- 1Facultad de Ciencias, Instituto de Ciencias Geológicas, Universidad de la República, Montevideo, Uruguay
- 2Facultad de Ciencias Exactas y Naturales, InGeBa, Universidad de Buenos Aires, Buenos Aires, Argentina
- 3Laboratorio de Sistemática e Historia Natural de Vertebrados, Facultad de Ciencias, IECA, Universidad de la República, Montevideo, Uruguay
- 4CR2P, UMR 7207, Centre National de la Recherche Scientifique/MNHN/UPMC, Sorbonne Universités, Paris, France
- 5Departamento de Paleontología, Facultad de Ciencias, Universidad de la República, Montevideo, Uruguay
Mesosaurs have been considered strictly aquatic animals. Their adaptations to the aquatic environment are well known and include putative viviparity, along with the presence of several skeletal characters such as a long, laterally compressed tail, long limbs, the foot larger than the manus, and presence of pachyosteosclerotic bones. They were also described as possessing non-coossified girdle bones and incompletely ossified epiphyses, although there could be an early fusion of the front girdle bones to form the scapulocoracoid in some specimens. Some of these features, however, are shared by most basal tetrapods that are considered semiaquatic and even some terrestrial ones. The study of vertebral columns and limbs provides essential clues about the locomotor system and the lifestyle of early amniotes. In this study, we have found that the variation of the vertebral centrum length along the axial skeleton of Mesosaurus tenuidens fits better with a semi-aquatic morphometric pattern, as shown by comparisons with other extinct and extant taxa. The present study allows us to suggest that whereas well-preserved mesosaur skeletons are mostly represented by juveniles and young adults that inhabited aquatic environments, more mature individuals might hypothetically have spent time on land. This is also supported, to an extent, by taphonomic factors such as the scarce representation and poor preservation of remains of mature individuals in the fossiliferous levels, and also by anatomy of the appendicular bones, and particularly the strongly ossified epiphyses and tarsus.
Introduction
Mesosaurs are widely thought to represent the earliest fully aquatic amniotes (MacGregor, 1908; Romer, 1966; Araújo, 1976; Oelofsen, 1981; Carroll, 1982; Laurin and Reisz, 1995; Modesto, 1996, 2006, 2010; Mazin, 2001; Canoville and Laurin, 2010; Villamil et al., 2015). They possessed a laterally compressed long tail, webbed limbs, long digits, a large foot (much larger than the manus), and nares placed far posteriorly from the tip of the snout, close to the eyes and fairly dorsal (MacGregor, 1908; von Huene, 1941; Piñeiro et al., 2016). All these characters can plausibly be considered adaptations to an aquatic lifestyle (Modesto, 1996; Piñeiro, 2004, 2008; Piñeiro et al., 2012a,c, 2016). Mesosaurs also developed pachyostosis (sensu stricto, Nopcsa, 1923; Houssaye, 2009) in the ribs and osteosclerosis in the humerus and presumably in other skeletal elements (Canoville and Laurin, 2010). They also have poorly ossified epiphyses of appendicular bones, at least in juvenile and early adult stages, suggesting that they were inhabitants of water bodies (de Ricqlès and de Buffrénil, 2001), most likely of shallow water because pachyostosis and osteosclerosis are passive buoyancy control adaptations to shallow water environments (de Ricqlès and de Buffrénil, 2001; Houssaye, 2009). Inferred viviparity or ovoviviparity in mesosaurs (Piñeiro et al., 2012a) is a typical reproductive strategy of aquatic amniotes as well (Angelini and Ghiara, 1984), though it could have originated in their terrestrial ancestors (Motani et al., 2014). Nevertheless, mesosaurs did not possess certain morphological adaptations present in ichthyosaurs, mosasaurs and plesiosaurs, such as reduction of the limbs, polydactyly, and polyphalangy, which are clear adaptations to live in open marine environments (Massare, 1988).
Mesosaurs may occupy a basalmost position in sauropsids, according to some recent phylogenetic (e.g., Laurin and de Buffrénil, 2016; Laurin and Piñeiro, 2017) and anatomical (e.g. Villamil et al., 2015; Piñeiro et al., 2016) studies. Others (Piñeiro et al., 2012a,b; Tsuji et al., 2012; Modesto et al., 2015) placed them as the basalmost parareptiles, but either way, the mesosaurid lifestyle is relevant to understand the evolutionary history of early amniotes.
Several studies have compared vertebral profiles of extant and extinct species and have shown patterns and convergences in this morphological trait as a result of similar locomotory behaviors (Fish, 1984, 1994; McShea, 1993; Buchholtz, 1998, 2001a,b, 2007; Buchholtz and Schur, 2004; Lindgren et al., 2007; Pierce et al., 2011; Felice and Angielczyk, 2014). In this paper, we test the supposed aquatic adaptations of Mesosaurus tenuidens by applying the vertebral centrum length methodology of Buchholtz (1998, 2001a,b) and by studying limb morphometrics of several extant and extinct taxa and various swimming styles that we compare in order to discriminate between semi-aquatic, aquatic and terrestrial lifestyles for mesosaurs. We also discuss mesosaurid ontogeny and the taphonomic factors (resulting in completeness and preservation quality of the specimens) that could have altered our results.
Materials
We examined a total of 50 skeletons: 40 of Mesosaurus tenuidens, four Hovasaurus boulei, two Thadeosaurus colcanapi, and four Claudiosaurus germaini (Figure 1). The other data were collected from the literature (see below).
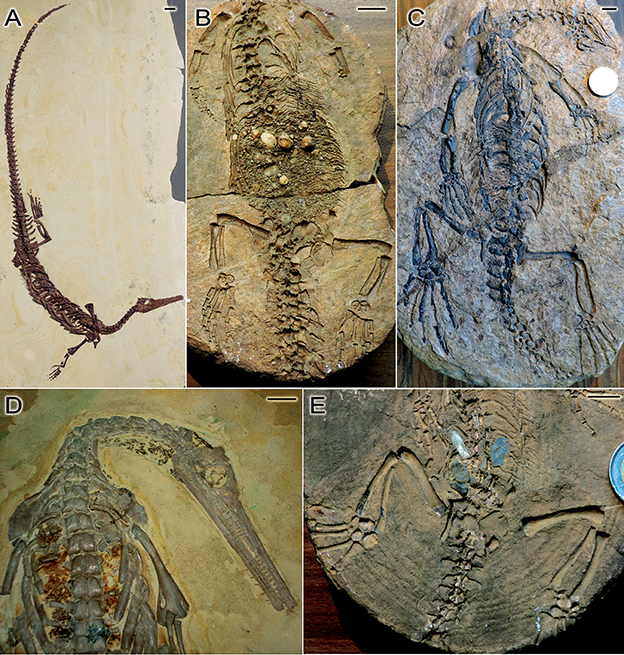
Figure 1. Taxa studied by direct specimen examination. (A) Mesosaurus tenuidens (PIMUZ A-III 591). (B) Hovasaurus boulei (MAP 1908-21-7). (C) Claudiosaurus germaini (MAP 1978-6-1a). (D) Mesosaurus tenuidens (GP/2E 669A). (E) Thadeosaurus colcanapi (MAP 1908-11-5a). All scales represent 10 mm.
The mesosaur specimens studied were all assigned to the species Mesosaurus tenuidens given that the observed phenotypic variability can be considered to reflect normal infraspecific variations. The specimens consist of well-preserved articulated individuals and partial skeletons, mostly featuring the axial skeleton (Figures 1A,D), belonging to the following paleontological collections: Fossil Vertebrates of the Facultad de Ciencias (FC-DPV), Montevideo, Uruguay; Instituto de Geociências (section Paleontology) of the São Paulo University (GP/2E), São Paulo, Brazil; Senckenberg Institute (SMF-R), Frankfurt, Germany; Institute of Paleobiology, Polish Academy of Science (ZPAL); Paleontological Institute and Museum, University of Zurich (PIMUZ), Suitzerland; Departamento Nacional de Produção Mineral, Museu de Ciências da Terra (DNPM), Rio de Janeiro, Brazil; Muséum national d'Histoire naturelle, Madagascan Permian (MNHN-MAP), and the American Museum of Natural History (AMNH), New York, USA.
Hovasaurus, Thadeosaurus, and Claudiosaurus were studied through direct examination and from photographs of the specimens housed at the Muséum National d'Histoire Naturelle of Paris, France (Figures 1B,C,E) and from drawings provided by Currie (1981b), Carroll (1981) and Currie and Carroll (1984). Profiles of basal synapsids and of extant diapsids were taken from Felice and Angielczyk (2014) for comparative purposes. Taxa used to construct Table S1 in Supplementary Material and perform the study on hind limb proportions were measured from the following sources: Stem Tetrapoda, and other stegocephalians of debated affinities (Marjanovi and Laurin, 2013), including temnospondyls and embolomeres (Romer, 1956; Carroll, 1970; Holmes, 1984, 2003; Godfrey, 1989; Panchen and Smithson, 1990; Sequeira and Milner, 1993; Smithson, 1993; Smithson et al., 1994; Lebedev and Coates, 1995; Coates, 1996; Clack, 1998; Ruta and Clack, 2006; Fröbisch et al., 2007); lepospondyls (Carroll, 1968; Carroll and Baird, 1968); Varanidae (Christian and Garland, 1996); phrynosomatid squamates (Herrel et al., 2002); Lacertidae Timon lepidus (Rewcastle, 1980); the earliest diapsid Petrolacosaurus (Peabody, 1952); the possible tangasaurid Kenyasaurus mariakaniensis (Harris and Carroll, 1977); Synapsids (Williston, 1910; Eaton, 1962; Reisz and Dilkes, 2003; Reisz and Fröbisch, 2014); various Permo-Carboniferous amniotes (Carroll, 1964, 1969; Fox and Bowman, 1966); Sauropterygians (Sues and Carroll, 1985; Rieppel, 1989; Caldwell, 1997a, 2002; Klein, 2012); Ichthyosauria (Carroll and Dong, 1991; Caldwell, 1997b); Mosasauridae (Caldwell, 1996).
Methods
We measured the centrum length for each available vertebra in the mesosaur skeletons. All measurements were taken on digital images (with a precision of less than 0.1 mm), all of the selected specimens have multiple centra articulated in sequence, or they are semi-articulated individuals where the position of each vertebra along the column was known, or could be determined.
Variations in vertebral length produce changes in the waves that are propagated through the column (Figure 2). When a wave passes through the body, each vertebra transmits the movement to the next one. If one vertebra is longer than the precedent, a bigger lateral displacement occurs, generating waves of higher amplitude and shorter wavelength (Figure 2E). On the contrary, if one vertebra is shorter than the precedent, a reduction in the amplitude is produced, as well as an increase in the wavelength (Figure 2D; Buchholtz, 2001b). Relative short centrum lengths are associated with column rigidity and stability through centrum shape changes and reduced joint mobility (Figures 2A–C) (Buchholtz, 1998, 2001a), while relatively long centrum lengths are related to column flexibility and enhanced mobility.
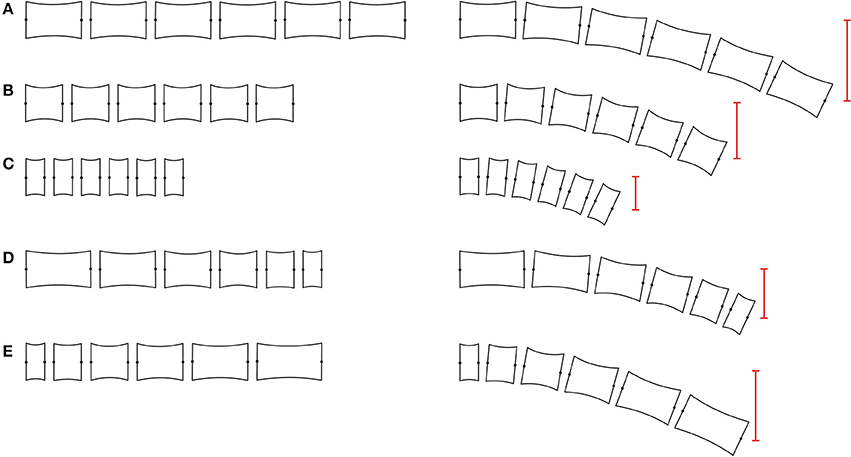
Figure 2. Vertebral column studied section. Models of different vertebral column sections (at the left) and the resulting vertebral amplitude after bending all the articulations five degrees (red line, right side). (A–C) vertebral amplitude of vertebral sections with homogeneous sized elements. Note that the bending amplitude depends directly of vertebral length. (D) vertebral section with progressive reduction in vertebral length. (E) vertebral section with progressive increment in vertebral length. The increment and reduction in bending amplitude is directly correlated with the vertebral length, increasing and decreasing respectively.
Of course, other non-osteological factors contribute to the flexibility of the spine such as the width of intervertebral disks, the interspinous ligaments, muscle architecture and size of the animal (De Smet, 1977; Long et al., 1997; Molnar et al., 2014, 2015).
In terrestrial locomotion, squamate trunks produce a standing or lateral traveling wave that bends the spine. The transmission of this wave is reflected with a localized increment in centrum length between the girdles, corresponding to the peak of the standing wave, where flexion and wave amplitude are higher (Ritter, 1992; Buchholtz, 1998; Felice and Angielczyk, 2014).
In aquatic locomotion, reptiles such as the marine iguana (Amblyrhynchus cristatus), as well as members of Crocodylia typically move with a carangiform undulation where lateral waves travel along the tail. In these taxa an increase in the caudal vertebral length is seen (Buchholtz, 1998; Felice and Angielczyk, 2014).
Fusion and reduction of cervical count and also reduction of vertebral length is frequently observed in the neck of aquatic and semi-aquatic mammals (Buchholtz, 2001a,b), and this feature increases in those species that developed a more aquatic adaptation. Some extinct taxa (like ichthyosaurs) share that characteristic (Buchholtz, 2001a), although plesiosaurs and their relatives do not, possibly because their propulsion mechanism is not axial, but appendicular.
It is expected that aquatic and semi-aquatic animals exhibit increments, peaks in caudal vertebral length, or plateau of constant vertebral lengths, corresponding to adaptations for generating or maintaining undulations of large amplitude, which are not found in terrestrial tetrapods (Buchholtz, 1998, 2001a; Felice and Angielczyk, 2014).
Measurement of Vertebral Centrum Length
As in previous works (Buchholtz, 1998, 2001a,b; Felice and Angielczyk, 2014), we plotted centrum length against vertebral position to obtain a vertebral profile for each specimen (Figures 3–6; see also Tables S2, S3 in Supplementary Material). Due to the variable number of vertebrae that can occur in the different sections of the column and due to the incompleteness of the mesosaurid specimens, all patterns were adjusted so that the sacral vertebrae are fixed in positions 35 and 36 in the plots. Thus, the longest complete mesosaurid has its first vertebra (atlas) in position 1, but some specimens can show different vertebral counts, particularly in the neck and possibly in the tail.
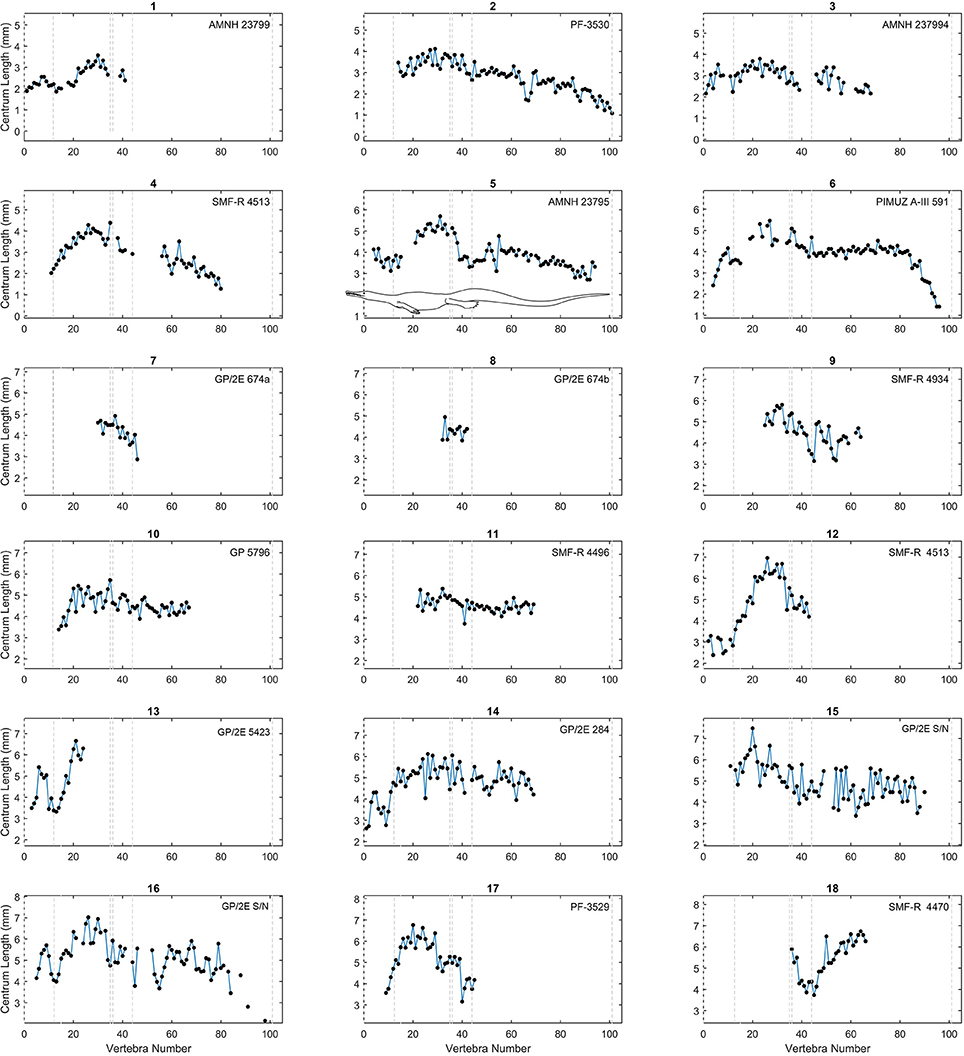
Figure 3. Centrum length profiles for Mesosaurus. Plots showing centrum length profiles for the Mesosaurus specimens sorted according their mean vertebral length. All patterns were adjusted so that the sacral vertebrae are fixed in position 35 and 36 in the plots. All graphs display the same scale in their y-axis (a 6 cm span of values). The dashed lines mark the approximate placement of the last cervical (12 or 13), the relative placement of the first thoracic vertebra (position 13 or 14), and of the two sacral vertebrae (positions 35 and 36), the end of the caudal lateral processes (position 44) and the assumed end of the tail (position 101). See the scaled mesosaurid representation of these limits in Figure 5. Raw data can be found in Table S2 (Supplementary Material).
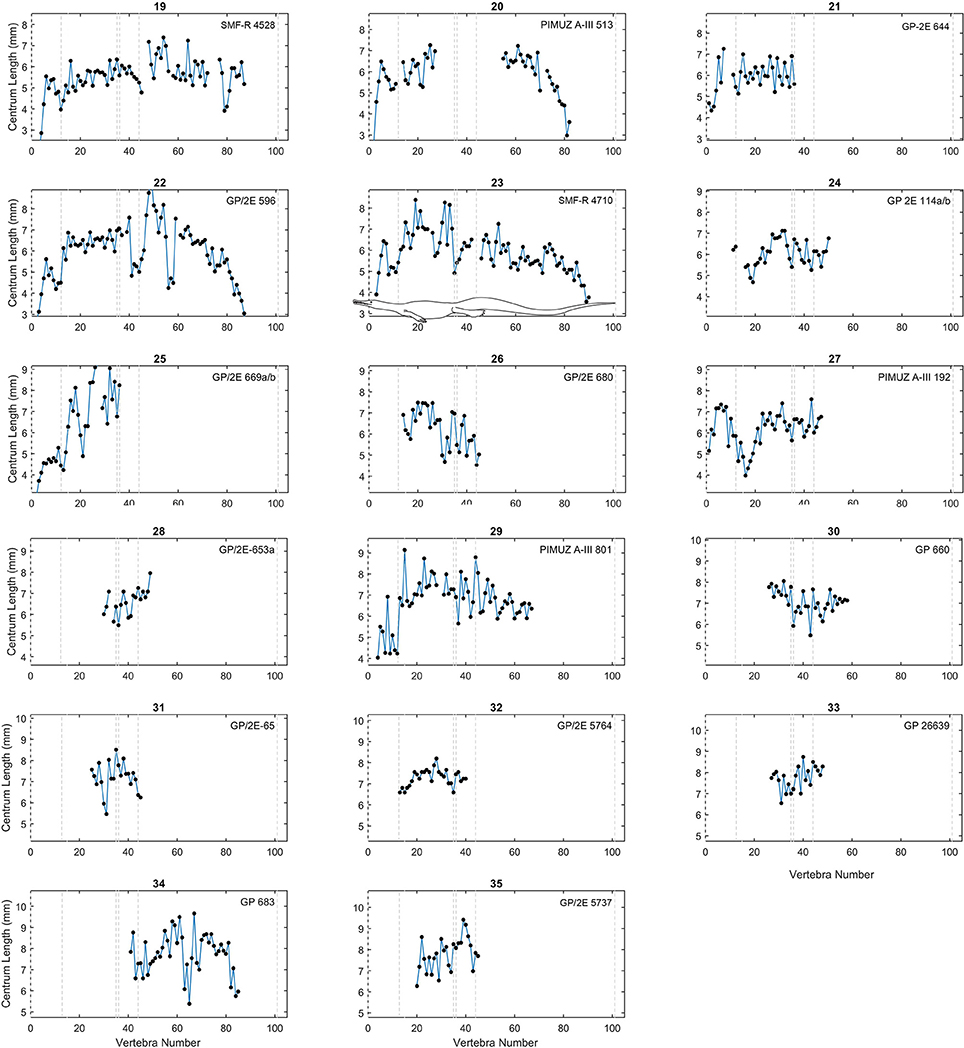
Figure 4. Centrum length profiles for Mesosaurus. Plots showing centrum length profiles for the Mesosaurus specimens sorted according their mean vertebral length (Figure 3). All patterns were adjusted so that the sacral vertebrae are fixed in position 35 and 36 in the plots. All graphs display the same scale in their y-axis as in Figure 3. The dashed lines mark the approximate placement of the last cervical (12 or 13), the relative placement of the first thoracic vertebra (position 13 or 14), and of the two sacral vertebrae (positions 35 and 36), the end of the caudal lateral processes (position 44) and the assumed end of the tail (position 101). See the scaled mesosaurid representation of these breaks is shown in graph 23. Raw data can be found in Table S2 (Supplementary Material).
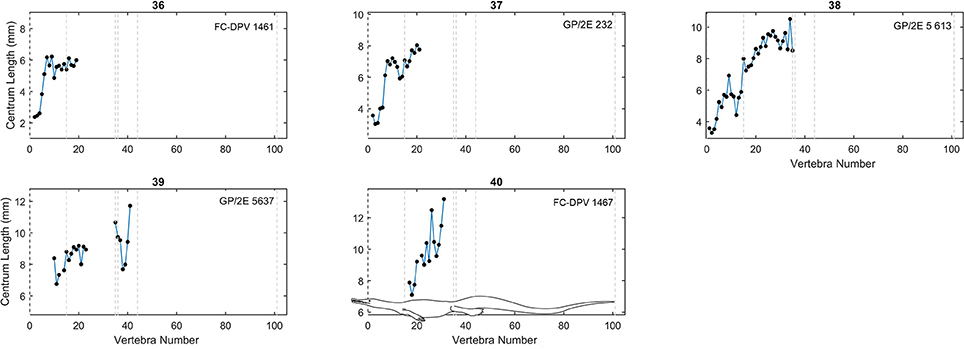
Figure 5. Centrum length profiles for Mesosaurus. Plots showing centrum length profiles for the Mesosaurus specimens sorted according their mean vertebral length (Figure 4). Specimens 36, 37, and 38 are not placed according its mean vertebral length, but considering the relative mean of the neck vertebrae. All patterns were adjusted so that the sacral vertebrae are fixed in position 35 and 36 in the plots. Contrary to the previous graphs (Figures 3, 4) these ones do not display the same scale in the y-axis. Dashed lines mark the approximate placement of the last cervical (12 or 13), the relative placement of the first thoracic vertebra (position 13 or 14), and of the two sacral vertebrae (positions 35 and 36), the end of the caudal lateral processes (position 44) and the assumed end of the tail (position 101). A scaled mesosaurid representation of these breaks is shown in graph 40.
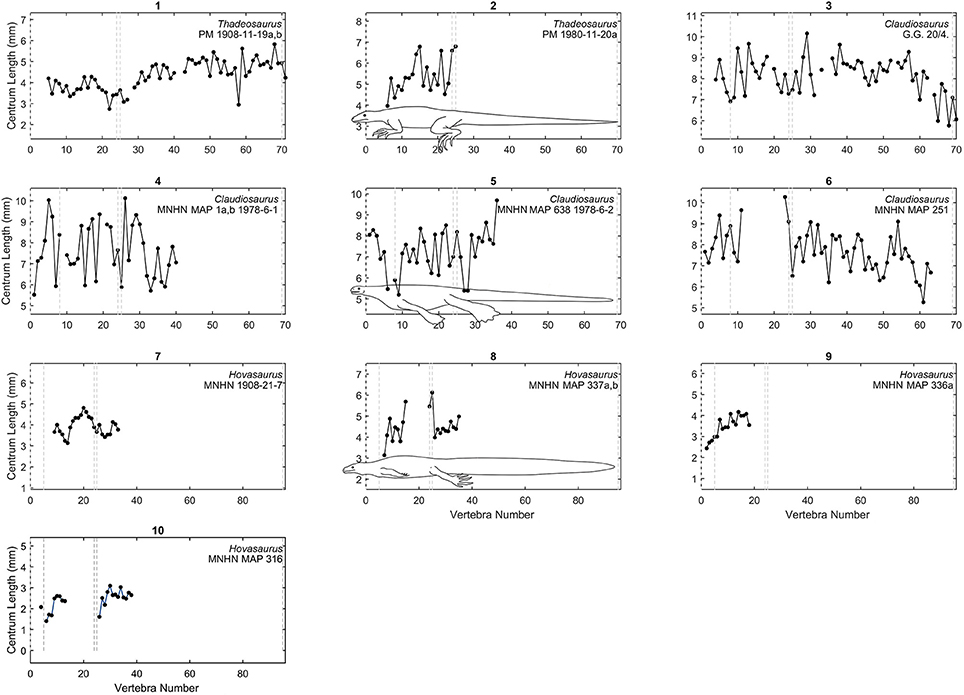
Figure 6. Centrum length profiles for Madagascar taxa. Plots showing centrum length profiles for Thadeosaurus, Claudiosaurus, and Hovasaurus. All the plots have the same vertical scale as in Figures 3, 4. The patterns were adjusted according to the vertebral descriptions of each species. Dashed lines mark the relative placement of the last cervical, the two sacral vertebrae, and the end of the tail as approximately shown by the species representation in the graphs 2, 5, and 9. Raw data are provided in Table S3 (Supplementary Material).
We compared our mesosaurid vertebral profiles with data of other extant and extinct diapsids and extinct synapsids provided by Felice and Angielczyk (2014): Alligatoridae (Caiman crocodylus- spectacled caiman, Melanosuchus niger- black caiman), Crocodylidae (Crocodylus rhombifer- Cuban crocodile), Gavialidae (Gavialis gangeticus- gharial), Iguanidae (Amblyrhynchus cristatus- marine iguana, Iguana iguana- green iguana), Varanidae (we sampled the same species as Felice and Angielczyk, 2014), Varanus bengalensis - Bengal monitor, V. dumerilii- Dumeril's monitor, V. exanthematicus-savannah monitor, V. komodoensis- Komodo dragon, V. rudicollis- roughneck monitor, for characterization of the terrestrial pattern and V. salvator- water monitor for the semiaquatic one), the basal diapsids Hovasaurus boulei, Thadeosaurus colcanapi and Claudiosaurus germaini basically from the Late Permian, but recently also found in Early Triassic deposits of Madagascar (cf. Ketchum and Barrett, 2004), and Caseidae (Casea broilii, Cotylorhynchus hancocki), Varanopidae (Varanops brevirostris), Ophiacodontidae (Ophiacodon retroversus). The selection of the extinct taxa was based on their grade of completeness to obtain the most robust and accurate profiles. Moreover, the selection of the extant taxa for comparison with mesosaurids was based on comparable skeletal anatomy, size, and on their observed locomotory specializations, covering species with aquatic, semi-aquatic and terrestrial life styles.
We measured the parameters developed by McShea (1992). The metric range (R), polarization (C), irregularity (Cm), concentration (E1), and smoothness (E2), were calculated for each specimen. However they could not be compared with more recent data (e.g., McShea, 1993; Buchholtz, 2001a; Felice and Angielczyk, 2014) because the original metric was modified, customizing the equations and thus making the comparisons operose, impractical and meaningless. The range R represents the logarithm of the difference between the maximum and minimum vertebral length in each specimen. The parameter C reflects the polarization of the data. The concentration E1 is the difference between R and C. As this parameter is the subtraction of two logarithmic operations, it results in normalization independent of the size. Cm is the mean of the distances between adjacent elements. The smoothness E2 is the subtraction of two logarithmic operations, resulting in a normalized parameter independent of size. E2 is the difference between C and Cm. The mathematical significance of applying these vertebral parameters to different species should be taken with caution because they are interrelated and are size- and noise-sensitive. Logarithmic size corrections (before or after calculations) may distort the statistical patterns to be analyzed, alter relationships among parameters, and consequently their interpretation. Thus, our parametric analysis was performed only within one species (the Mesosaurus specimens) in order to observe intraspecific variations.
The mean vertebral length was obtained for each specimen. Using this mean, the total length of the observed vertebral sections was calculated (mean value × number of vertebrae in the section) and compared with the real measurements. The comparison has a mean of zero with a three sigma deviation of 4 mm for Mesosaurus; in other words, the theoretical length of a Mesosaurus section, based on the mean vertebral length, almost does not differ from the real one. Calculations of the total section lengths using only ten vertebrae lead to a mean three sigma deviation of 60 mm. Meanwhile, increasing the vertebrae to 20 (when available) reduces the deviation to 6 mm. Considering the result obtained with mesosaurs sections, we extrapolated the theoretical total length for each specimen, using the mean vertebral length and assuming a total count of 101 vertebrae, as a first approximation to the real size of each specimen. As a simple observation reveals that calculation using only or mostly cervical and caudal vertebrae tend to underestimate mesosaurid length, and using only dorsal vertebrae tends to overestimate this length. Results using only 10 vertebrae can lead errors of up to 30 cm, whereas if more than 20 vertebrae are used, including more than one region of vertebral column, the total length errors can be reduced approximately to 6 cm.
As the mean length can be biased by the number of vertebrae present in each specimen and also by the body region which these vertebrae represent, we also tested the possibility of using the length of the sacral vertebrae to estimate the Mesosaurus body length. However, the section length estimation using the sacral vertebrae differs from real measurements by a range between 30 and 120 mm (N = 20) and the expected error in the estimation of the total body length is up to 25 cm. Consequently, we realized that the most reliable unit to estimate the size of incomplete skeletons in morphometric analyses is the mean centrum length, rather than the sacral vertebral length, on the condition that 20 or more vertebrae are used to estimate the mean. Comparing that measure to femur length, when available, provide a more reliable estimation of the body length for the 40 mesosaurid specimens studied herein.
The plots were normalized, using the mean vertebral length, in order to eliminate size effects and a mean pattern was calculated in order to observe the general pattern in Mesosaurus' vertebral column. Also, normalized patterns were used to provide a more trustworthy basis for interspecific comparisons and to overcome size differences.
As in almost all paleontological studies, it is very likely that some of the data obtained contain noise and errors introduced by differences in completeness, ontogeny and taphonomic artifacts such as rotation and deformation (McShea, 1993; Buchholtz, 2001a; Felice and Angielczyk, 2014).
Taphonomic Constraints for Mesosaurus Vertebral Measurements
The specimens were preserved as impressions, molds, casts or permineralized, half-buried bones embedded in a calcareous matrix. Due to this kind of preservation, and the difficulties in preparing the specimens, 3D measurements could not be taken accurately. However, vertebral rotation and superposition is an intriguing frequent preservation condition observed in the trunk column section of even perfectly articulated specimens. The vertebral distortion, which involves also the disarticulation of their corresponding ribs, is found particularly at the level of the chest and the abdominal cavity, being thus possibly related to carcass explosion after the decay of viscera (Keller, 1976 apud Reisdorf et al., 2012, 2014). Whether the specimen is preserved in dorsal or ventral view, when a segment of two, three or four vertebrae are preserved in different positions including dorso-lateral to lateral and ventro-lateral views, that displacement always implies the distortion of the ribs in the affected segment (Figure 7). This taphonomic condition can be misinterpreted as a pathological trait rather than a taphonomical feature, as was recently described in a well-preserved and almost complete skeleton assigned to Stereosternum tumidum (Szczygielski et al., 2017) without any rigorous anatomical and taxonomic analyses.
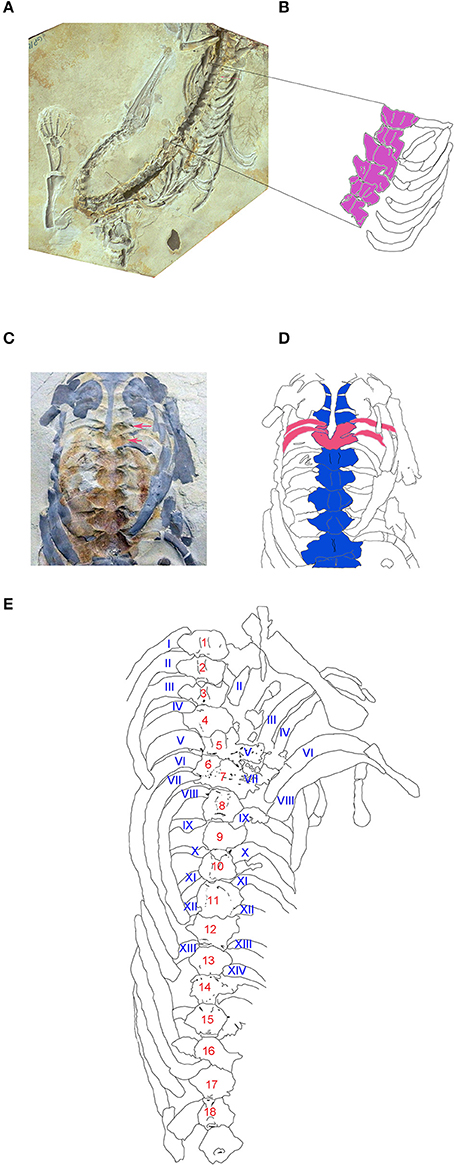
Figure 7. Taphonomic constraints to vertebral measurements (see text for additional information on this issue). (A) GP/2E 644, photograph of a well preserved incomplete mesosaurid specimen showing rotation of vertebral centra from lateroventral to ventral position (red arrow). (B) Schematic drawing of the vertebral dorsal segment of A where the vertebral rotation was gradually produced. (C) GP/2E 661, photograph of an incomplete, partially articulated individual showing vertebral overlap (red arrows). (D) Schematic drawing of C, remarking the involved overlapped dorsal vertebrae and the corresponding two pairs of ribs (in red). (E) Schematic drawing of partial trunk segment of the specimen ZPAL R VII/1 thought by Szczygielski et al. (2017) to possess the pathology known as hemivertebra. The supposed pathology seems to be an overlapping of vertebrae here numbered as 6 and 7 to show the correspondence between vertebrae and ribs from the right and left sides.
In consequence, we did not measure widths and heights since these measures are difficult or in some cases impossible to obtain on disturbed centra. Some dorsal measurements have to be considered as estimated, because they were taken over the neural arch rather than on the centrum.
Morphometric Study of Limb Proportions
We also studied the hindlimb proportions of Mesosaurus, Claudiosaurus and Hovasaurus, and several other extinct and extant species (Table S1 in Supplementary Material). According to some workers, there is a well-established relationship between morphology of hindlimbs and forelimbs and the locomotion style, which may implies a terrestrial, semi-terrestrial or aquatic lifestyle (e.g., Herrel et al., 2002; Joyce and Gauthier, 2004). We chose the hind limb because it is better preserved in mesosaurids and the other studied taxa. Locomotor changes have been observed in many evolutionary transitions, and, for example, a strong correlation has been observed between the hindlimb morphology and the lifestyle during phrynosomatid squamate evolution (Herrel et al., 2002). We measured femur and tibia length, and length of the longest toe of the hind foot, equivalent to the sum of phalanx length and metatarsal length. Many lengths were measured directly on specimens (e.g., mesosaurids and the Madagascan diapsids); the others were obtained from photographic material or from already published reliable reconstructions. Only specimens preserving all the segments that we measured were included into the analysis. Hindlimb proportions were then computed to find the rate values of tibia length over femur length and longest toe length over femur length for each taxon.
The diagrams that we produced allow comparing leg proportions, but some caution is necessary. Each species is represented by a point in the plot, which represents a mean value. For a precise analysis, the average proportions for each species should be calculated carefully taking into account ontogeny and sexual dimorphism. However, these features are difficult to infer from extinct species as they are typically represented by just one or few, often incompletely preserved specimens. As an example, of more than 40 studied mesosaurid specimens belonging to collections from around the world, only 4 specimens have the entire series of phalanges on their longest digit. Despite this, dispersion in the Mesosaurus, Hovasaurus, and Claudiosaurus hind limb ratios was found to be less than 0.2. Thus, we believe that despite the mentioned difficulties, this study, as a first approximation, has produced useful results.
Results
Mesosaurid Vertebral Column
The main difficulty in getting trustworthy total vertebral counts in mesosaurid vertebral columns lies in establishing the number of cervical and caudal vertebrae. This is becacuse the neck or the tail are not always completely preserved, and when they are, the first cervical vertebrae can be hidden under the skull and some cervical vertebrae are usually hidden by the pectoral girdle; the tail is completely preserved only in exceptional cases (Figure 1).
Some individuals gave abnormal profiles. This includes partially disarticulated specimens (the order of their vertebrae may have been misinterpreted) or for instance, specimen 5 (Figure 3), which has shorter vertebrae in the middle of the neck. However, we could not verify statistical evidence supporting two populations among the plotted mesosaurids, regarding the vertebral length pattern.
The vertebral length profiles of Mesosaurus are shown in Figures 3–5, ordered by their mean vertebral length; while those for the Madagascan diapsids are shown in Figure 6. All the patterns have the same scale (a 5 cm span of values, except Figure 5), facilitating the comparison between individuals and making it possible to distinguish them by size and completeness. The data show that in the cervical region, vertebrae of almost all the measured specimens, including juveniles and adults, are longest at the center of the neck. The trunk vertebrae increase their length progressively, forming a peak or a plateau toward the middle of the dorsal section (Figure 3, specimens 1 and 16; Figure 5, specimens 26 and 32) or in the posterior half of the trunk (Figure 3, specimens 5, 12; Figure 5, specimen 38). Two specimens instead show a peak in the anterior half of the trunk (Figure 3, specimen 15; Figure 4, specimen 26). Caudal vertebrae of some individuals show an increment in length posteriorly with a peak either around vertebrae 50–55 (Figure 3, specimens 3 and 5), or near the tail tip (Figure 3, specimen 18), and in others a plateau is seen (Figure 3, specimens 2, 6, and 11), maintaining the vertebral length along the first two thirds of the tail. The tail tip remains almost unknown because few specimens preserve it completely (Figures 3–6). Nevertheless, the distal portion of the tail shows a smoothly continuous decrease in vertebral length to the tip.
Mean Vertebral Length and Mesosaurus Size
The comparison between the total length of the observed vertebral sections and the one calculated using the mean centrum length for each specimen is shown in Figure 8A, while the estimated total length of each Mesosaurus specimen, with a total vertebral count of 101, is provided in Figure 8B.
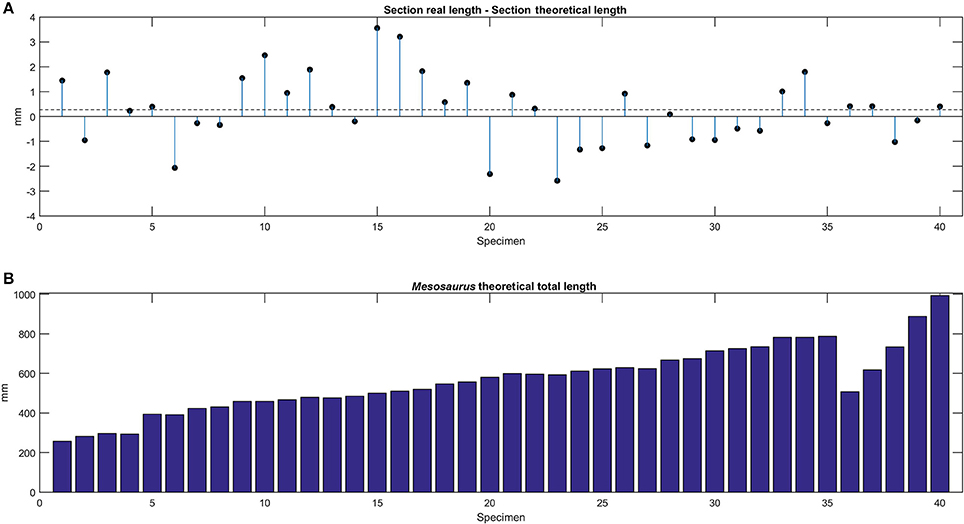
Figure 8. (A) Theoretical and estimated total length of studied specimens. Difference between the real length measured in the section preserved and the theoretical length calculated using the mean centrum length, for each specimen. (B) Theoretical total length estimated for each specimen of Mesosaurus. All the specimens were ordered by their mean vertebral length except for the specimens 36, 37, and 38; see also Figure 5.
The Parameters and Their Relation With the Specimen Size
The study performed on the vertebral parameters R, C, Cm, E1, E2 for Mesosaurus tenuidens is shown in Figure 9. The range R slightly increases with the size of the specimen. The parameter C reflects the polarization of the data, the closer C is to the value of R, the more polarized are the data, or in other words, they are less concentrated over the media. The formula of this parameter (C) is linked with the standard deviation and both exhibit the same pattern, except for a slight increment proportional to the size of the specimens that affect the parameter C. The concentration E1 results in normalization independent of size; thus, as expected, E1 shows no correlation with size increment, but features an oscillatory lifestyle with values close to 1. This can reflect a link to a real pattern behind size and distortion effects. The irregularity of Cm reflects how much variation is observed through the vertebral column. Cm is strongly related to the size of the specimens, meaning that longitudinal variation increases with the size of the mesosaurid body. E2 is inversely related to E1 as reflected in the graphics. We suggest that E1 and E2 reflect the random distortion that occurred in the bones; their proximity in values is a consequence of the real bone relations subjacent to the distortions.
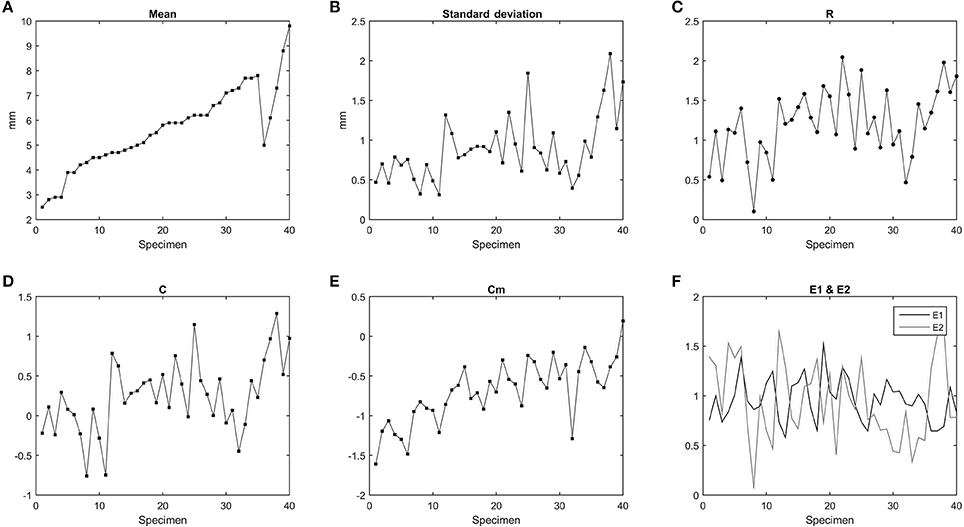
Figure 9. Statistical parameters related to each Mesosaurus tenuidens specimen according to the equations of McShea (1992). (A) Mean. (B) Standard deviation. (C) Range (R). (D) Polarization (C). (E) Irregularity (Cm). (F) Concentration (E1) and smoothness (E2).
The study of the vertebral parameters R, C, Cm, E1, E2, reflects and confirm what we have noted through direct observation of the specimens: the smallest individuals present less noise and smoother patterns and the completeness of the specimens decrease with the increment of size.
Vertebral profiles obtained from Mesosaurus are fairly constant among all individuals studied and normalization seems to reveal a general pattern. Nevertheless, larger specimens appear to show more extreme length values, even though the preservation quality is poor in these samples. As mentioned above, although some mesosaurids display abnormal profiles, visual examination of the data shows plainly that only one species is statistically supported.
A Comparative Profile of the Analyzed Vertebral Column in Extinct and Extant Species
The comparison between extant and extinct taxa is shown in Figures 10, 11. All patterns were re-scaled in their x-axis, so that the trunk of all individuals has the same length. Patterns of some species were averaged with the same method as for Mesosaurus, when more than one specimen was available. All patterns, including those obtained from data of Felice and Angielczyk (2014), were normalized with their mean centrum lengths so the y-axis reflects the variation in length with respect to their mean vertebral lengths.
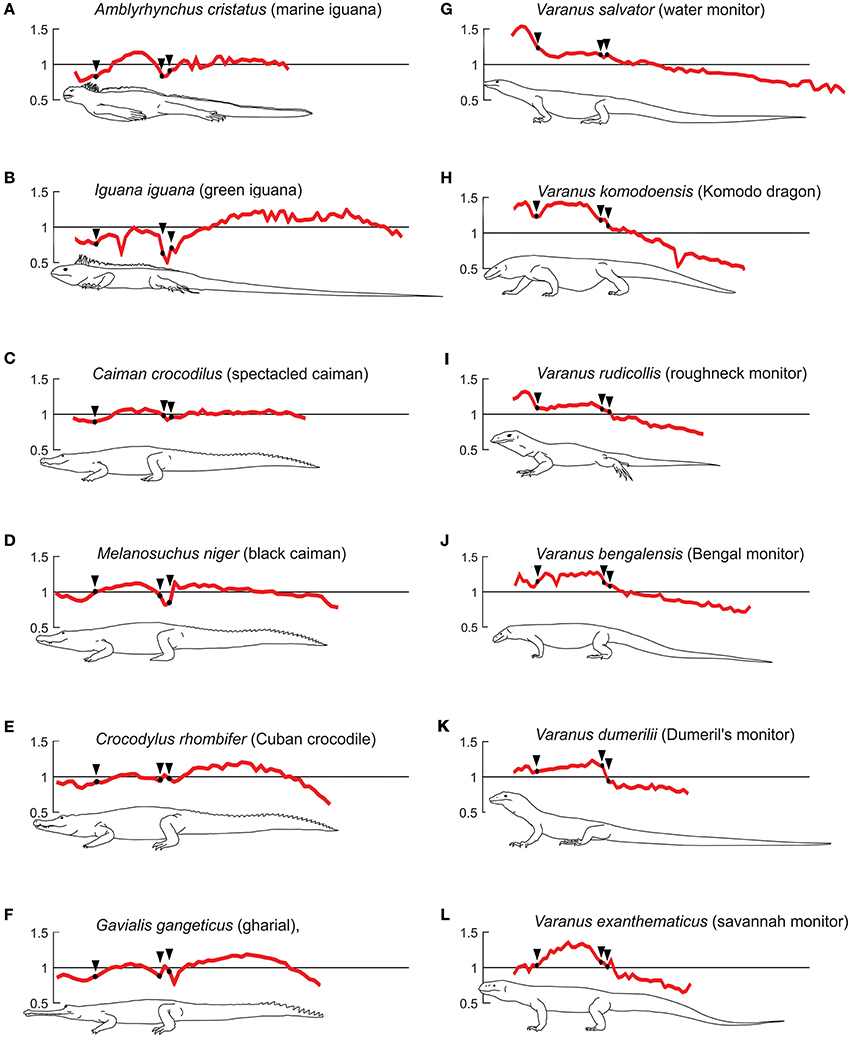
Figure 10. Semiaquatic and terrestrial profiles for extant taxa. Plots showing centrum length profiles for different semi-aquatic (A–F) and terrestrial (G–L) extant species. All patterns were normalized with their mean centrum length so the y-axis reflects the variation in length with respect to its mean vertebral length. The x-axis was re-scaled, so that the trunk-length of all individuals is represented by the same length in the plot. Black arrows mark the beginning of the chest and the first sacral vertebra. The pattern for Caiman crocodilius, Varanus bengalensis, and Varanus salvator are normalized average curves of the 3, 2, and 4 available specimen data. Data adapted from Felice and Angielczyk (2014).
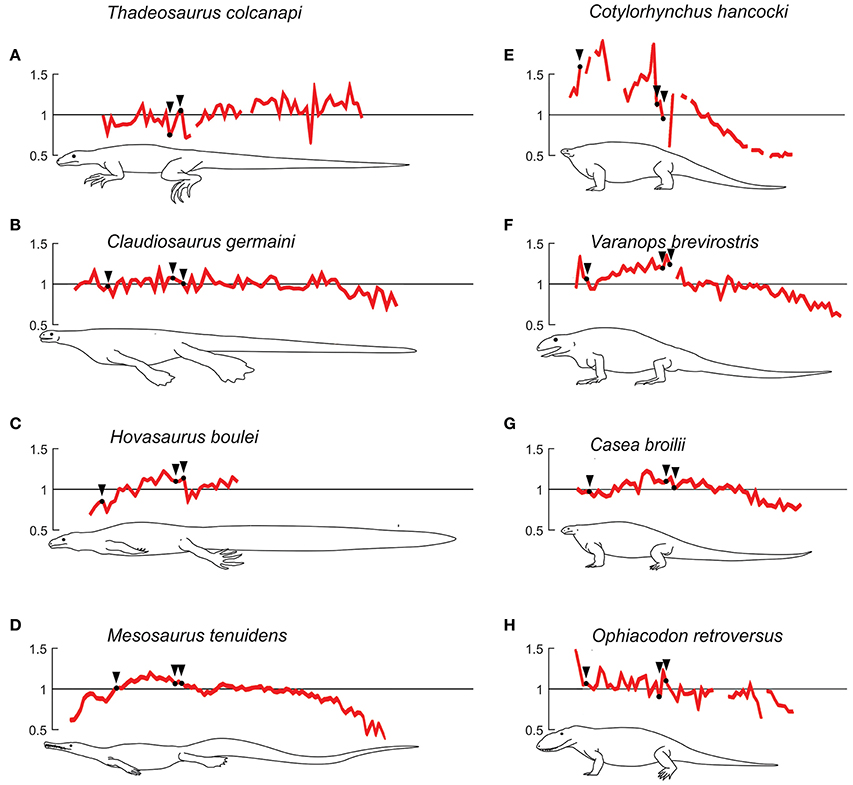
Figure 11. Semiaquatic and terrestrial profiles for extinct taxa. (A–D), taxa regarded to have developed a semiaquatic lifestyle. (E–H), taxa that represent the terrestrial type, although note that Ophiacodon's lifestyle is discused (see text). Plots showing centrum length profiles for different semi-aquatic (to the left) and terrestrial (to the right) extinct species. All patterns were normalized with their mean centrum length so the y-axis reflects the variation in length with respect to its mean vertebral length. All patterns are re-scaled, so that the trunk of all individuals has the same length. Black arrows mark the beginning of the chest and the first sacral vertebra. The patterns of Mesosaurus tenuidens, Hovasaurus boulei, Thadeosaurus colcanapi, Claudiosaurus germaini, and Cotylorhynchus hancocki, are normalized average curves obtained with data of the number of specimens available, respectively 40, 4, 2, 4, and 2. Data on synapsids were adapted from Felice and Angielczyk (2014).
The Madagascan Late Permian and Early Triassic Taxa
Centrum length profiles of stem diapsids Claudiosaurus, Thadeosaurus, and Hovasaurus are shown in Figure 6. The patterns were adjusted following previous vertebral descriptions of each species.
The pattern of Thadeosaurus (Figure 6, specimen 1) resembles that of Iguana iguana, because the vertebral length increases distally in the proximal caudal region.
In our vertebral profiles, Claudiosaurus shows one of the noisiest patterns and it is difficult to interpret from the graphs, but there is a peak in the cervical region derived from a progressive enlargement of the neck vertebrae, possibly toward the middle section (Figure 6, specimens 3,4,6) or near the head (Figure 6, specimen 5). Its mean vertebral length is approximately twice that of Hovasaurus, and is similar to the largest Mesosaurus. However, contrary to the largest Mesosaurus, the thoracic and caudal vertebrae of Claudiosaurus appear to maintain their relative size across the column, and become shorter toward the end of the tail.
Unfortunately, the analyzed specimens of Hovasaurus do not show a very clear pattern due to their incompleteness, but it seems that at least one peak is present in the middle of the thoracic region (Figure 6, specimens 7-10) and the proximal segment of the tail shows a clear continuous increment in vertebral length.
Earliest Basal Synapsids
The plots for the Permo-Carboniferous synapsids included in this study for comparative purposes are shown in Figures 11E–H. Cotylorhynchus, Varanops and Casea present a maximum in their vertebral profiles in the trunk, from which the vertebral length decreases toward both ends of the spine. Varanops also shows a maximum in the cervical region comparable to the trunk one and Cotylorhynchus displays two distinct peaks, in the anterior and posterior trunk. All these taxa show a continuous decrease toward the tail tip. In Cotylorhynchus this reduction is more abrupt, while in Varanops and Casea the vertebral length remains almost constant in the proximal portion of the tail. Ophiacodon has a very noisy pattern, with irregular values; it has its maximum vertebral length in the cervical region; however a general posteriorly decreasing trend is clear until the end of the tail. In all the sampled Permo-Carboniferous synapsids, the caudal vertebrae are shorter than the dorsal ones.
Extant Taxa
In the six sampled Varanus species, the presacral vertebrae are longer than the caudal vertebrae. Vertebral length also shows a steady decline in length toward the tail tip. Their caudal vertebrae are not longer than the shortest trunk vertebrae; even their cervical vertebrae are longer than the caudal ones. Also, all the species present a vertebral length increment in the middle portion of the neck.
The vertebral length profiles of the Varanus species examined display a continuum between extremes represented by V. salvator and V. exanthematicus. V. salvator displays its longest vertebrae forming a pronounced peak in the neck and a plateau in the trunk. V. rudicollis shows a very similar pattern. On the other end of the cline, V. exanthematicus shows a peak in maximum vertebral length between the girdles; all the other vertebrae decrease continuously in size toward both extremes of the spine. The other Varanus species present intermediate patterns in their dorsal regions.
The profiles of some of the analyzed taxa, such as Amblyrhynchus, Caiman, Melanosuchus, Crocodylus, and Gavialis show weaker gradients in the vertebral mean length throughout the column. Amblyrhynchus displays a peak among the dorsal vertebrae; its cervical vertebrae are shorter than the mean, even shorter than the dorsal ones. In contrast to Varanus, the neck of these taxa tends to present a minimum in vertebral length in its middle section. All these taxa show a maximum in vertebral centrum length in the dorsal series (slightly closer to the hip) (Figures 10A–F). Additionally, their pattern differs from that observed in Varanus in the sacral region where a local minimum is registered; pre- and post-sacral vertebrae are longer than the sacral ones. The caudal vertebral length exhibits two main patterns, one characterized by Caiman, Melanosuchus (Alligatoridae), and Amblyrhynchus, in which the vertebral length is homogeneous along the tail, and equal to the mean length, and the other characterized by Crocodylus (Crocodylidae) and Gavialis (Gavialidae), which show a vertebral length increment in the proximal part of the tail, with a maximum at the central region, and progressive decrease toward the end. The caudal vertebral length is only shorter than the mean vertebral length at the very end of the tail, in contrast with the terrestrial species in which all the caudal vertebrae are shorter than the mean length.
Among the extant taxa studied, the six Varanus species are all basically terrestrial (Thompson and Withers, 1997); although V. salvator seems to have more preferences for remain more time into the water (De Lisle, 2007), all varanid species can indeed swim, as they also develop arboreal specializations. V. rudicollis inhabits rainforests as an arboreal species (Horn, 2004), they spend almost all their life on land. Both species display their longest vertebrae in the neck and a plateau in the trunk, but none of the Varanus species show enlarged caudal vertebrae. They spend almost all their life on land and they even display a little dorsal skin-fold along their tail.
Some of the extant taxa analyzed, such as Amblyrhynchus, Caiman, Melanosuchus, Crocodylus, and Gavialis show profiles opposed to those of Varanus, particularly among the cervical vertebrae. This length reduction is possibly associated to their adaptation to aquatic environments (Buchholtz, 2001b). Nevertheless, they display a maximum in dorsal vertebral length toward the hip, which Buchholtz (1998) and Felice and Angielczyk (2014) suggest corresponds to the area of lateral undulation during terrestrial locomotion. The enlarged vertebrae in the tail represent the region where traveling undulatory waves pass along the tail during swimming (Buchholtz, 1998), and the differences between a plateau (Alligatoridae) and a peak (Crocodylidae and Amblyrhynchus) reflect the places where the transmitted waves may be amplified (Buchholtz, 1998; Felice and Angielczyk, 2014). It also reflects their behavior: although all extant crocodilians are semiaquatic and all have fixed vertebrae numbers, Crocodylidae are more aggressive and better predators (mainly on big prey), while Alligatoridae are not aggressive and prey on smaller preys.
The vertebral profile of Iguana iguana appears to differ strongly from the other extant taxa with smaller presacrals, cervicals that are not shorter than sacrals, and a tail pattern that resembles that of Crocodylus and Gavialis proximally, even though it maintains its length distally. It is also known that Iguana iguana possesses swimming capabilities as most other squamates and even though it is a terrestrial animal, it never moves far away the water sources (Morales-Mávil et al., 2007; Escobar et al., 2010). In its profile, Iguana resembles more the marine iguana than the tree dwelling Varanus rudicollis, and this reflects both taxonomy (Iguanidae vs. Varanidae) and differences in vertebral counts.
Iguana iguana and Varanus salvator, like many other squamate species, are known to use the tail as a whip-like weapon (Murphy and Mitchell, 1974); considering the differences observed in patterns of both species, we can infer that this lifestyle does not leave a clear signal in vertebral length profiles.
As pointed out in almost all the species studied, either extinct or extant, there is a length peak among the trunk vertebrae. As it appears in species of very different lifestyles, it may be a common attribute which obviously responds to the need these animals have to perform lateral undulation both during terrestrial and aquatic locomotion. Considering the plots for extant species, for which life habits are known (see Table S1 in Supplementary Material), we suggest that two patterns can be used to infer terrestrial and aquatic or semi-aquatic habits in extinct taxa:
A- In terrestrial animals: cervical vertebrae are longer than caudal vertebrae, and generally they are some of the longest in the spine. The longest cervical vertebrae are placed at the middle of the neck. Dorsal vertebrae either display a maximum or maintain the length almost constant. Caudal vertebrae are the smallest and decrease their length continuously toward the tail tip. Extant examples are most Varanus species; even V. salvator has cervical vertebrae longer than caudals.
B - In semi-aquatic species: cervical vertebrae are among the shortest ones, but the longest of this series are placed either at both ends of the neck or toward the head. Dorsal vertebrae display at least one peak, with values closest to the mean vertebral length. A local minimum is present in the sacral region. Caudal vertebrae either maintain their length almost constant over the tail, or may present at least one length peak. This pattern is represented by the crocodilians and Amblyrhynchus among extant taxa.
Femur/Tibia and Femur/Pes Ratios Plots (Figure 12)
The extant Varanus species and the phrynosomatid squamates fall at the same region in the graph. Most of these species have a tibia/femur ratio between 1:1 and 3:4 (0.75), while all had pes/femur ratio ranging between 1:1 and 2:1. That means that their tibia is not longer than the femur, and their pes is at least as long as their femur. Also, their pes is larger than their tibia but never more than twice the size of the latter. Some plotted species are characterized by their terrestrial lifestyle, like the selected varanids (Christian and Garland, 1996) and other squamates, which are represented by tree- and rock-climbers or ground-dwelling species (Herrel et al., 2002).
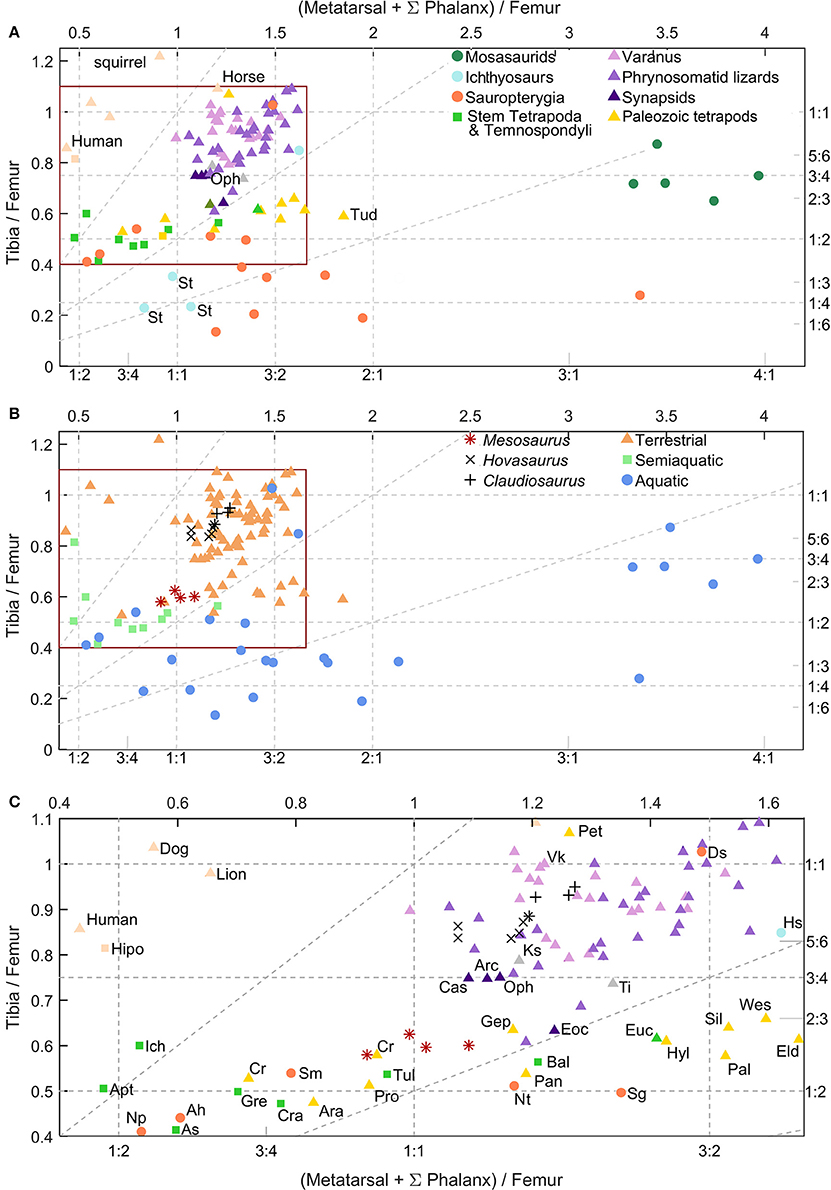
Figure 12. Hind limbs proportions; tibia/femur ratio vs. pes/femur ratio of different extant and extinct species. Selected mammals are plotted in pink as reference. Pes measurement is the sum of the length of phalanges and metatarsal of the longest toe. (A) Graph distinguishing species group. (B) Graph distinguishing observed or inferred species ecology. (C). Detail of the inset from (A,B). Axis display ratio values as decimals and fractions. Oblique dashed lines represents tibia/pes ratio, from left to right: 1:1, 1:2, 1:4. All the species are represented by the measurement of one individual except the varanids that represent the mean of several individuals; (see sources in main text). Mesosaurus, Hovasaurus, Claudiosaurs, Captorhinus, and Stenopterygius have more than one individual plotted. Stem Tetrapoda & Temnospondyli: [As], Acanthostega; [Apt], Apateon; [Gre], Greererpeton burkemorani; [Is], Ichthyostega; [Tul], Tulerpeton curtum; [Euc], Eucritta melanolimnetes; [Cra], Crassigyrinus scotic. Paleozoic tetrapods, embolomeres and other taxa that are often associated with the amniote stem: [Ara], Archeria; [Eld], Eldeceeon rolfei; [Gep], Gephyrostegus; [Pro], Proterogyrinus scheelei; [Sil], Silvanerpeton miripedes, [Wes], Westlothiana lizziae. Lepospondyli: [Tud], Tuditanus punctulatus; [Pan], Pantylus cordatus; Reptiles: [Vk], Varanus komodoensis; [Lac], Timon lepidus; [Ks], Kenyasaurus mariakaniensis; Sauropterygians: [Ah], Anarosaurus heterodontus; [Ds], Dactylosaurus schroederi; [Np], Neusticosaurus pusillus; [Pt], Psilotrachelosaurus toeplitschi; [Sg], Serpianosaurus germanicus; [Sm], Serpianosaurus mirigiolensis; early eureptiles: [Cr], Captorhinus; [Hyl], Hylonomus lyelli; [Pal], Paleothyris acadiana; [Pet], Petrolacosaurus kansensis; Synapsids: [Arc], Archaeovenator hamiltonensis; [Cas], Casea broilii; [Eoc], Eocasea martini; [Oph], Ophiacodon; Ichthyosaurs: [Hs], Hupehsuchus; [St], Stenopterygius; Taxa were measured from the following sources: Stem Tetrapoda, and other stegocephalians of debated affinities (Marjanovi and Laurin, 2013), including temnospondyls and embolomeres (Romer, 1956; Carroll, 1970; Holmes, 1984, 2003; Godfrey, 1989; Panchen and Smithson, 1990; Sequeira and Milner, 1993; Smithson, 1993; Smithson et al., 1994; Lebedev and Coates, 1995; Coates, 1996; Clack, 1998; Ruta and Clack, 2006; Fröbisch et al., 2007); Varanidae (Christian and Garland, 1996); lepospondyls (Carroll, 1968; Carroll and Baird, 1968); phrynosomatid lizards (Herrel et al., 2002); Lacertidae Timon lepidus (Rewcastle, 1980); the earliest diapsid Petrolacosaurus (Peabody, 1952); the possible tangasaurid Kenyasaurus mariakaniensis (Harris and Carroll, 1977); Synapsids (Williston, 1910; Eaton, 1962; Reisz and Dilkes, 2003; Reisz and Fröbisch, 2014); various Permo-Carboniferous amniotes (Carroll, 1964, 1969; Fox and Bowman, 1966); Sauropterygians; (Sues and Carroll, 1985; Rieppel, 1989; Caldwell, 1997a, 2002; Klein, 2012); Ichthyosauria (Carroll and Dong, 1991; Caldwell, 1997b); Mosasauridae (Caldwell, 1996). See Table S1 (Supplementary Material) as reference.
Stem-tetrapods like Acanthostega, Ichthyostega, Crassigyrinus, Tulerpeton and Grereerpeton and the temnospondyl Apateon have a tibia/femur ratio 1:2, and possess pes/femur ratios ranging between 1:2 and 1:1. This means that their tibia is approximately half as long as their femur, and their pes is equal to, or shorter than their femur. However the plotted Temnospondyli show more disparity, with a pes slightly shorter or larger than those of stem tetrapods.
The 13 sauropterygian species plotted show much variation in their pes/femur ratios; their pes can be longer or shorter than their femur. However, all the plotted taxa have a femur similar in length to more than twice as long as their tibiae. The ichthyosaur Stenopterygius (St) falls in the same region as sauropterygians. However, the early sauropterygian Dactylosaurus schroederi and the basal ichthyosaur Hupehsuchus appear in the squamate zone. This fact would be interpreted as preservation of more terrestrial characters derived from their ancestors (Storrs, 1993).
The five plotted mosasaurid taxa display the most extreme pes/femur ratio, larger than 3:1. Their tibia/femur ratio is similar to that of other reptiles, between 1:2 and 1:1. Thus, the mosasaur tibia is shorter than the femur but longer than half of the femur, and their pes is more than 3 times longer than their femur (Caldwell, 1996).
Synapsids are distributed next to the squamates, but have a slightly lower tibia/femur ratio.
Early tetrapods that are often interpreted as part of the amniote stem (e.g., Proterogyrinus, Gephyrostegus, and possibly Westlothiana) (Ruta et al., 2003; Piñeiro et al., 2016) are a very heterogeneous group. They are often considered aquatic or semiaquatic (except for Westlothiana), and appear close to mesosaurids in the plot, as well as to the purposed semiaquatic stem Tetrapoda Greererpeton (Gre) and Tulerpeton (Tul) (after Ruta et al., 2003) and to the captorhinid Captorhinus aguti, which is usually interpreted as terrestrial. They all possess a similar tibia/femur ratio lower than 3:4 (except Petrolacosaurus with a ratio larger than 1:1) and a pes/femur ratio ranging between 1:2 and 2:1 (Godfrey, 1989; Lebedev and Coates, 1995). Stem Tetrapoda & Temnospondyli, and part of the Permo-Carboniferous taxa (including anthracosaurs, lepospondyls, and early amniotes) appear to share the same region. Gephyrostegus displays a ratio that is similar to squamates and synapsids. Eldeceeon [Eld], Silvanerpeton [Sil], Westlothiana [Wes], Hylonomus [Hyl] Paleothyris [Pal], and Pantylus cordatus [Pan] share pes/femur ratios analogous to the extant reptiles. However, their tibia/femur ratio is lower than the ratio of the plotted reptiles with the same pes/femur ratio. These last taxa are characterized by the lowest tibia/pes ratios for terrestrial taxa (oblique dashed lines in the plot; triangles in Figure 12). In other words, while almost all plotted terrestrial species have tibia/pes ratios larger than 1:2, the mentioned six species had ratios lower than 1:2.
Mesosaurus has tibia/femur and pes/femur ratios lower than those seen in squamates and basal synapsids. Its pes/femur ratio is lower than the majority of the presumed stem Amniota although Mesosaurus has a tibia/femur ratio near to 1:2 and the pes/femur ratio is larger than in the majority of the stem tetrapods studied herein. It has also a slightly higher tibia/femur ratio. Curiously, Mesosaurus, Captorhinus (Cr), Proterogyrinus (Pro), and Tulerpeton (Tul) have similar ratios, in a region shared by both terrestrial and semiaquatic taxa. Even though Proterogyrinus was considered probably aquatic based on the presence of lateral-line canals (although poorly developed) (Holmes, 1984), many temnospondyls thought to be semiaquatic, also possess these sensory sulci (see Schoch and Milner, 2000; Yates and Warren, 2000; Damiani, 2001). Nevertheless, the absence of sulci does not necessarily imply terrestriality (Vallin and Laurin, 2004, p. 68; Danto et al., 2012). The hypothesis that any stegocephalian with lateral-line sulci was amphibious rather than aquatic contradicts what we know of extant taxa (because the neuromasts of this organ do not support dehydration), unless we postulate that the sulci persisted in the adult even though the organ regressed and was lost, presumably at metamorphosis.
Contrary to reptiles that mainly have tibia/pes ratios between 1:1 and 1:2, most mammals have tibia/pes ratios larger than 1:1 and thus, they fall in a separated area of the plot, which may reflect their parasagittal gait and other mammalian attributes (Kelly and Sears, 2011; Figure 12).
Claudiosarus and Hovasaurus occupy the same regions as the squamates, probably reflecting the retention of some terrestrial locomotion, as was previously suggested by other workers (e.g., Carroll, 1981, but see also Smith, 2000) and in agreement with our results on vertebral morphometrics.
Discussion
The Lifestyle of Basalmost Amniotes
Romer (1974) hypothesized that the earliest amniotes might have been aquatic or semi-aquatic. More efforts were recently done by applying different methodologies to investigate deeper into the lifestyle of basalmost amniotes (Germain and Laurin, 2005; Canoville and Laurin, 2010; Huttenlocker and Rega, 2012), including that of the basal synapsid Ophiacodon. Most of the previous studies have considered Ophiacodon as a semi-aquatic animal (Romer and Price, 1940; Kemp, 1982; Germain and Laurin, 2005; Canoville and Laurin, 2010). For example, Felice and Angielczyk (2014) applied morphometric studies including vertebral length profiles to several extinct and extant taxa and Ophiacodon and other early synapsids fell in the semi-aquatic category as they display a morphometric profile similar to most extant semi-aquatic reptiles and mammals. Nevertheless, a fairly terrestrial pattern can be observed in the plots that these authors provided for Ophiacodon, regarding the smooth decline in vertebral length toward the tip of the tail, which is present (although much more evident) in all the Varanus species included in our study. Because the lines of evidence are ambiguous, Felice and Angielczyk (2014) did not consider the length metrics of Ophiacodon useful to explore lifestyles for this taxon. Similarly, another work that inquired into the lifestyle of Ophiacodon through a study of bone microanatomy (Germain and Laurin, 2005) found evidence supporting a semi-aquatic to terrestrial pattern. However, a more recent study (Laurin and de Buffrénil, 2016) concluded (based on another bone section) that this basal synapsid may have been terrestrial and that this may reflect the early amniote condition because an older basal synapsid (Clepsydrops collettii) exhibited a microanatomical signature typical of terrestrial tetrapods. However, this could also reflect the condition in early synapsids.
Our results show that all the studied basal synapsids develop an intermediate pattern between the purposely semi-aquatic diapsids from the Late Permian of Madagascar (Thadeosaurus, Hovasaurus, and Claudiosaurus) and the extant terrestrial Varanus species.
Lambertz et al. (2016) regard the Caseidae as aquatic mainly for their osteoporotic-like limb bones and even suggest that they had a respiratory mechanism to cope with the oxygen needs while swimming. From our results, early synapsids display a terrestrial-like pattern with Cotylorhynchus as the most notable example (Figure 11E). Casea and Varanops resemble the patterns of Varanus rudicollis or V. dumerilii. Despite the limited data about the early synapsid neck, it can be said that, contrary to mesosaurids, Cotylorhynchus, Varanops and Ophiacodon have cervical vertebrae longer than the caudal ones, and in the case of Varanops and Ophiacodon, also longer than most of the dorsal ones (although some mesosaurids display a segment of long vertebrae at the middle of the neck). Cotylorhynchus, Casea, and Varanops have at least one peak of length in the dorsal region, whereas Ophiacodon appears to maintain a more constant value. Early synapsids exhibit a caudal pattern similar to that of Varanus: a progressive decrease along the tail, not developing a relative maximum. However, contrary to Varanus, early synapsids appear to maintain the vertebral length along the first portion of the tail (see Figures 10G–L, 11E–H).
Ophiacodon also resembles the terrestrial type (like Varanus salvator or V. rudicollis) in having the cervical vertebrae longer than the dorsals and the caudals, and the dorsals are also longer than the caudals. This is congruent with the latest bone microanatomical study (Laurin and de Buffrénil, 2016). However, this interpretation should be treated with caution because we did not study the specimens directly and there could be some distortion due to incompleteness of the available specimens plotted, a condition that is reflected in their noisy profile (Figure 11H).
The Madagascan taxa Thadeosaurus and Claudiosaurus were restored as having 25 and 24 presacral vertebrae respectively, and the former shows at least 47 caudal vertebrae (Carroll, 1981). According to (Carroll (1981), pp. 322–323) and Currie and Carroll (1984), the column and limb proportions of Thadeosaurus are similar to those of basal terrestrial squamates and, along with Claudiosaurus, Thadeosaurus lacked any aquatic specialization. Also, according to (Carroll, 1981, p. 357) the terminal phalanges of Thadeosaurus “are the typically trenchant, recurved and laterally compressed claws, associated with terrestrial carnivores”. However, it is sometimes considered as a semi-aquatic or swimmer species by its anatomical similarities with Claudiosaurus and Hovasaurus, though there was no morphological evidence in the skeleton to suggest swimming habits (Carroll, 1981; Currie, 1981a; Currie and Carroll, 1984). However, according to the patterns established by Buchholtz (1998), Thadeosaurus, with vertebral length increasing in the caudal region, resembles that of aquatic taxa.
Our results suggest that among the Madagascan taxa, Claudiosaurus may be the most terrestrial taxon (maybe semi-aquatic); it is intriguingly similar to Mesosaurus because of a smooth decrease in vertebral length toward the tip of the tail. Nevertheless, Claudiosaurus shows almost no length increase in dorsal or caudal vertebrae. Considering the cartilaginous nature of carpus and tarsus in young individuals of Claudiosaurus (Carroll, 1981), just as is the case in Mesosaurus (Piñeiro et al., 2016), this feature could be seen as useful for improving flexibility and thus adaptive to semiaquatic animals. Thadeosaurus, on the contrary, displays a more ossified carpus and tarsus, a feature putatively related to a more terrestrial habit. Carroll (1981) states that all the osteological features shown by Claudiosaurus do not forcibly make it aquatic, despite the poor ossification of carpus and tarsus and lateralized zygapophyses in the caudal vertebrae. Nevertheless, most extant terrestrial squamates have an outstanding capacity for aquatic locomotion despite the lack of skeletal and clear physiological specializations; intriguingly, the marine iguana displays very few of such specializations. It should be therefore kept in mind that even subtle skeletal modifications may reflect a notable difference in habitat preferences.
Hovasaurus, a stem diapsid up to 900 mm long, with pachyostotic ribs, strong caudal epiphyses and gastroliths, and a possible tail “fin” impression above the neural spines of the caudal vertebrae, is interpreted to have been adapted to a fully aquatic lifestyle (Carroll, 1981; Currie, 1981a; de Buffrénil and Mazin, 1989; Smith, 2000; Ketchum and Barrett, 2004; Reisz et al., 2011). Hovasarus possesses 25 presacral vertebrae, 5 of which are cervicals, and at least 70 caudal vertebrae (Currie, 1981b). Unfortunately, the analyzed specimens are incomplete, but the clear continuous increment in vertebral length in the proximal segment of the tail represents a feature seen in the semi-aquatic Crocodylia or Amblyrhynchus (Figures 10A, 11C).
Mesosaurus' vertebral column is composed of 12 (13 in some individuals) cervical, 21 dorsal, two sacrals and more than 64 caudal vertebrae, making a total count of around 100 elements (Villamil et al., 2015; Piñeiro et al., 2016). As occurs with other basal amniotes, the lifestyle of mesosaurids is not as easy to determine as previously believed. Mesosaurids probably spent most of the time swimming in shallow waters, but capabilities for land incursion cannot be totally ruled out. Mesosaurus has cervical vertebrae shorter than the dorsals, just as observed in semi-aquatic extinct and extant species. However, intriguingly, some Mesosaurus have the longest cervical vertebrae placed at the middle of the neck, as in terrestrial Varanus species. Also, in Mesosaurus, the longest vertebrae are located in the dorsal region, as in Amblyrhynchus, some basal synapsids and also some Varanus species. Mesosaurus seems to maintain the caudal vertebral length along the first portion of the tail, in a peak or a plateau, from which it decreases distally.
According to limb morphometrics, mesosaurids fall consistently in a region occupied by taxa that are usually considered both terrestrials and semiaquatic; they are positioned close to Gephyrostegus, Proterogyrinus, and Tulerpeton, as well as to the basal eureptile Captorhinus aguti, but intriguingly, they are not as close as expected to the oldest amniotes Hylonomus and Paleothyris (see Figure 12). However, the lifestyle of the two taxa that are often considered terrestrial (Gephyrostegus and Captorhinus aguti) is not well-established. Captorhinus is typically considered terrestrial because of faunal associations (MacDougall et al., 2017), but its thick long bone cortex suggests an amphibious lifestyle (Canoville and Laurin, 2010). Therefore, this result would suggest affinities of Captorhinus with aquatic or semiaquatic taxa. As for Gephyrostegus, the absence of lateral-line canals led Carroll (1970) to suggest a terrestrial lifestyle, but the lack of fusion between neural arches and centra of an embolomerous configuration in specimens that (Carroll (1970), p. 269) considered mature, suggests a fairly aquatic or a semiaquatic lifestyle.
Morphometrics of Mesosaurid Ontogenetic Stages
Mesosaurus' vertebral profiles seem to have a constant pattern through ontogeny as they are similarly independent of the size of the individuals (Figure 3, specimens 1-3; Figure 4, specimens 34, 35; Figure 5, specimen 39, Figure 8B). However, patterns are more marked in the very mature individuals, with larger differences between minimum and maximum lengths. Moreover, as the preservation quality of fossil samples decreases in the largest individuals, noise and disarticulation increase. The larger the specimen, the more difficult it is to find a complete skeleton.
Although some individuals exhibit abnormal profiles, normalization methods using the mean vertebral length of a population allow comparing individuals. Normalization (dividing the patterns by the mean vertebral length) does not modify the patterns; it simply rescales them so that they are comparable to each other (Figure 13).
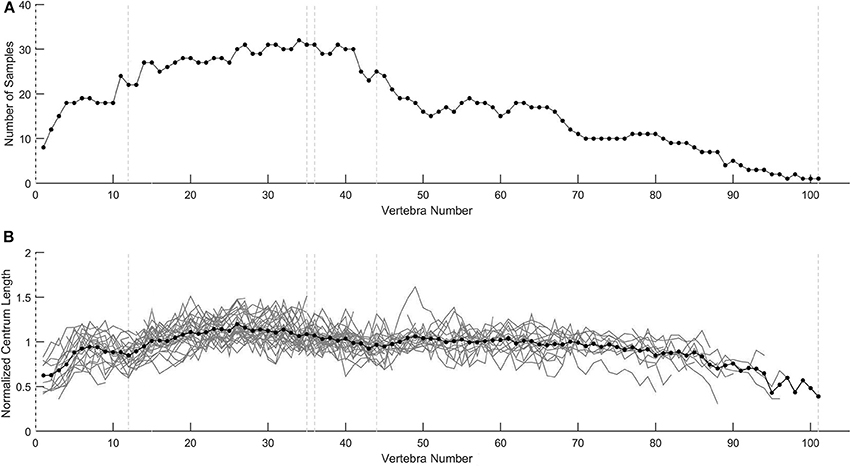
Figure 13. Profile normalization. (A) Number of vertebral samples available for each vertebra. (B) Normalized centrum length profiles for the 40 specimens represented individually in Figures 3–5). Normalization was performed with the mean vertebral length. Mean values for each vertebra position are indicated by black dots.
Previous studies have characterized the pattern of the species by studying only a few isolated individuals. However, this results in substantial uncertainty about the representative pattern of the species because some intraspecific variation is always present. Moreover, how can we know that the selected pattern was not distorted by fossil preservation? Once normalized, the profiles allow calculating a mean profile, which better reflects the genuine pattern shared by the whole population. Normalized patterns can be compared with this mean pattern (and between them) in order to identify intraspecific variations.
In our study, all 40 Mesosaurus tenuidens specimens plotted show profiles that follow a clear general pattern consistent with semi-aquatic adaptations (Figure 8B) in all sampled ontogenetic stages. Nevertheless, each individual has characteristics and noticeable deviations, which might represent intraspecific variation or may reflect differences in age, sex or preservation.
Paleobiological, Paleoecological and Taphonomic Evidence Supports the Transitional Semi-Aquatic Habits for Mesosaurids
Taking into account the 40 profiles obtained, Mesosaurus would fall into the semi-aquatic pattern seen in some extant crocodylians and also in the analyzed Madagascan taxa (Figures 10, 11). The graphs show that juvenile individuals, which are represented by more complete, articulated and less variable skeletons, have a clear semiaquatic profile, while the less complete and badly preserved adult individuals show a noisier pattern. This might imply a transitional lifestyle, where juvenile individuals are fairly aquatic, but adults spend more time on land. The caudal profile obtained for Mesosaurus tenuidens is peculiar because it appears to be more constant than in typically terrestrial forms, but there is generally no clear increment in caudal vertebral length or a clear plateau as in other semi-aquatic species (exceptions are noted; Figure 3, specimen 18; Figure 6, specimens 5 and 8), only a slight decreasing curve. The neck of Mesosaurus is long, and vertebrae are saddle-shaped, strongly linked to each other through a cervical rib contact, spanning two successive vertebrae, resembling those of some terrestrial species, which need to raise the head in subaerial conditions and move it differently than in the semi-aquatic forms studied herein.
The completeness degree of some specimens, as well as the number of available individuals to be plotted (better if different identifiable ontogenetic stages are represented), play an important role in the validity of this methodology. Mesosaurids are a good example to apply this method because an important number of well-preserved specimens are available, counting juvenile (including hatchlings) and adult stages.
Mesosaurids have been considered mainly aquatic, but derived from a terrestrial form that had subsequently become aquatic (Laurin and de Buffrénil, 2016) or to have derived directly from semi-aquatic ancestors (Romer, 1957). Mesosaurids fed on aquatic prey items and defecated in a transitional environment (possibly a lagoon or a hypersaline sea) in shallow water (Piñeiro et al., 2012a,c; Silva et al., 2017). Nares have migrated farther away from the tip of the rostrum and are located dorsally, eyes are large and the snout is long. These features, along with the presence of pachyosteosclerotic ribs and webbed manus and pes, are obvious aquatic adaptations. However, some of these features (though not pachyosteosclerosis) are also present in amphibious taxa. The presence of coprolites in close proximity to mesosaurid skeletons such as those we have recently described (Silva et al., 2017), which mostly appear not to have been expelled post mortem (see Hone et al., 2015), is rather uncommon because normally, it is difficult to identify the coprolite producer. Possible explanations for the numerous excrements associated to mesosaurid body remains include: (a), mesosaurids spent much time in confined areas in a transitional coastal environment and (b), they lived in dense populations (Dentzien-Dias et al., 2012). Common associations of adult skeletons with newborns suggest that some kind of parental care existed in Mesosaurus (Piñeiro et al., 2012a). Mesosaurus often scavenged on mesosaurid carrion; this lifestyle is compatible with the hypothesis that dense populations of mesosaurids lived in an environment with limited food resources (Silva et al., 2017). Some gastric contents found associated to mesosaurid skeletons include plant and insect remains that might have been scavenged on the water pool bottom (Silva et al., 2017) but alternatively, plants and insects could have been ingested on land. Exceptionally, mesosaurid coprolites show possible desiccation fractures (see Silva et al., 2017, Figure 3C).
Direct evidence that Mesosaurus spent a significant proportion of time on land is missing. Its environment was an inland sea, comparable to a shallow saline lake. At the time that mesosaurids colonized the basin, the sea may have been partially cut off from the ocean, which may have resulted in moderate evaporation and hypersalinity (see Piñeiro et al., 2012c; Callefo et al., 2018). In the Uruguayan part of the Paraná Basin, the shore was close, as biogeographic reconstructions suggest (Wopfner, 1999; Milani and De Wit, 2008; Figure 14); this is also suggested by the presence of well-preserved insects and plants as common components of the “mesosaur community” (Piñeiro et al., 2012c). Moreover, pygocephalomorph crustaceans are associated to shallow environments (Brooks, 1962; Hotton, 2002; Piñeiro, 2008) and that is probably why mesosaurids were not found in the deepest basin sections. Mesosaurus would have fed on algae as well as on mesosaurid carrion deposited in the shallow coastal plains and it is worth to note that mesosaurid skeletons from the deepest areas are in general not only well preserved, but also more articulated, perhaps due to the absence of pygocephalomorph reworking. Sedimentological analyses do not suggest that the basin was affected by tides, and the studied levels from Uruguay show no evidence of heavy storms, which allowed the delicate, exceptional preservation of the fossils (Piñeiro et al., 2012b,c). Subaerial preservation is more unlikely to have produced well-preserved specimens; this taphonomic bias is illustrated by the quality of the fossilized specimens. Large, mature mesosaurids are poorly preserved and less abundant than young adults and juveniles that were rapidly buried by volcanic ash that periodically affected the basin (see Santos et al., 2006; Piñeiro et al., 2012c). Alternatively, the difference in numbers of preserved specimens might simply reflect population structure in which few individuals are expected to reach a large body size. Remarkably, Silva et al. (2017) suggested that Mesosaurus from the Mangrullo and Iratí Formations lived under environmental stress. It is thus also possible that adult Mesosaurus were more terrestrial than younger ones, which would provide a simple taphonomic explanation of the relative rarity and poor preservation quality of mature individuals. Intriguingly, the largest mesosaurids known so far (Piñeiro, 2002, 2006) come from crystalline levels (gypsum), and are associated with desiccation marks and a thin bentonitic layer. The alternative hypothesis suggesting a more fully aquatic mesosaurid taxon (Modesto, 1996) or simply that the very mature individuals lived in deeper waters is not supported by the existent evidence: the largest individuals are solely preserved in the marginal (shore line) area of the basin (e.g., those from the Mangrullo Formation); the suggested presence of parental care in Mesosaurus supports the hypothesis that mature and juvenile individuals lived very close to each other at least seasonally, and the main food item of mesosaurids (see Silva et al., 2017) should have been most abundant in coastal areas, because of the unfavorable environmental conditions for bentonic biota such as the high salinity and anoxia of the deepest basin bottoms (Goldberg and Humayun, 2016).
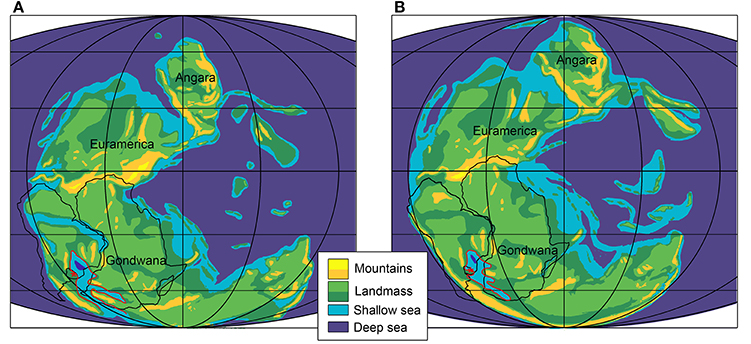
Figure 14. Gondwana during the Early and Middle Permian; (A) Sakmarian; (B) Wordian. The red line delimitates the sea where Mesosaurus is known to occur. Africa, Madagascar and North portion of South America contours are drawn with a black line. Modified after Faure and Cole (1999); Gibbs et al. (2002); Schlüter (2008); Stephenson (2018).
Additional Anatomical Features Studied for Evaluation of Mesosaurid Lifestyle
Although the rigid structure of the appendicular joints was considered as a constraint for mesosaurids to walk on land, it could not have been restrictive for making terrestrial incursions (Modesto, 1996; Piñeiro et al., 2012a). Nevertheless, the anatomy of the girdles and appendicular elements does not differ much from those present in supposedly terrestrial basal amniotes such as Hylonomus, the captorhinids and basal synapsids, or even the basal diapsid Petrolacosaurus (see Romer and Price, 1940; Peabody, 1952; Romer, 1956; Carroll, 1964, 1982; Reisz, 1977). These features include the fusion of coracoid and scapula to form the scapulocoracoid in adults, and co-ossification of the pelvic elements which abut weakly to the two sacral ribs through the iliac dorsal lamina, a joint that appears as co-ossified in the mature specimens (Piñeiro et al., 2012a).
The somewhat rigid axial skeleton of mesosaurids forms a deep chest where vertebrae lack intercentra but display poorly developed accessory articular structures (e.g., hyposphene and hypantrum) to stabilize the trunk (Seeley, 1892; von Huene, 1941; Sumida and Modesto, 2001), probably acting in association with the ribs and gastralia. Still allowing some flexibility, these apophyses restrain extreme torsion of the axial skeleton and helped mesosaurids to avoid injuries from vertebral twisting during rapid movements. The gastralia also increases the body stiffness, giving protection to the ventral body wall (Buchholtz, 2001a), but it could be plesiomorphic for tetrapods (Gegenbaur, 1898; Romer, 1956) and thus, it is not exclusive of aquatic forms.
Another noteworthy condition for evaluating the lifestyle of Mesosaurus is the different development of manus and pes. While Romer and Price (1940) considered that the substantially reduced size of fore limb compared to the hind limb was an adaptation for an aquatic lifestyle of mesosaurids, this inference is questionable. Many of the basal amniotes considered to be terrestrial forms have limb proportions between manus and pes similar to those of mesosaurids (e.g., Petrolacosaurus and other basal diapsids, most basal synapsids, captorhinomorphs, diadectomorphs) (see Romer and Price, 1940; Fox and Bowman, 1966; Carroll, 1969; Heaton and Reisz, 1980, 1986; Reisz, 1981; Reisz et al., 1984; Berman and Henrici, 2003; Berman et al., 2004, 2010). The manus is less developed than the foot in Paleothyris, Hylonomus, Hovasaurus, Claudiosaurus, and Mesosaurus, but also in anthracosaurs, such as Gephyrostegus, Greererpeton, Proterogyrinus and the microsaurs Tuditanus and possibly Pantylus (Carroll, 1964, 1968; Carroll and Baird, 1968; Holmes, 1984; Godfrey, 1989). However, most of these taxa with the exception of Paleothyris, Hylonomus, Tuditanus, and Pantylus, which are thought to be terrestrial (e.g., Falcon-Lang et al., 2007), have been considered as semi-aquatic forms. Interestingly, most extant squamates exhibit an important size difference between manus and pes, despite being terrestrial, but most swim well if necessary (e.g., escaping a predator).
Recently, it has been found that Mesosaurus possesses a navicular or navicular-like bone (Piñeiro et al., 2016). This bone, which is apparently formed by the fusion of centralia 1 and 2, is part of a tarsal innovation, including the origin of the astragalus and the calcaneum, which is shared by all the oldest amniotes. Thus, while it is not necessarily a direct evidence of a terrestrial lifestyle (O'Keefe et al., 2006), the earliest amniotic tarsus includes this bone, which is possibly an adaptation to a more terrestrial lifestyle. It could have originated in the semi-aquatic precursors of basal amniotes, in taxa resembling Westlothiana, Gephyrostegus and the microsaurs, which also possess an amniotic-like tarsus (see Piñeiro et al., 2016 and references therein).
Optimizations of the inferred aquatic habit in amniote phylogenies suggest that colonization of shallow environments is plesiomorphic with respect to a pelagic adaptation, which, according to some authors, could have been attained by Mesosaurus tenuidens, which thus would be the most aquatic mesosaurid species (Modesto, 1996, 1999). However, this interpretation may have been influenced by adaptations developed by Mesosaurus at different ontogenetic stages, as suggested in the present study and also observed in other aquatic and semi-aquatic reptiles (see Currie and Carroll, 1984; Caldwell, 1995, 2002).
The identification of Mesosaurus as one of the basalmost amniotes (Laurin and Reisz, 1995; Piñeiro et al., 2012b, 2016; Laurin and Piñeiro, 2017) or as the basalmost parareptile (e.g., Piñeiro et al., 2012a,b; Tsuji et al., 2012; Modesto et al., 2015) raises the possibility that they did not derive from terrestrial forms. According to Romer (1957), mesosaurids' ancestors were amphibious rather than terrestrial. The argument that supported Romer's hypothesis was that the temporal gap (from early Late Carboniferous to Early Permian) appears to be very short (Romer, 1974, but see also von Huene, 1940; Mazin, 2001; Piñeiro, 2006) for the development of such anatomically complex evolutionary transformations (from axial aquatic to paraxial terrestrial and again to axial aquatic locomotion). Mesosaurids represented one of the basalmost clades in the phylogenetic tree of amniotes (Laurin and Piñeiro, 2017) which is, interestingly, in accordance with Romer's idea that the earliest amniotes retained an amphibious lifestyle, as suggested for mesosaurids in this study.
Conclusions
Vertebral profiles seem to be a simple and useful method to discriminate locomotor habits. Profiles of terrestrial taxa exhibit clear differences from those of aquatic and semi-aquatic taxa in the studied species. Extinct species roughly match their expected patterns and are in general consistent with other adaptive anatomical features observed in the taxa. Basal synapsids fit well in the terrestrial pattern as expected, and the Madagascan taxa analyzed seem to fit the semi-aquatic and aquatic patterns identified here. The obtained results should be taken with caution due to the low number of specimens and taxa involved.
The quality of preservation appears to be more important to establish reliable vertebral length profiles than the completeness degree of fossils. Well-preserved specimens, even if they are incomplete (e.g., Hovasaurus), can provide discernible patterns, but complete and poorly preserved fossils generate noisy patterns whose interpretation may be misleading (e.g., Ophiacodon). As was previously suggested, ontogenetic changes in the proportions of the axial skeleton, sexual dimorphism or osteo-pathologies and other sources of variation in extinct species would make this study very defective if only a few specimens were used. The normalization method using the mean vertebral length resulted in a reliable tool in order to overcome intraspecific variations in specific ontogenetic stages, allowing calculating a mean profile that reflects the general pattern of the species.
The evidence suggests that Mesosaurus may have been slightly amphibious rather than strictly aquatic, at least when it attained a large size and an advanced ontogenetic age, though it is impossible to determine how much time was spent on land and what kind of activity was performed there. Thus, it is impossible to know if mesosaurids came onto land only to bask, like seals or crocodiles, or if they were a bit more agile. Their rather extensive pachyosteosclerosis implies limited terrestrial agility, which would have severely restricted terrestrial foraging abilities. Therefore, we suggest a more amphibious for Mesosaurus rather a fully aquatic lifestyle as previously suggested. Studies on limb proportions also support the resulting semiaquatic pattern suggested by the vertebral profiles. This interpretation is compatible with a hypothesis of semi-aquatic or amphibious direct ancestors of mesosaurids (Piñeiro et al., 2016), though this is not what an optimization of lifestyle on tetrapod phylogeny suggests (Laurin and de Buffrénil, 2016).
As suggested in previous works (e.g., Felice and Angielczyk, 2014), we also encourage further studies of vertebral profiles in extant and extinct species, in order to better understand how ontogeny, sexual dimorphism, osteo-pathologies, and preservation modify vertebral column patterns.
Author Contributions
PND and GP conceived the study, wrote the manuscript with input of all the authors; PND and GP produced the figures; PND is responsible for all the measurements and he performed all the morphometric analyses; and MM and ML contributed to the writing and interpretation of the results, and to the edition of the text.
Funding
This is a contribution to the Project ANII, Movilidad and Cooperación Internacional N° 1, 2014-2015 (Uruguay-France) to GP.
Conflict of Interest Statement
The authors declare that the research was conducted in the absence of any commercial or financial relationships that could be construed as a potential conflict of interest.
Acknowledgments
We are grateful to the Academic Editor Martín Ezcurra and reviewers Torsten Scheyer and Juan Carlos Cisneros for their constructive contribution to this paper, and to Ivone Cardozo and Marcelha Páez Landim (USP, São Paulo, Brazil), Rainer Brocke and Gunnar Riedel (Curators of the Paleontological Collection at the Natur Museum Senckenberg, Frankfurt, Germany), for kindly help us during the revision of several of the specimens used in this study. We are also grateful to the curator Heinz Furrer and collection managers of the Paläontologisches Institut und Museum der Universität Zürich (Switzerland) (PIMUZ) for provide good quality photographs of mesosaur specimens housed in that institution. We thank Pablo Velozo for taking the photographs of the Madagascan taxa deposited in the fossil collection of Muséum National d'Histoire Naturelle of Paris, and to Piotr Bajdek for helpful comments and suggestions on mesosaur cololites taphonomy.
Supplementary Material
The Supplementary Material for this article can be found online at: https://www.frontiersin.org/articles/10.3389/fevo.2018.00109/full#supplementary-material
Table S1. Forelimbs ratios. Species acronyms, colors and source of data as used in Figure 12 are provided. S, semiaquatic; A, aquatic; T, terrestrial.
Table S2. Centrum measurements for the studied Mesosaurus specimens. Code and collection number are provided.
Table S3. Centrum measurements for the studied Madagascan taxa Thadeosaurus, Claudiosaurus and Hovasaurus.
References
Angelini, F., and Ghiara, G. (1984). Reproductive modes and strategies in vertebrate evolution. Boll. Zool. 51, 121–203. doi: 10.1080/11250008409439459
Araújo, D. C. (1976). Taxonomia e relações dos Proganosauria da Bacia do Paraná. An. Acad. Bras. Cien. 48, 91–116.
Berman, D. S., and Henrici, A. C. (2003). Homology of the astragalus and structure and function of the tarsus of Diadectidae. J. Paleontol. 77, 172–188. doi: 10.1666/0022-3360(2003)077<0172:HOTAAS>2.0.CO;2
Berman, D. S., Henrici, A. C., Kissel, R. A., Sumida, S. S., and Martens, T. (2004). A new diadectid (Diadectomorpha), Orobates pabsti, from the Early Permian of central Germany. Bull. Carn. Mus. Nat. Hist. 35, 1–36.
Berman, D. S., Reisz, R. R., and Scott, D. (2010). Redescription of the skull of Limnoscelis paludis Williston (Diadectomorpha: Limnoscelidae) from the Pennsylvanian of Caño del Cobre, northern New Mexico. Bull. N. M. Mus. Nat. Hist. Sci. 49, 185–210. doi: 10.2992/0145-9058(2004)35[1:ANDDOP]2.0.CO;2
Brooks, H. K. (1962). The Paleozoic Eumalacostraca of North America. Bull. Amer. Paleont. 44, 163–338.
Buchholtz, E. A. (1998). “Implications of vertebral morphology for locomotor evolution in early Cetacea,” in The Emergence of Whales: The Evolutionary Patterns in the Origin of Cetacea, Ed J. G. M. Thewissen (New York, NY: Plenum Press). 325–352.
Buchholtz, E. A. (2001a). Swimming styles in Jurassic ichthyosaurs. J. Vert. Paleont. 21, 61–73. doi: 10.1671/0272-4634(2001)021[0061:SSIJI]2.0.CO;2
Buchholtz, E. A. (2001b). Vertebral osteology and swimming style in living and fossil whales (Order: Cetacea). J. Zool. 253, 175–190. doi: 10.1017/S0952836901000164
Buchholtz, E. A. (2007). Modular evolution of the cetacean vertebral column. Evol. Dev. 9, 278–289. doi: 10.1111/j.1525-142X.2007.00160.x
Buchholtz, E. A., and Schur, S. A. (2004). Vertebral osteology in Delphinidae (Cetacea). Zool. J. Linn. Soc. 140, 383–401. doi: 10.1111/j.1096-3642.2003.00105.x
Caldwell, M. (1995). Developmental constraints and limb evolution in Permian and modern lepidosauromorph diapsids. J. Vert. Paleontol. 14, 459–471.
Caldwell, M. W. (1996). Ontogeny and phylogeny of the mesopodial skeleton in mosasauroid reptiles. Zool. J. Linn. Soc. 116, 407–436.
Caldwell, M. W. (1997a). Limb osteology and ossification patterns in Cryptoclidus (Reptilia: Plesiosauroidea) with a review of sauropterygian limbs. J. Vert. Paleontol. 17, 295–307.
Caldwell, M. W. (1997b). Limb ossification patterns of the ichthyosaur Stenopterygius, and a discussion of the proximal tarsal row of ichthyosaurs and other neodiapsid reptiles. Zool. J. Linn. Soc. 120, 1–25.
Caldwell, M. W. (2002). From fins to limbs to fins: limb evolution in fossil marine reptiles. Am. J. Med. Genet. 112, 236–249. doi: 10.1002/ajmg.10773
Callefo, F., Arduim, D. H., Ricardi-Branco, F., Galante, D., Rodrigues, F., and Branco, F. C. (2018). The giant stromatolite field at Santa Rosa de Viterbo, Brazil (Paraná Basin) – A new paleoenvironmental overview and the consequences of the Irati Sea closure in the Permian. J. South. Am. Earth. Sci. 84, 299–314. doi: 10.1016/j.jsames.2018.04.008
Canoville, A., and Laurin, M. (2010). Evolution of humeral microanatomy and lifestyle in amniotes, and some comments on paleobiological inferences. Biol. J. Linn. Soc. 100, 384–406. doi: 10.1111/j.1095-8312.2010.01431.x
Carroll, R. L. (1964). The earliest reptiles. Zool. J. Linn. Soc. 45, 61–83. doi: 10.1111/j.1096-3642.1964.tb00488.x
Carroll, R. L. (1968). The postcranial skeleton of the Permian microsaur Pantylus. Can. J. Zool. 46, 1175–1192. doi: 10.1139/z68–168
Carroll, R. L. (1969). A Middle Pennsylvanian captorhinomorph, and the interrelationships of primitive reptiles. J. Paleontol. 43, 151–170.
Carroll, R. L. (1970). The ancestry of reptiles. Phil. Trans. R. Soc. Lond. B. Biol. Sci. 257, 267–308.
Carroll, R. L. (1981). Plesiosaur ancestors from the Upper Permian of Madagascar. Philos. Trans. R. Soc. Lond. B. Biol. Sci. 293, 315–383.
Carroll, R. L., and Baird, D. (1968). The Carboniferous amphibian Tuditanus (Eosauravus) and the distinction between microsaurs and reptiles. Am. Mus. Novit. no. 2337, 1–50.
Carroll, R. L., and Dong, Z. M. (1991). Hupehsuchus, an enigmatic aquatic reptile from the Triassic of China, and the problem of establishing relationships. Philos. Trans. R. Soc. Lond. B. Biol. Sci. 331, 131–153.
Christian, A., and Garland, T. Jr. (1996). Scaling of limb proportions in monitor lizards (Squamata: Varanidae). J. Herpetol. 30, 219–230.
Clack, J. A. (1998). A new Early Carboniferous tetrapod with a mélange of crown-group characters. Nature. 394, 66.
Coates, M. I. (1996). The Devonian tetrapod Acanthostega gunnari Jarvik: postcranial anatomy, basal tetrapod interrelationships and patterns of skeletal evolution. Trans. R. Soc. Edinb. 87, 363–421.
Currie, P. J. (1981a). The Osteology and Relationships of Aquatic Eosuchians From the Upper Permian of Africa and Madagascar. Unpublished Ph.D.Thesis, McGill University, Montreal, QC.
Currie, P. J. (1981b). Hovasaurus boulei, an aquatic eosuchian from the Upper Permian of Madagascar. Palaeontol. Afric. 21, 99–168.
Currie, P. J., and Carroll, R. L. (1984). Ontogenetic changes in the eosuchian reptile Thadeosaurus. J. Vert. Paleontol. 4, 68–84.
Damiani, R. J. (2001). A systematic revision and phylogenetic analysis of Triassic mastodonsauroids (Temnospondyli: Stereospondyli): Zool. J. Linn. Soc. 133, 379–482. doi: 10.1111/j.1096-3642.2001.tb00635.x
Danto, M., Witzmann, F., and Müller, J. (2012). Redescription and phylogenetic relationships of Solenodontosaurus janenschi Broili, 1924 from the Late Carboniferous of Nýrany, Czech Republic. Fossil Record. 15, 45–59. doi: 10.1002/mmng.201200003
de Buffrénil, V., and Mazin, J. M. (1989). Bone histology of Claudiosaurus germaini (Reptilia, Claudiosauridae) and the problem of pachyostosis in aquatic tetrapods. Hist. Biol. 2, 311–322.
Dentzien-Dias, P. C., de Figueiredo, A. E. Q., Horn, B., Cisneros, J. C., and Schultz, C. L. (2012). Paleobiology of a unique vertebrate coprolites concentration from Rio do Rasto Formation (Middle/Upper Permian), Paraná Basin, Brazil. J. S. Am. Earth Sci. 40, 53–62. doi: 10.1016/j.jsames.2012.09.008
de Ricqlès, A., and de Buffrénil, V. (2001). “Bone histology, heterochronies and the return of Tetrapods to life in water: where are we?” in Secondary Adaptation of Tetrapods to Life in Water, eds J.-M. Mazin and V. de Buffrénil (Munich: Friedrich Pfeil), 289–310.
De Smet, W. M. A. (1977). “The regions of the cetacean vertebral column,” in Functional Anatomy of Marine Mammals, Vol. 3. ed J. Harrison (New York, NY: Academic Press), 58–80.
Eaton, T. H. (1962). Adaptive features of the fore limb in primitive tetrapods and mammals. Am. Zool. 2, 157–160.
Escobar, R. A., Besier, E., and Hayes, W. K. (2010). Evaluating head starting as a management tool: post release success of green iguanas (Iguana iguana) in Costa Rica. Int. J. Biodivers. Conserv. 2, 204–214.
Falcon-Lang, H. J., Benton, M. J., and Stimson, M. (2007). Ecology of earliest reptiles inferred from basal Pennsylvanian trackways. J. Geol. Soc. 164, 1113–1118. doi: 10.1144/0016-76492007-015
Faure, K., and Cole, D. (1999). Geochemical evidence for lacustrine microbial blooms in the vast Permian Main Karoo, Paraná, Falkland Islands and Huab basins of southwestern Gondwana. Palaeogeogr. Palaeoclimatol. Palaeoecol. 152, 189–213.
Felice, R. N., and Angielczyk, K. D. (2014). “Was Ophiacodon (Synapsida, Eupelycosauria) a Swimmer? A test using vertebral dimensions,” in Early Evolutionary History of the Synapsida, Vertebrate Paleobiology and Paleoanthropology, eds C. F. Kammerer, K. D. Angielczyk, and J. Fröbisch (Dordrecht: Springer), 25–50.
Fish, F. E. (1984). Kinematics of undulatory swimming in the American Alligator. Copeia 1984, 839–843.
Fish, F. E. (1994). Association of propulsive swimming mode with behavior in river otters (Lutra canadensis). J. Mammal. 75, 989–997.
Fox, R. C., and Bowman, M. C. (1966). Osteology and relationships of Captorhinus aguti (Cope) (Reptilia: Captorhinomorpha). Univ. Kans. Paleontol. Cont. Vertebrata. 11, 1–179.
Fröbisch, N. B., Carroll, R. L., and Schoch, R. R. (2007). Limb ossification in the Paleozoic branchiosaurid Apateon (Temnospondyli) and the early evolution of preaxial dominance in tetrapod limb development. Evol. Dev. 9, 69–75. doi: 10.1111/j.1525-142X.2006.00138.x
Gegenbaur, C. (1898). Vergleichende Anatomie der Wirbelthiere mit Berücksichtigung der Wirbellosen, Vol 1. Leipzig: Wilhelm Engelmann.
Germain, D., and Laurin, M. (2005). Microanatomy of the radius and lifestyle in amniotes (Vertebrata, Tetrapoda). Zool. Scripta. 34, 335–350. doi: 10.1111/j.1463-6409.2005.00198.x
Gibbs, M. T., Rees, P. M., Kutzbach, J. E., Ziegler, A. M., Behling, P. J., and Rowley, D. B. (2002). Simulations of Permian climate and comparisons with climate-sensitive sediments. J. Geol. 110, 33–55. doi: 10.1086/324204
Godfrey, S. J. (1989). The postcranial skeletal anatomy of the Carboniferous tetrapod Greererpeton burkemorani Romer, 1969. Philos. Trans. R. Soc. Lond. B. Biol. Sci. 323, 75–133. doi: 10.1098/rstb.1989.0002
Goldberg, K., and Humayun, M. (2016). Geochemical paleoredox indicators in organic-rich shales of the Irati Formation, Permian of the Paraná Basin, southern Brazil. Braz. J. Geol. 46, 377–393. doi: 10.1590/2317-4889201620160001
Harris, J. M., and Carroll, R. L. (1977). Kenyasaurus, a new eosuchian reptile from the Early Triassic of Kenya. J. Paleontol. 51, 139–149.
Heaton, M. J., and Reisz, R. R. (1980). A skeletal reconstruction of the Early Permian captorhinid reptile Eocaptorhinus laticeps (Williston). J. Paleontol. 54, 136–143.
Heaton, M. J., and Reisz, R. R. (1986). Phylogenetic relationships of captothinomorph reptiles. Can. J. Earth. Sci. 23, 402–418.
Herrel, A., Meyers, J. J., and Vanhooydonck, B. (2002). Relations between microhabitat use and limb shape in phrynosomatid lizards. Zool. J. Linn. Soc. 77, 149–163. doi: 10.1046/j.1095-8312.2002.00101.x
Holmes, R. B. (1984). The Carboniferous amphibian Proterogyrinus scheelei Romer, and the early evolution of tetrapods. Philos. Trans. R. Soc. Lond. B. Biol. Sci. 306, 431–524. doi: 10.1098/rstb.1984.0103
Holmes, R. B. (2003). The hind limb of Captorhinus aguti and the step cycle of basal amniotes. Can. J. Earth. Sci. 40, 515–526. doi: 10.1139/e02-039
Hone, D., Henderson, D. H., Therrien, F., and Habib, M. B. (2015). A specimen of Rhamphorhynchus with soft tissue preservation, stomach contents and a putative coprolite. PeerJ. 3:e1191. doi: 10.7717/peerj.1191
Horn, H.-G. (2004). “Varanus salvadorii,” in Varanoid Lizards of the World, eds E. R. Pianka, D. King, and R. A. King (Bloomington: Indiana University Press), 234–244.
Hotton, N., Feldmann, R. M., Hook, R. W., and Dimichele, W. A. (2002). Crustacean-bearing continental deposits in the Petrolia Formation (Leonardian Series, Lower Permian) of North-Central Texas. J. Paleontol. 76, 486–494. doi: 10.1017/S002233600003732X
Houssaye, A. (2009). “Pachyostosis” in aquatic amniotes: a review. Integr. Zool. 4, 325–340. doi: 10.1111/j.1749-4877.2009.00146.x
Huttenlocker, A. K., and Rega, E. (2012). “The paleobiology and bone microstructure of pelycosaurian-grade synapsids, in Forerunners of Mammals: Radiation, Histology, Biology, ed A. Chinsamy-Turan (Bloomington, IN: Indiana University Press), 90–119.
Joyce, W. G., and Gauthier, J. A. (2004). Palaeoecology of Triassic stem turtles shed new light on turtle origins. Proc. R. Soc. Lond. B. 271, 1–5. doi: 10.1098/rspb.2003.2523
Keller, T. (1976). Magen-und Darminhalte von Ichthyosauriern des süddeutschen Posidonienschiefers. N. Jb. Geol. Paläont. Mh. 5, 266–283.
Kelly, M. E., and Sears, K. E. (2011). Limb specialization in living marsupial and eutherian mammals: constraints on mammalian limb evolution. J. Mammal. 92, 1038–1049. doi: 10.1644/10-MAMM-A-425.1
Ketchum, H. F., and Barrett, P. M. (2004). New reptile material from the Lower Triassic of Madagascar: implications for the Permian Triassic extinction event. Can. J. Earth. Sci. 41, 1–8. doi: 10.1139/e03-084
Klein, N. (2012). Postcranial morphology and growth of the pachypleurosaur Anarosaurus heterodontus (Sauropterygia) from the Lower Muschelkalk of Winterswijk, The Netherlands. Paläontologische Zeitschrift. 86, 389–408. doi: 10.1007/s12542-012-0137-1
Lambertz, M., Shelton, C. D., Spindler, F., and Perry, S. F. (2016). A caseian point for the evolution of a diaphragm homologue among the earliest synapsids. Ann. N.Y. Acad. Sci. 40, 1–18. doi: 10.1111/nyas.13264
Laurin, M., and de Buffrénil, V. (2016). Microstructural features of the femur in early ophiacodontids: a reappraisal of ancestral habitat use and lifestyle of amniotes. Comptes Rendus Palevol. 15, 115–127. doi: 10.1016/j.crpv.2015.01.001
Laurin, M., and Piñeiro, G. (2017). A reassessment of the taxonomic position of mesosaurs, and a surprising phylogeny of early amniotes. Front. Earth Sci. Topic Res. 13:88. doi: 10.3389/feart.2017.00088
Laurin, M., and Reisz, R. R. (1995). A reevaluation of early amniote phylogeny. Zool. J. Linn. Soc. 113, 165–223. doi: 10.1111/j.1096-3642.1995.tb00932.x
Lebedev, O. A., and Coates, M. I. (1995). The postcranial skeleton of the Devonian tetrapod Tulerpeton curtum Lebedev. Zool. J. Linn. Soc. 114, 307–348.
Lindgren, J., Jagt, J. W., and Caldwell, M. W. (2007). A fishy mosasaur: the axial skeleton of Plotosaurus (Reptilia, Squamata) reassessed. Lethaia 40, 153–160. doi: 10.1111/j.1502-3931.2007.00009.x
Long, J. H., Pabst, D. A., Shepherd, W. R., and McLellan, W. A. (1997). Locomotor design of dolphin vertebral columns: bending mechanics and morphology of Delphinus delphis. J. Exp. Biol. 200, 65–81.
MacDougall, M. J., Scott, D., Modesto, S. P., Williams, S. A., and Reisz, R. R. (2017). New material of the reptile Colobomycter pholeter (Parareptilia: Lanthanosuchoidea) and the diversity of reptiles during the Early Permian (Cisuralian). Zool. J. Linn. Soc. 180, 661–671. doi: 10.1093/zoolinnean/zlw012
MacGregor, J. H. (1908). “On Mesosaurus brasiliensis nov. sp. from the Permian of Brasil,” in ed. I.C. White IC Commissão dos Estudos das Minas de Carvão de Pedra do Brasil, Parte II (Rio de Janeiro: National Press), 301–336.
Marjanovi,ć, D., and Laurin, M. (2013). The origin(s) of extant amphibians: a review with emphasis on the “lepospondyl hypothesis”. Geodiversitas 35, 207–272. doi: 10.5252/g2013n1a8
Massare, J. A. (1988). Swimming capabilities of Mesozoic marine reptiles: implications for method of predation. Paleobiology 14, 187–205.
Mazin, J. M. (2001). “Mesozoic marine reptiles: an overview,” in Secondary Adaptation of Tetrapods to Life in Water, eds J.-M. Mazin and V. de Buffrénil (Munich: Friedrich Pfeil), 95–117.
McShea, D. W. (1992). A metric for the study of evolutionary trends in the complexity of serial structures. Biol. J. Linn. Soc. 45, 39–55.
McShea, D. W. (1993). Evolutionary change in the morphological complexity of the mammalian vertebral column. Evolution 47, 730–740.
Milani, E. J., and De Wit, M. J. (2008). Correlations between the classic Paraná and Cape–Karoo sequences of South America and southern Africa and their basin infill flanking the Gondwanides: du Toit revisited. Geol. Soc. Lond. Spec. Public. 294, 319–342. doi: 10.1144/sp294.17
Modesto, S. P. (1996). The Anatomy, Relationships, and Palaeoecology of Mesosaurus tenuidens and Stereosternum tumidum (Amniota: Mesosauridae) From the Lower Permian of Gondwana. PhD Thesis, University of Toronto, Toronto.
Modesto, S. P. (1999). Observations on the structure of the Early Permian reptile Stereosternum tumidum. Palaeontol. Afric. 35, 7–19.
Modesto, S. P. (2006). The cranial skeleton of the Early Permian aquatic reptile Mesosaurus tenuidens: implications for relationships and palaeobiology. Zool. J. Linn. Soc. 146, 345–368. doi: 10.1111/j.1096-3642.(2006)0.00205.x
Modesto, S. P. (2010). The postcranial skeleton of the aquatic parareptile Mesosaurus tenuidens from the Gondwanan Permian. J. Vert. Paleontol. 30, 1378–1395. doi: 10.1080/02724634.2010.501443
Modesto, S. P., Scott, D. M., MacDougall, M. J., Sues, H-D., Evans, D. C., and Reisz, R. R. (2015). The oldest parareptile and the early diversification of reptiles. Proc. R. Soc. B 282:20141912. doi: 10.1098/rspb.2014.1912
Molnar, J. L., Pierce, S. E., Bhullar, B. A. S., Turner, A. H., and Hutchinson, J. R. (2015). Morphological and functional changes in the vertebral column with increasing aquatic adaptation in crocodylomorphs. Royal Soc. Open. Sci. 2, 150–439. doi: 10.1098/rsos.150439
Molnar, J. L., Pierce, S. E., and Hutchinson, J. R. (2014). An experimental and morphometric test of the relationship between vertebral morphology and joint stiffness in Nile crocodiles (Crocodylus niloticus). J. Exp. Biol. 217, 758–768. doi: 10.1242/jeb.089904
Morales-Mávil, J. E., Vogt, R. C., and Gadsden-Esparza, H. (2007). Desplazamientos de la iguana verde, Iguana iguana (Squamata: Iguanidae) durante la estación seca en La Palma, Veracruz, México. Rev. Biol. Trop. 55, 709–715. doi: 10.15517/rbt.v55i2.6047
Motani, R., Jiang, D. Y., Tintori, A., Rieppel, O., and Chen, G. B. (2014). Terrestrial origin of viviparity in Mesozoic marine reptiles indicated by Early Triassic embryonic fossils. PLoS ONE 9:e88640. doi: 10.1371/journal.pone.0088640
Murphy, J. B., and Mitchell, L. A. (1974). Ritualized combat behavior of the pygmy mulga monitor lizard, Varanus gilleni (Sauria: Varanidae). Herpetologica 30, 90–97.
Nopcsa, F. B. (1923). Vorläufige Notiz über die Pachyostose und Osteosklerose einiger mariner Wirbeltiere. Anatomischer Anzeiger. 56, 353–359.
Oelofsen, B. W. (1981). An Anatomical and Systematic Study of the Family Mesosauridae (Reptilia, Proganosauria) With Special Reference to its Associated Fauna and Palaeoecological Environment in the Whitehill Sea. Unpublished PhD thesis, University of Stellenbosch.
O'Keefe, F. R., Sidor, C. A., Larsson, H. C. E., Maga, A., and Ide, O. (2006). Evolution and homology of the astragalus in early amniotes: new fossils, new perspectives. J. Morphol. 267, 415–425. doi: 10.1002/jmor.10413
Panchen, A. L., and Smithson, T. R. (1990). The pelvic girdle and hind limb of Crassigyrinus scoticus (Lydekker) from the Scottish Carboniferous and the origin of the tetrapod pelvic skeleton. Trans. R. Soc. Edinb. 81, 31–44.
Peabody, F. E. (1952). Petrolacosaurus kansensis Lane, a Pennsylvanian reptile from Kansas. Univ. Kans. Paleontol. Cont. Vertebrata 1, 1–41.
Pierce, S. E., Clack, J. A., and Hutchinson, J. R. (2011). Comparative axial morphology in pinnipeds and its correlation with aquatic locomotory behaviour. J. Anat. 219, 502–514. doi: 10.1111/j.1469-7580.2011.01406.x
Piñeiro, G. (2002). Paleofaunas del Pérmico-Eotriásico de Uruguay. Unpublished MSc. Thesis, Universidad de la República, PEDECIBA, Montevideo.
Piñeiro, G. (2004). Paleofaunas del Pérmico y Permo-Triásico de Uruguay. Bioestratigrafía, Paleobiogeografía y Sistemática. Unpublished PhD Thesis, Universidad de la República, Montevideo.
Piñeiro, G. (2006). “Nuevos aportes a la paleontología del Pérmico de Uruguay,” in Cuencas Sedimentarias de Uruguay– Paleozoico, eds G. Veroslavsky, S. Martínez, and M. Ubilla (Montevideo: DIRAC Facultad de Ciencias), 257–279.
Piñeiro, G. (2008). “Los mesosaurios y otros fósiles de fines del Paleozoico,” in Fósiles de Uruguay, ed D. Perea (Montevideo: DIRAC; Facultad de Ciencias), 179–205.
Piñeiro, G., Ferigolo, J., Meneghel, M., and Laurin, M. (2012a). The oldest known amniotic embryos suggest viviparity in mesosaurs. Hist. Biol. 24, 630–640. doi: 10.1080/08912963.2012.662230
Piñeiro, G., Ferigolo, J., Ramos, A., and Laurin, M. (2012b). Cranial morphology of the Early Permian mesosaurid Mesosaurus tenuidens and the evolution of the lower temporal fenestration reassessed. Comptes Rendus Palevol. 11, 379–391. doi: 10.1016/j.crpv.2012.02.001
Piñeiro, G., Núñez-Demarco, P., and Meneghel, M. (2016). The ontogenetic transformation of the mesosaurid tarsus: a contribution to the origin of the amniotic astragalus. PeerJ. 4:e2036. doi: 10.7717/peerj.2036
Piñeiro, G., Ramos, A., Goso, C., Scarabino, F., and Laurin, M. (2012c). Unusual environmental conditions preserve a Permian mesosaur-bearing Konservat-Lagerstätte from Uruguay. Acta Palaeontol. Pol. 57, 299–318. doi: 10.4202/app.2010.0113
Reisdorf, A. G., Anderson, G. S., Bell, L. S., Klug, C., Schmid-Röhl, A., Röhl, H. J., et al. (2014). Reply to “Ichthyosaur embryos outside the mother body: not due to carcass explosion but to carcass implosion” by van Loon (2013). Paleobiodiv. Paleoenviron. 94, 487–494. doi: 10.1007/s12549-014-0162-z
Reisdorf, A. G., Bux, R., Wyler, D., Benecke, M., Klug, C., Maisch, M. W., et al. (2012). Float, explode or sink: post-mortem fate of lung-breathing marine vertebrates. Paleobiodivers. Paleoenviron. 92, 67–81. doi: 10.1007/s12549-011-0067-z
Reisz, R. R. (1981). A diapsid reptile from the Pennsylvanian of Kansas. Univ. Kans. publ. Mus. Nat. Hist. 7, 1–74.
Reisz, R. R., Berman, D. S., and Scott, D. (1984). The anatomy and relationships of the Lower Permian reptile Araeoscelis. J. Vert. Paleontol. 4, 57–67.
Reisz, R. R., and Dilkes, D. W. (2003). Archaeovenator hamiltonensis, a new varanopid (Synapsida: Eupelycosauria) from the Upper Carboniferous of Kansas. Can. J. Earth. Sci. 40, 667–678. doi: 10.1139/e02-063
Reisz, R. R., and Fröbisch, J. (2014). The oldest caseid synapsid from the Late Pennsylvanian of Kansas, and the evolution of herbivory in terrestrial vertebrates. PLoS ONE 9:e94518. doi: 10.1371/journal.pone.0094518
Reisz, R. R., Modesto, S. P., and Scott, D. M. (2011). A new Early Permian reptile and its significance in early diapsid evolution. Proc. R. Soc. Lond. B. Biol. Sci. B. 278, 3731–3737. doi: 10.1098/rspb.2011.0439
Rewcastle, S. C. (1980). Form and function in lacertilian knee and mesotarsal joints; a contribution to the analysis of sprawling locomotion. J. Zool. 191, 147–170.
Rieppel, O. (1989). A new pachypleurosaur (Reptilia: Sauropterygia) from the Middle Triassic of Monte San Giorgio, Switzerland. Phil. Trans. R. Soc. Lond. B, 323, 1–73.
Romer, A. S. (1974). Aquatic adaptation in reptiles - primary or secondary? Ann. S. Afr. Mus. 64, 221–230.
Romer, A. S., and Price, I. L. (1940). Review of the Pelycosauria. Geol. Soc. Spec. Pub. 28, 1–538. doi: 10.1130/SPE28-p1
Ruta, M., Coates, M. I., and Quicke, D. L. J. (2003). Early tetrapod relationships revisited. Biol. Rev. 78, 251–345. doi: 10.1017/S1464793102006103
Ruta, M., and Clack, J. A. (2006). A review of Silvanerpeton miripedes, a stem amniote from the Lower Carboniferous of East Kirkton, West Lothian, Scotland. Trans. R. Soc. Edinb. Earth Sci. 97, 31–63. doi: 10.1017/S0263593300001395
Santos, R. V., Souza, P. A., Alvarenga, C. J. S., Dantas, E. L., Pimentel, E. L., Oliveira, C. G., et al. (2006). Shrimp U–Pb zircon dating and palynology of bentonitic layers from the Permian Irati Formation Paraná Basin, Brazil. Gondwana Res. 9, 456–463. doi: 10.1016/j.gr.2005.12.001
Schoch, R. R., and Milner, A. R. (2000). Handbuch der Paläoherpetologie: Stereospondyli, Teil 3, Verlag Dr. München: Friedrich Pfeil.
Sequeira, S. E. K., and Milner, A. R. (1993). The temnospondyl amphibian Capetus from the Upper Carboniferous of the Czech Republic. Palaeontology 36, 657–680.
Silva, R. R., Ferigolo, J., Bajdek, P., and Piñeiro, G. (2017). The feeding habits of Mesosauridae. Topic Res. Front. Earth Sci. 5:23. doi: 10.3389/feart.2017.00023
Smith, R. M. H. (2000). Sedimentology and taphonomy of Late Permian vertebrate fossil localities in Qsouthwestern Madagascar. Palaeontol. Afric. 36, 25–41.
Smithson, T. R. (1993). Eldeceeon rolfei, a new reptiliomorph from the Viséan of East Kirkton, West Lothian, Scotland. Trans. R. Soc. Edinb. Earth Sci. 84, 377–382. doi: 10.1017/S0263593300006180
Smithson, R. T., Carroll, R. L., Panchen, A. L., and Andrews, S. M. (1994). Westlothiana lizziae from the Viséan of East Kirkton, West Lothian, Scotland, and the amniote stem. Trans. R. Soc. Edinburgh. Earth Sci. 84, 383–412. doi: 10.1017/S0263593300006192
Stephenson, M. H. (2018). Permian palynostratigraphy: a global overview. Geol. Soc. Lond. Spec. Publ. 450, 321–347. doi: 10.1144/SP450.2
Storrs, G. W. (1993). Function and phylogeny in sauropterygian (Diapsida) evolution. Am. J. Sci. 293:63.
Sues, H. D., and Carroll, R. L. (1985). The pachypleurosaurid Dactylosaurus schroederi (Diapsida: Sauropterygia). Can. J. Earth. Sci. 22, 1602–1608.
Sumida, S. S., and Modesto, S. P. (2001). A phylogenetic perspective on locomotory strategies in early amniotes. Am. Zool. 41, 586–597. doi: 10.1093/icb/41.3.586
Szczygielski, T., Surmik, D., Kapuścinska, A., and Rothschild, B. M. (2017). The oldest record of aquatic amniote congenital scoliosis. PLoS ONE 12:e0185338. doi: 10.1371/journal.pone.0185338
Thompson, G. G., and Withers, P. C. (1997). Comparative morphology of western Australian varanid lizards (Squamata: Varanidae). J. Morphol. 233, 127–152.
Tsuji, L. A., Müller, J., and Reisz, R. R. (2012). Anatomy of Emeroleter levis and the phylogeny of the nycteroleter parareptiles. J.Vert. Paleontol. 32, 45–67. doi: 10.1080/02724634.2012.626004
Vallin, G., and Laurin, M. (2004). Cranial Morphology and Affinities of Microbrachis, and a Reappraisal of the Phylogeny and Lifestyle of the First Amphibians. J. Vertebr. Paleontol. 24, 56–72. doi: 10.1671/5.1
Villamil, J., Núñez Demarco, P., Meneghel, M., Blanco, R. E., Jones, W., Rinderknecht, A., et al. (2015). Optimal swimming speed estimates in the Early Permian mesosaurid Mesosaurus tenuidens Gervais, 1865 from Uruguay. Hist. Biol. 28, 963–971. doi: 10.1080/08912963.2015.1075018
von Huene, F. (1940). A idade Permiana Inferior de todas as camadas contendo messosáurios. Trad. G. Faria Alvin. Div. Min. Metal. Rio Janeiro 6, 64–68.
von Huene, F. (1941). Osteologie und systematische Stellung von Mesosaurus. Palaeontogr. Abt. A. 92, 45–58.
Wopfner, H. (1999). The early Permian deglaciation event between East Africa and northwestern Australia. J. Afr. Earth Sci. 29, 77–90.
Keywords: Mesosaurus tenuidens, morphometrics, lifestyle, semiaquatic, early permian, gondwanan pangaea, paleoecology
Citation: Nuñez Demarco P, Meneghel M, Laurin M and Piñeiro G (2018) Was Mesosaurus a Fully Aquatic Reptile?. Front. Ecol. Evol. 6:109. doi: 10.3389/fevo.2018.00109
Received: 19 April 2018; Accepted: 04 July 2018;
Published: 27 July 2018.
Edited by:
Martin Daniel Ezcurra, Museo Argentino de Ciencias Naturales Bernardino Rivadavia, ArgentinaReviewed by:
Torsten M. Scheyer, Universität Zürich, SwitzerlandJuan Carlos Cisneros, Federal University of Piauí, Brazil
Copyright © 2018 Nuñez Demarco, Meneghel, Laurin and Piñeiro. This is an open-access article distributed under the terms of the Creative Commons Attribution License (CC BY). The use, distribution or reproduction in other forums is permitted, provided the original author(s) and the copyright owner(s) are credited and that the original publication in this journal is cited, in accordance with accepted academic practice. No use, distribution or reproduction is permitted which does not comply with these terms.
*Correspondence: Pablo Nuñez Demarco, cG51bmV6QGZjaWVuLmVkdS51eQ==
Graciela Piñeiro, Zm9zc2lsQGZjaWVuLmVkdS51eQ==