were relative to the start of the spring treatment for all bees). We defined emergence as cocoons that had been torn open by a bee. Bees that did not emerge were inspected for the presence of parasitoids. Non-parasitized bees that appeared to be fully developed were identified to species, and used to analyze treatment effects on pre-emergence mortality. After emergence, we re-weighed the labeled capsules containing the emerged bee plus the cocoon, nesting materials, and feces. Post-emergence weights were recorded no more than 14 h after emergence and were used to calculate pre-emergence weight loss occurring during treatments. In some Osmia individuals, pre-emergence weight loss was negative, indicating that weight was gained between the start of treatments and emergence. Because Osmia individuals had reached a non-feeding life stage at the start of treatments, we speculate that moisture, either absorbed through the cuticle of the overwintering bees (May, 1972) or by the gelatin capsule encasing the bee cocoons, may have accounted for the observed weight gain. We considered any weight gain to be negligible and did not adjust or remove any data from our analysis of pre-emergence weight loss. Emerged bees were placed (still inside their capsule) in ambient indoor temperature (~21°C) without feeding, where they were monitored once per day until death to determine post-emergence lifespan without feeding (Bosch and Kemp, 2000). We determined death by probing the intertegular space of the thorax (i.e., between the base of the wings) with forceps; if no movement was observed for three seconds, we assumed the bee was dead. Deceased bees were identified to species and sex was determined (McGavin, 1994; Michener, 2007; Sheffield et al., 2011; Rightmyer et al., 2013). Intertegular distance (i.e., body size) was quantified by measuring the distance (i.e., ocular units) between tegulae (i.e., base of the wings) using a calibrated reticle ruler and measurements were converting to millimeters (Cane, 1987; Cariveau et al., 2016). Weight loss was divided by intertegular distance and analyzed as the proportion of weight loss (mg) per unit body size (mm).
Statistical analyses were conducted using R statistical software version 3.4.3 (R Core Team, 2013). In total, we included eight cavity-nesting solitary bee species in the analyses. These species were represented by at least 8 emerged male and 8 emerged female individuals (i.e., 458 total bees; see Table S2 for sample sizes in each treatment broken down by species and sex). Due to small sample sizes for some species, we could not analyze all response variables individually for each species. With the exception of pre-emergence mortality, and winter emergence (see below), we grouped species together by genus (i.e., Osmia spp. and Megachile spp.) and analyzed responses across species at the genus level. We investigated responses to fall and spring temperature, the duration of fall, the date of spring onset, and the duration of winter by analyzing four response variables individually for Osmia spp. and Megachile spp.: pre-emergence mortality, pre-emergence weight loss, days to emergence, and post-emergence lifespan. Days to emergence, post-emergence lifespan, and pre-emergence weight loss were analyzed using linear mixed-effects models (“nlme” package; Pinheiro et al., 2013). To improve normality, emergence was log-transformed in models fitted to Osmia spp. All linear mixed-effects models included a random intercept for “maternal identity” (i.e., bees from the same mother were contained in a single tube) nested within “collection location” (i.e., the field location of the trap-nest from which bees were collected). When necessary to achieve homogeneity of variances, we used the “varIdent” variance structure (Pinheiro et al., 2013). Only O. coloradensis and O. tersula had pre-emergence mortality numbers high enough to analyze (see Results). However, due to small sample sizes for O. tersula (Table S2), we combined pre-emergence mortality data for O. tersula with O. coloradensis, and analyzed them together. Only non-emerged bees that had developed into mature adults and had not been parasitized were included in the analysis of pre-emergence mortality. Pre-emergence mortality was analyzed using a generalized linear model with a “logit” link (R Core Team, 2013). In addition, a considerable number of O. lignaria emerged while still in the winter treatment. We assumed that emerging prior to the onset of spring would likely result in mortality, and were interested in whether treatments influenced this response. Thus, we analyzed the probability of O. lignaria emergence during winter using generalized linear models with a “logit” link (R Core Team, 2013).
All models included temperature (i.e., cool or warm), fall duration (i.e., short or long), spring onset (i.e., early or late), and sex as categorical independent variables. Given that body size could both influence the response to, and result from our treatments, we first fit the latter. We found treatment effects on body size for Megachile spp. but not Osmia spp. Therefore, we only included body size as a covariate in Osmia models. In each model, we included temperature × fall duration, temperature × spring onset, and fall duration × spring onset interactions to test for interactive effects of our treatment combinations. Interactions of sex and body size with each independent variable (i.e., temperature, fall, and spring) were included to assess, sex-, and size-specific responses to each treatment. A temperature × fall duration × spring onset three-way interaction was included to assess whether the mediating effects of winter duration on fall duration and spring onset depended on temperature. However, this interaction was not significant (i.e., α ≤ 0.05) in any models and we do not discuss it further. To determine the influence of each treatment and their interactions on each response variable, we used separate ANOVAs (R Core Team, 2013). We then evaluated our hypotheses with post-hoc tests using the “lsmeans” package (Lenth and Hervac, 2015), which tested for differences in means among pairwise comparisons between treatment levels (averaged over the other treatments) and generated an estimate of effect size. A “false discovery rate” p-value adjustment (Benjamini and Hochberg, 1995) was used when comparing means across multiple combinations of independent variables or covariates. Figures representing the model estimates (i.e., least square means) were produced with the ggplot2 package (Wickham, 2009).
To disentangle the direct and indirect effects of treatments on the response variables tested in this study (not including winter mortality), we fit Structural Equation Models (SEMs) separately for Osmia spp. and Meagachile spp. using the “PiecewiseSEM” package (Lefcheck, 2016). This approach allowed us to produce mechanistic models representing our a priori hypotheses for Osmia spp. and Meagachile spp. (Figure 2). The categorical independent variables temperature, fall duration, spring onset, and sex, were included in each PiecewiseSEM model fitted to days to emergence, pre-emergence weight loss, and post-emergence lifespan. As in each mixed-effects model, body size was only included as an independent variable in the Osmia model. We included pre-emergence weight loss as an independent variable in the days to emergence and post-emergence lifespan models fitted to the Osmia data. In the Megachile models, we included days to emergence as an independent variable in the pre-emergence weight loss and post-emergence lifespan models. All models fitted within the PiecewiseSEM framework used the “lme” function in the “nlme” package (Pinheiro et al., 2013) and included a random intercept for maternal identity nested within collection location. Emergence and lifespan were log-transformed to improve normality. To facilitate comparisons among each link, coefficients were range standardized (Grace and Bollen, 2005). That is, each effect is reported as the percent of the range of values for a given response variable (when a variable is categorical), or as the percent change per one unit increase in a continuous explanatory variable. To highlight potentially distinct mechanistic pathways operating between Osmia spp. and Megachile spp., we only report significant links (i.e., α ≤ 0.05) and focus on indirect treatment effects to supplement the results of the mixed effects analyses.
Pre-emergence mortality was low (< 6%) in O. lignaria, O. californica, O. montana, O. vallicola, M. lapponica, and M. relativa, and higher in O. coloradensis (26%) and O. tersula (75%). Osmia coloradensis and O. tersula were more likely to survive and emerge when spring onset was late compared to early (z = 3.01, p = 0.003; (Table 1 and Figure 3, see Table S3 for ANOVA table showing full results for pre-emergence mortality). The probability of pre-emergence mortality decreased as body size increased (Table 1), but body size did not interact with the spring onset treatment to affect pre-emergence mortality (Table S3). Sex did not affect the probability of pre-emergence mortality (Table S3).
Table 1. ANOVA tables of the generalized linear models fitted separately to pre-emergence mortality and the probability of winter emergence (i.e., emerging prior to the initiation of spring treatments).
Figure 3. The probabilities of pre-emergence mortality ± SE for O. coloradensis and O. tersula (top panel) and of winter emergence ± SE for O. lignaria (bottom panel). Probabilities ± SE shown for all treatment combinations separated by sex.
Osmia lignaria winter emergence (i.e., emergence prior to the start of the spring treatment) was influenced by the timing of spring onset, fall duration, and temperature, and differed between male and female bees (Table 1, see Table S3 for ANOVA table showing full results for winter emergence). When spring onset was late and fall duration was long, males were more likely to emerge during winter compared to females (z = 2.97, p < 0.001; Figure 3). In addition, when spring onset was late and fall duration was long, male bees in the warm temperature treatment were more likely to emerge during winter compared to male bees in the cool treatment (z = 2.23, p = 0.026; Figure 3). Body size did not influence the likelihood of O. lignaria emergence during winter (Table S3).
In Osmia spp. (i.e., adult-wintering species), males emerged in 2.3 ± 0.4 fewer days relative to the start of the spring treatment compared to females (t287 = 7.82, p < 0.001; Figure 4A and Table 2, see Table S4 for ANOVA tables showing full results for all response variables). The timing of spring onset influenced mean days to emergence in both male and female bees. When averaged across sex, bees in the early-spring onset treatment emerged in 1.4 ± 0.2 more days relative to the start of the spring treatment compared to the late-spring onset treatment (t287 = 6.82, p < 0.001; Figure 4A and Table 2). For females, the effect size of the spring onset treatment depended on fall duration (Table 2). On average, females emerged in the fewest days in the short-fall duration treatment when spring onset was late (i.e., 120 days winter), with the largest difference in days to emergence (3.6 ± 1.3 fewer days) occurring between the long-fall duration + early-spring onset treatment combination (i.e., 60 days of winter; t287 = 3.67, p = 0.001; Figure 4A).
Figure 4. Least square mean ± SE showing (A) Osmia spp. days to emergence, (B) Osmia spp. pre-emergence weight loss, (C) Osmia spp. post-emergence lifespan, (D) Megachile spp. days to emergence, (E) Megachile spp. pre-emergence weight loss, and (F) Megachile spp. post-emergence lifespan. Least square means ± SE shown for all treatment combinations separated by sex. Note negative weight loss values (i.e., weight gain) are likely due to moisture absorbed by the bee or the nesting materials during treatments.
Table 2. ANOVA tables of the linear mixed-effects models fitted separately to Osmia spp. (i.e., adult-wintering species) and Megachile spp. (i.e., prepupal-wintering species).
Neither the temperature treatment nor body size influenced days to emergence in Osmia spp. (Table S4).
Male Megachile emerged in 3.2 ± 0.5 fewer days relative to the start of the spring treatment compared to females (t38 = 6.50, p < 0.001; Figure 4D and Table 2, see Table S4 for ANOVA tables showing full results for all response variables). In contrast to Osmia spp., the temperature treatment had a strong effect on mean days to emergence in Megachile spp. (i.e., prepupal-wintering species; Table 2), causing bees in the warm treatment to emerge in 27.2 ± 0.4 fewer days on average compared to the cool treatment (t38 = 60.42, p < 0.001; Figure 4D). In addition, the spring onset treatment affected mean days to emergence in Megachile spp. (Table 2), but this effect was smaller compared to the temperature effect. On average, the late-spring onset treatment caused bees to emerge in 1.7 ± 0.5 fewer days relative to the start of the spring treatment compared to the early-spring onset treatment (t38 = 3.73, p = 0.001; Figure 4D).
The fall duration treatment did not affect days to emergence in Megachile spp. (Table S4).
In Osmia spp., mean pre-emergence weight loss per mm of intertegular distance was greater in females compared to males (0.9 ± 0.3 mg/mm greater loss, t252 = 3.06, p = 0.003 Figure 4B and Table 2). When averaged across sex, the spring onset treatment affected mean pre-emergence weight loss (Table 2), with bees in the early-spring onset treatment losing 1.4 ± 0.2 mg/mm more weight on average compared to the late spring treatment (t252 = 6.18, p < 0.001; Figure 4B). The temperature treatment also affected mean pre-emergence weight loss, but only in females (Table S4). On average, female bees lost 1.3 ± 0.4 mg/mm more weight in the warm temperature treatment compared to the cool treatment (t252 = 3.16, p = 0.004; Figure 4B), whereas the effect of the temperature treatment on mean pre-emergence weight loss in males was not significant (t252 = 0.90, p = 0.453; Figure 4B). In addition, body size influenced mean pre-emergence weight loss (Table 2). Pre-emergence weight loss increased by 0.1 mg/mm per each 0.1 mm increase in intertegular distance (t252 = 2.14, p = 0.033; Figure S1).
There was no effect of the fall duration treatment on pre-emergence weight loss in Osmia spp. (Table S4).
In Megachile spp., the effect of temperature on pre-emergence weight loss was opposite to that observed in Osmia spp. and depended on the spring onset treatment (Table 2). In the late-spring onset treatment, temperature affected pre-emergence weight loss, with male Megachile losing 4.0 ± 1.2 mg/mm more weight in the cool temperature treatment compared to the warm treatment (t38 = 3.44, p = 0.030; Figure 4E), and females losing 6.1 ± 0.9 mg/mm more weight in the cool treatment compared to the warm treatment (t38 = 6.83, p < 0.001; Figure 4E). The difference in mean pre-emergence weight loss between the cool temperature treatment and the warm treatment was not significant for either male or female bees when spring onset was early (p-values > 0.095; Figure 4E). These patterns resulted in the greatest pre-emergence weight loss occurring in the cool treatment when spring onset was late for both male and female bees (average weight loss across sex: 13.9 ± 1.1 mg/mm; Figure 4E).
Neither fall duration nor sex influenced pre-emergence weight loss in Megachile spp. (Table S4).
In Osmia spp., mean post-emergence lifespan (without feeding) was 1.0 ± 0.2 days longer in male bees compared to female bees (t279 = 4.48, p < 0.001; Figure 4C and Table 2). Temperature affected mean post-emergence lifespan in Osmia spp., but the effect of temperature depended on spring onset, fall duration, and sex (Table 2). In the early-spring onset treatment, mean male post-emergence lifespan was 2.0 ± 0.3 days shorter in the warm temperature treatment compared to the cool treatment (t279 = 6.28, p < 0.001; Figure 4C). In the late-spring onset treatment, the effect of temperature on mean male post-emergence lifespan depended on fall duration (Table 2). In the late-spring onset + long-fall duration treatment combination, mean male Osmia post-emergence lifespan was 1.3 ± 0.3 days shorter in the warm temperature treatment compared to the cool treatment (t279 = 3.93, p = 0.001; Figure 4C). In contrast, the effect of the temperature treatment on mean male post-emergence lifespan was not significant in the late-spring onset treatment when fall duration was short (t279 = 0.60, p = 0.558; Figure 4C). For female Osmia, post-emergence lifespan was 1.6 ± 0.4 days shorter on average in the warm temperature treatment compared to the cool treatment, but only in the early-spring onset treatment when fall duration was long (t279 = 4.02, p < 0.001; Figure 4C).
In contrast to Osmia spp., mean post-emergence lifespan in Megachile spp. was shorter in the cool temperature treatment compared to the warm treatment (1.8 ± 0.3 days shorter, t34 = 6.07, p < 0.001; Figure 4F), but the effect size of the temperature treatment depended on the spring onset treatment (Table 2). In the cool temperature treatment, Megachile individuals lived 2.8 ± 0.5 days longer on average compared to the warm treatment when spring onset was late (t34 = 5.60, p < 0.001; Figure 4F), but only 0.8 ± 0.3 days longer when spring onset was early (t34 = 2.74, p = 0.011; Figure 4F). These patterns resulted in the longest mean post-emergence lifespan (4.3 ± 0.4 days) occurring in the warm treatment when spring onset was late, and the shortest mean post-emergence lifespan (1.2 ± 0.4 days) occurring in the cool treatment when spring onset was late.
Sex did not influence mean post-emergence lifespan in Megachile spp. (Table S4).
The Osmia model (i.e., adult-wintering species) did not differ significantly from the data (Fisher C-score = 0.94, df = 2, p = 0.625; Figure 5), indicating our model provided an appropriate fit (Lefcheck, 2016). Our model was most effective at describing days to emergence (R2c = 0.65), followed by longevity (R2c = 0.49), then weight loss (R2c = 0.16).
Figure 5. Shows results of the Piecewise Structural Equation Analyses separately for Osmia spp. and Megachile spp. Directional arrows denote significant (α ≤ 0.05) effects. Percentages associated with each arrow are range standardized effect sizes (i.e., the percent of the range of values for a categorical response variable, or as the percent change per one unit increase in a continuous explanatory variable). Solid arrows represent a positive effect and dashed arrows represent a negative effect, with arrow thickness corresponding to the size of the effect. Effect sizes for categorical variables represent the effect of the stated level of the independent variable shown in each box compared to the other level of that variable. For example, in the Megachile spp. model, the warm temperature treatment caused a 72.4% decrease in the range of values for days to emergence in Megachile spp. compared to the cool temperature treatment.
There was a significant positive correlation between pre-emergence weight loss and the number of days to emergence relative to the start of the spring treatment, with a 29.5 ± 12.1% increase in days to emergence associated with each 1 mg/mm increase in weight loss (p = 0.016; Figure 5). The effect sizes of the significant (α ≤ 0.005) direct treatment effects on pre-emergence weight loss were small (> 0.03; Figure 5), which suggests that pre-emergence weight loss was more strongly influenced by the indirect effects of treatments via their influence on emergence timing, particularly early-spring onset. This also suggests that the timing of emergence responded more strongly to the direct effect of early-spring onset compared to the influence on pre-emergence weight loss. In addition, there was a significant negative correlation between pre-emergence weight loss and post-emergence lifespan, with post-emergence lifespan decreasing by 34.4 ± 7.4% (p < 0.001) per each 1 mg/mm increase in pre-emergence weight loss (Figure 5). Weak direct effects of treatments on pre-emergence weight loss suggest that post-emergence lifespan responded most strongly to the direct effect of early-spring onset (16 ± 1.8% decrease in lifespan compared to late-spring onset, p < 0.001) and to the indirect effect of early-spring onset via the effect of the early-spring treatment on the timing of emergence and pre-emergence weight loss (i.e., the early spring—emergence—weight loss—lifespan pathway; Figure 5).
The Megachile model (i.e., prepupal-wintering species) did not differ significantly from the data (fisher C-score = 1.01 df = 4, p = 0.909; Figure 5), suggesting an appropriate fit to the data (Lefcheck, 2016). Our model was most effective at describing emergence (R2c = 0.97), followed by weight loss (R2c = 0.56), and longevity (R2c = 0.46).
Days to emergence relative the start of the spring treatment influenced pre-emergence weight loss, with a 13.5 ± 5.5% (p = 0.017) increase in pre-emergence weight loss per each one day increase in days to emergence (Figure 5). The warm treatment indirectly reduced pre-emergence weight loss by 9.4% compared to the cool treatment via the effect of the temperature treatment on days to emergence (Figure 5). In addition, there was a significant negative correlation between pre-emergence weight loss and post-emergence lifespan, with post-emergence lifespan decreasing by 33.3 ± 13.0% (p = 0.014) per each 1 mg/mm increase in weight loss (Figure 5). Thus, it appears that the effect of temperature on post-emergence lifespan in Megachile spp. was mediated by the indirect effect of temperature on days to emergence and weight loss (i.e., the temperature—emergence—weight loss—lifespan pathway; Figure 5).
Our ability to understand and forecast the consequences of climate change for plant-pollinator interactions has benefited from linking plant life history traits to response patterns observed among plants species (e.g., Fitter and Fitter, 2002; Sherry et al., 2007; Cook et al., 2012; Burkle et al., 2013; Kudo and Ida, 2013; Gezon et al., 2016; Pardee et al., 2018). However, such progress with pollinator species is lacking. Here, we investigated the influence of overwintering life history, sex, and body size on solitary bee responses to controlled temperature and season length manipulations that spanned fall, winter, and spring. There were negative direct and indirect effects of early spring onset, increased temperature, and a long fall season on winter emergence, pre-emergence mortality, pre-emergence weight loss, and post-emergence lifespan in Osmia spp. (i.e., adult-wintering species), but not Megachile spp. (i.e., prepupal-wintering species). This indicates that climate change may be more detrimental to the fitness of adult-wintering species compared to prepupal-wintering species. In addition, there were treatment effects on emergence that depended on overwintering life history and sex. Observed phenological responses suggest that there may be implications of climate change for phenological synchrony among plant and pollinator species, as well as intraspecific male-female interactions that can be better understood by considering pollinator overwintering life histories.
Reduced cold exposure during adult diapause experienced by Osmia spp. in the early-spring onset treatment likely delayed emergence relative to the start of the spring treatment and increased pre-emergence weight loss, as well as increased pre-emergence mortality in O. coloradensis and O. tersula. In laboratory studies on Osmia lignaria, shorter durations of cold exposure can delay adult diapause termination, resulting in delayed emergence relative to the start of spring incubation (i.e., exposure to warm temperatures that elicit emergence, Bosch and Kemp, 2003, 2004; Sgolastra et al., 2010). In both laboratory and field studies on Osmia spp., delayed emergence can cause rapid metabolic expenditure, resulting in depleted stored-energy reserves at emergence, and increased pre-emergence mortality (i.e., O. lignaria, O. cornuta, and O. ribifloris, Bosch and Kemp, 2003, 2004; Sgolastra et al., 2010; CaraDonna et al., 2018). Responses to early-spring onset observed across Osmia spp. in this study were consistent with previous studies, suggesting that responses to cold exposure during adult diapause may be similar among species of Osmia. This interpretation is further supported by structural equation analyses in this study, which revealed a positive correlation between the number of days to emergence and weight loss in Osmia spp. Taken together, our findings indicate that Osmia spp. may be vulnerable to increased pre-emergence energy depletion and higher rates of pre-emergence mortality under shorter winter seasons caused by climate change.
However, the duration of winter was equal (i.e., 90 days) in both the early-spring + short-fall treatment combination and the late-spring + long-fall treatment combination, indicating that the timing of the spring treatment, and not just the duration of the winter treatment, drove the observed responses by Osmia spp. in this study. In O. lignaria, the timing of adult diapause initiation may influence the timing of emergence by determining when cold exposure begins accumulating, and in turn, when diapause termination occurs. Given that adult diapause is initiated in O. lignaria at the end of summer relative to adult eclosion, and is not cued by cold temperatures (Sgolastra et al., 2010), Osmia spp. in this study likely initiated adult diapause prior to or shortly after the start of the fall treatment. In the fall treatment, temperatures in both the warm and cool treatments were within the range at which Osmia spp. can successfully overwinter in the laboratory (Bosch and Kemp, 2003, 2004; Sgolastra et al., 2010). Therefore, Osmia spp. likely accumulated cold exposure outside of the “winter” period, especially prior to the start of the spring treatment when spring onset was late compared to early. These explanations align with reduced cold exposure as the most plausible cause of the observed responses by Osmia spp. in the early-spring onset treatment.
While the warm temperature treatment did not affect the timing of Osmia emergence, it resulted in increased weight loss. This weight loss was likely due to increased energetic expenditure during fall (Bosch and Kemp, 2003, 2004; Sgolastra et al., 2011; Schenk et al., 2018a) and when emergence was delayed by the early-spring onset treatment. However, only female Osmia were susceptible to this temperature-induced weight loss, suggesting that female Osmia may be more vulnerable to increased pre-emergence energy consumption under climate change compared to males. We also found an effect of body size on weight loss, suggesting that larger bees may store and expend more energy compared to smaller bees (O'Neill et al., 2014). But, because body size did not interact with any treatments, larger bees may not be likely to experience disproportionate effects of climate change via increased energy consumption. Increased female weight loss in response to the warm treatment and the early spring treatment indicate that multiple response mechanisms operating within different life cycle stages of solitary bees may interact to affect pre-emergence energy consumption. Investigating these mechanisms will be important while continuing to build a better understanding of how pollinator species are responding to climate change. Here, both temperature and season length resulted in increased weight loss, whereas only early-spring onset affected the timing of emergence. This suggests that increased pre-emergence energy consumption may be a consistent consequence of climate change for Osmia spp., whereas shifts in the timing of emergence may depend more on variation in winter temperature and duration (Forrest and Thomson, 2011; CaraDonna et al., 2018), compared to fall temperature and duration.
When assessing mortality, it is possible that factors unrelated to our treatments, such as parasitism (Wcislo and Cane, 1996), pathogens (Evison and Jensen, 2018), or inadequate provision quantity (Torchio, 1985), may have caused pre-emergence mortality in this study. Alternatively, some species of Osmia demonstrate parsivoltinism (i.e., can overwinter for one or two years prior to emerging (Torchio and Tepedino, 1982; Forrest et al., 2019), which could account for non-emerged bees following one winter in this study. However, we favor energy depletion caused by delayed emergence as the most plausible explanation of increased pre-emergence mortality in O. coloradensis and O. tersula for several reasons. First, we excluded parasitized bees from the analysis of pre-emergence mortality. Second, we only analyzed non-emerged bees that had developed into adults, and development to the adult life stage is inconsistent with mortality caused by pathogens (Evison and Jensen, 2018), as well as the decision to forgo emergence following a single winter (Torchio and Tepedino, 1982; Forrest et al., 2019). Provision quantity could have influenced mortality, but care taken to distribute individuals from the same mother and collection location equally across treatments likely reduced any bias in provision quantities among treatments.
In contrast to Osmia spp., neither the timing of spring onset nor the duration of fall caused increased weight loss or increased pre-emergence mortality in Megachile spp. As expected, the timing of emergence and weight loss in Megachile spp. was affected primarily by temperature. Because prepupal-wintering bees develop into adults following prepupal diapause, warmer spring temperature likely accelerated developmental rates and advanced the timing of emergence relative to the start of the spring treatment, an interpretation that is consistent with previous laboratory studies on Megachile rotundata (Kemp and Bosch, 2000; O'Neill et al., 2011). Less pre-emergence weight loss in the warm temperature treatment compared to the cool treatment is also consistent with previous work on M. rotundata, in which body fat content increased with post-winter temperatures within the range used in this study (O'Neill et al., 2011). In this study, reduced pre-emergence weight loss in the warm temperature treatment was likely a result of accelerated development. Megachile rotundata that develop more quickly in the laboratory tend to emerge earlier, which results in less time spent in spring incubation temperatures and less stored-energy depletion prior to emergence (Kemp and Bosch, 2000; O'Neill et al., 2011). However, the effect of temperature on pre-emergence weight loss in this study only occurred in the late-spring onset treatment, despite similar emergence responses to temperature in both the early- and late-spring onset treatments. This surprising result implies that the timing of spring onset, not just the interplay between post-winter developmental rates and the timing of emergence, influenced weight loss in Megachile spp. To our knowledge, interplay between the magnitude and timing of post-winter temperature has not been previously shown to affect pre-emergence weight loss in Megachile spp. Further research is needed to understand how post-winter temperature may interact with the timing of spring onset to influence physiological processes in Megachile spp.
Pre-emergence mortality responded inconsistently across Osmia spp., and was low in both species of Megachile, suggesting that increased pre-emergence mortality in response to shorter winters associated with climate change may be a species-specific response among species of Osmia, and not a widespread consequence for Megachile spp. On the other hand, increased weight loss in the early-spring onset treatment was observed across Osmia spp., and for female Osmia in the warm treatment. If pre-emergence weight loss is indicative of stored-energy consumption, the amount of energy available to Osmia spp. for fitness-related activities, such as gamete production, mating, nest construction, and offspring provisioning (Hahn and Denlinger, 2007; O'Neill et al., 2015) may be affected by climate change (Bosch et al., 2010). Although we cannot draw a causative link between increased pre-emergence weight loss and post-emergence fitness in this study, structural equation analyses revealed a significant negative correlation between pre-emergence weight loss and post-emergence lifespan without feeding in both Osmia spp. and Megachile spp. Post-emergence lifespan is an important predictor of fitness in solitary bees (Bosch and Vicens, 2006). Thus, if the observed relationship between pre-emergence weight loss and post-emergence lifespan holds in natural systems, our findings suggest that Osmia spp. may be vulnerable to post-emergence fitness consequences under climate change. This relationship was particularly strong in female Osmia in the early-spring onset treatment when fall was long, suggesting female Osmia may be disproportionately susceptible to post-emergence fitness consequences caused by shorter winter durations. Alternatively, solitary bees may be able to overcome any potential post-emergence fitness consequences of increased pre-emergence energy consumption by consuming nectar and pollen after they emerge (Sgolastra et al., 2016). More work is needed to understand how rearing conditions and post-emergence nectar and pollen availability may affect fitness in solitary bees.
In contrast to Osmia spp., a positive effect of early-spring onset and warmer temperatures on pre-emergence weight loss in Megachile spp. suggests that Megachile spp., and possibly other species of solitary bees that overwinter as prepupae, may be less vulnerable to potential negative fitness consequences associated with increased stored-energy depletion under climate change, a conclusion reached by previous authors (Bosch et al., 2010; Fründ et al., 2013). Furthermore, the negative correlation between pre-emergence weight loss and post-emergence lifespan without feeding revealed by structural equation analyses in this study suggests that warmer springs may aid fitness in Megachile spp. Importantly, however, spring temperatures used to rear Megachile spp. in this study were at the lower end of the temperature range suitable for development of M. rotundata reared in the laboratory (O'Neill et al., 2011). In nature, post-winter development in Megachile spp. is likely influenced by spring onset as well as daily mean and maximum temperatures during summer (Kemp and Bosch, 2000), which are likely higher than the temperatures used in this study. This is important because higher post-wintering temperatures cause non-linear effects on development, emergence timing, and fat loss in M. rotundata in the laboratory (O'Neill et al., 2011; Yocum et al., 2012). If M. lapponica and M. relativa respond to temperature in a similar manner to M. rotundata, the effects of temperature on the timing of emergence and pre-emergence weight loss likely depend on the temperature and timing of summer. In future work, it will be important to incorporate spring and summer treatments into experimental examinations of how Megachile spp. are responding to climate change.
In addition to weight loss, shifts in the timing of emergence can have implications for fitness in solitary bees by impacting temporal synchrony among plant and pollinator species (Forrest, 2015). For Osmia spp., delayed emergence in response to the early-spring onset treatment is seemingly at odds with the widely documented pattern showing earlier emergence dates in spring-active pollinator species under climate change (e.g., Bartomeus et al., 2011; Burkle et al., 2013; Kudo and Ida, 2013). However, treatment effects on the timing of emergence in Osmia spp. in this study were small (ca. 2–3 days). Regardless of the timing of spring onset, Osmia spp. emerged in <7 days on average relative to the start of the spring treatment. This indicates that the timing of emergence in Osmia spp. is highly plastic, and responsive to spring warming, which is consistent with advancing emergence phenologies reported for spring-active pollinator species by other authors. The degree to which shifts in phenology among Osmia spp. will affect synchrony with flowering periods of spring-flowering plants will largely depend on whether the cues that drive flowering phenologies are the same as those that drive the timing of emergence in Osmia spp., and, if separate cues are used, whether climate-driven changes in these separate cues are correlated (Lambert et al., 2010).
For Megachile spp., our findings indicate that the timing of emergence will advance under warmer spring temperatures. However, because changes in summer temperature under climate change will also influence the timing of emergence in Megachile spp., our ability to speculate about the consequences of climate change for emergence phenologies among Megachile spp. is limited. Importantly, summer-flowering plant species can be more prone to maintain or delay their phenologies in response to climate change compared to spring-flowering plants (Fitter and Fitter, 2002; Sherry et al., 2007; Cook et al., 2012). Thus, it will be important to understand whether phenological responses to temperature in summer-active pollinators, such as Megachile spp., will lead to divergent shifts in phenology with summer-flowering plants.
Interestingly, winter emergence in O. lignaria (i.e., emergence occurring prior to being transferred to the spring treatment) indicates that O. lignaria can emerge in the absence of perceiving spring warming (Bosch and Kemp, 2003). It could be that O. lignaria responded to depleted energy reserves by emerging, regardless of perceiving the onset of spring, a mechanism suggested to exist in some diapausing insects (Irwin and Lee, 2000; Hahn and Denlinger, 2007). A stronger effect of warm, extended fall conditions on winter emergence in males compared to females when spring onset was late suggests that emergence may be more constrained in male O. lignaria, potentially as a result of reduced energy availability at the start of the experiment (i.e., O. lignaria males entered the experiment with 8.5 mg/mm less mass compared to females; t252 = 9.96, p < 0.001). Emerging prior to the onset of spring would likely increase mortality in O. lignaria due to scarce or absent floral resource availability when they emerge (Schenk et al., 2018b), which could have important implications for O. lignaria populations under climate change. However, given that the likelihood of winter emergence was low when spring-onset was early suggests that climate change may not increase the occurrence of this response. On the other hand, sex-specific sensitivity to warmer, longer fall seasons could differentially alter the timing of male and female emergence, as well as disproportionately affect male mortality. Because O. lignaria populations have male-biased sex ratios (Torchio and Tepedino, 1980) and demonstrate protandry (i.e., males emerge prior to females), changes in population sex ratios driven by increased male mortality (Torchio and Tepedino, 1980), or changes in the mean population-level degree of protandry (Morbey and Ydenberg, 2001), could have ecological and evolutionary implications. This potential consequence of climate change deserves further investigation.
When speculating about the implications of our results for solitary bees under climate change, we acknowledge that the responses we observed under artificial temperature and season length manipulations may not accurately represent bee responses in natural settings. In addition to the potential for non-linear responses to temperature in Megachile spp. (O'Neill et al., 2011), bees typically experience fluctuating temperatures. In the laboratory, rearing solitary bees under fluctuating temperatures can cause different responses compared to constant temperatures (Kemp and Bosch, 2000; Rinehart et al., 2011). An important path forward will be to incorporate this variation into manipulative studies in the laboratory and in the field. In addition, we were largely unable to analyze responses at the species level, and our sample sizes differed by species. It is therefore possible that responses we observed when grouping species together by genus were driven by a subset of species. Assessing species-specific response patterns among species with shared traits will help build a better understanding of how pollinator life history traits mediate responses to climate change.
In summary, we demonstrated that overwintering life stage can mediate solitary bee responses to manipulated temperature and seasonal timing treatments that spanned fall, winter, and spring. Our findings suggest that Osmia spp., which overwinter as adults, may be more prone to increased stored-energy depletion prior to emergence compared to Megachile spp., which overwinter as prepupae. These results indicate that Osmia spp. may be more vulnerable to pre-emergence mortality and negative post-emergence fitness consequences of climate change compared to Megachile spp. In addition, sex-specific responses, particularly winter emergence by male O. lignaria, suggest that climate change may affect male and female emergence phenology and post-emergence fitness differently, with potential implications for population-level mating success and sex ratios. This study highlights the importance of combining experimental manipulations across multiple life cycle stages to help reveal the trait-mediated mechanisms by which climate change is impacting species. We urge future researchers to continue disentangling the complex roles of life history traits in mediating responses to climate change, so that we can better understand and preserve the species interactions that support essential ecosystem services such as pollination.
The raw data supporting the conclusions of this manuscript will be made available by the authors, without undue reservation, to any qualified researcher.
AS and LB conceived the project and obtained funding and contributed to revisions. AS designed the project, collected and analyzed the data, and wrote the first draft of manuscript.
Montana Academy of Science, Montana Institute on Ecosystems, and Montana State University.
The authors declare that the research was conducted in the absence of any commercial or financial relationships that could be construed as a potential conflict of interest.
We thank K. O'Neill, J. Hu, and J. Mangold for providing helpful comments on drafts of this manuscript, and D. Baumbauer for assistance with equipment integral to the project. We also thank the two reviewers for their time and contributions to this article.
The Supplementary Material for this article can be found online at: https://www.frontiersin.org/articles/10.3389/fevo.2019.00314/full#supplementary-material
Alexander, L. V., Zhang, X., Peterson, T. C., Caesar, J., Gleason, B., Tank, A., et al. (2006). Global observed changes in daily climate extremes of temperature and precipitation. J. Geophys. Res. Atmosph. 111, 1–22. doi: 10.1029/2005JD006290
Bartomeus, I., Ascher, J. S., Wagner, D., Danforth, B. N., and Colla, S. (2011). Climate-associated phenological advances in bee pollinators and bee-pollinated plants. Proc. Natl. Acad. Sci. U.S.A. 108, 20645–20649. doi: 10.1073/pnas.1115559108
Benjamini, Y., and Hochberg, Y. (1995). Controlling the false discovery rate - a practical and powerful approach to multiple testing. J. R. Stat. Soc. Seri. Methodol. 57, 289–300. doi: 10.1111/j.2517-6161.1995.tb02031.x
Bosch, J., and Kemp, W. P. (2000). Development and emergence of the orchard pollinator Osmia lignaria (Hymenoptera: Megachilidae). Environ. Entomol. 29, 8–13. doi: 10.1603/0046-225X-29.1.8
Bosch, J., and Kemp, W. P. (2003). Effect of wintering duration and temperature on survival and emergence time in males of the orchard pollinator Osmia lignaria (Hymenoptera: Megachilidae). Environ. Entomol. 32, 711–716. doi: 10.1603/0046-225X-32.4.711
Bosch, J., and Kemp, W. P. (2004). Effect of pre-wintering and wintering temperature regimes on weight loss, survival, and emergence time in the mason bee Osmia cornuta (Hymenoptera: Megachilidae). Apidologie 35, 469–479. doi: 10.1051/apido:2004035
Bosch, J., Sgolastra, F., and Kemp, W. P. (2010). Timing of eclosion affects diapause development, fat body consumption and longevity in Osmia lignaria, a univoltine, adult-wintering solitary bee. J. Insect Physiol. 56, 1949–1957. doi: 10.1016/j.jinsphys.2010.08.017
Bosch, J., and Vicens, N. (2006). Relationship between body size, provisioning rate, longevity and reproductive success in females of the solitary bee Osmia cornuta. Behav. Ecol. Sociobiol. 60, 26–33. doi: 10.1007/s00265-005-0134-4
Buckley, L. B., and Kingsolver, J. G. (2012). Functional and phylogenetic approaches to forecasting species' responses to climate change. Ann. Rev. Ecol. Evol. Syst. 43, 205–226. doi: 10.1146/annurev-ecolsys-110411-160516
Burkle, L., Marlin, J. C., and Knight, T. M. (2013). Plant-pollinator interactions over 120 years: loss of species, co-occurrence, and function. Science 339, 1611–1615. doi: 10.1126/science.1232728
Burrows, M. T., Schoeman, D. S., Buckley, L. B., Moore, P., Poloczanska, E. S., Brander, K. M., et al. (2011). The pace of shifting climate in marine and terrestrial ecosystems. Science 334, 652–655. doi: 10.1126/science.1210288
Cane, J. H. (1987). Estimation of bee size using intertegular span (apoidea). J. Kans. Entomol. Soc. 60, 145–147.
CaraDonna, P. J., Cunningham, J. L., and Iler, A. M. (2018). Experimental warming in the field delays phenology and reduces body mass, fat content and survival: implications for the persistence of a pollinator under climate change. Funct. Ecol. 32, 2345–2356. doi: 10.1111/1365-2435.13151
Cariveau, D. P., Nayak, G. K., Bartomeus, I., Zientek, J., Ascher, J. S., Gibbs, J., et al. (2016). The allometry of bee proboscis length and its uses in ecology. PLoS ONE 11:e0151482. doi: 10.1371/journal.pone.0151482
Cariveau, D. P., and Winfree, R. (2015). Causes of variation in wild bee responses to anthropogenic drivers. Curr. Opin. Insect Sci. 10, 104–109. doi: 10.1016/j.cois.2015.05.004
Cook, B., Wolkovich, E. M., and Parmesan, C. (2012). Divergent responses to spring and winter warming drive community level flowering trends. Proc. Natl. Acad. Sci. U.S.A. 109, 9000–9005. doi: 10.1073/pnas.1118364109
Deutsch, C. A., Tewksbury, J. J., Huey, R. B., Sheldon, K. S., Ghalambor, C. K., Haak, D. C., et al. (2008). Impacts of climate warming on terrestrial ectotherms across latitude. Proc. Natl. Acad. Sci. U.S.A. 105, 6668–6672. doi: 10.1073/pnas.0709472105
Diamond, S., Frame, A. M., Martin, R. A., and Buckley, L. B. (2011). Species' traits predict phenological responses to climate change in butterflies. Ecology 92, 1005–1012. doi: 10.1890/10-1594.1
Evison, S. E. F., and Jensen, A. B. (2018). The biology and prevalence of fungal diseases in managed and wild bees. Curr. Opin. Insect Sci. 26,105–113. doi: 10.1016/j.cois.2018.02.010
Fitter, A., and Fitter, R. S. R. (2002). Rapid changes in flowering time in British plants. Science 296, 1689–1691. doi: 10.1126/science.1071617
Forrest, J. R. K. (2015). Plant-pollinator interactions and phenological change: what can we learn about climate impacts from experiments and observations? Oikos 124, 4–13. doi: 10.1111/oik.01386
Forrest, J. R. K., Cross, R., and CaraDonna, P. J. (2019). Two-year bee, or not two-year bee? How voltinism is affected by temperature and season length in a high-elevation solitary bee. Am. Natural. 193, 560–574. doi: 10.1086/701826
Forrest, J. R. K., and Thomson, J. D. (2011). An examination of synchrony between insect emergence and flowering in Rocky Mountain meadows. Ecol. Monogr. 81, 469–491. doi: 10.1890/10-1885.1
Fründ, J., Zieger, S. L., and Tscharntke, T. (2013). Response diversity of wild bees to overwintering temperatures. Oecologia 173, 1639–1648. doi: 10.1007/s00442-013-2729-1
Gezon, Z. J., Inouye, D. W., and Irwin, R. E. (2016). Phenological change in a spring ephemeral: implications for pollination and plant reproduction. Glob. Chang. Biol. 22, 1779–1793. doi: 10.1111/gcb.13209
Grace, J., and Bollen, K. (2005). Interpreting the results from multiple regression and structural equation models. Bull. Ecol. Soc. Am. 86, 283–295. doi: 10.1890/0012-9623(2005)86[283:ITRFMR]2.0.CO;2
Hahn, D., and Denlinger, D. L. (2007). Meeting the energetic demands of insect diapause: nutrient storage and utilization. J. Insect Physiol. 53, 760–773. doi: 10.1016/j.jinsphys.2007.03.018
Hegland, S., Nielsen, A., Lazaro, A., Bjerknes, A. L., and Totland, O. (2009). How does climate warming affect plant-pollinator interactions? Ecol. Lett. 12, 184–195. doi: 10.1111/j.1461-0248.2008.01269.x
Hodek, I., and Hodkova, M. (1988). Multiple role of temperature during insect diapause - a review. Entomol. Exp. Appl. 49, 153–165. doi: 10.1111/j.1570-7458.1988.tb02486.x
IPCC (2014). Climate Change 2014: Synthesis Report Contribution of Working Groups I, II, and III to the Fifth Assessment Report of the Intergovernmental Panel on Climate Change, eds Core Writing Team, RK Pachauri and LA Meyer (Geneva: IPCC), 151.
Irwin, J. T., and Lee, R. E. (2000). Mild winter temperatures reduce survival and potential fecundity of the goldenrod gall fly, Eurosta solidaginis (Diptera: Tephritidae). J. Insect Physiol. 46, 655–661. doi: 10.1016/S0022-1910(99)00153-5
Kemp, W. P., and Bosch, J. (2000). Development and emergence of the alfalfa pollinator Megachile rotundata (Hymenoptera: Megachilidae). Ann. Entomol. Soc. Am. 93, 904–911. doi: 10.1603/0013-8746(2000)093[0904:DAEOTA]2.0.CO;2
Kemp, W. P., and Bosch, J. (2001). Postcocooning temperatures and diapause in the alfalfa pollinator Megachile rotundata (Hymenoptera: Megachilidae). Ann. Entomol. Soc. Am. 94, 244–250. doi: 10.1603/0013-8746(2001)094[0244:PTADIT]2.0.CO;2
Kemp, W. P., Bosch, J., and Dennis, B. (2004). Oxygen consumption during the life cycles of the prepupa-wintering bee Megachile rotundata and the adult-wintering bee Osmia lignaria (Hymenoptera: Megachilidae). Ann. Entomol. Soc. Am. 97, 161–170. doi: 10.1603/0013-8746(2004)097[0161:OCDTLC]2.0.CO;2
Kingsolver, J. G., Woods, H. A., Buckley, L. B., Potter, K. A., MacLean, H. J., and Higgins, J. K. (2011). Complex life cycles and the responses of insects to climate change. Integr. Comp. Biol. 51, 719–732. doi: 10.1093/icb/icr015
Kudo, G., and Ida, T. Y. (2013). Early onset of spring increases the phenological mismatch between plants and pollinators. Ecology 94, 2311–2320. doi: 10.1890/12-2003.1
Lambers, H., Chapin III, F. S., and Pons, T. L. (2008). Plant Physiological Ecology. New York, NY: Springer Science & Business Media.
Lambert, A. M., Miller-Rushing, A. J., and Inouye, D. W. (2010). Changes in snowmelt date and summer precipitation affect the flowering phenology of erythronium grandiflorum (glacier lily; Liliaceae). Am. J. Bot. 97, 1431–1437. doi: 10.3732/ajb.1000095
Lefcheck, J. S. (2016). PiecewiseSEM: piecewise structural equation modelling in R for ecology, evolution, and systematics. Methods Ecol. Evol. 7, 573–579. doi: 10.1111/2041-210X.12512
Lenth, R. V., and Hervac, M. Ó. (2015). Lsmeans: Least-Squares Means R Package Version 216. Available online at: https://cran.r-project.org/web/packages/lsmeans/index.html
May, D. G. K. (1972). Water uptake during larval development of a sweat bee, Augochlora pura (Hymenoptera: Apoidea). J. Kansas Entomol. Soc. 45, 439–449.
McGavin, G. C. (1994). The bee genera of North and Central-America - michener, cd, mcginley, rj, danforth, bn. Nature 370, 261–262. doi: 10.1038/370261b0
Memmott, J., Craze, P. G., Waser, N. M., and Price, M. V. (2007). Global warming and the disruption of plant-pollinator interactions. Ecol. Lett. 10, 710–717. doi: 10.1111/j.1461-0248.2007.01061.x
Menzel, A., Sparks, T. H., Estrella, N., Koch, E., Aasa, A., Ahas, R., et al. (2006). European phenological response to climate change matches the warming pattern. Glob. Chang. Biol. 12, 1969–1976. doi: 10.1111/j.1365-2486.2006.01193.x
Michener, C. D. (2007). The Bees of the World, 2nd Edn. Baltimore, MD: John Hopkins University Press.
Morbey, Y. E., and Ydenberg, R. C. (2001). Protandrous arrival timing to breeding areas: a review. Ecol. Lett. 4, 663–673. doi: 10.1046/j.1461-0248.2001.00265.x
Ogilvie, J. E., and Forrest, J. R. K. (2017). Interactions between bee foraging and floral resource phenology shape bee populations and communities. Curr. Opin. Insect Sci. 21, 75–82. doi: 10.1016/j.cois.2017.05.015
O'Neill, K. M., Delphia, C. M., and O'Neill, R. P. (2014). Oocyte size, egg index, and body lipid content in relation to body size in the solitary bee Megachile rotundata. Peerj. 2:e314. doi: 10.7717/peerj.314
O'Neill, K. M., Delphia, C. M., and Pitts-Singer, T. L. (2015). Seasonal trends in the condition of nesting females of a solitary bee: wing wear, lipid content, and oocyte size. Peerj 3:e930. doi: 10.7717/peerj.930
O'Neill, K. M., O'Neill, R. P., Kemp, W. P., and Delphia, C. M. (2011). Effect of temperature on post-wintering development and total lipid content of Alfalfa Leafcutting Bees. Environ. Entomol. 40, 917–930. doi: 10.1603/EN10320
Pardee, G. L., Inouye, D. W., and Irwin, R. E. (2018). Direct and indirect effects of episodic frost on plant growth and reproduction in subalpine wildflowers. Glob. Chang. Biol. 24, 848–857. doi: 10.1111/gcb.13865
Pinheiro, J., Bates, D., DebRoy, S., Sarkar, D., and R Development Core Team (2013). nlme: Linear and Nonlinear Mixed Effects Models R Package Version, 31–111.
Pitts-Singer, T. L., and James, R. R. (2009). Prewinter management affects Megachile rotundata (Hymenoptera: Megachilidae) prepupal physiology and adult emergence and survival. J. Econ. Entomol. 102, 1407–1416. doi: 10.1603/029.102.0402
Post, E. S., Pedersen, C., Wilmers, C. C., and Forchhammer, M. C. (2008). Phenological sequences reveal aggregate life history response to climatic warming. Ecology 89, 363–370. doi: 10.1890/06-2138.1
Potts, S. G., Imperatriz-Fonseca, V., Ngo, H. T., Aizen, M. A., Biesmeijer, J. C., Breeze, T. D., et al. (2016). Safeguarding pollinators and their values to human well-being. Nature 540, 220–229. doi: 10.1038/nature20588
R Core Team (2013). R: A Language and Environment for Statistical Computing. R Foundation for Statistical Computing,Vienna, Austria. Available online at: https://www.r-project.org/
Radmacher, S., and Strohm, E. (2010). Factors affecting offspring body size in the solitary bee Osmia bicornis (Hymenoptera, Megachilidae). Apidologie 41, 169–177. doi: 10.1051/apido/2009064
Rafferty, N. E. (2017). Effects of global change on insect pollinators: multiple drivers lead to novel communities. Curr. Opin. Insect Sci. 23, 22–27. doi: 10.1016/j.cois.2017.06.009
Rightmyer, M. G., Griswold, T., and Brady, S. G. (2013). Phylogeny and systematics of the bee genus Osmia (Hymenoptera: Megachilidae) with emphasis on North American Melanosmia: subgenera, synonymies and nesting biology revisited. Syst. Entomol. 38, 561–576. doi: 10.1111/syen.12013
Rinehart, J. P., Yocum, G. D., West, M., and Kemp, W. P. (2011). A fluctuating thermal regime improves survival of cold-mediated delayed emergence in developing Megachile rotundata (Hymenoptera: Megachilidae). J. Econ. Entomol. 104, 1162–1166. doi: 10.1603/EC11062
Schenk, M., Krauss, J., and Holzschuh, A. (2018b). Desynchronizations in bee-plant interactions cause severe fitness losses in solitary bees. J. Anim. Ecol. 87, 139–149. doi: 10.1111/1365-2656.12694
Schenk, M., Mitesser, O., Hovestadt, T., and Holzschuh, A. (2018a). Overwintering temperature and body condition shift emergence dates of spring-emerging solitary bees. Peerj 6:e4721. doi: 10.7717/peerj.4721
Sgolastra, F., Arnan, X., Pitts-Singer, T. L., Maini, S., Kemp, W. P., and Bosch, J. (2016). Pre-wintering conditions and post-winter performance in a solitary bee: does diapause impose an energetic cost on reproductive success? Ecol. Entomol. 41, 201–210. doi: 10.1111/een.12292
Sgolastra, F., Bosch, J., Molowny-Horas, R., Maini, S., and Kemp, W. P. (2010). Effect of temperature regime on diapause intensity in an adult-wintering Hymenopteran with obligate diapause. J. Insect Physiol. 56, 185–194. doi: 10.1016/j.jinsphys.2009.10.001
Sgolastra, F., Kemp, W. P., Buckner, J. S., Pitts-Singer, T. L., and Maini, S. (2011). The long summer: pre-wintering temperatures affect metabolic expenditure and winter survival in a solitary bee. J. Insect Physiol. 57, 1651–1659. doi: 10.1016/j.jinsphys.2011.08.017
Sheffield, C. S., Ratti, C., Packer, L., and Griswold, T. (2011). Leafcutter and mason bees of the genus Megachile Latreille (Hymenoptera: Megachilidae) in Canada and Alaska. Can. J. Arthropod Identif. 18:29. doi: 10.3752/cjai.2011.18
Sherry, R., Zhou, X., Gu, S., Arnone, J. A., and Schimel, D. S. (2007). Divergence of reproductive phenology under climate warming. Proc. Natl. Acad. Sci. U.S.A. 104, 198–202. doi: 10.1073/pnas.0605642104
Sparks, T. H., and Menzel, A. (2002). Observed changes in seasons: an overview. Int. J. Climatol. 22, 1715–1725. doi: 10.1002/joc.821
Stephen, W. P., Bohart, G. W., and Torchio, P. F. (1969). “The biology and external morphology of bees with a synopsis of the genera of northwestern America,” in The Biology and External Morphology of Bees with a Synopsis of the Genera of Northwestern America (Corvallis, OR: The Agricultural Research Station; Oregon State University), 140.
Stephen, W. P., and Torchio, P. F. (1961). Biological notes on the leafcutter bee Megachile (Eutricharaea) rotundata (Fabricius) (Hymenoptera: Megachilidae). Pan-Pac. Entomology 37, 89–93.
Torchio, P. F. (1985). Field experiments with the pollinator species, Osmia lignaria Propinqua Cresson, in apple orchards 5 (1979–1980), methods of introducing bees, nesting success, seed counts, fruit yields (Hymenoptera, Megachilidae). J. Kans. Entomol. Soc. 58, 448–464.
Torchio, P. F. (1989). In-nest biologies and development of immature stages of 3 Osmia species (Hymenoptera, Megachilidae). Ann. Entomol. Soc. Am. 82, 599–615. doi: 10.1093/aesa/82.5.599
Torchio, P. F., and Tepedino, V. J. (1980). Sex-ratio, body size and seasonality in a solitary bee, Osmia lignaria Propinqua Cresson (Hymenoptera, Megachilidae). Evolution 34, 993–1003. doi: 10.1111/j.1558-5646.1980.tb04037.x
Torchio, P. F., and Tepedino, V. J. (1982). Parsivoltinism in three species of Osmia bees. Psyche 89, 221–238. doi: 10.1155/1982/60540
Tylianakis, J. M., Didham, R. K., Bascompte, J., and Wardle, D. A. (2008). Global change and species interactions in terrestrial ecosystems. Ecol. Lett. 11, 1351–1363. doi: 10.1111/j.1461-0248.2008.01250.x
Vanbergen, A. J., Baude, M., Biesmeijer, J. C., Britton, N. F., Brown, M. J. F., Brown, M., et al. (2013). Threats to an ecosystem service: pressures on pollinators. Front. Ecol. Environ. 11, 251–259. doi: 10.1890/120126
Wcislo, W. T., and Cane, J. H. (1996). Floral resource utilization by solitary bees (Hymenoptera: Apoidea) and exploitation of their stored foods by natural enemies. Annu. Rev. Entomol. 41, 257–286. doi: 10.1146/annurev.en.41.010196.001353
Keywords: climate change, pollinators, overwinter, emergence, weight loss, lifespan
Citation: Slominski AH and Burkle LA (2019) Solitary Bee Life History Traits and Sex Mediate Responses to Manipulated Seasonal Temperatures and Season Length. Front. Ecol. Evol. 7:314. doi: 10.3389/fevo.2019.00314
Received: 01 March 2019; Accepted: 06 August 2019;
Published: 28 August 2019.
Edited by:
Gina Marie Wimp, Georgetown University, United StatesReviewed by:
Theresa L. Pitts-Singer, Pollinating Insect Biology, Management, Systematics Research (USDA-ARS), United StatesCopyright © 2019 Slominski and Burkle. This is an open-access article distributed under the terms of the Creative Commons Attribution License (CC BY). The use, distribution or reproduction in other forums is permitted, provided the original author(s) and the copyright owner(s) are credited and that the original publication in this journal is cited, in accordance with accepted academic practice. No use, distribution or reproduction is permitted which does not comply with these terms.
*Correspondence: Anthony H. Slominski, YW50aG9ueS5zbG9taW5za2lAbW9udGFuYS5lZHU=
Disclaimer: All claims expressed in this article are solely those of the authors and do not necessarily represent those of their affiliated organizations, or those of the publisher, the editors and the reviewers. Any product that may be evaluated in this article or claim that may be made by its manufacturer is not guaranteed or endorsed by the publisher.
Research integrity at Frontiers
Learn more about the work of our research integrity team to safeguard the quality of each article we publish.