- 1Department of Biology, Long Island University-Post, Greenvale, NY, United States
- 2Department of Science, Syosset High School, Syosset, NY, United States
- 3Department of Biology, Central Michigan University, Mount Pleasant, MI, United States
- 4Department of Biological Sciences, Auburn University, Auburn, AL, United States
In some areas of the Antarctic shelf, bryozoans are abundant, acting as ecosystem engineers creating secondary structures with wide benthic coverage and harboring numerous other species. As the combined forces of global warming and ocean acidification threaten these habitats, we measured the composition of habitat-forming bryozoan communities using two techniques for imaging the sea floor, a YoYo-camera system and the AWI Ocean Floor Observation System (OFOS). YoYo-camera transects of the Bellingshausen, Amundsen, and Ross Seas were conducted during a research cruise on the R/V Nathaniel B. Palmer in 2013. OFOS transects included sites in the northern Palmer Archipelago where it borders the Scotia Sea and the Weddell Sea as part of the DynAMO project during the PS81 and PS96 cruises of R/V Polarstern in 2013 and 2015-16, respectively. Areas of bryozoan colonies were measured from the sea floor images using machine-learning algorithms available through the Trainable Weka Segmentation plugin developed for FIJI software. Habitat-forming bryozoan communities in the Palmer Archipelago and Ross Sea were largely composed of anascan flustrid species with finely mineralized skeletons, and to a lesser extent by other ascophoran lepraliomorph and umbonulomorph species having more robustly mineralized skeletons. Although habitat-forming bryozoan communities in the shallower (200 m) sites of the Weddell Sea also contained flustrid species, percent area and composition of flustrid bryozoans declined with increasing depth. Lepraliomorph and umbonulomorph bryozoan morphotypes were more abundant in the Weddell Sea, maintaining their relative percent area and increasing their percent composition between 200 − 400 m. Moreover, our analyses of species composition based on externally gathered datasets show similar trends among sites, depths, and degrees of colony mineralization to our seabed imaging study. Variation present in the bryozoan species compositions of the Amundsen and Bellingshausen Seas suggest that these areas potentially represent divergent bryozoan communities requiring further validation via remote imaging surveys. Overall, compositional differences among Antarctic habitat-forming bryozoan communities are likely influenced by the combined effects of seasonal ice scour and carbonate chemistry, which in an increasingly acidified and warming ocean may put the communities of the eastern Weddell Sea at greater risk.
Introduction
Among the rich benthic invertebrate communities of the Antarctic shelf are diverse assemblages of echinoderms, brachiopods, crustaceans, sea spiders, octocorals, glass sponges, and bryozoans (Thatje et al., 2005). Many of these species are endemic (Pugh and Convey, 2008) and survive only in a narrow thermal range (Pörtner et al., 2007). Many Antarctic shelf invertebrates have body types supported by calcite, aragonite, or bimineralic skeletons requiring increased metabolic costs to build due to the combined influences of cold temperature, lower pH, increased hydrostatic pressure, and mineral dissolution (Andersson et al., 2008; McNeil and Matear, 2008). As a result of these natural physiological challenges, the skeletons of some Antarctic invertebrates are often noted for being thinner and more delicate than those of comparable shallow-water temperate or warm-water species (McClintock et al., 2009; Watson et al., 2012; Duquette et al., 2018). The effects of typical environmental pressures on Antarctic shelf invertebrates are compounded by the impacts of ocean acidification (Hofmann et al., 2010), global warming (Ingels et al., 2012), and the shoaling of the calcite compensation depth (Hillenbrand et al., 2003; Griffiths, 2010). For these reasons, identifying specialized communities of the Antarctic shelf rich with species dependent on mineralized body types that act as ecosystem engineers (e.g., bryozoans) and provide secondary structure for numerous other taxa is crucial for protecting Antarctic diversity. Bryozoan diversity in the Southern Ocean is estimated at more than 400 species, with the majority belonging to the clades Cheilostomata and Cyclostomata (De Broyer and Danis, 2011; Figuerola et al., 2014; Pabis et al., 2014). Within these clades bryozoan species tend to converge on different kinds of encrusting and erect colonial forms that can make significant secondary structures or reef-like habitats. Although some habitat-forming bryozoan communities have been documented worldwide (Wood et al., 2012), little is known about the species assemblages that create these unique habitats and their importance to Antarctic shelf communities as a whole.
Faunal communities inhabiting the deeper regions of the Antarctic shelf are, in general, less physically disturbed than benthic assemblages on the shallower regions of the shelf (Barnes and Conlan, 2007). Disturbances in modern shelf communities are most notably caused by seasonal ice scour, which has its greatest effect down to 100 m but can progress with lesser effects down to 500 m in some places (Gutt et al., 1996; Barnes and Conlan, 2007). The last glacial maximum that traversed the western Antarctic shelf likely limited shelf benthic communities to the deeper continental margin until about 12 and 15 thousand years ago (Anderson et al., 2002). Since many benthic invertebrates inhabiting the shelf exhibit eurybathic distributions (Brey et al., 1996), the post-glacial recolonization process may have been sourced by populations from the continental slope and abyssal regions, surrounding continents and islands, or other surviving refuge habitats (Barnes and Kuklinski, 2010). Studies of Antarctic shelf bryozoans and sea spiders support the refugia hypothesis (Barnes and Kuklinski, 2010; Leese et al., 2015; Barnes et al., 2016). Based on older and current patterns of ecological disturbance and subsequent recolonization, invertebrate communities inhabiting the shallower and deeper regions of the Antarctic shelf may be comprised of significantly different species assemblages (Jones et al., 2007; Boger, 2011; Barnes et al., 2016).
Antarctic shelf communities have often been described by their species compositions using standard sampling methods (e.g., Agassiz trawler and epibenthic sledge). The development of remote imaging systems allows for the non-destructive characterization of shelf fauna on significantly wider spatial scales (Grange and Smith, 2013; Piepenburg et al., 2017). Used together direct and remote sampling techniques provide powerful tools for comparing benthic species assemblages on both small and wide spatial scales that may be tracked over time. Our study focuses on measuring the coverage and composition of the most abundant systematic groups of bryozoans occurring on the Antarctic shelf: the anascan Flustrina (flustrids) and two ascophoran groups, the Lepraliomorpha and Umbonulomorpha. We lumped the latter two systematic groups together, as recent phylogenetic analyses have determined the Lepraliomorpha and Umbonulomorpha to be paraphyletic clades that interdigitate (Waeschenbach et al., 2012; Taylor et al., 2015). Taking advantage of more recent imaging surveys with wider geographic distributions and better-resolved images of the Antarctic benthos, we used novel machine learning-based segmentation algorithms to discern between major morphological grades and clades of bryozoans.
Specific aims for our study were to map and measure the relative coverage and clade compositions of habitat-forming bryozoan communities using our seabed image datasets. Bryozoan-rich habitats with greater benthic coverage were selectively investigated since these larger communities are more likely to be reinvestigated by future benthic studies thus facilitating the documentation of potential temporal shifts. We test whether differences in coverage and clade composition correlate significantly with select abiotic factors such as depth, temperature, and salinity. As the clade groupings listed above differ significantly in degree of biomineralization (Smith et al., 2006; Smith, 2014), we also identify specific Antarctic bryozoan communities more at risk from the effects of ocean acidification. Our criteria used to categorize habitat-forming bryozoan communities emphasizes select regions of the Antarctic shelf over wide spatial scales of the Ross Sea, Palmer Archipelago, and Weddell Sea, so we augmented our geographical and depth comparisons using bryozoan species records gathered from external datasets testing for similar trends in community structure.
Materials and Methods
Sampling
YoYo-camera transects were conducted during 2013 on the R/V Nathaniel B. Palmer in three western Antarctic Seas, with the majority occurring in the Ross Sea (Bellingshausen Sea: six; Amundsen Sea: two; and Ross Sea: 12). Sea floor imaging on the R/V Nathaniel B. Palmer consisted of a vertically oriented YoYo-camera system using a DSC 10,000 camera, an OIS 3821 strobe, and a bottom contact trigger positioned 2.5 m above the bottom (Halanych et al., 2013). YoYo-camera images were standardized to a single size (4.32 m2) using parallel laser beams set 10 cm apart for scale. Seabird SBE3 oceanographic sensors mounted on a CTD rosette maintained by the US Antarctic Program collected environmental variables such as salinity, temperature, and fluorometer readings at maximum depth. The AWI Ocean Floor Observation System (OFOS) imaging of the seabed in the Antarctic Peninsula and Weddell Sea was part of the DynAMO project during the PS81/96 cruises of R/V Polarstern in 2013 and 2015-16. Using the OFOS system, image size varies based on distance from the seabed and is calculated for each image (median = 5.4 m2). Salinity, temperature, and chlorophyll α readings at maximum depth were collected by CTD (see Segelken-Voigt et al., 2016; Piepenburg et al., 2017; for sampling details). OFOS transects included a subset from the Palmer Archipelago where it borders the Scotia Sea (n = four), but the majority of the OFOS transects were conducted throughout the Weddell Sea (n = 16). Respective depth ranges between OFOS and YoYo-camera transects were split between shallower (170–400 m) and deeper (600–1,200 m) areas of the shelf, with the greatest amount of overlap between 400 and 600 m (Figure 1 and Table 1).
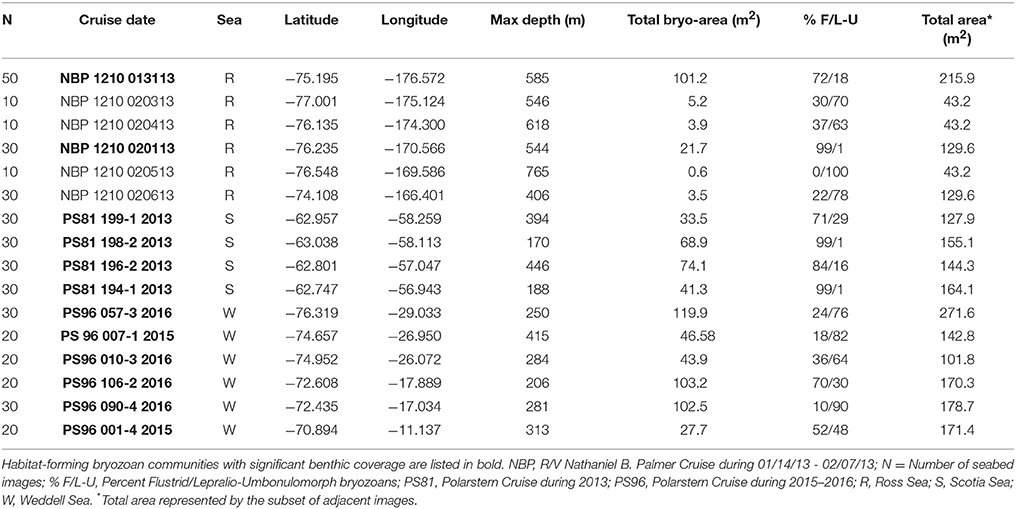
Table 1. Sites in the Ross, Scotia, and Weddell Seas where bryozoan-rich and habitat-forming bryozoan communities were found on the Antarctic Shelf.
Sea Floor Imaging Analysis
We used the machine learning algorithms included with the Trainable WEKA segmentation plugin (Arganda-Carreras et al., 2017) available through FIJI imaging software (Schindelin et al., 2012) to measure the area of bryozoan colonies. As the purpose of our study was to specifically document and characterize specialized habitat-forming bryozoan communities, the review and inclusion of images for further analysis was intentionally non-random. All YoYo-camera and OFOS images were visually inspected for the presence of bryozoan colonies. The ability to correctly identify and categorize bryozoan colony types in the sea floor images was crucial to this process. To that end, a database was created that includes macrophotographic images and scanning electron micrographs of bryozoan samples collected by benthic trawls that corresponded to the YoYo-camera transects as part the R/V Nathaniel B. Palmer cruise (n = 133 samples). Our classifications were also cross-referenced with the bryozoan taxa collected from these sites as listed in our species records gathered from external databases. We chose to measure areas of the Antarctic shelf with more extensive bryozoan coverage and not every observed bryozoan colony, so sea floor image series were chosen where bryozoans occurred in at least three successive images. Ten adjacent images were processed as a unit in FIJI (see Table 1 for the number of processed images per site). Resolution of all processed images was downsized to 1,024*1,024 pixels. Some image series gathered by YoYo-camera were color-corrected to improve contrast. Trainable Weka Segmentation plugin was used with the default settings plus the entropy and structure filters to reduce classification errors of the resulting three bryozoan groups (Flustrina, Lepralio-Umbonulomorpha, and Background Substrate/Other Taxa). The plugin requires that the user manually outline representative bryozoan colony types from each group, background substrate, and other taxa in a subset of images from the 10-image set. This process trains the algorithm to classify and outline each group in all provided images. Once the image segmentation is applied, all images are visually inspected for classification errors. If classification errors are found, then the user has the option of manually correcting the errors, improving the image segmentation, and then reapplying the improved classification to all images. From these segmented images, percent areas of each group were measured with MorphJLib for Region Morphometry. We processed 400 images in shelf areas of the Ross Sea, Palmer Archipelago/Scotia Sea, and Weddell Sea where more extensive bryozoan coverage was observed (total area analyzed from all photographs with bryozoans = 2232.7 m2).
Despite the reliability of the segmentation methods used here, we recognize that a low proportion of the bryozoans in the sea floor images may have been misclassified, as colony form varies significantly in some species (e.g., Adelascopora secunda and A. jeqolqa with flustriform and cellariiform colony morphologies, respectively). Since our main classification criterion between bryozoan groups was based on the presence or absence of flexible flustriform (foliaceous) colony morphologies, some cellariiform genera in the clade Flustrina (e.g., Melicerita and Cellaria) may have been misclassified with the lepralio-umbonulomorph morphotypes in some images. Although not as abundant as cheilostome bryozoans, moderately mineralized cyclostome bryozoans with erect colonies (e.g. Hornera and Fasciculipora) may also have been included with the lepralio-umbonulomorph morphotypes in some images. However, since cellariiform members of Flustrina and all cyclostome bryozoans have more mineralized skeletons than their flustriform counterparts (see Supplemental Figure 1; Hayward, 1995), our classification scheme succeeds in separating bryozoan species with predominantly chitinous epithelial body walls (cystids) from those with mainly mineralized epithelial cystids. This phylogenetic and functional split based on colony form and degree of epithelial mineralization further supports the compositional differences within our classification scheme.
Geographic and Depth-Related Trends
We plotted our processed sea floor imaging data with ArcGIS software (ver. 10.5). Relationships among bryozoan percent area, percent composition, bryozoan colony type, Antarctic sea, temperature, salinity, fluorometric readings, and depth were explored using JMP 14 software (SAS). Values for percent area and percent composition were arcsine transformed before conducting the ANOVA and principle component analyses. We then augmented our geographic and depth analyses listed above using the combined bryozoan collection records of the Smithsonian Institution National Museum of Natural History, the Census of Marine Antarctic Life, and the SCAR Marine Biodiversity Information Network (Barnes and Downey, 2014) from Antarctica. This dataset consisted of unique bryozoan species records listing bryozoan species, latitude, longitude, and depth. From this dataset we quantified the number of occurrences of 145 bryozoan species as an indirect proxy of relative abundance in the Ross Sea, Amundsen Sea, Bellingshausen Sea, Palmer Archipelago, and Weddell Sea (Ross Sea N = 629; Amundsen Sea = 183; Bellingshausen Sea = 134; Palmer Archipelago = 1,446, and Weddell Sea = 1,904 records; see Supplemental Table 1). Sampled depth ranges were not equally distributed among our geographic sites, so we focused on depth ranges of 200–1,000 m for all sites as these depth ranges best matched our seabed image dataset. Species counts for the Amundsen and Bellingshausen Seas were grouped together to offset the effects of decreased sampling intensity in these areas. We tested for trends in bryozoan community structure, biomineralization (see Supplemental Table 2), and depth among sites using non-metric dimensional scaling (nMDS) with the vegan package available through R (ver. 3.5). The species dataset was autotransformed with a square root transformation and a Wisconsin double standardization. The Bray Curtis method was used to create a dissimilarity matrix and differences between geographic areas and depth ranges were tested with a permutational MANOVA (AMOVA). The percentages of finely, moderately, and heavily mineralized species were measured within each depth range among sites. These percentages were plotted as environmental vectors and surfaces on the resulting ordination plots. More broadly, depth-related trends among bryozoan clades were explored worldwide, adding bryozoan species records (Smithsonian Institution National Museum of Natural History, N = 1,723) from other non-Antarctic sites in the Southern and Northern Hemispheres with reliable depth, latitude, and longitude values.
Results
Composition of Habitat-Forming Bryozoan Communities on the Antarctic Shelf
Habitat-forming bryozoan communities were found in a subset of sites in the Ross Sea (see Figure 2A) consisting mainly of leaf-like colonies of anascan flustrid species (e.g., Carbasea, Nematoflustra, Isosecuriflustra, and Kymella, etc.) with finely mineralized skeletons (see Figure 2B). Other common types, though present to a lesser extent than flustrid bryozoans, are the more robustly mineralized colonies of diverse ascophoran lepraliomorph (e.g., Reteporella and Adelascopora) and umbonulomorph (e.g., Bostrychopora, Cellarinella, and Systenopora) species. Figures 2C–E show typical sea floor images from the Ross Sea, Palmer Archipelago/Scotia Sea, and Weddell Sea where bryozoans are common, forming substantial secondary structures. Although different kinds of invertebrate communities were present in the Bellingshausen and Amundsen Sea sites, bryozoan habitats applicable to our study were not found. The resolution of both the YoYo-camera and OFOS systems allowed for bryozoan colonies to be readily distinguished from the surrounding background substrate and associated taxa (e.g., sponges, cnidarians, echinoderms, and fish). Furthermore, due to the more robust mineralized skeletons and divergent colony morphologies present in the vast majority of lepraliomorph and umbonulomorph bryozoans as compared to flustrid species, the Trainable Weka Segmentation plugin for FIJI software was able to reliably discriminate between these two bryozoan categories (see Figure 2F with flustrids shown in green, lepralio-umbonulomorphs in red, and background substrate and associated taxa in purple).
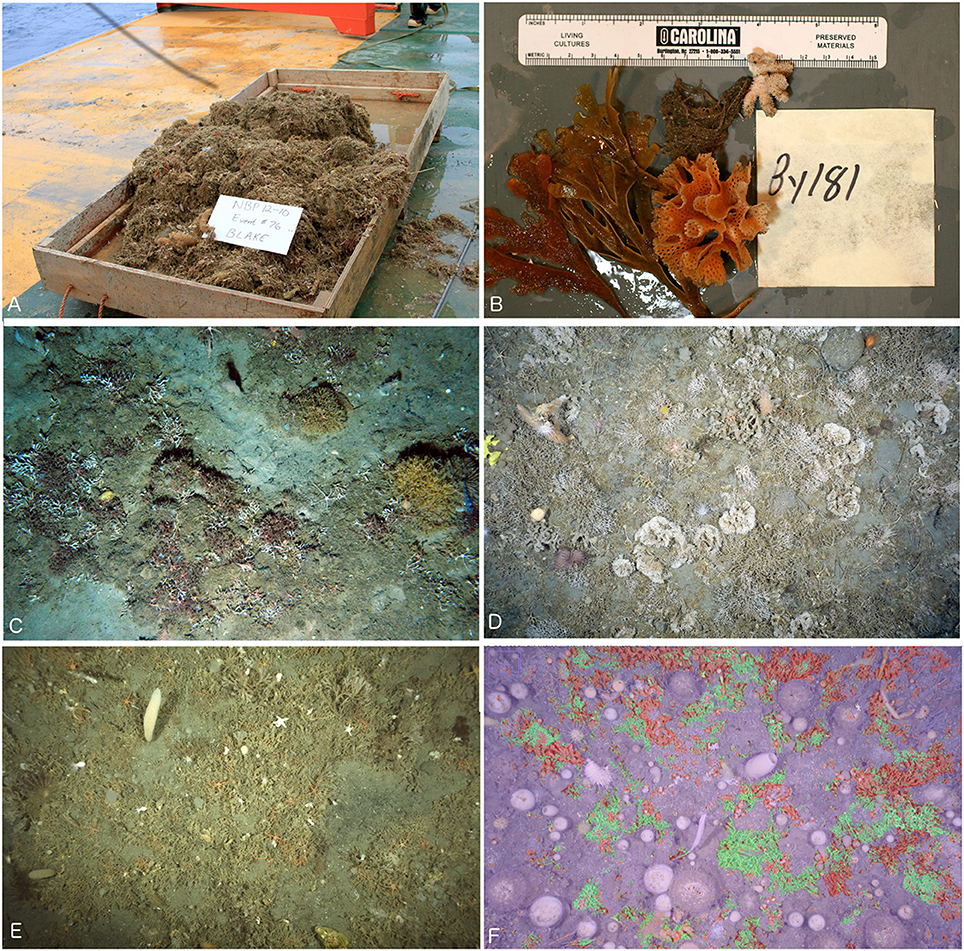
Figure 2. Overview of bryozoan colony types and habitats. (A) Blake trawl gathered from the Ross Sea containing mainly flustrid bryozoans. (B) Typical flustrid, lepraliomorph, and umbonulomorph colony types. (C) Habitat-forming bryozoan communities of the Ross Sea. (D) Habitat-forming bryozoan communities of the Weddell Sea. (E) Habitat-forming bryozoan communities of the Scotia Sea. (F) Segmented image where flustrids are shaded red, lepralio-umbonulomorphs are shaded green, and the background substrate and other taxa are shaded purple.
Our surveys of all imaging datasets found 16 out of 37 sites in the Ross Sea, Palmer Archipelago/Scotia Sea, and Weddell Sea (n = 6, 4, 6, respectively; see Table 1) with applicable bryozoan communities in a depth range of 170–765 m. Fifteen of these sites had a total bryozoan area >3 m2 (Table 1 and Figure 3). No obvious geographic trend in the distribution of habitat-forming bryozoan sites was found in the Ross Sea (see Figure 3B), and only one of the Ross Sea sites exhibited a total area that matched sites in the Palmer Archipelago/Scotia Sea and Weddell Sea (Figures 3A–D). All of the habitat-forming bryozoan sites in the Weddell Sea were found in its more eastern region (Figure 3D). The total percent compositions for flustrid and lepralio-umbonulomorphotypes within habitat-forming bryozoan communities were similar between the Ross Sea and Palmer Archipelago/Scotia Sea sites, but differed from the Weddell Sea (Ross Sea: 79% flustrid/21% lepralio-umbonulomorph; Palmer Archipelago/Scotia Sea: 90/10%; Weddell Sea: 34/66%).
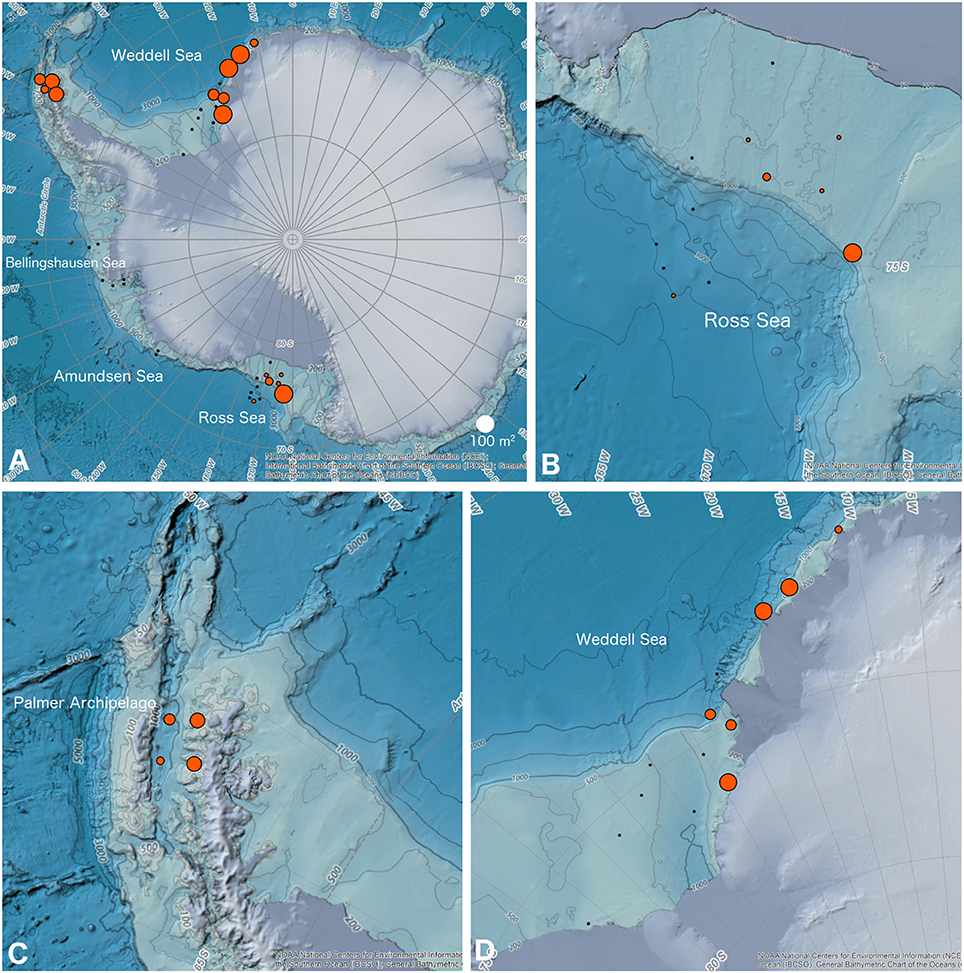
Figure 3. GIS maps of the location and area of bryozoan-rich and habitat-forming bryozoan communities considered in this paper. (A) Map of Antarctica showing all sites. (B) Ross Sea. (C) Sites along the northern portion of the Palmer Archipelago where it borders the Scotia Sea. (D) Weddell Sea. Black dots are areas included in the imaging surveys, but where habitat-forming bryozoan communities were not observed. Red circles are areas with habitat-forming bryozoan communities scaled by the total bryozoan area. The NOAA GIS base maps of Antarctica obtained from a web-shared repository.
Overall, the Ross and Palmer Archipelago/Scotia sites are largely comprised of flustrid bryozoans and to a lesser extent by lepralio-umbonulomorph morphotypes. However, the Palmer Archipelago/Scotia Sea sites are more variable with several high outliers for lepralio-umbonulomorph morphotypes. Percent area and percent clade composition of these two bryozoan morphotypes are significantly different in the Weddell Sea sites, as lepralio-umbonulomorphs account for more of the percent area and percent composition (Figures 4A,B). A one-way ANOVA found significant differences in the means for both percent area and percent clade composition between flustrid and lepralio-umbonulomorph morphotypes ignoring background substrate and other taxa within each of the three sea regions (Table 2). Significant differences were mostly found for the percent areas and percent clade compositions of flustrid and lepralio-umbonulomorph morphotypes among the three regions, except in a few comparisons (Table 2).
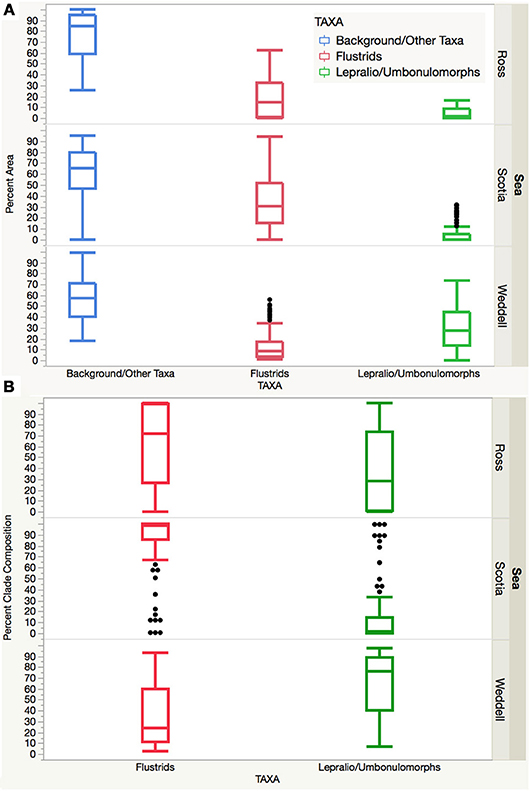
Figure 4. (A) Box plot showing the percent area of the background substrate/other taxa, flustrids, and lepralio-umbonulomorphs among the three Antarctic regions. (B) Box plot showing the percent clade composition of flustrid and lepralio-umbonulomorph bryozoans among the three Antarctic regions.
Environmental Variables
Environmental variables such as salinity, temperature, and fluorometric readings at depth varied slightly among sites with habitat-forming bryozoan communities (Supplemental Table 3). A principle components analysis including these variables along with transformed measurements of percent area and percent composition resulted in the eigenvector weightings shown in Table 3 that account for 66.3% of the total variation. Although environmental factors such as temperature and salinity were included as significant weightings in the first two eigenvectors, their relative importance is unclear since our data do not include any variation over time. The three Antarctic regions are differentiated largely by their different compositions of flustrid and lepralio-umbonulomorph bryozoans and depth (Figure 5). We explore the differences in bryozoan composition and depth in more detail in Figure 6. For this analysis images in which bryozoans comprised <10% of the total area were excluded to rule out the influence of pioneer colonies and to focus more on larger bryozoan communities. The trends observed between maximum depth and the percent areas of bryozoans are largely based on the compositional differences among the three Antarctic regions. The percent area of flustrid bryozoans increases with depth in the Ross Sea (r2 = 0.30, Figure 6A), but this trend is largely influenced by only two sites (NBP 1210 013113 and NBP 1210 020113, 585 and 544 m, respectively). In general, the deeper shelf sites in the Ross Sea are characterized by a greater prevalence of flustrid bryozoans as compared to lepralio-umbonulomorph bryozoans. Flustrid bryozoans comprise most of the shallower shelf sites in the northern Palmer Archipelago/Scotia Sea showing no significant correlation with maximum depth (Figure 6A). However, the percent area of lepralio-umbonulomorph bryozoans did increase with maximum depth (r2 = 0.31). In contrast to the Ross Sea and Palmer Archipelago/Scotia Sea sites, the percent area of flustrid bryozoans decreased in the shallower areas of the eastern Weddell Sea (r2 = 0.36), and lepralio-umbonulomorph bryozoans are more prevalent these habitats (Figure 6A). Similar trends are observed for the percent clade composition among regions and depths with the only obvious difference being that the percent composition of lepralio-umbonulomorph bryozoans increases with depth in the Weddell Sea sites (r2 = 0.25, Figure 6B).
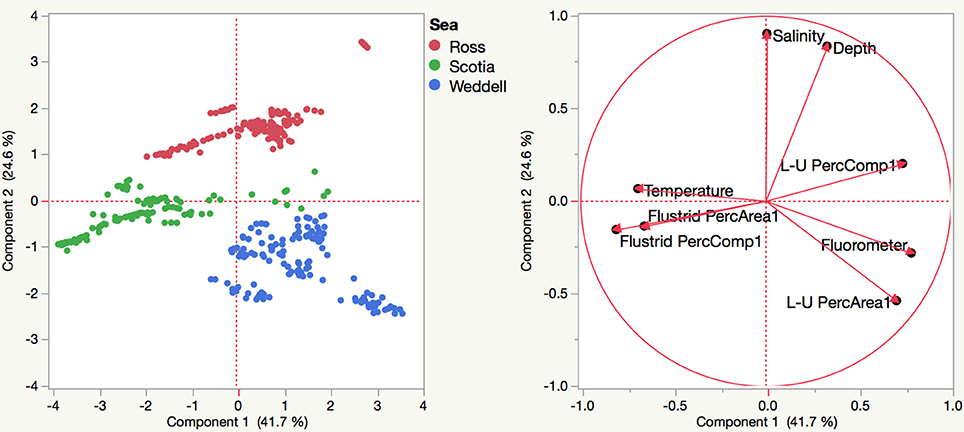
Figure 5. Principle components analysis based on the percent areas and percent compositions of bryozoans along with environmental variables collected at each site among the three Antarctic regions. L-U, Lepralio-Umbonulomorph bryozoans.
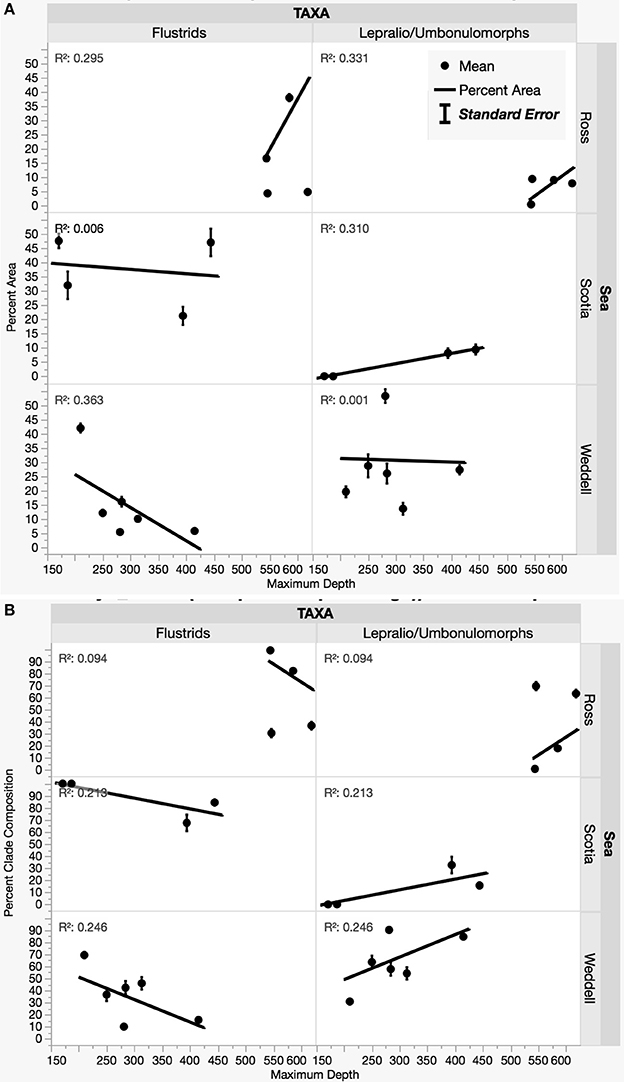
Figure 6. Relationships among the percent area of flustrids and lepralio-umbonulomorph bryozoans by geographic region and depth. (A) Percent bryozoan area and depth. (B) Percent clade composition and depth.
Significant differences in species composition and degree of mineralization are evident among sites and some depth groupings in our nMDS analyses. Overlap in species composition within the first 400 m is shown for the Ross Sea, Weddell Sea, and the Palmer Archipelago (Figures 7A,C). Deeper zones of these regions (> 600 m) exhibit more unique species compositions. Amundsen and Bellingshausen species compositions by depth range were significantly more variable and different from the other regions (Figure 7A). Species compositions among regions also separate based on the percentage of finely, moderately, and heavily mineralized species groups (Figures 7B,D,E). Trends in species degree of biomineralization are similar to our results based on our seabed images. More finely skeletonized species are found in the Palmer Archipelago and Ross Sea, but moderate and heavily mineralized species are more prevalent in the Amundsen and Bellingshausen Seas as well as deeper (>800 m) regions of the Palmer Archipelago and Weddell Sea (Figures 7B,E). Due to sampling biases it is difficult to test for significant differences in community structure beyond 1,000 m. Although based on a small number of samples the deepest Antarctic bryozoans are all flustrids occurring between 3,000 and 5,000 m (N = 3). Beyond Antarctica, worldwide bryozoan records show that cyclostome, flustrid, and lepralio-umbonulomorph bryozoans all occur down to depths of 2,700–2,800 m. However, only flustrid bryozoans are found down to 5,900–6,000 m (Figure 8). These flustrid bryozoans of the abyssal zone include both Antarctic and non-Antarctic species of Notoplites, Himantozoum, and Camptoplites.
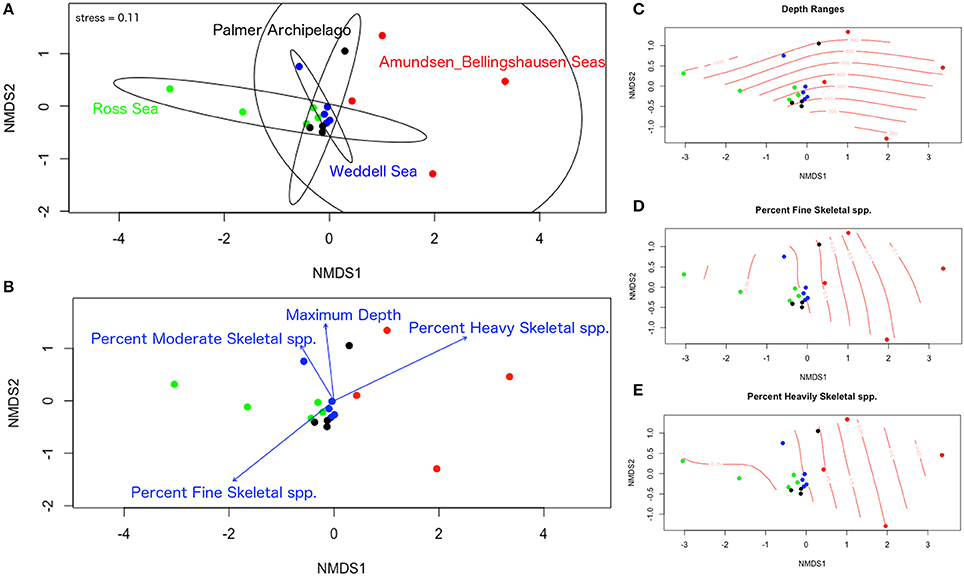
Figure 7. Non-metric dimensional scaling analysis of bryozoan species records gathered from external datasets. (A) Ordination plot of all sites and depth ranges with 95% confidence ellipses around each region. (B) Ordination plot of all sites and depth ranges with environmental vectors for maximum depth, percent fine skeletal species, percent moderate skeletal species, and percent heavily skeletal species. (C–E) Ordinal surface plots for depth ranges, percent fine skeletal species, and percent heavily skeletal species. All percentages are listed as decimals.
Discussion
Biogeography and Composition of Antarctic, Habitat-Forming Bryozoan Communities
Our study focused on mapping and characterizing unique bryozoan-rich communities producing significant coverage on areas of the Antarctic shelf. Although several studies include bryozoans as being one of the more common and diverse members of the shelf fauna (e.g., Barnes and Kuklinski, 2010; Barnes et al., 2016; Figuerola et al., 2018), the impact of expansive bryozoan secondary structures on the surrounding community has not been evaluated on a wide geographic scale (Wood et al., 2013). Evaluating the geographic distribution of habitat-forming bryozoan communities in other Antarctic regions based on published studies using direct sampling or remote-imaging techniques (or both) can be difficult due to interpretational differences in categorizing bryozoan-rich habitats. For example, based on trawl samples bryozoans have been found to be both species-rich and abundant at some Amundsen Sea sites (Linse et al., 2013), but without corresponding seabed images to measure benthic coverage it is not possible to assess if these sites include habitat-forming bryozoan communities as defined here. A recent YoYo-camera survey of the Sabrina Coast Shelf found abundant forms of bryozoans and many other invertebrate taxa, even though bryozoan dominated communities were not observed (Post et al., 2017). However, bryozoan-rich communities (including sponges and soft corals) were found in imaging surveys of the George V Ice Shelf (Post et al., 2011). In general, habitat-forming bryozoan communities are more expansive in Antarctic regions than in other sites worldwide (Wood et al., 2012), albeit only a subset of the Antarctic regions accessible for remote imaging have been explored in detail.
Compositional differences discussed here in habitat-forming bryozoan communities among the Ross Sea, Palmer Archipelago/Scotia Sea, and Weddell Sea are similarly noted for particular species of bryozoans. For example, the flustrid bryozoan Melicerita obliqua makes moderately mineralized cellariiform colonies that do not fit our clade categories, but is segmented correctly by its degree of mineralization. This species is widely distributed in low abundances in the Ross Sea (trawl data, Winston, 1983). However, Melicerita obliqua is one of the most common benthic suspension feeders found in an expansive coastal region of the eastern Weddell and Lazarev Seas [grand average of 595.6 colonies per 100 m2, see (Gutt and Starmans, 1998)] lending support to our finding that habitat-forming communities in the Weddell Sea have a greater prevalence of moderately to robustly calcifying species. Similar to the results of Gutt and Starmans (1998), we found bryozoans abundant in eastern Weddell Sea sites where suspension feeders dominate, but not in more western Weddell Sea sites which are characterized by a higher abundance of detritus feeders. Bryozoan-rich sites in the eastern Weddell and Lazarev Seas are also among the most diverse in other invertebrate taxa (Gutt and Starmans, 1998). Consistent with our study, Cellarinella spp. found on the Fimbul Ice Shelf region of the Weddell Sea are abundant in the shallower (245 m) sites, but decrease sharply at a maximum depth of 510 m (seabed imaging data, Jones et al., 2007). Other unidentified species of bryozoans are more common at the deeper Fimbul Ice Shelf sites (Jones et al., 2007). Similar depth-related differences in the abundances of bryozoan erect colony forms occur at sites from Signy Island (Scotia Sea), where flexible flustrid bryozoan species are more abundant with increasing depth down to 290 m as compared to other more heavily mineralized colony types (Barnes, 1995).
Mineralization Patterns of Antarctic Bryozoans
The composition of bryozoan skeletons varies with latitude, as species from high latitudes and polar regions (60–90°) predominantly use calcite, with a limited number of species being bimineralic (Kuklinski and Taylor, 2009; Taylor et al., 2009). This latitudinal trend in bryozoan skeletal composition may be due to aragonite skeletons' greater susceptibility to dissolution in colder water (Morse et al., 1980; Taylor et al., 2009). Another compounding factor is that bryozoan skeletons vary with respect to the weight percent of magnesium carbonate in the calcite, and skeletons with higher amounts of Mg-calcite are more soluble in colder water (Andersson et al., 2008). As the negative effects of seawater pH and hydrostatic pressure on biomineralization intensify with depth (Feely et al., 2009), selection should favor deep-water bryozoans with lower skeletal Mg content. However, no significant correlations have been found among pH, Mg-calcite content, and depth for species of Antarctic (n = 4, Figuerola et al., 2015) and Artic (n = 52, Borszcz et al., 2013) bryozoans, perhaps because polar bryozoans already exhibit lower levels of Mg-calcite content at both shallow and deeper depths. Despite these results, latitudinal patterns in skeletal composition suggest that there may be a more significant effect from temperature than from depth on aspects of the biomineralization process in bryozoans (Kuklinski and Taylor, 2009; Taylor et al., 2009, 2015). This assertion was not supported by more rigorous sampling of four species of Antarctic bryozoans that exhibited significant variability in the Mg-calcite skeletal content among sites and showed no significant correlation with temperature (Loxton et al., 2014). Therefore, selection may favor local adaptations in response to discrete environmental conditions among isolated populations of bryozoans over small spatial scales (Loxton et al., 2014). Local adaptive responses may be one reason why more significant correlations among calcite types and depth have not been found in the above studies. It should be noted that none of these studies measured the abundances of bryozoan colony morphotypes with different degrees of biomineralization as shown here.
Despite the variation present in the biomineralization patterns of Antarctic bryozoans, some studies do show their usefulness as ecological indicators of global change (Barnes et al., 2011; Fortunato, 2015). Moreover, Antarctic bryozoan species from shallow-water habitats exhibit wide variation in mass-specific metabolic rates (Peck and Barnes, 2004). Interestingly, one common flustrid species, Camptoplites bicornis, was found to have one of the highest mass-specific metabolic rates even when compared to some Antarctic molluscs (Peck and Barnes, 2004). Considering that species of Camptoplites are found in shallow and deep-water habitats alike (as deep as 5 km, see Hayward, 1981), this metabolic flexibility may be one key trait that allows species of Camptoplites, other lightly skeletonized flustrid bryozoans, and a small number of unmineralized ctenostome species (N = 5, Grischenko and Chernyshev, 2015) to occupy and potentially exploit areas of the deeper shelf and the deep sea.
Potential Abiotic Factors
Due to the inherent patchiness and abiotic complexity of benthic communities along the Antarctic shelf, other studies have not found specific environmental factors that account for most of the variation in percent area or species composition aside from depth (Jones et al., 2007) and latitude/longitude (Gutt and Starmans, 1998). However, the presence or absence of significant benthic faunal coverage may be correlated with patterns in the bottom-near current that provides sustenance to these communities (Gutt and Starmans, 1998). In terms of substrata, glacial dropstone densities correlate with increased species diversity and benthic coverage in sites off the Sabrina Coast and the West Antarctic Peninsula (Post et al., 2017; Ziegler et al., 2017). Although isolated bryozoan colonies are present on hard substrata in the seabed images included in our study, habitat-forming bryozoan communities largely occurred on soft bottom areas of the Ross Sea shelf. Dropstones were not observed in significant abundance at the Palmer Archipelago/Scotia Sea or Weddell Sea sites. These observations do not rule out that dropstone presence and specific sediment characteristics may in some areas facilitate recruitment and formation of habitat-forming bryozoan communities.
Sea surface temperatures largely influence seasonal and long-term sea ice coverage patterns surrounding Antarctica (Comiso et al., 2017). Although average sea ice cover increased for the whole of Antarctica over the last 34 years, seasonal patterns in sea ice expansion and melt in some seas of western Antarctica, the Antarctic Peninsula, and the Weddell Sea are different (Comiso et al., 2017). Winter-spring trends show ice melt in the Antarctic Peninsula, but ice expansion in the western Amundsen, Ross, and eastern Weddell Seas. Summer-autumn trends show significant ice retreat in the Bellingshausen and Amundsen Seas, but increases in the Weddell and Ross Seas (Comiso et al., 2017). Additionally, reduction in sea ice cover and subsequent increases in primary algal productivity promote growth in Antarctic benthic shelf communities that outweighs (but does not completely eliminate) the negative impacts of increased ice scour in the shallow shelf zones (Barnes and Conlan, 2007; Barnes, 2017). Interestingly, bryozoan recolonization patterns after recent ice scour differ between areas. Both the George V Ice Shelf (Post et al., 2011) and eastern Weddell Sea (Teixidó et al., 2004) have high bryozoan recolonization abundances, compared to low bryozoan recolonization abundances in the western Weddell (Gutt and Piepenburg, 2003). Based on environmental factors linked to patterns in sea ice coverage, zoobenthic communities in the Bellingshausen and Amundsen Seas would be predicted to increase with time. Interestingly, increased benthic colonization has already been observed in sites of the Antarctic Peninsula (Lagger et al., 2018). In general, the abundances of benthic suspension feeders in the western Ross Sea (Rowden et al., 2015), the northern Antarctic Peninsula (Gutt et al., 2016; Segelken-Voigt et al., 2016), and the eastern Weddell Sea (Gutt and Starmans, 1998) do positively correlate with long-term trends in seasonal ice melt. Spatial and temporal variability in Antarctic marine carbonate chemistry and seawater pH are modified by sea ice melt, primary productivity, freshwater inputs, ocean mixing, and photosynthetic production (Roden et al., 2013; Matson et al., 2014; Smith et al., 2014; Stark et al., 2018). Considering the complex interactions among these environmental drivers, heterogenic species assemblages varying in degrees of body mineralization are to be expected in different regions and depths of the Antarctic shelf. Understanding how future trends in Antarctic sea ice melt will influence ocean acidification and potentially negatively impact the composition of Antarctic shelf communities is crucial for protecting these specialized habitats.
Our study supports the conclusion that flustrid bryozoans are more prevalent in the habitat-forming bryozoan communities in the Ross Sea and northern Palmer Archipelago/Scotia Sea. In contrast, sites in the eastern Weddell Sea are comprised mainly of moderately to robustly mineralized, cellariiform flustrids, lepraliomorph, and umbonulomorph bryozoans. The species compositional differences observed among Antarctic sites and depths are likely influenced by the spatial and temporal variability inherent in seasonal ice scour, carbonate chemistry, and primary productivity. How these specialized communities will respond to the combined forces of future global warming and ocean acidification remains an open question (although see Ashton et al., 2017), but considering the overall species diversity characteristic of habitat-forming bryozoan communities, these habitats should be protected (Wood et al., 2012). Marine protection areas are designated in the Ross Sea and the sub-Antarctic Island of South Georgia (Hogg et al., 2018; Nyman, 2018), however surrounding Antarctic regions remain unprotected and, in particular, shelf communities of the eastern Weddell Sea should be given careful consideration.
Author Contributions
AM and KH conceived and planned the sampling on the R/V N.B. Palmer; SS, VA, and PW gathered and analyzed the data; SS wrote the paper; AM, VA, PW, and KH reviewed the manuscript.
Funding
KH, AM, and SS provided funding. NSF OPP Grant #1043745 to KH, NSF OPP 1043670 to AM, and LIU Grants in Aid of Research to SS.
Conflict of Interest Statement
The authors declare that the research was conducted in the absence of any commercial or financial relationships that could be construed as a potential conflict of interest.
Acknowledgments
The authors would like to thank the efforts of the crew of the R/V N.B. Palmer, D. Piepenburg, and crew of the Polarstern. The authors also thank the reviewers for helpful suggestions on improving the paper.
Supplementary Material
The Supplementary Material for this article can be found online at: https://www.frontiersin.org/articles/10.3389/fevo.2018.00116/full#supplementary-material
Supplemental Figure 1. Clade and mineralization classifications based on common genera of Antarctic bryozoans. Macrophotographic images of various genera are listed from top to bottom in the left panel. Across each panel the middle image is a scanning electron micrograph of the mineralized skeleton with the tissues removed. The right panel is an image taken from our seabed imaging dataset that corresponds to the particular bryozoan group or genus.
Supplemental Table 1. Bryozoan species records gathered from external datasets.
Supplemental Table 2. Antarctic bryozoan species grouped by degree of mineralization: fine, moderate, and heavy.
Supplemental Table 3. Environmental variables measured at bottom depth in bryozoan-rich and habitat-forming bryozoan sites.
References
Anderson, J. B., Shipp, S. S., Lowe, A. L., and Wellner, J. S. (2002). The Antarctic ice sheet during the last glacial maximum and its subsequent retreat history: a review. Quat. Sci. Rev. 21, 49–70. doi: 10.1016/S0277-3791(01)00083-X
Andersson, A. J., Mackenzie, F. T., and Bates, N. R. (2008). Life on the margin: implications of ocean acidification on Mg-calcite, high latitude and cold-water marine calcifiers. Mar. Ecol. Prog. Ser. 373, 265–273. doi: 10.3354/meps07639
Arganda-Carreras, I., Kaynig, V., Rueden, C., Eliceiri, K. W., Schindelin, J., Cardona, A., et al. (2017). Trainable Weka Segmentation: a machine learning tool for microscopy pixel classification. Bioinformatics 33, 2424–2426. doi: 10.1093/bioinformatics/btx180
Ashton, G. V., Morley, S. A., Barnes, D. K. A., Clark, M. S., and Peck, L. S. (2017). Warming by 1°C drives species and assemblage level responses in antarctica's marine shallows. Curr. Biol. 27, 2698–2705.e3. doi: 10.1016/j.cub.2017.07.048
Barnes, D. K., and Conlan, K. E. (2007). Disturbance, colonization and development of Antarctic benthic communities. Philosoph. Transac. R. Soc. B Biol. Sci. 362, 11–38. doi: 10.1098/rstb.2006.1951
Barnes, D. K., Kuklinski, P., Jackson, J. A., Keel, G. W., Morley, S. A., and Winston, J. E. (2011). Scott's collections help reveal accelerating marine life growth in Antarctica. Curr.Bio. 21, R147–R148. doi: 10.1016/j.cub.2011.01.033
Barnes, D. K. A. (1995). Sublittoral epifaunal communities at Signy Island, Antarctica. II. Below the ice-foot zone. Mar. Biol. 121, 565–572. doi: 10.1007/BF00349467
Barnes, D. K. A. (2017). Polar zoobenthos blue carbon storage increases with sea ice losses, because across-shelf growth gains from longer algal blooms outweigh ice scour mortality in the shallows. Glob. Change Biol. 23, 5083–5091. doi: 10.1111/gcb.13772
Barnes, D. K. A., and Downey, R. V. (2014). “Biogeographic Atlas of the Southern Ocean,” in Bryozoa, eds C. De Broyer, P. Koubbi, H. J. Griffiths, B. Raymond, C. Udekem d'Acoz, d', A. P. Van de Putte, B. Danis, B. David, S. Grant, J. Gutt, C. Held, G. Hosie, F. Huettmann, A. Post, and Y. Ropert-Coudert (Cambridge: SCAR), 195–199.
Barnes, D. K. A., and Kuklinski, P. (2010). Bryozoans of the Weddell Sea continental shelf, slope and abyss: did marine life colonize the Antarctic shelf from deep water, outlying islands or in situ refugia following glaciations? J. Biogeogr. 37, 1648–1656. doi: 10.1111/j.1365-2699.2010.02320.x
Barnes, D. K. A., Sands, C. J., Hogg, O. T., Robinson, B. J. O., Downey, R. V., and Smith, J. A. (2016). Biodiversity signature of the last glacial maximum at south georgia, southern ocean. J. Biogeogr. 43, 2391–2399. doi: 10.1111/jbi.12855
Boger, S. D. (2011). Antarctica—before and after Gondwana. Gondwana Res. 19, 335–371. doi: 10.1016/j.gr.2010.09.003
Borszcz, T., Kuklinski, P., and Taylor, P. D. (2013). Patterns of magnesium content in arctic bryozoan skeletons along a depth gradient. Polar Biol. 36, 193–200. doi: 10.1007/s00300-012-1250-z
Brey, T., Dahm, C., Gorny, M., Klages, M., Stiller, M., and Arntz, W. E. (1996). Do Antarctic benthic invertebrates show an extended level of eurybathy? Antarct. Sci. 8, 3–6. doi: 10.1017/S0954102096000028
Comiso, J. C., Gersten, R. A., Stock, L. V., Turner, J., Perez, G. J., and Cho, K. (2017). Positive trend in the Antarctic sea ice cover and associated changes in surface temperature. J. Climate 30, 2251–2267. doi: 10.1175/JCLI-D-16-0408.1
De Broyer, C., and Danis, B. (2011). How many species in the Southern Ocean? Towards a dynamic inventory of the Antarctic marine species. Deep Sea Res. II Top. Stud. Oceanogr. 58, 5–17. doi: 10.1016/j.dsr2.2010.10.007
Duquette, A., Halanych, K. M., Angus, R. A., and McClintock, J. B. (2018). Inter and intraspecific comparisons of the skeletal Mg/Ca ratios of high latitude Antarctic echinoderms. Antarctic Sci. 30, 1–10. doi: 10.1017/S0954102017000566
Feely, R., Doney, S., and Cooley, S. (2009). Ocean acidification: present conditions and future changes in a high-CO2 world. Oceanography 22, 36–47. doi: 10.5670/oceanog.2009.95
Figuerola, B., Gordon, D. P., and Cristobo, J. (2018). New deep Cheilostomata (Bryozoa) species from the Southwestern Atlantic: shedding light in the dark. Zootaxa 4375, 211–239. doi: 10.11646/zootaxa.4375.2.3
Figuerola, B., Gordon, D. P., Polonio, V., Cristobo, J., and Avila, C. (2014). Cheilostome bryozoan diversity from the southwest Atlantic region: is antarctica really isolated? J. Sea Res. 85, 1–17. doi: 10.1016/j.seares.2013.09.003
Figuerola, B., Kuklinski, P., and Taylor, P. D. (2015). Depth patterns in Antarctic bryozoan skeletal Mg-calcite: can they provide an analogue for future environmental changes? Mar. Ecol. Prog. Ser. 540, 109–120. doi: 10.3354/meps11515
Fortunato, H. (2015). Bryozoans in climate and ocean acidification research: a reappraisal of an under-used tool. Reg. Stud. Mar. Sci. 2, 32–44. doi: 10.1016/j.rsma.2015.08.010
Grange, L. J., and Smith, C. R. (2013). Megafaunal communities in rapidly warming fjords along the west Antarctic peninsula: hotspots of abundance and beta diversity. PLoS ONE 8:e77917–e77917. doi: 10.1371/journal.pone.0077917
Griffiths, H. J. (2010). Antarctic marine biodiversity–what do we know about the distribution of life in the Southern Ocean? PLoS ONE 5:e11683. doi: 10.1371/journal.pone.0011683
Grischenko, A. V., and Chernyshev, A. V. (2015). Triticella minini – a new ctenostome bryozoan from the abyssal plain adjacent to the Kuril–Kamchatka trench. Deep Sea Res. II Top. Stud. Oceanograp. 111, 343–350. doi: 10.1016/j.dsr2.2014.08.004
Gutt, J., Alvaro, M. C., Barco, A., Böhmer, A., Bracher, A., David, B., et al. (2016). Macroepibenthic communities at the tip of the Antarctic Peninsula, an ecological survey at different spatial scales. Polar Biol. 39, 829–849. doi: 10.1007/s00300-015-1797-6
Gutt, J., and Piepenburg, D. (2003). Scale-dependent impact on diversity of Antarctic benthos caused by grounding of icebergs. Mar. Ecol. Prog. Ser. 253, 77–83. doi: 10.3354/meps253077
Gutt, J., and Starmans, A. (1998). Structure and biodiversity of megabenthos in the Weddell and Lazarev Seas (Antarctica): ecological role of physical parameters and biological interactions. Polar Biol. 20, 229–247. doi: 10.1007/s003000050300
Gutt, J., Starmans, A., and Dieckmann, G. (1996). Impact of iceberg scouring on polar benthic habitats. Mar. Ecol. Prog. Ser. 137, 311–316. doi: 10.3354/meps137311
Halanych, K. M., Cannon, J. T., Mahon, A. R., Swalla, B. J., and Smith, C. R. (2013). Modern Antarctic acorn worms form tubes. Nat. Comms. 4, 2738–2738. doi: 10.1038/ncomms3738
Hayward, P. J. (1995). Antarctic Cheilostomatous Bryozoa. New York, NY: Oxford University Press. 1–355.
Hillenbrand, C.-D., Grobe, H., Diekmann, B., Kuhn, G., and Fütterer, D. K. (2003). Distribution of clay minerals and proxies for productivity in surface sediments of the Bellingshausen and Amundsen seas (West Antarctica)–Relation to modern environmental conditions. Mar. Geol. 193, 253–271. doi: 10.1016/S0025-3227(02)00659-X
Hofmann, G. E., Barry, J. P., Edmunds, P. J., Gates, R. D., Hutchins, D. A., Klinger, T., et al. (2010). The Effect of ocean acidification on calcifying organisms in marine ecosystems: an organism-to-ecosystem perspective. Annu. Rev. Ecol. Evol. Syst. 41, 127–147. doi: 10.1146/annurev.ecolsys.110308.120227
Hogg, O. T., Huvenne, V. A. I., Griffiths, H. J., and Linse, K. (2018). On the ecological relevance of landscape mapping and its application in the spatial planning of very large marine protected areas. Sci. Total Environ. 626, 384–398. doi: 10.1016/j.scitotenv.2018.01.009
Ingels, J., Vanreusel, A., Brandt, A., Catarino, A. I., David, B., De Ridder, C., et al. (2012). Possible effects of global environmental changes on Antarctic benthos: a synthesis across five major taxa. Ecol. Evol. 2, 453–485. doi: 10.1002/ece3.96
Jones, D. O. B., Bett, B. J., and Tyler, P. A. (2007). Depth-related changes to density, diversity and structure of benthic megafaunal assemblages in the Fimbul ice shelf region, Weddell Sea, Antarctica. Polar Biol. 30, 1579–1592. doi: 10.1007/s00300-007-0319-6
Kuklinski, P., and Taylor, P. D. (2009). Mineralogy of Arctic bryozoan skeletons in a global context. Facies 55, 489–500. doi: 10.1007/s10347-009-0179-3
Lagger, C., Nime, M., Torre, L., Servetto, N., Tatián, M., and Sahade, R. (2018). Climate change, glacier retreat and a new ice-free island offer new insights on Antarctic benthic responses. Ecography 41, 579–591. doi: 10.1111/ecog.03018
Leese, F., Dömel, J. S., and Convey, P. (2015). Genetic data support independent glacial refugia and open ocean barriers to dispersal for the Southern Ocean sea spider Austropallene cornigera (Möbius, 1902). J. Crustacean Biol. 35, 480–490. doi: 10.1163/1937240X-00002351
Linse, K., Griffiths, H. J., Barnes, D. K., Brandt, A., Davey, N., David, B., et al. (2013). The macro- and megabenthic fauna on the continental shelf of the eastern Amundsen Sea, Antarctica. Cont. Shelf Res. 68, 80–90. doi: 10.1016/j.csr.2013.08.012
Loxton, J., Kuklinski, P., Barnes, D., Najorka, J., Spencer Jones, M., and Porter, J. S. (2014). Variability of Mg-calcite in Antarctic bryozoan skeletons across spatial scales. Mar. Ecol. Prog. Ser. 507, 169–180. doi: 10.3354/meps10826
Matson, P. G., Washburn, L., Martz, T. R., and Hofmann, G. E. (2014). Abiotic versus biotic drivers of ocean pH Variation under Fast Sea Ice in McMurdo Sound, Antarctica. PLoS ONE 9:e107239–e107212. doi: 10.1371/journal.pone.0107239
McClintock, J. B., Angus, R. A., Mcdonald, M. R., Amsler, C. D., Catledge, S. A., and Vohra, Y. K. (2009). Rapid dissolution of shells of weakly calcified Antarctic benthic macroorganisms indicates high vulnerability to ocean acidification. Antarct. Sci. 21, 449–456. doi: 10.1017/S0954102009990198
McNeil, B. I., and Matear, R. J. (2008). Southern Ocean acidification: a tipping point at 450-ppm atmospheric CO2. Proc. Natl. Acad. Sci. U.S.A. 105, 18860–18864. doi: 10.1073/pnas.0806318105
Morse, J. W., Mucci, A., and Millero, F. J. (1980). The solubility of calcite and aragonite in seawater of 35%. salinity at 25°C and atmospheric pressure. Geochim. Cosmochim. Acta 44, 85–94. doi: 10.1016/0016-7037(80)90178-7
Nyman, E. (2018). Protecting the poles: marine living resource conservation approaches in the Arctic and Antarctic. Ocean Coast. Manage. 151, 193–200. doi: 10.1016/j.ocecoaman.2016.11.006
Pabis, K., Hara, U., Presler, P., and Sicinski, J. (2014). Structure of bryozoan communities in an Antarctic glacial fjord (Admiralty Bay, South Shetlands). Polar Biol. 37, 737–751. doi: 10.1007/s00300-014-1474-1
Peck, L. S., and Barnes, D. K. A. (2004). Metabolic flexibility: the key to long-term evolutionary success in Bryozoa? Proc. R. Soc. B Biol. Sci. 271(Suppl. 3), S18–S21. doi: 10.1098/rsbl.2003.0053
Piepenburg, D., Buschmann, A., and System, A. D. E. (2017). Seabed images from Southern Ocean shelf regions off the northern Antarctic Peninsula and in the southeastern Weddell Sea. Earth Syst. Sci. Data 9, 461–469. doi: 10.5194/essd-9-461-2017
Pörtner, H. O., Peck, L., and Somero, G. (2007). Thermal limits and adaptation in marine Antarctic ectotherms: an integrative view. Philos. Transac. R. Soc. B Biol. Sci. 362, 2233–2258. doi: 10.1098/rstb.2006.1947
Post, A. L., Beaman, R. J., O'Brien, P. E., Eléaume, M., and Riddle, M. J. (2011). Community structure and benthic habitats across the George V Shelf, East Antarctica Trends through space and time. Deep Sea Res. Part II: Top. Stud. Oceanograp. 58, 105–118. doi: 10.1016/j.dsr2.2010.05.020
Post, A. L., Lavoie, C., Domack, E. W., and Leventer, A. (2017). Environmental drivers of benthic communities and habitat heterogeneity on an East Antarctic shelf. Antarct. Sci. 29, 17–32. doi: 10.1017/S0954102016000468
Pugh, P. J. A., and Convey, P. (2008). Surviving out in the cold: antarctic endemic invertebrates and their refugia. J. Biogeogr. 35, 2176–2186. doi: 10.1111/j.1365-2699.2008.01953.x
Roden, N. P., Shadwick, E. H., Tilbrook, B., and Trull, T. W. (2013). Annual cycle of carbonate chemistry and decadal change in coastal Prydz Bay, East Antarctica. Mar. Chem. 155, 135–147. doi: 10.1016/j.marchem.2013.06.006
Rowden, A. A., Kröger, K., and Clark, M. R. (2015). Compositional patterns of benthic assemblages on the northwestern Ross Sea shelf, Antarctica: interacting environmental drivers operating at multiple spatial scales. Hydrobiologia 761, 211–233. doi: 10.1007/s10750-015-2305-2
Schindelin, J., Arganda-Carreras, I., Frise, E., and Kaynig, V. (2012). Fiji: an open-source platform for biological-image analysis. Nat. Methods 9, 676–682. doi: 10.1038/nmeth.2019
Segelken-Voigt, A., Bracher, A., Dorschel, B., Gutt, J., Huneke, W., Link, H., et al. (2016). Spatial distribution patterns of ascidians (Ascidiacea: Tunicata) on the continental shelves off the northern Antarctic Peninsula. Polar Biol. 39, 863–879. doi: 10.1007/s00300-016-1909-y
Smith, A. M. (2014). Growth and calcification of marine bryozoans in a changing ocean. Biol. Bull. 226, 203–210. doi: 10.1086/BBLv226n3p203
Smith, A. M., Key, M. M. Jr., and Gordon, D. P. (2006). Skeletal mineralogy of bryozoans: taxonomic and temporal patterns. Earth-Sci. Rev. 78, 287–306. doi: 10.1016/j.earscirev.2006.06.001
Smith, W. O., Dinniman, M. S., Hofmann, E. E., and Klinck, J. M. (2014). The effects of changing winds and temperatures on the oceanography of the Ross Sea in the 21st century. Geophys. Res. Lett. 41, 1624–1631. doi: 10.1002/2014GL059311
Stark, J. S., Roden, N. P., Johnstone, G. J., Milnes, M., Black, J. G., Whiteside, S., et al. (2018). Carbonate chemistry of an in-situ free-ocean CO2 enrichment experiment (antFOCE) in comparison to short term variation in Antarctic coastal waters. Sci. Rep. 8, 2861. doi: 10.1038/s41598-018-21029-1
Taylor, P. D., James, N. P., Bone, Y., Kuklinski, P., and Kyser, T. K. (2009). Evolving mineralogy of cheilostome bryozoans. Palaios 24, 440–452. doi: 10.2110/palo.2008.p08-124r
Taylor, P. D., Lombardi, C., and Cocito, S. (2015). Biomineralization in bryozoans: present, past and future. Biol. Rev. 90, 1118–1150. doi: 10.1111/brv.12148
Teixidó, N., Garrabou, J., Gutt, J., and Series, W. A. M. E. P. (2004). Recovery in Antarctic benthos after iceberg disturbance: trends in benthic composition, abundance and growth forms. Mar. Ecol. Prog. Ser. 278, 1–16. doi: 10.3354/meps278001
Thatje, S., Hillenbrand, C.-D., and Larter, R. (2005). On the origin of Antarctic marine benthic community structure. Trends Ecol. Evol. 20, 534–540. doi: 10.1016/j.tree.2005.07.010
Waeschenbach, A., Taylor, P. D., and Littlewood, D. T. (2012). A molecular phylogeny of bryozoans. Mol. Phylogenet. Evol. 62, 718–735. doi: 10.1016/j.ympev.2011.11.011
Watson, S. A., Peck, L. S., Tyler, P. A., Southgate, P. C., Tan, K. S., Day, R. W., et al. (2012). Marine invertebrate skeleton size varies with latitude, temperature and carbonate saturation: implications for global change and ocean acidification. Glob. Change Biol. 18, 3026–3038. doi: 10.1111/j.1365-2486.2012.02755.x
Winston, J. (1983). Patterns of growth, reproduction and mortality in bryozoans from the Ross Sea, Antarctica. Bull. Mar. Sci. 33, 608–702.
Wood, A. C., Rowden, A. A., Compton, T. J., Gordon, D. P., and Probert, P. K. (2013). Habitat-Forming Bryozoans in New Zealand: their known and predicted distribution in relation to broad-scale environmental variables and fishing effort. PLoS ONE 8:e75160–e75131. doi: 10.1371/journal.pone.0075160
Wood, A. C. L., Probert, P. K., Rowden, A. A., and Smith, A. M. (2012). Complex habitat generated by marine bryozoans: a review of its distribution, structure, diversity, threats and conservation. Aquat. Conserv. Mar. Freshw. Ecosyst. 22, 547–563. doi: 10.1002/aqc.2236
Keywords: bryozoa, sea floor imaging, Ross Sea, Weddell Sea, Antarctic shelf
Citation: Santagata S, Ade V, Mahon AR, Wisocki PA and Halanych KM (2018) Compositional Differences in the Habitat-Forming Bryozoan Communities of the Antarctic Shelf. Front. Ecol. Evol. 6:116. doi: 10.3389/fevo.2018.00116
Received: 19 April 2018; Accepted: 20 July 2018;
Published: 14 August 2018.
Edited by:
Peter Convey, British Antarctic Survey (BAS), United KingdomReviewed by:
Rachel Victoria Downey, Australian National University, AustraliaJuan Ernesto Guevara Andino, Field Museum of Natural History, United States
Copyright © 2018 Santagata, Ade, Mahon, Wisocki and Halanych. This is an open-access article distributed under the terms of the Creative Commons Attribution License (CC BY). The use, distribution or reproduction in other forums is permitted, provided the original author(s) and the copyright owner(s) are credited and that the original publication in this journal is cited, in accordance with accepted academic practice. No use, distribution or reproduction is permitted which does not comply with these terms.
*Correspondence: Scott Santagata, c2NvdHQuc2FudGFnYXRhQGxpdS5lZHU=