- 1Conservation Ecology Group, Groningen Institute for Evolutionary Life Sciences, University of Groningen, Groningen, Netherlands
- 2Department of Biological Sciences, University of South Carolina, Columbia, SC, United States
- 3Conservation Biology Research Group, Department of Anatomy, Cell Biology and Zoology, Faculty of Sciences, University of Extremadura, Badajoz, Spain
- 4DBIO/CESAM Centre for Environmental and Marine Studies, University of Aveiro, Campus Universitário de Santiago, Aveiro, Portugal
- 5South Iceland Research Centre, University of Iceland, Fjölheimar, Selfoss, Iceland
- 6Department of Zoology, University of Oxford, Oxford, United Kingdom
- 7NIOZ Royal Netherlands Institute for Sea Research, Department of Coastal Systems and Utrecht University, Den Burg, Netherlands
- 8Department of Life Sciences, Marine and Environmental Research Centre, University of Coimbra, Coimbra, Portugal
- 9Division of Biological Sciences, University of Montana, Missoula, MT, United States
Few studies have been able to directly measure the seasonal survival rates of migratory species or determine how variable the timing of migration is within individuals and across populations over multiple years. As such, it remains unclear how likely migration is to affect the population dynamics of migratory species and how capable migrants may be of responding to changing environmental conditions within their lifetimes. To address these questions, we used three types of tracking devices to track individual black-tailed godwits from the nominate subspecies (Limosa limosa limosa) throughout their annual cycles for up to 5 consecutive years. We found that godwits exhibit considerable inter- and intra-individual variation in their migratory behavior across years. We also found that godwits had generally high survival rates during migration, although survival was reduced during northward flights across the Sahara Desert. These patterns differ from those observed in most other migratory species, suggesting that migration may only be truly dangerous when crossing geographic barriers that lack emergency stopover sites and that the levels of phenotypic flexibility exhibited by some populations may enable them to rapidly respond to changing environmental conditions.
Introduction
Many migratory species are experiencing rapid and dramatic population declines (Wilcove and Wikelski, 2008). These declines have been linked with a variety of anthropogenic changes, including habitat loss (Rushing et al., 2016), and land-use (Gill et al., 2007) and climatic change (Both et al., 2006). Nonetheless, linking a species' or population's decline with specific environmental drivers remains difficult given the vast distances that separate areas occupied during different phases of the annual cycle (Piersma et al., 2016). The advent of new tracking technologies over the past two decades has begun to bridge this gap, but these new technologies remain expensive—meaning that many studies of migration are carried out over short timescales or with small sample sizes—and thus a plethora of questions about migration and the causes of declines in migratory species still remain (Hebblewhite and Haydon, 2010). This is especially true in regards to our understanding of how migratory behaviors may change over the course of an individual's lifetime and how migration itself may influence the population dynamics of migratory species (Piersma, 2011).
Recent work, however, has begun to deepen our understanding of the degree of variation in migratory behavior that individuals can be expected to display over the course of their lives. For instance, a number of studies have shown that individual migratory birds can exhibit highly repeatable migratory timing (Stanley et al., 2012; Gill et al., 2014). In some cases, individuals may vary their departure dates from non-breeding sites by as little as 3 days over 4 consecutive years (Conklin et al., 2013). In contrast, other studies have found that individuals of some species can exhibit marked improvement and flexibility in their migratory timing and performance, enabling them to adjust their behaviors to prevailing environmental conditions (Sergio et al., 2014; Pedler et al., 2018). What remains unclear, though, is how flexible migratory behaviors are generally and how both historical and contemporary selection pressures may mold the levels of flexibility exhibited by individuals within a population.
Similarly, our understanding of the location and timing of mortality events during the annual cycles of migratory species has developed rapidly over the past two decades. While most efforts to determine the seasonal survival rates of migratory species have relied on color-marking schemes and mark-recapture analyses to infer when and where individuals die (Sillett and Holmes, 2002; Lok et al., 2015), some recent studies have used satellite tracking devices to monitor the survival of individuals continuously throughout their annual cycles (Hebblewhite and Merrill, 2011; Klaassen et al., 2014; Hewson et al., 2016; Watts et al., 2019). In general, these studies have identified migration as the period during the annual cycle with the highest mortality rates. Nonetheless, work with two different sub-species of red knots (Calidris canutus islandica and C. c. canutus) has found the opposite—higher survival during migration than in stationary periods—indicating that migration may not be universally dangerous (Leyrer et al., 2013; Rakhimberdiev et al., 2015). As a result, the seasonal survival patterns of a larger number of migratory species still need to be documented in order to more fully understand how migration may influence a species' population dynamics.
The nominate subspecies of the black-tailed godwit (Limosa limosa limosa) breeds predominantly in The Netherlands and spends the non-breeding season disjunctively in sub-Saharan West Africa and the southern Iberian Peninsula (Hooijmeijer et al., 2013; Kentie et al., 2016). Previous work has indicated that individuals exhibit significant repeatability in the timing of their departure from staging sites during northward migration (r = 0.30–0.42; Lourenço et al., 2011) and arrival at the breeding grounds (r = 0.24; Kentie et al., 2017), irrespective of their non-breeding location. Individuals can also exhibit marked flexibility in their migratory behaviors, however. For instance, in response to a recent early spring snowstorm, many godwits were able to alter their arrival timing and use of stopover sites in order to avoid the most inclement conditions (Senner et al., 2015a). Similarly, recent work has found little evidence that godwits migrating longer distances incur costs that carry-over to affect reproduction, indicating the possibility that migration may not be the limiting event during the godwit annual cycle (Kentie et al., 2017). Godwits thus represent an intriguing opportunity to assess levels of flexibility in migratory timing across an individual's life, as well as to broaden our understanding of how mortality events are spread across migratory annual cycles.
To address these knowledge gaps, we used three types of tracking devices to monitor godwit migration timing and seasonal survival over the course of 6 years, from 2012 to 2017. Given their ability to flexibly respond to conditions encountered mid-migration and the lack of reversible state effects linking migration to reproductive success (Senner et al., 2015a; Kentie et al., 2017), we predicted that godwits would exhibit high levels of flexibility and high survival during migration. By broadening the spectrum of species for which seasonal survival estimates and measures of migratory repeatability have been determined, we aim to improve our understanding of the ways in which migratory species may be able to respond to environmental change.
Methods
Study Species
Godwits of the nominate subspecies breed across much of Western Europe, but nearly 80% of their population now breeds in The Netherlands (Kentie et al., 2016). Historically, the entire population was thought to spend the non-breeding season in West Africa and then migrate northward via stopover sites in Italy, Morocco, Portugal, and France (Beintema and Drost, 1986). However, coinciding with shrinking populations and the creation of fish ponds and seasonally flooded rice fields in Spain and Portugal in the 1980s, godwits have altered both their non-breeding distribution and migration routes (Lourenço and Piersma, 2008). Currently, nearly a quarter of the population spends the non-breeding season in and around Doñana Natural and National Parks in southern Spain (Márquez-Ferrando et al., 2014). Together with staging sites in Extremadura, Spanish sites now host approximately half of the population during northward migration as well (Masero et al., 2011), with the remainder using Portuguese staging sites (Lourenço et al., 2010; Verhoeven et al., 2018). Moreover, Italy is now rarely visited and the use of French and Moroccan stopovers has declined dramatically (Lourenço and Piersma, 2008; Alves and Lourenço, 2014).
In general, godwits spending the non-breeding season in West Africa depart from January to February and then join the remainder of the population in Iberia, where they can stage for as long as 4–5 weeks (Masero et al., 2011). Godwits begin leaving Iberia in early March and can fly directly to their breeding sites or stopover as many as four times en route (Senner et al., 2015a). Breeding ground arrival then spans a period from early March to mid-May. Once on the breeding grounds, a period of 5 weeks can elapse between arrival and clutch initiation (Senner et al., 2015b). Finally, adult godwits depart breeding areas on southward migration from mid-June onwards (Hooijmeijer et al., 2013).
General Methods and Tracking Devices
We employed three different tracking technologies: solar geolocation devices (“geolocators”), satellite transmitters, and GPS trackers. Geolocators and GPS trackers were both deployed during the breeding season (Apr–Jun) at our long-term demographic study area in southwest Friesland, The Netherlands (Senner et al., 2015a). This area encompasses 10,280 ha spanning from 53.0672°N, 5.4021°E in the north, to 52.8527°N, 5.4127°E in the south, 52.9715°N, 5.6053°E in the east, and 52.8829°N, 5.3607°E in the west. Satellite transmitters were deployed during northward migration (Jan–Feb) at staging sites in Extremadura, Spain (39.0364°N, 5.9112°W; Masero et al., 2011) and Santarém, Portugal (38.8525°N, 8.9695°W; Lourenço et al., 2011).
We deployed geolocators from Migrate Technology, Ltd. on adults captured on nests using walk-in traps or mist-nets placed over the nest (n = 126; 2012–2013: 0.65 g W65A9, 2014: 1 g Intigeo C65). Geolocators were attached to colored flags placed on the upper tibia; the combination of the geolocator and flag weighed ≤3.3 g, which was ≤1.5% of an individual's mass at the time of capture. In subsequent years, we then attempted to recapture geolocator-carrying individuals using similar methods as during the initial capture. We also attached 9.5 g solar-powered PTT-100s satellite transmitters from Microwave Technology Inc. (n = 60; 2013–2015) and 7.5 g solar-powered UvA-Bits GPS trackers (n = 20; 2013) developed by the University of Amsterdam (Bouten et al., 2013) using a leg-loop harness made of 2 mm nylon rope (see Senner et al., 2015a for more details). Because of the weight, we deployed these devices only on individuals weighing >300 g, meaning the majority were placed on females (n = 1 male). In total, the tracking device and harness weighed ~12 and 10 g, respectively, representing 3.43 ± 0.22 and 2.86 ± 0.19% of an individual's mass at the time of capture.
Tracking Data
Geolocators measure ambient light levels in order to identify the timing of sunrise and sunset, and, ultimately, estimate an individual's position on the globe. To transform our raw light data into twice-daily position estimates, we first passed it through the program IntiProc (v. 1.03; Migrate Technology, Ltd.) and then processed our transformed light data using the “BAStag” package (Wootherspoon et al., 2013) in the R software environment (R Development Core Team, 2016). We used a light threshold of 1.5 to demarcate all sunrises and sunsets and discarded sunrises/sunsets that had non-random shading events, such as when a geolocator was shaded during either the beginning or end of a twilight period. Finally, we processed our light data using the R package “FLightR” (Rakhimberdiev et al., 2017) following Rakhimberdiev et al. (2016). In FLightR, we used the period during which an individual was known to be on the breeding grounds (from resighting data) as a calibration period. We then analyzed the data without land or behavioral masks or automated outlier exclusion, but with movements constrained to the region between 18°W−13°E and 11–57°N (Hooijmeijer et al., 2013). All models were optimized with 1 million particles.
We programmed satellite transmitters to collect locations for 10 h and recharge for 48 h, which allowed us to identify locations used for ≥2 d. We retrieved all location fixes via the CLS tracking system (www.argos-system.org) and passed them through the Douglas Argos-filter (DAF) algorithm (Douglas et al., 2012). We retained all standard class locations (i.e., LC 3, 2, 1) and excluded all auxiliary class locations that did not meet our predefined threshold for maximum movement rate (120 km h−1). On average, this resulted in 8 ± 1 locations per 10-h duty cycle for each individual.
We programmed the GPS trackers to record an individual's location once every 5 min when the tracker's battery was fully charged and once every 15 min in all other instances (see Senner et al., 2018 for more details). Although GPS trackers generate many fewer erroneous locations than satellite transmitters, spurious locations are recorded. We thus also filtered our GPS tracker data with the DAF and used the same movement thresholds as those imposed on our satellite transmitter data.
Statistical Analysis
To detail the temporal flexibility of godwit annual cycles, we first characterized the timing of all movements made by tracked individuals. Because both the spatial and temporal resolution of the three types of tracking devices differed (Bouten et al., 2013; Boyd and Brightsmith, 2013; Rakhimberdiev et al., 2016), we took a conservative approach to identifying movements in order to make the data comparable across devices. Therefore, given that the average location estimates generated by FLightR have a deviation of ± ~40 km from an individual's true location and that our satellite transmitters were only able to detect stopovers lasting ≥2 d, we defined a migratory movement as a direct flight of ≥80 km and a stopover as a stationary period lasting ≥2 d. Additionally, geolocators have difficulty estimating latitude—but not longitude—within a week of the equinoxes (Rakhimberdiev et al., 2016). Unfortunately, the spring equinox coincides with godwit northward migration and the period of their arrival at their breeding sites. However, godwits migrate in both a northerly and easterly direction during this period, enabling us to document each individual's arrival date by identifying the date on which they crossed a longitude of 5°E, which corresponds to The Netherlands when arriving from the west (see Verhoeven et al., 2019 for more details).
Given these “decision rules” for identifying migratory movements, following our previously published studies (e.g., Hooijmeijer et al., 2013), we delineated the godwit annual cycle into 10 separate annual cycle events that are performed by most godwits—the breeding season, post-breeding season, southbound migration over Europe, Iberian stopover period, southbound migration over the Sahara Desert, the non-breeding season, northbound migration over the Sahara Desert, Iberian staging period, northbound flight over Europe, and European stopover period. Briefly, we defined non-breeding sites as the site where an individual was located on 20 September, while breeding sites were those sites where an individual was located on 15 May. We defined the post-breeding period as beginning for geolocator-carrying individuals the day they were last resighted in our study area (see Loonstra et al., 2019 for detailed information about our hemisphere-wide resighting efforts); for all other individuals, the post-breeding period began when they moved >25 km away from their breeding site. Finally, not all individuals migrate to sub-Saharan Africa. As a result, we only made comparisons among individuals performing the same event (e.g., northbound flight over Europe).
We then calculated: the repeatability (r) of the timing and number of movements with the R package “rptR” (Stoffel et al., 2017) using the linear mixed model method and including individual as a random-effect; the amount of variation exhibited by an individual in their migratory behavior across years (difference in the timing of an event between consecutive years; “intra-individual” variation); and the amount of population-level variation across all tracked individuals (difference in timing between earliest and latest individual within a single year ± SD; “inter-individual” variation). To ensure that the observed levels of intra-individual variation were not a byproduct of stochastic environmental conditions or the type of transmitter an individual carried, we used linear mixed models with individual included as a random effect, and year and type of tracking device as predictor variables. Additionally, as an anecdotal comparison, we obtained data on the amount of intra-individual variation in northward migratory timing exhibited by two other species of godwits—Hudsonian (L. haemastica) and bar-tailed godwits (L. lapponica baueri)—from the published literature (Conklin et al., 2013; Senner et al., 2014).
We also used satellite transmitter data to calculate event-specific survival rates. Retrieving data from both the GPS trackers and geolocators was dependent upon an individual returning to the breeding grounds, meaning we could not pinpoint when mortality occurred in individuals carrying those types of devices that did not return to The Netherlands. To calculate the event-specific survival rates, we first determined which type of annual-cycle event an individual was engaged in on each day that its transmitter provided location estimates using an individual's location and movement patterns (see above). Then, we identified on which day each individual died via its transmitter's activity sensor. Although the death of a transmitter does not necessarily imply that the individual carrying that transmitter also died, in all but five cases in which we documented the death of a transmitter, we also failed to subsequently observe the associated individual. In those cases in which we did observe the individual after the transmitter had stopped functioning, two birds had lost their transmitters, while the transmitters of the other three individuals had failed. These individuals were removed from our analyses in the season during which their transmitter failed or was lost; our estimates of godwit survival rates may therefore be biased low. Finally, to test for differences in daily survival rates among years and annual cycle events, we used an Andersen-Gill model—a type of hazards model—in the R package “survival” (Therneau and Lumley, 2015). In these models, we included the annual cycle event as the (categorical) predictor variable, survival between consecutive time steps as the dependent variable, year as the strata, and individual as a random effect. Because the hazards calculated by the model depend on which event of the annual cycle was used as the reference/baseline level, we reran the model with each separate event as the reference and then used model averaging in the R package “AICcmodavg” (Mazerolle, 2013) to determine their model-averaged coefficients.
For our linear regression model testing the effects of year and type of tracking device on intra-individual variation, we compared models including predictor variables to an intercept-only “null” model within an AICc framework, where the model with the lowest AICc score was considered the most well-supported model (Burnham and Anderson, 2002). Predictor variables whose 95% confidence intervals did not include zero were considered biologically relevant (Grueber et al., 2011). The regression models were run using the R package “lme4” (Bates et al., 2014); the statistical significance of random effects was tested using the R package “lmerTest” (Kuznetsova et al., 2015). All results are reported as mean ± SD unless otherwise noted.
Results
Return Rates and Migration Routes
We deployed 60 satellite transmitters, 20 GPS trackers, and 126 geolocators from 2012 to 2015 (Figure 1). Three satellite transmitters were inadvertently placed on Icelandic-breeding black-tailed godwits (L. l. islandica) and not included in our analyses; the remaining 57 individuals were tracked for ≤4 southward and ≤5 northward migrations per individual. Fourteen individuals with GPS trackers returned the following year, of which 4 provided data and 1 eventually provided two complete annual cycles. One-hundred and eighteen individuals with geolocators returned to the breeding grounds at least once, 43 of those individuals were recaptured, 28 of those geolocators provided data for at least one full migration period, and 2 individuals were tracked for two complete annual cycles.
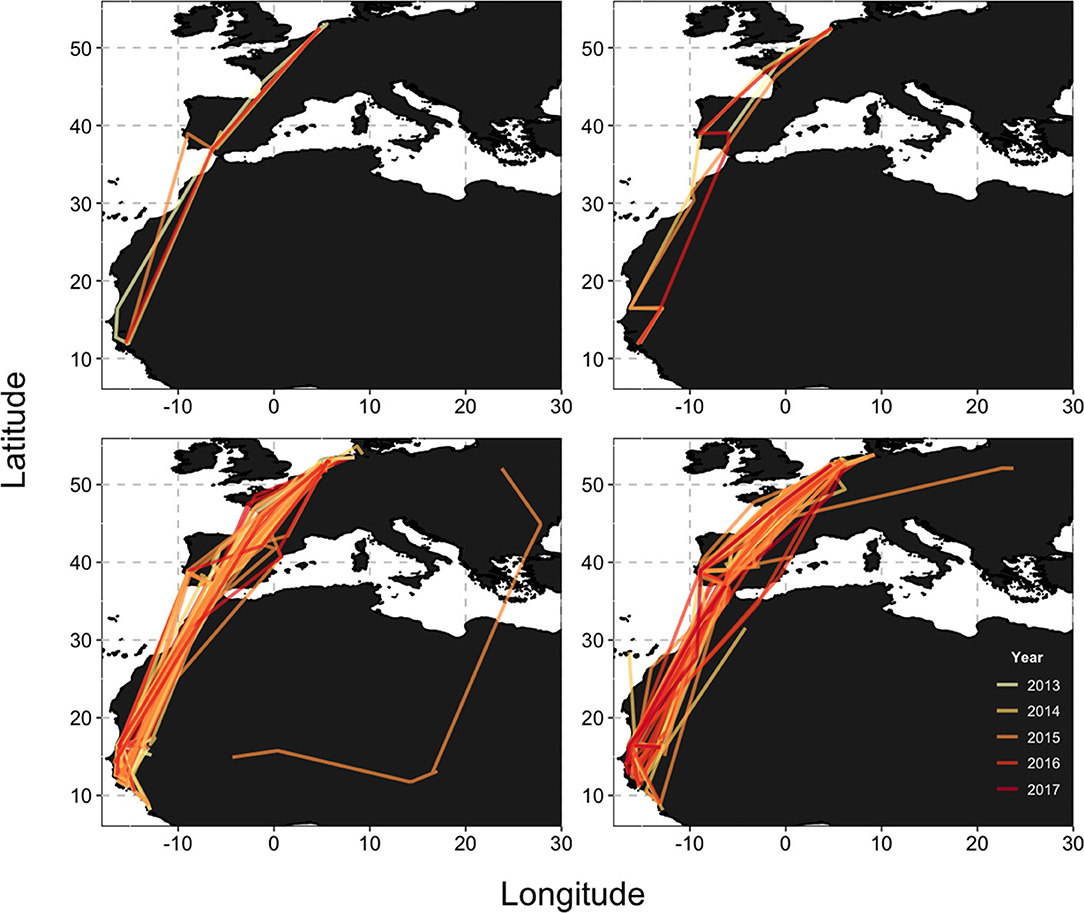
Figure 1. The migratory routes during southward (left) and northward (right) migrations for the entire tracked population (bottom; n = 57 individuals), as well as a representative individual, “Badajoz,” (top; n = 4 years). The legend applies to all panels.
Among the individuals with satellite transmitters, all but 10 bred in The Netherlands (n = 47); the remaining individuals bred in Germany (n = 3), Belgium (n = 2), and Poland (n = 1), or were not tracked long enough to determine their breeding location (n = 4). Across all individuals tracked to their non-breeding grounds (n = 64), irrespective of tracking device type, all but eight spent the non-breeding season in sub-Saharan West Africa, with the remainder spending that period either on the Iberian Peninsula (n = 7) or in Morocco (n = 1); 13 individuals died before reaching their non-breeding grounds. All individuals exhibited broad fidelity to both their breeding and non-breeding sites, as no individual changed either the province in which they bred nor whether they spent the non-breeding season north or south of the Sahara Desert (Figure 1; see also Kentie et al., 2017; Verhoeven et al., 2019).
Repeatability
The repeatability of migratory timing and behavior ranged from r = 0.78 (95% CI = 0.55, 0.91; Table 1) for the date of departure from the non-breeding grounds to r = 0.00 (95% CI = 0.00, 0.40) for the number of stops made during southward migration. In only three cases were aspects of migration not repeatable—the number of stops made during southward migration (see above), number of stops made during northward migration (r = 0.20, 95% CI = 0.00, 0.52), and duration of northward migration (r = 0.21, 95% CI = 0.00, 0.52).
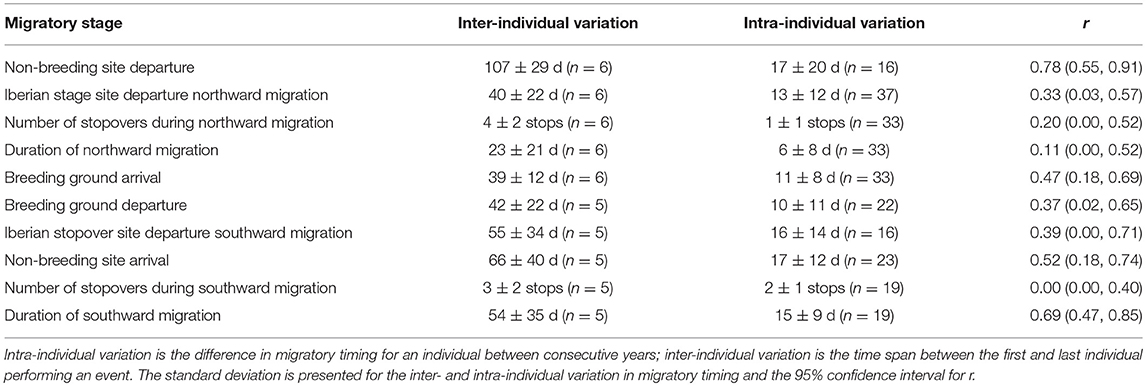
Table 1. Repeatability (r), and inter- and intra-individual variation in migratory timing and behavior of continental black-tailed godwits tracked with satellite transmitters, GPS trackers, and solar geolocation devices, 2012–2017.
Inter-individual Variation
Levels of inter-individual variation for the timing of migratory events ranged from an average of 96 ± 29 d (n = 6 years; Table 1) for the timing of departure from the non-breeding grounds to an average of 47 ± 9 d (n = 6 years) for the timing of departure from the breeding grounds. Inter-individual variation in the number of stops made was 4 ± 2 stops (n = 6 years) during northward migration and 4 ± 2 stops during southward migration (n = 5 years), while for the duration of migration, it was 25 ± 11 d (n = 6 years) for northward migration and 63 ± 23 d (n = 5 years) for southward migration.
Intra-individual Variation
Levels of intra-individual variation in the timing of migratory events ranged from an average of 17 ± 12 d (n = 17 individuals; Table 1) for the timing of arrival at non-breeding sites following southward migration to an average of 10 ± 11 d (n = 22 individuals) for the timing of arrival at the breeding grounds. Intra-individual variation in the number of stops made was 1 ± 1 stops (n = 33 individuals) during northward migration and 2 ± 1 stops during southward migration (n = 19 individuals), while, for the duration of migration, it was 6 ± 8 d (n = 33 individuals) for northward migration and 15 ± 9 d (n = 19 individuals) for southward migration. These levels of intra-individual variation did not vary across years or with the type of tracking device an individual carried (Supplementary Information Tables 1, 2). Additionally, black-tailed godwits exhibited qualitatively more flexibility than did either Hudsonian or bar-tailed godwits (Figure 2) over the course of northward migration.
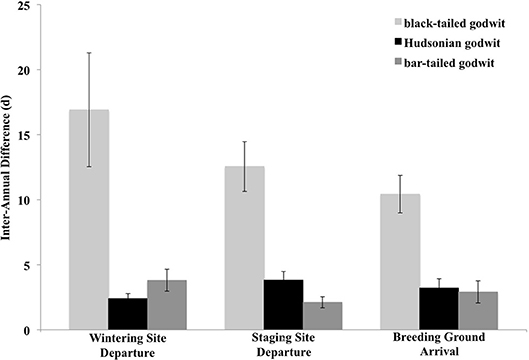
Figure 2. Intra-individual variation in the timing of northward migration among individual black-tailed (n = 92), Hudsonian (n = 26), and bar-tailed godwits (n = 9). Data for Hudsonian and bar-tailed godwits were taken from Conklin et al. (2013) and Senner et al. (2014), respectively. Error bars represent one standard deviation from the mean.
Seasonal Survival
Across all years and periods of the annual cycle, daily survival rates for godwits carrying satellite transmitters averaged 0.998 ± 0.001 (n = 57 individuals and 24,366 days), leading to an annual survival rate of 0.52 ± 0.12. Hazard rates were highest during the flight from non-breeding areas in sub-Saharan West Africa to staging areas in Spain and Portugal (β = 18.97, 95% CI = 11.75, 26.19; Table 2; Supplementary Information Tables 3, 4). In contrast, hazard rates were lowest during the post-breeding staging period (β = −15.6, 95% CI = −27.29, −3.91). No other portion of the annual cycle had hazard rates that differed significantly from the baseline (Table 2). Finally, the breeding season accounted for 29.8 ± 20.1% (n = 5 years; Table 2) of all mortality events, while northward flights over Africa accounted for 12.8 ± 16.0% (n = 5 years), and the post-breeding period 0.0 ± 0.0% (n = 4 years).
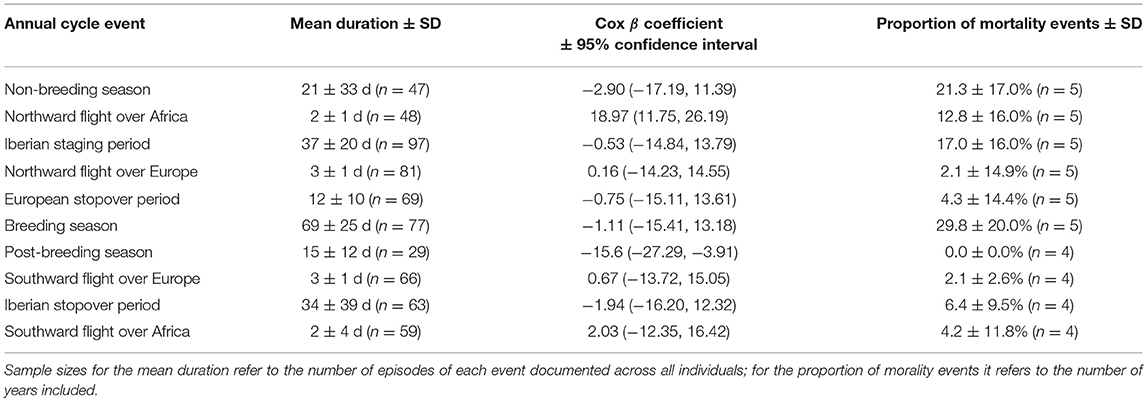
Table 2. The duration, model-averaged β coefficients from an Andersen-Gill model, and total proportion of mortality events observed during each annual cycle event.
Discussion
We found that black-tailed godwits breeding in northwestern Europe exhibited high-levels of inter- and intra-individual variation in migratory timing—but also high repeatability of departure and arrival dates—and generally high survival rates during migratory flights. Nonetheless, survival rates were lower during migratory flights over the Sahara Desert. Our results thus suggest that the relative danger of migration may be context dependent and that, under the right circumstances, some migratory species may be readily capable of responding to contemporary environmental changes.
Seasonal Survival in Migratory Species
Given the difficulty of tracking migrants throughout their annual cycles, few studies have explored their seasonal survival. The majority of studies that have succeeded in developing such estimates have identified migration as the period with the lowest daily (and seasonal) survival rates of the annual cycle (Sillett and Holmes, 2002; Hebblewhite and Merrill, 2011; Klaassen et al., 2014; Romer et al., 2015; Watts et al., 2019). Our results are not entirely consistent with these findings: migratory flights over Europe during both north- and southward migration were consistently characterized by hazard rates that were equivalent to those exhibited during other, stationary, portions of the godwit annual cycle. Nevertheless, hazard rates during the northward flight traversing the Sahara Desert were significantly higher—and thus survival rates lower—overall than during other periods of the annual cycle. Our results thus suggest that the relative danger of migration is likely context dependent and migration may only be truly dangerous during flights over geographic barriers that lack potential emergency stopover sites (see also Lok et al., 2015).
Two potential caveats, however, should also be noted: First, even after accounting for the potential biases in our survival estimates resulting from transmitter failures, the overall survival rates documented here are considerably lower than those found in other studies with color-marked godwits (Roodbergen et al., 2008; Kentie et al., 2016, 2018; Loonstra et al., 2019). This suggests our use of satellite transmitters weighing 9.5 g may have served as a handicap that reduced survival. Survival was lower than expected across nearly all annual-cycle events, however, indicating that these effects were not just experienced during migration. Second, our sample of godwits carrying satellite transmitters was heavily biased toward females and it is possible that males and females differ in their seasonal survival patterns. Previous godwit studies, though, have suggested that migratory patterns are roughly similar between the two sexes (Lourenço et al., 2011; Kentie et al., 2016), although adult females do have slightly lower annual survival rates (Loonstra et al., 2019). We therefore believe that our results are robust and broadly representative of the seasonal survival rates and migratory patterns exhibited by godwits breeding in northwest Europe.
Given these results and those from recent studies with red knots (Leyrer et al., 2013; Rakhimberdiev et al., 2015), how strongly should migration be expected to determine the population dynamics of migratory species? Previous studies of migratory birds making flights across large geographic barriers, such as the Sahara, have found that mortality events experienced during these flights may result from a suite of potentially interacting and sometimes unpredictable processes, including extremely high temperatures (Schmaljohann et al., 2007), violent sandstorms (Klaassen et al., 2010), poor body condition (Ward et al., 2018), and predation (Gangoso et al., 2013). In some cases, the mortality events experienced during these flights play an important role in determining a species' overall population dynamics (Lok et al., 2013). Many migratory species never cross such barriers, however. For these species, our results suggest that migration should not necessarily be more dangerous than any other activity, so long as high-quality stopover sites exist (Alves et al., 2013).
Even for those species whose migrations do include geographic barriers, it is unclear how strongly migration should generally be expected to regulate population dynamics. For instance, although hazard rates were highest during trans-Saharan flights in our study, these flights accounted for only a relatively small proportion of the total number of mortality events experienced by godwits across their annual cycle. Instead the breeding season, which is substantially longer in duration than a trans-Saharan flight (μ = 75 and 2 d, respectively), resulted in more than twice the number of mortality events as did trans-Saharan flights. Given that trans-Saharan flights occupy such a short period of time, survival rates during these flights would have to be severely reduced before they become the limiting component of the godwit annual cycle (but see Sillett and Holmes, 2002; Leyrer et al., 2013). Nonetheless, understanding what is influencing mortality rates during trans-Saharan flights, and during flights over geographic barriers more generally (e.g., Ward et al., 2018), is necessary to predict how changing conditions may impact the population dynamics of migratory species.
Flexibility in Migratory Timing
Godwits also exhibited significant inter- and intra-individual variation in their migratory timing within and among years. For instance, individual godwits varied their departure timing from non-breeding sites by an average of 17 days and their arrival on the breeding grounds by an average of 11 days between consecutive years. Furthermore, within a single year, departure dates across the population from non-breeding sites in Africa could span nearly 5 months and arrival dates at breeding sites in The Netherlands could cover almost 2 months (see also Verhoeven et al., 2019). In turn, our previous work has indicated that this variation is neither influenced by an individual's non-breeding site—as godwits spending the non-breeding season in sub-Saharan Africa exhibit similar levels of intra-individual variation and repeatability to those spending it in Iberia (see Kentie et al., 2017; Verhoeven et al., 2019)—nor the length of its life—as godwits do not directionally change the timing of their migrations over the course of their lives (see Verhoeven et al., 2019). To the best of our knowledge, godwits thus display the largest degree of variation in migratory timing yet documented among obligate migratory birds (Both et al., 2016). For example, in other godwit species, departure and arrival dates can span a period as small as 10 days across entire breeding populations (Senner et al., 2014), while individuals can vary their migratory timing by as little as 4 days over the course of their lifetimes (Figure 2; Conklin et al., 2013; Gill et al., 2014; Senner et al., 2014). More broadly, even among those bird species exhibiting low levels of repeatability in migratory timing, their absolute levels of intra- and inter-individual variation are lower than those observed in godwits (Both et al., 2016).
Intriguingly, in addition to their high levels of intra- and inter-individual variation, godwits also exhibited significant levels of repeatability in nearly all of their migratory behaviors. As discussed extensively by Conklin et al. (2013), high levels of repeatability can arise for a number of different reasons, as the measure represents the ratio between intra- and inter-individual variation within a population. In godwits, their measures of high repeatability appear to result from the large degree of inter-individual variation exhibited by the population (see Verhoeven et al., 2019 for more details). Thus, individual godwits tend to time their annual cycles differently from one another, yet show significant flexibility in the timing of their movements within their separate migratory windows.
This high degree of individual-level flexibility and population-level variability could mean that godwits are better able to respond to environmental change than other long-distance migrants. For instance, unlike Hudsonian and bar-tailed godwits (Conklin et al., 2010; Senner et al., 2017), black-tailed godwits do not appear to be time constrained during their northward migration. This means that the fitness benefits for an individual of flexibly altering its migration timing, as well as the number of stops it makes during migration, may outweigh those of arriving at a specific site at a specific time. In turn, this may enable them to minimize the initiation of reversible state effects that carry-over the conditions experienced during previous time periods, potentially affecting their survival and fitness (sensu Senner et al., 2015c). Accordingly, we have previously shown that many godwits delayed their migrations by arriving more than 20 days later than normal to their breeding sites in response to an early spring snowstorm in northwest Europe, enabling individuals that delayed their migrations to avoid the most inclement storm-related conditions, but also subsequently achieve high reproductive success (Senner et al., 2015a).
Nonetheless, their flexibility does not enable godwits to adequately respond to all types of environmental change. During the past nine decades, for example, godwits have failed to shift the onset of their breeding season earlier (Meltofte et al., 2018) and have become increasingly mismatched with the local insect phenology on their breeding grounds, leading to reductions in their reproductive success that have compounded the reductions simultaneously incurred by broad-scale agricultural intensification (Kleijn et al., 2010; Schroeder et al., 2012; Kentie et al., 2018). As such, heightened flexibility may enable godwits to respond to some, but not necessarily all, environmental changes.
The Drivers of Migratory Flexibility
More broadly, there is mounting evidence that plasticity can drive advances in migratory timing in response to climate change (Gill et al., 2014), as well as the colonization of novel migratory routes within only a few generations (Eichhorn et al., 2009; Verhoeven et al., 2018). Flexible migratory behaviors are also not unique to godwits, as some ungulates are able to flexibly alter whether or not they migrate in a given year in response to environmental conditions and herd size (Eggeman et al., 2016). Furthermore, the farthest flying migratory birds can fly more than 12,000 km non-stop and maintain annual survival rates among the highest recorded across all bird species, suggesting the ability to flexibly respond to a wide range of conditions in flight (Conklin et al., 2013). These studies thus suggest that under the right circumstances, many migratory populations can exhibit significant levels of plasticity and flexibility. But, what are those circumstances?
We propose that high levels of plasticity and flexibility in migratory behaviors could be related to: (1) high variability in the cues used to time migration (Senner, 2012; Winkler et al., 2014); (2) a relaxation of selection on migratory timing in response to a lack of density dependent selection pressures (Day and Kokko, 2015); or, (3) strong selection on flexibility in migratory behaviors (Nussey et al., 2005). In the case of godwits, we hypothesize that a relaxation of selection on migratory timing resulting from the on-going godwit population decline is the most likely scenario. For instance, if the cues godwits use to migrate were highly variable, we would expect to observe that godwits exhibit low repeatability in addition to their considerable population-level variation in migratory timing (Conklin et al., 2013). Instead, individual godwits, while displaying high flexibility in their migratory behaviors, still appear to time their migrations very differently from each other (Verhoeven et al., 2019). Similarly, if density dependence were acting strongly on godwits, we would expect survival rates to vary with the size of the godwit population (Rakhimberdiev et al., 2015). However, we found little inter-annual variation in survival rates despite the fact that the godwit population size has fluctuated in recent years (Kentie et al., 2016). Furthermore, an individual's migratory timing is not influenced by the number of other godwit pairs breeding nearby and is uncorrelated with their subsequent reproductive success (Senner et al., unpublished data), suggesting that selection on migratory timing has been relaxed. Finally, the creation of new, artificial, wetlands throughout their range has led to an expansion of the amount of habitat available for adult godwits during the non-breeding season and enabled a series of rapid changes to godwit migration patterns (Márquez-Ferrando et al., 2014; Verhoeven et al., 2018).
In combination, density dependent pressures do not currently appear to be influencing godwit survival or migratory timing and this may now mean that adult godwits exist below their carrying capacity throughout the year. Unfortunately, however, we currently lack the data to robustly assess these three possibilities and future studies should therefore endeavor to identify those circumstances that may enable migratory populations to exhibit high levels of flexibility and plasticity in their migratory behaviors. Developing a deeper understanding of why some populations are more flexible than others will both aid conservation efforts, but also help revise our view of what is normal and possible for migrants (Conklin et al., 2017).
Author Contributions
NS, MV, and TP designed the project. All authors carried out the fieldwork. NS and MS carried out the analyses. NS wrote the manuscript. All authors contributed edits.
Conflict of Interest Statement
The authors declare that the research was conducted in the absence of any commercial or financial relationships that could be construed as a potential conflict of interest.
Acknowledgments
We thank the many members of our field crews from 2004 to 2015 for their assistance in the field. We also thank S. Pardal, M. Parejo-Nieto, A. Villegas-Sánchez, and the rest of the teams from Badajoz and Lisboa for help with satellite transmitter instrumentation. A. Stokman, W. Nauta, S. Venema, Staatsbosbeheer, It Fryske Gea, ANV Súdwesthoeke, and Kuststripe, and many other land managers and farmers were gracious in allowing us access to their land. Local bird conservation communities—including Fûgelwachten Makkum, Warkum, Koudum-Himmelum, Oudega, Gaastmeer, and Stavoren-Warns—provided locations of many nests. E. Rakhimberdiev greatly assisted with the geolocator analyses, J. R. Conklin helpfully made available his bar-tailed godwit repeatability data, and T. L. Tibbitts curated the satellite tracking data from 2013 to 2015. Funding for NS, MV, and their fieldwork was provided by NWO-ALW TOP grant Shorebirds in space (854.11.004) awarded to TP. RK is funded by the Royal Society. JA benefited from a Fundação para a Ciência e Tecnologia grant (SFRH/BPD/91527/2012). Long-term godwit research was funded by the Kenniskring weidevogels of the former Ministry of Agriculture, Nature Management and Food Safety (2007–2010, 2012, 2016); the Province of Fryslân (2013–2016); and the Spinoza Premium 2014 of the Netherlands Organization for Scientific Research (NWO) awarded to TP. Additional financial support came from the Prins Bernhard Cultuurfonds (through It Fryske Gea), the Van der Hucht de Beukelaar Stichting, the Paul and Louise Cook Endowment Ltd., the University of Groningen, BirdLife-Netherlands, and WWF-Netherlands. This work was done under license number 6350A, C, and G following the Dutch Animal Welfare Act Articles 9 and 11.
Supplementary Material
The Supplementary Material for this article can be found online at: https://www.frontiersin.org/articles/10.3389/fevo.2019.00096/full#supplementary-material
References
Alves, J. A., Gunarsson, T. G., Hayhow, D. B., Appleton, G. F., Potts, P. M., Sutherland, W. J., et al. (2013). Costs, benefits, and fitness consequences of different migratory strategies. Ecology 94, 11–17. doi: 10.1890/12-0737.1
Alves, J. A., and Lourenço, P. M. (2014). Estimating flight ranges to unravel migratory strategies: spring migration of continental Black-tailed Godwits. Bird Conserv. Int. 24, 214–222. doi: 10.1017/S0959270913000324
Bates, D., Maechler, M., Bolker, B., Walker, S., Bojesen, R. H., Singmann, H., et al. (2014). Linear Mixed-Effect Models Using Eigen and S4. Available online at: http://www.r-project.org (accessed April 24, 2018).
Both, C., Bijlsma, R. G., and Ouwehand, J. (2016). Repeatability in spring arrival dates in pied flycatchers varies among years and sexes. Ardea 104, 3–21. doi: 10.5253/arde.v104i1.a1
Both, C., Bouwhuis, S., Lessells, C. M., and Visser, M. E. (2006). Climate change and population declines in a long-distance migratory bird. Nature 441, 81–83. doi: 10.1038/nature04539
Bouten, W., Baaij, E. W., Shamoun-Baranes, J., and Camphuysen, C. J. (2013). A flexible GPS tracking system for studying bird behaviour at multiple scales. J. Ornithol. 154, 571–580. doi: 10.1007/s10336-012-0908-1
Boyd, J. D., and Brightsmith, D. J. (2013). Error properties of Argos satellite telemetry locations using least squares and Kalman filtering. PLoS ONE 8:e63051. doi: 10.1371/journal.pone.0063051
Burnham, K. P., and Anderson, D. R. (2002). Model Selection and Multimodel Inference: A Practical Information-Theoretic Approach. New York, NY: Springer.
Conklin, J. R., Battley, P. F., and Potter, M. A. (2013). Absolute consistency: individual versus population variation in annual-cycle schedules of a long-distance migrant bird. PLoS ONE 8:e54535. doi: 10.1371/journal.pone.0054535
Conklin, J. R., Battley, P. F., Potter, M. A., and Fox, J. W. (2010). Breeding latitude drives individual schedules in a trans-hemispheric migrant bird. Nat. Commun. 1:67. doi: 10.1038/ncomms1072
Conklin, J. R., Senner, N. R., Battley, P. F., and Piersma, T. (2017). Extreme migration and the individual quality spectrum. J. Avian Biol. 48, 19–36. doi: 10.1111/jav.01316
Day, E., and Kokko, H. (2015). Relaxed selection when you least expect it: why declining bird populations might fail to respond to phenological mismatches. Oikos 124, 62–68. doi: 10.1111/oik.01340
Douglas, D. C., Weinzierl, R., Davidson, S. C., Kays, R., Wikelski, M., and Bohrer, G. (2012). Moderating Argos location errors in animal tracking data. Methods Ecol. Evol. 3, 999–1007. doi: 10.1111/j.2041-210X.2012.00245.x
Eggeman, S. L., Hebblewhite, M., Bohm, H., Whittington, J., and Merrill, E. H. (2016). Behavioural flexibility in migratory behaviour in a long-lived large herbivore. J. Anim. Ecol. 85, 785–797. doi: 10.1111/1365-2656.12495
Eichhorn, G., Drent, R. H., Stahl, J., Leito, A., and Alerstam, T. (2009). Skipping the Baltic: the emergence of a dichotomy of alternative spring migration strategies in Russian barnacle geese. J. Anim. Ecol. 78, 63–72. doi: 10.1111/j.1365-2656.2008.01485.x
Gangoso, L., López-López, P., Grande, J. M., Mellone, U., Limiñana, R., Urios, V., et al. (2013). Ecological specialization to fluctuating resources prevents long-distance migratory raptors from becoming sedentary on islands. PLoS ONE 8:e61615. doi: 10.1371/journal.pone.0061615
Gill, J. A., Alves, J. A., Sutherland, W. J., Appleton, G. F., Potts, P. M., and Gunnarsson, T. G. (2014). Why is timing of bird migration advancing when individuals are not? Proc. Biol. Sci. 281:20132161. doi: 10.1098/rspb.2013.2161
Gill, J. A., Langston, R. H. W., Alves, J. A., Atkinson, P. W., Bocher, P., Vieira, N. C., et al. (2007). Contrasting trends in two black-tailed godwit populations: a review of causes and recommendations. Wader Study Group Bull. 114, 43–50.
Grueber, C. E., Nakagawa, S., Laws, R. J., and Jamieson, I. G. (2011). Multimodel inference in ecology and evolution: challenges and solutions. J. Evol. Biol. 24, 699–711. doi: 10.1111/j.1420-9101.2010.02210.x
Hebblewhite, M., and Haydon, D. T. (2010). Distinguishing technology from biology: a critical review of the use of GPS telemetry data in ecology. Philos. Trans. R. Soc. B. 365, 2303–2312. doi: 10.1098/rstb.2010.0087
Hebblewhite, M., and Merrill, E. H. (2011). Demographic balancing of migrant and resident elk in a partially migratory population through forage-predation tradeoffs. Oikos. 120, 1860–1870. doi: 10.1111/j.1600-0706.2011.19436.x
Hewson, C. M., Thorup, K., Pearce-Higgins, J. W., and Atkinson, P. W. (2016). Population decline is linked to migration route in the common cuckoo. Nat. Commun. 7:12296. doi: 10.1038/ncomms12296
Hooijmeijer, J. C. E. W., Senner, N. R., Tibbitts, T. L., Gill, RE Jr, Douglas, D. C., Bruinzeel, L. W., et al. (2013). Post-breeding migration of Dutch-breeding black- tailed godwits: timing, routes, use of stopovers, and nonbreeding destinations. Ardea. 101, 141–152. doi: 10.5253/078.101.0209
Kentie, R., Coulson, T., Hooijmeijer, J. C. E. W., Howison, R. A., Loonstra, A. H. J., Verhoeven, M. A., et al. (2018). Warming springs and habitat alteration interact to impact timing of breeding and population dynamics in a migratory bird. Glob. Chang. Biol. 24, 5292–5303. doi: 10.1111/gcb.14406
Kentie, R., Márquez-Ferrando, R., Figuerola, J., Gangoso, L., Hooijmeijer, J. C. E. W., Loonstra, A. H. J., et al. (2017). Does wintering north or south of the Sahara correlate with timing and breeding performance in black-tailed godwits? Ecol. Evol. 7, 2812–2820. doi: 10.1002/ece3.2879
Kentie, R., Senner, N. R., Hooijmeijer, J. C. E. W., Márquez-Ferrando, R., Figuerola, J., Masero, J. A., et al. (2016). Estimating the size of the Dutch breeding population of continental black-tailed godwits from 2007–2015 using resighting data from spring staging sites. Ardea. 114, 213–225. doi: 10.5253/arde.v104i3.a7
Klaassen, R. H., Hake, M., Strandberg, R., Koks, B. J., Trierweiler, C., Exo, K. M., et al. (2014). When and where does mortality occur in migratory birds? Direct evidence from long-term satellite tracking of raptors. J. Anim. Ecol. 83, 176–184. doi: 10.1111/1365-2656.12135
Klaassen, R. H. G., Strandberg, R., Hake, M., Olofsson, P., Tøttrup, A. P., and Alerstam, T. (2010). Loop migration in adult marsh harriers Circus aeruginosus as revealed by satellite telemetry. J. Avian Biol. 41, 200–207. doi: 10.1111/j.1600-048X.2010.05058.x
Kleijn, D., Schekkerman, H., Dimmers, W. J., van Kats, R. J. M., Melman, D., and Teunissen, W. A. (2010). Adverse effects of agricultural intensification and climate change on breeding habitat quality of black-tailed godwits Limosa l. limosa in The Netherlands. Ibis. 152, 475–486. doi: 10.1111/j.1474-919X.2010.01025.x
Kuznetsova, A., Brockhoff, B., and Christensen, H. B. (2015). Tests in Linear Mixed Effect Models. Available online at: http://www.r-project.org (accessed April 24, 2018).
Leyrer, J., Lok, T., Brugge, M., Spaans, B., Sandercock, B. K., and Piersma, T. (2013). Mortality within the annual cycle: seasonal survival patterns in Afro-Siberian red knots Calidris canutus canutus. J. Ornithol. 154, 933–943. doi: 10.1007/s10336-013-0959-y
Lok, T., Overdijk, O., and Piersma, T. (2015). The cost of migration: spoonbills suffer higher mortality during trans-Saharan spring migrations only. Biol. Lett. 11:20140944. doi: 10.1098/rsbl.2014.0944
Lok, T., Overdijk, O., Tinbergen, J. M., and Piersma, T. (2013). Seasonal variation in density dependence in age-specific survival of a long-distance migrant. Ecology. 94, 2358–2369. doi: 10.1890/12-1914.1
Loonstra, A. H. J., Verhoeven, M. A., Senner, N. R., Hooijmeijer, J. C. E. W., Piersma, T., and Kentie, R. (2019). Natal habitat type and sex-specific survival rates result in a male-biased adult sex ratio. Behav. Ecol. 30. doi: 10.1093/beheco/arz021
Lourenço, P. M., Kentie, R., Schroeder, J., Alves, J. A., Groen, N. M., Hooijmeijer, J. C. E. W., et al. (2010). Phenology, stopover dynamics and population size of migrating black-tailed godwits Limosa limosa limosa in Portuguese rice plantations. Ardea 98, 35–42. doi: 10.5253/078.098.0105
Lourenço, P. M., Kentie, R., Schroeder, J., Groen, N. M., Hooijmeijer, J. C. E. W., and Piersma, T. (2011). Repeatable timing of northward departure, arrival and breeding in black-tailed godwits Limosa l. limosa, but no domino effects. J. Ornithol. 152, 1023–1032. doi: 10.1007/s10336-011-0692-3
Lourenço, P. M., and Piersma, T. (2008). Stopover ecology of black-tailed godwits Limosa limosa limosa in Portuguese rice fields: a guide on where to feed in winter. Bird Study 55, 194–202. doi: 10.1080/00063650809461522
Márquez-Ferrando, R., Figuerola, J., Hooijmeijer, J. C. E. W., and Piersma, T. (2014). Recently created man-made habitats in Doñana provide alternative wintering space for the threatened Continental European black-tailed godwit population. Biol. Conserv. 171, 127–135. doi: 10.1016/j.biocon.2014.01.022
Masero, J. A., Santiago-Quesada, F., Sánchez-Guzmán, J. M., Villegas, A., Abad-Gómez, J. M., Lopes, R. J., et al. (2011). Long lengths of stay, large numbers, and trends of the black-tailed godwit Limosa limosa in rice fields during spring migration. Bird Conserv. Int. 21, 12–24. doi: 10.1017/S0959270910000092
Mazerolle, M. J. (2013). Model Selection and Multimodel Inference Based on QAICc. Available online at: http://www.r-project.org (accessed April 24, 2018).
Meltofte, H., Amstrup, O., Petersen, T. L., Rigét, F., and Tøttrup, A. P. (2018). Trends in breeding phenology across ten decades show varying adjustments to environmental changes in four wader species. Bird Study. 65, 44–51. doi: 10.1080/00063657.2018.1444014
Nussey, D. H., Postma, E., Gienapp, P., and Visser, M. E. (2005). Selection on heritable phenotypic plasticity in a wild bird population. Science 310, 304–306. doi: 10.1126/science.1117004
Pedler, R. D., Ribot, R. F. H., and Bennett, A. T. D. (2018). Long-distance flights and high-risk breeding by nomadic waterbirds on desert salt lakes. Conserv. Biol. 32, 216–228. doi: 10.1111/cobi.13007
Piersma, T. (2011). Flyway evolution is too fast to be explained by the modern synthesis: proposals for an ‘extended’ evolutionary research agenda. J. Ornithol. 152, S151–S159. doi: 10.1007/s10336-011-0716-z
Piersma, T., Lok, T., Chen, Y., Hassell, C. J., Yang, H.-Y., Boyle, A., et al. (2016). Simultaneous declines in summer survival of three shorebird species signals a flyway at risk. J. Appl. Ecol. 53, 479–490. doi: 10.1111/1365-2664.12582
R Development Core Team (2016). R: A Language and Environment for Statistical Computing. Vienna: R Foundation for Statistical Computing. Available online at: http://www.r-project.org (accessed April 24, 2018).
Rakhimberdiev, E., Saveliev, A., Piersma, T., and Karagicheva, J. (2017). FLightR: an R package for reconstructing animal paths from solar geolocation loggers. Methods Ecol. Evol. 8, 1482–1487. doi: 10.1111/2041-210X.12765
Rakhimberdiev, E., Senner, N. R., Verhoeven, M. A., Winkler, D. W., Bouten, W., and Piersma, T. (2016). Comparing inferences of solar geolocation data against high-precision GPS data: annual movements of a double-tagged black-tailed godwit. J. Avian Biol. 47, 589–596. doi: 10.1111/jav.00891
Rakhimberdiev, E., van den Hout, P. J., Brugge, M., Spaans, B., and Piersma, T. (2015). Seasonal mortality and sequential density dependence in a migratory bird. J. Avian Biol. 46, 332–341. doi: 10.1111/jav.00701
Romer, J. D., Gitelman, A. I., Clements, S., and Schreck, C. B. (2015). Designing a monitoring program to estimate estuarine survival of anadromous salmon smolts: simulating the effect of sample design on inference. PLoS ONE. 10:e0132912. doi: 10.1371/journal.pone.0132912
Roodbergen, M., Klok, C., and Schekkerman, H. (2008). The ongoing decline of the breeding population of black-tailed godwits Limosa l. limosa in The Netherlands is not explained by changes in adult survival. Ardea. 96, 207–218. doi: 10.5253/078.096.0206
Rushing, C. S., Ryder, T. B., and Marra, P. P. (2016). Quantifying drivers of population dynamics for a migratory bird throughout the annual cycle. Proc. R. Soc. B. 283:20152846. doi: 10.1098/rspb.2015.2846
Schmaljohann, H., Liechti, F., and Bruderer, B. (2007). Songbird migration across the Sahara: the non-stop hypothesis rejected! Proc. R. Soc. B. 274, 735–739. doi: 10.1098/rspb.2006.0011
Schroeder, J., Piersma, T., Groen, N. M., Hooijmeijer, J. C. E. W., Kentie, R., Lourenço, P. M., et al. (2012). Reproductive timing and investment in relation to spring warming and advancing agricultural schedules. J. Ornithol. 153, 327–336. doi: 10.1007/s10336-011-0747-5
Senner, N. R. (2012). One species, but two patterns: populations of the Hudsonian Godwit (Limosa haemastica) differ in spring migration timing. Auk. 129, 670–682. doi: 10.1525/auk.2012.12029
Senner, N. R., Conklin, J. R., and Piersma, T. (2015c). An ontogenetic perspective on individual differences. Proc. R. Soc. B 282:20151050. doi: 10.1098/rspb.2015.1050
Senner, N. R., Hochachka, W. M., Fox, J. W., and Afanasyev, V. (2014). An exception to the rule: carry-over effects do not accumulate in a long-distance migratory bird. PLoS ONE 9:e86588. doi: 10.1371/journal.pone.0086588
Senner, N. R., Stager, M., and Sandercock, B. K. (2017). Ecological mismatches are moderated by local conditions for two populations of a long-distance migratory bird. Oikos 126, 61–72. doi: 10.1111/oik.03325
Senner, N. R., Stager, M., Verhoeven, M. A., Cheviron, Z. A., Piersma, T., and Bouten, W. (2018). High-altitude shorebird migration in the absence of topographical barriers: avoiding high air temperatures and searching for profitable winds. Proc. R. Soc. B. 285:20180569. doi: 10.1098/rspb.2018.0569
Senner, N. R., Verhoeven, M. A., Abad-Gómez, J. M., Gutiérrez, J. S., Hooijmeijer, J. C. E. W, Kentie, R., Masero, J. A., et al. (2015a). When Siberia came to The Netherlands: the response of black-tailed godwits to a rare spring weather event. J. Anim. Ecol. 84, 1164–1176. doi: 10.1111/1365-2656.12381
Senner, N. R., Verhoeven, M. A., Hooijmeijer, J. C., and Piersma, T. (2015b). Just when you thought you knew it all: new evidence for flexible breeding patterns in continental black-tailed godwits. Wader Study. 122, 18–24. doi: 10.18194/ws.00006
Sergio, F., Tanferna, A., De Stephanis, R., Jiménez, L. L., Blas, J., Tavecchia, G., et al. (2014). Individual improvements and selective mortality shape lifelong migratory performance. Nature. 515, 410–413. doi: 10.1038/nature13696
Sillett, T. S., and Holmes, R. T. (2002). Variation in survivorship of a migratory songbird throughout its annual cycle. J. Anim. Ecol. 71, 296–308. doi: 10.1046/j.1365-2656.2002.00599.x
Stanley, C. Q., MacPherson, M., Fraser, K. C., McKinnon, E. A., and Stutchbury, B. J. (2012). Repeat tracking of individual songbirds reveals consistent migration timing but flexibility in route. PLoS ONE. 7:e40688. doi: 10.1371/journal.pone.0040688
Stoffel, M. A., Nakagawa, S., and Schielzeth, H. (2017). rptR: repeatability estimation and variance decomposition by generalized linear mixed-effects models. Methods Ecol. Evol. 8, 1639–1644. doi: 10.1111/2041-210X.12797
Therneau, T. M., and Lumley, T. (2015). Survival Analysis. Available online at: http://lme4.r-forge.r-project.org (accessed November 1, 2015).
Verhoeven, M. A., Loonstra, A. H. J., Hooijmeijer, J. C. E. W., Masero, J. A., Piersma, T., and Senner, N. R. (2018). Generational shift in spring staging site use by a long-distance migratory bird. Biol. Lett. 14:20170663. doi: 10.1098/rsbl.2017.0663
Verhoeven, M. A., Loonstra, A. H. J., Senner, N. R., McBride, A. D., Both, C., and Piersma, T. (2019). Variation from an unknown source: large inter-individual differences in migrating black-tailed godwits. Front. Ecol. Evol. 7:31. doi: 10.3389/fevo.2019.00031
Ward, M. P., Benson, T. J., Deppe, J., Zenzal, T. J. Jr, Diehl, R. H., Celis-Murillo, A., et al. (2018). Estimating apparent survival of songbirds crossing the Gulf of Mexico during autumn migration. Proc. R. Soc. B 285:20181747. doi: 10.1098/rspb.2018.1747
Watts, B. D., Smith, F. M., Hamilton, D. J., Keyes, T., Paquet, J., Pirie-Dominix, L., et al. (2019). Seasonal variation in mortality rates for Whimbrels (Numenius phaeopus) using the Western Atlantic Flyway. Condor. 121, 1–13. doi: 10.1093/condor/duy001
Wilcove, D. S., and Wikelski, M. (2008). Going, going, gone: is animal migration disappearing? PLoS Biol. 6:e188. doi: 10.1371/journal.pbio.0060188
Winkler, D. W., Jørgensen, C., Both, C., Houston, A. I., McNamara, J. M., Levey, D. J., et al. (2014). Cues, strategies, and outcomes: how migrating vertebrates track environmental change. Mov. Ecol. 2:10. doi: 10.1186/2051-3933-2-10
Wootherspoon, S., Sumner, M., and Lisovski, S. (2013). Basic Data Processing for Light Based Geolocation Archival Tags. Available online at: http://lme4.r-forge.r-project.org (accessed April 24, 2018).
Keywords: repeatability, phenotypic flexibility, seasonal survival, migration, annual cycle
Citation: Senner NR, Verhoeven MA, Abad-Gómez JM, Alves JA, Hooijmeijer JCEW, Howison RA, Kentie R, Loonstra AHJ, Masero JA, Rocha A, Stager M and Piersma T (2019) High Migratory Survival and Highly Variable Migratory Behavior in Black-Tailed Godwits. Front. Ecol. Evol. 7:96. doi: 10.3389/fevo.2019.00096
Received: 23 September 2018; Accepted: 11 March 2019;
Published: 09 April 2019.
Edited by:
David Costantini, Muséum National d'Histoire Naturelle, FranceReviewed by:
Diego Rubolini, University of Milan, ItalyCas Eikenaar, Institute of Avian Research, Germany
Michael P. Ward, University of Illinois at Urbana-Champaign, United States
Copyright © 2019 Senner, Verhoeven, Abad-Gómez, Alves, Hooijmeijer, Howison, Kentie, Loonstra, Masero, Rocha, Stager and Piersma. This is an open-access article distributed under the terms of the Creative Commons Attribution License (CC BY). The use, distribution or reproduction in other forums is permitted, provided the original author(s) and the copyright owner(s) are credited and that the original publication in this journal is cited, in accordance with accepted academic practice. No use, distribution or reproduction is permitted which does not comply with these terms.
*Correspondence: Nathan R. Senner, bmF0aGFuLnNlbm5lckBnbWFpbC5jb20=