- 1Faculty of Engineering and Mathematical Sciences, Oceans Graduate School, The University of Western Australia, Perth, WA, Australia
- 2Faculty of Engineering and Mathematical Sciences, The Oceans Institute, The University of Western Australia, Perth, WA, Australia
- 3Anatomisches Institut der Universität Tübingen, Tübingen, Germany
Neuroanatomical studies of the peripheral sense organs and brains of deep-sea fishes are particularly useful for predicting their sensory capabilities and ultimately their behavior. Over the abyssal plane (between 2,000 and 6,000 m), communities of grenadiers (Gadiformes: Macrouridae) play an important ecological role as predator-scavengers. Previous studies suggest that these fishes rely heavily on chemosensation, especially olfaction. Furthermore, at least one species, Coryphaenoides armatus, undergoes an ontogenetic shift in the relative size of the optic tectum and the olfactory bulbs, suggesting. a shift from a reliance on vision to olfaction during ontogeny, apparently in association with a shift to a more scavenging lifestyle. Here, we compared the olfactory and visual sensory inputs to the brain in C. armatus, and in a second, closely-related species, Coryphaenoides profundicolus, by assessing the total number of axons (myelinated and unmyelinated) in the olfactory tract and optic nerve in a range of individuals from both species. In C. armatus, the numbers of axons in both tract and nerve increased with body size, with the total number of axons in the olfactory tract being far greater than the number of axons in the optic nerve. These differences became more pronounced in larger animals. In the two smaller C. profundicolus individuals (≤ 315 mm SL), there were more axons in the optic nerve than in the olfactory tract, but the opposite situation was found in larger individuals. As in C. armatus, the number of olfactory tract axons also increased with body size in C. profundicolus, but in contrast, the number of optic nerve axons decreased in this species. These results suggest that both C. armatus and C. profundicolus undergo an ontogenetic shift in sensory orientation, with olfaction becoming relatively more important than vision in larger animals. The differences in the ratio of olfactory tract to optic nerve axons in C. armatus indicate that olfaction is of particular importance to larger individuals of this species. In both species, the percentage of myelinated axons in the olfactory tract was relatively low, but we found evidence for interspecific and ontogenetic variation in the percentages of myelinated axons in the optic nerve.
Introduction
Grenadiers or rat-tails (Gadiformes, Macrouridae) are a diverse and abundant family of deep-sea, benthopelagic fishes, with just over 400 recognized species (Eschmeyer et al., 2018). These fishes generally have large heads, prominent eyes, and long, tapering bodies (Figure 1). Grenadiers have a global distribution, with most species found on the continental shelves and slopes at depths of between 200 and 2,000 m (Marshall, 1979; Cohen et al., 1990; Weitzman, 1997), although some species frequent abyssal depths from 2,000 to below 6,000 m (Gaither et al., 2016; Linley et al., 2016). Given their diversity and numerical abundance, grenadiers often comprise a large proportion of the biomass in deep-sea benthopelagic habitats and probably play an important ecological role as predator-scavengers in these communities (Haedrich, 1997; Drazen et al., 2008; Lee et al., 2008; Gerringer et al., 2017).
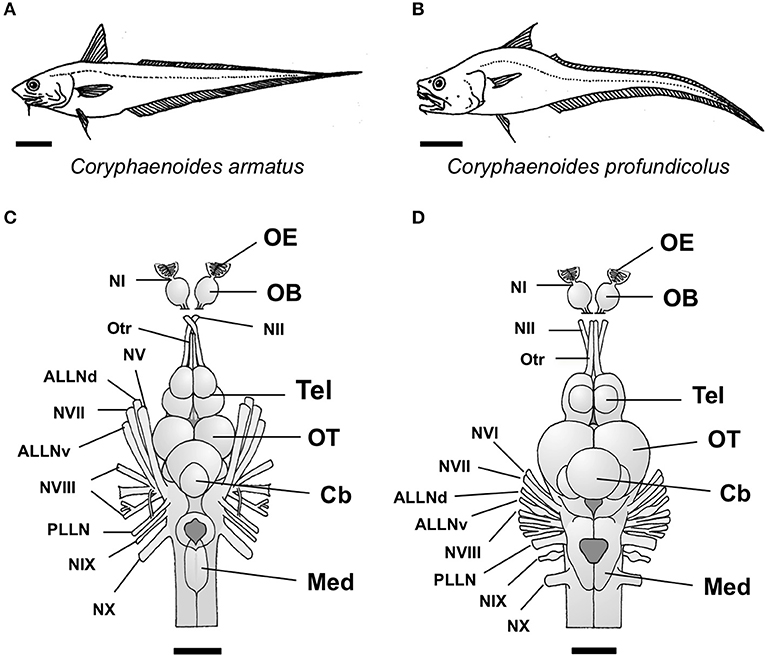
Figure 1. The two species of deep-sea grenadier investigated in this study, the abyssal grenadier Coryphaenoides armatus (A) and the deep-sea grenadier C. profundicolus (B). Dorsal views of the brain and the cranial and sensory nerves from the two largest individuals of these species investigated are presented in (C) C. armatus (900 mm SL) and (D) C. profundicolus (890 mm SL). In both species, the olfactory bulbs (OB) are stalked. The olfactory epithelium (OE) is positioned very close to the olfactory bulbs and the olfactory nerve (NI) is very short. The olfactory bulbs are connected to the telencephalon (Tel) by long olfactory tracts (Otr). ALLNd, dorsal root of the anterior lateral line nerve; ALLNv, ventral root of the anterior lateral line nerve; Cb, cerebellum; Med, medulla; NI, olfactory nerve (cranial nerve I); NII, optic nerve (cranial nerve II); NV, cranial nerve V; NVI, cranial nerve VI; NVII, cranial nerve VII; NVIII, cranial nerve VIII; NIX, cranial nerve IX; NX, cranial nerve X; OB, olfactory bulbs; OE, olfactory epithelium; OT, optic tectum; Otr, olfactory tract; PLLN, posterior lateral line nerve; Tel, telencephalon. Scale bars: 10 cm (A); 10 cm (B); 3 cm (C); 2.5 cm (D).
Of the abyssal grenadier species, the abyssal grenadier Coryphaenoides armatus, which is found at depths of ca. 2,000–5,200 m (Gaither et al., 2016), is the most widespread and abundant (Merrett and Haedrich, 1997). Analyses of stomach contents have confirmed that this species is euryphagous, ingesting both living and dead animal material, along with plant debris and even human refuse (Haedrich and Henderson, 1974; Sedberry and Musick, 1978). As well as being an active predator, adult C. armatus are heavily reliant on scavenging on carrion including food-falls, such as the carcasses of cephalopods, fishes, and cetaceans (Haedrich and Henderson, 1974; Mauchline and Gordon, 1991; Kemp et al., 2006), for survival (Drazen et al., 2008). Compared to other deep-sea fishes, adult C. armatus appear to be particularly well-equipped to detect the location of such food-falls using olfaction as they possess relatively large olfactory bulbs (Wagner, 2001a, 2002). Moreover, in situ video recorded by baited cameras on landers (autonomous vehicles deployed on the seafloor) has revealed that C. armatus is often the first species to appear when baited cameras are deployed and is presumably attracted to the bait by a very sensitive olfactory system (Wilson and Smith, 1984; Priede et al., 1990, 1994; Armstrong et al., 1992; Wagner, 2003). However, baits are predominantly taken only by large individuals (King et al., 2006), even in locations where trawls have demonstrated that smaller individuals are also present (Henriques et al., 2002; Collins et al., 2005). This suggests that the olfactory sense in smaller individuals of C. armatus is not as sensitive as that of larger animals, and/or that these smaller fish may be more dependent on different sources of food that require a greater reliance on sensory modalities other than olfaction. Interestingly, dietary studies show that carrion accounts for a lower proportion of the diet in small C. armatus compared to larger individuals, with epibenthic and benthic invertebrates being more numerous in the stomachs of smaller animals (Haedrich and Henderson, 1974; Martin and Christiansen, 1997; Drazen et al., 2008). Moreover, a functional analysis of the feeding apparatus suggests that feeding strategy differs between small and large individuals of C. armatus (McLellan, 1977). Smaller individuals have an elongated, shovel-like rostrum that they use to actively dig in muddy substrates for food. In contrast, larger animals, which have a less prominent rostrum, tend to swim above the bottom with their heads orientated downwards. A quantitative comparison of brain morphology across a broad size range also reveals changes in the relative sizes of the olfactory bulbs and the primary visual brain area, the optic tecta, in C. armatus, with the optic tectum being relatively larger in smaller animals and the olfactory bulbs being relatively larger in adults (Wagner, 2003).
In fishes, the nervous system grows continuously throughout life (Kaslin et al., 2008). Shifts in the relative size of different sensory brain areas, such as those described for C. armatus (Wagner, 2003) have been documented in a range of shallow water fishes and agnathans (lampreys; Cadwallader, 1975; Brandstätter and Kotrschal, 1989, 1990; Kotrschal et al., 1998; Lisney et al., 2007, 2017; Salas et al., 2015). These shifts are correlated with changes in the structure of the peripheral sense organs, and occur in association with ontogenetic shifts in habitat, diet and behavior, and may also coincide with the onset of sexual maturity. Therefore, Wagner's (2003) findings for C. armatus provide strong evidence that this species also undergoes a shift in sensory orientation (from vision to olfaction) during ontogeny, in association with changes in diet and feeding behavior.
Wagner's (2003) study on C. armatus used the “ellipsoid model” to quantify the volumes of different brain areas. This approach assumes that each brain area approximates the volume of an idealized ellipsoid or half ellipsoid (Huber et al., 1997), where linear measurements of the length, width, and depth of each brain area are translated into volumetric measures. This method has proved useful for quantifying variations in brain morphology in fishes, especially in species that are difficult or impossible to maintain in captivity, or to observe and study in detail in their natural environment, such as large, open water pelagic species (e.g., tunas, billfishes, and oceanic sharks; Lisney and Collin, 2006; Yopak and Lisney, 2012; Yopak et al., 2015) and deep-sea fishes (Collin et al., 2000; Wagner, 2001a,b, 2002, 2003). However, there are caveats associated with this approach. For example, the ellipsoid model can significantly under- or over-estimate the volume of brain areas compared to volumes obtained from other methods, such as stereological approaches on serial brain sections or the segmentation of brain regions following magnetic resonance imaging (MRI). This may be particularly relevant for multi-lobed brain areas (Ullmann et al., 2010), or those brain areas that enclose a large ventricular space (Yopak and Lisney, 2012). Furthermore, some brain areas, such as the optic tectum are multi-modal, where, in addition to visual input, the optic tectum also receives projections from other sensory modalities, such as the somatosensory and octavolateralis systems (Bodznick, 1991; Butler and Hodos, 2005). Therefore, some caution should be used when interpreting data obtained using the ellipsoid method, and if possible, complementary methods used to assess sensory input to the brain. One such method, which correlates well with differences in sensory orientation and behavior among species, is to compare the number of axons in the sensory (cranial) nerves associated with different senses. For example, in barn owls (Tyto alba), which are auditory specialists, there are more axons in their auditory nerve compared to other avian species (Köppl, 1997). In fishes and mammals, visually-oriented species have many more optic nerve axons compared to species that live in dim or turbid conditions (Huber and Rylander, 1992; Wohlert et al., 2016). Furthermore, the star-nosed mole (Condylura cristata), which has a unique and highly specialized star-shaped mechanosensory organ on the end of its snout, also possesses more than twice as many trigeminal nerve axons compared to other insectivores (Leitch et al., 2014).
In this study, we investigated olfactory and visual inputs to the brain in Coryphaenoides armatus, and in a second, closely-related abyssal grenadier species, the deep-sea grenadier C. profundicolus. This was achieved by assessing the number of axons in the olfactory tract and the optic nerve from different sized individuals of both species using transmission electron microscopy (TEM), which allows for the identification of very small axons that cannot be resolved using light microscopy (Vaney and Hughes, 1976). In C. armatus and C. profundicolus, the olfactory bulbs are stalked, and connected to the telencephalon by long olfactory tracts (Figure 1). The olfactory epithelium, which houses the olfactory receptor neurons (ORNs), is positioned very close to the olfactory bulbs (Døving, 1986). The ORNs detect odorants in the water and thus represent the first-order neurons in the olfactory pathway. Their unmyelinated axons, which comprise the olfactory nerve, project to the olfactory bulb, where they synapse with the second-order neurons (mitral cells) within specialized structures called glomeruli. In species with stalked olfactory bulbs, the olfactory nerves are very short and thus difficult to identify and isolate for neuroanatomical studies. Therefore, we opted to assess axon numbers in the olfactory tract in our specimens. The olfactory tract is primarily comprised of mitral cell axons, which project to the telencephalon and diencephalon, plus a small number of centrifugal fibers originating from the telencephalon that project back to the olfactory bulb (Westerman and Wilson, 1968; Døving, 1986; Hamdani and Døving, 2007). In contrast, the optic nerve contains the axons of the retinal ganglion cells, which are the third-order neurons in the visual pathway, receiving information from the photoreceptors (first-order neurons), via the bipolar cells (second-order neurons). The optic nerve also contains centrifugal fibers that project back to the retina, but the proportion of centrifugal axons within the optic nerve is very low across vertebrates (Itaya, 1980; Dunlop and Beazley, 1984; Brooks et al., 1999) including fishes (Schmidt, 1979; Gerwerzhagen et al., 1982; Collin and Collin, 1988).
For C. armatus, we predicted that, in accordance with Wagner's (2003) findings, we would see an increase in the ratio of axons in the olfactory tract compared to the optic nerve as body size increased. In comparison to C. armatus, much less is known about the biology of C. profundicolus. This species is found at similar depths to C. armatus (ca. 3,600–4,900 m; Gaither et al., 2016), but is not as abundant or cosmopolitan in its distribution. Nevertheless, in the eastern North Atlantic Ocean, both C. armatus and C. profundicolus are amongst the most abundant abyssal species and are often caught or observed together in the same locations (Merrett, 1992; Merrett and Fasham, 1998; Milligan et al., 2016). Moreover, C. profundicolus is morphologically similar to C. armatus (Figure 1) and adults this species also have relatively large olfactory bulbs, although not to the same extent as C. armatus (Wagner, 2001a, 2002). Therefore, for C. profundicolus, we predicted that we would also find a similar increase in the ratio of axons in the olfactory tract compared to the optic nerve with increasing body size.
Materials and Methods
Specimens
This study was carried out in accordance with the 5th edition of the National Health and Medical Research Council of Australia's code for the care and use of animals for scientific purposes (National Health Medical Research Council, 1990). The protocol was approved by the University of Western Australia animal ethics committee. Fish were collected on a scientific expedition on board the RRS Challenger in 1995 (Cruise No. 122) in the vicinity of 31–41°N (latitude) and 11–17°W (longitude) over the Madeira Abyssal Plain in the eastern North Atlantic Ocean, using a semi-balloon otter trawling net (OTSB 14) at depths between 100 and 4,000 m. The animals were dead when the nets were retrieved but, due to the advanced trawling techniques, the degree of external damage was minimized, and the time between death and the preservation of nervous material was also optimized. Each specimen was measured (standard length or SL in mm) and then, under a dissecting microscope, the cranium was removed thereby exposing the underlying brain and cranial nerves. Each whole head was preserved for electron microscopy by being immersed in 2.5% glutaraldehyde in 0.1 M phosphate buffer (pH, 7.4) for 24 h. The brain (with the cranial nerves still attached) was then carefully removed and stored in either fresh fixative or 0.1 M phosphate buffer until histological processing. Only specimens with suitable ultrastructural preservation were used in this study. In total, four individual C. armatus (size range 430–900 mm SL) and five individual C. profundicolus (size range 250–860 mm SL) were used in this study.
Olfactory Tract and Optic Nerve Analysis
Small pieces (1–2 mm) of the olfactory tract (close to the telencephalon) and the optic nerve (close to the back of the eye) of each specimen were removed and post-fixed in 1% osmium tetroxide before being dehydrated in an acetone series and embedded in araldite. Both the left and right sides were sampled for each specimen. Blocks were then cut for transmission electron microscopy (TEM) using a Leica ultramicrotome and a glass knife. Selected transverse sections of each tract/nerve were stained with uranyl acetate and lead citrate and examined on a Leo 912 Omega TEM (Carl Zeiss, Oberkochen, Germany). For each section, a series of low magnification (400–630 ×) electron micrographs of the whole tract/nerve were obtained with the aid of a computerized sampling program that enabled the total tract/nerve area to be photographed (with a 20% overlap). A series of high magnification (5,000–16,000 ×) photographs were then obtained by sampling at regular intervals throughout the tract/nerve so that any marked increases in axon density (especially within regions containing increased densities of unmyelinated axons) could be identified. Axon counts were made using photographic enlargements by manual counting with the aid of a Zeiss GSZ stereomicroscope (Carl Zeiss, Jena, Germany). In the olfactory tract in both species, three axonal regions could be identified, a central region occupied by high densities of unmyelinated axons surrounded by two regions containing predominantly myelinated axons (Figure 2B). Since the mean axon density in the unmyelinated region was appreciably higher than in the myelinated regions (Table 1), each of these areas of the olfactory tract was assessed separately. The total number of axons was obtained by multiplying the mean axon density in each region by the total tract area. The total area of each tract/nerve was assessed after the low magnification series of photographic enlargements were all incorporated into a montage and the borders of each tract/nerve traced, making sure to exclude non-axonal regions, such as blood vessels and the nerve sheath. Measurements of the tract/nerve area were performed by scanning these traced outlines into a computer using a scanner (Hewlett Packard Scan Jet IIcx; Palo Alto, CA, USA), and assessing the area using Digitrace software (Imatec, Miesbach, Germany). Axon densities were determined by dividing the total number of axons by the total area of each tract/nerve. The results for the left and right tracts/nerves were pooled and averaged for each individual. In the results section, species averages are presented ± standard deviation.
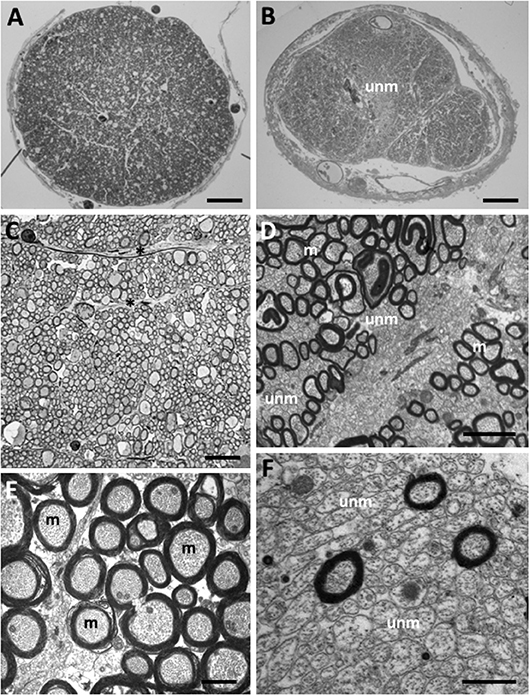
Figure 2. Myelinated and unmyelinated axons in the olfactory tract and optic nerve in Coryphaenoides armatus and C. profundicolus. (A) Light micrograph of the profile of a region of the optic nerve of C. profundicolus (390 mm SL) close to the back of the eye. (B) Light micrograph of the profile of a region of the olfactory tract in C. armatus (430 mm SL) close to the telencephalon, which appears to be divided into three regions including a central region occupied by high densities of unmyelinated axons (unm). (C) Low power electron micrograph of the optic nerve of C. armatus (900 mm SL) showing dense fascicles of myelinated axons separated by astroglial processes (*). (D) Electron micrograph of the olfactory tract of C. armatus (530 mm SL) showing a mixture of both myelinated (m) and unmyelinated (unm) axons. (E) Electron micrograph of the optic nerve axons in C. profundicolus (370 mm SL) showing the high density of myelinated axons (m). (F) Close up of the high density of unmyelinated axons (unm) in the central region of the olfactory tract of C. armatus (700 mm SL). Scale bars: 0.3 mm (A); 0.2 mm (B); 8.0 μm (C); 2.0 μm (D); 1.5 μm (E); 2.0 μm (F).
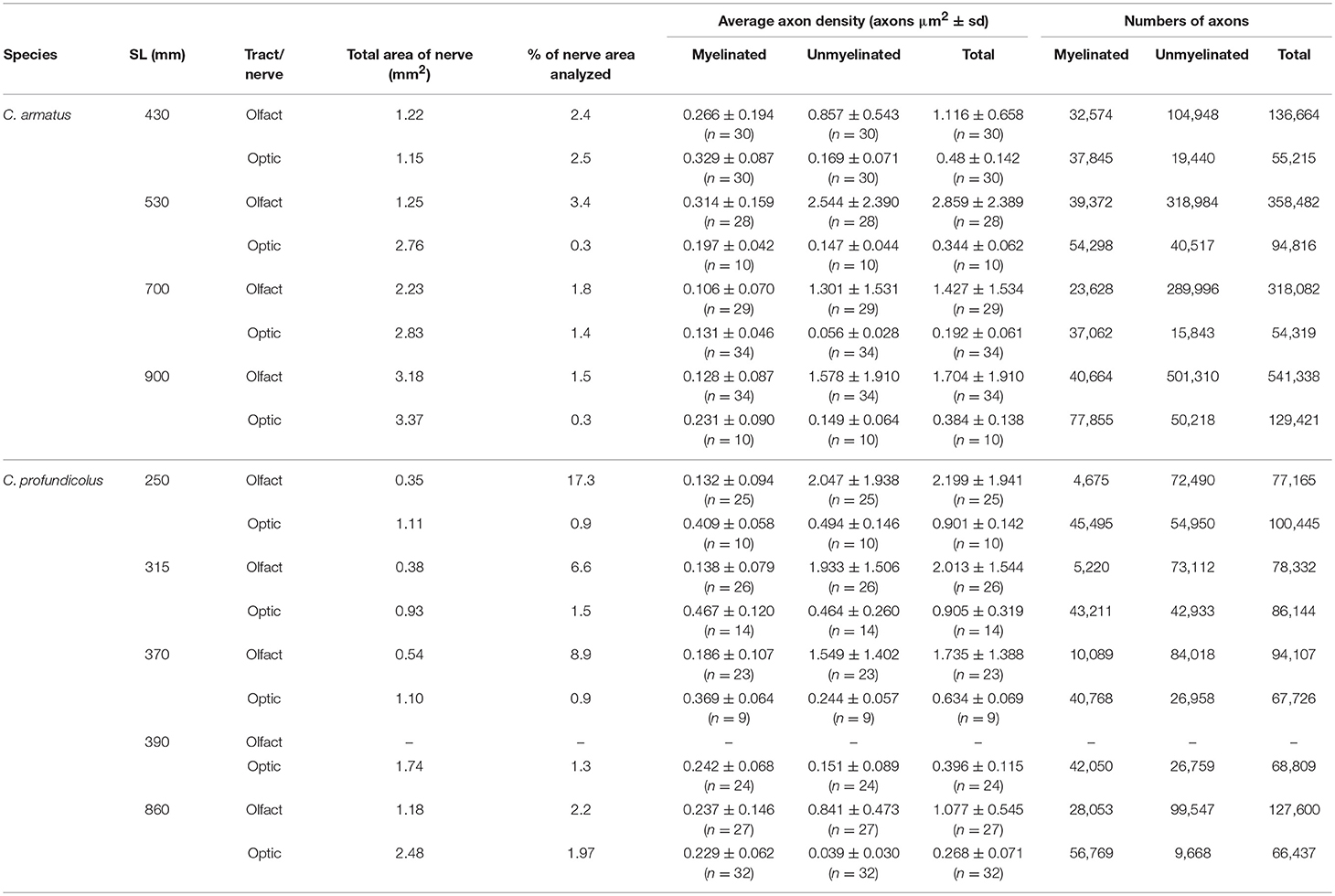
Table 1. Density and numbers of myelinated and unmyelinated axons in the olfactory tract and optic nerve from different sized individuals of Coryphaenoides armatus and C. profundicolus.
Results
In both species, the optic nerve was larger than the olfactory tract (C. armatus: average olfactory tract and optic nerve areas 1.97 ± 0.93 mm2 (n = 4) and 2.53 ± 0.96 mm2 (n = 4), respectively; C. profundicolus: average olfactory tract and optic nerve areas 0.61 ± 0.39 mm2 (n = 4) and 1.47 ± 0.64 mm2 (n = 5), respectively), and the area of both tract and nerve increased with increasing body size. Representative images of axons in the olfactory tract and optic nerve of both species are presented in Figure 2. Both myelinated and unmyelinated axons were identified in the olfactory tract and optic nerve in all specimens (also see Table 1). In general, the unmyelinated axons, which were smaller in size, were packed in higher densities than the myelinated axons (Table 1). There were also substantial differences in the proportion of myelinated and unmyelinated axons in the olfactory tract and the optic nerve across individuals and between species (Table 1; Figure 3; see below).
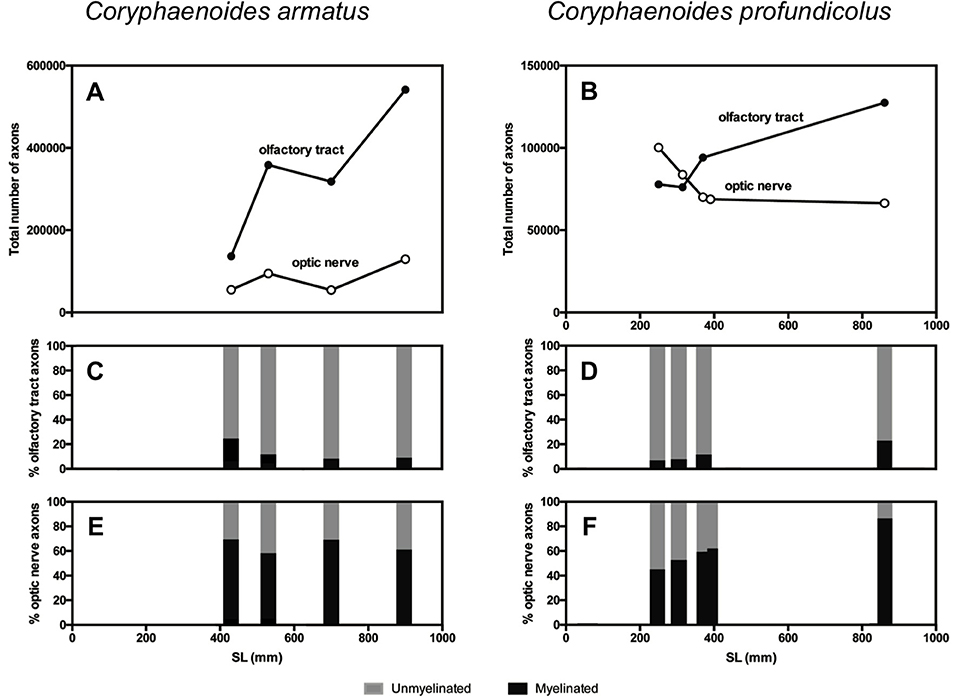
Figure 3. Axons in the olfactory tract and the optic nerve in Coryphaenoides armatus and C. profundicolus. The total number of axons in the olfactory tract (filled circles) and optic nerve (open circles) from different sized individuals in the two species is presented for (A) C. armatus and (B) C. profundicolus. Ontogenetic variation in the percentage of myelinated (black) and unmyelinated (gray) axons in the olfactory tract (C) and optic nerve (E) from different-sized individual C. armatus, and in the olfactory tract (D) and optic nerve (F) from different-sized individual C. profundicolus.
The results of the axon counts in the olfactory tract and the optic nerve in both species are presented in Table 1. When comparing the total number of axons across individuals and between species (Figure 3), the most noticeable finding is that the total number of axons in the olfactory tract in C. armatus far exceeds the numbers counted in the optic nerve in this species, as well as both the olfactory tract and optic nerve in C. profundicolus. This is particularly apparent in the larger individuals. For example, in the 900 mm SL individual C. armatus, the number of axons in the olfactory tract (541,338) was over four times greater than the total number of optic nerve axons (129,421), and over four and eight times greater than the total number of axons in the olfactory tract (127,600) and optic nerve (66,437), respectively, in the similarly-sized 860 mm SL individual of C. profundicolus. On average, the number of olfactory tract axons in C. armatus (338,642 ± 166,029; n = 4) was three and a half times greater than in the olfactory tract axons in C. profundicolus (93,900 ± 23,806; n = 4). Despite the substantial differences in the total number of axons in the olfactory tract between the two species, in both C. armatus and C. profundicolus, the total number of axons in the olfactory tract increased with SL (Figures 3A,B). In both species, the percentage of myelinated axons in the olfactory tract was relatively low (Table 1; Figures 3A,B), averaging 12.6 ± 7.6% (n = 4) of the total axon count in C. armatus and 11.4 ± 7.4% (n = 4) of the total axon count in C. profundicolus. However, in C. armatus, the percentage of total axons accounted for by myelinated axons decreased as SL increased, while the opposite was true for C. profundicolus.
On average, the total number of axons in the optic nerve was higher in C. armatus (83,443 ± 36,002; n = 4) compared to C. profundicolus (77,850 ± 14,213; n = 5). In C. armatus, the total number of axons in the optic nerve generally increased with increasing SL e.g., from 55,215 in the smallest individual to 129,421 in the largest individual (Figure 3C). In contrast, the total number of optic nerve axons in C. profundicolus declined steadily with increasing SL e.g., from 100,222 axons in the smallest individual to 66,437 axons in the largest individual (Figure 3D). The percentage of myelinated axons in the optic nerve in both species was similar on average C. armatus: 63.6 ± 5.7% (n = 4); C. profundicolus: 60.1 ± 15.6% (n = 5) and much greater than in the olfactory tract. However, while in C. armatus, the percentage remained relatively similar as SL increased (Figure 3C), in C. profundicolus the percentage of myelinated axons in the optic nerve increased as SL increased e.g., from 45.3% in the smallest individual to 85.5% in the largest individual (Figure 3D).
On average, the number of olfactory tract axons was greater than the number of optic nerve axons in both species (C. armatus: 338,642 vs. 83,443 axons; ratio: 4:1; C. profundicolus: 94,301 vs. 77,912 axons; ratio: 1.2:1). Given the very large numbers of axons in the olfactory tract in C. armatus, the ratios were higher in this species than in C. profundicolus. In both species, the ratio of olfactory tract axons to optic nerve axons generally increased with increasing SL (Figures 3E,F).
Discussion
Very little is known about the behavior of deep-sea fishes. While it is possible to film benthopelagic and benthic deep-sea animals using baited cameras attached to landers (Bagley et al., 2004; Jamieson et al., 2013), this process is logistically complicated and expensive. Moreover, bringing deep-sea animals to the surface alive is extremely difficult (Bagley et al., 2004; Drazen et al., 2005) making behavioral observations in aquaria all but impossible. Like all animals, deep-sea fishes are reliant on their sensory systems to gather information about their environment and to guide their behavior. Therefore, neuroanatomical studies of the peripheral sense organs and their afferent-recipient sites in the brain have proved a particularly useful approach for making deductions about their sensory capabilities, sensory orientation (i.e., which senses may be of particular overall importance), and behavior (Marshall, 1979; Wagner, 2001a,b). In abyssal food webs, grenadiers, such as the two species of Coryphaenoides studied here, occupy the top trophic positions (Drazen et al., 2008; Lee et al., 2008; Gerringer et al., 2017), and so knowledge regarding the sensory biology of these fishes is critical to understanding the biology of these ecologically important animals (Bailey et al., 2007).
Ontogenetic Shifts in Sensory Capability in Coryphaenoides
Previous work by Wagner (2001a, 2002, 2003) on the anatomy of the central nervous system in C. armatus resulted in two important conclusions: (1). As adults, the olfactory bulbs are relatively large, indicating that this species is an olfactory specialist, and (2). C. armatus undergoes an ontogenetic shift in brain morphology, whereby the relative size of the optic tectum decreases and the relative size of the olfactory bulbs increases as body size increases. This suggests that C. armatus undergoes a shift in sensory capability (from vision to olfaction) during ontogeny. Our study supports these findings. Across all of the different-sized individuals we studied, the number of olfactory tract axons was, on average, over four times greater than the number of axons in the optic nerve. Moreover, the ratio of olfactory tract axons to optic nerve axons increased as body size increased. In other words, the level of olfactory input exceeds the level of visual input to the brain in C. armatus, and this difference in olfactory vs. visual input increases ontogenetically. This neuroanatomical evidence for an ontogenetic shift in sensory capability appears to be closely associated with an an ontogenetic shift in diet and feeding behavior. As described previously, small C. armatus feed predominantly on epibenthic and benthic invertebrates (Haedrich and Henderson, 1974; Martin and Christiansen, 1997), which they take from or close to the bottom, or dig for in the soft, muddy substrate using their elongated rostrums (McLellan, 1977). Based on our results and those of Wagner (2003), we predict that vision may be more important than olfaction for the identification of these prey, especially epibenthic invertebrates that live on or just above the substrate. As the animals get larger, there is a shift away from benthic invertebrate prey and larger, more pelagic prey, such as cephalopods and fishes become more important. While some of these prey may be actively predated, scavenging on food-falls is particularly important source of food for larger C. armatus (Haedrich and Henderson, 1974; Mauchline and Gordon, 1991; Martin and Christiansen, 1997; Kemp et al., 2006). Larger C. armatus are also found at deeper depths, a trend seen in other scavenging deep-sea demersal fish (Merrett and Haedrich, 1997; Collins et al., 2005; King et al., 2006). This may be because a larger body size permits, for example, higher swimming speeds, larger energy reserves (and thus greater endurance), and a lower mass-specific metabolic rate (Collins et al., 2005). These factors would give larger individuals a better chance of surviving from meal to meal, allowing them to exploit deep-sea food resources, which although sparse and randomly distributed, tend to be large in size, such as the carcass of a whale or large elasmobranch (Higgs et al., 2014). In contrast, smaller C. armatus may occupy a different niche because until they reach a threshold minimum size they cannot compete with their larger conspecifics (Collins et al., 2005). Olfaction appears to become far more important in these larger animals, and their well-developed olfactory system likely helps these fishes to locate sparsely distributed food-falls in the abyss. Indeed, as previously noted, large C. armatus are often the first fish to appear at baited cameras (Wilson and Smith, 1984; Priede et al., 1990, 1994; Armstrong et al., 1992; Henriques et al., 2002; Wagner, 2003; Collins et al., 2005; Kemp et al., 2006; King et al., 2006). Wagner's (2003) results indicate that the ontogenetic shift in sensory capability in C. armatus occurs at a body size of between 400 and 500 mm SL, which is in agreement with the size range over which the shift in diet occurs in this species (Haedrich and Henderson, 1974; Martin and Christiansen, 1997). Unfortunately, we were not able to perform olfactory tract and optic nerve counts in any individuals smaller than 430 mm SL, but we predict that in smaller animals both the numbers of axons in the olfactory tract and optic nerve, and the ratio of olfactory tract axons to optic nerve axons, will be lower than the values presented for the 430 mm individual presented here.
Our results for C. profundicolus suggest that, in adults, olfaction is also important, although probably not to the same extent as in C. armatus. Wagner (2001a, 2002) found that, in comparison to other deep-sea fishes, the brain from an 890 mm SL C. profundicolus had relatively enlarged olfactory bulbs, and also an enlarged gustatory area, suggesting that chemoreception (olfaction and taste) is important to this species. Our data indicate that, like C. armatus, C. profundicolus also undergoes an ontogenetic shift in sensory orientation, with olfaction becoming relatively more important than vision in larger animals. Based on our axon counts, this shift appears to occur at a body size between 315 and 370 mm SL. However, the differences in olfactory vs. visual input as assessed by comparing the total number of axons, were not as great as those seen in C. armatus, as illustrated by the relatively low ratios of olfactory tract axons to optic nerve axons in C. profundicolus. A comparison of the brains of the two species illustrated in Figure 1 suggests that the optic tectum is relatively larger in C. profundicolus compared to C. armatus, which implies that vision may be relatively more important in the former. Unfortunately, no information is currently available on the brain morphology of different sized individuals of C. profundicolus, so it not known whether this species exhibits an ontogenetic shift in the relative size of the olfactory bulbs and optic tectum, such as that found in C. armatus. Moreover, little is known about the biology of C. profundicolus in general, especially in comparison to C. armatus. Dietary studies in C. profundicolus have not identified an ontogenetic shift (Denda et al., 2017), so it is not possible to establish whether the apparent ontogenetic shift from vision to olfaction identified here is correlated with a change in feeding strategy or behavior in this species.
Comparison of Olfactory Tract and Optic Nerve Axon Numbers With Other Species
Little work has been performed to quantify the numbers of axons in the olfactory tract in vertebrates using TEM, so it is difficult to make comparisons between our findings for C. armatus and C. profundicolus and other species. However, the average number of axons in both species is greater than the number reported for the crucian carp (Carassius carassius) (73,246; Westerman and Wilson, 1968). In another freshwater fish, the burbot (Lota lota), Döving and Gemne (1965) found that the number of myelinated axons was around 10,000, although these authors did not estimate the number of unmyelinated axons in L. lota. Since Westerman and Wilson (1968) found that 89.5% of the olfactory tract axons in C. carassius are unmyelinated, if similar proportions are assumed for L. lota, the total number of olfactory tract axons in this species could be around 100,000. These values for C. carassius and L. lota are over five times and three times lower than the highest and the average numbers of total olfactory tract axons that we counted in Coryphaenoides armatus, respectively. This supports Wagner's (2001a, 2002, 2003) designation of C. armatus as an olfactory specialist. In contrast, the numbers of axons in the olfactory tract in C. profundicolus are more similar to those for Carassius carassius and L. lota, further indicating that the olfactory system in C. profundicolus is not as well-developed as in C. armatus.
In contrast to the paucity of comparative information on axon numbers in the olfactory tract, quantitative analyses of the axons in the optic nerve using TEM have been made for a number of vertebrates (Brooks et al., 1999), including fishes. The total axon counts for both C. armatus and C. profundicolus are lower than those reported for some shallow water fishes, such as the striped mojarra (Eugerres plumieri) (200,000 axons; Tapp, 1974) and the goldfish (Carassius auratus) (180,000 axons; Easter et al., 1981). This suggests that vision may be relatively less important in the two species of grenadiers studied here compared to E. plumieri and C. auratus. However, the values we report here are more similar to those reported for minnows (Cyprinidae) (45,000–106,600; Huber and Rylander, 1992), the sandlance (Limnichthyes fasciatus) (104,452 axons; Collin and Collin, 1988) and the Australian lungfish (Neoceratodus forsteri) (74,100 axons; Bailes et al., 2006). Of these species, those with the lowest number of axons are fishes, like deep-sea grenadiers, that live in light-restricted habitats, such as minnows that live in turbid rivers (45,000–60,800 axons; Huber and Rylander, 1992) and N. forsteri. In dim environments, where the amount of light available for vision is limited, visual sensitivity is more important than visual acuity, and animals that live in such environments tend to have relatively low numbers of retinal ganglion cells and therefore retinal ganglion cell axons in the optic nerve. This relationship is consistent across vertebrates and similar examples can be found by comparing axon number in the optic nerve of diurnal and nocturnal birds, e.g., pigeons (Columbia livia) have 2.4 million axons (Binggelli and Paule, 1969) while barn owls (Tyto alba) have 680,000 axons (Wathey and Pettigrew, 1989) and the optic nerve of primates e.g., tufted capuchins (Cebus apella) have 1.09 million axons while Azara's night monkeys (Aotus azarae) have 480,000 axons (Finlay et al., 2008).
Ontogenetic Changes in Optic Nerve Axon Numbers
In both Coryphaenoides armatus and C. profundicolus, the total number of axons in the olfactory tract increased with body size. In C. armatus, this was also true for the total number of axons in the optic nerve. A similar situation has been shown to occur in the optic nerve in Carassius auratus and N. forsteri (Easter et al., 1981; Bailes et al., 2006) and also in the optic nerves of amphibians (Dunlop and Beazley, 1981, 1984). Like fishes, the central nervous system of amphibians exhibits intermediate growth and undergoes widespread and lifelong neurogenesis (Kaslin et al., 2008). It is also well-documented that the retina and the optic tectum grow continuously throughout life in both fishes and amphibians, through the addition of new neurons and tissue stretching (Straznicky and Gaze, 1972; Johns and Easter, 1977; Dunlop and Beazley, 1981, 1984; Easter et al., 1981; Raymond and Easter, 1983; Bakken and Stevens, 2012). Thus, the finding that there is a progressive decrease in the number of optic nerve axons in Coryphaenoides profundicolus is unexpected. To the best of our knowledge, this is the first example of a fish in which the optic nerve axons, and therefore their associated retinal ganglion cells, are either not being continually generated throughout life or the retina is undergoing appreciable periods of cell death and therefore axonal loss. Future work on visual development will hopefully confirm the mechanism of this findings in other models known to undergo continual retinal growth, such as the goldfish Carassius auratus, the zebrafish Danio rerio, the black bream Acanthopagrus butcheri (Shand et al., 2000), the bamboo shark Chiloscyllium punctatum (Harahush et al., 2014), deep-sea viperfish (Chauliodus sloani; Locket, 1980; Fröhlich and Wagner, 1998) and the lungfish N. forsteri (Bailes et al., 2006).
Proportions of Myelinated and Unmyelinated Axons
The percentage of myelinated axons in the olfactory tract was relatively low (around 11–12% on average) in Coryphaenoides armatus and C. profundicolus. Although little information exists on the axonal composition of the olfactory tract in fishes, our values correspond well with those of Westerman and Wilson (1968), who found that 10.5% of the olfactory tract axons in Carassius carassius are myelinated.
Regarding the optic nerve, we found that, on average, ~60% of the axons were myelinated in both Coryphaenoides. armatus and C. profundicolus. These values are considerably lower than the values reported for some other fishes, such as E. plumieri and Carassius auratus (≥96% of axons are myelinated; Tapp, 1974; Easter et al., 1981), and L. fasciatus (74% of axons are myelinated; Collin and Collin, 1988). However, in another genus of deep-sea fish, Conocara sp., only ~25% of the axons were found to be myelinated (Collin et al., 2000). In Coryphaenoides armatus, the percentage of myelinated axons was relatively similar across the different sized individuals, but in C. profundicolus, the percentage of myelinated axons increased progressively as body size increased. A similar situation has been described in N. forsteri, in which Bailes et al. (2006) found 17% of the optic nerve axons in a small individual to be myelinated, in contrast to 74% in a much larger fish. In some frogs, the proportion of myelinated axons also increases with age, but to a much smaller degree. For example, in moaning frogs (Heleioporus eyeri) and African clawed frogs (Xenopus laevis), the percentage of myelinated axons increases from 0.3 and 1.5% at metamorphic climax to 2.5 and 11% in adults, respectively (Dunlop and Beazley, 1981, 1984). It is known that the number of myelinated axons can vary along the length of the optic nerve (Cima and Grant, 1982; Playford and Dunlop, 1993), but we consider it highly unlikely that this accounts for the ontogenetic differences we report here, because all of our optic nerve samples were taken from the same location, just behind the eye, in each individual. Overall, it appears that in fishes, the proportions of myelinated and unmyelinated axons in the optic nerve can be highly variable, both ontogenetically within a species and among species. The reasons for this are unclear, but as myelin acts as an electrical insulator and serves to increase the velocity of propagation of nerve impulses along axons, the functional implications are that the speed at which visual information is relayed to the brain may vary among species and/or different size/age classes within a species, which in turn could influence behavioral reaction speeds (Bailey et al., 2007). Alternatively, as fish grow and the optic nerve gets longer (meaning that neural signals must travel increasing distances to reach the brain), increased levels of myelination could serve to maintain relatively constant conduction velocities (Bakken and Stevens, 2012). Interestingly, in C. profundicolus, while the total number of optic nerve axons decreases with body size, the degree of myelination increases with growth, perhaps as some form of compensatory function to maintain or increase the conduction velocity of neural signals along the optic nerve.
Author Contributions
All authors listed have made a substantial, direct and intellectual contribution to the work, and approved it for publication.
Conflict of Interest Statement
The authors declare that the research was conducted in the absence of any commercial or financial relationships that could be construed as a potential conflict of interest.
Acknowledgments
This study was generously supported by an Alexander von Humboldt Fellowship to SPC, and an Endeavour Award Postdoctoral Fellowship from the Australian Government to TJL. U. Mattheus provided expert help with the ultrastructure preparation and imaging. Special thanks are due to the organizers of the RRS Challenger deep-sea cruise No. 122, during which the specimens were collected, and the RV Sonne cruise No. 258-1, which provided the authors with the opportunity to reassess and conceptualize these data. The comments from three reviewers greatly improved the manuscript.
References
Armstrong, J. D., Bagley, P. M., and Priede, I. G. (1992). Photographic and acoustic tracking observations of the behaviour of the grenadier, Coryphaenoides (Nematonurus) armatus, the eel Synaphobranchus bathybius, and other abyssal demersal fish in the North Atlantic Ocean. Mar. Biol. 112, 535–544. doi: 10.1007/BF00346170
Bagley, P. M., Priede, I. G., Jamieson, A. D., Bailey, D. M., Battle, E. J. V., Henriques, C., et al. (2004). Lander techniques for deep-ocean biological research. Underw. Technol. 26, 3–12. doi: 10.3723/175605404783101567
Bailes, H. J., Trezise, A. E. O., and Collin, S. P. (2006). The number, morphology, and distribution of retinal ganglion cells and optic axons in the Australian lungfish Neoceratodus forsteri (Krefft 1870). Vis. Neurosci. 23, 257–273. doi: 10.1017/S0952523806232103
Bailey, D. M., Wagner, H.-J., Jamieson, A. J., Ross, M. F., and Priede, I. G. (2007). A taste of the deep-sea: the roles of gustatory and tactile searching behaviour in the grenadier fish Coryphaenoides armatus. Deep Sea Res. I 54, 99–108. doi: 10.1016/j.dsr.2006.10.005
Bakken, T. E., and Stevens, C. F. (2012). Visual system scaling in teleost fish. J. Comp. Neurol. 520, 142–153. doi: 10.1002/cne.22704
Binggelli, R. L., and Paule, N. J. (1969). The pigeon retina: qualitative aspects of the optic nerve and ganglion cell layer. J. Comp. Neurol. 137, 1–18. doi: 10.1002/cne.901370102
Bodznick, D. (1991). Elasmobranch vision: multimodal integration in the brain. J. Exp. Zool. Suppl. 256, 108–116. doi: 10.1002/jez.1402560515
Brandstätter, R., and Kotrschal, K. (1989). Life history of roach, Rutilus rutilus (Cyprinidae, Teleostei). A qualitative and quantitative study on the development of sensory brain areas. Brain Behav. Evol. 34, 35–42. doi: 10.1159/000116489
Brandstätter, R., and Kotrschal, K. (1990). Brain growth patterns in four European cyprinid species (Cyprinidae, Teleostei): roach (Rutilus rutilus), bream (Abramis brama), common carp (Cyprinus carpio) and sabre carp (Pelecus cultratus). Brain Behav. Evol. 35, 195–211. doi: 10.1159/000115867
Brooks, D. E., Komàromy, A. M., and Källberg, M. E. (1999). Comparative retinal ganglion cell and optic nerve morphology. Vet. Ophthalmol. 2, 3–11. doi: 10.1046/j.1463-5224.1999.00047.x
Cadwallader, P. L. (1975). Relationship between brain morphology and ecology in New Zealand Galaxiidae, particularly Galaxias vulgaris (Pisces: Salmoniformes). N. Z. J. Zool. 2, 35–43. doi: 10.1080/03014223.1975.9517860
Cima, C., and Grant, P. (1982). Development of the optic nerve in Xenopus laevis. II. Gliogenesis, myelination and metamorphic remodelling. J. Embryol. Exp. Morph. 72, 251–267.
Cohen, D. M., Inada, T., Iwamoto, T., and Scialabba, N. (1990). FAO Species Catalogue. Vol. 10. Gadiform Fishes of the World (Order Gadiformes). An Annotated and Illustrated Catalogue of Cods, Hakes, Grenadiers and Other Gadiform Fishes Known to Date. FAO Fisheries Synopsis 125. Rome: FAO.
Collin, S. P., and Collin, H. B. (1988). Topographic analysis of the retinal ganglion cell layer and optic nerve in the sandlance Limnichthyes fasciatus (Creeiidae, Perciformes). J. Comp. Neurol. 278, 226–241. doi: 10.1002/cne.902780206
Collin, S. P., Lloyd, D., and Wagner, H.-J. (2000). Foveate vision in deep-sea teleosts: a comparison of primary visual and olfactory inputs. Phil. Trans. R. Soc. Lond. B 355, 1315–1320. doi: 10.1098/rstb.2000.0691
Collins, M. A., Bailey, D. M., Ruxton, G. D., and Priede, I. G. (2005). Trends in body size across an environmental gradient: a differential response in scavenging and non-scavenging demersal deep-sea fish. Proc. R. Soc. Lond. B 272, 2051–2057. doi: 10.1098/rspb.2005.3189
Denda, A., Stefanowitsch, B., and Christiansen, B. (2017). From the epipelagic zone to the abyss: trophic structure at two seamounts in the subtropical and tropical eastern Atlantic–Part II Benthopelagic fishes. Deep Sea Res. I 130, 78–92. doi: 10.1016/j.dsr.2017.08.005
Døving, K. B. (1986). “Functional properties of the fish olfactory system” in Progress in Sensory Physiology, 6th Edn. eds H. Autrum, D. Ottoson, E. R. Perl, R. F. Schmidt, H. Simazu, and W. D. Willis (Berlin: Springer-Verlag), 39–104.
Döving, K. B., and Gemne, G. (1965). Electrophysiological and histological properties of the olfactory tract of the burbot (Lota lota L.). J. Neurophysiol. 28, 139–153. doi: 10.1152/jn.1965.28.1.139
Drazen, J. C., Bird, L. B., and Barry, J. P. (2005). Development of a hyperbaric trap-respirometer for the capture and maintenance of live deep-sea organisms. Limnol. Oceanogr. Methods 3, 488–498. doi: 10.4319/lom.2005.3.488
Drazen, J. C., Popp, B. N., Choy, C. A., Clemente, T., De Forest, L., and Smith, K. L. Jr. (2008). Bypassing the abyssal benthic food web: macrourid diet in the eastern north Pacific inferred from stomach content and stable isotope analyses. Limnol. Oceanogr. 53, 2644–2654. doi: 10.4319/lo.2008.53.6.2644
Dunlop, S. A., and Beazley, L. D. (1981). Changing retinal ganglion cell distribution in the frog Heleioporus eyeri. J. Comp. Neurol. 202, 221–237. doi: 10.1002/cne.902020208
Dunlop, S. A., and Beazley, L. D. (1984). A morphometric study of the retinal ganglion cell layer and optic nerve from metamorphosis in Xenopus laevis. Vision Res. 24, 417–427. doi: 10.1016/0042-6989(84)90040-3
Easter, S. S. Jr., Rusoff, A. C., and Kish, P. E. (1981). The growth and organization of the optic nerve and tract in juvenile and adult goldfish. J. Neurosci. 1,793–811. doi: 10.1523/JNEUROSCI.01-08-00793.1981
Eschmeyer, W. N., Fricke, R., and van der Laan, R. (Eds.),. (2018). Catalog of Fishes: Genera, Species, References. Available online at: http://researcharchive.calacademy.org/research/ichthyology/catalog/fishcatmain.asp (Accessed April 27, 2018).
Finlay, B. L., Franco, E. C. S., Yamada, E. S., Crowley, J. C., Parsons, M., Muniz, J. A. P. C., et al. (2008). Number and topography of cones, rods and optic nerve axons in New and Old World primates. Vis. Neurosci. 25, 289–299. doi: 10.1017/S0952523808080371
Fröhlich, E., and Wagner, H.-J. (1998). Development of multibank rod retinae in deep-sea fishes. Vis. Neurosci. 15, 477–483. doi: 10.1017/S095252389815304X
Gaither, M. R., Violi, B., Gray, H. W. I., Neat, F., Drazen, J. C., Grubbs, R. D., et al. (2016). Depth as a driver of evolution in the deep sea: insights from grenadiers (Gadiformes: Macrouridae) of the genus Coryphaenoides. Mol. Phylogenet. Evol. 104, 73–82. doi: 10.1016/j.ympev.2016.07.027
Gerringer, M. E., Popp, B. N., Linley, T. D., Jamieson, A. J., and Drazen, J. C. (2017). Comparative feeding ecology of abyssal and hadal fishes through stomach content and amino acid isotope analysis. Deep Sea Res. I 121, 110–120. doi: 10.1016/j.dsr.2017.01.003
Gerwerzhagen, K., Rickmann, M. J., Meyer, D. L., and Ebbesson, S. O. E. (1982). Optic tract cells projecting to the retina in the teleost, Pantodon bucholzi. Cell Tissue Res. 225, 23–28. doi: 10.1007/BF00216215
Haedrich, R. K. (1997). “Distribution and population ecology” in Deep-sea Fishes, ed. D. J. Randall, and A. P. Farrell San Diego: Academic Press), 79–114.
Haedrich, R. K., and Henderson, N. R. (1974). Pelagic food of Coryphaenoides armatus, a deep benthic rattail. Deep Sea Res. 21, 739–744.
Hamdani, E. H., and Døving, K. B. (2007). The functional organization of the fish olfactory system. Prog. Neurobiol. 82, 80–86. doi: 10.1016/j.pneurobio.2007.02.007
Harahush, B. K., Hart, N. S., and Collin, S. P. (2014). Ontogenetic changes in retinal ganglion cell distribution and spatial resolving power in the brown-banded bamboo shark Chiloscyllium punctatum (Elasmobranchii). Brain Behav. Evol. 83, 286–300. doi: 10.1159/000361036
Henriques, C., Priede, I. G., and Bagley, P. M. (2002). Baited camera observations of deep-sea demersal fishes of the northeast Atlantic Ocean at 15–28°N off West Africa. Mar. Biol. 141, 307–314. doi: 10.1007/s00227-002-0833-6
Higgs, N. D., Gates, A. R., and Jones, D. O. B. (2014). Fish food in the deep sea: revisiting the role of large food-falls. PLoS ONE 9:e96016. doi: 10.1371/journal.pone.0096016
Huber, R., and Rylander, M. K. (1992). Quantitative histological study of the optic nerve in species of minnows (Cyprinidae, Teleostei) inhabiting clear and turbid water. Brain Behav. Evol. 40, 250–255. doi: 10.1159/000113916
Huber, R., van Staaden, M. J., Kaufman, L. S., and Liem, K. F. (1997). Microhabitat use, trophic patterns, and the evolution of brain structure in African cichlids. Brain Behav. Evol. 50, 167–182. doi: 10.1159/000113330
Itaya, S. K. (1980). Retinal efferents from the pretectal are in the rat. Brain Res. 201, 436–441. doi: 10.1016/0006-8993(80)91049-5
Jamieson, A. J., Boorman, B., and Jones, O. B. (2013). “Deep-sea benthic sampling” in Methods for the Study of Marine Benthos, 4th Edn. ed A. Eleftheriou (Chichester: Wiley), 285–348.
Johns, P. R., and Easter, S. S. Jr. (1977). Growth of the adult goldfish eye. II. Increase in retinal cell number. J. Comp. Neurol. 176, 331–342. doi: 10.1002/cne.901760303
Kaslin, J., Ganz, J., and Brand, M. (2008). Proliferation, neurogenesis and regeneration in the non-mammalian vertebrate brain. Phil. Trans. R. Soc. Lond. B 363, 101–122. doi: 10.1098/rstb.2006.2015
Kemp, K. M., Jaieson, A. J., Bagley, P. M., McGarth, H., Bailey, D. M., Collins, M. A., et al. (2006). Consumption of large bathyal food fall, a six month study in the NE Atlantic. Mar. Ecol. Prog. Series 310, 65–76. doi: 10.3354/meps310065
King, N. J., Bagley, P. M., and Priede, I. G. (2006). Depth zonation and latitudinal distribution of deep-sea scavenging demersal fishes of the Mid-Atlantic Ridge, 42 to 53°N. Mar. Ecol. Prog. Series 319, 263–274. doi: 10.3354/meps319263
Köppl, C. (1997). Number and axon calibres of cochlear afferents in the barn owl. Aud. Neurosci. 3, 313–334.
Kotrschal, K., van Staaden, M. J., and Huber, R. (1998). Fish brains: evolution and environmental relationships. Rev. Fish Biol. Fish. 8, 373–408. doi: 10.1023/A:1008839605380
Lee, C. C., Chen, H. W., Hsu, C. C., and Shao, K. T. (2008). “Feeding ecology of three congeneric grenadiers in waters of northeastern Taiwan” in Grenadiers of the World Oceans: Biology, Stock Assessment, and Fisheries, Vol. 63, eds A. M. Orlov and T. Iwamoto (Bethesda, MD: American Fisheries Society), 185–201.
Leitch, D. B., Sarko, D. K., and Catania, K. C. (2014). Brain mass and cranial nerve size in shrews and moles. Sci. Rep. 4:6241. doi: 10.1038/srep06241
Linley, T. D., Gerringer, M. E., Yancey, P. H., Drazen, J. C., Weinstock, C. L., and Jamieson, A. J. (2016). Fishes of the hadal zone including new species, in situ observations and depth records of Liparidae. Deep Sea Res. I 114, 99–100. doi: 10.1016/j.dsr.2016.05.003
Lisney, T. J., Bennett, M. B., and Collin, S. P. (2007). Volumetric analysis of sensory brain areas indicates ontogenetic shifts in the relative importance of sensory systems in elasmobranchs. Raff. Bull. Zool. Suppl. 14, 7–15. Available online at: https://lkcnhm.nus.edu.sg/app/uploads/2017/06/s14rbz02_Lisney-pp7-15.pdf
Lisney, T. J., and Collin, S. P. (2006). Brain morphology in large pelagic fishes: a comparison between sharks and teleosts. J. Fish Biol. 68, 532–554. doi: 10.1111/j.0022-1112.2006.00940.x
Lisney, T. J., Yopak, K. E., Camilieri-Asch, V., and Collin, S. P. (2017). Ontogenetic shifts in brain organization in the bluespotted stingray, Neotrygon kuhlii (Chondrichthyes: Dasyatidae). Brain Behav. Evol. 89, 68–83. doi: 10.1159/000455223
Locket, N. A. (1980). Variation in the architecture with size in the multiple-bank retina of a deep-sea teleost, Chauliodus sloani. Proc. R. Soc. Lond. B 208, 223–242. doi: 10.1098/rspb.1980.0050
Martin, B., and Christiansen, B. (1997). Diets and standing stocks of benthopelagic fishes at two bathymetrically different midoceanic localities in the northeast Atlantic. Deep Sea Res. I 44, 541–558. doi: 10.1016/S0967-0637(97)00008-3
Mauchline, J., and Gordon, J. D. M. (1991). Oceanic pelagic prey of benthopelagic fish in the benthic layer of a marginal oceanic region. Mar. Ecol. Prog. Series 74, 109–115. doi: 10.3354/meps074109
McLellan, T. (1977). Feeding strategies of the macrourids. Deep Sea Res. 24, 1019–1036. doi: 10.1016/0146-6291(77)90572-0
Merrett, N. R. (1992). Demersal ichthyofaunal distribution in the abyssal eastern North Atlantic, with special reference to Coryphaenoides (Nematonurus) armatus (Macrouridae). J. Mar. Biol. Ass. U.K. 72, 5–24. doi: 10.1017/S002531540004875X
Merrett, N. R., and Fasham, M. J. R. (1998). Demersal ichthyofaunal distribution in the abyssal North Atlantic revisited: the effect of sample size on ordination. Mar. Ecol. Prog. Series 173, 267–274. doi: 10.3354/meps173267
Merrett, N. R., and Haedrich, R. L. (1997). Deep-sea Demersal Fish and Fisheries. London: Chapman and Hall.
Milligan, R. J., Morris, K. J., Bett, B. J., Durden, J. M., Jones, D. O. B., Robert, K., et al. (2016). High resolution study of the spatial distributions of abyssal fishes by autonomous underwater vehicle. Sci. Rep. 6:26095. doi: 10.1038/srep26095
National Health and Medical Research Council (1990). Australian Code for the Care and Use of Animals for Scientific Purposes. 5th Edn. Canberra: National Health and Medical Research Council.
Playford, D. E., and Dunlop, S. A. (1993). A biphasic sequence of myelination in the developing optic nerve of the frog. J. Comp. Neurol. 333, 83–93. doi: 10.1002/cne.903330107
Priede, I. G., Bagley, P. M., Smith, A., Creasey, S., and Merrett, N. R. (1994). Scavenging deep demersal fishes of the Porcupine Seabight, North-East Atlantic: observations by baited camera, trap and trawl. J. Mar. Biol. Ass. U.K. 74, 481–498. doi: 10.1017/S0025315400047615
Priede, I. G., Smith, K. L. Jr., and Armstrong, J. D. (1990). Foraging behavior of abyssal grenadier fish: inferences from acoustic tagging and tracking in the northern Pacific Ocean. Deep Sea Res. 37, 81–101 doi: 10.1016/0198-0149(90)90030-Y
Raymond, P. A., and Easter, S. S. Jr. (1983). Postembryonic growth of the optic tectum in goldfish. I. Location of germinal cells and numbers of neurons produced. J. Neurosci. 3, 1077–1091. doi: 10.1523/JNEUROSCI.03-05-01077.1983
Salas, C. A., Yopak, K. E., Warrington, R. E., Hart, N. S., Potter, I. C., and Collin, S. P. (2015). Ontogenetic shifts in brain scaling reflect behavioral changes in the life cycle of the pouched lamprey Geotria australis. Front. Neurosci. 9:251. doi: 10.3389/fnins.2015.00251
Schmidt, J. T. (1979). The laminar organisation of optic nerve fibers in the tectum of goldfish. Proc. R. Soc. Lond. B 205, 287–306. doi: 10.1098/rspb.1979.0066
Sedberry, G. R., and Musick, J. A. (1978). Feeding strategies of some demersal fishes of the continental slope and rise off the mid-Atlantic coast of the USA. Mar. Biol. 44, 357–375. doi: 10.1007/BF00390900
Shand, J., Chin, S. M., Harman, A. M., Moore, S., and Collin, S. P. (2000). Variability in the location of the retinal ganglion cell area centralis is correlated with ontogenetic changes in feeding behaviour in the black bream, Acanthopagrus butcheri (Sparidae, Teleostei). Brain Behav. Evol. 55, 176–190. doi: 10.1159/000006651
Straznicky, K., and Gaze, R. M. (1972). Development of the optic tectum in Xenopus laevis. An autoradiographic study. J. Embryol. Exp. Morphol. 26, 87–115.
Tapp, R. L. (1974). Axon numbers and distribution, myelin thickness, and the reconstruction of the compound action potential in the optic nerve of the teleost: Eugerres plumieri. J. Comp. Neurol. 153, 267–274. doi: 10.1002/cne.901530304
Ullmann, J. F. P., Cowin, G., and Collin, S. P. (2010). Quantitative assessment of brain volumes in fish: comparison of methodologies. Brain Behav. Evol. 76, 261–270. doi: 10.1159/000321467
Vaney, D. I., and Hughes, A. (1976). The rabbit optic nerve: fibre diameter spectrum, fibre count, and comparison with a retinal ganglion cell count. J. Comp. Neurol. 170, 241–252. doi: 10.1002/cne.901700208
Wagner, H.-J. (2001a). Brain areas in abyssal demersal fish. Brain Behav. Evol. 57, 301–316. doi: 10.1159/000047249
Wagner, H.-J. (2001b). Sensory brain areas in mesopelagic fishes. Brain Behav. Evol. 57, 117–133. doi: 10.1159/000047231
Wagner, H.-J. (2002). Sensory brain areas in three families of deep-sea fish (slickheads, eels and grenadiers): comparison of mesopelagic and demersal species. Mar. Biol. 141, 807–817. doi: 10.1007/s00227-002-0892-8
Wagner, H.-J. (2003). Volumetric analysis of brain areas indicates a shift in sensory orientation during development in the deep-sea grenadier Coryphaenoides armatus. Mar. Biol. 142, 791–797. doi: 10.1007/s00227-002-0990-7
Wathey, J. C., and Pettigrew, J. D. (1989). Quantitative analysis of the retinal ganglion cell layer and optic nerve of the barn owl Tyto alba. Brain Behav. Evol. 33, 279–292. doi: 10.1159/000115936
Weitzman, S. H. (1997). “Systematics of deep-sea fishes” in Deep-sea Fishes, ed. D. J. Randall, and A. P. Farrell (San Diego, CA: Academic Press), 43–77.
Westerman, R. A., and Wilson, J. A. F. (1968). The fine structure of the olfactory tract in the teleost Carassius carassius L. Z. Zellforsch. Mikrosk. Anat. 91, 186–199. doi: 10.1007/BF00364310
Wilson, R. R. Jr., and Smith, K. L. Jr. (1984). Effect of near-bottom currents on detection of bait by the abyssal grenadier fishes Coryphaenoides spp., recorded in situ with a video camera free-vehicle. Mar. Biol. 84, 83–91. doi: 10.1007/BF00394530
Wohlert, D., Kröger, J., Witt, M., Schmitt, O., Wree, A., Czech-Damal, N., et al. (2016). A comparative morphometric analysis of three cranial nerves in two phocids: the hooded seal (Cystophora cristata) and the harbor seal (Phoca vitulina). Anat. Rec. 299, 370–378. doi: 10.1002/ar.23298
Yopak, K. E., and Lisney, T. J. (2012). Allometric scaling of the optic tectum in cartilaginous fishes. Brain Behav. Evol. 80, 108–126. doi: 10.1159/000339875
Keywords: axons, brain, deep-sea fish, grenadier, olfaction, ontogenetic shift, sensory system, vision
Citation: Lisney TJ, Wagner H-J and Collin SP (2018) Ontogenetic Shifts in the Number of Axons in the Olfactory Tract and Optic Nerve in Two Species of Deep-Sea Grenadier Fish (Gadiformes: Macrouridae: Coryphaenoides). Front. Ecol. Evol. 6:168. doi: 10.3389/fevo.2018.00168
Received: 27 July 2018; Accepted: 03 October 2018;
Published: 09 November 2018.
Edited by:
Chuan-Chin Chiao, National Tsing Hua University, TaiwanReviewed by:
Wei Li, Retinal Neurophysiology Section, National Institutes of Health (NIH), United StatesFrancisco Nadal-Nicolas, Retinal Neurophysiology Section, National Institutes of Health (NIH), United States, in collaboration with reviewer WL
Karen Carleton, University of Maryland, College Park, United States
Wen-Sung Chung, The University of Queensland, Australia
Copyright © 2018 Lisney, Wagner and Collin. This is an open-access article distributed under the terms of the Creative Commons Attribution License (CC BY). The use, distribution or reproduction in other forums is permitted, provided the original author(s) and the copyright owner(s) are credited and that the original publication in this journal is cited, in accordance with accepted academic practice. No use, distribution or reproduction is permitted which does not comply with these terms.
*Correspondence: Shaun P. Collin, c2hhdW4uY29sbGluQHV3YS5lZHUuYXU=