- Department of Organisms and Ecosystems Research, National Institute of Biology, Ljubljana, Slovenia
Due to human perceptional bias in favor of air-borne sounds, substrate-borne vibrational signaling has been traditionally regarded as a highly specialized, inherently short-range and, consequently, a private communication channel, free from eavesdropping by sexual competitors and predators. In this review, we synthesize current knowledge pertinent to the view that most animals live in a rich vibratory world, where vibrational information is available to unintended receivers. In recent years, we realized that vibrational signaling is one of the oldest and taxonomically most widespread forms of communication by mechanical waves and that receptors detecting substrate vibrations are ubiquitous. In nature, substrate vibrations are reliable source of information readily available to all members of the animal community able to detect them. Viewing vibrational communication in more relevant ecological context reveals that animals relying on substrate vibrations live in complex communication networks. Long evolutionary history of this communication channel is reflected in varied and sophisticated predator-prey interactions guided by substrate-borne vibrations. Eavesdropping and exploitation of vibrational signals used in sexual communication have been so far largely neglected; however, existing studies show that generalist arthropod predators can intercept such signals emitted by insects to obtain information about prey availability and use that information when making foraging decisions. Moreover, males which advertise themselves for longer periods than females and with vibrational signals of higher amplitude face higher predation risk. It is likely that eavesdropping and exploitation of vibrational signals are major drivers in the evolution taking place in the vibratory world and we believe that studies of interspecific interactions guided by substrate vibrations will, in the future, offer numerous opportunities to unravel mechanisms that are central to understanding behavior in general.
Introduction
Signals produced by mechanical vibrations and transmitted from the signaller to the receiver through the medium (air, water or solid substrate) via mechanical waves are an important part of animal communication (Bradbury and Vehrencamp, 1998). Speech (i.e., air-borne sound communication) is such an important element of human interactions that our strong bias in favor of this form of mechanical communication is inevitable, in particular, since our hearing also allows us to perceive most of air-borne mechanical waves (i.e., sounds) emitted by other animals. Although cutaneous mechanoreceptors in human skin are capable of detecting substrate-borne mechanical waves (e.g., Brisben et al., 1999; O'Connell-Rodwell et al., 2001; Stuart et al., 2003), our own experience of the vibratory world around us depends almost exclusively on the use of sensitive equipment. It is therefore not surprising that in contrast to air-borne sound, substrate-borne vibrations have been traditionally considered a highly specialized and rare form of animal communication, and consequently, even regarded as an adaptation to avoid detection by eavesdropping enemies (Belwood and Morris, 1987; Lima and Dill, 1990; Henry, 1994; Zuk and Kolluru, 1998; Cooley, 2001; Lang et al., 2005; Römer et al., 2010), at best accessible only to specialized parasitoids (Hughes et al., 2012). Although the evidence emerging in the last decade shows that enemies and rivals use vibrational information available in their environment to guide their behaviors crucial for reproduction and survival (reviewed in Virant-Doberlet et al., 2014), the unfortunate general misconception that vibrational signaling is a private mode of communication (i.e., inaccessible to eavesdroppers sharing the same habitat) is unexpectedly hard to put at rest. This persistence is surprising, since it is widely accepted that incidental vibrational cues induced in the substrates by activities like moving and feeding are used by predators and parasitoids to find their prey or host (e.g., Barth, 1998; Meyhöfer and Casas, 1999; Brownell and van Hemmen, 2001; Devetak, 2014), although such vibrations are generally less conspicuous than vibrational signals used in sexual communication.
Here, we aim to provide a synthesis of the current state of knowledge relevant to the view that the majority of animals lives in a rich vibratory world, where vibrational information is readily available to eavesdroppers. While the body of existing literature supporting this view is large, the available information is scattered across different research topics and often does not directly address the issue of ecological context of vibrational communication. There are so far only a few studies directly dealing with exploitation of vibrational signals used in sexual communication by eavesdropping predators or parasitoids and to challenge the view that vibrational signaling is a safe mode of communication (e.g., Henry, 1994; Zuk and Kolluru, 1998; Hughes et al., 2012), we also review the evidence that substrate vibrations guide many behaviors related to predator-prey interactions. As it has been first proposed by Cocroft and Rodríguez (2005), we argue that in this communication modality eavesdropping may be particularly common and we wish to convey that besides our perceptional bias and general lack of understanding of the importance of the vibrational channel, there is no reason for a persisting view that enemies ignore vibrational signals as a source of information helping them to find their prey or host. In this review, it is not our intention to focus on a distinction between air-borne sounds and substrate-borne vibrations and differences between acoustic and vibrational communication, since this topic has been subject of several commentaries (e.g., Cocroft et al., 2014; Endler, 2014; Hill and Wessel, 2016; Hill et al., 2019) and comprehensive reviews (e.g., Cocroft and Rodríguez, 2005; Hill, 2008; Caldwell, 2014; Yack, 2016).
Due to their diversity, abundance and reliance on substrate vibrations, arthropods are the best animal group to provide an insight into life in the vibratory world and various aspects of their behavior that is guided by substrate vibrations have been studied in detail. In line with the existing literature and our own research, the review is therefore focused primarily on interactions in arthropods; however, we also include the evidence from other animal groups whenever appropriate. Throughout the review, we use eavesdropping in accordance with the existing literature, where it is defined as “extracting information from signaling interactions between others” (McGregor and Peake, 2000). Consequently, we do not consider interactions that do not include interception of vibrational signals used in communication (e.g., incidental vibrational cues, mimicry) as eavesdropping. We further define exploiters as a subset of eavesdroppers that use extracted information to gain advantage over the signallers thus incurring direct or indirect costs (Bradbury and Vehrencamp, 1998). In this context, eavesdropping rivals that may extract from vibrational signals relative information about the size and dominance of signaling males and use this information to avoid direct encounters, are not considered exploiters.
Vibrational Communication Networks
Viewing communication in a more ecological context, it becomes clear that in nature communication occurs not only in a signaller-intended receiver dyad but in a group of several animals within signaling and receiving range of each other (i.e., in a network environment) (McGregor and Peake, 2000; McGregor, 2005; Virant-Doberlet et al., 2014). Taking into account that each signal can be received by several receivers and that each receiver can receive signals from several signallers, an emerging property of the network environment is that signals can be exploited by eavesdropping receivers (e.g., rivals, enemies) (Peake, 2005) (Figure 1). The complexity of the overall network structure depends on the identities and numbers of signallers and receivers able to detect the emitted signals (i.e., ultimately on the animal community present in the habitat). The still prevailing perception that vibrational signaling may be a safer communication channel than air-borne sounds (e.g., Hughes et al., 2012; Römer, 2013; Yack, 2016) mainly results from an impression that substrate vibrations are a rare and inherently short-range form of communication and consequently, the opportunities for eavesdropping and exploitation are severely limited. The first step in changing this perception is therefore to raise the awareness that vibrational signaling is one of the most widespread forms of animal communication and that the emitted signals are accessible to many heterospecifics, including potential enemies.
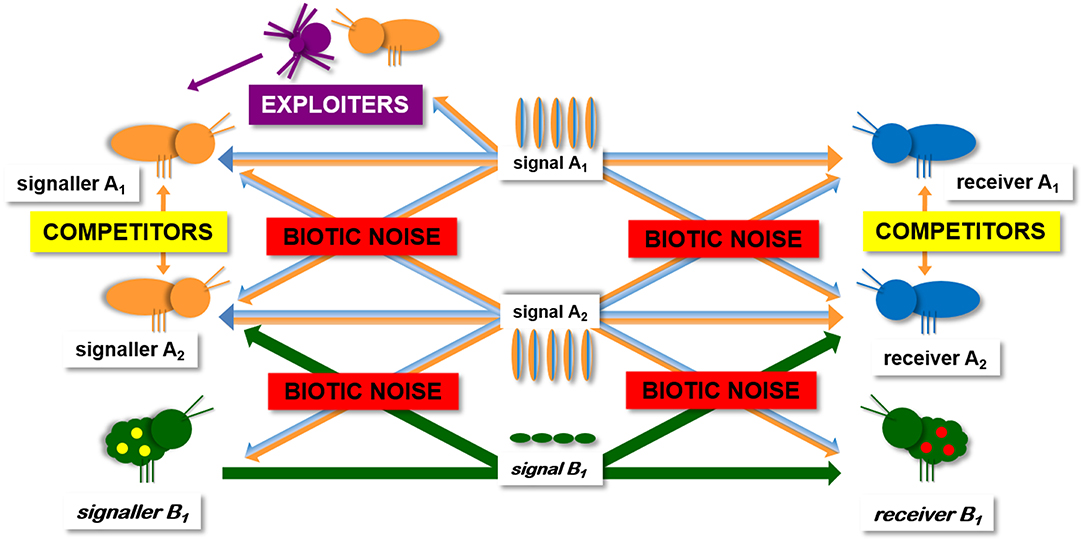
Figure 1. Schematic presentation of insect vibrational communication network. Natural habitat contains other conspecific and heterospecific signallers, as well as eavesdropping exploiters like rivals and enemies. The complexity of any real world communication network depends on the identities and numbers of signallers and receivers able to detect the emitted signals. In a duetting communication system, the same individual is the emitter and the receiver (A1, A2). From potential interactions within such a communication network, it emerges that both, signallers and receivers take on a range of costs and benefits that may have fundamental implications for their communication strategies.
How Common Is Vibrational Signaling and Reception?
As are substrate vibrations ubiquitous in the environment (Hill, 2009; Šturm et al., 2019), so are vibration receptors ubiquitous in organisms (e.g., Hill, 2008; Narins et al., 2016; Sugi et al., 2018) and vibrational signaling is now considered one of the most ancient forms of communication (Cocroft et al., 2014; Endler, 2014). It is likely that it is present in bacteria (e.g., Reguera, 2011) and vibrational stimuli affect plants as well (Appel and Cocroft, 2014). So far, behavioral response to vibrational stimuli has been documented in nematodes (Torr et al., 2004), molluscs (Roberts et al., 2015), annelids (Mitra et al., 2009), arthropods (Hill, 2008), and vertebrates (Narins et al., 2016).
Vibrational signaling, vibration reception and behavior guided by substrate-borne vibrations have been best studied in vertebrates and arthropods. In vertebrates, the use of vibrational signaling in intraspecific interactions has been described in all major groups (e.g., Whang and Janssen, 1994; Barnett et al., 1999; O'Connell-Rodwell, 2007; Caldwell et al., 2010a; Ota et al., 2015). The frequency range of vertebrate vibrational signals spans from infrasonic range below 20 Hz up to 1 kHz, with most of the energy found in the range below 500 Hz (Lewis and Narins, 1985; Narins et al., 1992; O'Connell-Rodwell, 2007; Caldwell et al., 2010a; Mason and Narins, 2010; Bishop et al., 2015; Halfwerk et al., 2016). Vertebrates detect substrate vibrations via somatosensory mechanoreceptors in their skin and joints and/or via an extratympanic pathway to their auditory system (reviewed in Hill, 2008; Narins et al., 2016). Greatest frequency and amplitude sensitivity to vibrations is species-specific and usually lies between 50 and 500 Hz, where animals can detect substrate displacements as small as 1 nm (reviewed in Gridi-Papp and Narins, 2010; Mason and Narins, 2010;Narins et al., 2016).
Relying on substrate-borne vibrations to gather information from the environment is particularly common in arthropods. Vibrational behavior is prevalent in insects (reviewed in Virant-Doberlet and Čokl, 2004; Cocroft and Rodríguez, 2005) and spiders (reviewed in Uhl and Elias, 2011). Studies suggest that it may also be common in crustaceans (Taylor and Patek, 2010), scorpions (Brownell and van Hemmen, 2001) and millipedes (Wesener et al., 2011). All arthropods possess sensitive mechanoreceptors to detect substrate vibrations (Hill, 2008, 2009) and it is currently estimated that around 200,000 insect and 40,000 spider species use vibrational communication in a variety of intraspecific interactions (Virant-Doberlet and Čokl, 2004; Cocroft and Rodríguez, 2005; Uhl and Elias, 2011). While many arthropods use vibrational signaling in combination with other modalities, a conservative estimate is at least 150,000 insect species rely exclusively on vibrational communication (Cocroft and Rodríguez, 2005). Vibrational signals used in sexual communication are species- and sex-specific and characterized by their distinct temporal and spectral properties (e.g., Barth and Schmitt, 1991; Čokl, 2008; Cocroft et al., 2010; Elias et al., 2012; Henry et al., 2013; Derlink et al., 2014). Frequency range of insect vibrational signals is usually between 50 Hz and 5 kHz; however, most energy is limited to the frequency range below 500 Hz. The morphological and physiological characteristics of vibration receptors differ greatly among groups (reviewed in Barth, 1998; Brownell and van Hemmen, 2001; Taylor and Patek, 2010; Lakes-Harlan and Strauß, 2014). Arthropods are most sensitive to vibrations below 1 kHz and they can detect displacements in the range of 0.1 nm (e.g., Barth, 1998; Čokl et al., 2006; Eriksson et al., 2011;Lakes-Harlan and Strauß, 2014).
Importantly, besides the signaller's identity, vibrational signals also provide information that enables the receiver to determine in which direction the source of vibration is positioned and to locate the signaller (Barth, 1993; Virant-Doberlet et al., 2006; Hebets, 2008; Gibson and Cocroft, 2018; Prešern et al., 2018). At least in some cases, vibrational signals most likely also enable individuals to estimate the distance to the source (e.g., Lewis and Narins, 1985; Hill and Shadley, 2001; Gridi-Papp and Narins, 2010). In arthropods, the most important directional cues are time differences in a signal's arrival to spatially separated sensory inputs (i.e., vibroreceptors located in each leg) and differences in amplitude resulting from signal damping during propagation through the substrate (reviewed in Virant-Doberlet et al., 2006). Propagation velocity and attenuation depend on signal frequency and signals of lower frequency propagate more slowly and with less attenuation (Michelsen et al., 1982; Barth, 1998; Elias and Mason, 2014; Mortimer, 2017). Vibrational signals are therefore well adapted for transmission through the substrate, as well as creating relevant time delays that can be processed in the central nervous system. Even insects with body size around 1 cm can accurately locate the source of vibrational signals by processing time delays between 0.2 and 0.5 ms (Hager and Kirchner, 2014; Prešern et al., 2018).
Perhaps the most impressive example of versatility of vibrational modality has been described in the blind mole rat Nannospalax ehrenbergi. This species produces vibrational signals by striking the head against the tunnel roof and uses these self-generated substrate vibrations to assess the size, physical properties and location of an obstacle blocking its underground tunnel. It can than dig an optimal bypass tunnel around it (Kimchi and Terkel, 2003a,b;Kimchi et al., 2005).
Active Space of Vibrational Signals
The probability that the emitted signal is intercepted by eavesdroppers does not increase only with the number of potential receivers, but also with the distance the signal travels through the habitat. The active space (i.e., effective range) of vibrational signals has been defined as the “area in which the signal amplitude is sufficiently above the detection threshold of potential receivers to have an effect on their behavioral response” (Mazzoni et al., 2014; Šturm et al., 2019). Signal active space therefore depends, on the one hand, on intrinsic factors like the signal amplitude at the source (i.e., “loudness” of the signaller) and the sensitivity of a receiver's vibroreceptors, and, on the other hand, on environmental factors like attenuation of the signal during the transmission through the substrate and the amplitude of interfering background noise. Behavioral studies show that active space of vibrational signals broadly depends on the size of the animal and differs enormously, from a few cm in fruitflies (Mazzoni et al., 2013), to several km in elephants (Narins et al., 2016).
The amplitude of arthropod vibrational signals may be low at the source; however the sensitivity of their vibroreceptors is well above the amplitude of emitted signals (Michelsen et al., 1982). For example, the amplitude of vibrational signals emitted by the green stink bug Nezara viridula, recorded from the vibrating body and expressed as velocity is in the range 0.3–0.8 mm/s, while the threshold sensitivity of its receptors in the relevant frequency range is between 0.01 and 0.03 mm/s (Čokl et al., 2007). Nevertheless, the environment imposes severe constraints on the effective range of vibrational signals and, in contrast to air-borne sound, the size and shape of the active space are highly unpredictable.
One limitation is continuity of the substrate. It is generally assumed that for plant-dwelling animals the active space of vibrational signals is limited to the plant on which the animal is signaling. However, at least in meadows, the effective range also extends to the neighboring plants connected by roots and touching leaves (Čokl and Virant-Doberlet, 2003; Šturm et al., 2019) and even across a several-cm-wide air gap between overlapping leaves (Eriksson et al., 2011). Moreover, many small plant-dwelling insects increase the effective range of their vibrational signals using the behavioral strategy “fly/jump-call,” where they randomly move through the habitat and emit vibrational signals from different plants (e.g., Šturm et al., 2019). It should also be pointed out that the plant on which the animal is signaling is not necessarily a small herbaceous plant, but can also be a shrub or a tree, and in this case the active space of vibrational signals can extend up to 4 m even within one plant (McVean and Field, 1996; Barth, 2002b).
The effective range of vibrational signals is also limited by damping due to transmission properties of the substrate, which ultimately result in reduced amplitude (Mortimer, 2017). Moreover, heterogeneity of the natural substrates encountered in the habitat imposes unpredictable changes to signal structure observed in selective frequency filtering and temporal distortions (reviewed in Elias and Mason, 2014; Mortimer, 2017). Consequently, the longer the distance a vibrational signal travels from the source, the more likely it will be degraded to the extent that it cannot be recognizable by the receiver. Nevertheless, studies have shown that animal vibrational signals are tuned and adapted to specific transmission properties of their environment (host plants, leaf litter, soil) (Günther et al., 2004; Čokl, 2008; Hebets et al., 2008; McNett and Cocroft, 2008; Elias et al., 2010; Narins et al., 2016). Heterogeneity of the substrate can also affect availability and reliability of directional cues, since besides frequency, signal propagation velocity and attenuation, which create time delays and amplitude differences at sensory inputs, are strongly dependent on physical properties of the transmission medium (reviewed in Virant-Doberlet et al., 2006).
Studies of the natural vibroscape (i.e., vibrations emanating from a given landscape) show that the vibrational channel is dominated by frequencies below 1 kHz. This frequency range also includes the majority of arthropod vibrational signals used in communication (Šturm et al., 2019). In this frequency range, interfering background vibrational noise results from wind and rain (e.g., Barth et al., 1988; Caldwell et al., 2010b; McNett et al., 2010). Vertebrates and arthropods can distinguish incidental vibrations induced by wind and rain from incidental vibrational cues resulting from animal movements (e.g., Warkentin, 2005; Castellanos and Barbosa, 2006; Guedes et al., 2012). One known exception is the túngara frog Physalaemus pustulosus, which appears not to discriminate between vibrations used in intraspecific communication and incidental vibrations induced by rain (Halfwerk et al., 2016). Nevertheless, the frequency overlap of spectra of vibrational signals, vibroreceptor frequency sensitivity and frequency range of abiotic environmental noise indicates that the active space of vibrational signals is likely to be reduced. While it has been shown that wind-induced vibrations had a negative effect on frog and insect vibrational behavior (McNett et al., 2010; Hamel and Cocroft, 2012; Tishechkin, 2013; Halfwerk et al., 2016), current evidence suggests that some arthropod predators exploit background vibrational noise that impairs the ability of prey to detect incidental vibrational cues induced by their approach (Wilcox et al., 1996; Wignall et al., 2011; Soley, 2016).
In summary, laboratory and field studies show that animals live in a complex vibrational environment. The ongoing studies of the natural meadow vibroscape show that substrate vibrations are a constantly available, rich and reliable source of information (Šturm et al., 2019). The complexity of vibrational information available in the environment is best exemplified by the diversity of a vibrational community (i.e., the assemblage of animals in a particular habitat that produce vibrational signals and are active over a specified time). Assessment of species richness reflected in species-specific vibrational signals revealed that on a single plant the vibrational channel can be shared daily by more than 20 species (Figure 2).
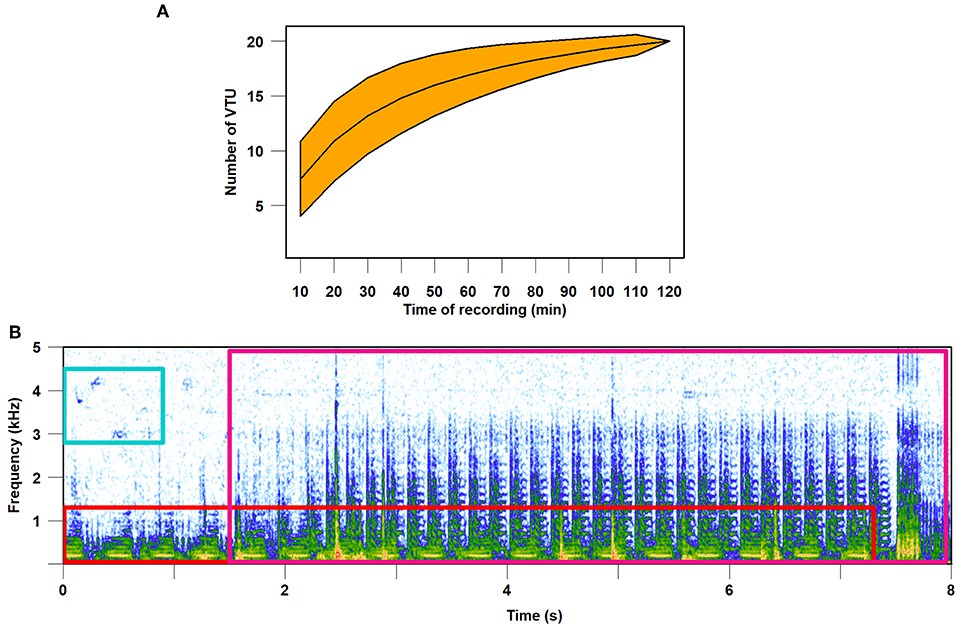
Figure 2. Complexity of meadow vibroscape. Vibrational signals were registered with a laser vibrometer on Carex hirta during 2-h (14:30–16:30) recording session on July 9, 2018. (A) Species accumulation curve of vibrational taxonomic units (VTU). VTU is equivalent to species and each 10 min of the recording was taken as a sample. Orange area around the curve indicates 95% confidence interval. The curve approaches 20 VTUs; however, it does not reach the plateau. (B) Visualization in the form of spectrogram of vibroscape, which includes overlapping vibrational signals of two unknown insect species (red and purple frames) and vibrational component of a bird air-borne song (blue frame).
Predator-Prey and Parasitoid-Host Interactions Guided by Substrate Vibrations
Taking into account that every movement of the body induces incidental vibrations in the substrate and that animals possess highly sensitive receptors to detect them, it is not surprising that one of the most widespread functions of substrate vibrations in interspecific interactions directly linked to survival and reproductive success is to avoid predators, capture prey or find the host to deposit eggs. In these contexts, detecting, identifying and locating the source is crucial. In this section we provide an overview of interactions guided by substrate vibrations that do not include eavesdropping on prey or host vibrational communication. Humans are aware of such interactions, since we can observe them in the field; either orb-weaving spiders approaching their struggling prey caught in the web, or crickets ceasing singing when they detect vibrations created by our footsteps.
Finding Prey or Host
Arthropod predators often rely on incidental vibrational cues to capture their prey. Spiders and scorpions locate their arthropod prey using incidental vibrations created by prey movements (reviewed in Barth, 1998, 2002b; Brownell and van Hemmen, 2001). Incidental vibrational cues induced by moving prey have, in general, lower amplitude than vibrational signals used in communication and also contain higher frequencies that are subject to stronger attenuation and higher propagation velocities (Barth et al., 1988). Nevertheless, spiders and scorpions can accurately orient toward and locate the source of such vibrations. Although both groups can also use amplitude cues to determine the location of the vibrational source (reviewed in Barth, 1998, 2002a; Brownell and van Hemmen, 2001), at least for scorpions, the more reliable directional cue is the difference in arrival times of a vibrational wave reaching receptors located in eight legs positioned on the substrate and they can detect time delays as short as 0.2 ms. The predatory stinkbug Podisus maculiventris (Hemiptera, Pentatomidae) reliably locates the source of vibrations created by chewing caterpillars (Pfannenstiel et al., 1995). The use of incidental vibrational cues to locate endophytic hosts appears to be widespread among parasitoid wasps (reviewed in Meyhöfer and Casas, 1999). However, wasp parasitoids of pupae locate their immobile hosts by using self-generated vibrations induced by tapping the substrate with modified antennae and detecting the echoes with enlarged subgenual vibration receptors in their legs (vibrational sounding) (reviewed in Broad and Quicke, 2000). It was suggested that wasps find the position of a hidden host by analyzing the contrast in resonance between hollow and solid sections of the substrate (Wäckers et al., 1998).
Antlion larvae (Neuroptera, Myrmeleontidae) wait for their prey at the bottom of funnel-shaped pit traps and their reaction to incidental vibrational cues generated by walking prey is to accurately toss the sand in its direction, in order to prevent its escaping from the sand pit (Fertin and Casas, 2009; Devetak, 2014). Moreover, studies show that antlions can associate a behaviourally neutral vibrational cue with the arrival of the prey and that such learning increases fitness by improving their digestive efficiency and, ultimately also decrease the time spent in the larval stage (Guillete et al., 2009; Hollis et al., 2011).
In arthropods, the use of substrate vibrations to catch the prey or find the host can also involve more complex behavioral strategies that do not include accurate location of the prey. The orb weaving spider Nephila pilipes uses incidental vibrational cues induced by prey caught in the web to assess the type of prey available in its environment and modify web architecture accordingly (Blamires et al., 2011). Araneophagic jumping spiders from the genus Portia produce deceptive vibrational signals to capture their prey (aggressive mimicry) (reviewed in Jackson and Cross, 2013). Portia is also well known for its cognitive abilities and uses a trial-and-error approach to generate vibrational signals that elicit appropriate responses from each prey spider species. The aggressive mimicry repertoire includes male vibrational courtship display of another jumping spider species (Jackson and Wilcox, 1990), incidental vibrations induced by prey caught in the web adapted according to the species and size of the spider prey (Jackson et al., 1998; Tarsitano et al., 2000; Jackson and Nelson, 2011), as well as female courtship display by subadult females to prey on conspecific males (Jackson and Cross, 2013). Similarly, the assassin bug Stenolemus bituberus (Hemiptera, Reduviiidae), which preys on web-building spiders, mimics incidental vibrations generated by struggling prey to attract the spider within striking distance (Wignall and Taylor, 2011). In playback experiments, the ambush bug Phymata crassipes (Hemiptera, Phymatidae) alternated with vibrational stimuli and imitated their duration (Gogala et al., 1984). The authors suggested that by imitation of heterospecific courtship vibrational signals this sit-and-wait predatory bug may attract their potential prey; however, this hypothesis was not tested. Acoustic signals emitted by pupae and larvae of the parasitic butterfly Maculinea rebeli mimic distinct sounds produced by queen ants, thus providing them superior treatment from workers (Barbero et al., 2009). Although these authors did not describe these stridulatory signals as substrate vibrations, it is likely that this social parasite-host communication is mediated by a vibrational component of these signals (DeVries et al., 1993), since most of the current evidence suggests that ants perceive only substrate-borne mechanical waves (Hunt and Richard, 2013; Golden and Hill, 2016).
Reliance on vibrational cues for finding prey is not limited to arthropods. Entomopathogenic nematodes use vibrational cues induced by insects moving in the soil to locate their hosts (Torr et al., 2004). In vertebrates, the use of vibrational information in prey detection has been documented in reptiles and mammals. The sandfish lizard Scincus scincus and sand viper Cerastes cerastes detect and locate their prey using incidental vibrations (Hetherington, 1989; Young and Morain, 2002). For the Namib Desert golden mole (Eremitalpa granti namibiensis) dune termites that live among the roots of grass are the principal insect prey. The blind and nocturnal golden mole relies on substrate vibrations to locate its food; however, for orientation it uses vibrations created by grass rattling in the wind, since these distinct vibrations with dominant frequency around 300 Hz transmit well through the sand and golden moles may detect them at distances of 20–25 m (Narins et al., 1997; Lewis et al., 2006). Golden moles also possess hypertrophied ossicles in the middle ear and such adaptation enables them better directional hearing in the frequency range up to 300 Hz (Mason and Narins, 2010; Narins et al., 2016).
Avoiding Enemies
While some insects appear to be able to walk near the predator without inducing typical incidental vibrations associated with locomotion (vibrocrypticity) (Barth et al., 1988), remaining still is probably the most common prey behavior in response to incidental vibrational cues arising from a potential enemy, since it also ceases to provide vibrational cues that reveal prey location (e.g., Meyhöfer et al., 1997; Djemai et al., 2001; Kojima et al., 2012a). Wolf spiders freeze in response to perceived presence of a predator and they are not only able to discriminate between incidental vibrations emanating from predators and non-predators, but also can perceive the substrate component of bird calls and use it to differentiate between threatening and non-threatening species (Lohrey et al., 2009; Sitvarin et al., 2016). Interestingly, non-predators can also exploit such a defense freezing response. Pupae of the soil-living beetle Trypoxylus dichotoma emit vibrational signals that mimic vibrations induced by a foraging mole to stop burrowing of the conspecific larvae in order to protect themselves from accidental damage to their pupal cell (Kojima et al., 2012a,b). Another form of passive defense is avoidance. Termites rely on avoiding ant predators by hiding behind clay walls and they monitor incidental vibrations generated by ant footsteps and discriminate them from incidental vibrational cues from other sources (Oberst et al., 2017).
Another common reaction is escape behavior that in arthropods includes dropping from the plant (e.g., Losey and Denno, 1998). Larvae of the moth Semiothisa aemulataria living on leaves escape invertebrate predators walking on the leaf by hanging from silk threads and they can differentiate between incidental vibrations induced by predator and non-predators or abiotic environmental sources, as well as adapt the length of the thread according to the identity of the predator (Castellanos and Barbosa, 2006). Similar behavior has been observed in treefrog embryos, where vibrational cues emanating from predator attack trigger early hatching and escape behavior from the egg clutch deposited on vegetation, and result in tadpoles falling into the water (Warkentin, 2005; Caldwell et al., 2010b). To avoid false alarms, embryos assess incidental vibrations to distinguish between lethal and benign sources. Earthworms emerge from soil in response to incidental vibrations resulting from digging moles; however, their escape is clearly directional away from the source of vibrations (i.e., a foraging mole) (Catania, 2008; Mitra et al., 2009).
Some animals use vibrational signals as part of an active defense strategy. Kangaroo rats footdrum in the presence of snakes, where drumming functions as a direct signal to the predator. In response to the vibrational component of drumming, snakes cease their stalking behavior (Randall, 2001, 2010). Vibrational signaling is often part of antipredator behavior in group-living insects (reviewed in Cocroft and Hamel, 2010). For example, when attacked by parasitoid wasps, an aphid colony produces coordinated collective kicking and twitching response that induces substrate vibrations. These substrate vibrations play a role in recruiting colony members, synchronizing a defense and potentially also repelling attackers (Hartbauer, 2010). However, besides synchronized signaling within a group, vibrational signaling as part of anti-predator protection has also been described in parent-offspring interactions (Cocroft, 1996; Ramaswamy and Cocroft, 2009; Hamel and Cocroft, 2012), as well as interactions between insects and their ant mutualists, where ant attendance increases the signaller's survival (e.g., Travassos and Pierce, 2000; Morales et al., 2008).
Alarm signals used to inform members of the group about danger can also be considered as a part of defense behavior. Vibrational signals that function to warn conspecifics have been described in termites (e.g., Rosengaus et al., 1999; Hager and Kirchner, 2013; Delattre et al., 2015) and elephants (O'Connell-Rodwell et al., 2007). At present, it is not clear whether footdrumming alarm signals emitted by many mammals in the presence of a predator are perceived as air-borne sound or substrate vibrations, or both (Randall, 2001, 2010).
Eavesdropping Vibrational Communication Networks
Eavesdropping in animal communication is defined as “the use of information in signals by individuals other than the primary target” (Peake, 2005) and eavesdropping network applies to situations where, the receiver eavesdrops on the signaling interaction in which it is not directly involved to obtain information (Burt and Vehrencamp, 2005). Due to their reliance on vibrational signals in many intraspecific and interspecific interactions and their highly sensitive vibration receptors, arthropods are the best group to study eavesdropping vibrational communication networks. Besides exploitation of vibrational signaling in the context of sexual communication by enemies (predators, parasitoids), eavesdropping applies also to other interactions like intraspecific competition (Virant-Doberlet et al., 2014).
Intraspecific Eavesdropping
In many species relying on vibrational communication, a coordinated reciprocal exchange of male and female vibrational signals is essential for recognition and successful location of the female (e.g., Derlink et al., 2014; Polajnar et al., 2014; Kuhelj et al., 2015a; Lujo et al., 2016). In leafhoppers (Hemiptera, Cicadellidae), communication is mediated exclusively via substrate vibrations and the male-female vibrational duet appears to be easily exploited by intruding males that silently approach a female duetting with the calling male (Mazzoni et al., 2009a,b; Kuhelj and Virant-Doberlet, 2017). The most important factor in obtaining the female in such a competitive setting appears to be the ability to locate the female before the rival. Evidence shows that intruding eavesdropping exploiters are at a competitive advantage. In the leafhopper Aphrodes makarovi, each exchange of male and female vibrational signals is initiated by the male and his walking, associated with the search for the replying stationary female, is limited to the duration of her reply (Kuhelj et al., 2016). In a competitive setting, that included two males and a female, the winners (i.e., males that mated with the female) were males that were better at exploiting female replies emitted in response to the rival's triggering call (i.e., in comparison with their competitors, they more often searched for the female during her reply to the rival's call) (Kuhelj and Virant-Doberlet, 2017). In this species, male signaling effort is negatively correlated with longevity (Kuhelj et al., 2015b) and eavesdropping on a male-female duet maintained by another male allows exploiters to invest less in energetically demanding signaling and to survive longer. In this context, it should be noted, that in the threehopper Enchenopa binotata, which also relies on vibrational communication, longevity was the strongest predictor of male lifetime mating success (Sullivan-Beckers and Cocroft, 2010).
Although the role of intraspecific eavesdropping and exploitation of vibrational signaling in the context of sexual communication has only rarely been systematically studied, current evidence suggests that, at least in mating systems based on stereotyped vibrational duets, this may be a widespread strategy to increase male mating success. It may also have important implications for sexual selection and evolution of vibrational communication. In general, in communication systems in which eavesdroppers exploit female replies to signals emitted by another male, males maintaining a duet have no advantage over males exploiting it, since information about the identity and location contained in the female reply is available to all males present in the active space of female signals. So far, in systems based on substrate-borne vibrations, in the perceived presence of an eavesdropping intruding male, the only observed defensive tactic displayed by the calling male was to emit distinct masking signals overlapping the last part of the female reply emitted in response to their own triggering call (Kuhelj et al., 2016; Kuhelj and Virant-Doberlet, 2017). Although the adaptive significance of these masking vibrational signals is not clear, the most likely benefit results from preventing the eavesdropper from locating the female by confounding him with the second vibrational source.
Eavesdropping by Enemies
The fact that we cannot observe by chance predators and parasitoids eavesdropping and exploiting vibrational communication in the field probably underlies our long persisting belief that enemies ignore vibrational signals used by their prey or host in intraspecific communication (Cocroft and Rodríguez, 2005; Cocroft, 2011; Virant-Doberlet et al., 2011, 2014). Arthropod predator-prey interactions usually involve small animals and take place in thick vegetation. Moreover, even when we notice predation, we are not aware that the predator may be exploiting prey intraspecific vibrational communication due to our inadequate perception of substrate vibrations. In previous sections, we reviewed the evidence that in the vibrational channel there are ample opportunities for eavesdropping and that many animals rely on information provided by incidental vibrations to guide their predator-prey interactions. Here below, we focus on the evidence that enemies also exploit prey or host vibrational signaling.
Laboratory studies showed that in the absence of other cues the egg parasitoid Telenomous podisi (Hymenoptera: Scelionidae) is selectively attracted to species- and sex-specific female vibrational song of its preferred host, the stink bug Euschistus heros (Laumann et al., 2007, 2011). This continuously emitted song composed of repetitive low-frequency pulses provides a reliable directional cue to locate a stationary female. While searching behavior mediated by female vibrational signals may increase the probability of finding egg masses on the same plant, it seems likely that host location is guided by multimodal cues that also include vision and volatile chemical compounds (e.g., Michereff et al., 2016).
Spiders have highly sensitive vibroreceptors and prey detection is commonly mediated by substrate vibrations (Uetz, 1992; Barth, 1998; Roberts et al., 2007). While Morris et al. (1994) suggested that in the Ecuadorean rainforest katydids use low frequency vibrational signaling instead of ultrasonic air-borne sounds in order to avoid eavesdropping by bats, they also observed that many spiders were catching them. Anecdotal evidence from the field and unpublished laboratory results also indicated that jumping spiders may use leafhopper vibrational signals to locate their prey (Narhardiyati and Bailey, 2005). A laboratory study also showed that vibrational signals incorporated into the multimodal courtship display of the wolf spider Schizocosa ocreata increased detectability to its predator jumping spider Phidippus clarus (Roberts et al., 2007).
Direct evidence that spider foraging behavior is influenced by prey vibrational signals used in sexual communication was obtained in the study of predator-prey interactions between the spider Enoplognatha ovata (Theridiidae) and the leafhopper A. makarovi (Virant-Doberlet et al., 2011). Using molecular diagnostics to identify A. makarovi DNA in the E. ovata gut, authors were able to show that in the field predation rate on leafhoppers was significantly higher when signaling adults were present. At that time 25% of spiders were feeding on them. Microcosm and playback experiments showed that spiders caught significantly more males than females and that spider residence time was significantly longer only when a plant was vibrated with the male call. Although taken together results suggest that E. ovata spiders exploit prey vibrational signaling primarily to obtain information about prey availability, in response to playback of A. makarovi male calls some spiders located the source of vibrations and authors suggested that differences observed in behavior of individual spiders may be attributed to learning and previous experience of A. makarovi signals in the field. Importantly, molecular diagnostics also showed that only a few spider species found at the study site were feeding on A. makarovi and that Pardosa wolf spiders, which were not consuming them in the field, were also ignoring live leafhoppers in microcosms, as well as their vibrational signals in playback experiments.
Avoiding Eavesdropping Enemies
So far, various interactions within vibrational communication network have been studied in more detail only in the leafhopper A. makarovi. With our current knowledge we cannot distinguish trade-offs and adaptive behavioral strategies that arise from selection pressure imposed by eavesdropping predators (Virant-Doberlet et al., 2011) from selection resulting from eavesdropping intraspecific competitors (Kuhelj and Virant-Doberlet, 2017), biotic noise and competition for signaling space in the vibrational channel (Šturm et al., 2019), as well as sexual selection and indirect costs due to energetically demanding signaling (Kuhelj et al., 2015b, 2016). This is particularly so, since selection pressures from different sources can reinforce or oppose each other. However, here below, we discuss some potential behavioral strategies in leafhoppers that may also serve as a defense against eavesdropping predators.
Taking into account the diversity and density of potential predators in the habitat that exploit leafhopper vibrational signaling, as well as unpredictability in the structure of the predator community at each individual location, it is likely that leafhoppers' behavioral anti-predator strategies would not be adapted to specific predator species and their foraging strategies. The rich and complex vibroscape perceived by predators may provide general information about the food availability important for choosing a good foraging site, as well as directional cues to locate the signallers, depending on the foraging strategy (e.g., Uetz, 1992; Persons and Uetz, 1996). As our own observations in the field show, spider webs attached to several plants also enable the resident spider to eavesdrop on vibrational signals emitted by their potential prey signaling on these plants (Figure 3).
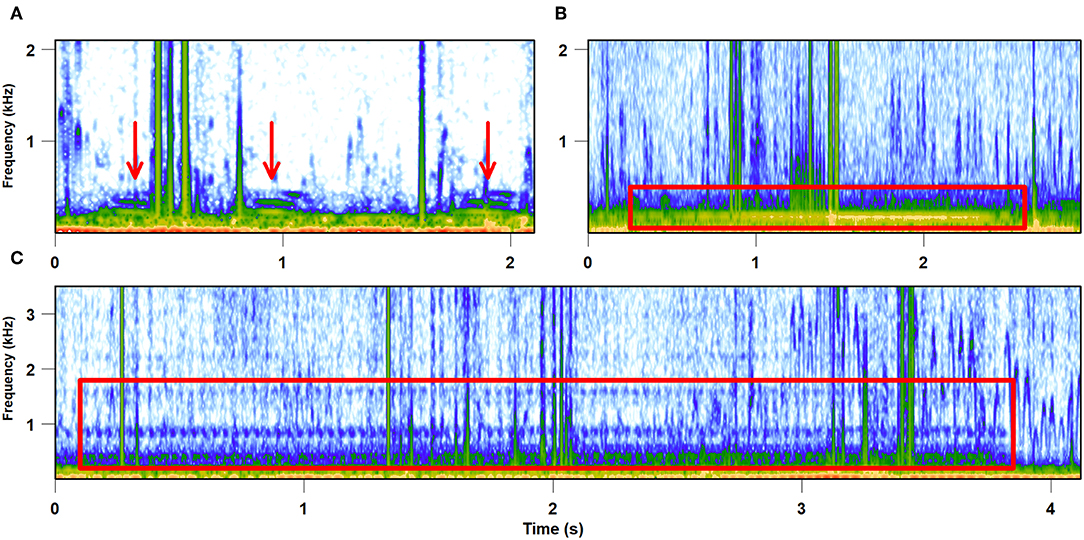
Figure 3. Vibrational signals recorded from spider web. Vibrations were recorded on July 27, 2017 with a laser vibrometer from the web of Argiope bruennichi built between several grass stems. (A,B) Vibrational signals of two unknown species, (C) male advertisement call of the leafhopper Aphrodes makarovi.
The most obvious strategy to avoid enemies eavesdropping on sexual communication would be to emit as few and as quiet vibrational signals as possible without compromising the goal of reliably and quickly finding a suitable partner. In contrast to the majority of mating systems based on air-borne sound communication, where males produce continuous songs and silent females approach them, in mating systems based on substrate-borne vibrations partners exchange signals and males approach stationary females (e.g., Bailey, 2003; Derlink et al., 2014; Polajnar et al., 2014; Gibson and Cocroft, 2018). Duetting, in which both partners coordinate and modify their vibrational signals and behavior according to the partner's reply, is also a communication strategy that enables high signaling activity only at times when a partner is actually present. In the leafhopper Scaphoideus titanus the male vibrational repertoire aimed at a female includes several signals. The male emits short and structurally simple calls during the call-fly stage and during the searching phase and starts emitting longer and more complex courtship phrase only after he arrives on the leaf where the female is located (Polajnar et al., 2014). In A. makarovi, the long and structurally complex male call is the only vibrational signal directed to the female; however, male calling rate is low during the call-fly stage and increases only after a male receives the female reply (Kuhelj et al., 2015b). After vibrational contact is established, higher calling rate is advantageous and has significant positive effects on the probability to locate a female quickly.
In leafhoppers, pair formation includes male call-fly behavior, as well as a more localized search for the replying female. Hence high predation risk may also result from increased probability of encounters with predators while moving through the habitat. Males can also reduce predation risk by modifying their movements in the perceived presence of the predators. In the treehopper E. binotata, male behavioral response to the presence of spider silk was to reduce call-fly behavior but not their calling rate (Fowler-Finn et al., 2014).
In general, males are easier to detect than females, because they advertise themselves over a longer period of time, with more conspicuous signals of high amplitude (Zuk and Kolluru, 1998; Haynes and Yeargan, 1999). However, in duetting systems females are potentially equally exposed to eavesdropping predators as males, not only by proximity to the signaling male, but also because they remain stationary during vibrational exchange and therefore might be easier to locate. Virant-Doberlet et al. (2011) showed that in microcosms predation rate on A. makarovi females was also significant. In this study, the natural amplitude ratio between the male call and the female reply remained unchanged. Therefore, the male call which was more effective in increasing residence time of E. ovata predators in playback experiments, had higher amplitude. In this species, female vibrational replies can be even longer than male calls; however, their amplitude is always lower (Kuhelj et al., 2016). In this context, the observation that a female mates the first male that locates her may also be a strategy to reduce the duration of exposure to predators.
So far, confounding the conspecific competitors exploiting male-female duets appears to be the only observed defense against eavesdropping (Kuhelj et al., 2016; Kuhelj and Virant-Doberlet, 2017). Duet structures described in some leafhoppers, in which male and female signals share temporal and spectral characteristics and are partly overlapping, or female replies appear randomly among similar elements in male vibrational signals (Mazzoni et al., 2009a; Kuhelj et al., 2016), probably result in difficulties in locating the signallers. In a basically one-dimensional vibrational environment on the plant (de Groot et al., 2011), an eavesdropping exploiter may perceive such duets as one compound vibrational signal arriving from two spatially separated sources and from different directions.
Conclusions and Suggestions for Further Studies
Despite increased interest in vibrational communication in the last decade and the progress we made in our perception and understanding of this communication modality, vibrational signaling still remains a poorly known and understood mode of communication (Cocroft et al., 2014; Endler, 2019). As it is obvious from this review, vibrational signaling is far from being a safe communication channel, inaccessible to enemies. It is evolutionarily older and more widespread than air-borne sound communication and highly sensitive receptors detecting substrate vibrations are common. Although active space of vibrational signals is undoubtedly more complex and unpredictable than in air-borne sounds, animal vibrational signals are, in general, well adapted to their natural environment and frequency sensitivity of their receptors. As the first studies of the vibroscape show, substrate vibrations are a readily available and reliable source of information, both in intraspecific communication and in predator-prey interactions. Long evolutionary history of this communication channel is also reflected in the breadth of varied predator-prey interactions guided by substrate-borne vibrations. However, our own perceptional bias in favor of air-borne sound communication still hampers our understanding of challenges that animals relying on vibrational signals are facing in their environment. Eavesdropping and exploitation of vibrational signals used in sexual communication have been so far largely neglected; although, it is likely they are major drivers in the evolution taking place in the vibratory world.
What is urgently needed, are more studies on different model species in different environments, since the only existing study providing more comprehensive insight into exploitation of vibrational signaling included one prey species at a single field location (Virant-Doberlet et al., 2011). While leafhoppers provide an excellent model for studying predator-prey interactions within the vibratory world, since their communication is based exclusively on vibrational signals, we should also bear in mind that production of air-borne insect songs also creates a vibrational component of these signals (Caldwell, 2014). In order to establish that exploitation by eavesdropping predators can influence the evolution of vibrational signals, as well as signaling and searching behavior, it is not enough to observe that predation occurs. We must also determine that predation during the mating period occurs frequently enough to be a significant source of mortality and that there is a positive relationship between signaling and risk of predation (Kotiaho et al., 1998; Virant-Doberlet et al., 2011). Moreover, such studies should also be carried out under relevant ecological conditions. As existing data show, when focusing on arthropod predator-prey interactions, it should be also taken into account that only a subset of suitable predators may prey on a particular species (Virant-Doberlet et al., 2011) and that predation behavior can show geographic variation (Jackson and Carter, 2001). Furthermore, experience and learning are also likely to influence prey preference and such selective attention can be formed after a single encounter (Jackson and Li, 2004). We also need to ask whether spiders learn vibrational signals of locally abundant prey species or respond to signal characteristics that are common across many prey species (Cocroft, 2011; Virant-Doberlet et al., 2011). To bridge some gaps in our understanding of vibrational modality, we should also focus on how predators and prey perceive the vibratory world they share. Recent studies showing that plants can also respond to substrate vibrations induced by insects, provide additional evidence about the interconnected complexity of vibrational interactions in nature (Appel and Cocroft, 2014; Veits et al., 2019). Taking into account the long evolutionary history of vibrational communication, we have no doubt that studies of interspecific interactions guided by substrate vibrations will in the future offer numerous opportunities to unravel mechanisms that are central to our understanding behavior in general.
Author Contributions
All authors listed have made a substantial, direct and intellectual contribution to the work, and approved it for publication.
Funding
We received financial support from the Slovenian Research Agency (Javna Agencija za raziskovalno dejavnost RS) (research core funding no. P1-0255, research project J1-8142, postdoc research project Z1-8144 awarded to AK and Ph.D. fellowship awarded to RŠ).
Conflict of Interest Statement
The authors declare that the research was conducted in the absence of any commercial or financial relationships that could be construed as a potential conflict of interest.
Acknowledgments
We would like to thank Ximena Bernal and Rachel Page for an invitation to contribute to the symposium How enemies shape communication systems at the 2018 ABS meeting, as well as to Frontiers Research Topics. We would also like to thank all our colleagues, past and present, who over the years shared with us the interest in research of vibrational communication. Our sincere thanks also go to numerous other researchers, whose papers we re-read in preparation of this review, since without their dedicated work and insight such a review would not be possible. Unfortunately, we could not cite all of your excellent studies. We are grateful to Peggy Hill who helped to improve upon the manuscript. Last but not least, we gratefully acknowledge the support and patience of the editors.
References
Appel, H. M., and Cocroft, R. B. (2014). Plants respond to leaf vibrations caused by insect herbivore chewing. Oecologia 175, 1257–1266. doi: 10.1007/s00442-014-2995-6
Bailey, W. J. (2003). Insect duets: underlying mechanisms and their evolution. Physiol. Entomol. 28, 157–174. doi: 10.1046/j.1365-3032.2003.00337.x
Barbero, F., Thomas, J. A., Bonelli, S., Balletto, E., and Schönrogge, K. (2009). Queen ants make distinctive sounds that are mimicked by a butterfly social parasite. Science 323, 782–785. doi: 10.1126/science.1163583
Barnett, K. E., Cocroft, R. B., and Fleishman, L. J. (1999). Possible communication by substrate vibration in a chameleon. Copeia 1, 225–228. doi: 10.2307/1447408
Barth, F. G. (1993). Sensory guidance in spider pre-copulatory behaviour. Comp. Biochem. Physiol. 104A, 717–733. doi: 10.1016/0300-9629(93)90148-W
Barth, F. G. (1998). “The vibrational sense in spiders,” in Comparative Hearing: Insects, eds R. R. Hoy, A. N. Popper, and R. R. Fay (New York, NY: Springer Verlag), 228–278.
Barth, F. G. (2002a). Spider senses – technical perfection and biology. Zoology 105, 271–285. doi: 10.1078/0944-2006-00082
Barth, F. G., Bleckmann, H., Bohnenberger, J., and Seyfarth, E.-A. (1988). Spiders of genus Cupiennius Simon 1891 (Araneae, Ctenidae). II. On the vibratory environment of a wandering spider. Oecologia 77, 194–201. doi: 10.1007/BF00379186
Barth, F. G., and Schmitt, A. (1991). Species recognition and species isolation in wandering spiders (Cupiennius spp.; Ctenidae). Behav. Ecol. Sociobiol. 29, 333–339. doi: 10.1007/BF00165957
Belwood, J. J., and Morris, G. K. (1987). Bat predations and its influence on calling behaviour in neotropical katydids. Science 238, 64–67. doi: 10.1126/science.238.4823.64
Bishop, A. M., Denton, P., Pomeroy, P., and Twiss, S. (2015). Good vibrations by the beach boys: magnitude of substrate vibrations is a reliable indicator of male grey seal size. Anim. Behav. 100, 74–82. doi: 10.1016/j.anbehav.2014.11.008
Blamires, S. J., Chao, Y.-C., Liao, C.-P., and Tso, I.-M. (2011). Multiple prey cues induce foraging flexibility in a trap-building predator. Anim. Behav. 81, 955–961. doi: 10.1016/j.anbehav.2011.01.022
Bradbury, J. W., and Vehrencamp, S. L. (1998). Principles of Animal Communication. Sunderland: Sinauer.
Brisben, A. J., Hsiao, S. S., and Johnson, A. K. (1999). Detection of vibrations transmitted through an object grasped in the hand. J. Neurophysiol. 81, 1548–1558. doi: 10.1152/jn.1999.81.4.1548
Broad, G. R., and Quicke, D. L. J. (2000). The adaptive significance of host location by vibrational sounding in parasitoid wasps. Proc. R. Soc. B 267, 2403–2409. doi: 10.1098/rspb.2000.1298
Brownell, P. H., and van Hemmen, J. L. (2001). Vibration sensitivity and a computational theory for prey-localizing behavior in sand scorpions. Am. Zool. 41, 1229–1240. doi: 10.1093/icb/41.5.1229
Burt, J. M., and Vehrencamp, S. L. (2005). “Dawn chorus as an interactive communication network,” in Animal Communication Networks, ed P. K. McGregor (Cambridge: Cambridge University Press), 320–343.
Caldwell, M. S. (2014). “Interactions between airborne sound and substrate vibration in animal communication,” in Studying Vibrational Communication, eds R. B. Cocroft, M. Gogala, P. S. M. Hill, and A. Wessel (Berlin: Springer Verlag), 65–92.
Caldwell, M. S., Johnston, G. R., McDaniel, J. G., and Warkentin, K. M. (2010a). Vibrational signalling in the agonistic interactions of red-eyed treefrogs. Curr. Biol. 20, 1012–1017. doi: 10.1016/j.cub.2010.03.069
Caldwell, M. S., McDaniel, J. G., and Warkentin, K. M. (2010b). Is it safe? Red-eyed treefrog embryos assessing predation risk use two features of rain vibrations to avoid false alarms. Anim. Behav. 79, 255–260. doi: 10.1016/j.anbehav.2009.11.005
Castellanos, I., and Barbosa, P. (2006). Evaluation of predation risk by a caterpillar using substrate-borne vibrations. Anim. Behav. 72, 461–469. doi: 10.1016/j.anbehav.2006.02.005
Catania, K. C. (2008). Worm grunting, fiddling and charming-humans unknowingly mimic a predator to harvest bait. PLoS ONE 3:e3472. doi: 10.1371/journal.pone.0003472
Cocroft, R. B. (1996). Insect vibrational defence signals. Nature 382, 679–680. doi: 10.1038/382679a0
Cocroft, R. B. (2011). The public world of insect vibrational communication. Mol. Ecol. 20, 2041–2043. doi: 10.1111/j.1365-294X.2011.05092.x
Cocroft, R. B., Gogala, M., Hill, P. S. M., and Wessel, A. (2014). “Fostering research progress in a rapidly growing field,” in Studying Vibrational Communication, eds R. B. Cocroft, M. Gogala, P. S. M. Hill, and A. Wessel (Berlin: Springer Verlag), 3–12.
Cocroft, R. B., and Hamel, J. A. (2010). “Vibrational communication in the ‘other societies': a diversity of ecology, signals and signal functions,” in The use of Vibrations in Communication: Properties, Mechanisms and Function Across Taxa, ed C. E. O'Connell-Rodwell (Thiruvananthapuram: Research Signpost), 47–68.
Cocroft, R. B., and Rodríguez, R. L. (2005). The behavioral ecology of insect vibrational communication. BioScience 55, 323–334. doi: 10.1641/0006-3568(2005)055[0323:TBEOIV]2.0.CO;2
Cocroft, R. B., Rodríguez, R. L., and Hunt, R. E. (2010). Host shifts and signal divergence: mating signals covary with host use in a complex of specialized plant-feeding insects. Biol. J. Linn. Soc. 99, 60–72. doi: 10.1111/j.1095-8312.2009.01345.x
Čokl, A. (2008). Stink bug interaction with host plants during communication. J. Insect Physiol. 54, 1113–1124. doi: 10.1016/j.jinsphys.2008.06.004
Čokl, A., and Virant-Doberlet, M. (2003). Communication with substrate-borne signals in small plant-dwelling insects. Annu. Rev. Entomol. 48, 29–50. doi: 10.1146/annurev.ento.48.091801.112605
Čokl, A., Virant-Doberlet, M., and Zorović, M. (2006). “Sense organs involved in the vibratory communication of bugs,” in Insects Sounds and Communication: Physiology, Behaviour, Ecology and Evolution, eds M. F. Claridge and S. Drosopoulos (Boca Raton, FL: Taylor and Francis), 71–80.
Čokl, A., Zorović, M., and Millar, J. G. (2007). Vibrational communication along plants by stink bugs Nezara viridula and Murgantia histrionica. Behav. Processes 75, 40–54. doi: 10.1016/j.beproc.2007.01.003
Cooley, J. R. (2001). Long-range acoustic signals, phonotaxis and risk in the sexual pair-forming behaviors of Okanagana canadensis and O. rimosa (Hemiptera: Cicadidae). Ann. Entomol. Soc. Am. 94, 755–760. doi: 10.1603/0013-8746(2001)094[0755:LRASPA]2.0.CO;2
de Groot, M., Čokl, A., and Virant-Doberlet, M. (2011). Species identity cues: possibilities for errors during vibrational communication on plant stems. Behav. Ecol. 22, 1209–1217. doi: 10.1093/beheco/arr115
Delattre, O., Sillam-Dussès, D., Jandák, V., Brothánek, M., Rücker, K., Bourguignon, T., et al. (2015). Complex alarm strategy in the most basal termite species. Behav. Ecol. Sociobiol. 69, 1945–1955. doi: 10.1007/s00265-015-2007-9
Derlink, M., Pavlovčič, P., Stewart, A. J. A., and Virant-Doberlet, M. (2014). Mate recognition in duetting species: the role of male and female vibrational signals. Anim. Behav. 90, 181–193. doi: 10.1016/j.anbehav.2014.01.023
Devetak, D. (2014). “Sand-borne vibrations in prey detection and orientation of antlions,” in Studying Vibrational Communication, eds R. B. Cocroft, M. Gogala, P. S. M. Hill, and A. Wessel (Berlin: Springer Verlag), 319–330.
DeVries, P. J., Cocroft, R. B., and Thomas, J. (1993). Comparison of acoustical signals in Maculinea butterfly caterpillars and their obligate host Myrmica ants. Biol. J. Linn. Soc. 49, 229–238. doi: 10.1111/j.1095-8312.1993.tb00902.x
Djemai, I., Casas, J., and Magal, C. (2001). Matching host reactions to parasitoid wasp vibrations. Proc. R. Soc. B. 268, 2403–2408. doi: 10.1098/rsbp.2001.1811
Elias, D. O., Maddison, W. P., Peckmezian, C., Girad, M. B., and Mason, A. C. (2012). Orchestrating the score: complex multimodal courtship in the Habronattus coecatus group of Habronattus jumping spiders. Biol. J. Linn. Soc. 105, 522–547. doi: 10.1111/j.1095-8312.2011.01817.x
Elias, D. O., and Mason, A. C. (2014). “The role of wave and substrate heterogeneity in vibratory communication; practical issues in studying the effect of vibratory environment in communication,” in Studying Vibrational Communication, eds R. B. Cocroft, M. Gogala, P. S. M. Hill, and A. Wessel (Berlin: Springer Verlag), 215–247.
Elias, D. O., Mason, S. A. C., and Hebets, E. A. (2010). A signal-substrate match in the substrate-borne component of a multimodal courtship display. Curr. Zool. 56, 370–378.
Endler, J. A. (2014). “The emerging field of tremology,” in Studying Vibrational Communication, eds R. B. Cocroft, M. Gogala, P. S. M. Hill, and A. Wessel (Berlin: Springer Verlag), VII-X.
Endler, J. A. (2019). “Biotremology and sensory ecology,” in Biotremology–Studying Vibrational Behaviour, eds P. S. M. Hill, R. Lakes-Harlan, V. Mazzoni, P. M. Narins, M. Virant-Doberlet, and A. Wessel (Berlin: Springer Verlag). Available online at: https://www.springer.com/gp/book/9783030222925
Eriksson, A., Anfora, G., Lucchi, A., Virant-Doberlet, M., and Mazzoni, V. (2011). Inter-plant vibrational communication in a leafhopper insect. PLoS ONE 6:e19692. doi: 10.1371/journal.pone.0019692
Fertin, A., and Casas, J. (2009). Orientation towards prey in antlions: efficient use of wave propagation in sand. J. Exp. Biol. 210, 3337–3343. doi: 10.1242/jeb.004473
Fowler-Finn, K. D., Al-Wathiqui, N., Cruz, D., Al-Wathiqui, M., and Rodríguez, R. L. (2014). Male Enchenopa binotata treehoppers (Hemiptera: Membracidae) vary mate-searching behavior but not signalling behavior in response to spider silk. Naturwissenschafen 101, 211–220. doi: 10.1007/s00114-014-1145-7
Gibson, J. S., and Cocroft, R. B. (2018). Vibration-guided mate searching in treehoppers: directional accuracy and sampling strategies in a complex sensory environment. J. Exp. Biol. 221:jeb175083. doi: 10.1242/jeb.175083
Gogala, M., Virant, M., and Blejec, A. (1984). Mocking bug Phymata crassipes (Heteroptera). Acoust. Lett. 8, 44–51.
Golden, T. M. J., and Hill, P. S. M. (2016). The evolution of stridulatory communication in ants, revisited. Insect. Soc. 63, 309–319. doi: 10.1007/s00040-016-0470-6
Gridi-Papp, M., and Narins, P. M. (2010). “Seismic detection and communication in amphibians,” in The use of Vibrations in Communication: Properties, Mechanisms and Function Across Taxa, ed C. E. O'Connell-Rodwell (Thiruvananthapuram: Research Signpost), 69–83.
Guedes, R. N., Matheson, S. M., Frei, B., Smith, M. L., and Yack, J. E. (2012). Vibration detection and discrimination in the masked birch caterpillar (Drepana arcuata). J. Comp. Physiol. A 198, 325–335. doi: 10.1007/s00359-012-0711-8
Guillete, L. M., Hollis, K. L., and Markatian, A. (2009). Learning in a sedentary insect predator: antlions (Neuroptera: Myrmeleodontidae) anticipate long wait. Behav. Processes 80, 224–232. doi: 10.1016/j.beproc.2008.12.015
Günther, R. H., O'Connell-Rodwell, C. E., and Klemperer, S. L. (2004). Seismic waves from elephant vocalizations: a possible communication mode. Geophys. Res. Lett. 31, 1–4. doi: 10.1029/2004GL019671
Hager, F. A., and Kirchner, W. H. (2013). Vibrational long-distance communication in the termites Macrotermes natalensis and Odontotermes sp. J. Exp. Biol. 216, 3249–3256. doi: 10.1242/jeb.086991
Hager, F. A., and Kirchner, W. H. (2014). Directional sensing in the termite Macrotermes natalensis. J. Exp. Biol. 217, 2526–2530. doi: 10.1242/jeb.103184
Halfwerk, W., Ryan, M. J., and Wilson, P. S. (2016). Wind- and rain-induced vibrations impose different selection pressures on multimodal signalling. Am. Nat. 188, 279–288. doi: 10.1086/687519
Hamel, J. A., and Cocroft, R. B. (2012). Negative feedback from maternal signals reduces false alarms by collectively signalling offspring. Proc. R. Soc. B 279, 3820–3826. doi: 10.1098/rspb.2012.1181
Hartbauer, M. (2010). Collective defense of Aphis nerii and Uroleucon hypochoeridis (Homoptera, Aphididae) against natural enemies. PLoS ONE 5:e10417. doi: 10.1371/journal.pone.0010417
Haynes, K. F., and Yeargan, K. V. (1999). Exploitation of intraspecific communication system: illicit signallers and receivers. Ann. Entomol. Soc. Am. 92, 960–970. doi: 10.1093/aesa/92.6.960
Hebets, E. A. (2008). Seismic signal dominance in the multimodal courtship display of the wolf spider Schizocosa stridulans Stratton 1991. Behav. Ecol. 19, 1250–1257. doi: 10.1093/beheco/arn080
Hebets, E. A., Elias, D. O., Mason, A. C., Miller, G. L., and Stratton, G. E. (2008). Substrate-dependent signalling success in the wolf spider Schizocosa retrosa. Anim. Behav. 75, 605–615. doi: 10.1016/j.anbehav.2007.06.021
Henry, C. S. (1994). Singing and cryptic speciation in insects. Trends Ecol. Evol. 9, 388–392. doi: 10.1016/0169-5347(94)90061-2
Henry, C. S., Brooks, S. J., Duelli, P., Johnson, J. B., Wells, M. M., and Mochizuki, A. (2013). Obligatory duetting behaviour in the Chrysoperla carnea-group of cryptic species (Neuroptera: Chrysopidae): its role in shaping evolutionary history. Biol. Rev. 88, 787–808. doi: 10.1111/brv.12027
Hetherington, T. E. (1989). Use of vibratory cues for detection of insect prey by the sandswimming lizard Scincus scincus. Anim. Behav. 37, 290–297. doi: 10.1016/0003-3472(89)90118-8
Hill, P. S. (2009). How do animals use substrate-borne vibrations as an information source? Naturwissenschaften 96, 1355–1371. doi: 10.1007/s00114-009-0588-8
Hill, P. S., and Wessel, A. (2016). Biotremology. Curr. Biol. 26, R181–191. doi: 10.1016/j.cub.2016.01.054
Hill, P. S. M., and Shadley, J. R. (2001). Talking back: sending soil vibration signals to lekking prairie mole cricket males. Am. Zool. 41, 1200–1214. doi: 10.1093/icb/41.5.1200
Hill, P. S. M., Virant-Doberlet, M., and Wessel, A. (2019). “What is biotremology?,” in Biotremology–Studying Vibrational Behaviour, eds P. S. M. Hill, R. Lakes-Harlan, V. Mazzoni, P. M. Narins, M. Virant-Doberlet, and A. Wessel (Berlin: Springer Verlag). Available online at: https://www.springer.com/gp/book/9783030222925
Hollis, K. L., Cogswell, H., Snyder, K., Guillette, L. M., and Nowbahari, E. (2011). Specialized learning in antlions (Neuroptera: Myrmeleontidae), pit-digging predators, shortens vulnerable larval stage. PLoS ONE 6:e17958. doi: 10.1371/journal.pone.0017958
Hughes, N. K., Kelley, J. L., and Banks, P. B. (2012). Dangerous liaisons: the predation risk of receiving social signals. Ecol. Lett. 15, 1326–1339. doi: 10.1111/j.1461-0248.2012.01856.x
Hunt, J. H., and Richard, F.-J. (2013). Intracolony vibroacoustic communication in social insects. Insect. Soc. 60, 403–417. doi: 10.1007/s00040-013-0311-9
Jackson, R. R., and Carter, C. M. (2001). Geographic variation in reliance on trial-and-error signal derivation by Portia labiata, an araneophagic jumping spider from Philippines. J. Insect. Behav. 14, 799–827. doi: 10.1023/A:1013041501584
Jackson, R. R., and Cross, F. R. (2013). A cognitive perspective on aggressive mimicry. J. Zool. 290, 161–171. doi: 10.1111/jzo.12036
Jackson, R. R., and Li, D. (2004). One-encounter, search-image formation by araneophagic spiders. Anim. Cogn. 7, 247–254. doi: 10.1007/s10071-004-0219-x
Jackson, R. R., Li, D., Fijn, N., and Barrion, A. (1998). Predator-prey interactions between aggressive mimic jumping spiders (Salticidae) and araneophagic spitting spiders (Scytodidae) from the Philippines. J. Insect. Behav. 11, 319–342. doi: 10.1023/A:1020946529246
Jackson, R. R., and Nelson, X. J. (2011). Reliance on trial and error signal derivation by Portia africana an araneophagic jumping spider from East Africa. J. Ethol. 29, 301–307. doi: 10.1007/s10164-010-0258-5
Jackson, R. R., and Wilcox, R. S. (1990). Aggressive mimicry, prey specific predatory behaviour and predator-recognition in the predator-prey interactions of Portia fimbriata and Euryattus sp. jumping spider from Queensland. Behav. Ecol. Sociobiol. 26, 111–119. doi: 10.1007/BF00171580
Kimchi, T., Reshef, M., and Terkel, J. (2005). Evidence for the use of reflected self-generated seismic waves for spatial orientation in a blind subterranean mammal. J. Exp. Biol. 208, 647–659. doi: 10.1242/jeb.01396
Kimchi, T., and Terkel, J. (2003a). Mole rats (Spalax ehrenbergi) select bypass burrowing strategies in accordance with obstacle size. Naturwisseschaften 90, 36–39. doi: 10.1007/s00114-002-0383-2t
Kimchi, T., and Terkel, J. (2003b). Detours by the blind mole-rat follow assessment of location and physical properties of underground obstacles. Anim. Behav. 66, 885–891. doi: 10.1006/anbe.2003.2267
Kojima, W., Ishikawa, Y., and Takanashi, T. (2012a). Deceptive vibratory communication: pupae of a beetle exploit the freeze response of larvae to protect themselves. Biol. Lett. 8, 717–720. doi: 10.1098/rsbl.2012.0386
Kojima, W., Takanashi, T., and Ishikawa, Y. (2012b). Vibratory communication in the soil: pupal signals deter larval intrusion in a group-living beetle Trypoxylus dichotoma. Behav. Ecol. Sociobiol. 66, 171–179. doi: 10.1007/s00265-011-1264-5
Kotiaho, J., Alatalo, R. V., Mappes, J., Parri, S., and Rivero, A. (1998). Male mating success and risk of predation in a wolf spider: a balance between sexual and natural selection. J. Anim. Ecol. 67, 287–291. doi: 10.1046/j.1365-2656.1998.00192.x
Kuhelj, A., de Groot, M., Blejec, B., and Virant-Doberlet, M. (2015a). The effect of timing of female vibrational reply on male signalling and searching behaviour in the leafhopper Aphrodes makarovi. PLoS ONE 10:e0139020. doi: 10.1371/journal.pone.0139020
Kuhelj, A., de Groot, M., Blejec, B., and Virant-Doberlet, M. (2016). Sender-receiver dynamics in leafhopper vibrational duetting. Anim. Behav. 114, 139–146. doi: 10.1016/j.anbehav.2016.02.001
Kuhelj, A., de Groot, M., Pajk, F., Simčič, T., and Virant-Doberlet, M. (2015b). Energetic cost of vibrational signalling in a leafhopper. Behav. Ecol. Sociobiol. 69, 815–828. doi: 10.1007/s00265-015-1898-9
Kuhelj, A., and Virant-Doberlet, M. (2017). Male-male interactions and male mating success in the leafhopper Aphrodes makarovi. Ethology 123, 425–433. doi: 10.1111/eth.12613
Lakes-Harlan, R., and Strauß, J. (2014). “Functional morphology and evolutionary diversty of vibration receptors in insects,” in Studying Vibrational Communication, eds R. B. Cocroft, M. Gogala, P. S. M. Hill, and A. Wessel (Berlin: Springer Verlag), 277–302.
Lang, A. B., Teppner, I., Hartbauer, M., and Römer, H. (2005). “Predation and noise in communication networks of neotropical katydids,” in Animal Communication Networks, ed P. K. McGregor (Cambridge: Cambridge University Press), 152–169.
Laumann, R. A., Blassioli Moraes, M. C., Čokl, A., and Borges, M. (2007). Eavesdropping on sexual vibratory signals of stink bugs (Hemiptera: Pentatomidae) by egg parasitoid Telenomus podisi. Anim. Behav. 73, 637–649. doi: 10.1016/j.anbehav.2006.09.011
Laumann, R. A., Čokl, A., Lopes, A. P. S., Fereira, J. B. C., Moraes, M. C. B., and Borges, M. (2011). Silent singers are not safe: selective response of a parasitoid to substrate-borne vibratory signals of stink bugs. Anim. Behav. 82, 1175–1183. doi: 10.1016/j.anbehav.2011.08.017
Lewis, E. R., and Narins, P. M. (1985). Do frogs communicate with seismic signals? Science 227, 187–189. doi: 10.1126/science.227.4683.187
Lewis, E. R., Narins, P. M., Jarvis, J. U., Bronner, G., and Mason, M. J. (2006). Preliminary evidence for the use of microseimic cues for navigation by the Namib golden mole. J. Acoust. Soc. Am. 119, 1260–1268. doi: 10.1121/1.2151790
Lima, S. L., and Dill, L. M. (1990). Behavioral decisions made under risk of predation: a review and prospectus. Can. J. Zool. 68, 619–640. doi: 10.1139/z90-092
Lohrey, A. K., Clark, D. L., Gordon, S. D., and Uetz, G. W. (2009). Antipredator responses of wolf spiders (Araneae: Lycosidae) to sensory cues representing an avian predator. Anim. Behav. 77, 813–821. doi: 10.1016/j.anbehav.2008.12.025
Losey, J. E., and Denno, R. F. (1998). The escape response of pea aphids to foliar-foraging predators: factors affecting dropping behaviour. Ecol. Entomol. 23, 53–61. doi: 10.1046/j.1365-2311.1998.00102.x
Lujo, S., Hartman, E., Norton, K., Pregmon, E. A., Rohde, B. B., and Mankin, R. W. (2016). Disrupting mating behaviour of Diaphorina citri (Liviidae). J. Econ. Entomol. 109, 2373–2379. doi: 10.1093/jee/tow202
Mason, M. J., and Narins, P. M. (2010). “Seismic sensitivity and communication in subterranean mammals,” in The use of Vibrations in Communication: Properties, Mechanisms and Function Across Taxa, ed C. E. O'Connell-Rodwell (Thiruvananthapuram: Research Signpost), 121–139.
Mazzoni, V., Anfora, G., and Virant-Doberlet, M. (2013). Substrate vibrations during courtship in three Drosophila species. PLoS ONE 8:e80708. doi: 10.1371/journal.pone.0080708
Mazzoni, V., Eriksson, A., Anfora, G., Lucchi, A., and Virant-Doberlet, M. (2014). “Active space and the role of amplitude in plant-borne vibrational communication,” in Studying Vibrational Communication, eds R. B. Cocroft, M. Gogala, P. S. M. Hill, and A. Wessel (Berlin: Springer Verlag), 125–145.
Mazzoni, V., Lucchi, A., Čokl, A., Prešern, J., and Virant-Doberlet, M. (2009a). Disruption of the reproductive behaviour of Scaphoideus titanus by playback of vibrational signals. Entomol. Exp. Appl. 133, 174–185. doi: 10.1111/j.1570-7458.2009.00911.x
Mazzoni, V., Prešern, J., Lucchi, A., and Virant-Doberlet, M. (2009b). Reproductive strategy of the Nearctic leafhopper Scaphoideus titanus Ball (Hemiptera: Cicadellidae). Bull. Entomol. Res. 99, 401–413. doi: 10.1017/S0007485308006408
McGregor, P. K. (2005). “Introduction,” in Animal Communication Networks, ed P. K. McGregor (Cambridge: Cambridge University Press), 1–6.
McGregor, P. K., and Peake, T. M. (2000). Communication networks: social environments for receiving and signalling behaviour. Acta Ethol. 2, 71–81. doi: 10.1007/s102110000015
McNett, G. D., and Cocroft, R. B. (2008). Host shift favour vibrational signal divergence in Enchenopa binotata treehoppers. Behav. Ecol. 19, 650–656. doi: 10.1093/beheco/arn017
McNett, G. D., Luan, L. H., and Cocroft, R. B. (2010). Wind-induced noise alters signaller and receiver behaviour in vibrational communication. Behav. Ecol. Sociobiol. 64, 2043–2051. doi: 10.1007/s00265-010-1018-9
McVean, A., and Field, L. H. (1996). Communication by substratum vibration in the New Zealand tree weta Hemideina femorata (Stenopelmatidae: Orthoptera). J. Zool. 239, 101–122. doi: 10.1111/j.1469-7998.1996.tb05440.x
Meyhöfer, R., and Casas, J. (1999). Vibrational stimuli in host location by parasitic wasps. J. Insect Physiol. 45, 967–971. doi: 10.1016/S0022-1910(99)00060-8
Meyhöfer, R., Casas, J., and Dorn, S (1997). Vibration-mediated interactions in a host-parasitoid system. Proc. R. Soc. B 264, 261–266. doi: 10.1098/rspb.1997.0037
Michelsen, A., Flemming, F., Gogala, M., and Traue, D. (1982). Plants as transmission channels for insect vibrational songs. Behav. Ecol. Sociobiol. 11, 269–281. doi: 10.1007/BF00299304
Michereff, M. F., Borges, M., Aquino, M. F., Laumann, R. A., Mendes Gomes, A. C., and Blassioli-Moraes, M. C. (2016). The influence of volatile semiochemicals from stink bug eggs and oviposition-damaged plants on the foraging behaviour of the egg parasitoid Telenomus podisi. Bull. Entomol. Res. 106, 663–671. doi: 10.1017/S0007485316000419
Mitra, O., Callaham, M. A., Smith, M. L., and Yack, J. E. (2009). Grunting for worms; seismic vibrations cause Diplocardia earthworms to emerge from soil. Biol. Lett. 5, 16–19. doi: 10.1098/rsbl.2008.0456
Morales, M. A., Barone, J. L., and Henry, C. S. (2008). Acoustic alarm signalling facilitates predator protection of treehoppers by mutualist ant bodyguards. Proc. R. Soc. B 275, 1935–1194. doi: 10.1098/rspb.2008.0410
Morris, G. K., Mason, A. C., and Wall, P. (1994). High ultrasonic and tremulation signals in neotropical katydids (Orthoptera: Tettigoniidae). J. Zool. 233, 129–163. doi: 10.1111/j.1469-7998.1994.tb05266.x
Mortimer, B. (2017). Biotremology: do physical constraints limit the propagation of vibrational information? Anim. Behav. 130, 165–174. doi: 10.1016/j.anbehav.2017.06.015
Narhardiyati, M., and Bailey, W. J. (2005). Biology and natural enemies of the leafhopper Balclutha incisa (Matsumura) (Hemiptera: Cicadellidae). Austr. J. Entomol. 44, 104–109. doi: 10.1111/j.1440-6055.2005.00460.x
Narins, P. M., Lewis, E. R., Jarvis, J. M., and O'Riain, J. (1997). The use of seismic signals by fossorial southern african mammals: a neuroethological gold mine. Brain Res. Bull. 44, 641–646. doi: 10.1016/S0361-9230(97)00286-4
Narins, P. M., Reichman, O. J., Jarvis, J. U. M., and And lewis, E. R. (1992). Seismic signal tramsmission between burrows of the cape mole-rat Georychus capensis. J. Comp. Physol. A 170, 13–21. doi: 10.1007/BF00190397
Narins, P. M., Stoeger, A. S., and O'Connell-Rodwell, C. (2016). “Infrasonic and seismic communication in the vertebrates with special emphasis on the Afroteria; an update and future directions,” in Vertebrate Sound Production and Acoustic Communication, eds R. A. Suthers, W. T. Fitch, R. R. Fay, and A. N. Popper (Heidelberg: Springer Verlag), 191–227.
Oberst, S., Bann, G., Lai, J. C., and Evans, T. A. (2017). Cryptic termites avoid predatory ants by eavesdropping on vibrational cues from their footsteps. Ecol. Lett. 20, 212–221. doi: 10.1111/ele.12727
O'Connell-Rodwell, C. E. (2007). Keeping ear to the ground: seismic communication in elephants. Physiology 22, 287–294. doi: 10.1152/physiol.00008.2007
O'Connell-Rodwell, C. E., Hart, L. A., and Arnason, B. T. (2001). Exploring the potential use of seismic waves as a communication channel by elephants and other large mammals. Am. Zool. 41, 1157–1170. doi: 10.1093/icb/41.5.1157
O'Connell-Rodwell, C. E., Wood, J. D., Kinzley, C., Rodwell, T. C., Poole, J. H., and Puria, S. (2007). Wild African elephants (Loxodonta africana) discriminate between familiar and unfamiliar seismic alarm calls. J. Acoust. Soc. Am. 122, 823–830. doi: 10.1121/1.2747161
Ota, N., Gahr, M., and Soma, M. (2015). Tap dancing birds: the multimodal mutual courtship display of males and females in a socially monogamous songbird. Sci. Rep. 5:16614. doi: 10.1038/srep16614
Peake, T. M. (2005). “Eavesdropping in communication networks,” in Animal Communication Networks, ed P. K. McGregor (Cambridge: Cambridge University Press), 13–37.
Persons, M. H., and Uetz, G. W. (1996). The influence of sensory information on patch residence time in wolf spiders (Araneae; Lycosidae). Anim. Behav. 51, 1285–1293. doi: 10.1006/anbe.1996.0133
Pfannenstiel, R. S., Hunt, R. E., and Yeargan, K. V. (1995). Orientation of a Hemipteran to vibrations produced by feeding caterpillars. J. Insect Behav. 8, 1–9. doi: 10.1007/BF01990965
Polajnar, J., Eriksson, A., Rossi Stacconi, M. V., Lucchi, A., Anfora, G., Virant-Doberlet, M., et al. (2014). The process of pair formation mediated by substrate-borne vibrations in a small insect. Behav. Processes 107, 68–78. doi: 10.1016/j.beproc.2014.07.013
Prešern, J., Polajnar, J., de Groot, M., Zorović, M., and Virant-Doberlet, M. (2018). On the spot: utilization of directional cues in vibrational communication of stink bug. Sci. Rep. 8:5418. doi: 10.1038/s41598-018-23710-x
Ramaswamy, K., and Cocroft, R. B. (2009). Collective signals in treehopper broods provide predator localization cues to the defending mother. Anim. Behav. 78, 697–704. doi: 10.1016/j.anbehav.2009.06.017
Randall, J. A. (2001). Evolution and function of drumming as communication in mammals. Am. Zool. 41, 1143–1156. doi: 10.1093/icb/41.5.1143
Randall, J. A. (2010). “Drummers and stompers: vibrational communication in mammals,” in The use of Vibrations in Communication: Properties, Mechanisms and Function Across Taxa, ed C. E. O'Connell-Rodwell (Thiruvananthapuram: Research Signpost), 99–120.
Reguera, G. (2011). When microbial conversations get physical. Trend Microbiol. 19, 105–113. doi: 10.1016/j.tim.2010.12.007
Roberts, J. A., Taylor, P. W., and Uetz, G. W. (2007). Consequences of complex signalling: predator detection of multimodal cues. Behav. Ecol. 18, 236–240. doi: 10.1093/beheco/arl079
Roberts, L., Cheesman, S., Breithaupt, T., and Elliott, M. (2015). Sensitivity of the mussel Mytilus edulis to substrate-borne vibration in relation to anthropogenically generated noise. Mar. Ecol. Prog. Ser. 538, 185–195. doi: 10.3354/meps11468
Römer, H. (2013). “Masking by noise in acoustic insects: problems and solutions,” in Animal Communication and Noise, ed H. Brumm (Berlin: Springer Verlag), 33–63.
Römer, H., Lang, A., and Hartbauer, M. (2010). The signallers dilemma: a cost-benefit analysis of public and private communication. PLoS ONE 5:e13325. doi: 10.1371/journal.pone.0013325
Rosengaus, R. B., Jordan, C., Lefebvre, M. L., and Traniello, J. F. A. (1999). Pathogen alarm behavior in a termite: a new form of communication in social insect. Naturwissenschaften 86, 544–548. doi: 10.1007/s001140050672
Sitvarin, M. I., Gordon, S. D., Uetz, G. W., and Rypstra, A. L. (2016). The wolf spider Pardosa milvina detects predator threat level using only vibratory cues. Behaviour 153, 159–173. doi: 10.1163/1568539X-00003332
Soley, F. G. (2016). Fine-scale analysis of an assassin bug's behaviour: predatory strategies to bypass the sensory systems of prey. R. Soc. Open Sci. 3:160573. doi: 10.1098/rsos.160573
Stuart, M., Turman, A. B., Shaw, J., Walsh, N., and Nguyen, V. (2003). Effects of aging on vibration detection thresholds at various body regions. BMC Geriatrics 3:1. doi: 10.1186/1471-2318-3-1
Šturm, R., Polajnar, J., and Virant-Doberlet, M. (2019). “Practical issues in studying natural vibroscape and biotic noise,” in Biotremology–Studying Vibrational Behaviour, eds P. S. M. Hill, R. Lakes-Harlan, V. Mazzoni, P. M. Narins, M. Virant-Doberlet, and A. Wessel (Berlin: Springer Verlag). Available online at: https://www.springer.com/gp/book/9783030222925
Sugi, T., Igarashi, R., and Nishimura, M. (2018). Noninvasive mechanochemical imaging in unconstrained Caenophabditis elegans. Materials 11:2013. doi: 10.3390/ma11061034
Sullivan-Beckers, L., and Cocroft, R. B. (2010). The importance of female choice, male-male competition and signal transmission as causes of selection on male mating signals. Evolution 64, 3158–3171. doi: 10.1111/j.1558-5646.2010.01073.x
Tarsitano, M., Jackson, R. R., and Kirchner, W. H. (2000). Signals and signal choices made by the araneophagic jumping spider Portia fimbriata while hunting the orb-weaving web spiders Zygiella x-notata and Zosis geniculatus. Ethology 106, 595–615. doi: 10.1046/j.1439-0310.2000.00570.x
Taylor, J. R. A., and Patek, S. N. (2010). “Crustacean seismic communication: heard but not present?,” in The use of Vibrations in Communication: Properties, Mechanisms and Function Across Taxa, ed C. E. O'Connell-Rodwell (Thiruvananthapuram: Research Signpost), 9–24.
Tishechkin, D. Y. (2013). Vibrational background noise in herbaceous plants and its impact on acoustic communication of small Auchenorrhyncha and Psyllinea (Homoptera). Entomol. Rev. 93, 1179–1189. doi: 10.1134/S0013873813050035
Torr, P., Heritage, S., and Wilson, M. J. (2004). Vibrations as a novel signal for host location by parasitic nematodes. Int. J. Parasitiol. 34, 997–999. doi: 10.1016/j.ijpara.2004.05.003
Travassos, M. A., and Pierce, N. E. (2000). Acoustics, context and function of vibrational signalling in a lycaenid butterfly-ant mutualism. Anim. Behav. 60, 13–26. doi: 10.1006/anbe.1999.1364
Uetz, G. W. (1992). Foraging strategies of spiders. Trend Ecol. Evol. 7, 155–159. doi: 10.1016/0169-5347(92)90209-T
Uhl, G., and Elias, D. O. (2011). “Communication,” in Spider Behaviour, ed M. E. Herberstein (Cambridge: Cambridge University Press), 127–189.
Veits, M., Khait, I., Obolski, U., Zinger, E., Boonman, A., Goldshtein, A., et al. (2019). Flowers respond to pollinator sound within minutes by increasing nectar sugar concentration. bioRxiv. doi: 10.1101/507319
Virant-Doberlet, M., and Čokl, A. (2004). Vibrational communication in insects. Neotrop. Entomol. 33, 121–134. doi: 10.1590/S1519-566X2004000200001
Virant-Doberlet, M., Čokl, A., and Zorović, M. (2006). “Use of substrate vibrations for orientation: from behaviour to physiology,” in Insects Sounds and Communication: Physiology, Behaviour, Ecology and Evolution, eds M. F. Claridge and S. Drosopoulos (Boca Raton, FL: Taylor and Francis), 81–97.
Virant-Doberlet, M., King, R. A., Polajnar, J., and Symondson, W. O. (2011). Molecular diagnostics reveal spiders that exploit prey vibrational signals used in sexual communication. Mol. Ecol. 20, 2204–2216. doi: 10.1111/j.1365-294X.2011.05038.x
Virant-Doberlet, M., Mazzoni, V., de Groot, M., Polajnar, J., Lucchi, A., Symondson, W. O. C., et al. (2014). “Vibrational communication networks: eavesdropping and biotic noise,” in Studying Vibrational Communication, eds R. B. Cocroft, M. Gogala, P. S. M. Hill, and A. Wessel (Berlin: Springer Verlag), 93–123.
Wäckers, F. L., Mitter, E., and Dorn, S. (1998). Vibrational sounding by the pupal parasitoid Pimpla (Coccygomimus) turionellae: an additional solution to the reliability-detectabilioty problem. Biol. Contr. 11, 141–146. doi: 10.1006/bcon.1997.0592
Warkentin, K. M. (2005). How embryos assess risk? Vibrational cues in predator-induced hatching of red-eyed treefrogs. Anim. Behav. 70, 59–71. doi: 10.1016/j.anbehav.2004.09.019
Wesener, T., Köhler, J., Fuchs, S., and van den Spiegel, D. (2011). How to uncoil your partner - “mating songs” in giant pill-millipedes (Diplopoda: Sphaerotheriida). Naturwissenschaften 98, 967–975. doi: 10.1007/s00114-011-0850-8
Whang, A., and Janssen, J. (1994). Sound production through the substrate during reproduction in mottled sculpin Cottus bairdi (Cottidae). Environ. Biol. Fish. 40, 141–148. doi: 10.1007/BF00002540
Wignall, A. E., Jackson, R. R., Wilcox, R. S., and Taylor, P. W. (2011). Exploitation of environmental noise by an araneophagic assassin bug. Anim. Behav. 82, 1037–1042. doi: 10.1016/j.anbehav.2011.07.038
Wignall, A. E., and Taylor, P. W. (2011). Assassin bug uses aggressive mimicry to lure spider prey. Proc. R. Soc. B. 278, 1427–1433. doi: 10.1098/rspb.2010.2060
Wilcox, R. S., Jackson, R. R., and Gentile, K. (1996). Spiderweb smokescreens: spider trickster uses background noise to mask stalking movements. Anim. Behav. 51, 313-326. doi: 10.1006/anbe.1996.0031
Yack, J. (2016). “Vibrational signaling,” in Insect Hearing, eds G. S. Pollack, A. C. Mason, A. N. Popper, and R. R. Fay (Berlin: Springer Verlag), 99–123.
Young, B. A., and Morain, M. (2002). The use of ground-borne vibrations for prey localization in the Saharan sand vipers (Cerates). J. Exp. Biol. 205, 661–665.
Keywords: biotremology, vibrational communication, communication network, predator-prey interactions, eavesdropping
Citation: Virant-Doberlet M, Kuhelj A, Polajnar J and Šturm R (2019) Predator-Prey Interactions and Eavesdropping in Vibrational Communication Networks. Front. Ecol. Evol. 7:203. doi: 10.3389/fevo.2019.00203
Received: 12 December 2018; Accepted: 16 May 2019;
Published: 04 June 2019.
Edited by:
Ximena E. Bernal, Purdue University, United StatesReviewed by:
Shawn M. Wilder, Oklahoma State University, United StatesReginald B. Cocroft, University of Missouri, United States
Copyright © 2019 Virant-Doberlet, Kuhelj, Polajnar and Šturm. This is an open-access article distributed under the terms of the Creative Commons Attribution License (CC BY). The use, distribution or reproduction in other forums is permitted, provided the original author(s) and the copyright owner(s) are credited and that the original publication in this journal is cited, in accordance with accepted academic practice. No use, distribution or reproduction is permitted which does not comply with these terms.
*Correspondence: Meta Virant-Doberlet, bWV0YS52aXJhbnRAbmliLnNp