- 1Appalachian Laboratory, University of Maryland Center for Environmental Science, Frostburg, MD, United States
- 2Department of Plant Biology, University of Vermont, Burlington, VT, United States
Animal mating systems have fascinated biologists for thousands of years. Ways to describe a mating system include determining social organization, observing copulations, or using genetics to assign parentage. Social organization can be difficult to quantify, however, documentation of copulations is often challenging, many copulations do not produce offspring, and genetic variation is sometimes minimal. Here we use data from a 7-year study of wild white-tailed prairie dogs (WTPDs, Cynomys leucurus) living in Colorado USA to estimate the frequencies of polyandry (i.e., copulation with ≥2 males) and polygyny (i.e., copulation with ≥2 females) from three independent approaches: (1) determination of the number of males and females living in the same territory (social organization); (2) observations of copulations; and (3) genetic assignments of paternity from seven polymorphic microsatellites. We predicted that our three approaches would yield similar estimates of polyandry and polygyny. Because a WTPD female's period of sexual receptivity each spring is limited to several hours on a single day, we also predicted that frequencies of polyandry and polygyny would be lower for WTPDs than for animals with longer periods of receptivity. Our results did not support these predictions. For polyandry, observations of copulations and genetics indicated similar overall percentages (27%), but social organization indicated a much lower percentage (3%). For polygyny, observations of copulations indicated the highest overall percentage (84%), then social organization (59%), then genetics (46%). All three approaches showed striking annual variation in the frequencies of WTPD polyandry and polygyny. Long-term studies that integrate behavioral and genetic insights can provide a detailed view of a mating system, but feasibility will depend on ease of capture, visibility of copulations, length of mating season, research objectives, and genetic variation.
Introduction
Biologists have been studying mating systems for >2,300 years (Darwin, 1871; Aristotle, 1943; Trivers, 1972; Smith, 1984; Eberhard, 1996; Parker and Birkhead, 2013). Despite this longstanding interest, the descriptions of mating systems can be confusing and controversial, and subject to different interpretations depending on how they are quantified (Wickler and Seibt, 1983; Gowaty, 1985; Westneat et al., 1990; Klug, 2018). For example, behavioral ecologists used the term “polyandry” in the 1970s to describe an overall mating system in which the variance in female lifetime reproductive success (LRS) is greater than the variance in male LRS (Trivers, 1972; Emlen and Oring, 1977; Alexander et al., 1979). More recently, however, behavioral ecologists have used the term “polyandry” to indicate copulation with ≥2 males by a single female (Zeh and Zeh, 2003; Bretman and Tregenza, 2005; Firman and Simmons, 2008; Johnson and Brockmann, 2010; Gowaty, 2012; Zhao et al., 2016). In an attempt to reduce confusion, we use the terms defined in Table 1 throughout this report to describe a mating system.
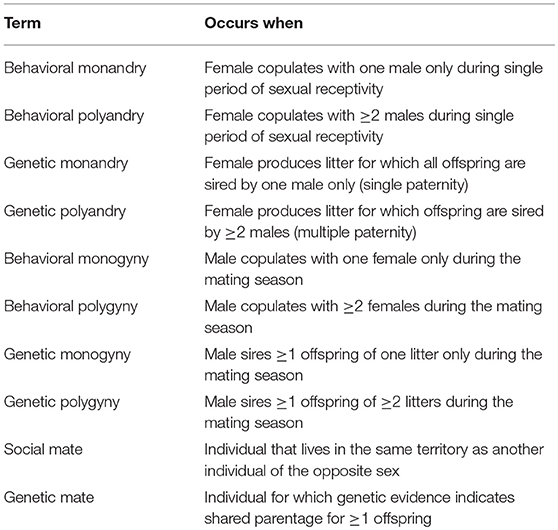
Table 1. Behavioral and genetic definitions for monandry, polyandry, monogyny, polygyny, social mate, and genetic mate for white-tailed prairie dogs.
An early way to estimate the number of sexual partners for males and females of a species was to observe the social organization. For an avian or mammalian species in which the typical pattern is for a single male and a single female to care for juveniles in an isolated nest or den, for example, a behavioral ecologist might hypothesize that females are behaviorally monandrous and males are behaviorally monogynous (Lack, 1968; Kleiman, 1977; Wittenberger and Tilson, 1980; Getz et al., 1990; Mock and Fujiok, 1990; Reichard and Boesch, 2003; Klug, 2018). For species for which the typical pattern is for several sexually mature females to rear offspring in separate nests in the same territory (i.e., the same physical area) with a single resident sexually mature male, we might hypothesize that females are behaviorally monandrous and males are behaviorally polygynous (Orians, 1969; Bartholomew, 1970; Downhower and Armitage, 1971; Emlen and Oring, 1977; Thornhill, 1981). For many species, however, a clear social organization that shows ≥1 specific female(s) living in the same territory with a specific male is not evident (e.g., one sex or the other frequently moves between territories) (Birkhead and Moller, 1992; Shuster and Wade, 2003; Danchin et al., 2008; Oliveria et al., 2008; Raveh et al., 2010; Clutton-Brock, 2016).
A better way to estimate the number of sexual partners for males and females for certain species is to observe copulations. If both males and females usually copulate with a single partner of the opposite sex, we might hypothesize that most females are behaviorally monandrous and most males are behaviorally monogynous (Kleiman, 1977; Black, 1996; Lukas and Clutton-Brock, 2013). If each female usually copulates with a single male and each male usually copulates with several females, however, we might hypothesize that most females are behaviorally monandrous and most males are behaviorally polygynous (Gladstone, 1979; Berger and Cunningham, 1991; Gibson et al., 1991; Alatalo et al., 1996). One problem with describing a mating season solely from observations of copulations is that one can almost never be certain that (s)he has detected every single mating for a male or female, for at least three reasons. First, for many species, copulations under natural conditions are often difficult to observe (Westneat et al., 1990; Birkhead and Moller, 1992, 1998; Byers, 1997). Second, a female's period of sexual receptivity extends over several days for many species (Dewsbury, 1975; Beach, 1976; Smuts et al., 1987; Birkhead and Moller, 1992, 1998), so that documenting every copulation is challenging. Third, a female of many species sometimes roams over long distances while she is sexually receptive (Westneat et al., 1990; Berger and Cunningham, 1991, 1994; Birkhead and Moller, 1992, 1998; Byers, 1997), so that tracking a receptive female and observing all her copulations are difficult.
A third way to define a mating system is to identify the possible sexual partners for males and females, and then use genetic markers to assign probability of maternity and paternity (Hanken and Sherman, 1981; Burke et al., 1989; Moore and Ball, 2002; Solomon et al., 2004; Weinman et al., 2015). If clutches or litters typically show single maternity and paternity, while also showing that males frequently sire juveniles of several clutches, then we can hypothesize that females are usually genetically monandrous and males are usually genetically polygynous (Ribble, 1991; Decker et al., 1993). Genetic assignments of paternity have the potential to reveal subtle nuances in a mating system that might not be apparent from behavioral observations alone (e.g., extra-pair paternity arising from undetected copulations). However, while genetic methods can be powerful for resolving paternity when genotypic diversity within a population is high, they suffer from reduced precision in situations when candidate males are close kin (e.g., two full brothers) and therefore genetically similar, or when the number of polymorphic loci and the number of alleles per locus are small (Blouin, 2003; Bonin et al., 2004; Coltman, 2005; Csilléry et al., 2006; Fernandez and Toro, 2006).
Single paternity of a clutch or litter (i.e., genetic monandry) can result even when a female copulates with ≥2 males (i.e., behavioral polyandry), for at least two reasons. First, via “sperm competition,” sperm from one male are sometimes better than sperm from other males at fertilizing eggs (Parker, 1970, 1984; Dewsbury, 1984; Moller and Birkhead, 1989; Wigby and Chapman, 2004; Firman and Simmons, 2008). Second, via “cryptic female choice,” a female that copulates with ≥2 males might selectively use sperm from only one male to fertilize all her eggs (Eberhard, 1996; Reeder, 2003).
Combining information from several approaches can lead to a richer understanding of animal mating systems, and reveal behavioral complexity that might not be apparent from relying on just one approach. Here we describe the results of research that compares behavioral and genetic estimates of polyandry and polygyny from social organization, observations of copulations, and assignments of paternity from microsatellites for white-tailed prairie dogs (Cynomys leucurus, hereafter “WTPDs”) living under natural conditions over 7 consecutive years. Many previous short-term studies with other species have yielded estimates of behavioral or genetic polyandry and polygyny from one or two of these methods (e.g., Lack, 1968; Orians, 1969; Westneat et al., 1990; Birkhead and Moller, 1992, 1998; Davies, 1992; Alatalo et al., 1996; Goossens et al., 1998; Dugdale et al., 2007; Raveh et al., 2011; Gowaty, 2012), but none has integrated large sample sizes for all three approaches in a wild population over many years.
Study Animals and Methods
Study Animals
WTPDs are large (500–900 g for adults, which are ≥9 months old), colonial, diurnal, burrowing, herbivorous rodents of the squirrel family (Sciuridae) (Clark et al., 1971; Hoogland and Brown, 2016) (Figures 1, 2). Within colonies, WTPDs live in territorial, contiguous family groups called clans, which typically contain 1 sexually mature male (hereafter, simply “male”), 2–5 sexually mature females (hereafter, simply “females”), 1–2 yearling adult males that have not reached sexual maturity, and (in June and July) 5–10 weaned juveniles (≤2 months after weaning). A female usually remains in the natal territory for life, and first copulates in her first spring (when about 9 months old). A WTPD male, by contrast, usually remains in the natal territory until he reaches sexual maturity, and then disperses in the first or second year after weaning (Hoogland, 2013a); most males do not first copulate until the second spring (when about 21 months old), but a few copulate in the first spring.
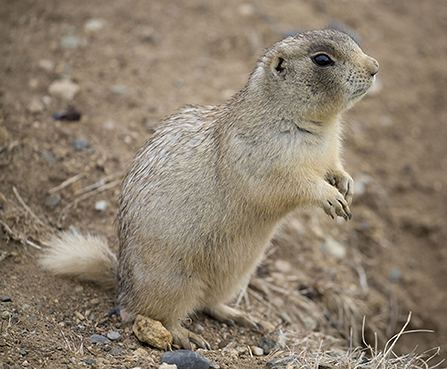
Figure 1. Adult white-tailed prairie dog at Arapaho National Wildlife Refuge, Colorado. Photo by Elaine Miller Bond, www.elainemillerbond.com (Reproduced with permission).
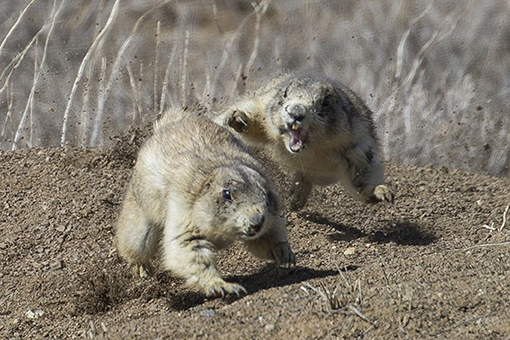
Figure 2. Two male white-tailed prairie dogs fighting during the mating season in April at the Arapaho National Widlife Refuge, Colorado. Males that win fights and chases are better able to monopolize, and copulate with, estrous females. Photo by Elaine Miller Bond, www.elainemillerbond.com (Reproduced with permission).
WTPD adults of both sexes defend the home territory against WTPDs from other clans. Within the home territory, each WTPD mother defends, and commonly forages in, a small area around the nursery-burrow containing her offspring against WTPDs of both sexes of the home clan. This maternal defense ceases soon after almost-weaned 5.5 week old juveniles emerge from their nursery-burrows in late May or early June (Clark, 1977). Males occasionally interact (mostly amicably) with juveniles of the home territory after the juveniles appear aboveground, but they avoid the nursery burrows with offspring throughout the period of lactation.
We studied WTPDs at the Case Ranch (40.6634°N, 106.3210°W) of the Arapaho National Wildlife Refuge (ANWR) near Walden, Jackson County, Colorado, USA, at an elevation of approximately 2,500 m, from 2006 through 2012.
Marking and Observing
We used 15 × 15 × 60 cm double-door Tomahawk livetraps (Tomahawk Livetrap Company, Tomahawk, Wisconsin, USA) baited with whole oats to capture adult WTPDs (Hoogland, 1995). We used unbaited 13 × 13 × 40 cm single-door Tomahawk livetraps to capture juveniles. We used a conical canvas bag that could be unzipped from either end to handle adult prairie dogs (Hoogland, 1995). We handled juveniles directly with gloves and no bag.
For permanent identification of each WTPD, we used uniquely numbered fingerling eartags (National Band and Tag Company, Newport, Kentucky, USA) (Hoogland, 1995; Hoogland and Brown, 2016). For identification of every adult and juvenile WTPD at the study-colony each year from a distance of ≤150 meters (Mean = 48 adults and 115 juveniles per year from 2007 through 2012), we used Nyanzol black fur dye (Greenville Colorants, Clifton, New Jersey, USA) to apply a unique number or symbol on both sides (Figure 3). Eartags usually remained with each WTPD for life, but Nyanzol markers remained only until molting of the winter fur in late spring or until molting of the summer fur in late summer. The combination of eartags and Nyanzol markers enabled us to identify the same individual every day within the same year and across years as well.
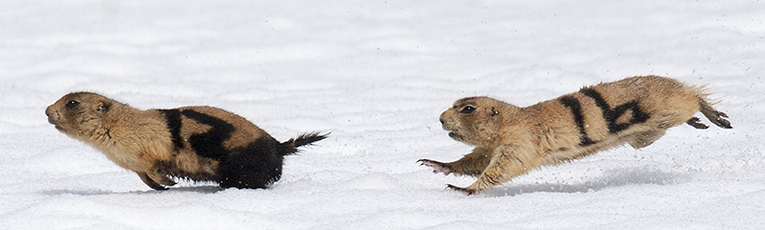
Figure 3. Earatagged, marked prairie dogs. Here male-16 (right) chases male Black-bottom-12 (left) during the mating season. Note silver eartag in left ear of each male. Every year, each WTPD at the study colony had a unique marker (with Nyanzol fur dye) and two National eartags with unique numbers. Photo by Elaine Miller Bond, www.elainemillerbond.com (Reproduced with permission).
Students (4–5 per year) and Hoogland used binoculars to observe marked WTPDs from 2-m high towers. We observed every day from dawn until dusk from early March through early July of seven consecutive years (2006 through 2012), for a total of >30,000 person-hours of watching.
Our research for handling, marking, and observing WTPDs complied with current laws of the USA, and was approved by the Institutional Animal Care and Use Committee (IACUC) of the University of Maryland Center for Environmental Science.
Assignment of Sibships and Maternity
Each WTPD mother reared her litter in an isolated nursery-burrow from parturition in late April or early May until her nearly-weaned offspring first appeared aboveground in late May or June when they were about 5.5 weeks old (Clark, 1977; Hoogland, 2013b). We captured, eartagged, marked, and took an ear sample from, all of a female's offspring. We determined sibships by livetrapping entire litters at the primary entrance (i.e., the entrance used most often by the mother and her offspring) to each nursery-burrow as soon as juveniles first appeared aboveground, and before the juveniles in a litter had an opportunity to mingle with young from other litters. We determined the mother of each litter by watching every day for the following three specific maternal behaviors that were salient during all of lactation (see also King, 1955; Hoogland, 2001, 2013b). First and most important, the lactating mother spent the night, with no other adults, in a single burrow from which her offspring eventually appeared aboveground. Second, the lactating mother defended the nursery-burrow containing her offspring during the day from other WTPDs of the home territory. Third, the mother frequently took mouthfuls of nest material (i.e., dry grass) into the nursery-burrow containing her offspring.
Determination of Social Organization From Compositions of Clans
We determined compositions of clans from behavioral observations in order to identify candidate fathers for genetic assignment of paternity for juveniles within a female's litter. Living in the same territory with a WTPD of the opposite sex did not necessarily lead to copulation with that WTPD. Further, the composition of WTPD clans changed over time within and across years. For our analyses we used the compositions of clans for late May and June, when almost-weaned juveniles emerged from their natal burrows for the first time, and when biologists are most likely to see and study WTPDs (e.g., Tileston and Lechleitner, 1966; Clark, 1977; Hoogland, 1981; Menkens et al., 1987). A WTPD male sometimes visited a territory for a day or two during the mating season and copulated with the female(s) there, and then returned to his home territory where he resided until June (see also Hoogland, 1995, 2013b). We scored this as a copulation with a male from a different clan. Similarly, when a female visited an adjacent territory on her day of estrus and copulated with a male living there, and then promptly returned to her home territory until June, we scored this as a copulation with a male from a different clan.
The best way to determine composition of clans is to document where WTPDs spend the night and where they forage shortly before (≤20 min) final submergence into a burrow for the night and shortly after (≤20 min) first emergence from a burrow in the morning (Hoogland, 1999; see also Hoogland et al., 2012). After juveniles had emerged from their natal burrows in June and when WTPD mothers were no longer defending their nursery-burrows, we classified WTPDs as members of the same clan if they consistently submerged for the night into the same burrow-entrance, or consistently emerged for the first time in the morning from the same burrow-entrance, or foraged in the same areas shortly before final submergences for the night or shortly after first emergences in the morning.
Documentation of Copulations
Of the 190 WTPD females for which we recorded estrus and copulations, 83 (44%) copulated aboveground; recording sexual partners for these females was straightforward. The other 107 females (56%) copulated exclusively underground, but several aboveground behaviors usually allowed us to identify sexual partners for these females. Specifically, we inferred a copulation when ≥3 of the following four criteria were satisfied (see also Hoogland, 2013b): (1) Usually after sniffing or licking a female's vulva, a male then followed her into a burrow for ≥5 min, and usually for ≥30 min, for an “underground consortship.” (2) With the estrous female nearby (≤2 m away), the male gave a unique vocalization (the “mating call”) within 5 min before or after an underground consortship. (3) The male or the female licked its genitals within 5 min after emerging from an underground consortship. (4) The estrous female remained aboveground much later than usual, typically 60–90 min after non-estrous females living in her territory had submerged into burrows for the night. Females that copulated aboveground consistently satisfied these same criteria (except 1). Other studies have used these same diagnostic behaviors to document underground copulations of three species of ground squirrels (Sherman, 1989; Lacey et al., 1997; Raveh et al., 2010) and three other species of prairie dogs (Hoogland, 1995, 1998a,b, 2007, 2013b).
Evidence that an underground consortship involved copulation and insemination was threefold (Hoogland, 1995, 1998a, 2007, 2013b): (1) The date of putative copulation(s) varied directly and strongly with the date of parturition (r ≥ 0.935 and P < 0.001 for each year, Pearson correlation test), which occurred 27–31 days after the putative date of copulation(s). (2) Over 95% of females had a sealed vulva 1–5 days before the date of putative copulation(s), but the vulva was invariably open for several days after putative copulation(s). (3) As explained below, patterns of paternity revealed by genetics were usually consistent with patterns inferred from observations of copulations.
By observing from dawn to dusk for the entire reproductive season of each year (i.e., ≥1 week before the first copulation in March through capture of the last weaned juvenile in June or July of 2006 through 2012), we documented 273 copulations by 190 females, and the weaning of 167 litters. The percentage per year for which we documented the number of sexual partners for females ranged from 16.1% (2007) to 100% (2012), with a mean ± SE of 75.2 ± 10.6%.
Collection of Tissue Samples for DNA Analyses
To obtain tissue for analyses of DNA, we collected a blood sample (2006 and 2007) or took a circular 2-mm tissue sample (2008 through 2012) from the right ear from each WTPD at the study-colony when we captured it for the first time as an adult or juvenile. To preserve the DNA, we immersed the sample into a labeled 2-ml vial with a solution composed of 0.25M EDTA pH7.5, 20% DMSO, and NaCl. We refrigerated the samples at approximately 7°C for 1–4 months until transport at the end of the field season to the Appalachian Laboratory in Frostburg, Maryland, where they were stored at −80° until we extracted DNA. Twenty-two samples (all from 2007) were lost during storage, leaving 801 samples available for genetic analysis.
Laboratory Analyses and Assignments of Maternity and Paternity
Total genomic DNA was extracted from either blood or ear-samples using the Qiagen DNeasy Blood and Tissue Kit (Qiagen, Hilden, Germany). With a Qiagen Multiplex PCR Kit, multiplex PCR was then conducted on the following seven microsatellite loci, all known to be polymorphic for WTPDs (Sackett et al., 2009): A119, C101, CA40-2, D12, D109, D115, and Taga27. Reactions were conducted in a 10 μl volume and consisted of the following final concentrations: 1X multiplex master mix; 0.5X Q-solution; 0.2 μM of each primer; 10–20 ng of DNA and brought up to volume with water. PCR reactions were run on an Eppendorf Mastercycler pro (Eppendorf, Hamburg, Germany) under the following parameters: 95°C for 15 min followed by 30 cycles of 94°C for 30 s; 60°C for 1.5 min; 72°C for 1.5 min; and a final extension step of 72°C for 30 min. PCR products were run on a 1.5% agarose gel to screen for amplification. Multiplex reactions were then sent to the Penn State Huck Institute of the Life Sciences Genomics Core Facility for genotyping on an Applied Biosystems 3730XL (Applied Biosystems, Foster City, California). Fragments were sized against the standard GS500LIZ using Applied Biosystem's Peak Scanner V1.0 software.
To assign genetic parentage to each WTPD offspring, we obtained maximum likelihood paternity assignments using Cervus 3.03 (Kalinowski et al., 2007). We assigned maternity from detailed behavioral observations (see Assignment of Sibships and Maternity), allowing us to focus statistical power on the more difficult issue of assigning paternity. For our analyses we deleted two offspring for which maternity was uncertain from behavioral observations. For every WTPD mother, we knew the male(s) that lived in her territory during the mating season, and all the males that lived in territories adjacent to her home territory (see “Determination of Social Organization from Compositions of Clans”). We used this information to create a list of candidate males for paternity for every weaned offspring. To determine appropriate confidence levels for assigning paternity in our dataset, we used Cervus 3.03 to simulate genotypes and determine the power of assigning parentage based on mating parameters that reflect the biology of WTPDs living at our study-colony. Specifically, we simulated a mating population with allele frequencies that matched our 7 microsatellite loci; we assumed 7 candidate fathers per offspring and a proportion of 0.98 loci typed (both based on observed averages from our datasets). We set a value of 0.95 for the proportion of candidate fathers sampled (i.e., we assumed we included 95% of all possible fathers in our analyses). We observed all males in our study-colony daily during the mating season each year.
We incorporated genotyping error into the analysis by genotyping sample duplicates that represent separate tissue collections from the same individual (N = 27) collected on different dates. These tissues were extracted independently for DNA, genotyped for the 7 loci, and compared for the reproducibility of their assigned alleles. Out of a total of 378 allelic comparisons (27 individuals × 7 loci × 2 diploid alleles per locus), we observed 2 mismatches, yielding an estimated genotyping error rate of 0.005. We used simulations with Cervus to obtain confidence thresholds for the log of odds score (LOD-score) of paternity for each candidate male under both strict (95% confidence, Critical LOD-score ≥3.51) and relaxed (80% confidence, Critical LOD-score ≥1.60) probabilities of accurate assignment. We then assigned the likelihood of paternity (LOP) based on identifying the candidate male with the highest LOD-score that exceeded the confidence threshold.
The accuracy of genetic assignments of paternity is driven in large part by the number of loci and their allelic diversity (Bernatchez and Duchesne, 2000; Blouin, 2003; Coltman, 2005). Despite extensive optimization of different primer sets, we identified only 7 polymorphic microsatellite loci at our study-colony that amplified reliably and showed high repeatability in genotype scoring. These 7 loci possessed only modest levels of diversity (see Results), and yielded a combined non-exclusion probability (i.e., no allelic mismatches between a juvenile and candidate male) of 0.925. Consequently, genetic assignments with this dataset alone were unlikely to provide sufficient resolution to obtain accurate estimates of paternity for every WTPD offspring at our study-colony. We therefore combined information from genetic assignments, observations of copulations, and social organization to estimate paternity. Specifically, for each offspring we evaluated combined support for paternity among candidate males from (1) genetic assignments from Cervus 3.03; (2) whether a candidate male was observed copulating with the mother; and (3) whether a candidate male lived in the mother's home territory (i.e., the resident male) or in an adjacent territory. For these assessments from combined support, we evaluated the genetic evidence in two ways: (a) from the maximum likelihood of paternity (LOP) with an 80% probability threshold, and (b) from the non-exclusion of candidate males having zero allelic mismatches with the offspring being examined. Regarding non-exclusion, if multiple candidate males had zero mismatches with an offspring, we assigned paternity to the male observed copulating with the mother; this latter procedure yielded assignments for 482 offspring (68%).
Results
Evaluation of the WTPD Mating System From Social Organization
The majority of the clans at our study-colony in June contained a single resident male along with ≥1 female that all lived in the same territory (Figures 4, 5); these one-male clans suggested behavioral monandry. Forty-two percent of clans shared a male who spent time with two groups of females, so we called each of these groups a “half-male” clan, which also suggested behavioral monandry (see also Hoogland, 1995). Three clans contained two resident males, which suggested behavioral polyandry. Twenty (16%) of the 125 clans contained zero males; 4 of these zero-male clans resulted when a male died or dispersed to an unknown location after the mating season, and the other 16 resulted when a resident male abandoned a territory with females after the mating season and spent all his time in an adjacent territory.
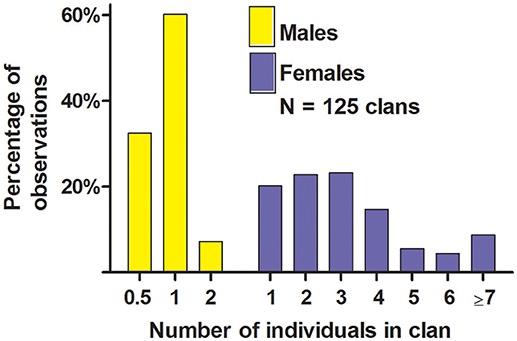
Figure 4. Number of sexually mature males and sexually mature females in clans of white-tailed prairie dogs at the Arapaho National Wildlife Refuge, Colorado USA, in June of each year from 2006 through 2012. These data are only from clans with ≥1 female (i.e., we have not included solitary males). For males, “0.5” refers to a territory (and group of females) in which a male spent some of his time; he spent the rest of his time in a different territory with a different group of females; we call each group of females in this scenario a “half-male” clan. One or two sexually immature yearling males sometimes live within clans, but are not shown here. Not every clan produced ≥1 weaned litter each spring.
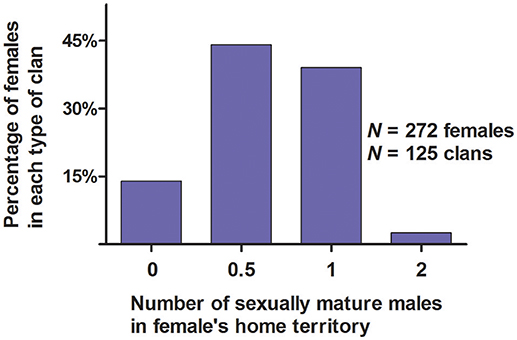
Figure 5. Percentage of females in the different types of clans of white-tailed prairie dogs at the Arapaho National Wildlife Refuge, Colorado USA, in June of each year from 2006 through 2012. These data are only from clans with ≥1 female (i.e., we have not included solitary males). “0” on the X-axis is for territories that did not have a resident sexually mature male. “0.5” on the X-axis is for “half-male” territories in which a male spent some of his time; he spent the rest of his time in a different territory with a different group of females.
Evaluation of the WTPD Mating System From Observations of Copulations
The WTPD mating season—i.e., the span over which copulations occurred—usually began in late March or early April, and lasted for about 3 weeks each year (Figure 6). From behavioral observations, we determined that each female was sexually receptive for only several hours on a single day of the mating season; we have no additional information about estrus from either cytology or hormone levels. Four lines of evidence indicate that our detection of a female's estrus, and the limitation of that estrus and sexual receptivity to a single day, were accurate. First, as noted above, the date of parturition (identified by a precipitous loss of maternal body mass over a 24-h period; Hoogland, 1995, 1998a) varied closely and positively with the date of putative estrus and copulations. Second, in those 83 cases (44% of all estrous females) when a female copulated aboveground rather than underground, all the aboveground copulations occurred on a single day. Third, the diagnostic behaviors associated with both underground and aboveground copulations (mating calls by the male, self- licking of genitals by the copulating male and the estrous female, and late final submergence at the end of the day by the estrous female) occurred on only a single day during the mating season for each female. Fourth, the inordinate amount of attention from males (anal sniffs, chases, and fights) generated by females presumed to be in estrus always lasted for only a single day during the mating season (see also Hoogland, 1995, 1998b).
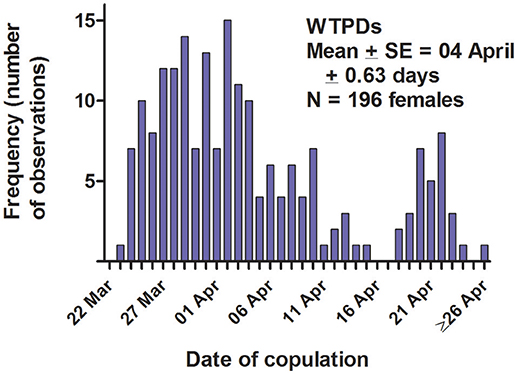
Figure 6. Date of copulation for 196 female white-tailed prairie dogs at the Arapaho National Wildlife Refuge, Colorado USA, from 2006 through 2012. Each female was sexually receptive for only a single day of the mating season each year. Within a year, the mating season (first copulation through last copulation) usually lasted for about 3 weeks.
Seventy percent of females copulated with a single male during the single period of sexual receptivity, and the other 30% copulated with 2, 3, 4, or 5 males (Figure 7). Eighteen percent of males copulated with a single female, and the other 82% copulated with 2–8 females (Figure 7). These data indicate that most females were behaviorally monandrous and most males were behaviorally polygynous.
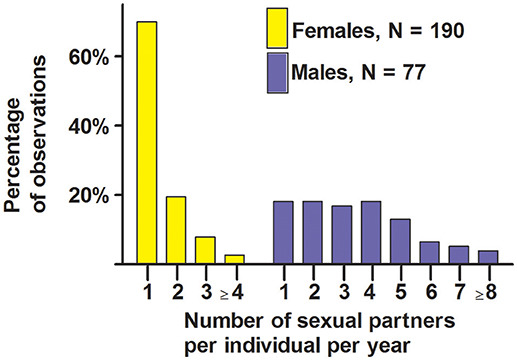
Figure 7. Number of sexual partners per year for male and female white-tailed prairie dogs at the Arapaho National Wildlife Refuge, Colorado USA, from 2006 through 2012. These data are from behavioral observations of copulations involving marked individuals.
Females copulated (a) exclusively with the resident adult male(s) of the home territory, or (b) with the male(s) of the home territory and with ≥1 male from outside territories, or (c) exclusively with ≥1 male from outside territories (Figure 8).
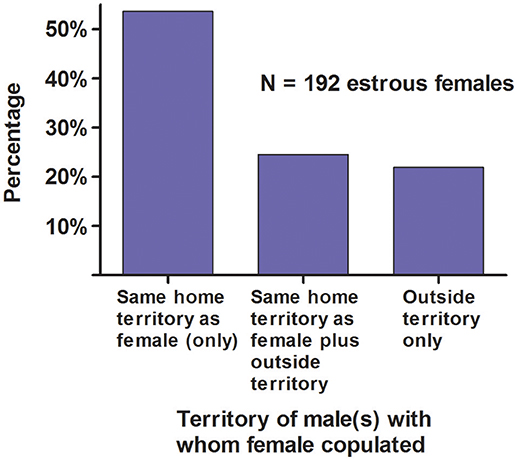
Figure 8. Territory of the male(s) with whom a female copulated for white-tailed prairie dogs at the Arapaho National Wildlife Refuge, Colorado USA, from 2006 through 2012.
Fifty-seven WTPD females (30%) were polyandrous (Figure 7), and their copulations with a 2nd, 3rd, or 4th male (N = 87 total copulations with later males) occurred under three circumstances: (a) when the home territory contained 2 males and the female copulated with both of those males (1%), (b) when the estrous female visited a male in an adjacent territory and copulated with him, with or without copulating with the resident male of the home territory (18%), or (c) when a male from an adjacent territory visited the estrous female's home territory and she copulated with him, with or without copulating with the resident male (81%).
Our longterm research allowed us to track many of the same eartagged, marked WTPDs regarding their tendency to copulate with ≥2 partners. For 48 WTPD females for which we observed copulations in ≥2 years, 48% were behaviorally monandrous every year, 6% were behaviorally polyandrous every year, and 46% were behaviorally monandrous in some years and behaviorally polyandrous in other years. Of the 19 WTPD males for which we observed copulations in ≥2 years, 63% were behaviorally polygynous every year, and 37% were behaviorally monogynous in 1 year and behaviorally polyandrous in other years. No male for which we had ≥2 years of information on copulations was behaviorally monandrous for more than 1 year.
Evaluation of the WTPD Mating System From Genetic Assignments of Paternity
Litter size at first emergence of almost-weaned WTPD juveniles from the natal burrow in late May or early June ranged from 1 through 8, with a mean ± SE of 5.16 ± 0.11 (N = 167 litters). From 2006 through 2012 we collected tissue samples (blood or ear) from the mother, all potential sires, and all juveniles from 156 of the 167 litters, for which we identified sexual partners for the mother of 104 litters.
We genotyped 801 individuals at 7 polymorphic microsatellite loci. We observed low allelic diversity at these loci (mean number of alleles per locus = 3.57; mean expected heterozygosity per locus = 0.325). Together, these loci produced a combined non-exclusion probability for parent-pair analysis of 0.075, indicating low power for determining paternity based on genetic assignments alone. Of 724 offspring weaned during the study period, 702 (97%) yielded genotype data at ≥4 loci. Because of the low non-exclusion probability, only 133 of 701 (19%) offspring could be assigned likelihood of paternity (LOP) with strict 95% confidence (LOD-score ≥ 3.51). However, a much larger proportion (506/701 = 73%) of offspring were assigned LOP when the confidence threshold was relaxed to 80% (LOD-score ≥ 1.60).
Combining Behavioral and Genetic Information for Assessment of Paternity and Mating System
An analysis of non-exclusion probabilities showed that an average of 27% of litters each year showed genetic evidence of multiple paternity—i.e., only about one quarter of females were genetically polyandrous (Figure 9).
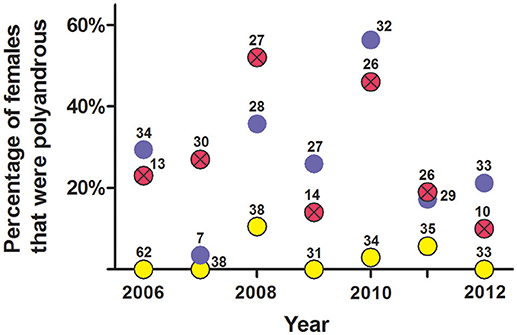
Figure 9. Estimate of the percentage of females per year that were polyandrous from (a) social organization, (b) observations of copulations, and (c) probability of genetic non-exclusion analyses from seven polymorphic microsatellites. These data are from female white-tailed prairie dogs at the Arapaho National Wildlife Refuge, Colorado USA, from 2006 through 2012. Yellow circles are from composition of clans (mean ± SE estimate of females that were polyandrous = 2.7% ± 1.5%); blue circles are from observations of copulations (mean ± SE = 27% ± 6.5%); red circles with Xs are from probability of genetic non-exclusion (mean ± SE = 27% ± 6.0%). The number above each dot indicates the number of females for which we had information for each method.
An analysis of non-exclusion probabilities showed that rates of genetic polygyny were high, with an average of 46% of males siring offspring from ≥2 litters each year (Figure 10).
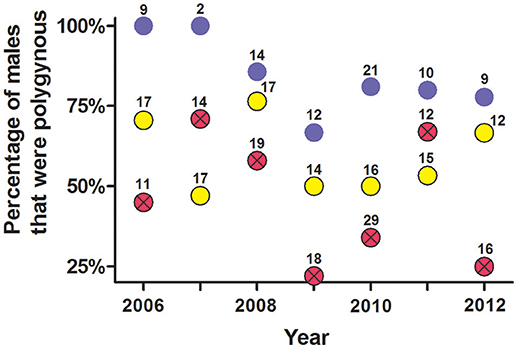
Figure 10. Estimate of the percentage of males per year that were polygynous from (a) social organization, (b) observations of copulations, and (c) probability of genetic non-exclusion analyses from seven polymorphic microsatellites. These data are from male white-tailed prairie dogs at the Arapaho National Wildlife Refuge, Colorado USA, from 2006 through 2012. Yellow circles are from composition of clans (mean ± SE estimate of males that were polygynous = 59% ± 4.5%); blue circles are from observations of copulations (mean = 84% ± 4.6%); red circles with Xs are from probability of genetic non-exclusion (mean = 46% ± 7.5%). The number above each dot indicates the number of males for which we had information for each method.
Behavioral and genetic evidence for polyandry varied considerably within and across years: Some years indicated low rates of multiple paternity vs. the frequency of observed behavioral polyandry (2006, 2009, 2010, and 2012), while other years indicated higher rates of multiple paternity vs. the frequency of observed behavioral polyandry (2007, 2008, 2011) (Figure 9). The frequency of behavioral polyandry estimated from social organization—with the assumption that females living in one-male, half-male, and zero-male clans were behaviorally monandrous, and females living in clans with ≥2 males were behaviorally polyandrous—was consistently low across years, with an overall mean of only 3% (Figure 9).
When a female was behaviorally polyandrous, multiple paternity occurred in 76% of the litters (LOP) or 64% of litters (non-exclusion) (Table 2). For the remaining litters, females were behaviorally polyandrous but with single paternity of the resulting litters. In these latter cases, the 1st-copulating male was more than twice as likely as 2nd and later-copulating males to have the highest LOP for every juvenile in the litter (Figure 11). For 86 of the 135 juveniles (64%) weaned by behaviorally polyandrous females, the 1st-copulating male had a higher LOP than 2nd- and later- copulating males. By contrast, when a female was behaviorally monandrous, her litter showed single paternity at a frequency of 80% (99/123 litters) (Table 2). Rates of genetic polygyny estimated from non-exclusion and rates of behavioral polygyny from social organization were always lower than rates of behavioral polygyny from observations of copulations, which indicated that a mean of 84% of males copulated with ≥2 females (Figure 10).
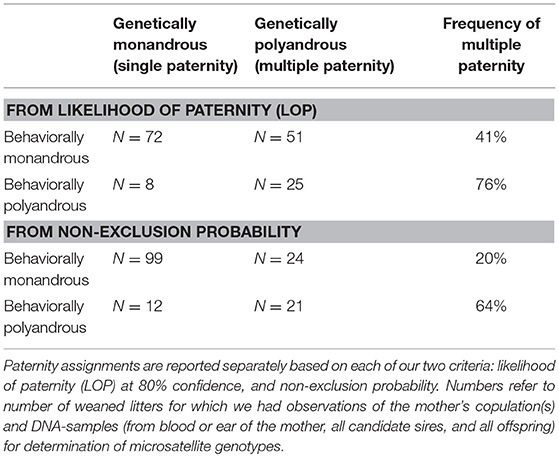
Table 2. Comparison of the number of sexual partners inferred for each white-tailed prairie dog female and her litter from observations of copulations and genetic paternity.
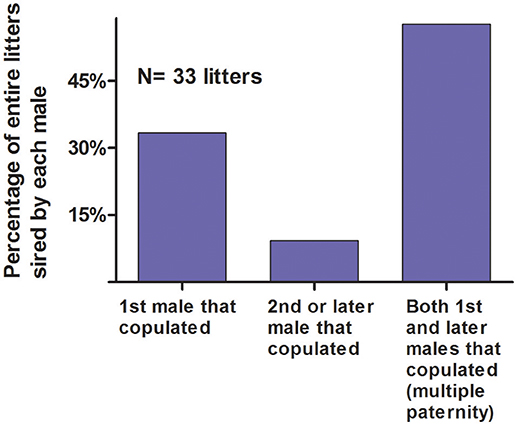
Figure 11. Probability of siring an entire litter vs. order of copulation with the mother. These data are from likelihood of paternity analyses (LOP) from seven polymorphic microsatellites for male white-tailed prairie dogs at the Arapaho National Wildlife Refuge, Colorado USA, from 2006 through 2012. Of the 135 juveniles weaned by polyandrous females, the 1st-copulating had a higher LOP than 2nd- and later- copulating males for 86 (64%).
Variation in the number of sexual partners per female across years depicted in Figure 9 was significant (P = 0.002, Kruskal–Wallis ANOVA).
For each offspring weaned, we determined combined genetic and behavioral support for paternity for three different (often overlapping) criteria for candidate males: (1) whether the male lived in the mother's home territory, (2) whether the male was observed to copulate with the mother, and (3) whether the male could be assigned paternity from genetics. Out of the 718 juveniles tested, 489 (68%) showed overlap among 2 or more criteria for paternity, but only 151 (21%) of juveniles had support across all three criteria, indicating they were sired by a male who lived in the mother's home territory and who copulated with the mother (Figure 12). At a confidence level of 80%, we were able to assign likelihood of paternity (LOP) to 506 of the 718 juveniles, of which 244 (48%) were estimated to be sired by males that were also observed copulating with the mother when she was in estrus, while 342 (68%) were sired by males that either lived in the mother's home territory or who were observed copulating with the mother (Figure 12). When the genetic assignment of paternity was evaluated on the principle of non-exclusion (i.e., no allelic mismatches between a juvenile and a candidate male), overlap with observations of copulations increased (Figure 13). Of the 718 juveniles, 707 could be assigned paternity based on non-exclusion; for 488 of these juveniles (69%), the male assigned paternity was also observed to copulate with the mother, while 629 (89%) of assigned fathers were either observed copulating with the mother or lived in the mother's home territory. In a minority of cases, paternity was assigned to a male that was not observed to copulate with the mother and did not live in the mother's home territory (LOP criteria: 164/718 = 23%; non-exclusion criteria: 78/718 = 11%).
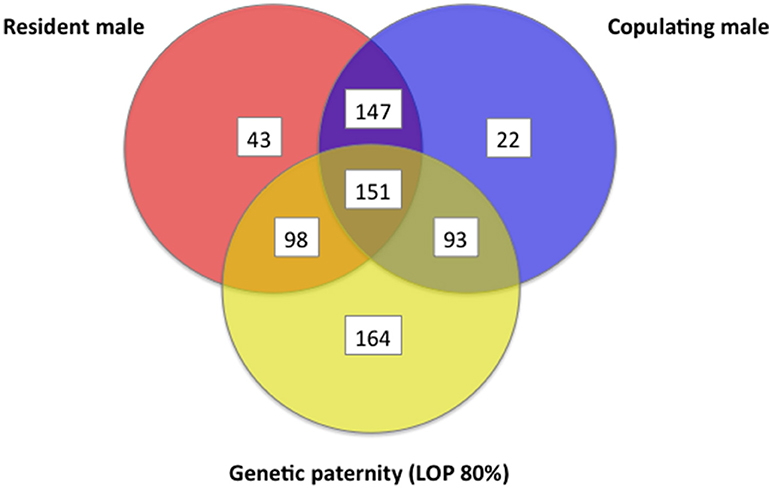
Figure 12. Venn diagram that shows information about a female's sexual partner(s) regarding (a) whether the male was a resident of the female's home territory, (b) whether the male was observed to copulate, and (c) whether the male had the highest likelihood of paternity (LOP), as determined by seven polymorphic microsatellites. These data are from white-tailed prairie dogs at the Arapaho National Wildlife Refuge, Colorado USA, from 2006 through 2012.
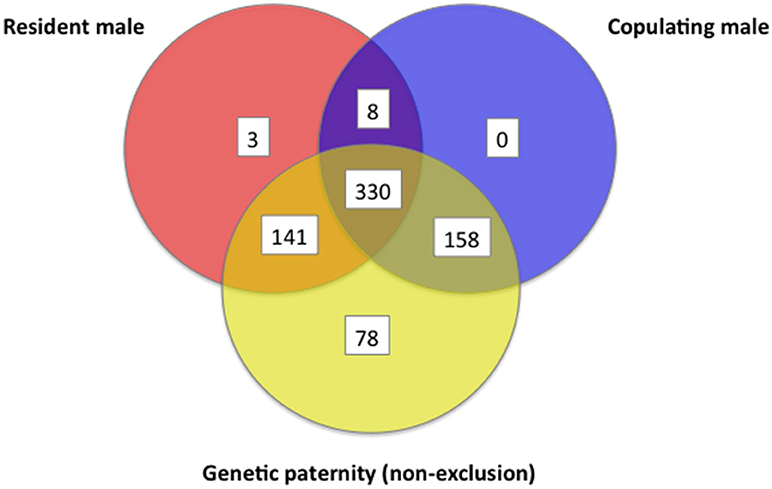
Figure 13. Venn diagram that shows information about a female's sexual partner(s) regarding (a) whether the male was a resident of the female's home territory, (b) whether the male was observed to copulate, and (c) whether one of the copulating males could be assigned paternity by non-exclusion, as determined by seven polymorphic microsatellites. These data are from white-tailed prairie dogs at the Arapaho National Wildlife Refuge, Colorado USA, from 2006 through 2012.
When we observed a WTPD female copulate with a single male and later wean a litter, the copulating male had the highest LOP for 66% (130/198) of offspring assigned paternity. This increased to 92% (274/297) when we assigned paternity based on non-exclusion. When we observed a WTPD female copulate with ≥2 males and later wean a litter, one of the copulating males had the highest LOP for 68% of the offspring (68/100); this increased to 95% of offspring for paternities based on non-exclusion (140/148).
Discussion
Studies of mating systems offer intriguing examples of how social organization and copulations are often related to, but are not necessarily fully compatible with, the effective pattern of parentage revealed by genetics (Westneat et al., 1990; Birkhead and Moller, 1992; Solomon and Keane, 2007; Lukas and Clutton-Brock, 2013; Klug, 2018). Because each female remains in or near (within 20 m) her home territory and is sexually receptive for only a single day each year during a short mating season, WTPDs are exemplary animals for quantifying frequencies of behavioral polyandry and behavioral polygyny. In theory, careful watching of a WTPD female on her single day of estrus should reveal all males with whom she might have copulated. Further, live-trapping of all WTPDs within a colony to obtain tissue-samples is practical, so that estimates of genetic polyandry and genetic polygyny are also feasible. Our long-term research allowed us to compare estimates of polyandry and polygyny from social organization, observations of estrus and copulations, and assignments of paternity from genetics. Our combination of these different approaches offers unique and complementary insights into the mating system of WTPDs living under natural conditions. Our results show that the highly structured WTPD social organization (with multiple females commonly living in the same territory with a single male) influences the number and identity of copulatory partners. However, the frequencies of behavioral polyandry and behavioral polygyny are not strictly translatable to the frequencies of genetic polyandry (multiple paternity) and genetic polygyny, because some multiply mated females produce litters of single-paternity, and others produce no litters at all. Further, females observed to copulate with only a single male sometimes produced litters with multiple paternity, indicating the occurrence of both cryptic, undetected copulations, and sperm competition.
Our research indicates that 83% (226/272) of WTPD females appeared to have only one “social mate” because they lived in a discrete clan with only one male, or shared a male with females living in an adjacent clan. Similar designation of social mates is possible for some species, but not for many other species for which discrete social groupings are not obvious (Birkhead and Moller, 1992; Shuster and Wade, 2003; Danchin et al., 2008; Oliveria et al., 2008; Raveh et al., 2011; Clutton-Brock, 2016). Compositions of clans also suggested that 3% of females had ≥2 social mates, and that 14% of females had no social mate, because they lived in a clan with no male (Figure 5). For males, compositions of clans suggested that 41% had only one social mate, because they lived in a clan with only one female; compositions suggested that the other 59% of males had ≥2 social mates, because they lived in a clan with ≥2 females (Figure 10).
When compared to the frequencies estimated from social organization (i.e., compositions of clans), observations of copulations indicated a higher frequency for both behavioral polyandry and behavioral polygyny. These observations show that 27% of all females (N = 190 for which we were able to document estrus and all putative sexual partners per year) copulated with ≥2 males during the mating season, much higher than the 3% for which social organization (i.e., clans with ≥2 males) indicated polyandry (Figure 9). Our observations of copulations also showed that 84% of males (N = 77 for which we could record all putative sexual partners per year) copulated with ≥2 females during the mating season, substantially higher than the 59% inferred from social organization alone (Figure 10).
Even though each WTPD female's period of sexual receptivity was limited to several hours on a single day each year, 27% of females copulated with two or more males. Polyandrous WTPD females produced more offspring that lived for at least 9 months after weaning (our best estimate of female reproductive success) than monandrous females, and this advantage resulted mainly because polyandrous females were more likely to conceive and get pregnant (Hoogland, 2013b). With this clear advantage to fitness, why were not more females polyandrous? In proximate terms, mate-guarding by the first male to copulate with a female sometimes precluded copulations by additional males. The first-copulating male chased away other males, and tried to sequester the female in a burrow so that she was unable to search for additional males (Figures 2, 3). In ultimate terms, polyandrous WTPD females were less likely than monandrous females to survive until the next mating season (Hoogland, 2013b). This scenario indicates a life history tradeoff between current-year and future reproduction.
With an understanding that other factors are also important (Trivers, 1972; Emlen and Oring, 1977; Kleiman, 1977; Smith, 1984; Clutton-Brock, 2016), we predicted that frequencies of polyandry and polygyny would be lower for WTPDs than for many other species of animals with longer periods of sexual receptivity. Support for this prediction is minimal. Our estimated frequencies for behavioral polyandry (27%) and behavioral polygyny (84%) are similar to estimates of the frequency of polyandry and polygyny for animals for which females have longer periods of sexual receptivity that sometimes last for several days (Westneat et al., 1990; Birkhead and Moller, 1992, 1998; Davies, 1992; Alatalo et al., 1996; Dugdale et al., 2007; Raveh et al., 2011; Gowaty, 2012). These WTPD frequencies are also similar to estimates of polyandry and polygyny for other species of ground-dwelling squirrels ((Hanken and Sherman, 1981; Lacey et al., 1997; Goossens et al., 1998; Hoogland, 2007, 2013b; Raveh et al., 2010); Raveh et al., 2011).
Because of the diagnostic aboveground behaviors we observed just before and just after a WTPD underground consortship (anal sniffing of the female by males, mating calls by the male, self-licking of the genitals by both sexes, and late final submergence at the end of the day by the female), we are confident that we usually were able to determine which male(s) consorted with a female on the single day when she was sexually receptive. Fifty-six percent of females copulated exclusively underground, however, so we could not be 100% certain that every underground consortship involved a copulation. Further, some females were especially secretive with their estrus and copulations, so that we could not accurately record their sexual partners. Consequently, litters showing multiple paternity sometimes resulted for mothers that appeared to be behaviorally monandrous. By contrast, however, litters that showed single paternity almost always resulted from females that were behaviorally monandrous. Thus, while genetic paternity assignments usually agreed with expectations from social organization and copulations (Figures 12, 13), overall about 11% of juveniles (78/718) were assigned paternity to a male outside a mother's home territory and for which we observed no copulations with the mother.
What does it mean when a WTPD male from an adjacent territory had the highest LOP for a juvenile but evidently had no copulations with that juvenile's mother on her day of estrus? At least three explanations are possible. The first is that the copulating male and the female had an underground consortship that was exceedingly subtle, so that we did not detect it—even though we were watching every day from dawn to dusk during the entire mating season, we focused on males and unmated females, and we especially concentrated on females that showed one or more signs of estrus. Support for this first explanation is our finding that some WTPD females were more covert than others with estrus and copulations. Some estrous females, for example, had only 1 underground consortship, elicited no mating calls, and had <5 interactions with only 1 male; documenting copulations for these secretive females was challenging. Estrous females at the other end of the spectrum, by contrast, had as many as 21 underground consortships with as many as 5 males, elicited as many as 26 mating calls, and had as many as 84 interactions with several males; documenting copulations for these conspicuous females was much easier.
The second possible explanation for differences between behavioral and genetic data concerns statistical errors (i.e., false positives or false negatives) in our assignments of paternity. When amplifying and scoring microsatellite loci, we included a large number of sample duplicates (N = 27 individuals with duplicate tissue samples) that confirmed we had a low genotyping error rate (0.5% of scored genotypes), and Cervus 3.03 considered this error rate during calculations of LOPs. We are therefore confident that scoring errors were highly unlikely to lead to false positives in assignments of paternity.
A third possible explanation for the discrepancy between behavioral and genetic data is the low variability across microsatellite loci at our study colony. Despite extensive initial screening efforts, we were able to find only seven microsatellite loci that were polymorphic, and the combined non-exclusion probability across all seven loci was relatively low at 0.075. Greater resolution for assigning genetic paternity might be possible in the future via development of a large number of diagnostic genome-wide markers such as single nucleotide polymorphisms (SNPs). Other studies have shown the utility of SNPs for genetic paternity assignment in recently bottlenecked or kin-structured populations (e.g., Tokarska et al., 2009; Weinman et al., 2015).
The low frequency of microsatellite polymorphisms we observed has been noted previously (Sackett et al., 2009), and likely reflects a biological characteristic of WTPDs in general. Perhaps this low frequency results from a historically low or bottlenecked effective population size that might have occurred at some point (Seglund et al., 2006). Nevertheless, the low levels of microsatellite variation at our study-colony limited our statistical power to assign paternity with a high confidence threshold (95%), and prompted us to use a less stringent confidence threshold (80%) when assigning paternity based on LOP. Thus, even though 164/718 (23%; see Figure 12) of juveniles were assigned paternity from LOP to a male from an adjacent territory when that male was never observed to consort underground or copulate aboveground with the mother when she was in estrus, this frequency is about what we would expect by chance alone at an 80% confidence threshold. The frequency of paternity assigned to males who were never observed to copulate drops to 11% when we instead consider the criteria based on non-exclusion (Figure 13). In these cases, when male-A copulated with female-B and could not be excluded from paternity, we think that he is a more likely sire than a male with a higher LOP (based on 80% threshold) who was not observed to copulate with the mother. We consider 11% to be our most accurate estimate of the true rate of cryptic genetic paternity at our WTPD study-colony over our 7 years of research there.
We predicted that our three approaches would yield similar estimates of polyandry and polygyny, but our results provide only minimal support for this prediction. Our results over seven consecutive years from both copulations and genetic assignments of paternity indicate an average rate of 27% for both behavioral and genetic polyandry (Figure 9). However, the year-to-year variability in these estimates was substantial (Figure 9). Further, annual variation in our estimates of behavioral and genetic polygyny was also large (Figure 10). A single year of observations of copulations and genetic assignments of paternity would have given a misleading impression of the overall frequencies of behavioral and genetic estimates of polyandry and polygyny for WTPDs. Several other studies have documented large annual variation in the frequencies of behavioral and genetic polyandry and polygyny as reported here for WTPDs (e.g., Schwagmeyer and Brown, 1983; Birkhead and Moller, 1992, 1998; Hare et al., 2004; Isvaran and Clutton-Brock, 2006; Bergeron et al., 2011; Wells et al., 2017). Possible reasons for so much annual variation include yearly differences in factors such as weather (Bergeron et al., 2011; Martin et al., 2014), density of females (Emlen and Oring, 1977; Wells et al., 2017), female age and female body mass (Cotton et al., 2006; Jones et al., 2012; Hoogland, 2013b), reproductive synchrony among females (Emlen and Oring, 1977; Hoogland, 2007), and the operational sex ratio (i.e., ratio of the number of sexually mature females ready to copulate to the number of sexually mature males ready to copulate; Emlen and Oring, 1977; Michener and McLean, 1996). With so many variables and only 7 years of information, we made no attempt to make a quantitative dissection of the underlying causes of the extreme annual variation in the mating system of the WTPDs at our study-colony.
For 86 of the 135 juveniles (64%) weaned by behaviorally polyandrous females, the 1st-copulating male had a higher LOP than 2nd- and later- copulating males (for similar results, see Hanken and Sherman, 1981; Schwagmeyer and Foltz, 1990; Hoogland, 1995; Lacey et al., 1997; Raveh et al., 2010). By contrast, when a female was behaviorally monandrous, she showed single paternity of her litter at a frequency of 80% (99/123 litters) (Table 2). Rates of genetic polygyny estimated from non-exclusion and rates of behavioral polygyny from social organization were always lower than rates of behavioral polygyny from observations of copulations, which indicated that a mean of 84% of males copulated with ≥2 females (Figure 10).
Previous studies documenting both behavioral and genetic polyandry and polygyny for a diverse array of other animals typically have involved smaller sample sizes over a shorter time span (Hanken and Sherman, 1981; Burke et al., 1989; Moore and Ball, 2002; Solomon et al., 2004). Some previous researchers have used the term “polygynandry” to describe a species in which behavioral and genetic estimates of polyandry and polygyny are both high (Davies, 1992; Baker et al., 2004; Dugdale et al., 2007; Munroe and Koprowski, 2011; Raveh et al., 2011; Schwanz et al., 2016). Perhaps polygynandry is also a good term to describe WTPDs, for which females commonly are both monandrous and polyandrous (behaviorally and genetically) and males commonly are both monogynous and polygynous (behaviorally and genetically).
Male and female WTPDs appear to have a conflict of interest regarding the optimal number of sexual partners per year for each female. Because polyandry yields more offspring that live for at least 9 months after weaning, a female maximizes annual reproductive success by mating with more than one male. A male, by contrast, maximizes annual reproductive success by monopolizing, and then being the only male to copulate with, as many females as possible (Figures 2, 3). Neither sex is completely “winning” this intersexual conflict of interest regarding the optimal number of sexual partners for females (Figure 9), and the opposing sexual selection pressures on males and females evidently have produced the polygynandry that we observed.
The overall frequencies of polyandry from observations of copulations vs. polyandry from genetics were identical (27%; Figure 9). This suggests that cryptic (post-copulatory) female choice is minimal, but pre-copulatory female choice might still be possible. Consistent with this latter notion, we observed that a WTPD female commonly did not copulate with all the available males that resided in, or temporarily visited, her home territory on her single day of estrus (J. L. Hoogland, personal observation). These insights emphasize the benefits and insights that are possible from combining both behavioral and genetic data measured at different points in the processes of copulation through the weaning of offspring.
Each of our three methods for documenting polyandry and polygyny among WTPDs has advantages and disadvantages (see also Hanken and Sherman, 1981; Gowaty, 1985, 2012; Westneat et al., 1990; Hunter et al., 1992; Kempenaers et al., 1995; DeWoody et al., 2000; Raveh et al., 2010; Mooring and Penedo, 2014). Estimating behavioral polyandry and behavioral polygyny from social organization requires neither observations during the mating season nor collection and analysis of DNA samples, for example, but this approach is only tangentially related to male and female annual reproductive success. Indeed, a study of social organization alone is the only approach that does not provide unequivocal evidence for either polyandry (behavioral or genetic) or polygyny (behavioral or genetic). Observations of copulations offer the best way to document behavioral polyandry and behavioral polygyny. Behavioral polyandry does not always produce multiple paternity, however, and the observation of copulation(s) with a single male does not necessarily rule out cryptic behavioral polyandry via undetected copulations with other males. Further, direct observations during the mating season require an inordinate number of person-hours of watching to generate large sample sizes. Estimates of paternity from genetics provide a powerful complement in these cases, and sometimes can provide the ultimate, unequivocal documentation of which, and how many, juveniles a male actually sires (Burke et al., 1989; Moore and Ball, 2002; Solomon et al., 2004; Weinman et al., 2015). Genetic analyses of paternity sometimes have little statistical power when genetic variation is low (as occurred at our study-colony), however. The choice of method(s) for estimating the frequencies of behavioral and genetic polyandry and polygyny will depend on the biology of the study-animal (e.g., degree of coloniality, ease of livetrapping, visibility of copulations, length of the mating season, and genetic variation) and research objectives. The combination of behavioral and genetic methods not only capitalizes on the contrasting strengths and weaknesses of each approach, but also reveals information about dynamic mating systems that would not be possible from using only one method. Information from WTPD social organization, for example, helped us to know which males were likely to copulate with an estrous female, and information from both social organization and copulations helped us to know which males to consider as possible candidate sires for each offspring. Our research was an extension of previous research on other species of ground-dwelling squirrels (e.g., Hanken and Sherman, 1981; Lacey et al., 1997; Goossens et al., 1998; Raveh et al., 2011; Wells et al., 2017), and should be informative for other social species as well. Our results underscore the valuable insights that can result from integrating diverse perspectives on the mating system gathered from long-term behavioral and genetic studies of natural populations.
Data Availability
Behavioral and genetic data analyzed in this study are in the tables and figures or have been deposited into Dryad (doi: 10.5061/dryad.jn365c2).
Author Contributions
With help from research assistants, JH was responsible for collecting all the information on social organization and copulations. SK and RT were responsible for all the genetic analyses of paternity. JH and SK wrote the manuscript.
Conflict of Interest Statement
The authors declare that the research was conducted in the absence of any commercial or financial relationships that could be construed as a potential conflict of interest.
Acknowledgments
For financial assistance, we thank Colorado Parks and Wildlife, the Denver Zoological Foundation, and the National Science Foundation. We thank 35 assistants for help in the field, especially M. Betzhold, J. Bowser, K. Eddy, S. Hale, A. Kirk, J. Stephens, Y. Sui and E. van Manen. For help with the manuscript, we thank M. Griesser, K. Tsuji, and C. Wells. We are grateful to D. Boesch, E. Davidson, R. Gardner, R. Morgan, and P. Goodwin of the University of Maryland Center for Environmental Science for facilitating our long-term research with prairie dogs.
References
Alatalo, R. V., Burke, T., Dann, J., Hanotte, O., Hoglund, J., Lundberg, A., et al. (1996). Paternity, copulation disturbance and female choice in lekking black grouse. Anim. Behav. 52, 861–873. doi: 10.1006/anbe.1996.0234
Alexander, R. D., Hoogland, J. L., Howard, R. D., Noonan, K. M., and Sherman, P. W. (1979). “Sexual dimorphisms and breeding systems in pinnipeds, ungulates, primates, and humans,” in Evolutionary Biology and Human Social Behavior, eds N. A. Chagnon and W. Irons (New York, NY: Duxbury Press), 402–435.
Baker, P. J., Funk, S. M., Bruford, M. W., and Harris, S. (2004). Polygynandry in a red fox population: implications for the evolution of group living in canids? Behav. Ecol. 15, 766–778. doi: 10.1093/beheco/arh077
Bartholomew, G. A. (1970). A model for the evolution of pinniped polygyny. Evolution 24, 546–559. doi: 10.1111/j.1558-5646.1970.tb01790.x
Beach, F. A. (1976). Sexual attractivity, proceptivity, and receptivity in female mammals. Hormones Behav. 7, 105–138. doi: 10.1016/0018-506X(76)90008-8
Berger, J., and Cunningham, C. (1991). Bellows, copulations, and sexual selection in bison (Bison bison). Behav. Ecol. 2, 1–6. doi: 10.1093/beheco/2.1.1
Berger, J., and Cunningham, C. (1994). Bison: Mating and Conservation in Small Populations. New York, NY: Columbia University Press.
Bergeron, P., Reale, D., Humphries, M. M., and Garant, D. (2011). Evidence of multiple paternity and mate selection for inbreeding avoidance in wild eastern chipmunks. J. Evol. Biol. 24:1685–1694. doi: 10.1111/j.1420-9101.2011.02294.x
Bernatchez, L., and Duchesne, P. (2000). Individual-based genotype analysis in studies of parentage and population assignment: how many loci, how many alleles? Can. J. Fish Aquat. Sci. 57, 1–12. doi: 10.1139/f99-271
Birkhead, T. R., and Moller, A. P. (1992). Sperm Competition in Birds: Evolutionary Causes and Consequences. New York, NY: Academic Press.
Birkhead, T. R., and Moller, A. P. (eds.). (1998). Sperm Competition and Sexual Selection. London: Academic Press.
Black, J. M. (eds.). (1996). Partnerships in Birds: The Study of Monogamy. New York, NY: Oxford University Press.
Blouin, M. S. (2003). DNA-based methods for pedigree reconstruction and kinship analysis in natural populations. Trends Ecol. Evol. 18, 503–511. doi: 10.1016/S0169-5347(03)00225-8
Bonin, A., Bellemain, E., Eidesen, P. B., Pompanon, F., Brochmann, C., and Taberlet, P. (2004). How to track and assess genotyping errors in population genetics studies. Mol. Ecol. 13, 3261–3273. doi: 10.1111/j.1365-294X.2004.02346.x
Bretman, A., and Tregenza, T. (2005). Measuring polyandry in wild populations: a case study using promiscuous crickets. Mol. Ecol. 14, 2169–2179. doi: 10.1111/j.1365-294X.2005.02556.x
Burke, T., Davies, N. B., Bruford, M. W., and Hatchwell, B. J. (1989). Parental care and mating behaviour of polyandrous dunnocks Prunella modularis related to paternity by DNA fingerprinting. Nature 338, 249–251. doi: 10.1038/338249a0
Byers, J. A. (1997). American Pronghorn: Social Adaptations and Ghosts of Predators Past. Chicago, IL: University of Chicago Press.
Clark, T. W. (1977). Ecology and ethology of the white-tailed prairie dog. Publ. Biol. Geol. 3, 1–97.
Clark, T. W., Hoffmann, R. S., and Nadler, C. F. (1971). Cynomys leucurus. Mamm. Spec. 7, 1–4. doi: 10.2307/3503914
Coltman, D. W. (2005). Testing marker-based estimates of heritability in the wild. Mol. Ecol. 14, 2593–2599. doi: 10.1111/j.1365-294X.2005.02600.x
Cotton, S., and Small, J., Pomiankowki, A. (2006). Sexual selection and condition-dependent mate preferences. Curr. Biol. 16:R755–R765. doi: 10.1016/j.cub.2006.08.022
Csilléry, K., Johnson, T., Beraldi, D., Clutton-Brock, T. H., Coltman, D., Hansson, B., et al. (2006). Performance of marker-based relatedness estimators in natural populations of outbred vertebrates. Genetics 173, 2091–2101. doi: 10.1534/genetics.106.057331
Danchin, E., Giraldeau, L. A., and Cezilly, F. C. (2008). Behavioural Ecology: An Evolutionary Perspective on Behavior. Oxford: Oxford University Press.
Davies, N. B. (1992). Dunnock Behaviour and Social Evolution. New York, NY: Oxford University Press.
Decker, M. D., Parker, P. G., Minchella, D. J., and Rabenold, K. N. (1993). Monogamy in black vultures: genetic evidence from DNA fingerprinting. Behav. Ecol. 4, 29–35. doi: 10.1093/beheco/4.1.29
DeWoody, J. A., Fletcher, D. E., Wilkins, S. D., Nelson, W. S., and Avise, J. C. (2000). Genetic monogamy and biparental care in an externally fertilizing fish, the largemouth bass (Micropterus salmoides). Proc. Roy. Soc. Lond. Ser. B Biol. Sci. 267, 2431–2437. doi: 10.1098/rspb.2000.1302
Dewsbury, D. A. (1975). Diversity and adaptation in rodent copulatory behavior. Science 190, 947–954. doi: 10.1126/science.1188377
Dewsbury, D. A. (1984). “Sperm competition in muroid rodents,” in Sperm Competition and the Evolution of Mating Systems, ed R. L. Smith (Orlando, FL: Academic Press), 547–571. doi: 10.1016/B978-0-12-652570-0.50023-3
Downhower, J. F., and Armitage, K. B. (1971). The yellow-bellied marmot and the evolution of polygamy. Am. Nat. 105, 355–370. doi: 10.1086/282730
Dugdale, H. L., Macdonald, D. W., Pope, L. C., and Burke, T. (2007). Polygynandry, extra-group paternity and multiple-paternity litters in European badger (Meles meles) social groups. Mol. Ecol. 16, 5294–5306. doi: 10.1111/j.1365-294X.2007.03571.x
Eberhard, W. G. (1996). Female Control: Sexual Selection by Cryptic Female Choice. Princeton, NJ: Princeton University Press.
Emlen, S. T., and Oring, L. W. (1977). Ecology, sexual selection, and the evolution of mating systems. Science 197, 215–223. doi: 10.1126/science.327542
Fernandez, J., and Toro, M. A. (2006). A new method to estimate relatedness from molecular markers. Mol. Ecol. 15, 1657–1667. doi: 10.1111/j.1365-294X.2006.02873.x
Firman, R. C., and Simmons, L. W. (2008). Polyandry facilitates postcopulatory inbreeding avoidance in house mice. Evolution 62, 603–611. doi: 10.1111/j.1558-5646.2007.00307.x
Getz, L. L., McGuire, B., Hofmann, J., Pizzuto, T., and Frase, B. (1990). “Social organization and mating system of the prairie vole,” in Social Systems and Population Cycles in Voles, eds R. H. Tamarin, R. S. Ostfeld, S. R. Pugh, and G. Bujalska (Basal: Birkhauser-Verlag), 69–80. doi: 10.1007/978-3-0348-6416-9_7
Gibson, R. M., Bradbury, J. W., and Vehrencamp, S. L. (1991). Mate choice in lekking sage grouse revisited: the roles of vocal display, female site fidelity, and copying. Behav. Ecol. 2, 165–180. doi: 10.1093/beheco/2.2.165
Gladstone, D. E. (1979). Promiscuity in monogamous colonial birds. Am. Nat.114, 545–557. doi: 10.1086/283501
Goossens, B., Graziani, L., Waits, L. P., Farand, E., Magnolon, S., Coulon, J., et al. (1998). Extra-pair paternity in the monogamous Alpine marmot revealed by nuclear DNA microsatellite analysis. Behav. Ecol. Sociobiol. 43, 281–288. doi: 10.1007/s002650050492
Gowaty, P. A. (1985). Multiple parentage and apparent monogamy in birds. Ornithol. Monogr. 37, 11–21.
Gowaty, P. A. (2012). The evolution of multiple mating. Costs and benefits of polyandry to females and of polygyny to males. Fly 6, 3–11. doi: 10.4161/fly.18330
Hanken, J., and Sherman, P. W. (1981). Multiple paternity in Belding's ground squirrel litters. Science 212, 351–353.
Hare, J. F., Todd, G., and Untereiner, W. A. (2004). Multiple mating results in multiple paternity in Richardson's ground squirrels, Spermophilus richardsoni. Can. Field Nat. 118, 90–94. doi: 10.22621/cfn.v118i1.888
Hoogland, J. L. (1981). The evolution of coloniality in white-tailed and black-tailed prairie dogs (Sciuridae: Cynomys leucurus and C. ludovicianus). Ecology 62, 252–272.
Hoogland, J. L. (1995). The Black-Tailed Prairie Dog: Social Life of a Burrowing Mammal. Chicago, IL: University of Chicago Press.
Hoogland, J. L. (1998a). Estrus and copulation among Gunnison's prairie dogs. J. Mammal. 79, 887–897.
Hoogland, J. L. (1998b). Why do female Gunnison's prairie dogs copulate with more than one male? Anim. Behav. 55, 351–359. doi: 10.1006/anbe.1997.0575
Hoogland, J. L. (1999). Philopatry, dispersal, and social organization of Gunnison's prairie dogs. J. Mammal. 80, 243–251. doi: 10.2307/1383224
Hoogland, J. L. (2001). Black-tailed, Gunnison's, and Utah prairie dogs all reproduce slowly. J. Mammal. 82, 917–927. doi: 10.1644/1545-1542(2001)082<0917:BTGSAU>2.0.CO;2
Hoogland, J. L. (2007).“Alarm calling, multiple mating, and infanticide among black-tailed Gunnison's, and Utah prairie dogs,” in Rodent Societies, eds J. O. Wolf and P. W. Sherman (Chicago, IL: University of Chicago Press), 438–449.
Hoogland, J. L. (2013a). Prairie dogs disperse when all close kin have disappeared. Science 339, 1205–1207. doi: 10.1126/science.1231689
Hoogland, J. L. (2013b). Why do females copulate with more than one male?—Insights from longterm research. J. Mammal. 94, 731–744. doi: 10.1644/12-MAMM-A-291.1
Hoogland, J. L., and Brown, C. R. (2016). Prairie dogs increase fitness by killing interspecific competitors. Proc. Soc. B 283, 1–7. doi: 10.1098/rspb.2016.0144
Hoogland, J. L., Cully, J. F., Rayor, L. S., and Fitzgerald, J. P. (2012). Conflicting research on the demography, ecology, and social behavior of Gunnison's prairie dogs (Cynomys gunnisoni). J. Mammal. 93, 1075–1085. doi: 10.1644/11-MAMM-A-034.3
Hunter, F. M., Burke, T., and Watts, S. E. (1992). Frequent copulation as a method of paternity assurance in the northern fulmar. Anim. Behav. 44, 149–156. doi: 10.1016/S0003-3472(05)80764-X
Isvaran, K., and Clutton-Brock, T. H. (2006). Ecological correlates of extra-group paternity in mammals. Proc. Biol. Sci. 74, 219–224. doi: 10.1098/rspb.2006.3723
Johnson, S. L., and Brockmann, H. J. (2010). Costs of multiple mates: an experimental study in horseshoe crabs. Anim. Behav. 80, 773–782. doi: 10.1016/j.anbehav.2010.07.019
Jones, P. H., Van Zant, J. L., and Dobson, F. S. (2012). Variation in reproductive success in male and female Columbian ground squirrels (Urocitellus columbianus). Can. J. Zool. 90, 736–743. doi: 10.1139/z2012-042
Kalinowski, S. T., Taper, M. L., and Marshal, T. C. (2007). Revising how the computer program CERVUS accommodates genotyping error increases success in paternity assignment. Mol. Ecol. 16, 1099–1106. doi: 10.1111/j.1365-294X.2007.03089.x
Kempenaers, B., Verheyen, G. R., and Dhondt, A. A. (1995). Mate guarding and copulation behaviour in monogamous and polygynous blue tits: do males follow a best-of-a-bad-job strategy? Behav. Ecol. Sociobiol. 36, 33–42. doi: 10.1007/BF00175726
King, J. A. (1955). Social behavior, social organization, and population dynamics in a black-tailed prairie-dog town in the Black Hills of South Dakota. Contrib. Lab. Vert. Biol. Univ. Mich. 67, 1–123.
Klug, H. (2018). Why monogamy? A review of potential ultimate drivers. Front. Ecol. Evol. 6:30. doi: 10.3389/fevo.2018.00030
Lacey, E., Wieczorek, J. R., and Tucker, P. K. (1997). Male mating behaviour and patterns of sperm precedence in Arctic ground squirrels. Anim. Behav. 53, 767–779. doi: 10.1006/anbe.1996.0342
Lukas, D., and Clutton-Brock, T. H. (2013). The evolution of social monogamy in mammals. Science 341, 526–530. doi: 10.1126/science.1238677
Martin, J. G. A., Petelle, M. M., and Blumstein, D. T. (2014). Environmental, social, morphological, and behavioral constraints on opportunistic multiple paternity. Behav. Ecol. Sociobiol. 68:1531–1538. doi: 10.1007/s00265-014-1762-3
Menkens, G. E., Miller, B. J., and Anderson, S. H. (1987). “White-tailed prairie dog ecology in Wyoming,” in Great Plains Wildlife Damage Control Workshop Proceedings (Lincoln, NE), 34–38.
Michener, G. R., and McLean, I. G. (1996). Reproductive behavior and operational sex ratio in Richardson's ground squirrels. Anim. Behav. 52, 743–758.
Mock, D. W., and Fujiok, M. (1990). Monogamy and long-term pair bonding in vertebrates. Trends Ecol. Evol. 5, 39–43. doi: 10.1016/0169-5347(90)90045-F
Moller, A. P., and Birkhead, T. R. (1989). Copulation behavior in mammals: evidence that sperm competition is widespread. Biol. J. Linn. Soc. 38, 119–131. doi: 10.1111/j.1095-8312.1989.tb01569.x
Moore, M. K., and Ball, R. M. (2002). Multiple paternity in loggerhead turtle (Caretta caretta) nests on Melbourne Beach, Florida: a microsatellite analysis. Mol. Ecol. 11, 281–288. doi: 10.1046/j.1365-294X.2002.01426.x
Mooring, M. S., and Penedo, M. C. T. (2014). Behavioral versus genetic measures of fitness in bison bulls (Bison bison). J. Mammal. 95, 913–924. doi: 10.1644/13-MAMM-A-209
Munroe, K., and Koprowski, J. (2011). Sociality, Bateman's gradients, and the polygynandrous genetic mating system of round-tailed ground squirrels (Xenospermophilus tereticaudus). Behav. Ecol. Sociobiol. 65, 1811–1824. doi: 10.1007/s00265-011-1189-z
Oliveria, R., Taborsky, M., and Brockmann, H. J. (2008). Alternative Reproductive Tactics: An Integrative Approach. Cambridge: Cambridge University Press.
Orians, G. H. (1969). On the evolution of mating systems in birds and mammals. Am. Nat. 103, 589–603. doi: 10.1086/282628
Parker, G. A. (1984). “Sperm competition and the evolution of animal mating systems,” in Sperm Competition and the Evolution of Mating Systems, ed R. L. Smith (Orlando, FL: Academic Press), 1–60.
Parker, G. A., and Birkhead, T. R. (2013). Polyandry: the history of a revolution. Philos. Trans. Roy. Soc. B Biol. Sci. 368:20120335. doi: 10.1098/rstb.2012.0335
Parker, G. H. (1970). Sperm competition and its evolutionary significance in insects. Biol. Rev. 45, 525–567. doi: 10.1111/j.1469-185X.1970.tb01176.x
Raveh, S., Heg, D., Dobson, F. S., Coltman, D. W., Gorrele, J. C., Balmer, A., et al. (2010). Mating order and reproductive success in male Columbian ground squirrels (Urocitellus columbianus). Behav. Ecol. 21, 537–547. doi: 10.1093/beheco/arq004
Raveh, S., Heg, D., Viblanc, V. A., Coltman, D. W., Gorrell, J. C., Dobson, F. S., et al. (2011). Male reproductive tactics to increase paternity in the polygynandrous Columbian ground squirrel (Urocitellus columbianus). Behav. Ecol. Sociobiol. 65, 695–706. doi: 10.1007/s00265-010-1071-4
Reeder, D. M. (2003). “The potential for cryptic female choice in primates: Behavioral, anatomical, and physiological considerations,” in Sexual Selection and Reproductive Competition in Primates: New Perspectives and Directions, ed C. B. Jones (Norman, OK: American Society of Primatologists), 255–303.
Reichard, U. H., and Boesch, C. (eds.). (2003). Monogamy: Mating Strategies and Partnerships in Birds, Humans and other Mammals. Cambridge: Cambridge University Press.
Ribble, D. O. (1991). The monogamous mating system of Peromyscus californicus as revealed by DNA fingerprinting. Behav. Ecol. Sociobiol. 29, 161–166. doi: 10.1007/BF00166397
Sackett, L. C., Etchberger, L. K., Mazzella, M. N., Lim, D. D., and Martin, A. P. (2009). Characterization of 18 microsatellite loci for three species of prairie dogs. Mol. Ecol. Resour. 10, 232–236. doi: 10.1111/j.1755-0998.2009.02796.x
Schwagmeyer, P. L., and Brown, C. H. (1983). Factors affecting male-male competition in thirteen-lined ground squirrels. Behav. Ecol. Sociobiol. 13, 1–6. doi: 10.1007/BF00295069
Schwagmeyer, P. L., and Foltz, D. W. (1990). Factors affecting the outcome of sperm competition in thirteen-lined ground squirrels. Anim. Behav. 39, 156–162. doi: 10.1016/S0003-3472(05)80735-3
Schwanz, L. E., Sherwin, W. B., Ognenvoska, K., and Lacey, E. A. (2016). Paternity and male mating strategies of a ground squirrel (Ictidomys parvidens) with an extended mating season. J. Mammal. 97, 576–588. doi: 10.1093/jmammal/gyv204
Seglund, A. E., Ernst, M., Grenier, B., Luce, A., Puchniak, A., and Schnurr, P. (2006). White-Tailed Prairie Dog Conservation Assessment. Western Association of Fish and Wildlife Agencies, Laramie, WY. Unpublished Report.
Sherman, P. W. (1989). Mate guarding as paternity insurance in Idaho ground squirrels. Nature 338, 418–420. doi: 10.1038/338418a0
Shuster, S. M., and Wade, M. J. (2003). Mating Systems and Strategies. Princeton, NJ: Princeton University Press.
Smith, R. L. (ed.). (1984). Sperm Competition and the Evolution of Animal Mating Systems. Orlando, FL: Academic.
Smuts, B. B., Cheney, D. L., Seyfarth, R. M., Wrangham, R. W., and Struhsaker, T. T. (eds.). (1987). Primate Societies. Chicago, IL: University of Chicago Press.
Solomon, N. G., and Keane, B. (2007). “Reproductive strategies in female rodents,” in Rodent Societies, eds J. O. Wolff and P. W. Sherman (Chicago, IL: University of Chicago Press), 42–56.
Solomon, N. G., Keane, B., Knoch, L. R., and Hogan, P. H. (2004). Multiple paternity in socially monogamous prairie voles (Microtus ochrogaster). Can. J. Zool. 82, 1667–1671. doi: 10.1139/z04-142
Thornhill, R. (1981). Panorpa (Mecoptera: Panorpidae) scorpionflies: systems for understanding resource-defense polygyny and alternative male reproductive efforts. Ann. Rev. Ecol. Syst. 12, 355–338. doi: 10.1146/annurev.es.12.110181.002035
Tileston, J. V., and Lechleitner, R. R. (1966). Some comparisons of the black-tailed and white-tailed prairie dogs in north-central Colorado. Am. Midl. Nat. 75, 292–316. doi: 10.2307/2423393
Tokarska, M., Marshall, T., Kowalczyk, R., Wojcik, J. M., Pertoldi, C., Kristensen, T. N., et al. (2009). Effectiveness of microsatellite and SNP markers for parentage and identity analysis in species with low genetic diversity: the case of European bison. Heredity 103:326. doi: 10.1038/hdy.2009.73
Trivers, R. L. (1972). “Parental investment and sexual selection,” in Sexual Selection and the Descent of Man, ed B. Campbell (Chicago, IL: Aldine), 136–179.
Weinman, L. R., Solomon, J. W., and Rubenstein, D. R. (2015). A comparison of single nucleotide polymorphism and microsatellite markers for analysis of parentage and kinship in a cooperatively breeding bird. Mol. Ecol. Res. 15, 502–511. doi: 10.1111/1755-0998.12330
Wells, C. P., Tomalty, K. M., Floyd, C. H., McElreath, M. B., May, B. P., and Van Vuren, D. H. (2017). Determinants of multiple paternity in a fluctuating population of ground squirrels. Behav. Ecol. Sociobiol. 71, 1–13. doi: 10.1007/s00265-017-2270-z
Westneat, D. F., Sherman, P. W., and Morton, M. L. (1990). The ecology and evolution of extra-pair copulations in birds. Curr. Ornithol. 331–369.
Wickler, W., and Seibt, U. (1983). “Monogamy: an ambiguous concept,” in Mate Choice, ed P. Bateson (Cambridge: Cambridge University Press), 33–50.
Wigby, S., and Chapman, T. (2004). Sperm competition. Curr. Biol. 14, R100–R103. doi: 10.1016/j.cub.2004.01.013
Wittenberger, J. F., and Tilson, R. L. (1980). The evolution of monogamy: hypotheses and evidence. Ann. Rev. Ecol. Syst. 11, 197–232. doi: 10.1146/annurev.es.11.110180.001213
Zeh, J. A., and Zeh, D. W. (2003). Toward a new sexual selection paradigm: polyandry, conflict, and incompatibility. Ethology 109, 929–950 doi: 10.1046/j.1439-0310.2003.00945.x
Keywords: Cynomys leucurus, mating system, polyandry, polygyny, white-tailed prairie dog
Citation: Hoogland JL, Trott R and Keller SR (2019) Polyandry and Polygyny in a Social Rodent: An Integrative Perspective Based on Social Organization, Copulations, and Genetics. Front. Ecol. Evol. 7:3. doi: 10.3389/fevo.2019.00003
Received: 13 August 2018; Accepted: 07 January 2019;
Published: 19 February 2019.
Edited by:
Heikki Helanterä, University of Oulu, FinlandReviewed by:
Kazuki Tsuji, University of the Ryukyus, JapanMichael Griesser, University of Zurich, Switzerland
Caitlin P. Wells, Colorado State University, United States
Copyright © 2019 Hoogland, Trott and Keller. This is an open-access article distributed under the terms of the Creative Commons Attribution License (CC BY). The use, distribution or reproduction in other forums is permitted, provided the original author(s) and the copyright owner(s) are credited and that the original publication in this journal is cited, in accordance with accepted academic practice. No use, distribution or reproduction is permitted which does not comply with these terms.
*Correspondence: John L. Hoogland, am9obi5ob29nbGFuZEB1bWNlcy5lZHU=