- 1Australian Institute of Marine Science, Crawley, WA, Australia
- 2Department of Marine Biology and Aquaculture, ARC Centre of Excellence for Coral Reef Studies, College of Science and Engineering, James Cook University, Townsville, QLD, Australia
- 3College of Life and Environmental Sciences, University of Exeter, Exeter, United Kingdom
- 4Department of Biology, University of Saskatchewan, Saskatoon, SK, Canada
- 5Department of Biomedical Sciences, WCVM, University of Saskatchewan, Saskatoon, SK, Canada
Although the behavior of most organisms evolves in response to harvest, teleost fishes in marine systems have remained susceptible to the same basic fishing techniques of hook and lines and nets for millennia. We argue that this has occurred because these techniques circumvent the evolutionary arms race that exists between all other non-human marine predators and their fish prey that codifies effective tactics of foraging and predator evasion. By removing size relationships between predator and prey, avoiding predator recognition, disrupting learning cues and through the rapid evolution of technology, fishing by humans subverts natural processes of selection on fishes that act to reduce mortality to non-human predators. This engenders high capture efficiency and explains why non-human predators in marine systems are forced to focus on naïve and young individuals as prey, whereas humans are able to target adult fishes. Our very high rates of harvest and disruption of predator-prey relationships shifts the morphology and life history of target species toward traits (small adult size etc.) that are a disadvantage in situations where they must avoid non-human predators and thus has the potential to contribute to reduced resilience of fished populations and impair the recovery of stocks when harvesting ceases.
Introduction
Any organism that is subject to high rates of mortality due to human behavior rapidly evolves behavioral, anatomical, and demographic adaptations to reduce mortality rates. Examples of this process occur across terrestrial and aquatic taxa of all sizes, phylogenies, and life history strategies (Sullivan et al., 2017). They include the evolution of female tusklessness in elephants as a response to poaching (Jachmann et al., 1995), rodenticide resistance (Song et al., 2011), and trap avoidance in small mammals (Parkes and Panetta, 2009) reductions in size due to harvesting in wild plants (Sullivan et al., 2017) and antibiotic resistance in microbes (Chopra and Roberts, 2001). In aquatic systems, experimental studies show that for teleost fishes, this process is remarkably swift; size-selective harvesting in fish populations can generate changes in adult size, reproductive output, activity and personality in only five generations (Uusi-Heikkilä et al., 2015) and these changes have a strong genetic basis that is slow to revert after the cessation of harvest (Conover et al., 2009; Uusi-Heikkilä et al., 2017). Notably, reductions in the adult body size of fishes (and accompanying reproductive output) over limited time spans of a few decades in response to fisheries are common across many wild populations (Jørgensen et al., 2007).
Teleost fishes have been collected from the wild by humans as a source of protein for a very long time. The first archeological evidence of targeted pelagic fishing of teleosts in marine environments has been recovered from cave systems in Timor in South-East Asia and dates back 42,000 years (O'Connor et al., 2011). The practice was probably occurring well before this time in the seafaring communities of humans colonizing the edges of archipelagos and continents in the region. The same excavations also revealed the first evidence of the manufacture and use of recognizable fish hooks somewhere between 23,000–16,000 years ago (O'Connor et al., 2011). Despite relatively low population sizes until recent centuries, humans have used these tools to great effect, proving themselves to be extremely efficient hunters, with evidence of overharvesting of fish stocks dating back centuries or even thousands of years in many freshwater (Finney et al., 2000) and marine habitats (e.g., Lopes et al., 2016). In coral reef systems, it has been argued that subsistence fishing by humans has been so effective that the practices of marine tenure and spatial closures evolved in traditional societies as a means of avoiding the over-harvesting of resources (Johannes, 1978, 2002; Cinner, 2007). Today, with the onset of global commercial fisheries, mortality imposed by humans is likely to be the single greatest source of loss for populations of heavily-targeted species, exceeding rates of natural mortality by up to 400% (Mertz and Myers, 1998).
The long history of human exploitation (>10,000 years) using the same basic techniques of hooks, lines and nets, high rates of fishing mortality, and the demonstrated malleability of fish morphology and genetics in the face of selective fishing practices, prompts an obvious question: why hasn't the behavior of fishes evolved to completely avoid these types of fishing? In order to provide an answer, we compare the behavioral interactions between fish and their non-human and human predators, focusing on the types of fishing practices (hook and line, nets, spears) that have the longest historical antecedents. We argue that fishing using these types of techniques has been successful for millennia because it directly circumvents all of the evolutionary constraints that allow fish as prey to detect, recognize, and learn about their predators. We show that whenever these constraints are relaxed, particularly where fish are able to learn about human predators, their behavior evolves very rapidly to reduce mortality to fishing pressure.
We argue that our subversion of predator-prey relationships underlies the ability of humans to act as “super predators” in aquatic systems, allowing us to harvest prey at 14 times the rate of other predators (Darimont et al., 2015). Moreover, because our fishing practices can act in direct opposition to selection that promotes survivorship in the face of non-human predators, we may enhance the evolution of traits that make fishes more susceptible to natural mortality. This has the potential to reduce the resilience of populations targeted by fisheries and to delay their recovery from over-fishing.
Here, we show that fishing by humans disrupts and in some cases counteracts evolved predator-prey relationships through four principal means: the removal of size-structured relationships between predator and prey; the avoidance of predator recognition systems; the disruption of learning cues; and the rapid evolution of technology.
Size-structured Food Chains in the Sea
Once they die, most organisms in the ocean sink. This poses a challenge for many fish as predators, because it restricts the opportunity for them to dismember prey before it descends beyond their reach. Over evolutionary time, this has provided strong selective pressure for these predators to engulf and swallow prey whole (Brose et al., 2006). Because the capture of prey is also energetically expensive due to high rates of failure, this selection pressure is reinforced by the need to minimize prey handling time due to strong competition from other predators attempting to steal prey and capitalize on a cheap meal (Iyengar, 2008). As a result, most carnivorous fishes swallow their prey whole.
The key determinant of the ability of a predatory fish to consume whole prey is the size of the mouth gape, a trait directly related to body size. Gapes of many teleost fishes are relatively small for their size. The evolution of protractile jaws has allowed predators to suck in prey via a vacuum created by the rapid expansion of the buccal cavity, which dramatically increases their foraging efficiency but forces the gape to remain small to maintain vacuum efficiency (Bellwood et al., 2015). This morphology also greatly limits the ability of the predator to chew food (Bellwood et al., 2015). Ultimately, this link between mouth gape and body size has resulted in marine ecosystems being inherently size-structured. Because predators are, on average, heavier by one to three orders of magnitude (Woodward et al., 2005; Brose et al., 2006; Bersier and Kehrli, 2008; Naisbit et al., 2011; Riede et al., 2011; Trebilco et al., 2013) and between three to four times longer (Pauly and Watson, 2005) than their prey, an easy way for prey to evade predation is to simply avoid animals that are larger than they are. The largest fishes will also pose the greatest threats due to their wider gapes (Urban, 2007), superior visual acuity (Breck and Gitter, 1983; Li et al., 1985; Walton et al., 1994) and swimming ability (Weihs, 1977).
Fishing by humans, notably hook, and line techniques, subverts any attempts by fish to avoid predators based on their size by cloaking predation as prey. The use of baited hooks and lures not only makes the predator much smaller than the prey they target, the low cost of this fishing gear also allows the predator to be very numerous (e.g., longlines). From the prey's viewpoint, gape-limitation of non-human predators selects for fast growth rate and consequently, high foraging rates. For this reason, it also favors opportunistic, low-cost foraging, which explains the occurrence of kleptoparasitism (Nilsson and Brönmark, 1999) and facultative scavenging (Dudley et al., 2000; Jenkins et al., 2004) in many fishes. Any avoidance of baits or lures acts contrary to this very strong selective pressure and likely explains the effectiveness of very similar types of fishing lures and hooks over very long time periods (centuries; Figure 1). Fishes do have the ability to learn to recognize and avoid hooks and lures (see below), but in many cases, this only occurs where there are high rates of escapement or where fishes are deliberately returned to the water after capture (e.g., angling, recreational fisheries).
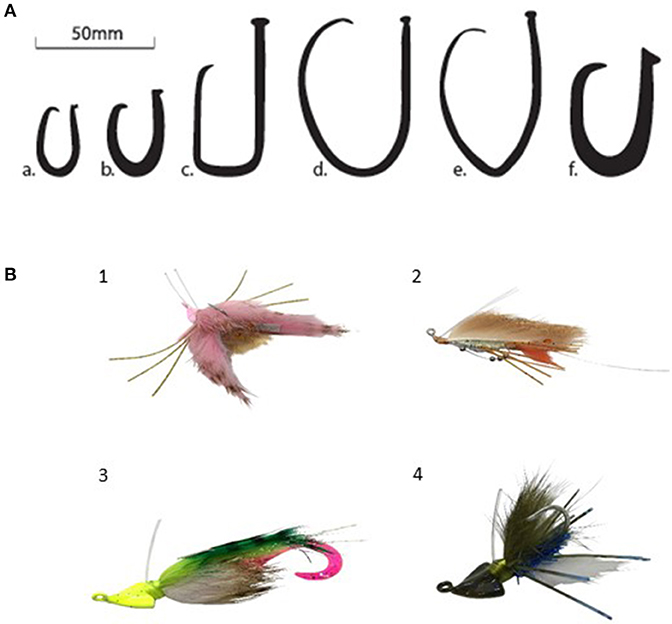
Figure 1. The rate at which fishes learn to avoid fishing techniques such as baits and lures probably reflects both the proportion of the stock that is likely to interact with a fisher and the degree of escapement from the technique. Evidence for this idea is shown by the history of technological innovations in fisheries for species such as bonefishes (Albula spp., family Albulidae) across the tropical Pacific (Allen, 2014). The line drawings (A) (from photographs in Allen, 2014) show bonefish hooks from indigenous fisheries in the Cook Islands. These include hooks manufactured from pearl shell between thirteenth and sixteenth centuries AD (a,b) and recovered from excavations of rock shelters at Aitutaki Island, southern Cook Islands, metal bonefish hooks collected at Aitutaki Island in early twentieth century (c) and in the same location in the late twentieth century (d, e) and a shell bonefish hook (f) collected in early twentieth century in the northern Cook Islands. Note the consistency of the form of these hooks through many centuries of time. Today, many of these now depleted stocks of bonefishes (Adams et al., 2014) are major targets for catch-and-release recreational fisheries. In contrast to earlier subsistence fisheries, the recreational fishery uses many different types of lures, mimicking both fish and invertebrates, made from a wide range of materials including feathers, plastics and metals, with new designs evolving continually. (B) Photographs of lures for bonefish that mimic crabs (1 and 4) shrimp (2 and baitfishes 3) in modern-day recreational fisheries.
This reversal of size-structured trophic interactions does not exist across all fisheries. In the case of techniques such as nets, humans can share the same size relationship with non-human predators, because fishing gear can be many times larger than the prey they seek to capture, particularly where forage fishes (typically small, filter-feeding fishes) are the target. Fishing using nets exploits the same behavioral response of schooling developed by fishes to deal with non-human predators in order to capture target species. Because the monofilament lines from which some nets are made are largely invisible to fish, the technique still subverts the ability of fishes to recognize humans (nets) as a predator and severely limits opportunities to evolve a learned response.
Predator Recognition
In addition to size, many other visual cues can also be used by prey to identify non-human predators. For example, the position and size of the mouth and eyes in relation to the head provide a good gauge of trophic role and feeding strategies of other fishes and might indicate the level of predatory threat that they pose. As piscivorous predators require binocular vision for precision striking, their eyes are forward-facing, whereas herbivores will tend to have laterally-placed eyes, allowing for broader-range monocular vision used for vigilance and predator detection. In addition to general rules-of-thumb relating to size and morphology, prey can also learn to recognize individual species of predators and use the characteristic morphology and behavior of one predator to make educated guesses as to the risk posed by unknown, but similar-looking fishes (Ferrari et al., 2007; Mitchell et al., 2013). Such generalization of predator recognition is likely common in many predator–prey systems.
Although visual cues are accurate in space and time, they are of limited value under low-light conditions or in complex habitats. For this reason, many aquatic species rely on chemical information to detect and avoid predators. The chemicals that make up the smell of a predator are species-specific and allow accurate detection of predators by the prey, as long as the smell of the predator is diffused widely across the prey's surroundings. Most aquatic species also show an innate avoidance of the smell of damaged conspecifics. These “alarm cues” are known to mediate a wide suite of overt antipredator responses that decrease the likelihood of prey being captured by predators, such as decreased foraging and activity and increased shelter use (Ferrari et al., 2010). These cues also mediate learned recognition of predators and dangerous habitats (Brown et al., 2011). Exposure to a novel predator in conjunction with the alarm cues of conspecifics enables young fishes to label this novel species as risky (Ferrari et al., 2010). Similarly, young fish exposed to new habitats in conjunction with alarm cues label the new habitat as one with high predator risk (Chivers and Smith, 1995). Both types of labeling can occur after a single exposure to the cues, making this one of the most efficient learning mechanisms described to date (Ferrari et al., 2010). Because alarm cues can also be detected in the feces of the predator, they can facilitate the labeling of predators long after an attack on conspecifics has occurred and can thus provide information regarding the home range of the predator (Dixson et al., 2012; Manassa and McCormick, 2012). Over longer time scales (weeks), risk cues not only elicit changes in prey behavior but also prey morphology and life-history traits that reduce the likelihood of predation. In the laboratory, damselfishes (Pomacentridae) exposed to the odor or sight of a predator in the presence of alarm cues over a 6-week period developed deeper bodies, smaller eyes and larger false eye spots on the posterior part of the dorsal fins than individuals that were not exposed to this combination of cues. When transferred to the field, fish with morphologies modified by the threat of predation had 50% higher survivorship (Lönnstedt et al., 2013).
Although visual and chemical cues are thought to be central to predator recognition by fishes, we know little about the use of other sensory systems such as hearing and electroreception. Fish can be trained with alarm cues to recognize particular noises (Wisenden et al., 2008), so it appears possible that sound may also play an important role in predator detection and recognition. Evidence for this phenomenon is provided by fishes that appear to be sensitive to the echolocation signals of cetaceans, so that noises produced during spawning choruses are suppressed while these predators are nearby (Luczkovich et al., 2000) and fishes that initiate escape behaviors in response to the sonar clicks of toothed whales (Wilson et al., 2011).
Despite the many ways in which prey fish have evolved to accurately detect and recognize predatory threats, humans are capable of bypassing all of these defense mechanisms under certain conditions. When humans behave like a typical predator, as occurs during spear fishing, fish show a great ability to gauge and respond to our presence in a threat-sensitive manner (Gotanda et al., 2009; Feary et al., 2011; Januchowski-Hartley et al., 2011, 2015; Côté et al., 2014; Tran et al., 2016). This occurs because spearfishing offers prey visual, chemical, auditory, and mechanical cues by which to assess risk. However, for the most part, fishing is a remote process where humans are not physically present, removing the ability of fish to label us as risky. Indirect cues, such as boat sound are available, but humans have also managed to decouple these cues from danger through the use of passive fishing techniques. Nets, hooks and traps can be deployed and left unattended, so that all cues indicating our predatory intent disappear. This ability is exclusive to humans; even the most cryptic of predators will still be detectable by prey via other sensory cues. Active fishing such as the trawling of nets offers a fish a noise cue of the vessel and net that can be used for avoidance of entrapment, but even with this cue, the effectiveness of any escape response can be very limited (Suuronen et al., 1997). Reactions to vessel noise are often inconsistent (De Robertis and Handegard, 2012), may only occur when fish are harvested in shallow water, can cause fish to be driven into, rather than away from nets and can be compensated for by altering fishing techniques (Ona and Godø, 1990; Mitson and Knudsen, 2003). Some mesopelagic fishes appear to be able to effectively avoid towed nets, but in this case the cues for escapement are thought to be visual rather than acoustic (Kaartvedt et al., 2012). Additionally, some fishing techniques exploit behavioral aspects of the prey species that are not involved in the context of predation. For example, many fish traps preferentially collect species that use confined spaces such as holes and caves as resting or shelter sites (Rakitin and Kramer, 1996).
Why is fishing gear usually not labeled as threatening by fish? Often motion is necessary for visual recognition of threats (Wisenden and Harter, 2001) making passive gear such as stationary nets, hook, and lines or traps difficult to label. Monofilament nets are virtually invisible to fishes. Fishing gear does not smell unless it is baited, in which case the odor is an attractant, and most passive techniques do not make noise. The absence of visual, chemical and auditory cues that would arouse prey to the threat of predation by humans thus makes labeling a very difficult task. Effectively, in the context of fish sensory awareness and predator recognition, humans are often an invisible predator.
Learning About Predators
Although it makes intuitive sense for prey in marine systems to be wary of fishes bigger than themselves, the costs associated with predator avoidance need to be carefully balanced with the benefits received from other fitness-promoting activities such as foraging, mating, and territory defense (Ydenberg and Dill, 1986). Based on their experiences, prey need to carefully select habitats that will allow them to optimize this trade-off and quickly learn to discriminate predators from non-predators. This balance is thought to be the principal determinant of patterns of habitat selection by fishes and the key driver of changes in habitat occupancy during ontogeny (Werner and Hall, 1988; Walters and Juanes, 1993; Dahlgren and Eggleston, 2000).
Learning about predators can occur directly, for instance, when a fish is attacked and survives the encounter. While a very reliable mode of learning, there are obvious costs associated with closely interacting with a predator. As discussed above, the simultaneous detection of conspecific alarm cues and a novel predator cue (sight, smell, or sound) leads to the immediate association between risk and the predator. Cues from closely-related species or prey guild members can also be used to mediate learning (Mitchell et al., 2012). More remarkable is the fact that naïve fish can observe the fright response of knowledgeable individuals responding to a predator cue and subsequently learn the association between the cue and the level of threat (Ferrari et al., 2005). Such social learning enables rapid transmission of information through the population. Learning of predators, either via alarm cues or social learning has been shown to dramatically increase survival in young fishes (Manassa and McCormick, 2013).
For learning to be possible, prey need to associate risk with a recognizable cue. The simple fact that many cues associated with fishing are unavailable means that learning is not an effective solution for prey. There are, however, situations when the cues are available and learning occurs. For example, intense catch-and-release fishing using lures quickly leads to a steep decrease in the number of strikes, as fish learn to avoid flies and spinners (Beukemaj, 1970; Askey et al., 2006; Lennox et al., 2015). Indeed, many of the examples of behavioral changes in fishes in response to fishing, such as a reduction in boldness, foraging or activity involve angling, which has a high opportunity for escapement (see reviews by Arlinghaus et al., 2017; Diaz Pauli and Sih, 2017). As these examples show, for learning to occur, the individual needs to survive the predatory encounter. In a catch-and-release context, learning is possible and the first-hand acquisition of information about the lure or bait could even be transmitted through the population very quickly via experienced individuals (Young and Hayes, 2004). In the absence of experienced individuals (i.e., if all captured fishes are removed from the population), learning cannot occur. Consequently, the efficiency of fishing will depend in part on the number of experienced individuals that survive and pass information to others. Commercial fishing gear has evolved to be very efficient, leaving little-to-no chance of escape. This keeps learning to a minimum. If humans used gear that was clearly visible and allowed a low level of escape, there would likely be fast and efficient learning among any social species.
Evidence for this is shown in situations such as coral reefs subject to subsistence fishing, an activity that generally relies on little technology, thus is likely to be less efficient. In these systems the role of traditional cycles of spatial closures can be to increase the naivety of fish to fishing, making them more susceptible to capture when the area is once again open (Cinner et al., 2006; Cohen and Foale, 2013; Goetze et al., 2017). Analogs of this process of learning and social transmission are shown in many terrestrial habitats, notably when humans use trapping to eradicate pest species. Typically, the decision to deploy traps depends on the frequency of escape, so that where there are likely to be high numbers of escapees, trapping may be ineffective as the target species quickly becomes wary of the trap and efficacy of trapping rapidly declines (King et al., 2009; Parkes and Panetta, 2009; Zabala et al., 2010). Notably, it is the older, experienced individuals that are most likely to become “trap shy” (King et al., 2009).
Why the Super-predator has Super-powers
The relationship between gape width and body size and the ability of prey to recognize and rapidly learn about predator threats forces non-human predators to focus on smaller and naïve (juvenile) prey in the ocean. Conversely, this provides very strong selective pressure for young fish to grow fast in order to escape gape-limited predators both in space and time, and thus to select habitats where predation risk can be balanced with foraging opportunities. As fish increase in size and gain the ability to deal with different types of food items, some habitats may no longer support these growth rates (Werner and Hall, 1988; Dahlgren and Eggleston, 2000). This forces fish to shift between habitats and to enter novel environments, offering predators a major advantage because they may encounter naïve prey. Although the processes of predator recognition and learning will occur very swiftly, this explains why such transitions between habitats (larval-juvenile, juvenile-adult) are often major mortality bottlenecks for most species (Doherty et al., 2004; Almany and Webster, 2006) and why survivorship at these times is typically very strongly linked to growth rates (Bergenius et al., 2002; Vigliola and Meekan, 2002; Wilson and Meekan, 2002; McCormick and Hoey, 2004; Raventós and MacPherson, 2005; Gagliano et al., 2007). These vulnerable transition periods can also occur later in life, for example when sex-changing fishes switch between sexes and undertake new social roles that expose them to a new or wider range of predators (Clifton and Robertson, 1993).
Humans have subverted this process of habitat selection through technological developments such as sonar, which removes any possibility that fish can occupy habitats where they cannot be detected and fishing techniques that allow access to all habitat types (deep sea, open oceans, coasts, and estuaries). This negates the key driver of habitat selection as a balance between foraging and predation risk for prey fishes (Werner and Hall, 1988). Once acquainted with a new habitat, prey fishes will have identified and cataloged the suite of non-human predators likely to pose a threat and are capable of generalizing this experience to include any novel predators that may be encountered (Mitchell et al., 2013). Because humans can disguise predation as prey, are effectively invisible and the capture technologies they use are highly efficient and can be indiscriminate, these evolved responses of fishes to predators are inappropriate and easily overwhelmed. Consequently, all sizes of fish prey are available for human exploitation, with adults providing the greatest return in terms of harvest for the cost of capture. Industrial fishing typically focuses on times and places where adult fishes gather together (spawning and feeding aggregations and optimal habitats) so that the costs of capture can be further minimized (Claydon, 2004). Apex predators such as sharks, billfishes, tunas and cetaceans may also focus on these aggregations, but their catch rates in marine ecosystems are limited both by the relative rarity of these top-order predators and by the mechanics of capture that typically involve swallowing no more than a few individuals at a time.
The imposition of size limits on fisheries may, in some cases, compensate for the efficiency of gear by offering learning opportunities for targeted species. Size-selectivity can result in the release and survival of small (undersize) fishes in populations, which typically occurs in many fisheries that rely on passive techniques, notably angling. Similar to any catch-and-release fishery, this can provide an opportunity for learning and thus selection and there are numerous examples of this process influencing the behavior (e.g., boldness, foraging) and distribution of target species (Olsen et al., 2012; Klefoth et al., 2013, 2017; Alós et al., 2014; Härkönen et al., 2014; Arlinghaus et al., 2017). However, in many commercial fisheries, this opportunity for learning and thus eventual adaptation could be reduced through the behavior of fishers, who actively seek to avoid capturing size classes (typically smaller cohorts) that they cannot retain, since bringing these on board the vessel reduces efficiency of the fishing effort. This is usually done in two ways, either mechanically (e.g., mesh size in nets, hook size in longlines Kennelly and Broadhurst, 2002; Minsund et al., 2002; Lennox et al., 2017) or through selection of habitats that are fished (Dunn et al., 2011). Avoidance of small size classes through the use of gear means that there might be no negative consequences for fishes that, for example, escape through the mesh of a trawl or gill net or feed on baits attached to hooks that are too large to capture them. Indeed, in the latter case, feeding on the bait could be a positive reinforcement for susceptibility that does not have any consequences until fish attain a size large enough to ingest the hook. Similarly, the entry of undersize crabs, lobsters and fishes into traps to feed on baits provides the same form of behavioral reinforcement if they are able to exit the trap or are returned to the water unharmed. Studies have shown that more than 90% of American lobsters (Homarus americanus) exit traps before they are recovered (Jury et al., 2001), and similar rates of trap exit also occur for some fishes (Bacheler et al., 2013). Food within traps is a very significant source of nourishment for juvenile (undersize) lobsters that increases their growth and ultimately the yield and economic value of these fisheries off the east coast of North America (Grabowski et al., 2010). Fishermen can avoid, or are often prohibited from fishing in, habitats where juveniles are abundant (e.g., nursery grounds Dunn et al., 2011) and many fisheries target times and places such as spawning aggregations where only adults are present. If small size classes are taken as by-catch in active fisheries such as trawling, they are often subjected to very high rates of mortality once discarded (Benoît et al., 2012). These scenarios may counter-act opportunities for small size classes to learn about the threat fishing poses to later life history stages; some could actively increase susceptibility. It is true however, that upper size limits may provide an opportunity for learning, but only if the fish that attain these larger sizes have passed through the gauntlet of fishing to enter the size refuge. This will not occur if these fish have managed to survive through either serendipity (never encountering fishing gear) or some form of spatial/temporal refuge from fishing (habitat, management zones). In any event, upper size limits on fisheries are relatively rare compared to lower limits that seek to allow some proportion of the stock to reproduce before being harvested (Sissenwine and Shepherd, 1987). Active forms of fishing (trawling, netting) offer little opportunity for this type of management and it is mostly a feature of passive approaches such as angling and trap fisheries. Furthermore, traditional management strategies for trawl and net fisheries target the removal of older, larger individuals in order to increase the productivity of a stock by reducing competition for resources and cannibalism on smaller size classes (Garcia et al., 2012).
Humans are the only predator in the ocean that has developed the ability to subvert every step of the evolved sequence of prey responses to predation threats (Figure 2). Many non-human predators use camouflage or mimicry to reduce the chances of predator recognition and ambush prey (Randall, 2005). This may even be combined with specialized morphologies that mimic food in order to draw potential prey within striking range, as occurs in anglerfishes (Chadwick, 1929; Pietsch and Grobecker, 1978). Additionally, some non-human predators have evolved highly sophisticated sensory systems that can use acoustic and vibratory (e.g., marine mammals Dehnhardt et al., 2001; Berta et al., 2005), electrosensory and olfactory (e.g., sharks Hueter et al., 2004; Gardiner et al., 2012) cues to maximize their chances of detecting prey. But no single species, other than humans, has the ability to circumvent all of the evolved antipredator strategies of fishes. The long history of collapses of a multitude of commercial fisheries due to over-harvesting throughout the world's oceans show that humans can do so with great effect (Christensen et al., 2003).
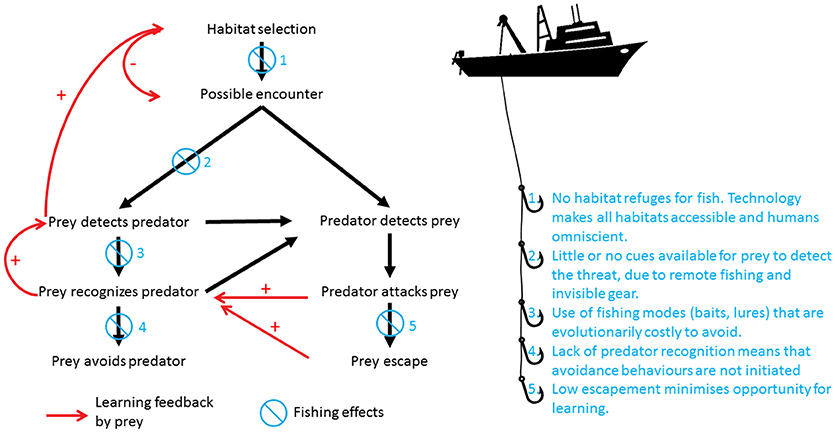
Figure 2. Unlike any other predator in marine systems, humans have the ability to influence the outcome of the entire predation sequence. This Figure summarizes how this occurs. Initially, prey choose habitats that provide a balance between risk of predation and opportunities to access resources (food, mates, shelter). If a predator and a prey are within their limits of sensory detection, the prey can detect the predator first, or alternatively, the predator can detect the prey without it being aware of it. In the case where the threat is detected visually, chemically, or by a combination of both cues, the prey has the opportunity to avoid the threat. In the alternate case where the predator detects the prey and the predator attacks, if the prey escapes it has the opportunity to learn. Moreover, those prey that witnessed the attack but were not directly subjected to it also have the opportunity to recognize the threat posed by the predator through social learning. This learning initiates a feedback loop, in which prey become better equipped to detect, recognize, and avoid predator threats in the future (red arrows in Figure). Ultimately, this might influence their choice of habitat, so that they select those habitats with lower chances of predator encounters. Fishing by humans disrupts the outcomes of every step of this predation sequence. Due to technology (sonar, satellite communications, deep water trawling, long-lining etc.), there is no habitat in which prey can seek refuge from humans as predators (1). Humans fish using remote methods, where there are little or no cues on which prey can focus in order to reduce encounter rates. Additionally, passive fishing gear is effectively invisible to the fish (monofilament lines and nets), and is generally odorless (2). When detection is possible (lures, baits, traps), the cues used for capture are evolutionarily costly to avoid, because they promote low-cost foraging or refuge, two highly selected traits for fitness (3). Even when recognized, the response to the threat may be co-opted by the fisher to increase catches, as is the case for purse-seining, trawling and fish drives (4). All fishing gears are highly efficient and have become more so over time, so that opportunities for escapement and learning are minimized (5). In contrast, evolution has forced non-human predators to focus on only one or two steps of this process using strategies such as crypsis, swimming performance, and enhanced sensory systems.
Conclusions
Humans are unique because unlike non-human predators, we have the ability to target adult life history stages of fishes, rather than juveniles (Darimont et al., 2015). This is possible because our fishing techniques circumvent and even directly counteract the evolutionary constraints that allow fish as prey to detect, recognize, and learn about non-human predators. This results in high efficiency, slowing the evolution of appropriate behavioral repertoires to avoid fishing by humans, despite the millennia-long use of very similar techniques. Populations of fishes can, and do, show behavioral adaptations to fishing, but only under restricted circumstances. The best evidence for this process comes from angling and recreational fisheries, often in small bodies of water such as rivers or lakes where catch and release techniques or high rates of escapement offer fish the opportunity to learn about humans as predators. Resulting behavioral traits are more likely to spread because all habitats are accessible to fishing and species have relatively small effective population sizes. In marine systems, the evidence for behavioral adaptions to active techniques such as trawling is limited and comes mostly from stocks of demersal fishes that have been driven to very low levels of abundance (often commercial extinction; for review see Diaz Pauli and Sih, 2017). It is also notable that many of these fisheries operate in situations where humans have also extirpated or severely reduced the abundance of non-human predators (e.g., marine mammals) that compete for the same prey. The combination of these factors will reduce selective pressures that might counter-act the evolution of behavioral adaptations to fishing and will facilitate the spread of these behavioral traits due to severe restrictions in population size. Over-fished populations are also less likely to host spatial or temporal refuges from fishing where naïve individuals might still contribute to future generations.
Because our methods of harvest act in direct opposition to the selective pressures imposed by non-human predators, our current rates of exploitation of fisheries (up to 400% greater than non-human sources) shift the morphology and life histories of fishes toward traits that are likely to make them more susceptible to natural sources of predation mortality. This is critical because it has the potential to reduce the resilience of fished populations and to delay the recovery of over-exploited stocks (Enberg et al., 2009) once harvesting has ceased. The role of non-human predators in delaying stock recovery is contentious, although we argue that it is unlikely to be coincidental that some of the best evidence for this process comes from demersal fisheries that display both the traits of over-fishing and reduced numbers of non-human predators mentioned above. For example, recovering populations of seal predators are argued to have significantly retarded the rebound of over-fished demersal stocks (see Yodzis, 2001; Trzcinski et al., 2006). Recent evidence from over-fished cod (Gadus morhua) stocks in the west of Scotland suggest that predation by gray seals (Halichoerus grypus) significantly increased once fishing ceased and that this now inhibits stock recovery (Cook et al., 2015). This study notes that as the size of the stock declined due to overfishing, the proportion eaten by seals increased, a pattern consistent with the idea that the spread of traits associated with the avoidance of fishing could have enhanced vulnerability of cod to these predators through time.
An understanding of the means by which humans subvert the predator defenses of fishes offers a new perspective for fisheries management. Given the multiple anthropogenic threats now faced by the world's fisheries (climate change, acidification, increasing demand for fish as a source of protein) it is imperative that we explore these options. All fishes have the ability to recognize and learn about predators, given the opportunity. By co-opting, rather than disrupting this behavior, humans may be able to achieve greater sustainability and resilience of fish stocks—a better outcome for both the species that are the targets of fisheries and the billions of people that depend on fishes as a food source.
Author Contributions
Theme conceived by MM and MF. All authors contributed to the writing and revisions.
Conflict of Interest Statement
The authors declare that the research was conducted in the absence of any commercial or financial relationships that could be construed as a potential conflict of interest.
Acknowledgments
We acknowledge financial support of the Australian Institute of Marine Science, James Cook University and the University of Saskatchewan.
References
Adams, A. J., Horodysky, A. Z., Mcbride, R. S., Guindon, K., Shenker, J., Macdonald, T. C., et al. (2014). Global conservation status and research needs for tarpons (Megalopidae), ladyfishes (Elopidae) and bonefishes (Albulidae). Fish Fish. 15, 280–311. doi: 10.1111/faf.12017
Allen, M. S. (2014). The historical role of bonefishes (Albula spp.) in Polynesian fisheries. Hawaiian Archaeol. 2014, 51–72.
Almany, G. R., and Webster, M. S. (2006). The predation gauntlet: early post-settlement mortality in reef fishes. Coral Reefs 25, 19–22. doi: 10.1007/s00338-005-0044-y
Alós, J., Palmer, M., Trías, P., Díaz-Gil, C., and Arlinghaus, R. (2014). Recreational angling intensity correlates with alteration of vulnerability to fishing in a carnivorous coastal fish species. Can. J. Fish. Aquat. Sci. 72, 217–225. doi: 10.1139/cjfas-2014-0183
Arlinghaus, R., Laskowski, K. L., Alós, J., Klefoth, T., Monk, C. T., Nakayama, S., et al. (2017). Passive gear-induced timidity syndrome in wild fish populations and its potential ecological and managerial implications. Fish Fish. 18, 360–373. doi: 10.1111/faf.12176
Askey, P. J., Richards, S. A., Post, J. R., and Parkinson, E. A. (2006). Linking angling catch rates and fish learning under catch-and-release regulations. N. Am. J. Fish. Manage. 26, 1020–1029. doi: 10.1577/M06-035.1
Bacheler, N. M., Schobernd, Z. H., Berrane, D. J., Schobernd, C. M., Mitchell, W. A., and Geraldi, N. R. (2013). When a trap is not a trap: converging entry and exit rates and their effect on trap saturation of black sea bass (Centropristis striata). ICES J. Mar. Sci. 70, 873–882. doi: 10.1093/icesjms/fst062
Bellwood, D. R., Goatley, C. H., Bellwood, O., Delbarre, D. J., and Friedman, M. (2015). The rise of jaw protrusion in spiny-rayed fishes closes the gap on elusive prey. Curr. Biol. 25, 2696–2700. doi: 10.1016/j.cub.2015.08.058
Benoît, H. P., Plante, S., Kroiz, M., and Hurlbut, T. (2012). A comparative analysis of marine fish species susceptibilities to discard mortality: effects of environmental factors, individual traits, and phylogeny. ICES J. Mar. Sci. 70, 99–113. doi: 10.1093/icesjms/fss132
Bergenius, M. A., Meekan, M. G., Robertson, R. D., and Mccormick, M. I. (2002). Larval growth predicts the recruitment success of a coral reef fish. Oecologia 131, 521–525. doi: 10.1007/s00442-002-0918-4
Bersier, L.-F., and Kehrli, P. (2008). The signature of phylogenetic constraints on food-web structure. Ecol. Complex. 5, 132–139. doi: 10.1016/j.ecocom.2007.06.013
Berta, A., Sumich, J. L., and Kovacs, K. M. (2005). Marine Mammals: Evolutionary Biology. London: Academic Press.
Beukemaj, J. (1970). Acquired hook-avoidance in the pike Esox lucius L. fished with artificial and natural baits. J. Fish Biol. 2, 155–160. doi: 10.1111/j.1095-8649.1970.tb03268.x
Breck, J. E., and Gitter, M. J. (1983). Effect of fish size on the reactive distance of bluegill (Lepomis macrochirus) sunfish. Can. J. Fish. Aquat. Sci. 40, 162–167. doi: 10.1139/f83-026
Brose, U., Jonsson, T., Berlow, E. L., Warren, P., Banasek-Richter, C., Bersier, L. F., et al. (2006). Consumer-resource body-size relationships in natural food webs. Ecology 87, 2411–2417. doi: 10.1890/0012-9658(2006)87[2411:CBRINF]2.0.CO;2
Brown, G. E., Ferrari, M. C., and Chivers, D. P. (2011). “Learning about danger: chemical alarm cues and threat-sensitive assessment of predation risk by fishes,” in Fish Cognition and Behavior, 2nd Edn, eds C. Brown, L. Kevin, and K. Jens (Oxford: Blackwell Publishing), 59–80.
Côté, I. M., Darling, E. S., Malpica-Cruz, L., Smith, N. S., Green, S. J., Curtis-Quick, J., et al. (2014). What doesn't kill you makes you wary? effect of repeated culling on the behaviour of an invasive predator. PLoS ONE 9:e94248. doi: 10.1371/journal.pone.0094248
Chadwick, H. (1929). Feeding habits of the angler-fish, Lophius piscatorius. Nature 124:337. doi: 10.1038/124337a0
Chivers, D. P., and Smith, R. J. F. (1995). Fathead minnows (Pimephales promelas) learn to recognize chemical stimuli from high-risk habitats by the presence of alarm substance. Behav. Ecol. 6, 155–158. doi: 10.1093/beheco/6.2.155
Chopra, I., and Roberts, M. (2001). Tetracycline antibiotics: mode of action, applications, molecular biology, and epidemiology of bacterial resistance. Microbiol. Mol. Biol. Rev. 65, 232–260. doi: 10.1128/MMBR.65.2.232-260.2001
Christensen, V., Guenette, S., Heymans, J. J., Walters, C. J., Watson, R., Zeller, D., et al. (2003). Hundred-year decline of North Atlantic predatory fishes. Fish Fish. 4, 1–24. doi: 10.1046/j.1467-2979.2003.00103.x
Cinner, J. (2007). Designing marine reserves to reflect local socioeconomic conditions: lessons from long-enduring customary management systems. Coral Reefs 26, 1035–1045. doi: 10.1007/s00338-007-0213-2
Cinner, J., Marnane, M. J., Mcclanahan, T. R., and Almany, G. R. (2006). Periodic closures as adaptive coral reef management in the Indo-Pacific. Ecol. Soc. 11:31. Available online at: http://www.ecologyandsociety.org/vol11/iss1/art31/
Claydon, J. (2004). Spawning aggregations of coral reef fishes: characteristics, hypotheses, threats and management. Oceanogr. Mar. Biol. 42, 265–302. doi: 10.1201/9780203507810.ch7
Clifton, K. E., and Robertson, D. R. (1993). Risks of alternative mating tactics. Nature 366, 520–520. doi: 10.1038/366520b0
Cohen, P. J., and Foale, S. J. (2013). Sustaining small-scale fisheries with periodically harvested marine reserves. Mar. Policy. 37, 278–287. doi: 10.1016/j.marpol.2012.05.010
Conover, D. O., Munch, S. B., and Arnott, S. A. (2009). Reversal of evolutionary downsizing caused by selective harvest of large fish. Proc. B Biol. Sci. 276, 2015–2020. doi: 10.1098/rspb.2009.0003r
Cook, R. M., Holmes, S. J., and Fryer, R. J. (2015). Grey seal predation impairs recovery of an over-exploited fish stock. J. Appl. Ecol. 52, 969–979. doi: 10.1111/1365-2664.12439
Dahlgren, C. P., and Eggleston, D. B. (2000). Ecological processes underlying ontogenetic habitat shifts in a coral reef fish. Ecology 81, 2227–2240. doi: 10.1890/0012-9658(2000)081[2227:EPUOHS]2.0.CO;2
Darimont, C. T., Fox, C. H., Bryan, H. M., and Reimchen, T. E. (2015). The unique ecology of human predators. Science 349, 858–860. doi: 10.1126/science.aac4249
De Robertis, A., and Handegard, N. O. (2012). Fish avoidance of research vessels and the efficacy of noise-reduced vessels: a review. ICES J. Mar. Sci. 70, 34–45. doi: 10.1093/icesjms/fss155
Dehnhardt, G., Mauck, B., Hanke, W., and Bleckmann, H. (2001). Hydrodynamic trail-following in harbor seals (Phoca vitulina). Science 293, 102–104. doi: 10.1126/science.1060514
Diaz Pauli, B., and Sih, A. (2017). Behavioural responses to human-induced change: why fishing should not be ignored. Evol. Appl. 10, 231–240. doi: 10.1111/eva.12456
Dixson, D. L., Pratchett, M. S., and Munday, P. L. (2012). Reef fishes innately distinguish predators based on olfactory cues associated with recent prey items rather than individual species. Anim. Behav. 84, 45–51. doi: 10.1016/j.anbehav.2012.04.001
Doherty, P., Dufour, V., Galzin, R., Hixon, M., Meekan, M., and Planes, S. (2004). High mortality during settlement is a population bottleneck for a tropical surgeonfish. Ecology 85, 2422–2428. doi: 10.1890/04-0366
Dudley, S. F., Anderson-Reade, M. D., Thompson, G. S., and McMullen, P. B. (2000). Concurrent scavenging off a whale carcass by great white sharks, Carcharodon carcharias, and tiger sharks, Galeocerdo cuvier. Fish. Bull. 98, 646–649.
Dunn, D. C., Boustany, A. M., and Halpin, P. N. (2011). Spatio-temporal management of fisheries to reduce by-catch and increase fishing selectivity. Fish Fish. 12, 110–119. doi: 10.1111/j.1467-2979.2010.00388.x
Enberg, K., Jørgensen, C., Dunlop, E. S., Heino, M., and Dieckmann, U. (2009). Implications of fisheries-induced evolution for stock rebuilding and recovery. Evol. Appl. 2, 394–414. doi: 10.1111/j.1752-4571.2009.00077.x
Feary, D. A., Cinner, J. E., Graham, N. A., and Januchowski-Hartley, F. A. (2011). Effects of customary marine closures on fish behavior, spear-fishing success, and underwater visual surveys. Conser. Biol. 25, 341–349. doi: 10.1111/j.1523-1739.2010.01613.x
Ferrari, M. C., Gonzalo, A., Messier, F., and Chivers, D. P. (2007). Generalization of learned predator recognition: an experimental test and framework for future studies. Proc. B Biol. Sci. 274, 1853–1859. doi: 10.1098/rspb.2007.0297
Ferrari, M. C., Trowell, J. J., Brown, G. E., and Chivers, D. P. (2005). The role of learning in the development of threat-sensitive predator avoidance by fathead minnows. Anim. Behav. 70, 777–784. doi: 10.1016/j.anbehav.2005.01.009
Ferrari, M. C., Wisenden, B. D., and Chivers, D. P. (2010). Chemical ecology of predator-prey interactions in aquatic ecosystems: a review and prospectus. Can. J. Zool. 88, 698–724. doi: 10.1139/Z10-029
Finney, B. P., Gregory-Eaves, I., Sweetman, J., Douglas, M. S., and Smol, J. P. (2000). Impacts of climatic change and fishing on Pacific salmon abundance over the past 300 years. Science 290, 795–799. doi: 10.1126/science.290.5492.795
Gagliano, M., McCormick, M. I., and Meekan, M. G. (2007). Survival against the odds: ontogenetic changes in selective pressure mediate growth-mortality trade-offs in a marine fish. Proc. Biol. Sci. 274, 1575–1582. doi: 10.1098/rspb.2007.0242
Garcia, S. M., Kolding, J., Rice, J., Rochet, M. J., Zhou, S., Arimoto, T., et al. (2012). Reconsidering the consequences of selective fisheries. Science 335, 1045–1047. doi: 10.1126/science.1214594
Gardiner, J. M., Hueter, R. E., Maruska, K. P., Sisneros, J. A., Casper, B. M., Mann, D. A., et al. (2012). “Sensory physiology and behavior of elasmobranchs,” in Biology of Sharks and Their Relatives, 2nd Edn, eds J. C. Carrier, J. A. Musick, and M. R. Heithaus (Baton Roca, FL: CRC Press), 349–401.
Goetze, J. S., Januchowski-Hartley, F. A., Claudet, J., Langlois, T. J., Wilson, S. K., and Jupiter, S. D. (2017). Fish wariness is a more sensitive indicator to changes in fishing pressure than abundance, length or biomass. Ecol Appl. 27, 1178–1189. doi: 10.1002/eap.1511/full
Gotanda, K. M., Turgeon, K., and Kramer, D. L. (2009). Body size and reserve protection affect flight initiation distance in parrotfishes. Behav. Ecol. Sociobiol. 63, 1563–1572. doi: 10.1007/s00265-009-0750-5
Grabowski, J. H., Clesceri, E. J., Baukus, A. J., Gaudette, J., Weber, M., and Yund, P. O. (2010). Use of herring bait to farm lobsters in the gulf of maine. PLoS ONE 5:e10188. doi: 10.1371/journal.pone.0010188
Härkönen, L., Hyvärinen, P., Paappanen, J., and Vainikka, A. (2014). Explorative behavior increases vulnerability to angling in hatchery-reared brown trout (Salmo trutta). Can. J. Fish. Aquat. Sci. 71, 1900–1909. doi: 10.1139/cjfas-2014-0221
Hueter, R. E., Mann, D. A., Maruska, K. P., Sisneros, J. A., and Demski, L. S. (2004). “Sensory biology of elasmobranchs,” in Biology of Sharks and Their Relatives, eds J. C. Carrier, J. A. Musick, and M. R. Heithaus (Boca Raton, FL: CRC Press), 325–368.
Iyengar, E. V. (2008). Kleptoparasitic interactions throughout the animal kingdom and a re-evaluation, based on participant mobility, of the conditions promoting the evolution of kleptoparasitism. Biol. J. Linnean Soc. 93, 745–762. doi: 10.1111/j.1095-8312.2008.00954.x
Jachmann, H., Berry, P., and Imae, H. (1995). Tusklessness in African elephants: a future trend. Afr. J. Ecol. 33, 230–235. doi: 10.1111/j.1365-2028.1995.tb00800.x
Januchowski-Hartley, F. A., Graham, N. A., Cinner, J. E., and Russ, G. R. (2015). Local fishing influences coral reef fish behavior inside protected areas of the Indo-Pacific. Biol. Conserv.182, 8–12. doi: 10.1016/j.biocon.2014.11.024
Januchowski-Hartley, F. A., Graham, N. A., Feary, D. A., Morove, T., and Cinner, J. E. (2011). Fear of fishers: human predation explains behavioral changes in coral reef fishes. PLoS ONE 6:e22761. doi: 10.1371/journal.pone.0022761
Jenkins, S., Mullen, C., and Brand, A. (2004). Predator and scavenger aggregation to discarded by-catch from dredge fisheries: importance of damage level. J. Sea Res. 51, 69–76. doi: 10.1016/j.seares.2003.05.002
Johannes, R. E. (1978). Traditional marine conservation methods in Oceania and their demise. Annu. Rev. Ecol. Syst. 9, 349–364. doi: 10.1146/annurev.es.09.110178.002025
Johannes, R. E. (2002). The renaissance of community-based marine resource management in Oceania. Annu. Rev. Ecol. Syst. 33, 317–340. doi: 10.1146/annurev.ecolsys.33.010802.150524
Jørgensen, C., Enberg, K., Dunlop, E. S., Arlinghaus, R., Boukal, D. S., Brander, K., et al. (2007). Ecology-managing evolving fish stocks. Science 318, 1247–1248. doi: 10.1126/science.1148089
Jury, S. H., Howell, H., O'grady, D. F., and Watson Iii, W. H. (2001). Lobster trap video: in situ video surveillance of the behaviour of Homarus americanus in and around traps. Mar. Freshw. Res. 52, 1125–1132. doi: 10.1071/MF01096
Kaartvedt, S., Staby, A., and Aksnes, D. L. (2012). Efficient trawl avoidance by mesopelagic fishes causes large underestimation of their biomass. Mar. Ecol. Prog. Ser. 456, 1–6. doi: 10.3354/meps09785
Kennelly, S. J., and Broadhurst, M. K. (2002). By-catch begone: changes in the philosophy of fishing technology. Fish Fish. 3, 340–355. doi: 10.1046/j.1467-2979.2002.00090.x
King, C. M., Mcdonald, R. M., Martin, R. D., and Dennis, T. (2009). Why is eradication of invasive mustelids so difficult? Biol. Conserv. 142, 806–816. doi: 10.1016/j.biocon.2008.12.010
Klefoth, T., Pieterek, T., and Arlinghaus, R. (2013). Impacts of domestication on angling vulnerability of common carp, Cyprinus carpio: the role of learning, foraging behaviour and food preferences. Fish. Manage. Ecol. 20, 174–186. doi: 10.1111/j.1365-2400.2012.00865.x
Klefoth, T., Skov, C., Kuparinen, A., and Arlinghaus, R. (2017). Toward a mechanistic understanding of vulnerability to hook-and-line fishing: boldness as the basic target of angling-induced selection. Evol. Appl. 10, 994–1006. doi: 10.1111/eva.12504
Lennox, R. J., Alós, J., Arlinghaus, R., Horodysky, A., Klefoth, T., Monk, C. T., et al. (2017). What makes fish vulnerable to capture by hooks? A conceptual framework and a review of key determinants. Fish Fish. 18, 986–1010. doi: 10.1111/faf.12219
Lennox, R. J., Diserud, O. H., Cooke, S. J., Thorstad, E. B., Whoriskey, F. G., Solem, Ø., et al. (2015). Influence of gear switching on recapture of Atlantic salmon (Salmo salar) in catch and release fisheries. Ecol. Freshw. Fish 25, 422–428. doi: 10.1111/eff.12223
Li, K. T., Wetterer, J. K., and Hairston, N. G. Jr. (1985). Fish size, visual resolution, and prey selectivity. Ecology 66 1729–1735. doi: 10.2307/2937368
Lönnstedt, O. M., Mccormick, M. I., and Chivers, D. P. (2013). Predator-induced changes in the growth of eyes and false eyespots. Sci. Rep. 3:2259. doi: 10.1038/srep02259
Lopes, M. S., Bertucci, T. C., Rapagn,ã, L., Tubino Rde, A., Monteiro-Neto, C., Tomas, A. R. G., et al. (2016). The path towards endangered species: prehistoric fisheries in Southeastern Brazil. PLoS ONE 11:e0154476. doi: 10.1371/journal.pone.0154476
Luczkovich, J. J., Daniel, H. J., Hutchinson, M., Jenkins, T., Johnson, S. E., Pullinger, R. C., et al. (2000). Sounds of sex and death in the sea: bottlenose dolphin whistles suppress mating choruses of silver perch. Bioacoustics 10, 323–334. doi: 10.1080/09524622.2000.9753441
Manassa, R. P., and McCormick, M. I. (2012). Social learning and acquired recognition of a predator by a marine fish. Anim. Cogn. 15, 559–565. doi: 10.1007/s10071-012-0484-z
Manassa, R. P., and McCormick, M. I. (2013). Social learning improves survivorship at a life-history transition. Oecologia 171, 845–852. doi: 10.1007/s00442-012-2458-x
McCormick, M. I., and Hoey, A. S. (2004). Larval growth history determines juvenile growth and survival in a tropical marine fish. Oikos 106, 225–242. doi: 10.1111/j.0030-1299.2004.13131.x
Mertz, G., and Myers, R. (1998). A simplified formulation for fish production. Can. J. Fish. Aquat. Sci. 55, 478–484. doi: 10.1139/f97-216
Minsund, O., Kolding, J., and Fréon, P. (2002). “Fish capture devices in industrial and artisanal fisheries and their influence on management,” Handbook of Fish Biology and Fisheries, Vol. 2, eds P. J. B. Hart and J. D. Reynolds (Oxford: Blackwell Science), 13–36.
Mitchell, M. D., Cowman, P. F., and McCormick, M. I. (2012). Chemical alarm cues are conserved within the coral reef fish family Pomacentridae. PLoS ONE 7:e47428. doi: 10.1371/journal.pone.0047428
Mitchell, M. D., Mccormick, M. I., Chivers, D. P., and Ferrari, M. C. (2013). Generalization of learned predator recognition in coral reef ecosystems: how cautious are damselfish? Funct. Ecol. 27, 299–304. doi: 10.1111/1365-2435.12043
Mitson, R. B., and Knudsen, H. P. (2003). Causes and effects of underwater noise on fish abundance estimation. Aquat. Living Resourc. 16, 255–263. doi: 10.1016/S0990-7440(03)00021-4
Naisbit, R. E., Kehrli, P., Rohr, R. P., and Bersier, L. F. (2011). Phylogenetic signal in predator–prey body-size relationships. Ecology 92, 2183–2189. doi: 10.1890/10-2234.1
Nilsson, P. A., and Brönmark, C. (1999). Foraging among cannibals and kleptoparasites: effects of prey size on pike behavior. Behav. Ecol. 10, 557–566. doi: 10.1093/beheco/10.5.557
O'Connor, S., Ono, R., and Clarkson, C. (2011). Pelagic fishing at 42,000 years before the present and the maritime skills of modern humans. Science 334, 1117–1121. doi: 10.1126/science.1207703
Olsen, E. M., Heupel, M. R., Simpfendorfer, C. A., and Moland, E. (2012). Harvest selection on Atlantic cod behavioral traits: implications for spatial management. Ecol. Evol. 2, 1549–1562. doi: 10.1002/ece3.244
Ona, E., and Godø, O. R. (1990). Fish Reaction to Trawling Noise: the Significance for Trawl Sampling. Rapports et Proces-verbaux des Réunions. Conseil International pour l'Éxploration de la Mer. 189, 159–166.
Parkes, J., and Panetta, F. (2009). “Eradication of invasive species: progress and emerging issues in the 21st century,” in Invasive Species Management. A Handbook of Principles and Techniques, eds M. N. Clout and P. A. Williams (Oxford, UK: Oxford University Press), 47–60.
Pauly, D., and Watson, R. (2005). Background and interpretation of the ‘Marine Trophic Index’as a measure of biodiversity. Philos. Trans. R. Soc. Lond. B Biol. Sci. 360, 415–423. doi: 10.1098/rstb.2004.1597
Pietsch, T. W., and Grobecker, D. B. (1978). The compleat angler: aggressive mimicry in an antennariid anglerfish. Science 201, 369–370. doi: 10.1126/science.201.4353.369
Rakitin, A., and Kramer, D. L. (1996). Effect of a marine reserve on the distribution of coral reef fishes in Barbados. Oceanogr. Literat. Rev. 9:922.
Raventós, N., and MacPherson, E. (2005). Effect of pelagic larval growth and size at hatching on the post-settlement survivorship in two temperate labrid fishes of the genus Symphodus. Mar. Ecol. Prog. Ser. 285, 205–211. doi: 10.3354/meps285205
Riede, J. O., Brose, U., Ebenman, B., Jacob, U., Thompson, R., Townsend, C. R., et al. (2011). Stepping in Elton's footprints: a general scaling model for body masses and trophic levels across ecosystems. Ecol. Lett. 14, 169–178. doi: 10.1111/j.1461-0248.2010.01568.x
Sissenwine, M., and Shepherd, J. (1987). An alternative perspective on recruitment overfishing and biological reference points. Can. J. Fish. Aquat. Sci. 44, 913–918. doi: 10.1139/f87-110
Song, Y., Endepols, S., Klemann, N., Richter, D., Matuschka, F. R., Shih, C. H., et al. (2011). Adaptive introgression of anticoagulant rodent poison resistance by hybridization between old world mice. Curr. Biol. 21, 1296–1301. doi: 10.1016/j.cub.2011.06.043
Sullivan, A. P., Bird, D. W., and Perry, G. H. (2017). Human behaviour as a long-term ecological driver of non-human evolution. Nat. Ecol. Evol. 1:0065. doi: 10.1038/s41559-016-0065
Suuronen, P., Lehtonen, E., and Wallace, J. (1997). Avoidance and escape behaviour by herring encountering midwater trawls. Fish. Res. 29, 13–24. doi: 10.1016/S0165-7836(96)00523-1
Tran, D. S. C., Langel, K. A., Thomas, M. J., and Blumstein, D. T. (2016). Spearfishing-induced behavioral changes of an unharvested species inside and outside a marine protected area. Curr. Zool. 62, 39–44. doi: 10.1093/cz/zov006
Trebilco, R., Baum, J. K., Salomon, A. K., and Dulvy, N. K. (2013). Ecosystem ecology: size-based constraints on the pyramids of life. Trends Ecol. Evol. 28, 423–431. doi: 10.1016/j.tree.2013.03.008
Trzcinski, M. K., Mohn, R., and Bowen, W. D. (2006). Continued decline of an Atlantic cod population: how important is gray seal predation? Ecol. Appl. 16, 2276–2292. doi: 10.1890/1051-0761(2006)016[2276:CDOAAC]2.0.CO;2
Urban, M. C. (2007). The growth-predation risk trade-off under a growing gape-limited predation threat. Ecology 88, 2587–2597. doi: 10.1890/06-1946.1
Uusi-Heikkilä, S., Sävilammi, T., Leder, E., Arlinghaus, R., and Primmer, C. R. (2017). Rapid, broad-scale gene expression evolution in experimentally harvested fish populations. Mol. Ecol. 26, 3954–3967. doi: 10.1111/mec.14179
Uusi-Heikkilä, S., Whiteley, A. R., Kuparinen, A., Matsumura, S., Venturelli, P. A., Wolter, C., et al. (2015). The evolutionary legacy of size-selective harvesting extends from genes to populations. Evol. Appl. 8, 597–620. doi: 10.1111/eva.12268
Vigliola, L., and Meekan, M. G. (2002). Size at hatching and planktonic growth determine post-settlement survivorship of a coral reef fish. Oecologia 131, 89–93. doi: 10.1007/s00442-001-0866-4
Walters, C. J., and Juanes, F. (1993). Recruitment limitation as a consequence of natural selection for use of restricted feeding habitats and predation risk taking by juvenile fishes. Can. J. Fish. Aquat. Sci. 50, 2058–2070. doi: 10.1139/f93-229
Walton, W. E., Easter, S. S. Jr., Malinoski, C., and Hairston, N. G. Jr. (1994). Size-related change in the visual resolution of sunfish (Lepomis spp.). Can. J. Fish. Aquat. Sci. 51, 2017–2026. doi: 10.1139/f94-204
Weihs, D. (1977). Effects of Size on Sustained Swimming Speeds of Aquatic Organisms. New York, NY: Academic Press.
Werner, E. E., and Hall, D. J. (1988). Ontogenetic habitat shifts in bluegill: the foraging rate-predation risk trade-off. Ecology 69, 1352–1366. doi: 10.2307/1941633
Wilson, D. T., and Meekan, M. G. (2002). Growth-related advantages for survival to the point of replenishment in the coral reef fish Stegastes partitus (Pomacentridae). Mar. Ecol. Prog. Ser. 231, 247–260. doi: 10.3354/meps231247
Wilson, M., Schack, H. B., Madsen, P. T., Surlykke, A., and Wahlberg, M. (2011). Directional escape behavior in allis shad (Alosa alosa) exposed to ultrasonic clicks mimicking an approaching toothed whale. J. Exp. Biol. 214, 22–29. doi: 10.1242/jeb.043323
Wisenden, B. D., and Harter, K. R. (2001). Motion, not shape, facilitates association of predation risk with novel objects by fathead minnows (Pimephales promelas). Ethology 107, 357–364. doi: 10.1046/j.1439-0310.2001.00667.x
Wisenden, B. D., Pogatshnik, J., Gibson, D., Bonacci, L., Schumacher, A., and Willett, A. (2008). Sound the alarm: learned association of predation risk with novel auditory stimuli by fathead minnows (Pimephales promelas) and glowlight tetras (Hemigrammus erythrozonus) after single simultaneous pairings with conspecific chemical alarm cues. Environ. Biol. Fishes 81, 141–147. doi: 10.1007/s10641-006-9181-6
Woodward, G., Ebenman, B., Emmerson, M., Montoya, J. M., Olesen, J. M., Valido, A., et al. (2005). Body size in ecological networks. Trends Ecol. Evol. 20, 402–409. doi: 10.1016/j.tree.2005.04.005
Ydenberg, R. C., and Dill, L. M. (1986). The economics of fleeing from predators. Adv. Study Behav. 16, 229–249. doi: 10.1016/S0065-3454(08)60192-8
Yodzis, P. (2001). Must top predators be culled for the sake of fisheries? Trends Ecol. Evol. 16, 78–84. doi: 10.1016/S0169-5347(00)02062-0
Young, R. G., and Hayes, J. W. (2004). Angling pressure and trout catchability: behavioral observations of brown trout in two New Zealand backcountry rivers. N. Am. J. Fish. Manage. 24, 1203–1213. doi: 10.1577/M03-177.1
Keywords: predator recognition, learning, alarm cue, social facilitation, size-structure, trophic, sensory
Citation: Meekan MG, McCormick MI, Simpson SD, Chivers DP and Ferrari MCO (2018) Never Off the Hook—How Fishing Subverts Predator-Prey Relationships in Marine Teleosts. Front. Ecol. Evol. 6:157. doi: 10.3389/fevo.2018.00157
Received: 27 March 2018; Accepted: 18 September 2018;
Published: 16 October 2018.
Edited by:
Ann Valerie Hedrick, University of California, Davis, United StatesReviewed by:
Shawn M. Wilder, Oklahoma State University, United StatesMike S. Allen, University of Florida, United States
Rebecca Lee Selden, Rutgers University, The State University of New Jersey, United States
Copyright © 2018 Meekan, McCormick, Simpson, Chivers and Ferrari. This is an open-access article distributed under the terms of the Creative Commons Attribution License (CC BY). The use, distribution or reproduction in other forums is permitted, provided the original author(s) and the copyright owner(s) are credited and that the original publication in this journal is cited, in accordance with accepted academic practice. No use, distribution or reproduction is permitted which does not comply with these terms.
*Correspondence: Mark G. Meekan, bS5tZWVrYW5AYWltcy5nb3YuYXU=