- 1Department of Biology, Elon University, Elon, NC, United States
- 2Division of Biological Sciences, University of Missouri, Columbia, MO, United States
Unintended receivers can be an important source of selection on social signals. Vibrational social signals are produced by diverse taxa, but most work on eavesdropping on social communication has focused on airborne signals. Few studies have examined whether predators and parasitoids exploit vibrational social signals, and whether vibrational communication systems have features to reduce apparency to unintended receivers. For a subsocial insect species (Hemiptera: Membracidae: Platycotis vittata), we first used a field playback experiment to show that offspring vibrational signals evoke maternal defense, and that maternal signals can inhibit offspring signaling. We next evaluated two potential benefits of inhibiting offspring signaling. We tested whether such inhibition increases the accuracy of offspring signals, as it does in a closely related species. We also tested whether by inhibiting offspring signals, mothers reduce the risk of attracting eavesdropping predators. Using playback experiments, we found that a vibrationally-sensitive predator attends to offspring but not maternal signals. In contrast, we found no evidence that inhibition increases the accuracy of offspring signals. Because predator eavesdropping is a likely cost of social communication for vibrationally signaling animals, we suggest that mechanisms to reduce apparency of such social signals may be common.
Introduction
Predators and parasitoids eavesdrop on airborne mate advertisement and courtship signals to locate prey and hosts (reviewed in Zuk and Kolluru, 1998; Haynes and Yeargan, 1999), and can act as powerful agents of selection, even driving the evolutionary loss of signaling behavior (Zuk et al., 2006). For animals communicating with substrate vibrations, however, there has been controversy about the potential for predator eavesdropping. Henry (1994) suggested that vibrational communication is essentially a private channel. In contrast, Morris et al. (1994) and (Römer et al., 2010) note that when rainforest katydids switch from airborne to substrate-borne signaling, they avoid eavesdropping bats but remain detectable by nearby spiders. Other authors have argued that substrate vibration is likely to be among the most vulnerable of modalities to unintended receivers, given the wide array of vibrationally-sensitive taxa (Cocroft and Rodríguez, 2005; Cocroft, 2011; Virant-Doberlet et al., 2019). Although Zuk and Kolluru's (1998) review of predator eavesdropping listed no examples from the vibrational modality, more recent studies provide evidence of the importance of unintended receivers in vibrational interactions. To date, however, such evidence is restricted to predator eavesdropping on mate advertisement signals (Narhardiyati and Bailey, 2005; Roberts et al., 2007; Virant-Doberlet et al., 2011) and eavesdropping on incidental vibrational cues by predators (Pfannenstiel et al., 1995; Barth, 1998; Meyhöfer and Casas, 1999) and competitors (Evans et al., 2009).
It is not known if predators eavesdrop on vibrational social signals, as they do on social signals in the other signaling modalities. For example, for airborne acoustic communication, such eavesdropping occurs on aggregating signals (reviewed in Haynes and Yeargan, 1999), offspring begging signals (reviewed in Magrath et al., 2010), alarm signals (Allan et al., 1996), and mobbing signals (Krams et al., 2007). This is unsurprising, as when multiple individuals signal in close proximity, they should provide an amplified and persistent source of information to unintended receivers. However, to our knowledge, there are no examples of predator eavesdropping on social vibrational signals. Yet many invertebrates are group-living for at least one life stage (Costa, 2006), and vibrational communication is widespread in group-living insects (reviewed in Cocroft and Hamel, 2010).
Because eavesdropping predators and parasites can act as strong agents of selection and impose costs on signalers, we should expect to see evidence of such selection in group-living, vibrationally-signaling species. For example, selection might result in strategies to mitigate costs while preserving signal function, such as reducing the apparency of signals to unintended receivers and increasing receiver sensitivity. Here, we test the hypothesis that for an insect species in which mothers and offspring use vibrational communication, maternal signals function to reduce the apparency of their offspring to eavesdropping predators by inhibiting offspring signaling.
In oak treehoppers (Hemiptera: Membracidae: Platycotis vittata), mothers and clustered offspring communicate with one another during predator encounters via substrate vibrations. A family typically consists of 40 to 50 sedentary offspring that live on the new growth tip of an oak branch with their mother (Wood, 1976). Oak treehopper family groups often develop in the presence of invertebrate predators on the same host tree, and even the same branch (Figure 1), and all known invertebrate predators of oak treehoppers are vibrationally-sensitive (Hamel, 2011). Predation is likely a strong source of offspring mortality: in one population, all offspring were lost from 45% of families, and invertebrate predators were commonly seen on the same trees as treehopper families (Hamel, 2011). We have observed attacks by invertebrate predators that resulted in 100% mortality.
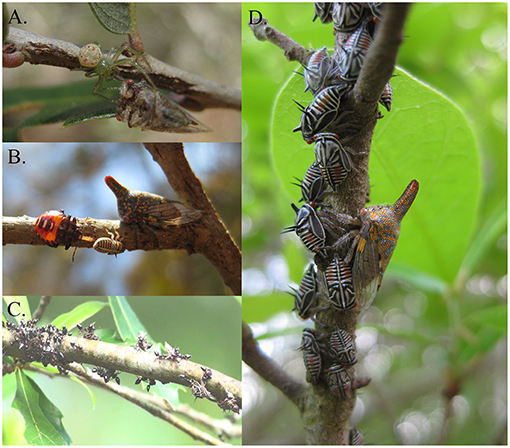
Figure 1. Vibrationally-sensitive insect predators of oak treehoppers (A–C) and an oak treehopper family (D). (A) Crab spider carrying oak treehopper mother; egg clutch is visible on the branch, underneath the spider. (B) Predatory stink bug nymph (introduced by author) feeding on oak treehopper nymph. (C) Arboreal ants (Crematogaster ashmeadii) preying upon oak treehopper nymphs. (D) Oak treehopper mother and late instar nymphs.
Predator attacks can be prolonged, and offspring groups produce synchronized vibrational signals throughout such attacks (Hamel, 2011). Mothers defend their offspring from predators (Beamer, 1930), and in response to offspring signals, a mother searches for the predator and produces her own vibrational signals (this study). After predator encounters end, mothers produce semi-continuous trains of signals for several minutes, and offspring generally cease signaling.
Here, we first show that offspring collective signals evoke maternal antipredator behavior (Experiment 1), and that vibrational signals by oak treehopper mothers reduce signaling by offspring groups during simulated predator attacks (Experiment 2). We then evaluate two hypotheses to explain why mothers reduce signaling by offspring groups. First, maternal signals may inhibit continued signaling by offspring after attacks end and thereby increase the accuracy with which offspring signals indicate predator presence. Juveniles in closely related thornbug treehoppers (Umbonia crassicornis) evoke maternal defense with group vibrational signals (Cocroft, 1996), and often continue signaling after attacks end (Cocroft, 1999a), producing false alarms unless they are inhibited by maternal signals (Hamel and Cocroft, 2012). This hypothesis predicts that maternal signals should reduce offspring signaling when a predator cue is removed. To test this hypothesis, we elicited signaling by offspring groups with simulated predator attacks, removed the predator cue, and then measured continued offspring signaling response as we played maternal signals, silence, or a common source of environmental noise (Experiment 3).
We also tested a second hypothesis, that mothers signal to decrease the risk of attracting eavesdropping predators (Experiments 4 and 5). We tested this hypothesis by measuring the responses of one generalist, vibrationally-sensitive predator to offspring and maternal signals. A predatory stinkbug (Hemiptera: Pentatomidae: Podisus maculiventris) is an appropriate focal predator because individuals of this species have been observed preying upon oak treehopper juveniles in the field (Mark Rothschild, pers. comm.), and because it uses incidental vibrations to locate caterpillar prey (Pfannenstiel et al., 1995).
Finally, because preliminary observations suggested that offspring and maternal signals differ temporally and spectrally, we characterized maternal and offspring signals recorded during this work.
Methods
Experimental Settings
We tested the effects of offspring signals on maternal behavior and of maternal signals on offspring behavior with field experiments (Experiments 1, 2, and 3) in 2009 and 2010, at the University of Florida Ordway-Swisher Biological Station (Putnam Co., FL). These three experiments were conducted with a naturally occurring field population of oak treehoppers, and each treehopper family was only used in a single experiment. We located female oak treehoppers on eggs by scanning branches of several oak species (Quercus spp.), and we covered each experimental family with a mesh sleeve to prevent predation. All nymphal aggregations tested were between the 2nd and 4th nymphal stages; oak treehoppers develop through a total of five nymphal stages. We tested predator responses to offspring and maternal signals in the laboratory (Experiments 4 and 5) at the University of Missouri.
Detection and Playback of Vibrational Signals
We detected treehopper vibrational signals with an accelerometer (PCB Piezotronics, NY, USA; Model 352A24, weight 0.8 g, frequency range: 0.8 Hz to 10 kHz ± 10% or Vibra-Metrics, NJ, USA; Model 9002A, weight 0.8 g, frequency range: 8 Hz to 18 kHz ± 10%) affixed to the branch < 10 cm from each family using mounting wax and powered by a signal conditioner and power supply (PCB Model 480E09 or Vibra-Metrics Model P5000). We recorded playbacks and signaling responses using a Marantz PMD660 digital audio recorder with a sampling rate of 44,100 Hz. To ensure that playbacks closely matched the frequency and amplitude spectra of original recordings (Figure S1), we matched the relative positions of transducer and signal source between each respective stimulus recording and playback. Background noise in vibrational stimuli was minimized, as we recorded stimuli during periods without vibrational noise from environmental factors (e.g., rain and wind), except when the stimuli in question were wind-induced vibrations (see Experiments 4 and 5). In a few stimulus recordings, when unavoidable background noise was present on a stimulus recording but did not overlap with the stimulus, we replaced the background noise with silence of the same duration using Audacity (v. 1.3.13).
For playbacks, we first set up vibration recording and video equipment and allowed each treehopper family 1 h to acclimate. We recorded treehopper and predator behavior using a digital video recorder (Sony Handycam Models HDR-HC7 and HDR-SR11). We scored video of behavioral responses using QuickTime Player (v. 7) and vibrational signaling responses using Audacity (v. 1.3.13). Because substrate-borne vibrations from abiotic factors such as wind can influence treehopper signaling (McNett et al., 2010), we conducted field playback experiments very early in the mornings, when wind is infrequent and occurs at low intensity, and these experiments were situated at a site with minimal anthropogenic noise.
To play vibrational stimuli, we glued a small neodymium magnet (United Nuclear Scientific, Laingsburg, MI) to a branch. We positioned an electromagnet 1 to 2 mm from the magnet so that faces were parallel (Rodríguez et al., 2006). We then played stimuli to the electromagnet from Audacity v.1.3.12 on a MacBook 2.4 GHz Intel Core Duo via a RadioShack 40-watt PA amplifier. To ensure that playback signals had the correct amplitude spectrum, we used a custom program in MatLab v.R2008bSV to assess frequency filtering by the branch and build an inverse filter (Cocroft et al., 2014). We used this inverse filter to filter signals being played through each branch. To ensure we played stimuli at biologically relevant amplitudes, we matched playback peak acceleration to signal peak acceleration from the original field recording. We generated silence for control treatments in audio editing and recording software (Audacity v.1.3.12).
Experiment 1: Do Offspring Vibrational Signals Communicate Increased Risk to Mothers?
To test whether offspring signals evoke maternal antipredator behavior and describe maternal responses, we played offspring vibrational signals to nine oak treehopper families on separate trees (Supplementary Video 1), with each family consisting of a single mother and her offspring. Each family was played its own offspring vibrational signals. As a baseline for comparison and to control for effects of observer presence, equipment, and any electrical noise generated by equipment during trials, we also played silence to each family. We controlled for possible carryover effects by alternating the treatment order between families and by waiting 30 min between playback treatments.
Based on preliminary observations, we expected mothers to walk and signal in response to offspring signals. We scored maternal signals using Audacity (v. 1.3.13 beta), and the proportion of time each mother walked in QuickTime Player (v. 7). We compared responses to playbacks of offspring signals against those produced during silence with the Wilcoxon Signed-Rank test; all comparisons were two-tailed. Because we scored both walking and signaling by mothers to test whether offspring signals elicited maternal defense, we adjusted comparison P-values for false discovery rate (FDR) (Benjamini and Hochberg, 1995).
Experiment 2: Do Maternal Vibrational Signals Reduce Offspring Signaling?
To test whether maternal signals reduce offspring signaling, we removed the mother from each of 28 oak treehopper families, each of which was located on a separate tree. We then simulated predation to elicit signaling by each offspring aggregation, played either the mother's signals (one of the two maternal signal types, see below) or silence, and recorded offspring signals. To obtain vibrational playback stimuli, we recorded each focal mother's signals by simulating predation as described below 1 day before testing each family. When offspring began signaling, the mother patrolled the family, signaled, searched for the source of disturbance, and found the predator cue, which she kicked as she would a predator. As soon as the mother kicked the predator cue, we withdrew it from the aggregation, simulating a predator eviction. Each mother then returned to her typical position at rest at the trunk end of the aggregation and produced steady bouts of signals. Mothers produced short syllables during the early stages of a simulated attack, and long syllables after locating and evicting the simulated predator. We therefore used two vibrational stimulus treatments in this experiment: one with short maternal syllables (hereafter, “early encounter signals”), and one with semi-continuous trains of long and short syllables (hereafter, “post search signals”).
We returned to each family the following day, removed the mother, set up vibration recording and video equipment, and allowed the family 1 h to acclimate. We then simulated a predator encounter with only the offspring aggregation, by presenting a predator cue and simultaneously playing vibrational stimuli or silence from the mother's resting position. Our predator cue was a chemical cue from a crushed treehopper nymph (Nault et al., 1974) which reliably elicits offspring signaling (J.H., pers. obs.). We sacrificed nymphs from oak treehopper families not included in an experiment and held them on a stainless steel probe ~ 1 cm under the center of each aggregation; a different crushed nymph was presented under the nymphal aggregation for the 10 min duration of each simulated attack. Nymphs were humanely euthanized (frozen) before being crushed, and we rinsed the probe with 70% ethanol after each presentation. Mothers were kept in plastic vials during these simulations and returned to their offspring after recording was completed. Because there were three treatments (early encounter signals, post search signals, and silence), we controlled for treatment order effects by randomly assigning families to one of the six possible treatment sequences, and by waiting 1 h between treatments.
We scored offspring group signaling rates in response to each playback treatment. Because we had a larger sample size for this experiment than for Experiment 1, we assessed the effect of early encounter and post-search maternal signaling on offspring signaling with a generalized linear mixed model (GLMM) (package glmmadmb, http://glmmadmb.r-forge.r-project.org/) fitted to the negative binomial error distribution. By using a mixed-effects model, we accounted for experimental design parameters and environmental factors that might have influenced offspring signaling response. The negative binomial was an appropriate distribution choice as the data were counts (i.e., number of offspring group signals per 10 min trial), and this distribution fit the error distribution of the response variable. As fixed effects, we included playback treatment, carryover effects, treatment sequence, temperature, energy from wind-induced vibrations, and interactions between temperature and treatment and temperature and carryover effects. We included family nested within treatment sequence as a random term. We set contrasts in the model to compare responses to playbacks against those from the silence treatment.
Because oak treehopper vibrational signals have not been previously characterized, we took frequency (peak frequency and 90% bandwidth) and temporal (90% duration) measures of maternal and offspring vibrational signals produced during the stimulus recordings for this experiment. Signal analysis was conducted in Raven Pro 1.5, and statistical analyses were conducted with R statistical software, version 2.13.0.
Experiment 3: Do Maternal Vibrational Signals Reduce False Alarms by Offspring?
To assess whether maternal signals reduce group signals in the absence of a predator, we evoked offspring signals from 10 oak treehopper families as described in Experiment 2, but here we withdrew the predator cue after eliciting 10 group signals, rather than leaving the cue in place, as in Experiment 2. As we removed the predator cue, we began playing vibrational stimuli (i.e., maternal signals, silence, or wind-induced vibrations) and recording offspring response.
As in Experiment 2, we first obtained a recording of each mother's signals. For playbacks, our treatments were maternal vibrational signals (post-search), wind vibrations, or silence. Each family received all three playback treatments, and each playback was a loop composed of 30 s of stimulus followed by 30 s of silence. We included silent intervals to facilitate accurate scoring of offspring signaling response, in case the presence of playback signals on the audio track interfered with scoring. However, because offspring group signals contain energy at higher frequencies than do the maternal signals or wind vibrations, we were easily able to score all group signals, including those produced during vibrational stimuli. We controlled for possible effects of treatment order by randomly assigning each family to a pre-determined treatment sequence and by waiting 1 h between treatments.
We scored offspring group signaling rates for each family using XBAT (Harold Figueroa, Ithaca, NY). Because sample size was limited, we compared signaling responses among treatments in both experiments using the Quade test (Quade, 1979), a non-parametric analog of repeated-measures ANOVA, rather than using a mixed-model approach. Statistical tests were conducted with R statistical software, version 2.13.0.
Experiments 4 & 5: Do Offspring and Maternal Signals Attract Potential Predators?
Oak treehopper maternal and offspring signals have different acoustic properties (see Results), and may differ in their salience to a predator. Here, we tested the hypothesis that offspring signals, but not maternal signals, attract vibrationally-sensitive insect predators. With two separate experiments, we assessed the responses of predatory stinkbugs (Hemiptera: Pentatomidae: Podisus maculiventris) to oak treehopper offspring and then maternal signals in the laboratory.
In both experiments, we allowed juvenile stinkbugs to walk up a thin string tied to the center of a branch of a potted oak (Q. alba) sapling (Figure 2). Each predator (N = 30 for offspring signals; N = 51 for maternal signals) received only one playback treatment. Sample size differences are an artifact of the two experiments being done in different years (2010 and 2009, respectively). Here, we wanted to test whether predators are attracted to oak treehopper offspring or maternal signals in particular, as compared with vibrations from other sources, such as abiotic factors. We therefore played offspring signals or maternal signals, wind vibrations, and silence for 3 min in continuous loops. We randomized stimulus order and stimulus exemplars. For the first experiment, we recorded two exemplars each of offspring signals and of wind vibrations, and for the second experiment, five exemplars each of maternal signals and wind vibrations. All exemplars were drawn from field recordings of simulated predator attacks and wind vibrations. When playing wind vibrations, we matched peak acceleration to that of the offspring signals being played.
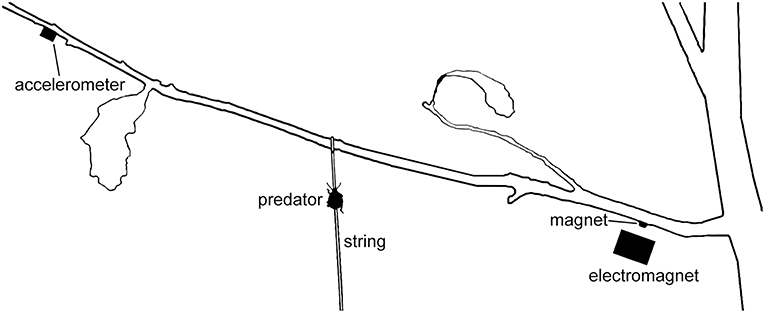
Figure 2. Playback setup for Experiments 4 and 5. Predatory stinkbugs were introduced to string and allowed to walk up to the branch of a potted Q. alba sapling. Vibrational stimuli were imparted using an electromagnet driving a magnet attached to the branch at the typical resting location of a female oak treehopper and monitored using an accelerometer.
We were provided with stinkbugs by the USDA-ARS Biological Control of Insects Research Laboratory (Columbia, MO). We maintained a laboratory colony at ~ 25°C on a 14:10 h light:dark cycle. We fed stinkbugs a combination of fourth instar larvae of Trichoplusia ni (Hübner) and a zoophytogenous artificial diet (Coudron et al., 2002) and provided water via moist dental wicks (Richmond Dental) in small plastic weigh boats (Fisher Scientific).
We began scoring predator behavior when all of a predator's legs made contact with a branch and ended after 180 s or when the predator dropped from the branch. Stinkbugs detect substrate vibrations with sensory organs in their legs (Čokl and Virant-Doberlet, 2003). Stinkbugs have been shown to remain in the local area of an attractive stimulus (Mazzoni et al., 2017), and pause when attending to attractive vibrational stimuli, as when choosing a direction at a Y-junction (Ota and Čokl, 1991; Čokl et al., 1999). We therefore scored the proportion of time that each predator remained stationary and the time spent on the half of the branch nearest to the playback vibration source as indices of stimulus attractiveness.
To assess the effect of playback treatment on the proportion of time predators remained stationary, we fitted a generalized linear model to the quasibinomial distribution (Warton and Hui, 2011). We set contrasts to compare predator responses during the signal treatment against those during silence and wind vibration treatments. The second response variable, proportion of time spent near the vibration source, had a nearly binary distribution: most (28/30) predators spent very little (< 1/4 of total time) or almost all (> 3/4 of total time) near the vibration source. We therefore treated these responses as binary data (success: predators spend ≥ half of observation time near the vibration source), fitted a logistic regression model with contrasts set as in the binomial model, and evaluated treatment level effects with likelihood ratio tests (LRTs). For all three response variables, we first tested for effects of playback exemplars. We found no significant exemplar effects (all P > 0.15) and pooled data within each treatment. Because there were three measures of predator attraction, we corrected P-values for false discovery rate (FDR) (Benjamini and Hochberg, 1995).
Results
Experiment 1: Do Offspring Vibrational Signals Communicate Increased Risk to Mothers?
Mothers signaled at a higher rate and walked for a greater proportion of time when offspring signals were played than when silence was played (for both maternal walking and signaling: offspring signals vs. silence, Wilcoxon W = 0, PFDR = 0.016, Figure 3). The offspring in each family also produced some vibrational signals during trials, but we found no difference in the number of group signals produced by nymphs according to playback treatment (offspring signals vs. silence, Wilcoxon W = 0, PFDR = 0.125). Maternal walking and signaling responses did not differ between trials in which their offspring signaled along with playbacks and those with playback signals alone (maternal signaling: Wilcoxon W = 9, P = 0.905; walking: Wilcoxon W = 12, P = 0.712).
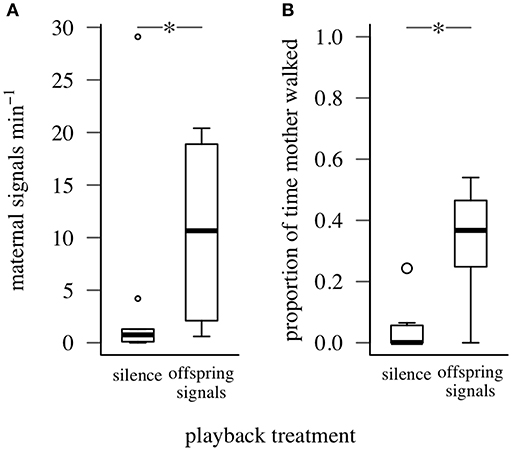
Figure 3. Signaling and walking response of mothers to playbacks of silence and offspring group signals (Experiment 1). *represents PFDR < 0.05, Wilcoxon Signed-Rank Test. Box plots show distributions of (A) maternal signaling rate, (B) proportion of time mothers walked (minimum, first quartile, median, third quartile, maximum; open circles represent outliers).
Experiment 2: Do Maternal Vibrational Signals Reduce Offspring Signaling?
In response to simulated predator attacks, oak treehopper mothers produced vibrational signals consisting of short syllables (69 ± 38 ms) as they began walking, and a combination of short and longer (613 ± 208 ms) syllables after ending their search and resettling at the base of the offspring aggregation (Figure 4) (N = 11 females, 7–10 of each signal type were analyzed from each female). Maternal signals then continued at high rates for several minutes. The frequency spectra of both short and long maternal signals were broadband (90% Bandwidth: 1793 ± 862 Hz and 1964 ± 392 Hz for short and long signals, respectively); the frequencies with greatest amplitude were 126 ± 87 Hz (short signals) and 192 ± 57 Hz (long signals).
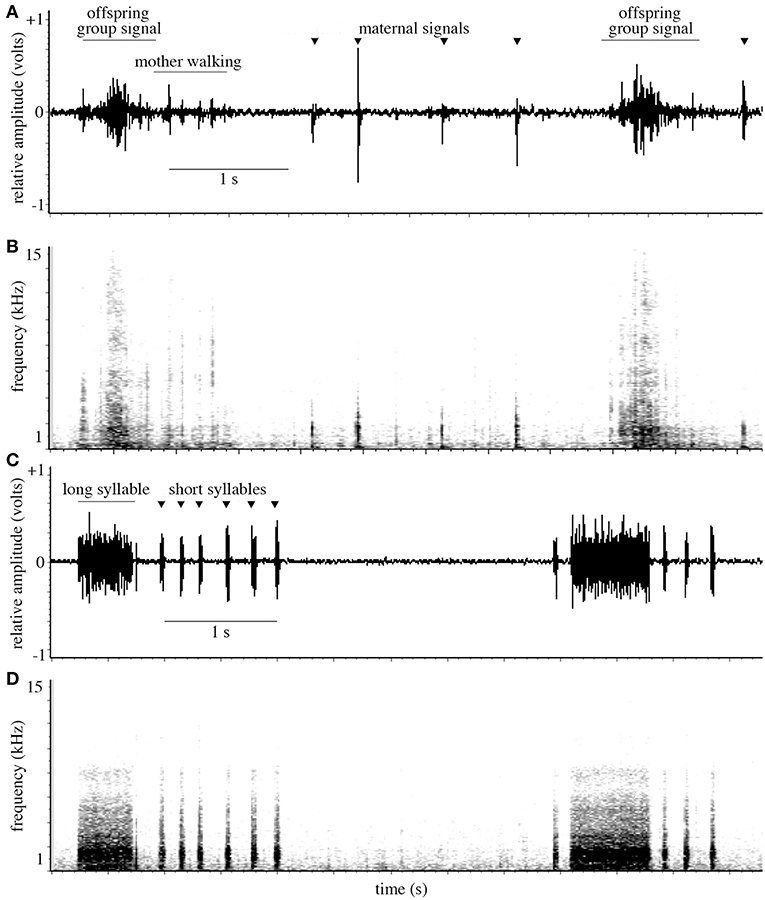
Figure 4. (A) Waveform and (B) spectrogram showing oak treehopper offspring vibrational signals; vibrations from mother walking; and maternal early encounter signals (marked with triangular points). (C) Waveform and (D) spectrogram showing oak treehopper maternal post-search vibrational signals with long and short syllables, produced after a mother has returned to her resting position at the base of the offspring aggregation.
Offspring produced signals individually and as synchronized groups. Individual signals had a mean duration of 75 ± 26 ms (N = 11 aggregations, 7–10 signals analyzed from each). Group signals are formed by overlapping individual signals and have a mean duration of 570 ± 207 ms (N = 11 aggregations, 2–10 signals analyzed from each). Group signals have peak amplitude near the midpoint of each signal and a characteristic waveform (Figure 4). Frequency spectra of individual and group signals were broadband (90% Bandwidth: individual: 7166 ± 862 Hz; group: 8955 ± 4796 Hz). For group signals, the frequency with the greatest amplitude was 386 ± 299 Hz; for individual signals, it was 83 ± 120 Hz.
Maternal post-search vibrational signals reduced offspring collective signaling during simulated predator attacks. The full model explained 68% of the variation in offspring signaling rate. After accounting for the effect of temperature on offspring behavior (GLMM, coefficient = 0.801, SE = 0.241, P < 0.001), offspring aggregations produced fewer group signals during playbacks of maternal post-search signals than during silence (maternal post-search signals: 3.41 ± 3.52 / min; maternal early encounter signals: 5.00 ± 3.37 / min; silence: 6.03 ± 4.56 / min; values are means ± SDs) (GLMM, coefficient = −0.609, SE = 0.195, P = 0.002; Figure 5). In contrast, offspring aggregations produced similar numbers of group signals during playbacks of maternal early encounter signals and silence (GLMM, coefficient = −0.267, SE = 0.177, P = 0.130). The experimental design parameters we expected to influence offspring signaling response accounted for 7.03% of variation (carryover: 4.89%; treatment sequence: 2.14%), and interactions between temperature and design parameters another 11.53%.
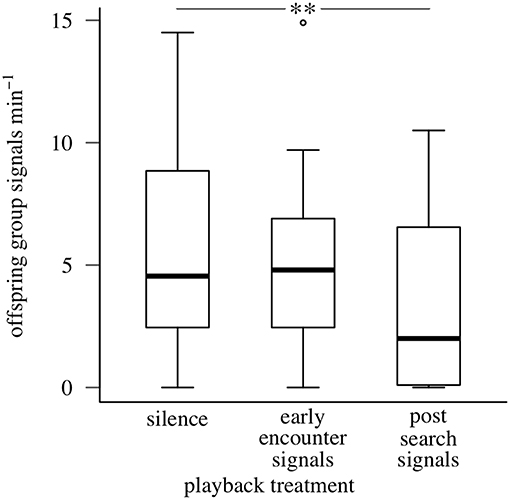
Figure 5. Signaling responses of oak treehopper offspring aggregations to playback of silence, maternal early encounter signals, or post-search signals during simulated predator attacks (Experiment 2). Box plots show distributions of offspring group signaling rate (minimum, first quartile, median, third quartile, maximum; open circles represent outliers). N = 28 families. **represents P < 0.01, GLMM.
Experiment 3: Do Maternal Vibrational Signals Reduce False Alarms by Offspring?
Maternal vibrational signals did not reduce false alarms by offspring after simulated predator attacks: offspring group signaling rate did not differ by playback treatment (Quade F = 1.519, df = 2/18, P = 0.25; Figure 6). Offspring aggregations produced group signals at similar (low) rates after simulated attacks, regardless of which vibrational stimulus type was played (maternal post-search signals: 2.25 ± 1.61/min; wind vibrations: 1.75 ± 1.14/min; silence: 2.86 ± 2.57/min).
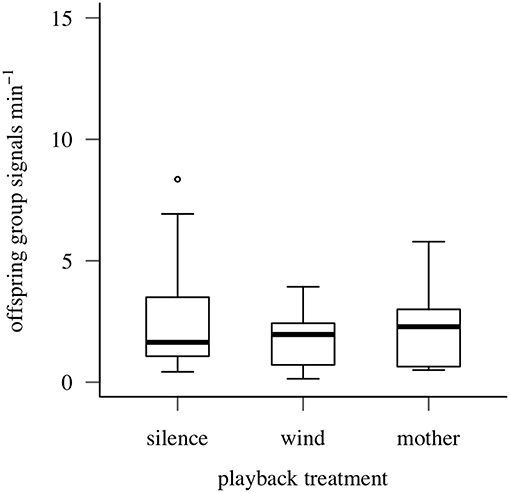
Figure 6. Signaling responses of oak treehopper offspring aggregations to playback of silence, wind vibrations, or maternal post search signals after simulated predator attacks (Experiment 3). Box plots show distributions of offspring group signaling rate (minimum, first quartile, median, third quartile, maximum; open circles represent outliers). N = 10 families.
Experiments 4 & 5: Do Offspring and Maternal Signals Attract Potential Predators?
Playback of offspring signals influenced the proportion of time that predators remained stationary (N = 30, [F(2, 27) = 8.458, P = 0.001, PFDR = 0.003]: predators were still for a greater proportion of each trial when offspring signals were played than when silence or wind vibrations were played (Figure 7; Supplementary Table 1). Additionally, the proportion of predators spending more than 50% of the observed time near the vibration source was higher during playback of offspring signals than during silence, although this did not differ between offspring signals (0.70 of individuals) and wind vibrations (0.40 of individuals) (Figure 7; Supplementary Table 1), and the overall effect of playback treatment on predator location was not significant (N = 30, LRT = 5.368, P = 0.068, PFDR = 0.068). In contrast, playback of maternal signals did not influence the proportion of time that predators remained stationary [N = 51, F(2, 48) = 1.989, P = 0.148, PFDR = 0.296], or the proportion of predators spending ≥ 50% time near the vibration source (N = 51, LRT = 2.124, P = 0.346, PFDR = 0.346) (Figure 7; Supplementary Table 1).
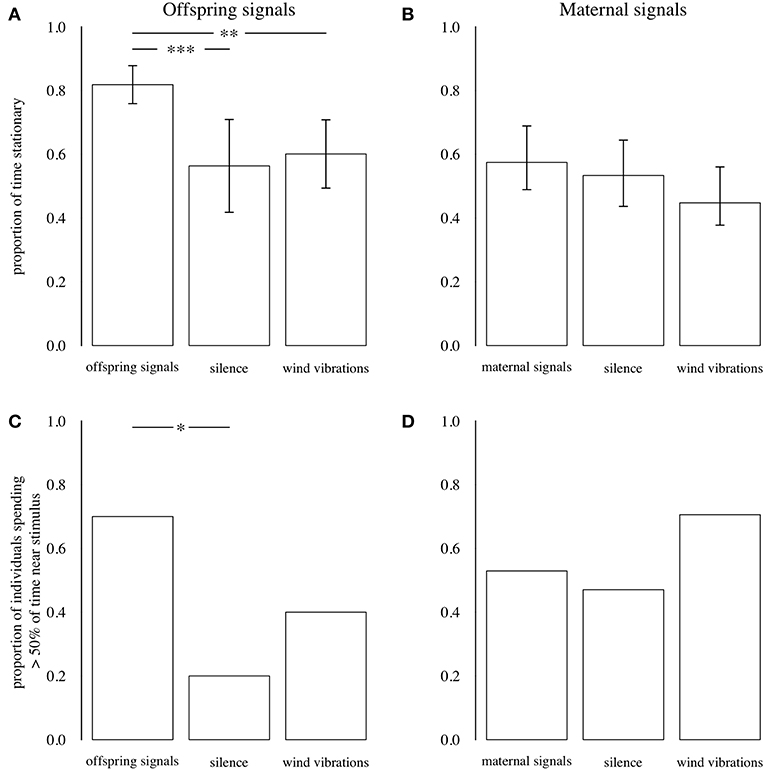
Figure 7. Top: Proportion of time that predators remained stationary during playbacks of offspring (A) and maternal (B) signals, silence, and wind vibrations. Bars show means; error bars are 95% CIs. Bottom: Proportion of individuals who spent > 50% of total time near the vibration stimulus during playbacks of offspring (C) and maternal (D) signals, silence, and wind vibrations. Data from Experiments 4 and 5. *P ≤ 0.05, **P ≤ 0.01, ***P ≤ 0.001, GLMs.
Discussion
We have established the functions of vibrational signals produced by oak treehopper mothers and offspring in a field population during simulated predator attacks, and we have provided evidence that as with social communication in other modalities, social vibrational signals may be exploited by unintended receivers. Our results also suggest that by reducing offspring vibrational signaling, maternal signaling may reduce the likelihood of attracting invertebrate predators.
Oak treehopper mothers and offspring both produce vibrational signals during simulated predator attacks. Offspring group signals evoke defensive behavior by mothers, and maternal signals inhibit offspring from signaling. Offspring signaling and maternal responses to playbacks of offspring signals, including walking and signaling, were all consistent with preliminary observations of familial responses during predator attacks (Hamel, 2011).
We consider our two hypotheses to explain why mothers inhibit offspring signaling, beginning with whether mothers might improve the accuracy of offspring signals. By inhibiting offspring signaling, maternal signals do not reduce the number of false alarms by offspring groups, as they do in a closely related species, in which offspring often continue signaling after predator attacks have ended, and even spontaneously begin signaling again after ceasing (Cocroft, 1999a). Oak treehopper offspring signaled during simulated predator attacks, but they produced very few signals after attacks ended. In other words, they tend not to produce false alarms, whether or not the mother is signaling.
Our second hypothesis was that by silencing offspring, maternal signaling might reduce a family's apparency to predators. A generalist invertebrate predator that locates other species of insect prey via incidentally produced substrate-borne vibrations (Pfannenstiel et al., 1995) attends to oak treehopper offspring signals, but not to maternal signals, with a stationary posture. Although animals adopt stationary postures in response to both attractive stimuli and to perceived threats, the predators in this study also spent more time near the stimulus source when offspring signals were played. In the field, offspring aggregations occur on the same trees, and sometimes the same branches, as vibrationally sensitive invertebrate predators (J.H., pers. obs.). Silencing oak treehopper offspring may therefore reduce the risk of attracting predators occurring in close proximity to relatively immobile offspring groups.
Although the long and semi-continuous signals that oak treehopper mothers produce after evicting predators silenced offspring, the short signals that mothers typically produce while searching for a predator did not inhibit offspring signaling. Interplay between short maternal signals and offspring signals may help the mother locate the source of disturbance. In a closely related species, those offspring closest to the predator are most likely to participate in each group signal, and those farther away are less likely to do so, potentially providing cues to the mother on predator location (Ramaswamy and Cocroft, 2009). However, these position-dependent signaling differences only occur when the mother is present, suggesting that the offspring are responding to some maternal cue, such as vibrational signals.
How Likely Is Predator Attraction by Vibrational Offspring Signals in Nature?
Our findings support work with other animal taxa (Allan et al., 1996; Krams et al., 2007; Magrath et al., 2010) showing that social signals attract predators to groups. Although most studies of predator eavesdropping focus on mate advertisement signals (Zuk and Kolluru, 1998; Haynes and Yeargan, 1999; Peake, 2005), social communication produces a concentrated and persistent source of signals and may be especially vulnerable to eavesdropping. Adaptations to reduce the apparency of social signals to predators and parasitoids are likely to be a general, if often overlooked, feature of social communication. For species with maternal care, offspring signals are produced primarily during periods of social interaction such as soliciting food or protection (Leech and Leonard, 1997; Lingle et al., 2012), and these are the periods of greatest risk of attracting eavesdropping predators (Leech and Leonard, 1997; Wise et al., 1999; Haff and Magrath, 2011). Likewise, signaling by oak treehopper mothers and offspring during predator attacks will make families conspicuous to eavesdroppers, as multiple invertebrate predators often occur simultaneously on the same tree, and even in the same local area on a given tree.
The predators in this study were naïve, lab-reared study animals, and experience may affect predator responses to offspring and maternal signals in the field. For example, female oak and thornbug treehoppers both defend offspring against invertebrate predators with kicks and wing-buzzes, behaviors that effectively deter predators and that are commonly interspersed with maternal vibrational signals (Wood, 1976; Cocroft, 1999b; Hamel, 2011; Hamel and Cocroft, 2012). In laboratory studies, invertebrates associate vibrational cues with both aversive stimuli (Abramson, 1986) and food rewards (Guillette et al., 2009). Therefore, predators may learn to avoid vibrational signals associated with maternal kicks, to approach group signals produced by abundantly occurring offspring aggregations, and to ignore common environmental sources of vibrational noise, such as wind.
Evidence that plant-borne vibrational communication is subject to predator eavesdropping is growing (reviewed in Virant-Doberlet et al., 2014). Although the vibrational modality has been hypothesized as a means of escaping eavesdroppers (Henry, 1994; Zuk and Kolluru, 1998), predator eavesdropping on vibrational signals is likely, given the abundance and diversity of vibrationally-sensitive invertebrate predators in the environments where communication occurs (Cocroft and Rodríguez, 2005), and the ability of invertebrate predators to learn associations between vibration cues and food rewards (Guillette et al., 2009). Invertebrate predators can locate prey via incidental vibrations (Pfannenstiel et al., 1995; Barth, 1998; Meyhöfer and Casas, 1999), and invertebrate predators home in on vibrational mate advertisement signals as well (Narhardiyati and Bailey, 2005; Laumann et al., 2007, 2011; Roberts et al., 2007; Virant-Doberlet et al., 2011).
In summary, this study provides evidence that predators can exploit social vibrational signals, and that a benefit of inhibiting offspring signaling may be reducing predation risk. Consequently, we suggest that predator eavesdropping is a probable cost of within-group communication for invertebrate groups using vibrational signals, and such signaling groups may experience strong selection by predators and parasitoids to reduce the apparency of their signals.
Author Contributions
JH and RC contributed to the conception and design of the study. JH conducted the experiments, performed the statistical analysis, and wrote the first draft of the manuscript. RC contributed to data collection and analysis and provided extensive and thoughtful revisions to the manuscript. All authors have contributed to manuscript revision, read, and approved the submitted version.
Funding
This study was funded by a U.S. Department of Education Graduate Assistance in Areas of National Need (GAANN) Fellowship, an Explorers Club Exploration Fund Grant, and a University of Missouri TransWorld Airlines Scholarship. Open access publication fees were provided by the Elon University College of Arts and Sciences and the Elon University Department of Biology.
Conflict of Interest Statement
The authors declare that the research was conducted in the absence of any commercial or financial relationships that could be construed as a potential conflict of interest.
Acknowledgments
We thank Kevin Bender, Lexie Dorfzaun, Michelle Feole, Tim Golden, Mark Rothschild, and Holley Short for assistance in the field, and J. Benjamin Christ, Megan Johnson, and Rodrigo Landeros for assistance in the laboratory. Judith Toms provided advice on statistical methods. We thank the staff and faculty of the University of Florida Ordway-Swisher Biological Station for facilitating work in the field, and Tom Coudron and James Smith of the USDA-ARS Biological Control of Insects Research Laboratory in Columbia, MO for providing Podisus maculiventris. We are grateful to Flavia Barbosa, Eric Bauer, and Laurel Symes for helpful comments on earlier versions of this manuscript.
Supplementary Material
The Supplementary Material for this article can be found online at: https://www.frontiersin.org/articles/10.3389/fevo.2019.00204/full#supplementary-material
Supplementary Video 1. Vibrational playback from Experiment 1, in which offspring group signals were recorded from and played back to mothers and offspring of nine oak treehopper families. Here, a mother walks and produces early-encounter signals in response to playback of offspring vibrational signals.
References
Abramson, C. I. (1986). Aversive conditioning in honeybees (Apis mellifera). J. Comp. Psych. 100, 108–116. doi: 10.1037/0735-7036.100.2.108
Allan, R. A., Elgar, M. A., and Capon, R. J. (1996). Exploitation of an ant chemical alarm signal by the zodariid spider Habronestes bradleyi Walckenaer. Proc. Royal Society B: Biol. Sci. 263, 69–73. doi: 10.1098/rspb.1996.0012
Barth, F. G. (1998). “The vibrational sense of spiders,” in Comparative Hearing: Insects, eds. R. R. Hoy, A. N. Popper, and R. R. Fay (Springer-Verlag), 228–278. doi: 10.1007/978-1-4612-0585-2_7
Beamer, R. H. (1930). Maternal instinct in a membracid (Platycotis vittata) (Homop.). Entomol. News 41, 330–331.
Benjamini, Y., and Hochberg, Y. (1995). Controlling the false discovery rate: a practical and powerful approach to multiple testing. J. R. Stat. Soc. Series B Stat. Methodol. 57, 289–300. doi: 10.1111/j.2517-6161.1995.tb02031.x
Cocroft, R., and Rodríguez, R. L. (2005). The behavioral ecology of insect vibrational communication. BioScience 55, 323–334. doi: 10.1641/0006-3568(2005)055[0323:TBEOIV]2.0.CO;2
Cocroft, R. B. (1996). Insect vibrational defence signals. Nature 382, 679–680. doi: 10.1038/382679a0
Cocroft, R. B. (1999a). Offspring-parent communication in a subsocial treehopper (Hemiptera: Membracidae: Umbonia crassicornis). Behaviour 136, 1–21. doi: 10.1163/156853999500640
Cocroft, R. B. (1999b). Parent-offspring communication in response to predators in a subsocial treehopper (Hemiptera: Membracidae: Umbonia crassicornis). Ethology 105, 553–568.
Cocroft, R. B. (2011). The public world of insect vibrational communication. Mol. Ecol. 20, 2041–2043. doi: 10.1111/j.1365-294X.2011.05092.x
Cocroft, R. B., and Hamel, J. A. (2010). “Vibrational communication in the ‘other' social insects: a diversity of ecology, signals, and signal function,” in Vibrational Communication in Animals, eds. C. O'Connell-Rodwell (Trivandrum: Research Signposts, 47–68.
Cocroft, R. B., Hamel, J. A., Su, Q., and Gibson, J. (2014). “Vibrational playback experiments: challenges and solutions,” in Studying Vibrational Communication, eds R. B. Cocroft, M. Gogala, P. S. M. Hill, and A. Wessel (Berlin; Heidelberg: Springer-Verlag), 249–274. doi: 10.1007/978-3-662-43607-3_13
Čokl, A., and Virant-Doberlet, M. (2003). Communication with substrate-borne signals in small plant-dwelling insects. Annu. Rev. Entomol. 48, 29–50. doi: 10.1146/annurev.ento.48.091801.112605
Čokl, A., Virant-Doberlet, M., and McDowell, A. (1999). Vibrational directionality in the southern green stink bug, Nezara viridula (L.), is mediated by female song. Anim. Behav. 58, 1277–1283. doi: 10.1006/anbe.1999.1272
Coudron, T. A., Wittmeyer, J., and Kim, Y. (2002). Life history and cost analysis for continuous rearing of Podisus maculiventris (Say) (Heteroptera: Pentatomidae) on a zoophytophagous artificial diet. J. Econ. Entomol. 95, 1159–1168. doi: 10.1603/0022-0493-95.6.1159
Evans, T. A., Inta, R., Lai, J. C. S., Prueger, S., Foo, N. W., Fu, E. W., et al. (2009). Termites eavesdrop to avoid competitors. Proc. Royal Society B: Biol. Sci. 276, 4035–4041. doi: 10.1098/rspb.2009.1147
Guillette, L. M., Hollis, K. L., and Markarian, A. (2009). Learning in a sedentary insect predator: Antlions (Neuroptera: Myrmeleontidae) anticipate a long wait. Behav. Proc. 80, 224–232. doi: 10.1016/j.beproc.2008.12.015
Haff, T. M., and Magrath, R. D. (2011). Calling at a cost: elevated nestling calling attracts predators to active nests. Biol. Lett. 7, 493–495. doi: 10.1098/rsbl.2010.1125
Hamel, J. A. (2011). Communication About Predation Risk between Parents and Offspring Groups in Treehoppers. Columbia, SC: University of Missouri.
Hamel, J. A., and Cocroft, R. (2012). Negative feedback from maternal signals reduces false alarms by collectively signalling offspring. Proc. Royal Society B: Biol. Sci. 279, 3820–3826. doi: 10.1098/rspb.2012.1181
Haynes, K. F., and Yeargan, K. V. (1999). Exploitation of intraspecific communication systems: illicit signalers and receivers. Ann. Entomol. Soc. Am. 92, 960–970. doi: 10.1093/aesa/92.6.960
Henry, C. S. (1994). Singing and cryptic speciation in insects. Trends Ecol. Evol. 10, 388–392. doi: 10.1016/0169-5347(94)90061-2
Krams, I., Krama, T., Igaune, K., and Mand, R. (2007). Long-lasting mobbing of the pied flycatcher increases the risk of nest predation. Behav. Ecol. 18, 1082–1084. doi: 10.1093/beheco/arm079
Laumann, R. A., Čokl, A., Lopes, A. P. S., Fereira, J. B. C., Moraes, M. C. B., and Borges, M. (2011). Silent singers are not safe: selective response of a parasitoid to substrate-borne vibratory signals of stink bugs. Anim. Behav. 82, 1175–1183. doi: 10.1016/j.anbehav.2011.08.017
Laumann, R. A., Moraes, M. C. B., and Čokl, A. (2007). Eavesdropping on sexual vibratory signals of stink bugs (Hemiptera: Pentatomidae) by the egg parasitoid Telenomus podisi. Anim. Behav. 73, 637–649. doi: 10.1016/j.anbehav.2006.09.011
Leech, S. M., and Leonard, M. L. (1997). Begging and the risk of predation in nestling birds. Behav. Ecol. 8, 644–646. doi: 10.1093/beheco/8.6.644
Lingle, S., Wyman, M. T., Kotrba, R., Teichroeb, L. J., and Romanow, C. A. (2012). What makes a cry a cry? A review of infant distress vocalizations. Curr. Zool. 58, 698–726. doi: 10.1093/czoolo/58.5.698
Magrath, R. D., Haff, T. M., Horn, A. G., and Leonard, M. L. (2010). Calling in the face of danger: predation risk and acoustic communication by parent birds and their offspring. Adv. Study Behav. 187–253. doi: 10.1016/S0065-3454(10)41006-2
Mazzoni, V., Polajnar, J., Baldini, M., Rossi Stacconi, M. V., Anfora, G., Guidetti, R., et al. (2017). Use of substrate-borne vibrational signals to attract the Brown Marmorated Stink Bug, Halyomorpha halys. J. Pest Sci. 90, 1219–1229 doi: 10.1007/s10340-017-0862-z
McNett, G. D., Luan, L. H., and Cocroft, R. B. (2010). Wind-induced noise alters signaler and receiver behavior in vibrational communication. Behav. Ecol. Sociobiol. 64, 2043–2051. doi: 10.1007/s00265-010-1018-9
Meyhöfer, R., and Casas, J. (1999). Vibratory stimuli in host location by parasitic wasps. J. Insect Physiol. 45, 967–971. doi: 10.1016/S0022-1910(99)00060-8
Morris, G. K., Mason, A. C., and Wall, P. (1994). High ultrasonic and tremulation signals in neotropical katydids (Orthoptera: Tettigoniidae). J. Zool. Lond. 233, 129–163. doi: 10.1111/j.1469-7998.1994.tb05266.x
Narhardiyati, M., and Bailey, W. J. (2005). Biology and natural enemies of the leafhopper Balclutha incisa (Matsumura) (Hemiptera: Cicadellidae: Deltocephalinae) in south-western Australia. Austral. Entomol. 44, 104–109. doi: 10.1111/j.1440-6055.2005.00460.x
Nault, L. R., Wood, T. K., and Goff, A. M. (1974). Treehopper (Membracidae) alarm pheromones. Nature 249, 387–388. doi: 10.1038/249387a0
Ota, D., and Čokl, A. (1991). Mate location in the southern green stink bug, Nezara viridula (Heteroptera: Pentatomidae), mediated through substrate-borne signals on ivy. J. Insect Behav. 4, 441–447. doi: 10.1007/BF01049329
Peake, T. (2005). “Eavesdropping in communication networks,” in Animal Communication Networks, ed. P. K. McGregor (Cambridge: Cambridge University Press), 13–37. doi: 10.1017/CBO9780511610363.004
Pfannenstiel, R. S., Hunt, R. E., and Yeargan, K. V. (1995). Orientation of a hemipteran predator to vibrations produced by feeding caterpillars. J. Insect Behav. 8, 1–9. doi: 10.1007/BF01990965
Quade, D. (1979). Using weighted rankings in the analysis of complete blocks with additive block effects. J. Am. Stat. Assoc. 74, 680–683. doi: 10.1080/01621459.1979.10481670
Ramaswamy, K., and Cocroft, R. B. (2009). Collective signals in treehopper broods provide predator localization cues to the defending mother. Anim. Behav. 78, 697–704. doi: 10.1016/j.anbehav.2009.06.017
Roberts, J. A., Taylor, P. W., and Uetz, G. W. (2007). Consequences of complex signaling: predator detection of multimodal cues. Behav. Ecol. 18, 236–240. doi: 10.1093/beheco/arl079
Rodríguez, R. L., Ramaswamy, K., and Cocroft, R. B. (2006). Evidence that female preferences have shaped male signal evolution in a clade of specialized plant-feeding insects. Proc. Royal Society B: Biol. Sci. 273, 2585–2593. doi: 10.1098/rspb.2006.3635
Römer, H., Lang, A., and Hartbauer, M. (2010). The signaller's dilemma: a cost–benefit analysis of public and private communication. PLoS ONE 5, e13325. doi: 10.1371/journal.pone.0013325
Virant-Doberlet, M., King, R. A., Polajnar, J., and Symondson, W. O. C. (2011). Molecular diagnostics reveal spiders that exploit prey vibrational signals used in sexual communication. Mol. Ecol. 20, 2204–2216. doi: 10.1111/j.1365-294X.2011.05038.x
Virant-Doberlet, M., Kuhelj, A., Polajnar, J., and šturm, R. (2019). Predator-prey interactions and eavesdropping in vibrational communication networks. Front. Ecol. Evol. 7:203. doi: 10.3389/fevo.2019.00203
Virant-Doberlet, M., Mazzoni, V., de Groot, M., Polajnar, J., Lucchi, A., Symondson, W. O. C., et al. (2014). “Vibrational communication networks: eavesdropping and biotic noise,” in Studying Vibrational Communication (Springer), 93–123. doi: 10.1007/978-3-662-43607-3_7
Warton, D. I., and Hui, F. K. C. (2011). The arcsine is asinine: analysis of proportions in ecology. Ecology 92, 3–10. doi: 10.1890/10-0340.1
Wise, K. K., Conover, M. R., and Knowlton, F. F. (1999). Response of coyotes to avian distress calls: testing the startle-predator and predator-attraction hypotheses. Behaviour 136, 935–949. doi: 10.1163/156853999501658
Wood, T. K. (1976). Biology and presocial behavior of Platycotis vittata (Homoptera: Membracidae). Ann. Entomol. Soc. Am. 69, 807–811. doi: 10.1093/aesa/69.5.807
Zuk, M., and Kolluru, G. R. (1998). Exploitation of sexual signals by predators and parasitoids. Q. Rev. Biol. 73, 415–438. doi: 10.1086/420412
Keywords: predator eavesdropping, social signals, vibrational communication, parent-offspring behavior, collective behavior
Citation: Hamel JA and Cocroft RB (2019) Maternal Vibrational Signals Reduce the Risk of Attracting Eavesdropping Predators. Front. Ecol. Evol. 7:204. doi: 10.3389/fevo.2019.00204
Received: 15 December 2018; Accepted: 20 May 2019;
Published: 14 June 2019.
Edited by:
Ximena E. Bernal, Purdue University, United StatesReviewed by:
Francisco Garcia-Gonzalez, Estación Biológica de Doñana (EBD), SpainWendy Hood, Auburn University, United States
Copyright © 2019 Hamel and Cocroft. This is an open-access article distributed under the terms of the Creative Commons Attribution License (CC BY). The use, distribution or reproduction in other forums is permitted, provided the original author(s) and the copyright owner(s) are credited and that the original publication in this journal is cited, in accordance with accepted academic practice. No use, distribution or reproduction is permitted which does not comply with these terms.
*Correspondence: Jennifer A. Hamel, amhhbWVsMkBlbG9uLmVkdQ==