- 1Department of Biology, Elmhurst College, Elmhurst, IL, United States
- 2Department of Plant Biology and Conservation, Northwestern University, Evanston, IL, United States
- 3Chicago Botanic Garden, Glencoe, IL, United States
Gene flow between populations can help maintain genetic diversity and prevent inbreeding, which is especially important for small, fragmented habitats. Many plant species rely on pollinators to move pollen between populations. In urban areas, insufficient pollinator services may result in limited gene flow, which can have negative consequences such as genetic drift and inbreeding depression. Furthermore, restored populations that are established with few founders of low genetic diversity may have limited long-term population persistence. Here, we tested the hypotheses that populations of a self-compatible forb established on urban green roofs from nursery stock are genetically depauperate and that limited gene (pollen) flow between populations will result in increased inbreeding. We compared the neutral genetic diversity of Penstemon hirsutus, using nine microsatellite loci, between three green roof populations established from nursery stock and three natural populations. We also established ten experimental populations on green roofs and measured rates of outcrossing and inbreeding and identified the movement of pollen within and between roofs using a paternity analysis. We found that neutral genetic diversity of populations established from nursery stock was lower than that of natural populations, although the level of inbreeding was also lower on the green roofs. In our experimental populations, we found that the rates of outcrossing and inbreeding varied between the roof populations. Our results suggest that inbreeding may be correlated with cover of co-flowering species but not with any of the other measured site properties. The location of likely pollen donors suggested that on average, 75% of pollen was derived from plants within the population (including self) and 25% came from plants on different roofs. Our results document realized pollen movement within and between green roofs, demonstrating that these habitats provide important connectivity in a fragmented environment.
Introduction
Green spaces are increasingly valued for their role in providing habitat for the conservation of native plants and wildlife in urban and suburban areas (Goddard et al., 2009; Kowarik, 2011). These spaces encompass a range of habitat types and composition, including parks, gardens, vacant lots and constructed green roofs (Given, 1994; Dunn and Heneghan, 2011; Müller et al., 2013). The resultant heterogeneous environment may provide unique opportunities to support biodiversity (Miller and Hobbs, 2002), including providing refuge for rare species (Ives et al., 2016), but can also impose challenges not faced in natural ecosystems, leading to increased extinction (Williams et al., 2005). The extent to which urban green spaces can contribute to the conservation of biodiversity is only beginning to be assessed.
Habitat fragmentation dominates the urban ecosystem and contributes to population isolation. While many plant species naturally occur in heterogeneous landscapes, the intervening matrix in urban areas may make urban populations more isolated than they would be in the wild (Frankham et al., 2002; Johnson et al., 2015; Hejkal et al., 2017). For plant species in particular, seed and pollen dispersal may be inhibited or limited in ways that can suppress important life history events (i.e., reproduction, mating dynamics, recruitment) and thus long-term population persistence (Given, 1994; Handel et al., 2013; Dubois and Cheptou, 2017). Ecological circumstances, such as a variation in pollination services, can influence reproductive assurance (i.e., increase selfing rates; Kalisz et al., 2004). For example, isolated patches of Crepis sancta in urban areas experience lower pollinator visitation rates than do populations in rural areas (Andrieu et al., 2009). Such differences can contribute to high rates of inbreeding and increasing population differentiation when compared to those in naturally-fragmented habitats or larger, more continuous populations (Breed et al., 2012, 2015). Furthermore, constructed urban populations like managed parks and gardens are commonly established using nursery-sourced plants that may be genetically depauperate compared to natural populations (Fant et al., 2008; Basey et al., 2015). Lower standing genetic diversity of the pollen donors can directly affect progeny fitness (Breed et al., 2015). The combined effects of increased isolation and limited genetic diversity may restrict the ability of urban plant populations to respond to environmental change and to limit their contribution to larger-scale (metapopulation) dynamics required for long-term persistence and survival (Reed and Frankham, 2003; Frankham, 2005; Van Rossum, 2009). While long-distance gene flow is important for maintaining genetic diversity in small populations (Ashley, 2010; Breed et al., 2011; Van Rossum and Triest, 2012), few studies have investigated the extent to which pollen and seed dispersal maintains genetic connectivity of plant populations in cities (La Point et al., 2015).
While theory predicts that one migrant per generation is enough to maintain diversity and counteract the negative effects of genetic drift (Wright, 1932; Lowe and Allendorf, 2010), highly managed populations, like those in urban areas, may require higher migration rates (Mills and Allendorf, 1996) and benefit from genetic rescue (Whiteley et al., 2015; Hendrick and Garcia-Dorado, 2016) if they have low standing genetic diversity. The ability to self-fertilize can ameliorate the effects of pollinator and pollen limitation in the short-term but inbreeding can create long-term challenges to population persistence if fitness is reduced (i.e., inbreeding depression; Frankham et al., 2002; Keller and Waller, 2002). Due to their spatial isolation (both vertical and horizontal), infrequent distribution, and occurrence in densely urbanized areas, green roof systems are an ideal setting for assessing the ability of urban ecosystems to achieve larger biodiversity conservation goals. Green roof populations may be especially susceptible to the negative effects of small population size, especially if they are established with nursery stock of low genetic diversity (Lundholm, 2015). In addition, outcrossing may be limited if pollinator abundance is low and movement (gene flow) between green roofs is infrequent (Colla et al., 2009; MacIvor and Lundholm, 2011; Tonietto et al., 2011; Ksiazek et al., 2012). For these reasons, self-compatible plant species may experience higher selfing rates in cities (Kalisz et al., 2004) and lower fitness on green roofs (Ksiazek et al., 2012) compared to those in natural areas. Conversely, if there is evidence of gene flow between green roof populations, this would suggest that small habitat patches may contribute to larger urban metapopulations and mitigate the detrimental outcomes associated with limited dispersal. Here, we test the following hypotheses using the self-compatible forb, Penstemon hirsutus: (1) Plant populations established on green roofs using nursery-stock are genetically depauperate compared to natural populations, and (2) Pollen movement is restricted in green roof populations, resulting in high selfing, restricted outcrossing events, and higher pollen flow within than between populations. We used nine microsatellite markers to compare genetic diversity metrics of three planted green roof populations in Chicago, IL and Glencoe, IL USA with those of three natural populations in the region (Putman and Carbone, 2014). We then established ten experimental populations on green roofs and used paternity assignment to assess outcrossing rates and patterns of gene flow within and between urban green roofs.
Materials and Methods
Study System
Penstemon hirsutus (L.) Willd (Scrophulariaceae) is a perennial forb native to the naturally-fragmented, dry, and rocky limestone grasslands of eastern North America (Clements, 1995; USDA, NRCS, 2006). It is occasionally used in urban and suburban plantings and is one of a handful of native species that can survive on green roofs in the Midwestern United States (Sutton et al., 2012). Mature P. hirsutus seeds lack specialized structures for dispersal (Clements, 1995), making pollen dispersal the primary mechanism of between-population gene flow. Penstemon hirsutus has a mixed mating system that includes xenogamy, geitonogamy and autogamy (Clements et al., 1999). Although P. hirsutus is self-compatible, it primarily relies on small solitary bees (Hoplitis and Ceratina species) for pollination (Crosswhite and Crosswhite, 1966). Both Hoplitis and Ceratina have been found in prairies within the study region (Tonietto et al., 2011) and Ceratina occur within the Chicago city limits (Tonietto et al., 2011; Lowenstein et al., 2014). Bee species of similar size and body shape that may also provide pollination services to P. hirsutus are present in Chicago (Lowenstein et al., 2014) and on green roofs specifically (Tonietto et al., 2011; Ksiazek et al., 2014).
Genetic Diversity of Green Roof vs. Natural Populations
Leaf tissue from 30 P. hirsutus individuals (Hale et al., 2012) from each of three green roofs and three natural populations (six populations and 180 individuals total) was collected, dried with silica gel, and stored at 2–5°C for subsequent genetic analysis. Green roof populations were located on the Quinlan Life Science Building on the campus of Loyola University and two subpopulations at the Rice Conservation Science Center at the Chicago Botanic Garden (all located in Cook County, Illinois, USA: Table 1). Each green roof population had an estimated size of between 50 and 100 individuals and each was established using plant material from different commercial nurseries. The natural populations were remnants located at the Midewin National Tallgrass Prairie and in two subpopulations at the Lockport Prairie Nature Preserve (all located in Will County, Illinois, USA: Table 1). Natural populations of P. hirsutus were small (<50 individuals) and no other patches of the species occurred within 1 km. Penstemon hirsutus has a high coefficient of conservatism (C = 9/10) in the Chicago region (Wilhelm and Rericha, 2017) and Will County, Illinois is urbanized and located at the western limit of P. hirsutus' range (Clements et al., 1998; USDA, NRCS, 2006; iNaturalist.org, 2019). Therefore, the natural populations observed in our study are likely smaller and more isolated than they would be elsewhere within North America.
Assessing Gene Flow Within and Between Green Roofs
We established 10 populations of P. hirsutus on green roofs of the Loyola University campus in Chicago, IL, USA during the summer of 2014 (Table 1, Figure 1) using bare root stock from Prairie Moon Nursery®. These plants served as the parental generation and were the only flowering individuals within the vicinity of the study that could have contributed to the pollen pool. Each of the ten populations consisted of five potted individuals grown in a greenhouse until the initiation of flowering. Pots were randomly selected using a number generator and spaced 2.5 m from a central individual and 5 m from others in the same repeated pattern like the 5 side of a die, centered on the vegetated portion of each green roof. Leaf tissue was collected from all parental plants, dried with silica gel, and stored at 2–5°C for DNA extraction and subsequent analyses. Potted plants remained on the green roofs until flowering was complete (6 weeks) and then were moved to an outdoor greenhouse and were covered with mesh exclusion bags (Delnet® Pollinator Bags, Delstar Technologies, Inc.; 0.5 mm diameter pore size) to prevent fruit and seed predation and to retain seeds as fruits matured. The following estimates of reproductive output were recorded for each of the 50 experimental plants: fruit to flower ratio, and mean fruit length (mm), mean fruit weight (g), and seed to ovule ratio for seven fruits per plant.
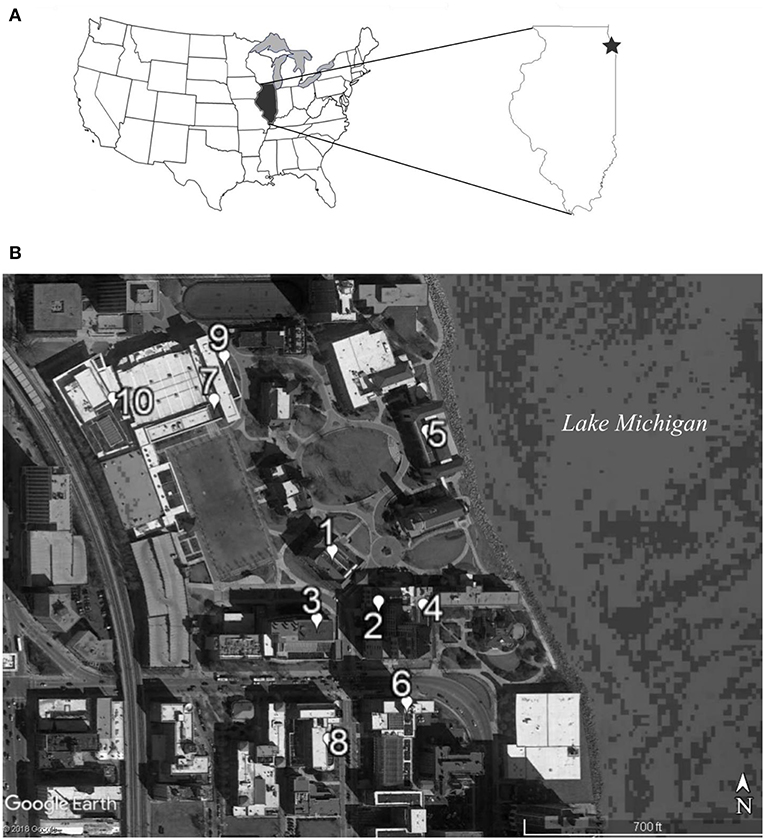
Figure 1. The 10 green roof sites used for the gene flow experiment were located in Chicago, IL USA (A) within a 0.12 km area on the western shore of Lake Michigan (B). Site numbers indicate the degree of proximity to all other sites, with site number 1 having the shortest mean distance to all other sites and site number 10 having the largest mean distance.
Offspring resulting from successful pollination events were genotyped to assess within- vs. between-roof gene flow. Seeds were removed from four fruits per individual, washed in a 10% bleach solution and double rinsed in deionized water before being plated on 0.75% agar plates and placed into cold dark stratification for 10 weeks at 2°C. Following cold stratification, seeds were germinated in a growth chamber set to a diurnal schedule of 10°C/12 h day, 2°C/12 h night for 4 weeks and then 17°C/13 h day, 3°C 11 h night for an additional 4 weeks with 40% relative humidity. We harvested four germinated seedlings per fruit and stored them at −80°C for subsequent DNA extraction and analysis.
We recorded biotic and abiotic characteristics of the 10 experimental green roof sites that may influence pollinator attractiveness and pollen movement: percent cover of flowering and non-flowering vegetation, co-flowering species diversity (during the study period once in June and again in July 2014), roof height (m), vegetated area of the roof (m2), latitude, and longitude. All green roof sites were located within a 0.12 km radius in a high-density urban environment, which lacked other populations of P. hirsutus (Figure 1). The mean distance between each population and the nine others was calculated from the center of each population. The distance between the center points of the experimental populations on all roofs was used to calculate the mean distance to all other sites. The next nearest large green space in the vicinity was an urban golf course ~3.5 km away, so additional pollen resources for the effective pollinators were most likely only available from small private gardens and street-side plantings.
DNA Extraction, Microsatellite Screening, and Genotyping
We extracted genomic DNA from ~1 cm2 of dried leaf tissue (or entire seedlings, in the case of the offspring generation) using a modified cetyltrimethylammonium bromide (CTAB) procedure (Doyle and Doyle, 1987). DNA quantity and quality were verified (Nanodrop 2000, ThermoScientific, USA). We tested a total of 46 primer pairs; 26 developed for Penstemon rostiflorus by Kramer and Fant (2007) and 20 developed for the Penstemon genus by Dockter et al. (2013). Nine of the 46 tested microsatellite markers that amplified reliably and were polymorphic in P. hirsutus were used for genotyping [Pen02, Pen03, Pen04, Pen06, Pen07, and Pen23 from Kramer and Fant (2007) and PS25, PS32, and PS53 from Dockter et al. (2013)]. The primer pair PS32 amplified two separate regions that did not appear to be linked and were counted as separate loci in the analyses. PCR reactions (10 μl) amplified DNA fragments using 5 μl 2x MyTaq Mix (Bioline, Taunton, MA, USA), 2.25 μl DNA-free H2O, 2 μl genomic DNA, 0.25 μl 2x bovine serum albumin (BSA Thermo Fisher Scientific, Waltham, MA, USA), 0.25 μl of each direct-labeled forward primer (WellRed D2, D3, or D4 fluorescent dye; Sigma-Aldrich Co. LLC St. Louis MO, USA), and 0.25 μl of each reverse primer. Initial denaturation was set at 94°C for 5 min, followed by 35 cycles of 94°C for 30 s, annealing for 30 s and 72°C for 2 min, and ending with a 10 min extension at 72°C. The following annealing temperatures were used: 48.8°C for Pen03, Pen04, and PS53, 53°C for Pen02, Pen06, and PS25 and 58.7°C for Pen07, Pen23, and PS32. PCR products were quantified using a CEQ 8000 Genetic Analysis System version 9.0 (Beckman Coulter, Brea CA, USA) with GenomeLab™ 600 (for PS25, PS32, and PS53) and GenomeLab™ 400 internal DNA size standards (for all remaining loci). We manually verified all fragment lengths and reran ~2% of the samples to ensure a low genotyping error rate. We excluded all samples that lacked fragment data at more than two loci.
Data Analyses
We first tested for the presence of null alleles in the full data set (natural and green roof maternal and offspring populations) using MicroChecker (Van Oosterhout et al., 2004). We conducted a STRUCTURE (v. 2.3.4) analysis using a LOCPRIOR model set to a burn-in of 10,000 with 10,000 iterations at K = 6 to confirm that the number of loci and number of alleles per loci were sufficient to detect genetic differentiation between the natural and green roof populations (Supplementary Information). We then used GenAlEx version 6.41 (Peakall and Smouse, 2006, 2012) to determine the population differentiation (FST) of the natural and established green roof populations and to estimate the following population genetic parameters: number of alleles (A), expected heterozygosity (HE), and inbreeding coefficient (FIS). We used FSTAT version 2.9.3 (Goudet, 2001) to estimate allelic richness (AR) per population corrected for sample size. We performed one-way ANOVAs to test for a significant effect of site type (natural prairie vs. green roof) on genetic diversity, measured as AR, HE, and FIS.
To compare genetic diversity between the green roof maternal and offspring populations, we took the mean of each diversity metric (FIS, AR, and HE) of each maternal individual's measured offspring and subtracted that from the diversity metric for the maternal individual. We used backward selection of generalized linear models (GLMs) to look for effects of site properties (vegetation and co-flowering cover, co-flowering species diversity, roof height and area, latitude, longitude, and mean distance to other sites) on the HE, AR and FIS of the offspring. We confirmed the best models from the GLM model-fitting using linear mixed effects models (LMEs, R package “lme4”) with site as a fixed effect and by performing one-way ANOVAs on each site property x diversity metric combination. We also used backward GLM selection and performed one-way ANOVAs to test for the effects of genetic diversity on reproductive traits (fruit to flower ratio, fruit weight, fruit length, and seed to ovule ratio).
We used a maximum-likelihood method in CERVUS version 3.0.7 (Marshall et al., 1998) to identify the most likely father for each offspring. CERVUS uses the natural log of the likelihood-odds ratio (LOD) score for each possible father using either a relaxed (80%) or strict confidence interval (95%). The individual with the highest LOD score of the population is deemed the most likely father. When there were multiple potential fathers, we assigned paternity to the individual with the highest LOD score at the greatest confidence interval. If there were multiple candidate fathers with the same LOD score, we conservatively selected the individual that was geographically closest. For each population, we calculated mean pollen flow distance using the average of the distances to the other populations when pollen flow between roofs occurred. We used backward elimination of GLMs to determine significant effects of site characteristics on mean pollen flow distance and confirmed the results of this model-fitting with an ANOVA of LMEs (R package “lme4”). We then used one-way ANOVAs to test for any stand-alone effects of site characteristics on mean pollen flow distance and proportion of offspring per population that were a result of between-site pollen flow, within-site pollen flow, or self-pollination. Unless otherwise noted, all statistical analyses were performed in R, version 3.3.1 (R Development Core Team., 2016).
Results
Genetic Diversity of Green Roof vs. Natural Populations
We amplified 94 alleles from 10 polymorphic loci from the three natural populations (mean 9 alleles per locus) and 95 alleles from the same loci in the three urban green roof populations (mean 10 alleles per locus). The number of alleles in our populations falls within the expected range of 7–13, as predicted by Kramer and Fant (2007). The mean number of alleles per locus per site was 6 in the natural populations and 5 in the green roof populations. The natural prairie populations of P. hirsutus were significantly higher in allelic richness (p = 0.001, R2 = 0.930, F = 67.721, 4) than the green roof populations, indicating greater genetic diversity (Table 2). Expected heterozygosity was also higher in the natural populations, although not significantly so (p = 0.381). However, the natural populations had significantly greater FIS values (p = 0.028, R2 = 0.672, F = 11.251, 4), indicating a higher incidence of inbreeding compared to green roof populations (Table 2). The average pairwise FST among green roof populations (0.193) was greater than the average pairwise FST among natural populations (0.084) and near the average calculated between green roof and natural populations (0.179).
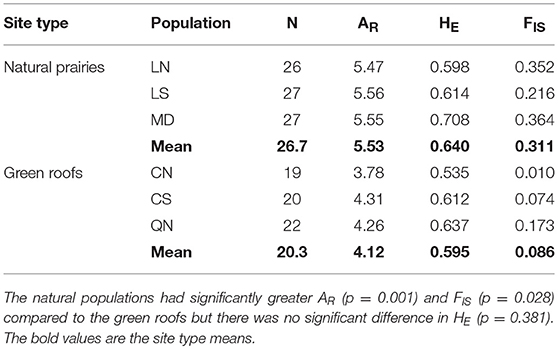
Table 2. Genetic summary statistics for three natural prairie and three green roof populations: Number of individuals (N), allelic richness (AR), expected heterozygosity (HE), and mean inbreeding coefficient (FIS).
Gene Flow Within and Between Green Roofs
Genetic diversity was low in the 10 experimental P. hirsutus populations established using nursery-stock, with only 34 alleles from 9 polymorphic loci in both the parental (mean 2 alleles per locus) and the offspring (mean 3 alleles per locus) generations. Both the average AR and HE were lower in the offspring generation than in the parental generation (Figure 2). The mean inbreeding coefficient remained close to zero for both generations, although the generational shift in FIS varied by experimental population, with roughly a third of populations showing an increase in inbreeding between parental and offspring generations, a third showing a decrease, and the remaining third showing no difference (Figure 2). Both the backward GLM selection (ΔAIC = 1.34) and the LME [ = 4.09, p = 0.043] indicated that greater cover of co-flowering species had a significant positive correlation with lower inbreeding coefficients (FIS) in the offspring. Of all the site characteristics, only longitude had a significant effect on offspring AR [ΔAIC = 5.32; = 6.62, p = 0.010] and HE [ΔAIC = 3.62; = 4.90, p = 0.027]. The ANOVAs failed to detect a relationship between any of the site characteristics and the AR, HE, or FIS of offspring or a relationships between site effects and the difference in inbreeding between parental and offspring populations (Table 3).
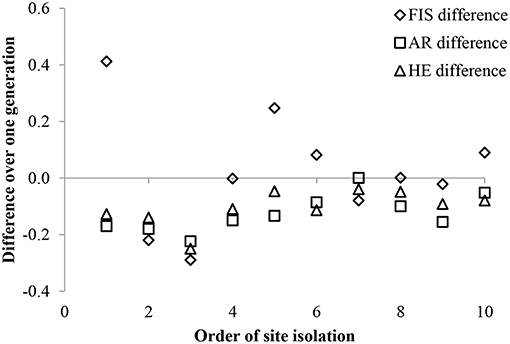
Figure 2. The difference in inbreeding (FIS) between offspring and parental generations varied by site and was not related to proximity to neighboring populations of P. hirsutus on 10 green roofs. Both average allelic richness (AR), and expected heterozygosity (HE) were lower in offspring than parental generations in all populations. Data for the populations are arranged by their relative degree of isolation compared to the other populations in the study. There were no significant linear relationships detected between the mean distance to other populations and FIS (p = 0.783), AR (p = 0.889), or HE (p = 0.443).
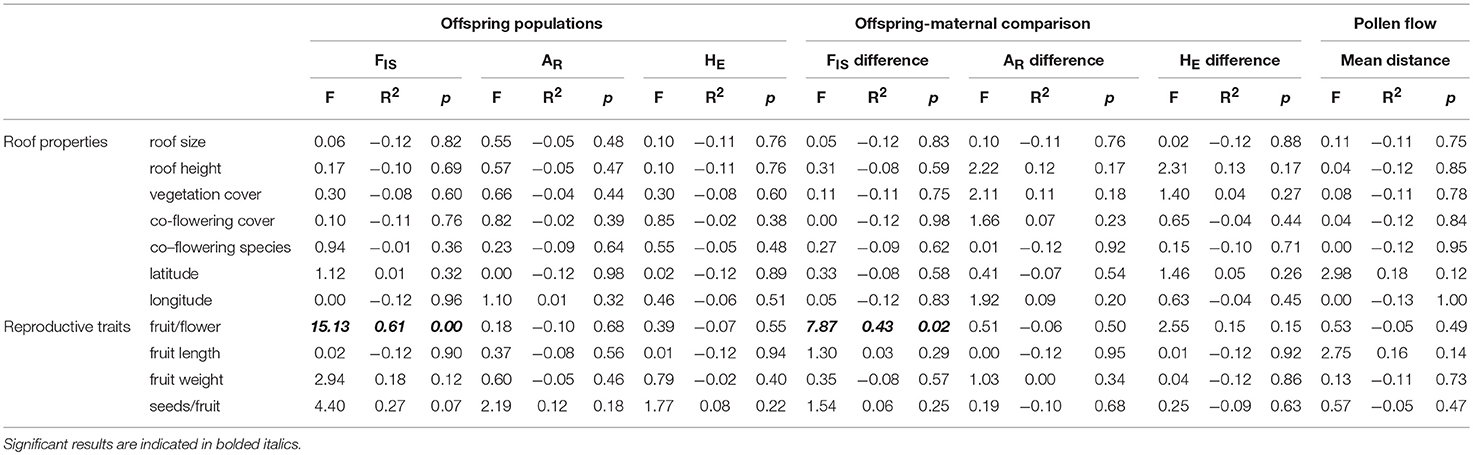
Table 3. The relationships between green roof characteristics and measured reproductive traits on the genetic diversity (FIS is inbreeding coefficient, AR is allelic richness, and HE is expected heterozygosity) of experimental populations of P. hirsutus.
We detected a significant negative relationship between rates of inbreeding and reproduction in the experimental populations (p = 0.005, R2 = 0.611, F = 15.131, 8), with higher FIS in the offspring associated with lower fruit-to-flower ratio in the parental generation (Table 3). We also found a significant negative relationship between fruit-to-flower ratio and the difference in inbreeding coefficients in the parental and offspring populations (p = 0.023, R2 = 0.433, F = 7.871, 8, Table 3, Figure 3). No other measures of reproductive output significantly differed with inbreeding coefficients of the offspring (Table 3), although we observed a trend of decreased seed set with increased rates of inbreeding (p = 0.069, R2 = 0.274, F = 4.3971, 8).
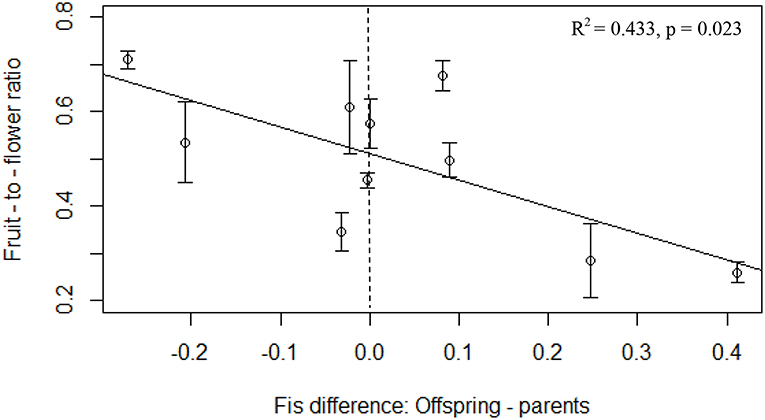
Figure 3. Fruit-to-flower ratio in the maternal P. hirsutus populations decreased with the generational difference in FIS. Populations represented by points to the right of the vertical dashed line have more inbreeding in the offspring generation than in the parental generation while those to the left of the line experienced more inbreeding in the parental than the offspring generation. Error bars depict standard error of the mean.
The conservative estimates from the paternity analysis revealed that the primary pollen source for offspring was from selfing (Figure 4). For all offspring (N = 235), 48% were likely the result of self-pollination (± 16% sd), 27% had fathers from the same roof (± 14% sd), and 25% had fathers from a different roof (± 14% sd). Of the offspring that resulted from pollen flow between roofs, the mean distance was 126.7 ± 71.3 m sd, with a maximum pollen flow distance of 351 m. According to the GLM, LME, and ANOVAs, none of the site characteristics were significant predictors of mean pollen flow distance (Table 3). Additionally, site characteristics were not correlated to the relative proportion of offspring that resulted from between-roof, within-roof, or self-pollination events.
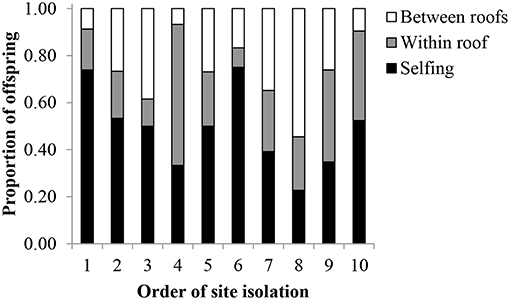
Figure 4. The proportion of P. hirsutus offspring resulting from self-pollination (“selfing,” mean = 48% ± 16 sd), within-roof-pollination (mean = 27% ± 14 sd), and between-roof-pollination (25% ± 14 sd) on 10 green roofs. Populations are ordered from least to most isolated, according to the mean distance to all other roofs included in the study.
Discussion
Our data support the hypotheses that P. hirsutus plants from nursery-stock are genetically depauperate compared to natural populations and that while between-roof gene flow occurs, plant populations on green roofs frequently experience selfing. To our knowledge, this is the first study to document gene (pollen) flow among green roof populations in urban habitats. About one-quarter of the offspring in our study resulted from pollen from a roof population an average of 127 m away. Our data suggest that gene flow between green roof populations is sufficient to avoid genetic differentiation and to overcome the negative effects of genetic drift (Mills and Allendorf, 1996; Lowe and Allendorf, 2010). Some pollinators may not be adversely affected by the urban environment and may be able to provide pollination services. Furthermore, our results are consistent with previous findings that distance is not the main factor explaining pollen movement (Ellstrand, 2014) and floral mating patterns (Ashley, 2010).
The lack of genetic diversity in the established green roof populations compared to the natural populations, together with the decreases in allelic richness and expected heterozygosity in green roof offspring compared to their parents, suggests that caution should be exercised when using commercially-propagated plant material for conservation purposes. Typically, highly diverse local plant material is necessary to best maintain genetic diversity and long-term population stability (McKay et al., 2005). On the other hand, some commercial sources of seed survive and have similar fitness levels in field conditions compared to plants from wild-collected seed (Gibbs et al., 2012). The fact that we found higher pairwise FST values between green roof populations than between natural populations suggests that urban populations established from different sources of nursery stock may have considerable genetic differentiation (Lowe and Allendorf, 2010); more so than one would find in the wild. Sufficient gene flow between urban populations may eventually lead to genetically robust metapopulations. However, long-term persistence could be impeded if increased inbreeding leads to reduced fitness (inbreeding depression) and if genetic drift leads to increased homozygosity (Booy et al., 2000; Spielman et al., 2004).
Many self-compatible insect-pollinated plant species like P. hirsutus are expected to show an increased propensity for self-pollination as a method of reproductive assurance (Johnson et al., 2015), which may be particularly relevant in urban areas that lack a diversity of pollinators. The urban environment provides unique challenges for plant and pollinator populations. As such, future studies are needed to determine the extent to which plant species rely on pollinators for reproduction, the consequences of mating events (increased inbreeding or outcrossing), how specific elements of the urban environment impact the diversity and abundance of various pollinator species, and how these factors combine to influence the likelihood of population persistence in an increasingly urbanized environment.
We found that nearly half of the offspring generation resulted from self-fertilization but also that about a quarter resulted from long-distance pollen movement. These results are consistent with expectations for small, fragmented populations within an inhospitable matrix (Hendrix and Kyhl, 2000; Trapnell and Hamrick, 2005; Evans et al., 2017). Small population sizes, such as those typically found in cities, can lead to longer and more pollinator foraging bouts within both individuals and populations, which can result in higher rates of geitonogamy and inbreeding (Severns, 2003). For example, Andrieu et al. (2009) observed fewer pollinator visits to Crepis sancta in small, urban compared to large, natural habitats but found that pollinators spent more time on each individual plant and within each urban patch. In addition, limited resources and lower densities, common features of many small populations, can promote between-population movement resulting in more long-distance pollen dispersal (Levin, 1979; Schmitt, 1983; Ison and Wagenius, 2014). The current study best supports the theory that pollinators maximize their time at individual plants but also indicates that long-distance pollen dispersal occurs between green roofs. In cities, pollinator foraging is often sufficient to maintain gene flow between fragmented patches. For example, the higher reproductive success of the forb Centaurea jacea in parks compared to semi-natural sites (Van Rossum, 2010) and the maintenance of pollen flow between urban populations of the perennial forb Viola pubescens (Culley et al., 2007) demonstrate that not only are pollinators capable of moving long distances through cities but that they might actually be suited to foraging in an urban matrix if floral and nesting resources are available.
Pollinators that move long distances have been documented to maintain population connectivity, limit inbreeding and reduce genetic differences among populations (Ehrlich and Raven, 1969; Lenormand, 2002). In urban habitats specifically, high pollinator visitation rates between patches (Parker, 1997; Verboven et al., 2014) may counteract the negative consequences of genetic drift. Although small, solitary Hoplitis and Ceratina bees have not been directly observed on green roofs in the study region to our knowledge (Tonietto et al., 2011; Ksiazek et al., 2014), P. hirsutus relies primarily on bees from these genera for pollination (Crosswhite and Crosswhite, 1966). Solitary bees of similar size are capable of foraging across the maximum observed distance between sites (351 m), although most Hoplitis and Ceratina individuals would be expected to forage <300 m from their nests in natural habitats (Gathmann and Tscharntke, 2002; Zurbuchen et al., 2010). Furthermore, foraging ranges of solitary bees may be reduced in cities compared to natural areas (Lopez-Uribe et al., 2008). Such evidence is supported by the mean distance between the outcrossed pollen donors and recipients observed in our current study (127 m). These results suggest that long-distance dispersal is occurring but the close proximity of patches is important for maintaining pollen flow between urban populations pollinated by small bees.
Although genetic diversity of a population tends to be positively correlated with overall fitness (Leimu et al., 2006), low genetic diversity itself does not necessarily impact population stability unless inbreeding depression results (Frankham, 2005). Self-compatible plant species like P. hirsutus benefit from reproductive assurance when pollinators are limited, but outcrossing with unrelated individuals can also provide the genetic material needed for populations to respond to environmental changes and persist in the long term (Nassar and Ramìrez, 2004). Many studies of both woody and herbaceous species have demonstrated increased fitness (such as greater seed size, fruit set, seedling size, survival rate, and seed production) when self-compatible individuals are outcrossed rather than self-pollinated (Kittelson and Maron, 2000; Vange, 2002; Vaughton and Ramsey, 2006; Frye and Neel, 2017). While the current study documented gene flow, we also identified lower reproductive output (reduced fruit to flower ratio) in populations that experienced high inbreeding. These results suggest either that there is inbreeding depression or pollinator limitation in P. hirsutus populations, which could lead to eventual population extinction.
While most common measures of pollinator attraction (i.e., the size of the vegetated area, and the proximity to the next nearest population) did not have a significant effect on inbreeding in this study, the large range in inbreeding coefficients that we observed between experimental offspring populations may be due to their small size and number. Although one-way analyses failed to demonstrate a significant relationship between site characteristics and inbreeding, the inverse correlation between percent cover of co-flowering species and FIS revealed by our two multi-variable analyses suggests that differences between sites may affect pollinator visitation. Alternatively, other unmeasured factors may explain the observed patterns of gene flow. The physical arrangement and architecture of buildings may have profound impacts on visitation rates if plant populations are not easily located by pollinators. For example, the two populations with the largest generational increase in inbreeding were each situated in a “courtyard” one story below the roof line so that the vegetated portion of the roof was at least four meters lower than the rest of the roof surface. To be attracted to a P. hirsutus plant at these sites, a bee would have to see the plant from directly above the population rather than from an angle and at a distance. Additionally, best-fit models predicted a correlation between longitude and AR and HE. In other words, green roof populations closest to the shore of Lake Michigan had offspring with lower genetic diversity, implying that a large landscape feature devoid of resources may have negative impacts on pollinator visitation. Such topographic and landscape-level factors are expected to lead to increased inbreeding over time; a prediction that needs to be tested more vigorously in subsequent experiments.
Our data suggest that populations of genetically-diverse native species can be created by using plants propagated from seeds collected from natural populations or by using plants from more than one nursery. Establishing plant populations with high genetic diversity has the potential to better support biodiversity far into the future (Lacy, 1987). In cities, more careful consideration of genetic diversity during ecosystem design could lead to increased urban restoration success, biodiversity support, and bolstering of long-term population persistence (Given, 1994; Bussell et al., 2006). Designing green roof habitats using targeted native plant species can, therefore, help meet the dual goals of creating self-sustaining, low maintenance green spaces, and enhancing urban conservation efforts (Ksiazek, 2014). For species that are self-compatible, inbreeding depression might be avoided by planting large populations to ensure that mating events are less likely to occur within or between closely-related individuals. In addition, establishing metapopulations, and supporting robust, and diverse pollinator communities may increase the likelihood of outcrossing within and between populations, thereby providing the genetic material needed to face future environmental change and avoid population extinction. Green roof managers can facilitate gene flow, which is particularly important for species that are self-incompatible, by providing resources for pollinators including plants that provide sufficient pollen and nectar throughout the foraging season and nesting materials (bare ground, twigs, etc.) that support resident bee populations. As modern cities continue to encroach upon natural habitat, the lines between urban and non-urban will become less defined. Managing for biodiversity, including genetic diversity, will allow all types of habitats to sustain a variety of species for generations to come.
Data Availability
Microsatellite data are deposited in the Dryad repository: doi: 10.5061/dryad.1j86179. All other data generated for this study are available upon request from the corresponding author.
Author Contributions
KK-M performed field and lab work and analyzed the data. All authors contributed to writing, editing the manuscript and to conceiving and designing the experiments.
Funding
Funding for this research was generously provided by the Botanical Society of America Graduate Student Research Award, The Graduate School at Northwestern University Presidential Fellowship, the Institute for Sustainability and Energy at Northwestern (ISEN) Student Grant, the Phipps Conservatory and Botanical Gardens Botany in Action Fellowship program, and the Program in Plant Biology and Conservation at Northwestern University and The Chicago Botanic Garden Research Award.
Conflict of Interest Statement
The authors declare that the research was conducted in the absence of any commercial or financial relationships that could be construed as a potential conflict of interest.
Acknowledgments
The authors thank Loyola University for access to green roof sites and Bala Chaudhary, Dan Larkin, Manfred Köhler, and two reviewers for comments on earlier versions of this manuscript.
Supplementary Material
The Supplementary Material for this article can be found online at: https://www.frontiersin.org/articles/10.3389/fevo.2019.00299/full#supplementary-material
Figure S1. The output from a LOCPRIOR model in STRUCTURE (burn-in of 10,000 with 10,000 iterations and K = 6) shows genetic differentiation between the natural prairie populations (LN, LS, and MD) and the green roof populations (CN, CS, and QN) used in this study.
References
Andrieu, E., Dornier, A., Rouifed, S., Schatz, B., and Cheptou, P. O. (2009). The town Crepis and the country Crepis: how does fragmentation affect a plant-pollinator interaction? Acta Oecol. 35, 1–7. doi: 10.1016/j.actao.2008.07.002
Ashley, M. V. (2010). Plant parentage, pollination, and dispersal: How DNA microsatellites have altered the landscape. Crit. Rev. Plant Sci. 29, 148–161. doi: 10.1080/07352689.2010.481167
Basey, A., Fant, J. B., and Kramer, A. T. (2015). Producing native plant materials for restoration: ten rules to maximize genetic diversity. Nat. Plants J. 16, 37–53. doi: 10.3368/npj.16.1.37
Booy, G., Hendriks, R. J. J., Smulders, M. J. M., Van Groenendael, J. M., and Vosman, B. (2000). Genetic diversity and the survival of populations. Plant Biol. 2, 379–395. doi: 10.1055/s-2000-5958
Breed, M. F., Marklund, M. H. K., Ottewell, K. M., Gardner, M. G., Harris, J. B., and Lowe, A. J. (2012). Pollen diversity matters: revealing the neglected effect of pollen diversity on fitness in fragmented landscapes. Mol. Ecol. 21, 5955–5968. doi: 10.1111/mec.12056
Breed, M. F., Ottewell, K. M., Gardner, M. G., and Lowe, A. J. (2011). Clarifying climate change adaptation responses for scattered trees in modified landscapes. J. Appl. Ecol. 48, 637–641. doi: 10.1111/j.1365-2664.2011.01969.x
Breed, M. F., Ottwell, K. M., Gardner, M. G., Marklund, M. H., Dormontt, E. E., and Lowe, A. J. (2015). Mating patterns and pollinator mobility are critical traits in forest fragmentation genetics. Heredity 115, 108–114. doi: 10.1038/hdy.2013.48
Bussell, J. D., Hood, P., Alacs, E. A., Dixon, K. W., Hobbs, R. J., and Krauss, S. L. (2006). Rapid genetic delineation of local provenance seed-collection zones for effective rehabilitation of an urban bushland remnant. Aust. Ecol. 31,164–175. doi: 10.1111/j.1442-9993.2006.01576.x
Clements, R. K. (1995). Studies on the Taxonomy and General Ecology of the Two Closely-Related Species Penstemon Tenuiflorus Pennel and P. hirsutus, (L.). Willd., (Scrophulariaceae section Graciles). Doctoral Dissertation. Lexington, KY: University of Kentucky.
Clements, R. K., Baskin, J. M., and Baskin, C. C. (1998). The comparative biology of the two closely-related species Penstemon tenuiflorus Pennell and P. hirsutus, (L.). Willd., (Scrophulariaceae, Section Graciles): Taxonomy, I., and geographical distribution. Castanea 63, 138–153.
Clements, R. K., Baskin, J. M., and Baskin, C. C. (1999). The comparative biology of the two closely-related species Penstemon tenuiflorus Pennell and P. hirsutus, (L.). Willd., (Scrophulariaceae, Section Graciles): II. Reproductive biology. Castanea 64, 299–309.
Colla, S. R., Willis, E., and Packer, L. (2009). Can green roofs provide habitat for urban bees, (Hymenoptera: Apidae)? Cities Environ. 2:4. doi: 10.15365/cate.2142009
Crosswhite, F. S., and Crosswhite, C. D. (1966). Insect pollinators of Penstemon series Graciles, (Scrophulariaceae). with notes on Osmia and other Megachilidae. Am. Midland Natural. 76, 450–467. doi: 10.2307/2423097
Culley, T. M., Sbita, S. J., and Wick, A. (2007). Population genetic effects of urban habitat fragmentation in the perennial herb Viola pubescens, (Violaceae). using ISSR markers. Ann. Bot. 100, 91–100. doi: 10.1093/aob/mcm077
Dockter, R. B., Elzinga, D. B., Geary, B., Maughan, P. J., Johnson, L. A., Tumbleson, D., et al. (2013). Developing molecular tools and insights into the Penstemon genome using genomic reduction and next-generation sequencing. BioMed Cent. Genet. 14:66. doi: 10.1186/1471-2156-14-66
Doyle, J. J., and Doyle, J. L. (1987). A rapid DNA isolation procedure for small quantities of fresh leaf tissue. Phytochem. Bull. 19, 11–15.
Dubois, J., and Cheptou, P. O. (2017). Effects of fragmentation on plant adaptation to urban environments. Philos. Trans. R. Soc. B 372:20160038. doi: 10.1098/rstb.2016.0038
Dunn, C. P., and Heneghan, L. (2011). “Composition and diversity of urban vegetation,” in Urban Ecology, ed J. Niemelä (New York, NY: Oxford University Press), 103–115. doi: 10.1093/acprof:oso/9780199563562.003.0013
Ehrlich, P. R., and Raven, P. H. (1969). Differentiation of populations. Science 165, 1228–1232. doi: 10.1126/science.165.3899.1228
Ellstrand, N. C. (2014). Is gene flow the most important evolutionary force in plants? Am. J. Bot. 101, 737–753. doi: 10.3732/ajb.1400024
Evans, T. M., Cavers, S., Ennos, R., Vanbergen, A. J., and Heard, M. S. (2017). Florally rich habitats reduce insect pollination and the reproductive success of isolated plants. Ecol. Evol. 7, 6507–6518. doi: 10.1002/ece3.3186
Fant, J. B., Holmstrom, R. M. R., Sirkin, E., Etterson, J. R., and Masi, S. (2008). Genetic structure of threatened native populations and propagules used for restoration in a clonal species, american beachgrass, (Ammophila breviligulata Fern.). Restorat. Ecol. 16, 594–603. doi: 10.1111/j.1526-100X.2007.00348.x
Frankham, R. (2005). Genetics and extinction. Biol. Conserv. 126, 131–140. doi: 10.1016/j.biocon.2005.05.002
Frankham, R., Ballou, J. D., and Briscoe, D. A. (2002). Introduction to Conservation Genetics. Cambridge: Cambridge University Press.
Frye, C. T., and Neel, M. C. (2017). Benefits of gene flow are mediated by individual variability in self-compatibility in small isolated populations of an endemic plant species. Evol. Appl. 10, 551–562. doi: 10.1111/eva.12437
Gathmann, A., and Tscharntke, T. (2002). Foraging ranges of solitary bees. J. Anim. Ecol. 71, 757–764. doi: 10.1046/j.1365-2656.2002.00641.x
Gibbs, J. P., Smart, L. B., Newhouse, A. E., and Leopold, D. J. (2012). A molecular and fitness evaluation of commercially available versus locally collected blue lupine Lupinus perennis L. seeds for use in ecosystem restoration efforts. Restorat. Ecol. 20, 456–461. doi: 10.1111/j.1526-100X.2011.00809.x
Goddard, M. A., Dougill, A. J., and Benton, T. G. (2009). Scaling up from gardens: biodiversity conservation in urban environments. Trends Ecol. Evol. 25, 90–98. doi: 10.1016/j.tree.2009.07.016
Goudet, J. (2001). FSTAT, a Program to Estimate and Test Gene Diversities and Fixation Indices, (version 2.9.3). Available online at: http://www2.unil.ch/popgen/softwares/fstat.htm (accessed May 1, 2017).
Hale, M. L., Burg, T. M., and Steeves, T. E. (2012). Sampling for microsatellite-based population genetic studies: 25 to 30 individuals per population is enough to accurately estimate allele frequencies. PLoS ONE 7:e45170. doi: 10.1371/journal.pone.0045170
Handel, S. N., Saito, O., and Takeuchi, K. (2013). “Restoration ecology in an urbanizing world,” in Urbanization, Biodiversity and Ecosystem Services: Challenges and Opportunities, eds T. Elmqvist, M. Fragkias, J. Goodness, B. Güneralp, P. J. Marcotullio, R. I. McDonald, S. Parnell, M. Schewenius, M. Sendstad, K. C. Seto, and C. Wilkinson (Dordrecht: Springer), 665–698. doi: 10.1007/978-94-007-7088-1_31
Hejkal, J., Buttschart, T. K., and Klaus, V. H. (2017). Connectivity of public urban grasslands: implications for grassland conservation and restoration in cities. Urban Ecosyst. 20, 511–519. doi: 10.1007/s11252-016-0611-8
Hendrick, P. W., and Garcia-Dorado, A. (2016). Understanding inbreeding depression, purging, and genetic rescue. Trends Ecol. Evol. 31, 940–952. doi: 10.1016/j.tree.2016.09.005
Hendrix, S. D., and Kyhl, J. F. (2000). Population size and reproduction in Phlox pilosa. Conserv. Biol. 14, 304–313. doi: 10.1046/j.1523-1739.2000.98253.x
iNaturalist.org (2019). iNaturalist Research-Grade Observations. Occurrence dataset https://doi.org/10.15468/ab3s5x via GBIF.org (accessed July 3, 2019).
Ison, J. L., and Wagenius, S. (2014). Both flowering time and distance to conspecific plants affect reproduction in Echinacea angustifolia, a common prairie perennial. J. Ecol. 102, 920–929. doi: 10.1111/1365-2745.12262
Ives, C. D., Lentini, P. E., Therlfall, C. G., Ikin, K., Shanahan, D. F., Garrard, G. E., et al. (2016). Cities are hotspots for threatened species. Glob. Ecol. Biogeogr. 25, 117–126. doi: 10.1111/geb.12404
Johnson, M. T., Thompson, K. A., and Saini, H. S. (2015). Plant evolution in the urban jungle. Am. J. Bot. 102, 1951–1953. doi: 10.3732/ajb.1500386
Kalisz, S., Vogler, D. W., and Hanley, K. M. (2004). Context-dependent autonomous self-fertilization yields reproductive assurance and mixed mating. Nature 430, 884–887. doi: 10.1038/nature02776
Keller, L. F., and Waller, D. M. (2002). Inbreeding effects in wild populations. Trends Ecol. Evol. 17, 230–241. doi: 10.1016/S0169-5347(02)02489-8
Kittelson, P. M., and Maron, J. L. (2000). Outcrossing rate and inbreeding depression in the perennial yellow bush lupine, Lupinus arboreus, (Fabaceae). Am. J. Bot. 87, 652–660. doi: 10.2307/2656851
Kowarik, I. (2011). Novel urban ecosystems, biodiversity, and conservation. Environ. Pollut. 159, 1974–1983. doi: 10.1016/j.envpol.2011.02.022
Kramer, A. T., and Fant, J. B. (2007). Isolation and characterization of microsatellite loci in Penstemon rostiflorus, (Plantaginaceae). and cross-species amplification. Mol. Ecol. Notes 7, 998–1001. doi: 10.1111/j.1471-8286.2007.01754.x
Ksiazek, K. (2014). “Chapter 6: The potential of green roofs to support urban biodiversity,” in Green Cities in the World, eds J. Briz, M. Köhler, and I. de Felipe (Madrid: Pronatur, 101–124.
Ksiazek, K., Fant, J., and Skogen, K. (2012). An assessment of pollen limitation on Chicago green roofs. Landsc. Urban Plann. 107, 401–408. doi: 10.1016/j.landurbplan.2012.07.008
Ksiazek, K., Tonietto, R., and Ascher, J. S. (2014). Ten bee species new to green roofs in the Chicago area. Great Lakes Entomol. 47, 87–92. Available online at: https://scholar.valpo.edu/tgle/vol47/iss1/13/
La Point, S., Balkenhol, N., Hale, J., et al. (2015). Ecological connectivity research in urban areas. Funct. Ecol. 29, 868–878. doi: 10.1111/1365-2435.12489
Lacy, R. (1987). Loss of genetic diversity from managed populations: Interacting effects of drift, mutation, immigration, selection, and population subdivision. Conserv. Biol. 1, 143–158. doi: 10.1111/j.1523-1739.1987.tb00023.x
Leimu, R., Mutikainen, P., Koricheva, J., and Fischer, M. (2006). How general are positive relationships between plant population size, fitness, and genetic variation? J. Ecol. 94,942–952. doi: 10.1111/j.1365-2745.2006.01150.x
Lenormand, T. (2002). Gene flow and the limits to natural selection. Trends Ecol. Evol. 17, 183–189. doi: 10.1016/S0169-5347(02)02497-7
Levin, D. A. (1979). The nature of plant species. Science 204, 381–384. doi: 10.1126/science.204.4391.381
Lopez-Uribe, M. M., Oi, C. A., and Del Lama, M. A. (2008). Nectar-foraging behavior of Euglossine bees, (Hymenoptera: Apidae). in urban areas. Apidologie 39, 410–418. doi: 10.1051/apido:2008023
Lowe, W. H., and Allendorf, F. W. (2010). What can genetics tell us about population connectivity? Mol. Ecol. 19, 3038–3051. doi: 10.1111/j.1365-294X.2010.04688.x
Lowenstein, D. M., Matteson, K. C., Xiao, I., Silva, A. M., and Minor, E. S. (2014). Humans, bees, and pollination services in the city: the case of Chicago, IL, (USA). Biodiver. Conserv. 23, 2857–2874. doi: 10.1007/s10531-014-0752-0
Lundholm, J. T. (2015). The ecology and evolution of constructed ecosystems as green infrastructure. Front. Ecol. Evol. 3:106. doi: 10.3389/fevo.2015.00106
MacIvor, J. S., and Lundholm, J. (2011). Insect species composition and diversity on intensive green roofs and adjacent level-ground habitats. Urban Ecosyst. 14, 225–241. doi: 10.1007/s11252-010-0149-0
Marshall, T. C., Slate, J., Kruuk, L. E. B., and Pemberton, J. M. (1998). Statistical confidence for likelihood-based paternity inference in natural populations. Mol. Ecol. 7, 639–655. doi: 10.1046/j.1365-294x.1998.00374.x
McKay, J. K., Christian, C. E., Harrison, S., and Rice, K. J. (2005). “How local is local?” – A review of practical and conceptual issues in the genetics of restoration. Rest. Ecol. 13, 432–440. doi: 10.1111/j.1526-100X.2005.00058.x
Miller, J. R., and Hobbs, R. J. (2002). Conservation where people live and work. Conserv. Biol. 16, 330–337. doi: 10.1046/j.1523-1739.2002.00420.x
Mills, L. S., and Allendorf, F. W. (1996). One-migrant-per-generation rule in conservation and management. Conserv. Biol. 10, 1509–1518. doi: 10.1046/j.1523-1739.1996.10061509.x
Müller, N., Ignatieva, M., Nilon, C. H., Werner, P., and Zipperer, W. C. (2013). “Patterns and trends in urban biodiversity and landscape design,” in Urbanization, Biodiversity and Ecosystem Services: Challenges and Opportunities, eds T. Elmqvist, M. Fragkias, J. Goodness, B. Güneralp, P. J. Marcotullio, R. I. McDonald, S. Parnell, M. Schewenius, M. Sendstad, K. C. Seto, and C. Wilkinson (Dordrecht: Springer), 123–174. doi: 10.1007/978-94-007-7088-1_10
Nassar, J. M., and Ramìrez, N. (2004). Reproductive biology of the melon cactus, Melocactus curvispinus, (Cactaceae). Plant Syst. Evol. 248, 31–44. doi: 10.1007/s00606-004-0193-4
Parker, I. M. (1997). Pollinator limitation of Cytisus scoparius, (Scotch broom), an invasive exotic shrub. Ecology 78, 1457–1470. doi: 10.1890/0012-9658(1997)078[1457:PLOCSS]2.0.CO;2
Peakall, R., and Smouse, P. E. (2006). GENALEX 6: genetic analysis in Excel. Population genetic software for teaching and research. Mol. Ecol. Notes 6, 288–295. doi: 10.1111/j.1471-8286.2005.01155.x
Peakall, R., and Smouse, P. E. (2012). GenAlEx 6.5: genetic analysis in Excel. Population genetic software for teaching and research – an update. Bioinformatics 28, 2537–2539. doi: 10.1093/bioinformatics/bts460
Putman, A. I., and Carbone, I. (2014). Challenges in analysis and interpretation of microsatellite data for population genetic studies. Ecol. Evol. 4, 4399–4428. doi: 10.1002/ece3.1305
R Development Core Team. (2016). R: A Language and Environment for Statistical Computing. Vienna: R Foundation for Statistical Computing.
Reed, D. H., and Frankham, R. (2003). Correlation between fitness and genetic diversity. Conserv. Biol. 17, 230–237. doi: 10.1046/j.1523-1739.2003.01236.x
Schmitt, J. (1983). Density-dependent pollinator foraging, flowering phenology, and temporal pollen dispersal patterns in Linanthus bicolor.” Evolution 37, 1247–1257. doi: 10.1111/j.1558-5646.1983.tb00241.x
Severns, P. (2003). Inbreeding and small population size reduce seed set in a threatened and fragmented plant species, Lupinus sulphureus ssp. Kincaidii, (Fabaceae). Biol. Conserv. 110, 221–229. doi: 10.1016/S0006-3207(02)00191-X
Spielman, D., Brook, B. W., and Frankham, R. (2004). Most species are not driven to extinction before genetic factors impact them. PNAS 101, 15261–15264. doi: 10.1073/pnas.0403809101
Sutton, R. K., Harrington, J. A., Skabelund, L., and MacDonagh, L. P. (2012). Prairie-based green roofs: Literature, templates, and analogs. J. Green Build. 7, 143–172. doi: 10.3992/jgb.7.1.143
Tonietto, R. K., Fant, J., Ascher, J., Ellis, K., and Larkin, D. (2011). A comparison of bee communities of Chicago green roofs, parks and prairies. Lands. Urban Plann.103, 102–108. doi: 10.1016/j.landurbplan.2011.07.004
Trapnell, D. W., and Hamrick, J. L. (2005). Mating patterns and gene flow in the neotropical epiphytic orchid, Laelia rubescens. Mol. Ecol. 14, 75–84. doi: 10.1111/j.1365-294X.2004.02383.x
USDA, NRCS. (2006). The PLANTS Database, 6 March 2006. Available online at: http://www.plants.usda.gov. Baton Rouge, LA: National Plant Data Center. (accessed December 18, 2014 and June 18, 2019).
Van Oosterhout, C., Hutchinson, W. F., and Wills, D. P. M. (2004). MICRO-CHECKER: software for identifying and correcting genotyping errors in microsatellite data. Mol. Ecol. Res. 4, 535–538. doi: 10.1111/j.1471-8286.2004.00684.x
Van Rossum, F. (2009). Pollen dispersal and genetic variation in an early-successional forest herb in a peri-urban forest. Plant Biol. 11, 725–737. doi: 10.1111/j.1438-8677.2008.00176.x
Van Rossum, F. (2010). Reproductive success and pollen dispersal in urban populations of an insect-pollinated hay-meadow herb. Perspect. Plant Ecol. Evol. Syst. 12, 21–29. doi: 10.1016/j.ppees.2009.08.002
Van Rossum, F., and Triest, L. (2012). Stepping-stone populations in linear landscape elements increase pollen dispersal between urban forest fragments. Plant Ecol. Evol. 145, 332–340. doi: 10.5091/plecevo.2012.737
Vange, V. (2002). Breeding system and inbreeding depression in the clonal plant species Knautia arvensis, (Dipsacaceae): implications for survival in abandoned grasslands. Biol. Conserv. 108, 59–67. doi: 10.1016/S0006-3207(02)00090-3
Vaughton, G., and Ramsey, M. (2006). Selfed seed set and inbreeding depression in obligate seed populations of Banksia marginata. Proc. Linnean Soc. New South Wales 127, 19–25. Available online at: https://search.informit.com.au/documentSummary;dn=044042065077416;res=IELHSS
Verboven, H. A. F., Aertsen, W., Brys, R., and Hermy, M. (2014). Pollination and seed set of an obligatory outcrossing plant in an urban-peri-urban gradient. Perspect. Plant Ecol. Evol. Syst. 16, 121–131. doi: 10.1016/j.ppees.2014.03.002
Whiteley, A. R., Fitzpatrick, S. W., Funk, W. C., and Tallmon, D. A. (2015). Genetic rescue to the rescue. Trends Ecol. Evol. 30, 42–49. doi: 10.1016/j.tree.2014.10.009
Wilhelm, G., and Rericha, L. (2017). Flora of the Chicago Region: A Floristic and Ecological Synthesis. Indianapolis: Indiana Academy of Science.
Williams, N. S. G., Morgan, J. W., McDonnell, M. J., and McCarthy, M. A. (2005). Plant traits and local extinctions in natural grasslands along an urban-rural gradient. J. Ecol. 93, 1203–1213. doi: 10.1111/j.1365-2745.2005.01039.x
Wright, S. (1932). “The roles of mutation, inbreeding, crossbreeding, and selection in evolution,” in Proceedings of the Sixth International Congress of Genetics (Chicago), 356–366.
Keywords: genetic diversity, green infrastructure, metapopulations, inbreeding, pollen flow, urban biodiversity
Citation: Ksiazek-Mikenas K, Fant JB and Skogen KA (2019) Pollinator-Mediated Gene Flow Connects Green Roof Populations Across the Urban Matrix: A Paternity Analysis of the Self-Compatible Forb Penstemon hirsutus. Front. Ecol. Evol. 7:299. doi: 10.3389/fevo.2019.00299
Received: 11 May 2019; Accepted: 24 July 2019;
Published: 07 August 2019.
Edited by:
Jeremy Lundholm, Saint Mary's University, CanadaReviewed by:
Anna L. Johnson, University of Maryland, Baltimore County, United StatesLillian Ruth Rivkin, University of Toronto, Canada
Copyright © 2019 Ksiazek-Mikenas, Fant and Skogen. This is an open-access article distributed under the terms of the Creative Commons Attribution License (CC BY). The use, distribution or reproduction in other forums is permitted, provided the original author(s) and the copyright owner(s) are credited and that the original publication in this journal is cited, in accordance with accepted academic practice. No use, distribution or reproduction is permitted which does not comply with these terms.
*Correspondence: Kelly Ksiazek-Mikenas, kelly.mikenas@elmhurst.edu