- 1Department of Primatology, Max Planck Institute for Evolutionary Anthropology, Leipzig, Germany
- 2German Centre for Integrative Biodiversity Research (iDiv), Leipzig, Germany
- 3Max Planck Institute for Evolutionary Anthropology, Leipzig, Germany
- 4Department of Anthropology, Texas State University, San Marcos, TX, United States
- 5Department of Anthropology, Iowa State University, Ames, IA, United States
- 6Taï Chimpanzee Project, Centre Suisse de Recherches Scientifiques, Abidjan, Côte d'Ivoire
Savanna-mosaic habitats are thought to represent exceptional circumstances for chimpanzees (Pan troglodytes), and owing to the virtues of their habitat as well as peripheral biogeographic location, they are often regarded as marginal to the chimpanzee ecological niche. If these habitats are marginal, then we should expect that chimpanzees living in these habitats demonstrate physiological consequences of the extremity of this environment. We therefore compared seasonal variation in physiological responses to climatic and ecological factors in chimpanzees inhabiting Fongoli, a savanna-mosaic habitat at the margins of the chimpanzee range with chimpanzees from Taï National Park, a lowland rainforest centrally located within the West African chimpanzee subspecies (P. troglodytes verus) range. We accomplished this using urinary biomarkers of hydration (creatinine), energetic status (c-peptide), and stress (cortisol) collected simultaneously from research stations at each location. We found that Fongoli was both more extreme and seasonal in climatic measures like temperature and rainfall, although food availability was more variable at Taï than at Fongoli. Although living in an extreme and seasonally more variable environment, Fongoli chimpanzees were more stable in their c-peptide values than Taï chimpanzees, but showed more extreme variation in their cortisol values. Although chimpanzees at both sites demonstrated significant seasonal effects of dehydration (creatinine), the more extreme environmental variation at Fongoli promoted higher physiological seasonal costs in the form of elevated cortisol levels. Overall, these results supported the assertion that Fongoli as a savanna-mosaic habitat is more extreme in its climate and ecology than a forested site. It appears that extreme savanna-mosaic habitats represent a limit to the chimpanzee ecological niche with regard to thermoregulation, in that seasonal environmental conditions, namely the hot and dry conditions of the dry season, strain an individual's ability to maintain homeostasis. However, Taï chimpanzees also faced dehydration as a limitation, which highlights that chimpanzees may experience certain challenges ubiquitously across their range, while other challenges remain habitat-specific. Hence, categorizing savanna habitats as universally more severe and challenging to chimpanzees than more heavily forested habitats does not yet appear to be warranted.
Introduction
Marginal habitats, typically peripheral in a spatial perspective of a species' biogeographic range (Kawecki, 2008; Sexton et al., 2009), are useful in understanding a species' ecological niche and therefore its environmental tolerance. These habitats typically represent “fuzzy transitional zones” between habitats in which conditions are favorable and those which are inhospitable, and fall beyond the limits of the “n-dimensional niche space” of a species (Hutchinson, 1961; Holt, 2009). In this sense, marginal habitats therefore can be defined as “edge of niche” habitats, in which “environmental conditions in the area represent only a marginal part of the species' fundamental niche” (pg. 154 Braunisch et al., 2008). In comparison with “core” habitats, populations in marginal habitats are sometimes representations of range disequilibrium and are typically population sinks characterized by low densities as well as low rates of survival and reproduction, which are largely sustained by immigration (Pulliam, 2000; Kawecki, 2008; Sexton et al., 2009). A marginal habitat for a species may fit niche requirements most of the time, but allow little safety-margin for environmental fluctuation, thereby creating the risk that this habitat may periodically exceed tolerable limits for individuals living there (Kawecki, 2008). Therefore, these habitats offer great potential for our understanding of the potential physiological consequences for individuals already living near the edge of their tolerable ecological niche space.
Most research centered on species' habitat marginality is either theoretically- or experimentally-based work concerning small-bodied and short lived species (see Sexton et al., 2009 for a review). Some exceptions exist, such as Chilvers et al.'s (2006) work with sea lions (Phocarctos hookeri). The West African chimpanzee (Pan troglodytes verus), however, offers a unique opportunity to examine marginality in a large-bodied and long lived mammal, as its biogeographic range extends across an extensive ecological gradient ranging from lush rainforest to dry savanna mosaics.
Savanna-mosaic habitats generally lie on the periphery of the chimpanzee's historical range, and as such, most research on savanna-mosaic chimpanzees was conducted under the assumption that these habitats are marginal to the chimpanzee ecological niche (McGrew et al., 1981; Baldwin et al., 1982; Moore, 1992; Hunt and McGrew, 2002; Pruetz and Bertolani, 2009). This assumption persists despite little effort to define potential chimpanzee environmental limits (McGrew et al., 1981; Kortlandt, 1983), and the predominant focus of chimpanzee research undertaken in wetter, more forested habitats (e.g., forested research sites significantly outnumber open habitat sites, e.g., 3 of 17 sites in Kühl et al., 2016). Conversely, however, others have argued that the extent of savanna habitats within the chimpanzee biogeographical range indicates that this is a habitat to which they are equally suited (Kortlandt, 1983).
In absence of comparative demographic or life history characteristics, some circumstantial evidence appears to support this marginal categorization. For example, it is well established that chimpanzees in savanna habitats occur at lower densities than chimpanzees in more heavily forested habitats (e.g., Baldwin et al., 1982; Pruetz et al., 2002; Matthews and Matthews, 2004; Kouakou et al., 2009; Potts et al., 2009; Arandjelovic et al., 2011; Després-Einspenner et al., 2017). By definition, savanna-mosaic habitats have lower tree density and diversity than habitats with continuous forest cover (Crowther et al., 2015), which in turn is expected to necessitate larger chimpanzee home range size to maintain dietary requirements (Mitani and Rodman, 1979; Baldwin et al., 1982; Pruetz and Bertolani, 2009). Floral diversity is likewise consistently lower in savanna habitats, which has itself been proposed to be limiting to the chimpanzee biogeographical range (Kortlandt, 1983). This reduced floral diversity in savanna habitats is reflected in lower chimpanzee dietary breadth (Suzuki, 1969; McGrew et al., 1988; Pruetz, 2006; Lindshield et al., 2017; Piel et al., 2017), and has been argued as an indicator that savanna chimpanzees face resource scarcity (Pruetz, 2006; Bertolani and Pruetz, 2011; Lindshield, 2014).
Additionally, savanna habitats are notably drier than rainforest or forested habitats (Figure 1; McGrew et al., 1981; Moore, 1992), and at some chimpanzee research sites, considerably hotter as well. Drier sites are assumed to impose considerable hydration pressure on chimpanzees, which we have previously demonstrated (Wessling et al., 2018). This dehydration pressure likely originates from the coupling of high temperatures and the timing of particularly water-scarce periods, when little to no standing water is available, and chimpanzees must choose between meeting other basic requirements (i.e., feeding, socializing) and hydration maintenance. Lastly, savannas are also expected to be considerably more seasonal than more moderate habitats (Alberts et al., 2005; Piel et al., 2017). In order to counter the challenges of heat, water, and nutritional or energetic stresses faced by chimpanzees at the margins of their ecological niche, flexible dietary or behavioral strategies to meet shifting requirements may be needed if and when these challenges are encountered simultaneously.
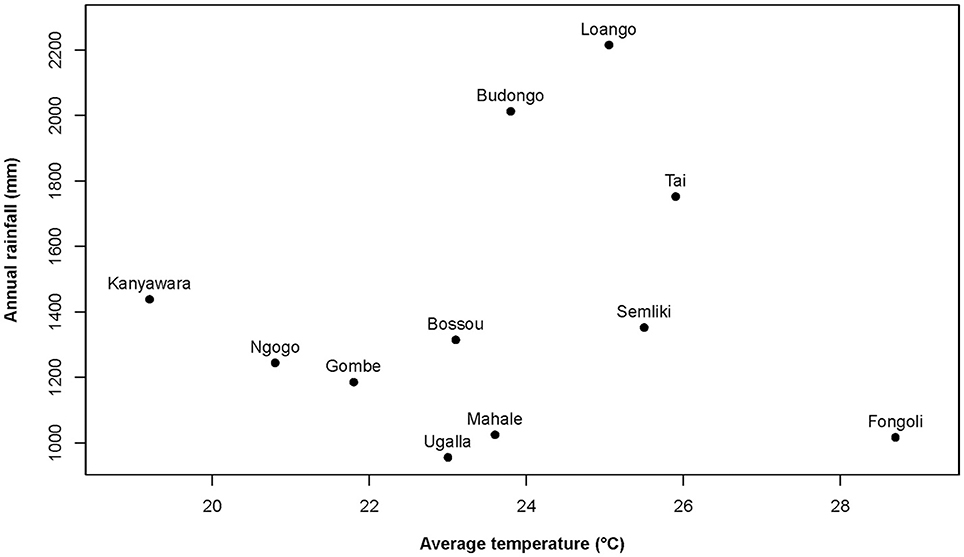
Figure 1. Average annual temperature (°C) and annual rainfall (mm) for chimpanzee long-term research sites (n = 10) (Data are derived from Head et al., 2011; Matsuzawa et al., 2011, this study; Lindshield, 2014; Piel et al., 2017).
As such, our interpretation of the breadth of chimpanzee ecological and environmental tolerance shapes our perception regarding how adaptable and flexible a species like the chimpanzee can be in their behavior, physiology, or ecology, as well as to the range of conditions which can be considered suitable habitat. Additionally, implicit in the “marginal” denomination is the presumption that forested habitats are more suitable habitats for chimpanzees (Boesch, 2009), and that savanna habitats represent an environment more extreme in its nature and in which chimpanzees should be expected to experience physiological stress.
Fongoli, Senegal, at the edge of the West African chimpanzee range, is considered to be at the extremes of a habitable ecological continuum for chimpanzees, and a coarse comparison of climatic data supports this assumption (Figure 1). McGrew et al. (1981) surmised that the savanna-woodlands of southeastern Senegal are the most marginal of all ecosystems inhabited by chimpanzees within the whole of their range. We have previously demonstrated that Fongoli chimpanzees experience seasonal periods of heat and water constraints, but although they do show variation in energetic status, energetically difficult periods do not appear to be stressful enough to elevate cortisol excretion (Wessling et al., 2018). However, it is only by comparison with a relatively non-seasonal site that we can understand the relevance of seasonal variation in biomarkers of physiological conditions and how they relate to the limits of the chimpanzee ecological niche. Therefore, if these habitats are marginal, we should expect that chimpanzees living in these habitats demonstrate more variable physiological responses to the extremity of this environment than chimpanzees in more moderate habitats. More specifically, if savanna chimpanzees are challenged by seasonal variation in environmental conditions to greater degrees than chimpanzees living in wetter, more closed-canopy habitats, then we should expect these constraints to be realized physiologically in two possible ways. Either we should expect that biomarkers of these constraints in chimpanzees at this site indicate greater physiological stress at an absolute scale, or that the degree of seasonal variation of these biomarkers is greater relative to forest-dwelling chimpanzees.
We therefore compared physiological, climatic, and ecological data collected at Fongoli (Wessling et al., 2018) with data collected during the same period from two chimpanzee communities at Taï National Park, Côte d'Ivoire. If Fongoli is assumed to represent the limit of a habitable environmental continuum for chimpanzees, then Taï might be near the opposite end of this continuum, as it is the wettest of research sites for the West African subspecies, and demonstrates comparatively minor seasonal variation in climatic parameters (Anderson et al., 2006, this study). Although moderate seasonal fluctuations in rainfall and food availability are known to occur at Taï, seasonal variation in responses to food and water availability are expected to be less pronounced in Taï chimpanzees, who are not expected to experience stress associated with heat or dehydration (Anderson et al., 2006). We accomplished this comparison using three well established urinary biomarkers (creatinine, c-peptide, and cortisol) of hydration, energetic status, and stress, respectively. Typically used as a measure of urinary concentration (Anestis et al., 2009), we employed the byproduct creatinine as a marker of an individual's hydration status. C-peptide, a peptide of insulin and therefore indicative of an individual's metabolic status, has recently been demonstrated to be a suitable biomarker for monitoring energetic status, especially in non-invasive studies of wild primates (Sherry and Ellison, 2007; Emery Thompson and Knott, 2008; Emery Thompson et al., 2009, 2016; Girard-Buttoz et al., 2011; McCabe et al., 2013; Grueter et al., 2014; Surbeck et al., 2015). Lastly, although cortisol variation is a non-specific measure of general physiological stress (Beehner and Bergman, 2017), we mirror other vertebrate research (Christison and Johnson, 1972; El-Halawani et al., 1973; Edens and Siegel, 1975; Harikai et al., 2003; Beehner and Bergman, 2017; Wessling et al., 2018), which has linked cortisol response to thermal challenges. While substantial literature on these biomarkers has already provided valuable insights into the lives of chimpanzees (e.g., creatinine as an indicator of muscle mass and growth: Emery Thompson et al., 2012, c-peptide variation in response to food availability and dominance status: Emery Thompson et al., 2009, and cortisol as a marker of multiple forms of physiological and psychological stress: Muller and Lipson, 2003; Anestis and Bribiescas, 2004; Muller and Wrangham, 2004; Emery Thompson et al., 2010), these results are not easily comparable across laboratories that employ different methods and tools for measuring them.
We intend to offer insight into the manner in which the assumed marginal savanna habitat imposes stresses on chimpanzees in comparison to presumably lusher habitats considered to promote higher chimpanzee population density (Boesch, 2009; Watts et al., 2012), thereby contributing to a better understanding of the chimpanzee ecological niche itself. Specifically, we hypothesize that the extreme and seasonal environment at Fongoli is physiologically more taxing, either overall or seasonally (or both), than less extreme and seasonal sites like Taï. As such, we predicted that biomarkers of dehydration, energetic status, and thermoregulatory stress at Fongoli (a) will be higher overall in levels of creatinine and cortisol and lower levels in c-peptide, and (b) show stronger seasonal variation than biomarkers of Taï chimpanzees. Additionally, we compare seasonal variation in environmental variables, and explore the contribution of these variables to variation in these biomarkers, with the expectation that these variables differ in their contribution to physiological constraints among the sites.
Materials and Methods
We compare our previously published dataset (Wessling et al., 2018) collected at the Fongoli Savanna Chimpanzee Project (FSCP; Pruetz and Bertolani, 2009) field site in south-eastern Senegal (12°40′N, 12–13′W) with data collected simultaneously from two chimpanzee communities (i.e., Taï East and Taï South) at the Taï Chimpanzee Project (TCP; Boesch and Boesch-Achermann, 2000) in south-western Côte d'Ivoire (5°45′N, 7°07′W) between January 2013 and January 2014. Previously, we have demonstrated that Fongoli chimpanzees exhibit seasonal variation in biomarkers of hydration, energetic status, and thermoregulatory stress, and that this variation was best explained by expected environmental correlates (Wessling et al., 2018). Nearly identical data collection protocols were followed in all three communities; below we describe key methodologies at both sites, with particular attention to the few methodological differences when they exist. A full description of methods specific to Fongoli analyses can be found in Wessling et al. (2018).
The Fongoli community (n = 31 individuals, 90 km2 home range; Pruetz, 2018) inhabits a savanna-mosaic environment characterized by distinct annual changes in precipitation between the wet (June to September) and the dry (November to April) season, with May and October representing transitional months (Pruetz and Bertolani, 2009). Temperatures at Fongoli reach as high as 48°C (Wessling et al., 2018) with an average annual rainfall of 945 mm that is typically confined only to four wet season months (FSCP database, unpublished, 2005 to 2012). The two TCP communities (Taï South: n = 41 individuals, 25 km2 home range; Taï East = 33 individuals, 28 km2 home range; Luncz and Boesch, 2015) have adjacent territories located in the Taï National Park, which consists of continuous primary lowland rainforest characterized by two wet (March-June, August- November) and two dry seasons (December- February, July; Boesch and Boesch-Achermann, 2000). Rainfall at Taï averages 1,759 mm annually, with temperatures averaging 25. 9°C but reaching as high as 36°C (Boesch, 2009, this study).
In order to test our predictions, we first determined the extent to which the Taï environment varied seasonally relative to Fongoli. We next investigated whether or not Taï chimpanzees demonstrated seasonal physiological variation in three key biomarkers of heat (cortisol), hydration (creatinine) and nutritional (c-peptide) stress, and to relate this variation to seasonal variation of these biomarkers in Fongoli chimpanzees. Finally, we investigated potential environmental measures responsible for the biomarker variation observed at each site. In order to perform these tests, we acquired a wealth of environmental, behavioral, and physiological data collected at both sites, which we have detailed below. This non-invasive research was carried out in accordance with the recommendations of the American Society of Primatologists (ASP) Principles for the Ethical Treatment of Non-Human Primates, and the protocol was approved by the Max Planck Society.
Field Data Collection
Climate Data Collection
Climatic and rainfall data were collected daily at the base camps of each community, located within each community's home range. Rainfall data were collected using a rain gauge, and measurements below the measureable limit of the gauge were set to the detection limit (0.1 mm). At Fongoli, a data logger (Onset Hobo Temp/RH Data Logger) was used to collect ambient temperature and relative humidity at 10-min intervals, in addition to a min-max thermohygrometer that was checked manually and reset daily on days when a researcher was at camp. At Taï, min-max thermohygrometers were checked and reset daily for each community. Daily temperature and humidity means were calculated as the average of the minimum and maximum temperature for the day, following the methods described in Wessling et al. (2018). We calculated daily mean heat index following the methods used by the National Oceanic and Atmospheric Administration (NOAA) using daily mean temperature and humidity.
Food Availability Index (FAI) Data Collection
For chimpanzees, a ripe-fruit specialist, we calculated three potentially relevant food availability indices (total food [FAItotal], total fruit [FAIfruit,], and ripe fruit [FAIfruit,]), monthly for each community, using data collected within each community home range, using the methods described in Wessling et al. (2018). We calculated FAItotal, FAIfruit, and FAIripe based on all plant-based food items, ripe, and unripe fruit items, and only ripe fruit items eaten by chimpanzees at each site, respectively. At Fongoli, Taï East and Taï South, phenological data on 887, 925, and 623 trees were1 collected monthly within each community's territory, respectively. Phenological data from the Taï South community territory were not available for January 2014; therefore these data were not included in any of the models. For Fongoli, we calculated density based upon the 6.8 km2 transect from which phenological data were collected. For Tai, we obtained tree density estimates from Goné Bi (2007), and used food tree density estimates from the Taï South community territory for Taï South group, but averaged food tree densities from three Taï community territories (Taï North, Taï Middle, and Taï South territories) for the Taï East community territory as these data were not available. Due to the difficulty in species identification of specific genera, we grouped species within two genera (Ficus and Lannea) and calculated the FAIs accordingly for all dietary items present.
Urine Sample Collection
We used urinary c-peptide, creatinine, and cortisol levels to determine potential physiological responses to variation in nutrition, water consumption, and heat exposure respectively. At Fongoli, samples were collected in the morning below night nests and during focal follows of adult individuals, although sample collection was not restricted to focal individuals but were collected ad libitum. As urine trickles off leaves and infiltrates the soil rather rapidly, we estimate that no urine sample collected under a night nest was voided more than 1 h prior to aspiration beneath a nest. We therefore used the time a sample was collected as a proxy for urination time and used these values when correcting for diurnal variation in each statistical model (see below). At Taï, samples were only collected if an individual was seen urinating. Histograms on the distribution of samples collected across the day for both sites are given in the supplementary information. All samples were stored frozen until shipped to the Endocrinology Laboratory at the Max Planck Institute for Evolutionary Anthropology in Leipzig, Germany.
For comparison with our dataset of Fongoli urine samples (N = 368), we selected a subset of 220 Taï adult urine samples from the TCP long-term database, collected from Taï East (N = 124) and Taï South (N = 96) communities during the study period. Following a power analysis, we determined one sample per individual per month to be an adequate sample size for comparison with the Fongoli dataset (N = 368, mean ± SD = 18.4 ± 8.6 samples/individual, range = 2–33 samples/individual) and used this as a goal in our sample selection. However, this sampling frequency was not available for all Taï adults and we therefore only included individuals for whom there were no gaps of longer than 2 months between samples. If more than one sample was available per individual per month, preference was given to samples collected before 1200 h to better match the Fongoli dataset (Figure S2). Lastly, if more than one sample fitting the aforementioned requirements was available, we selected samples with the highest sample volume to allow for later analyses of the same samples. Using these criteria, we were able to include 12 adult individuals from Taï East community (seven females, five males) and nine adult individuals from Taï South community (six females, three males) into our analyses. These sample sizes (N = 220, mean ± SD: 10.5 ± 1.2 samples / individual, range: 7–12 samples/individual) are comparable to the Fongoli dataset.
Behavioral Data Collection
To control for the potential influence of behavioral correlates on biomarker variation, we collected behavioral data using full-day focal animal sampling (Altmann, 1974) of adult individuals (>11 years) on 289, 280, and 267 days during the study period (total focal hours across all communities = 12675.3 h) at Fongoli, Taï East, and Taï South, respectively. Focal follows (period of behavioral data collection from a single individual) were used with the assumption that focal follow behavior are representative of the behavior of all sampled individuals on that day, as our behaviors of interest (see below) are typically performed by all present individuals in the party. To account for the effects of drinking behavior on creatinine levels, we determined the number of drinks per focal observation time on the day prior to the sampling day as an estimation of water consumption. If more than one observer collected data on a community on a given day, the drinks per observation time were averaged over the focal follows unless there was a corresponding urine sample on the day following a focal follow of that individual, at which occasion behavioral data pertaining to that individual were used instead of the community average.
Samples collected from females at Taï who gave birth within 8.5 months' post-sample collection were classified as pregnant samples. Furthermore, since miscarriages may occur unnoticed by researchers, we examined all remaining female samples for exceptionally high pregnanediol levels indicative of pregnancy and detected four samples with high pregnanediol values. We therefore performed pregnancy tests upon these samples; all tests were negative and therefore these samples were included in our dataset as non-pregnant. No Fongoli females were pregnant during the study period.
We used the number of oestrus females seen over the course of a focal follow at Fongoli and the total number of oestrus females observed within each community by all observers at Taï as a control variable in all cortisol models. We used this variable as a rough proxy for perceived social stress (Muller et al., 2007; Emery Thompson et al., 2010; Surbeck et al., 2012), as periods when a female is in oestrus have been shown to be a particularly stressful time for both males and females. Chimpanzee pant-grunt vocalizations are commonly used for the measurement of dominance hierarchies, as they are highly uni-directional indicators of relative dominance among dyads (Goodall, 1986; Muller and Wrangham, 2004). We therefore used pant-grunt vocalizations observed between December 2012 and January 2014 (N = 532, 557, and 441 for Fongoli, Taï South, and Taï East communities, respectively) among same sex dyads during focal follows (as chimpanzee females are ubiquitously lower ranking than adult males across field sites), to rank individuals using David's Scores (1 = lowest, nmax = group alpha; Gammell et al., 2003). There was one observed rank change in Taï South during the study period (November 2013) when the alpha position was overtaken by the beta male, at which point we reversed their assigned ranks accordingly. To allow for comparison of ranks across different communities of different sizes, we standardized rank by transforming them to a range of 0 (most subordinate) to 1 (alpha).
Chimpanzees live in groups characterized by fission-fusion social organization, and are well-known to vary their traveling parties according to ecological factors (Goodall, 1986; Anderson et al., 2002). Therefore, to control for the confounding effects of varying party size, we included party size as a control predictor in all c-peptide models. At Fongoli, party size included all of the observed individuals, however two different observer methods for party size definition were included in our dataset; either as daily means of 15 min scans, or as the number of unique individuals observed throughout the course of a day. At Taï, party composition was continuously recorded, therefore we calculated daily average party size using a weighted mean incorporating duration of each party composition. Accordingly, we also included the type of data summary (daily average or total seen) and its interaction with party size into the c-peptide models to account for the discrepancy in methods used at Fongoli.
Laboratory Analyses
Full details about sample analysis can be found in Wessling et al. (2018). In brief, we successfully analyzed 569 samples for c-peptide levels using a commercially available immunoassay kit (DIAsource, C-Pep-EASIA KAP0401). Intra- and inter-assay coefficients of variation of low and high value quality controls for assays of both Taï and Fongoli samples were 2.2 and 6.6% (N = 4) and 6.2 and 5.6% (N = 23), respectively. We were unable to obtain reliable measurements for four Taï samples before aliquots were depleted, which fell above the linear range of the assay after multiple attempts. Ten Taï samples measured below the minimum measurable value of the assay, so we therefore assigned the value of 0.078 ng/ml [half the lowest measureable value of the assay (0.155 ng/ml)]. C-peptide values were corrected by creatinine and are expressed in ng/mg creatinine, with the exception of their use as a predictor in cortisol models, for which they were corrected by specific gravity (SG) and are expressed in ng / ml SG (to avoid confounding c-peptide and creatinine).
Urinary cortisol levels were measured using liquid chromatography–tandem mass spectrometry using an adaptation of Hauser et al.'s (2008) method (see Wessling et al. (2018) for details), and are expressed in ng/ml SG. We successfully measured all but three Taï samples for cortisol following Hauser et al. (2008). However, we corrected cortisol levels of chimpanzee urine samples using deuterated testosterone because a coelution at the retention time of the traditional internal standard for cortisol, prednisolone, prevented us from using this standard. In total 577 samples were successfully measured for cortisol from Fongoli and Taï. Lastly, specific gravity was measured using a refractometer (TEC, Ober-Ramstadt, Germany), and creatinine was measured via colorimetric reaction with picric acid (Bahr et al., 2000) for samples from all three communities (N = 587).
Statistical Analyses
To test our hypotheses, we conducted four sets of analyses (see Supplementary Material 1 for model sets and individual model construction) using the statistical program R (version 3.3.1; R Core Team, 2016). Models were fitted across the entire dataset (both Fongoli and Taï sample sets) using Linear Mixed Models (LMMs; Baayen, 2008) with a Gaussian error structure and identity link (McCullagh and Nelder, 1989) using the package “lme4” (Bates et al., 2015) unless otherwise noted. All quantitative predictors (except sine and cosine of Julian date and rank) were z-transformed (to a mean of zero and a standard deviation of one) to ease model convergence and achieve comparability of estimates. All physiological models across sites also included a random effect of individual and random slopes of all quantitative predictors (except party size) within the effect of individual, but we did not include the correlation parameters between random slope terms and their intercepts (Schielzeth and Forstmeier, 2009; Barr et al., 2013) as such models did not converge.
Environmental and Physiological Seasonal Analyses
Initially, we tested for differences between the sites in seasonal variation of 13 environmental variables (models 1.1–1.13) as well as the three physiological indicators (creatinine, c-peptide, cortisol; models 2.1–2.3). For the physiological models to meet the assumptions of normally distributed and homogenous error, c-peptide, and cortisol were log transformed whereas creatinine was square root transformed. To test for seasonal effects, we included into each model a seasonal term allowing for a single seasonal cycle and represented by both sine and cosine of Julian date (divided by 365.25 and then multiplied by 2π; Stolwijk et al., 1999) at which a urine sample was collected (physiological models 2.1–2.3) or to which the data correspond (environmental models 1.1–1.13). In order to test for the differences between the two sites we included an interaction between the two seasonal terms (sine and cosine of Julian date) and site, while controlling for potential differences between the two Taï communities with an additional term (“south”).
To account for potentially confounding effects on physiological status, we included several additional control predictors in the three biomarker models. None of the effects of these control predictors are expected to differ between sites, and therefore we did not include their interactions with the “site” term. Sample collection time was used to control for any potential diurnal effects on the indicators of interest. We also included individuals' dominance rank and sex to account for physiological differences among individuals. We used the proxy of number of oestrus females present in the focal party on the day prior to sample collection to control for potential social stress in the cortisol model. Additionally, we included the number of times the focal animal drank on the previous day, as well as the interaction between average party size and the method used to measure it as control predictors in the creatinine and c-peptide models, respectively (see Wessling et al. (2018) for details). To control for seasonal variation of cortisol independent of potential influence from creatinine or c-peptide variation, we also included both biomarkers as control predictors (log and z-transformed) in the cortisol model.
Inter-Site Seasonal Comparison
In order to test for the differences in each response between the two sites, we compared the fit of the full models (using a likelihood ratio test) with that of a respective null model lacking the term “site” (and therefore also the respective interactions), but being otherwise identical. As we were interested in determining whether the seasonal variation at the two sites differed in magnitude but not whether it differed in timing, we fitted a seasonal model with date adjusted such that the seasonal pattern was synchronous in the two sites (i.e., identical timing of peaks; models 2.1–2.3). Then to test the interaction between site and season we conducted a likelihood ratio test of the adjusted date seasonal model with a reduced model lacking only the interaction.
Second, as we were also interested in the differences between sites in the effect of both creatinine and c-peptide on cortisol variation, we fitted an identical model (model 3.1) to that described above for cortisol, however using the original unadjusted seasonal variation. To evaluate the effect of creatinine and c-peptide on cortisol, we compared the full model with models lacking each predictor one at a time (Barr et al., 2013).
We used visual inspection of qq-plots and residuals plotted against fitted values to verify that each model met the assumptions of normally distributed and homogeneous residuals. These plots indicated three extreme outliers (residual values <-3) in the cortisol model originating from exceptionally low cortisol values in the Fongoli dataset. We therefore re-fitted the same model excluding these three samples, and found clear improvement in adherence to assumptions of normality and homogeneity of residuals and dropped these three points from the data. We evaluated model stability by excluding levels of the random effects one at a time and comparing the estimates derived from these datasets with those derived for the full dataset and found that all three models were sufficiently stable. Variance Inflation Factors (VIF) determined for a standard linear model excluding the random effects and interactions using the function vif of the R-package car (Fox and Weisberg, 2011) indicated minor problems with collinearity between specific predictors, namely site and sex in the physiological models (maximum VIFs for creatinine: 3.17, c-peptide: 3.25, and cortisol models: 3.19). This was likely due to the imbalance between each site in sexes sampled: our dataset from Fongoli was strongly male-biased whereas Taï samples were strongly biased toward female individuals. However, as we included a considerable number of female and male samples from both sites, such level of collinearity does not pose a problem. Sample sizes for each models are listed in the captions of the corresponding tables.
Environmental Drivers of Physiological Variation
Thirdly, to test for the effect of environmental variables on the three biomarkers, we replaced the generic seasonal term in models 2.1–2.3 with several potentially relevant predictors interacting with site (models 4.1–4.3). We fitted these models regardless of the outcome of the adjusted-seasonal models, as although seasonal variation may or may not differ between sites, the influence of potential environmental predictors should explain differences in timing in seasonal variation of the three biomarkers between the two sites.
To reduce redundancy among the environmental variables, we condensed several similar climatic variables (daily means, minima, maxima) of relative humidity, and temperature as well as mean heat index of all three communities into two uncorrelated factors using Factor Analysis (Supplementary Tables S2, S3). In the subsequent models we included the factors scores revealed by the Factor Analysis that we extracted using the regression method as predictors. We excluded daily minimum temperature from the Factor Analysis because when included, this variable alone loaded on a third factor. We therefore excluded it from our models as this variable showed strong correlations with our two climate Factors when included separately. Maximum, minimum, and mean relative humidity loaded strongest on factor one, which is hereafter referred to as “relative humidity,” whereas maximum, minimum, and mean temperature loaded strongest upon factor two, which we hereafter simply referred to as “temperature.” Following the Factor Analysis, we replaced the generic seasonal term in the creatinine and cortisol models with these two factors, whereas in the c-peptide model we replaced this term with our three food availability indices. Additionally, we included the amount of rainfall on the day prior to each sample to account for the possibility of potential sample dilution from standing rainwater on surfaces from which samples were aspirated. We included this term as an interaction with site, as sample collection (although ideally identical) may have varied between sites. Lastly, in absence of a direct or indirect measure of overall water availability at both sites, we also included total monthly rainfall as a rough proxy of overall wetness at each location.
VIFs of models comprising the environmental factors indicated problems with collinearity among predictors (maximum VIF: 50.35). Subsequently, we discovered a lack of overlap of the distributions of most environmental predictors (i.e., both climate factors and FAIs) between the sites, thereby greatly limiting the explanatory power of our models. To overcome this issue, we applied within-community centering for all environmental predictors except rain and monthly rain, whereby we subtracted the respective community mean per variable from each value of a given variable. Following the centering of environmental variables, VIFs suggested collinearity problems had been reasonably resolved (max VIF: 3.22 for “sex”).
We then compared the fit of each environmental model to a null model comprising all original predictors except “site” and the corresponding two-way interactions using a likelihood ratio test (Dobson, 2002). If this test was significant, we evaluated the effect of each site-environmental predictor interaction on the biomarkers by comparing the full with a respective reduced model lacking the interaction using a likelihood ratio test. We verified that residuals were normally distributed and homogenous, as well as that the models were sufficiently stable and that predictors did not show collinearity problems, as described above.
Post-hoc Analyses
Lastly, to further understand the effects of environmental variables on c-peptide and cortisol individually at each site, we re-fitted these environmental models on datasets from each site (models 5.1–5.4). These were not specific tests of our hypotheses and therefore are considered exploratory post-hoc analyses. For all tests, we considered p-values 0.05 as statistically significant and p-values between 0.05 and 0.10 as trends. Model formulations of all model sets can be found in Supplementary Material 1.
Results
Environmental Seasonal Variation (Models 1.1 Through 1.13)
At Taï, all climatic variables (temperature, rainfall, humidity, heat index) and food availability indices significantly varied seasonally within the year (models 1.1–1.13; Figure 2; Supplementary Table S2; Supplementary Figure S1). Moreover, the magnitude of seasonal variation in climatic variables was generally stronger in Fongoli as compared to Taï (all but one p < 0.001; rainfall: p = 0.018). On the contrary, food availability and total fruit availability showed higher intra-annual variation at Taï (both p < 0.001) than in Fongoli. Community averages for all environmental and physiological variables can be found in Supplementary Table S1.
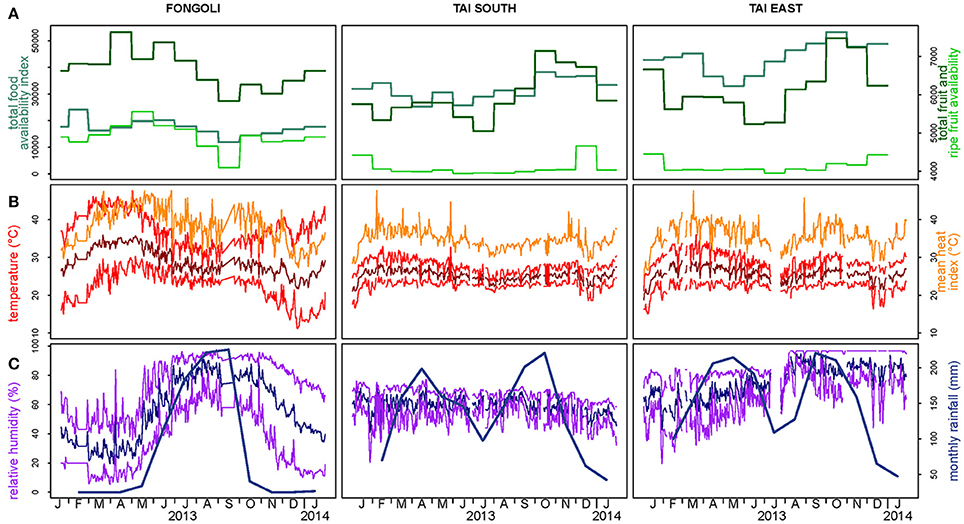
Figure 2. Variation during one annual cycle (2013–2014) of environmental variables for three chimpanzee communities. These include (A) total (dark green, bold), total fruit (green), and ripe fruit availability (light green) indices, (B) daily temperature maxima, means, and minima (red) and daily mean heat index (orange), and (C) daily relative humidity maxima, means, and minima (purple) and rainfall per month (blue).
Comparison of Physiological Variation (Models 2.1 Through 3.1)
The magnitude of seasonal variation in creatinine values did not significantly differ between the two sites, nor did average creatinine values significantly differ between sites (model 2.1, controlling for the timing of seasonal; full-null model comparison: χ2 = 0.569, df = 3, p = 0.903). These results were further supported when testing the interaction between site and season specifically, which did not reveal significance (χ2 = 0.239, df = 2, p = 0.887; Table 1A, Figure 3A). In fact, the two sites showed strong overlap in their average creatinine values as well as the amplitude of seasonal variation.
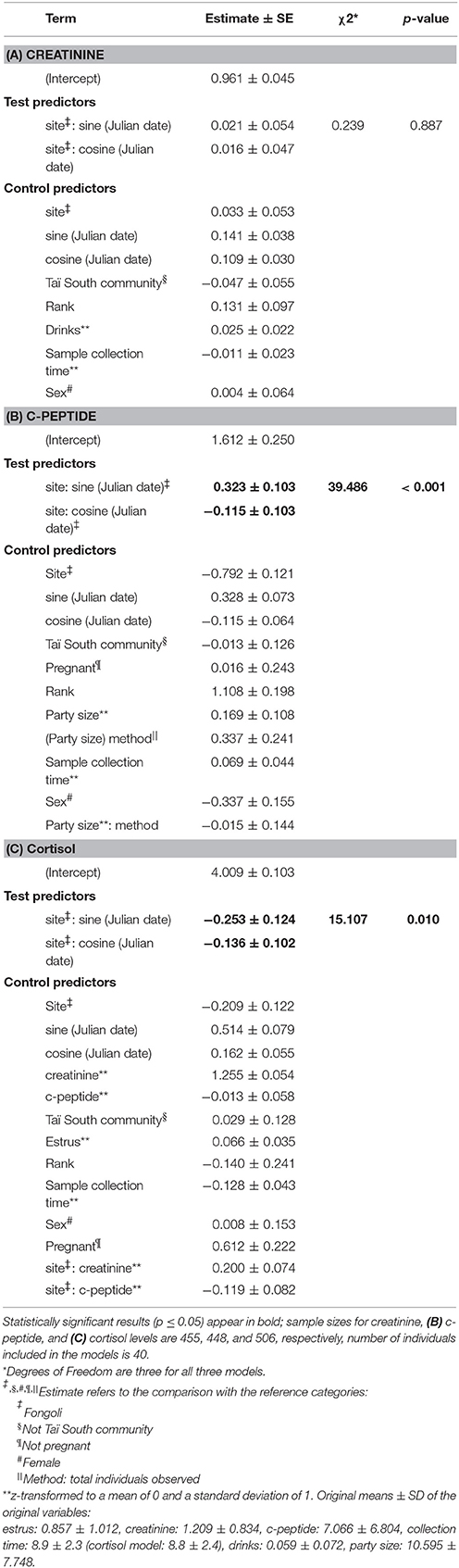
Table 1. Results of models comparing seasonal variation in (A) creatinine, (B) c-peptide, and (C) cortisol levels.
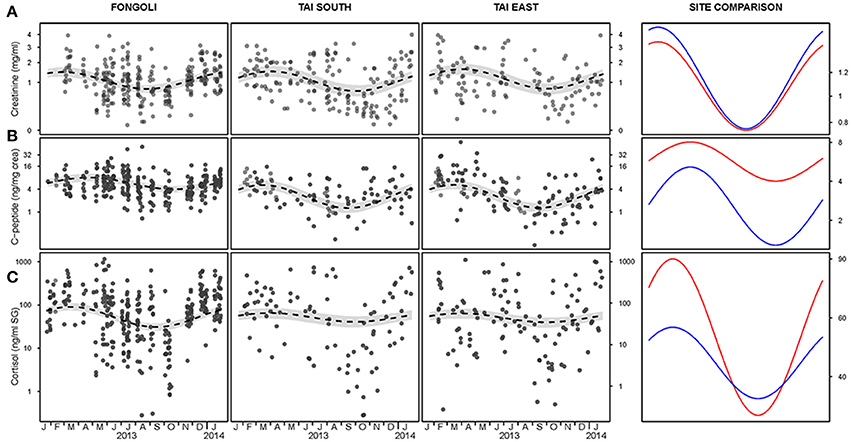
Figure 3. Adjusted and unadjusted seasonal variation of creatinine (A), c-peptide (B), and cortisol (C) levels at three chimpanzee communities. Fongoli is displayed in red and Taï in blue in the adjusted seasonal comparison (right column). All y-axes are displayed on a log scale except for creatinine (A) which is displayed on the square-root scale; confidence intervals are displayed in gray.
In contrast to creatinine, Fongoli and Taï chimpanzees differed either significantly in the magnitude of seasonal variation or in overall levels of their c-peptide values (model 2.2; full-null model comparison: χ2 = 39.486, df = 3, p < 0.001). More specifically, when testing the interaction between site and season (after adjustment of seasonal timing), we found that this difference was borne of larger intra-annual c-peptide variation in Taï samples but lower average levels than Fongoli samples (χ2 = 10.357, df = 2, p = 0.006; Figure 3B, Table 1B).
Lastly, we found that Fongoli and Taï significantly differed either overall or in the variation of their cortisol values (model 2.3; full-null model comparison: χ2 = 15.107, df = 3, p = 0.010). More specifically, the magnitude of seasonal variation tended to differ between the two sites (χ2 = 4.911, df = 2, p = 0.086; Figure 3C, Table 1C), with higher seasonal variation and averages in Fongoli cortisol values than in Taï chimpanzees.
We next tested the independent effects of creatinine and c-peptide on cortisol variation at the two sites, while still controlling for seasonal variation at each site. We found that the contribution of these biomarkers to cortisol variation significantly differed between Fongoli and Taï chimpanzees (model 3.1, Figure 4, Table 2; χ2 = 8.268, df = 2, p = 0.016). This difference was solely explained by the difference in effect of creatinine values on cortisol; Taï chimpanzees not only showed a significant effect of creatinine on cortisol values, but a significantly stronger effect of creatinine upon cortisol than was observed at Fongoli (χ2 = 7.231, df = 1, p = 0.007). The two sites did not significantly differ in their lack of an obvious effect of c-peptide on cortisol (χ2 = 2.041, df = 1, p = 0.153) as this relationship was effectively weak at both sites.
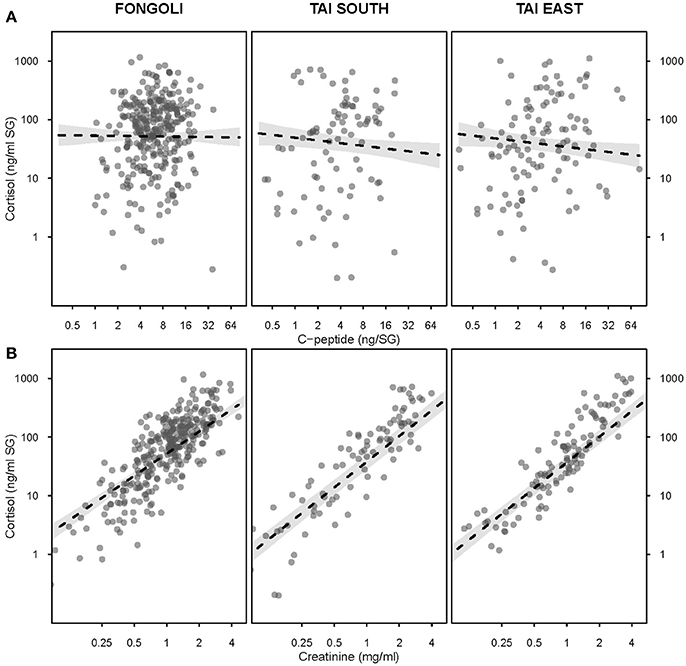
Figure 4. Effects of urinary c-peptide (A) and creatinine levels (B) on urinary cortisol concentration in three chimpanzee communities. All axes are displayed on a logarithmic scale; 95% confidence intervals are displayed in gray.
Environmental Drivers of Physiological Variation (Models 3.1 Through 5.4)
The effect of four environmental predictors on creatinine values clearly differed between sites (model 4.1; χ2 = 20.472, df = 5, p = 0.001). More specifically, both rainfall and monthly rainfall showed a positive effect on creatinine levels at Fongoli and a negative effect at Taï (test of the interactions; rainfall: χ2 = 6.255, df = 1, p = 0.0124; monthly rainfall: χ2 = 9.658, df = 1, p = 0.0019; Table 3A, Figure 5). Likewise, factor two (temperature) tended to interact with “site” (χ2 = 3.347, df = 1, p = 0.0673), whereby higher temperatures at Taï predicted higher creatinine values, while this relationship was weakly negative at Fongoli. Factor one unanimously had a negative impact on creatinine levels, and periods of higher humidity correlated with lower creatinine levels at both sites.
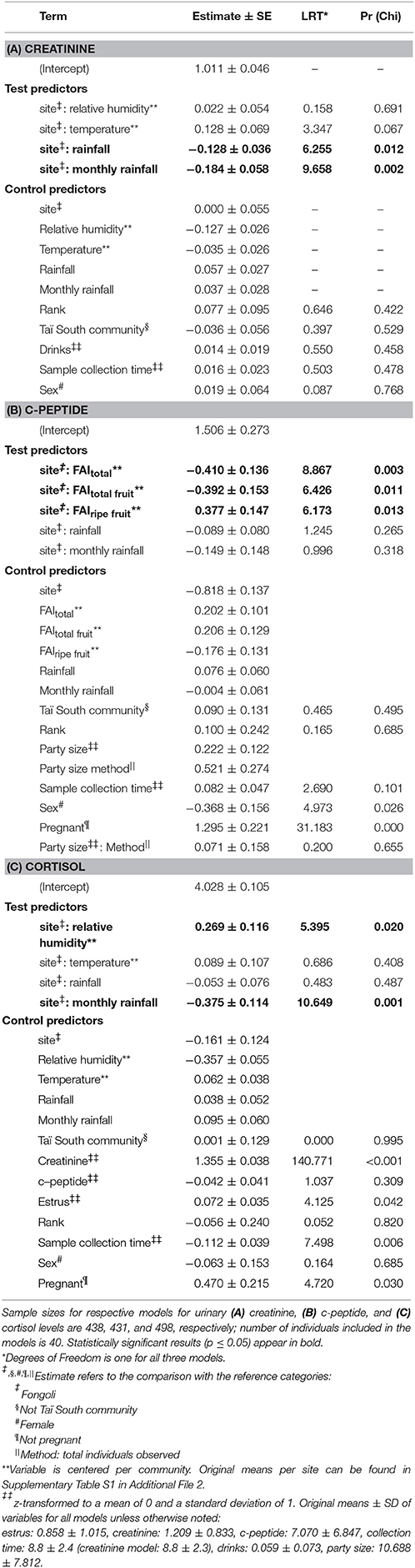
Table 3. Model results for inter-site differences of environmental variables on urinary biomarkers at Fongoli and Taï.
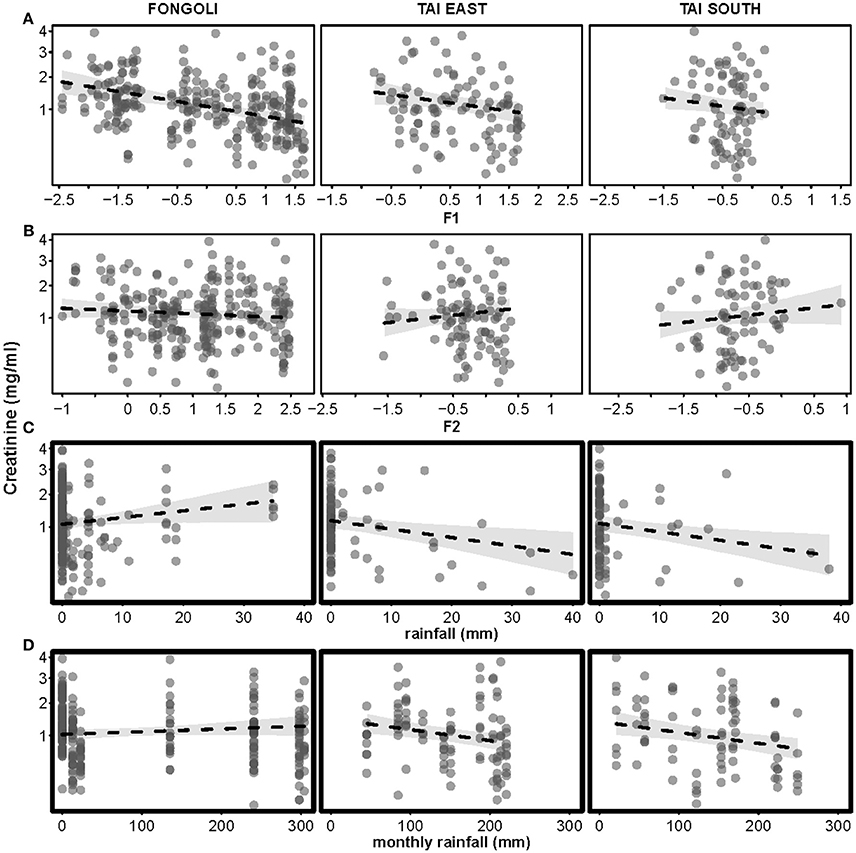
Figure 5. Effects of four environmental variables on urinary creatinine levels in three chimpanzee communities. Environmental variables displayed are: Factor 1 (relative humidity, centered to site; (A), Factor 2 (temperature, centered to site; (B), rainfall (C), and monthly rainfall (D). Creatinine levels appear on a square-root scale. Statistically significant inter-site differences (p ≤ 0.05) appear with bold margins; 95% confidence intervals are displayed in gray.
The impact of three food availability indices as well as rainfall the day prior and monthly rainfall differed between the two sites (model 4.2; full-null model comparison: χ2 = 66.929, df = 6, p < 0.001). Specifically, all three food availability indices differed significantly between the two sites, where higher total food availability and total fruit availability predicted higher c-peptide levels at Fongoli but lower c-peptide levels at Taï. This effect was reversed for ripe fruit availability (Table 3B, Figure 6). Post-hoc analyses indicated that total food availability predicted higher c-peptide values in Fongoli, whereas c-peptide levels at Taï were lower during periods of high total food availability (Supplementary Table S5). Additionally, higher ripe fruit availability significantly predicted higher c-peptide values for Taï chimpanzees.
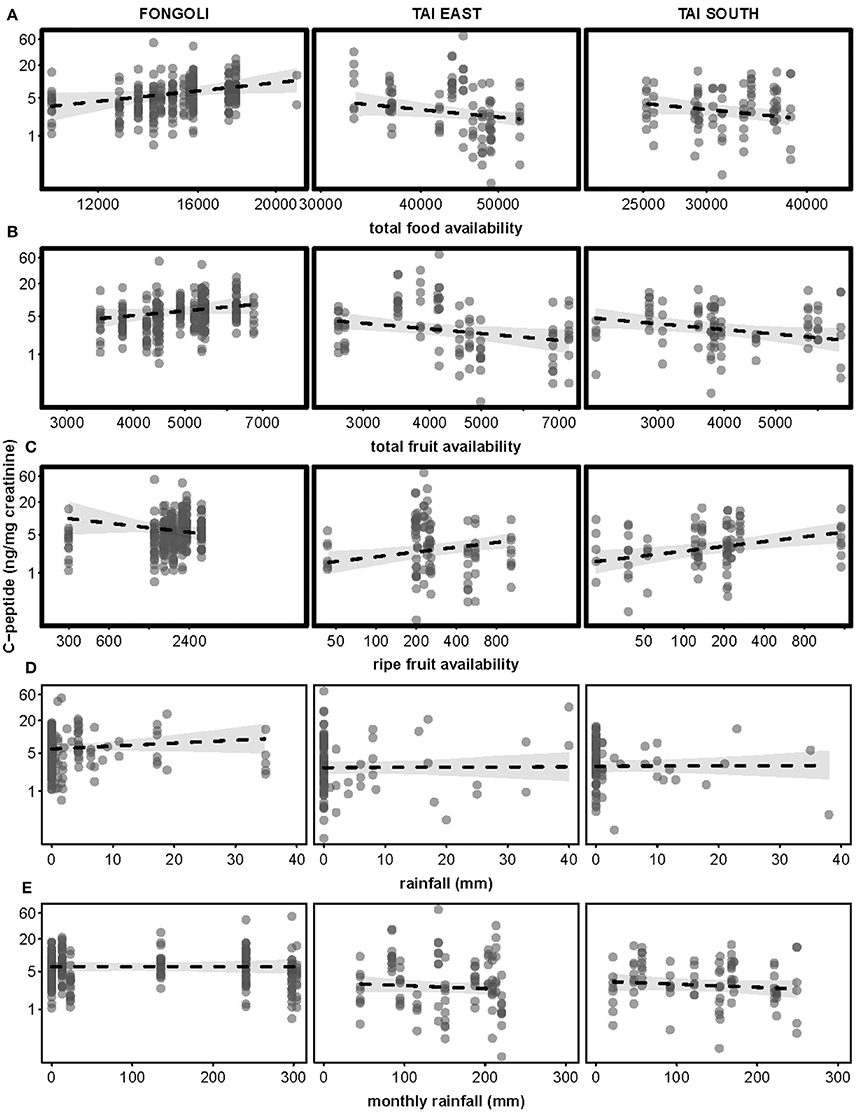
Figure 6. Effects of five environmental variables on urinary c-peptide levels in three chimpanzee communities. Environmental variables displayed are: total food availability (A, centered to community), total fruit availability (B, centered to community), ripe fruit availability (C, centered to community), rainfall (D), and monthly rainfall (E). C-peptide levels are displayed on a logarithmic scale. Statistically significant inter-site differences (p ≤ 0.05) appear with bold margins; 95% confidence intervals are displayed in gray.
When replacing the generic seasonal term with the four environmental variables (as in creatinine model), we found that Fongoli and Taï chimpanzees significantly differed in the contribution of these variables to cortisol variation (model 4.3; full-null model comparison: χ2 = 17.820, df = 5, p = 0.003). For both sites, increases in relative humidity coincided with decreases in cortisol variation, although this pattern was significantly stronger in Fongoli (Table 3C, Figure 7). Additionally, the amount of monthly rainfall had a positive effect on cortisol values at Fongoli, but a negative one at Taï. Post-hoc analyses indicated that only monthly rainfall significantly impacted cortisol levels at both sites, whereas relative humidity was also highly significant in its impact on Fongoli cortisol values but not in Taï. Furthermore, cortisol levels at Taï tended to increase with temperature (Supplementary Table S4).
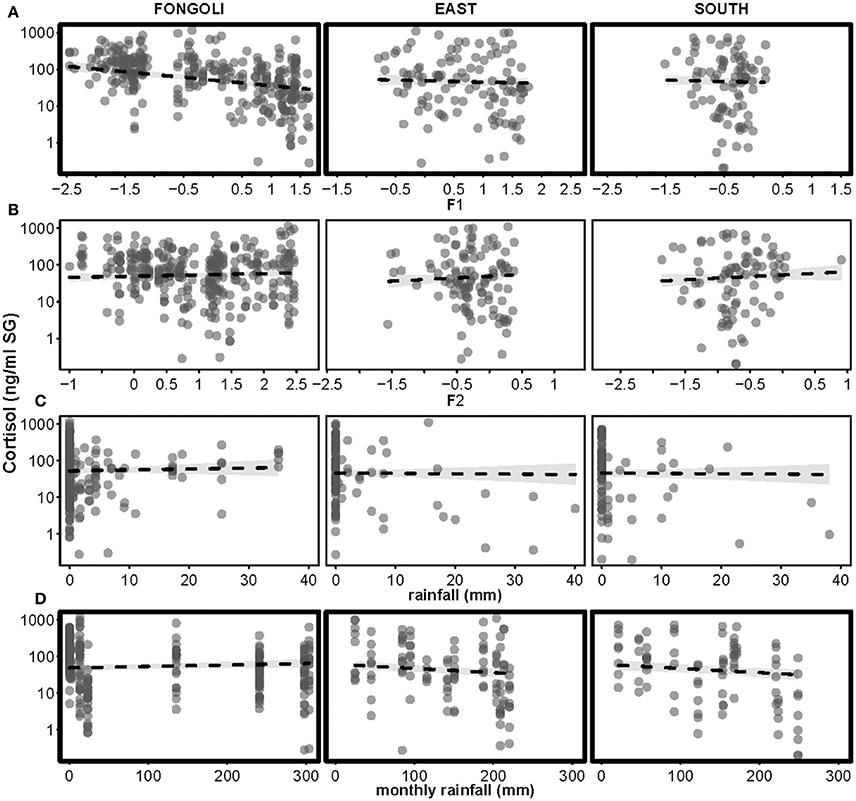
Figure 7. Effects of four environmental variables on urinary cortisol levels in three chimpanzee communities. Environmental variables displayed are: Factor 1 (relative humidity, centered to site; A), Factor 2 (temperature, centered to site; B), rainfall (C), and monthly rainfall (D). Cortisol levels appear on a logarithmic scale. Statistically significant inter-site differences (p ≤ 0.05) appear with bold margins; 95% confidence intervals are displayed in gray.
Discussion
Our results support the assertion that Fongoli as a savanna-mosaic habitat is more extreme and seasonal in its climate and ecology than a closed-canopy site independently of its effect upon chimpanzees. As for how this extreme and seasonal environment impacted chimpanzees, our results both supported and refuted our expectations. Fongoli chimpanzees appeared more seasonably stable in their c-peptide values than Taï chimpanzees, but showed more extreme variation in their cortisol values. Chimpanzees at both sites struggled equally with issues of dehydration, as indicated by seasonal creatinine variation and its impact upon cortisol values. As a result, categorizing savanna habitats as more challenging to chimpanzees than more heavily forested habitats, in the sense that they are singularly more physiologically costly and represent a niche to which they are not adapted, does not appear to be necessarily warranted as these habitats appear to exert constraints upon chimpanzees along different niche axes (Holt, 2009). Additionally, in other niche axes, savanna chimpanzees appear to endure similar stresses as those of chimpanzees inhabiting environments traditionally considered more species-typical.
Environmental Variation
We found Fongoli to be significantly more extreme in both absolute levels as well as in the seasonal variation of all climatic markers. These results support previous comparisons among long term chimpanzee research sites that have concluded that savanna-woodland or open habitat environments are generally more extreme, with Senegalese habitats considered particularly exceptional in their extremity (McGrew et al., 1981). Although Taï showed intra-annual variation in all markers, this variation was considerably more moderate (see Supplementary Figure S1) than Fongoli, suggesting that Fongoli and Taï are suitable comparative partners for investigations regarding chimpanzee physiological reactions to extreme versus moderate habitats.
Comparison of Seasonal Variation and Drivers of Physiological Change
C-peptide
Possibly as a consequence of greater seasonality in food availability metrics, specifically overall food availability and total fruit availability in Taï, Taï chimpanzees appeared to be less energetically stable than Fongoli chimpanzees. Specifically, we found that Taï chimpanzees' c-peptide levels varied more strongly and were on average lower than Fongoli c-peptide levels, and that this variation was more heavily driven by fruit availability (specifically ripe fruit availability). These results contrast with the assumptions that savanna habitats offer fewer nutritional or energetic possibilities to the primates that inhabit them. Therefore, limitations stemming from lower arboreal density and diversity have been proposed to be restrictive to savanna chimpanzees, potentially necessitating alternative behavioral adaptations to accommodate reduction of opportunities (Kortlandt, 1983), such as tool-assisted hunting (Pruetz and Bertolani, 2007; Pruetz et al., 2015), party size variation (e.g., Wrangham, 1977; Wrangham et al., 1992; Chapman et al., 1995; Boesch, 1996; Matsumoto-Oda et al., 1998; Mitani et al., 2002; Hashimoto et al., 2003), and incorporation of fallback foods (Wrangham et al., 1991; Furuichi et al., 2001; Hernandez-Aguilar et al., 2007), including crop-raiding (Hockings et al., 2009).
However, although Fongoli does have lower tree density in comparison to Taï, and the chimpanzees a reduced dietary breadth (Pruetz, 2006), we did not find that our c-peptide results supported the assumption that such limitations pose significant ecological challenges to Fongoli chimpanzees. While Fongoli chimpanzees certainly exhibit some degree of seasonal variation in c-peptide levels corresponding to expected periods of food scarcity (Wessling et al., 2018), this variation paled in comparison to the variation observed at Taï. To add to this, chimpanzees at neither site showed a significant influence of c-peptide levels upon cortisol excretion, as would have been expected if periods of scarcity and reduced energetic status were physiologically challenging. Therefore, savanna chimpanzees must employ behavioral, social, or ecological strategies to maximize resource utilization and acquisition in energetic maintenance needs to offset the apparent paucity in tree abundance and diversity offered by this habitat. Alternatively, it is possible that Fongoli chimpanzees exploit higher quality food resources than what has been observed in the Taï diet, although direct inter-site comparison of food quality has not yet been conducted. Accordingly, we are left to question the reliability of the assumption that savanna habitats represent an ecological limit of food availability tolerance for chimpanzees, and likewise the underlying implications of tree diversity and density in forming hypotheses related to energetic maintenance.
It therefore appears that Fongoli chimpanzees are able to cope with reduced food tree density and more seasonally extreme variation in preferred ripe fruit food items. Coping mechanisms range from dietary adjustment according to availability, depending on fallback or staple food items (Bogart and Pruetz, 2011), to employing behavioral strategies like varying foraging party size and utilizing an exceptionally large home range in a seasonal pattern (Baldwin et al., 1982; Pruetz and Bertolani, 2009). In this sense, the differences in the drivers of c-peptide variation between the sites appear to support observational evidence. Fongoli chimpanzees are known to consume higher amounts of non-fruit items (i.e., flowers, new leaves, and even bark; Pruetz, 2006) in comparison to other sites when preferred food items are scarcer (Pruetz, 2006; Lindshield, 2014) whereas Taï chimpanzees show a more typical chimpanzee dietary profile with a heavy reliance on ripe fruit and nuts (Boesch and Boesch-Achermann, 2000; Goné Bi, 2007). This view was supported by our c-peptide comparisons: while Taï chimpanzee c-peptide levels were largely explained by the availability of fruit (both ripe and total), Fongoli c-peptide levels were best explained by overall food availability. It has already been suggested that Fongoli chimpanzees rely on staple food items like termites as a dietary supplement (Bogart and Pruetz, 2011), and it appears that dietary flexibility in the face of seasonal scarcity of fruit items affords them higher energetic stability. In comparison, the Taï chimpanzees are more dependent on seasonal availability of ripe fruits and nuts (Boesch and Boesch-Achermann, 2000). It is thus probable that dietary flexibility in the face of seasonal variation in fruit abundance serves as an adequate adaptation to a relatively scarcer environment and seasonally available foods for the Fongoli chimpanzees. Similar patterns have also been suggested to be an adaptation employed by savanna-dwelling baboons who face similar conditions, in that they “forage widely in large, undefended home ranges and employ a handoff strategy to utilize a broad and constantly changing set of foods” (Alberts et al., 2005).
However, as several variables contribute to energetic status in wild organisms, we must also offer concessions regarding these conclusions. First, we were unable to account for potential seasonal differences in energetic expenditure in our models. Therefore, it is possible that these three communities may not only differ in their energetic consumption but also that energetic expenditure effects inter-site differences in c-peptide levels. Taï chimpanzees regularly patrol territorial boundaries (Samuni et al., 2017) while Fongoli chimpanzees do not; an activity that has been shown to have substantial energetic consequences (Amsler, 2010). Additionally, Taï chimpanzees are well known for their nut-cracking behavior, a seasonally fruiting and calorie-rich food item (N'guessan et al., 2009), and have likewise shown seasonality in hunting behavior (Boesch and Boesch-Achermann, 2000). It is possible that the influx of exceptional amounts of calories during nut-cracking or hunting seasons elicits exceptional seasonal variation in c-peptide values, which is supported to some degree by the simultaneity of the seasonal peak of the c-peptide model in both Taï communities with the nut-cracking season. However, exceptionally high c-peptide levels are not immediately discernable during this season in our dataset, and therefore exceptional caloric intake from nut consumption is unlikely to be the cause of higher intra-annual c-peptide variation at Taï. Further investigations of potential site differences in c-peptide levels that account for potential variation in energetic expenditure as well as consumption would be particularly illuminating.
Creatinine
Contrary to c-peptide, Fongoli and Taï chimpanzees did not differ in the degree of seasonal variation of creatinine values, and at both sites creatinine showed a strong positive relationship with cortisol variation. To add to this, this relationship was significantly stronger for Taï than Fongoli chimpanzees, suggesting that not only do Taï chimps also show signs of hydration constraints, but that they do to a greater degree than chimpanzees occupying a much hotter and drier environment. While puzzling, these results may be indicative that either the climate at Taï is sufficiently dry to elicit dehydration challenges, that preformed water in their diet is an insufficient contributor to their hydration status, and/or that the behavioral strategies adopted by the Fongoli chimpanzees afford them partial evasion of potential stress otherwise expected by an even harsher environment.
Although among the wettest of all chimpanzee long term research sites, Taï chimpanzees exhibited virtually identical hydration challenges during the late dry season as Fongoli chimpanzees, indicating that annual rainfall as a measure of habitat wetness is likely not a reliable indicator of the potential dehydration challenges an individual may face in a particular environment. Although dryer sites like Fongoli, Mt. Assirik, and Ugalla are expected to be the most challenging for maintaining hydration, a site's “dryness” is not a dependable indicator of potential dehydration stress, as “wet” sites like Taï may also impose dehydration challenges. Rather, McGrew et al. (1981) suggested that the amount of rainfall in a location should not be the only relevant indicator of dryness considered, but that also the distribution of rainfall over the year has important consequences. Like Fongoli, Taï has distinct wet and dry seasons; although the major dry season at Taï may only last 3 months (December–February), it appears that this dryer period is sufficiently dry to elicit dehydration challenges. Additionally, energetically rich but exceptionally dry nuts are in abundance during this time (Boesch and Boesch-Achermann, 2000), which may lead to drier diet during water scarce periods, thereby reducing the potential contribution of preformed water in ripe fruits to an individual's hydration status. Therefore, although overall annual rainfall may be an important habitat characteristic considered when evaluating the chimpanzee niche, on a day-to-day basis it may rather be the temporal distribution of rainfall that is of paramount relevance for delimiting chimpanzee habitat suitability with regards to daily hydration needs.
Additionally, marked decreases in rainfall have been observed both regionally and locally at Taï over decades of observation (Paturel et al., 1995; Servat et al., 1997; Boesch and Boesch-Achermann, 2000; Kühl et al., 2012), and chimpanzees there have exhibited observable demographic responses to such changes (Kühl et al., 2012). Given that these changes are rather recent relative to the expected timescale of evolutionary adaptation, and likely started in the 1980's based on data records from the site (Paturel et al., 1995; Servat et al., 1997), it may be that Taï chimpanzees have not had sufficient time to develop adequate behavioral repertoires to counter new seasonal dehydration challenges due to climatic shifts. Alternatively, Fongoli chimpanzees, which are expected to be well-adapted to their hot and dry habitat, could be expected to be more able to compensate behaviorally for habitat-typical challenges imposed by this environment, thereby potentially dampening the relationship between creatinine and cortisol levels. Such behavioral adaptations include strategies to maintain access and proximity to water sources during periods of highest necessity (Lindshield et al., 2017). Furthermore, Fongoli chimpanzees are observed to drink more often during seasonal periods of water scarcity (Wessling, unpublished data). That we found a negative relationship between creatinine values and both rainfall the day prior and monthly rainfall potentially suggests that in contrast to Fongoli, Taï chimpanzees do not employ this strategy but simply drink whenever water sources are encountered. Therefore, in the absence of a strategy specifically adapted to counter dehydration, Taï chimpanzees may suffer from dehydration when available water is infrequently encountered, perhaps explaining the existence of seasonally stressful periods of dehydration at a wet site like Taï.
At its core, our results suggest that chimpanzee habitats do not need to be relatively “dry” to pose a risk of dehydration to chimpanzees. Even in a moderately cooler and more stable site like Taï, chimpanzees experience dehydration, and therefore possible that dehydration challenges are experienced by chimpanzees throughout their range, at least in areas where rainfall is seasonal. Further investigations of creatinine and cortisol variation due to environmental changes across multiple chimpanzee localities will help elucidate this relationship. In our recent paper (Wessling et al., 2018), we posited that it is possible that Fongoli chimpanzees incorporate water-content properties of food into their dietary decision-making. If Taï chimpanzees also experience dehydration risks and constraints, then our results underline the importance of considering the contribution of dehydration risks to chimpanzee behavior and food selection, and the strategies employed to counter these constraints.
Cortisol
Ultimately, we found that cortisol levels of Fongoli chimpanzees tended to vary in a more extreme seasonal fashion than cortisol levels from Taï, indicating stronger seasonal constraints upon Fongoli chimpanzees. These results follow our prediction that the extreme habitat of Fongoli coupled with exceptional seasonality in climatic and ecological factors elicits stronger seasonal stress, specifically during the late dry season. Such conclusions are concordant with other range margin literature that has found that range margins are typically defined by abiotic or climate factors (see Sexton et al., 2009 for review).
Surprisingly, we found that differences in temperature variation appeared to have little effect on cortisol variation, but rather that relative humidity impacted chimpanzee cortisol at both sites. However, this effect was stronger in Fongoli than in Taï chimpanzees. Cortisol peaks toward the end of the dry season when both water is scarce (low humidity), temperatures are high, and humidity is low. It is likely that relative humidity more effectively captures the extremity of the end of the dry season than temperature, which remains high throughout the dry season. Relative humidity's inverse relationship with conditions of the dry season indicates that these conditions are significant, and further underlines the importance of adequate hydration levels for chimpanzees during the hottest period of the year.
Conclusions
In concert, these results supported our hypothesis that Fongoli, a site generally considered marginal, poses significantly higher seasonal costs to chimpanzees than less seasonal habitats. However, the extent of ecological differences in chimpanzee habitats, such as rainfall or food availability, yet a lack of difference in physiological response between these habitats suggests that behavioral flexibility is an important contribution to widening chimpanzee ecological tolerance, and perhaps even the chimpanzee niche. Such range expansion or ecological tolerance due to behavioral flexibility is a widely observed phenomenon found in many other taxa (e.g., Suarez et al., 1999; Yeh and Price, 2004; Sol et al., 2005; Duckworth and Badyaev, 2007; Liebl and Martin, 2014; Varner and Dearing, 2014; Varner et al., 2016).
Here we have provided a first attempt at examining in situ effects of ecological and range marginality in a long-lived, large-bodied mammal. Our results have important implications for our understanding of biogeography, ecological tolerance, and more specifically, the limits of both for extant chimpanzees and extinct hominins. Although chimpanzees at both sites appeared to exhibit significant seasonal effects of dehydration, the more extreme environmental variation and general conditions at Fongoli prompted higher physiological seasonal costs (in the form of cortisol). Therefore, it appears that extreme savanna habitats serve as a thermoregulatory limit to the chimpanzee ecological niche in the hot and dry conditions of the dry season, and strain an individual's ability to maintain homeostasis during parts of the year. Such results support the use of savanna chimpanzees as models for understanding adaptation to marginal environments, such as that which has been proposed during the expansion of hominins into savanna habitat from forested habitat (Reed, 1997; Bobe and Behrensmeyer, 2004; White et al., 2009; Copeland et al., 2011), and therefore likewise the significance of savannas within the hominin ecological niche (Quinn et al., 2013). These results further underline the uniqueness of thermoregulatory stress to individuals in savanna environments, thereby supporting established theory that thermoregulation was a novel and necessary evolutionary hurdle to be overcome in the expansion of hominins into similar environments (Wheeler, 1984, 1991), and supporting their use as models for understanding the mechanisms involved in early hominin adaptations to thermoregulation.
Nonetheless and contrary to expectation, Taï chimpanzees also appeared to face dehydration pressure, therefore refuting the assumption that savanna habitats are the only habitats in which water is a limiting factor (McGrew et al., 1981), and highlighting that certain challenges may be more ubiquitous than previously assumed. If these constraints are not unique to Fongoli, this begs the question as to whether or not savanna-mosaic habitats can be appropriately labeled as marginal habitats based on this criterion alone. Additionally, the lack of stronger seasonal effects in c-peptide levels at Fongoli in comparison with Taï suggests that this site does not necessarily reflect energetic or nutritional limits to chimpanzee ecological tolerance, as Kortlandt (1983) has suggested. Rather, our c-peptide results may even suggest that savanna-mosaic habitats represent favorable conditions with regard to maintaining adequate energetic status in contrast to lusher habitats like Taï.
Although stress response alone does not indicate long-term effects on an individual nor reduced habitat suitability, and fitness measures would better serve to address questions of marginality of chimpanzee habitat, we provide the first steps needed to approach such questions. For now, assumptions that the chimpanzee is strictly forest adapted (Boesch, 2009) may be premature and warrant caution. We therefore offer a new definition of ecological marginality for chimpanzees in which particular conditions may be considered marginal if they represent the limit of the chimpanzee ecological niche across multiple niche axes, and that such limitations have an observed effect upon measures of chimpanzee fitness. In this sense, savanna chimpanzees might appear marginal, however only along certain niche axes, whereas forest chimpanzees may be considered marginal along others. What is clear is that chimpanzees are behaviorally plastic enough to adapt to a range of environments and challenges, yet further investigation should be conducted to understand what the long-term consequences of these adaptations may mean in an evolutionary sense. Therefore, further investigation of seasonal effects of these or other biomarkers in a larger number of chimpanzee research sites would contribute significantly to discussions of ecological tolerance and what constitutes marginal habitats to chimpanzees, as we have presented only two examples of contrasting ecological conditions.
At a minimum, it appears therefore that savanna habitats still lie within the chimpanzee ecological niche. This should encourage researchers to view chimpanzee behavioral syndromes in these environments as generalizable to the species and within the range of species-typical behavior, and not as only originating in extraordinary circumstances. Therefore, given the range of habitats chimpanzees have been observed to occupy, the consideration of certain habitats to be ideal chimpanzee habitat should be revisited, or avoided entirely. Further comparisons across the chimpanzee ecological continuum therefore will allow us to understand the range of natural adaptation to their environment, especially with regard to the species' ability to adapt to an outstanding range of habitats. Such an initiative may be imperative in the face of climate change, habitat loss, and other threats to chimpanzee populations as a proper understanding of what constitutes suitable habitat for chimpanzees is a necessary prerequisite for adequate conservation effort and planning. Unquestionably, the categorization of savanna habitats as marginal or not impacts how these habitats are regarded for their conservation value to the species as a whole, thereby further underlining the complexity and importance of employing caution when evaluating chimpanzee habitat suitability across a range of habitats.
Additionally, these results highlight potential sensitivities of chimpanzee populations in savanna areas, for example seasonal periods of higher stress, such as the dry season. Erstwhile these results highlight sensitivities like water availability across habitats for chimpanzees that had previously been thought to be savanna-specific. As such, the consequences of threats like climate change may be far more severe than previously thought. Therefore, the effects of climate change will need to be taken into account when performing conservation evaluations like population viability analyses, as climate change may possibly amplify stochastic effects in vulnerable populations. Until now, all current attempts to model chimpanzee distribution or habitat suitability have been based upon static statistical models (with the exception of Lehmann et al., 2007). Here, however, we offer insights for building more mechanistic models of chimpanzee distribution, which would assist in both assessment of future change scenarios (such as climate and land use change), but also in the evaluation of potential changes that have occurred previously (such as Plio-Pleistocene climate and habitat shifts) in a more realistic way than that which can be offered by static models. To conclude, we must first understand what the chimpanzee niche is before we can understand how its change in the face of anthropogenic and climatic change will affect chimpanzee populations.
Author Contributions
EW, TD, and HK: conceived and designed the study; EW: performed the research; EW and RM: analyzed the data; TD, RW, and JP: provided material; EW, TD, RM, RW, JP, and HK: drafted the manuscript. All authors approved the submission of this manuscript.
Funding
This research was funded by the Max Planck Society, the National Geographic Society, and Iowa State University.
Conflict of Interest Statement
The authors declare that the research was conducted in the absence of any commercial or financial relationships that could be construed as a potential conflict of interest.
The reviewer AP declared a past collaboration with one of the authors, EGW, to the handling Editor.
Acknowledgments
We would like to thank the République du Sénégal and the Direction des Eaux et Forêts et Chasses, as well as the Ivoirian Ministère de la Recherches Scientifiques, the Ministère de l'Environnement et des Eaux et Forêts, the Office Ivoirien des Parcs et Réserves, the directorship of the Taï National Park, and the Centre Suisse de Recherche Scientifique for permission to conduct this research. At Fongoli we thank Jacques Tamba Keita, Michel Sadiakho, Dondo Kante, and Stacy Lindshield and at Taï we thank Florent Goulei, Ble Fabrice, Apollinaire Gnahe Djirian, and Oulai Yehanon Frederic for their support in the field. For support in the lab we thank Roísín Murtaugh, Vera Schmeling, Verena Behringer, and Juliane Damm for their significant contributions, and Gaëlle Bocksberger for support with data processing and analysis. Sincere thanks are due to Liran Samuni, Karline Janmaat, Anna Preis, Alex Mielke, and Sylvain Lemoine who all contributed significant aspects and understanding which made such analyses possible. We thank Liran Samuni, Alex Piel, and one reviewer for helpful comments on an earlier draft of this manuscript.
Supplementary Material
The Supplementary Material for this article can be found online at: https://www.frontiersin.org/articles/10.3389/fevo.2018.00060/full#supplementary-material
Abbreviations
FSCP, Fongoli Savanna Chimpanzee Project; TCP, Taï Chimpanzee Project; LMM, Linear Mixed Model; VIF, Variance Inflation Factor.
Footnotes
1. ^NOAA The Heat Index Equation. Available online at: http://www.wpc.ncep.noaa.gov/html/heatindex_equation.shtml (Accessed Oct 13, 2017).
References
Alberts, S. C., Hollister-Smith, J. A., Mututua, R. S., Sayialel, S. N., Muruthi, P. M., Warutere, J. K., et al. (2005). “Seasonality and long-term change in a savanna environment,” in Seasonality in Primates: Studies of Living and Extinct Human and Non-Human Primates, eds D. K. Brockman and C. P. van Schaik (Cambridge: Cambridge University Press), 157–196.
Altmann, J. (1974). Observational study of behavior: sampling methods. Behaviour 49, 227–266. doi: 10.1163/156853974X00534
Amsler, S. J. (2010). Energetic costs of territorial boundary patrols by wild chimpanzees. Am. J. Primatol. 72, 93–103. doi: 10.1002/ajp.20757
Anderson, D. P., Nordheim, E. V., and Boesch, C. (2006). Environmental factors influencing the seasonality of estrus in chimpanzees. Primates 47, 43–50. doi: 10.1007/s10329-005-0143-y
Anderson, D. P., Nordheim, E. V., Boesch, C., and Moermond, T. (2002). “Factors influencing fission-fusion grouping in chimpanzees in the Taï National Park, Côte d'Ivoire,” in Behavioural Diversity in chimpanzees and Bonobos, eds C. Boesch, G. Hohmann and L. Marchant (Cambridge: Cambridge University Press), 90–101.
Anestis, S. F., Breakey, A. A., Beuerlein, M. M., and Bribiescas, R. G. (2009). Specific gravity as an alternative to creatinine for estimating urine concentration in captive and wild chimpanzee (Pan troglodytes) samples. Am. J. Primatol. 71, 130–135. doi: 10.1002/ajp.20631
Anestis, S. F., and Bribiescas, R. G. (2004). Rapid changes in chimpanzee (Pan troglodytes) urinary cortisol excretion. Horm. Behav. 45, 209–213. doi: 10.1016/j.yhbeh.2003.09.015
Arandjelovic, M., Head, J., Rabanal, L. I., Schubert, G., Mettke, E., Boesch, C., et al. (2011). Non-invasive genetic monitoring of wild central chimpanzees. PLoS ONE 6:e14761. doi: 10.1371/journal.pone.0014761
Baayen, R. H. (2008). Analyzing Linguistic Data: a Practical Introduction to Statistics Using R. Cambridge: Cambridge University Press.
Bahr, N. I., Palme, R., Möhle, U., Hodges, J. K., and Heistermann, M. (2000). Comparative aspects of the metabolism and excretion of cortisol in three individual nonhuman primates. Gen. Comp. Endocrinol. 117, 427–438. doi: 10.1006/gcen.1999.7431
Baldwin, P. J., McGrew, W. C., and Tutin, C. E. (1982). Wide-ranging chimpanzees at Mt. Assirik, Senegal. Int. J. Primatol. 3, 367–385. doi: 10.1007/BF02693739
Barr, D. J., Levy, R., Scheepers, C., and Tily, H. J. (2013). Random effects structure for confirmatory hypothesis testing: keep it maximal. J. Mem. Lang. 68, 255–278. doi: 10.1016/j.jml.2012.11.001
Bates, B., Maechler, M., Bolker, B., and Walker, S. (2015). Fitting linear mixed-effects models using lme4. J. Stat. Softw. 67, 1–48. doi: 10.18637/jss.v067.i01
Beehner, J. C., and Bergman, T. J. (2017). The next step for stress research in primates: to identify relationships between glucocorticoid secretion and fitness. Horm. Behav. 91, 68–83. doi: 10.1016/j.yhbeh.2017.03.003
Bertolani, P., and Pruetz, J. D. (2011). Seed reingestion in Savannah Chimpanzees (Pan troglodytes verus) at Fongoli, Senegal. Int. J. Primatol. 32, 1123–1132. doi: 10.1007/s10764-011-9528-5
Bobe, R., and Behrensmeyer, A. K. (2004). The expansion of grassland ecosystems in Africa in relation to mammalian evolution and the origin of the genus Homo. Palaeogeogr. Palaeoclimatol. Palaeoecol. 207, 399–420. doi: 10.1016/j.palaeo.2003.09.033
Boesch, C. (1996). “Social grouping in Tai chimpanzees,” in Great Ape Societies, eds W. McGrew, L. Marchant, and T. Nishida (Cambridge: Cambridge University Press), 101–113.
Boesch, C. (2009). The Real Chimpanzee: Sex Strategies in the Forest. Cambridge: Cambridge University Press.
Boesch, C., and Boesch-Achermann, H. (2000). The Chimpanzees of the Taï Forest: Behavioural Ecology and Evolution. Oxford: Oxford University Press.
Bogart, S. L., and Pruetz, J. D. (2011). Insectivory of savanna chim panzees (Pan troglodytes verus) at Fongoli, Senegal. Am. J. Phys. Anthropol. 145, 11–20. doi: 10.1002/ajpa.21452
Braunisch, V., Bollmann, K., Graf, R. F., and Hirzel, A. H. (2008). Living on the edge—modelling habitat suitability for species at the edge of their fundamental niche. Ecol. Model. 214, 153–167. doi: 10.1016/j.ecolmodel.2008.02.001
Chapman, C. A., Chapman, L. J., and Wrangham, R. (1995). Ecological constraints on group size: an analysis of spider monkey and chimpanzee subgroups. Behav. Ecol. Sociobiol. 36, 59–70. doi: 10.1007/BF00175729
Chilvers, B., Wilkinson, I., Duignan, P., and Gemmell, N. (2006). Diving to extremes: are New Zealand sea lions (Phocarctos hookeri) pushing their limits in a marginal habitat? J. Zool. 269, 233–240. doi: 10.1111/j.1469-7998.2006.00059.x
Christison, G., and Johnson, H. (1972). Cortisol turnover in heat-stressed cows. J. Anim. Sci. 35, 1005–1010. doi: 10.2527/jas1972.3551005x
Copeland, S. R., Sponheimer, M., de Ruiter, D. J., Lee-Thorp, J. A., Codron, D., le Roux, P. J., et al. (2011). Strontium isotope evidence for landscape use by early hominins. Nature 474, 76–78. doi: 10.1038/nature10149
Crowther, T. W., Glick, H., Covey, K., Bettigole, C., Maynard, D., Thomas, S., et al. (2015). Mapping tree density at a global scale. Nature 525, 201–205. doi: 10.1038/nature14967
Després-Einspenner, M. L., Howe, E. J., Drapeau, P., and Kühl, H. S. (2017). An empirical evaluation of camera trapping and spatially explicit capture-recapture models for estimating chimpanzee density. Am. J. Primatol. 79:e22647. doi: 10.1002/ajp.22647
Dobson, A. J. (2002). An Introduction to Generalized Linear Models. Boca Raton, FL: Chapman & Hall/CRC.
Duckworth, R. A., and Badyaev, A. V. (2007). Coupling of dispersal and aggression facilitates the rapid range expansion of a passerine bird. Proc. Nat. Acad. Sci.U.S.A. 104, 15017–15022. doi: 10.1073/pnas.0706174104
Edens, F. W., and Siegel, H. S. (1975). Adrenal responses in high and low ACTH response lines of chickens during acute heat stress. Gen. Comp. Endocrinol. 25, 64–73. doi: 10.1016/0016-6480(75)90040-4
El-Halawani, M., Waibel, P., and Good, A. (1973). Effects of temperature stress on catecholamines and corticosterone of male turkeys. Am. J. Physiol. 224, 384–388. doi: 10.1152/ajplegacy.1973.224.2.384
Emery Thompson, M., and Knott, C. D. (2008). Urinary C-peptide of insulin as a non-invasive marker of energy balance in wild orangutans. Horm. Behav. 53, 526–535. doi: 10.1016/j.yhbeh.2007.12.005
Emery Thompson, M., Muller, M. N., Kahlenberg, S. M., and Wrangham, R. W. (2010). Dynamics of social and energetic stress in wild female chimpanzees. Horm. Behav. 58, 440–449. doi: 10.1016/j.yhbeh.2010.05.009
Emery Thompson, M., Muller, M. N., and Wrangham, R. W. (2012). Technical note: variation in muscle mass in wild chimpanzees: application of a modified urinary creatinine method. Am. J. Phys. Anthropol. 149, 622–627. doi: 10.1002/ajpa.22157
Emery Thompson, M., Muller, M. N., Wrangham, R. W., Lwanga, J. S., and Potts, K. B. (2009). Urinary C-peptide tracks seasonal and individual variation in energy balance in wild chimpanzees. Horm. Behav. 55, 299–305. doi: 10.1016/j.yhbeh.2008.11.005
Emery Thompson, M., Muller, M. N., Sabbi, K., Machanda, Z. P., Otali, E., and Wrangham, R. W. (2016). Faster reproductive rates trade off against offspring growth in wild chimpanzees. Proc. Natl. Acad. Sci. U.S.A. 113, 7780–7785. doi: 10.1073/pnas.1522168113
Fox, J., and Weisberg, S. (2011). An R Companion to Applied Regression, 2nd Edn. Thousand Oaks, CA: Sage.
Furuichi, T., Hashimoto, C., and Tashiro, Y. (2001). Fruit availability and habitat use by chimpanzees in the Kalinzu Forest, Uganda: examination of fallback foods. Int. J. Primatol. 22, 929–945. doi: 10.1023/A:1012009520350
Gammell, M. P., de Vries, H., Jennings, D. J., Carlin, C. O. M., and Hayden, T. J. (2003). David's score: a more appropriate dominance ranking method than Clutton-Brock et al.'s index. Anim. Behav. 66, 601–605. doi: 10.1006/anbe.2003.2226
Girard-Buttoz, C., Higham, J. P., Heistermann, M., Wedegärtner, S., Maestripieri, D., and Engelhardt, A. (2011). Urinary C-peptide measurement as a marker of nutritional status in macaques. PLoS ONE 6:e18042. doi: 10.1371/journal.pone.0018042
Goné Bi, Z. (2007). Régime Alimentaire des Chimpanzés, Distribution Spatiale et Phénologie des Plantes Dont les Fruits Sont Consommés Par les Chimpanzés du Parc National de Tai, en Côte d'Ivoire. Ph.D. Doctoral thesis, University of Cocody Abidjan.
Goodall, J. (1986). The Chimpanzees of Gombe: Patterns of Behavior. Cambridge, MA: The Belknap Press of Harvard University Press.
Grueter, C. C., Deschner, T., Behringer, V., Fawcett, K., and Robbins, M. M. (2014). Socioecological correlates of energy balance using urinary C-peptide measurements in wild female mountain gorillas. Physiol. Behav. 127, 13–19. doi: 10.1016/j.physbeh.2014.01.009
Harikai, N., Tomogane, K., Miyamoto, M., Shimada, K., Onodera, S., and Tashiro, S.-I. (2003). Dynamic responses to acute heat stress between 34 C and 38.5 C, and characteristics of heat stress response in mice. Biol. Pharm. Bull. 26, 701–708. doi: 10.1248/bpb.26.701
Hashimoto, C., Suzuki, S., Takenoshita, Y., Yamagiwa, J., Basabose, A. K., and Furuichi, T. (2003). How fruit abundance affects the chimpanzee party size: a comparison between four study sites. Primates 44, 77–81. doi: 10.1007/s10329-002-0026-4
Hauser, B., Deschner, T., and Boesch, C. (2008). Development of a liquid chromatography-tandem mass spectrometry method for the determination of 23 endogenous steroids in small quantities of primate urine. J. Chromatogr. B Analyt. Technol. Biomed. Life Sci. 862, 100–112. doi: 10.1016/j.jchromb.2007.11.009
Head, J. S., Boesch, C., Makaga, L., and Robbins, M. M. (2011). Sympatric Chimpanzees (Pan troglodytes troglodytes) and Gorillas (Gorilla gorilla gorilla) in Loango National Park, Gabon: dietary composition, seasonality, and intersite comparisons. Int. J. Primatol. 32, 755–775. doi: 10.1007/s10764-011-9499-6
Hernandez-Aguilar, R. A., Moore, J., and Pickering, T. R. (2007). Savanna chimpanzees use tools to harvest the underground storage organs of plants. Proc. Natl. Acad. Sci. U.S.A. 104, 19210–19213. doi: 10.1073/pnas.0707929104
Hockings, K. J., Anderson, J. R., and Matsuzawa, T. (2009). Use of wild and cultivated foods by chimpanzees at Bossou, Republic of Guinea: feeding dynamics in a human-influenced environment. Am. J. Primatol. 71, 636–646. doi: 10.1002/ajp.20698
Holt, R. D. (2009). Bringing the Hutchinsonian niche into the 21st century: ecological and evolutionary perspectives. Proc. Nat. Acad. Sci. U.S.A. 106(Suppl. 2), 19659–19665. doi: 10.1073/pnas.0905137106
Hunt, K. D., and McGrew, W. C. (2002). “Chimpanzees in the dry habitats of Assirik, Senegal and Semliki wildlife reserve, Uganda,” in Behavioural Diversity in Chimpanzees and Bonobos, eds C. Boesch, L. F. Marchant, and G. Hohmann (Cambridge: Cambridge University Press), 35–51.
Kawecki, T. J. (2008). Adaptation to marginal habitats. Annu. Rev. Ecol. Evol. 39, 321–342. doi: 10.1146/annurev.ecolsys.38.091206.095622
Kortlandt, A. (1983). Marginal habitats of chimpanzees. J. Hum. Evol. 12, 231–278. doi: 10.1016/S0047-2484(83)80149-3
Kouakou, C. Y., Boesch, C., and Kuehl, H. (2009). Estimating chimpanzee population size with nest counts: validating methods in Taï National Park. Am. J. Primatol. 71, 447–457. doi: 10.1002/ajp.20673
Kühl, H. S., Kalan, A. K., Arandjelovic, M., Aubert, F., D'Auvergne, L., Goedmakers, A., et al. (2016). Chimpanzee accumulative stone throwing. Sci. Rep. 6:22219. doi: 10.1038/srep22219
Kühl, H. S., N'Guessan, A., Riedel, J., Metzger, S., and Deschner, T. (2012). The effect of climate fluctuation on chimpanzee birth sex ratio. PLoS ONE 7:e35610. doi: 10.1371/journal.pone.0035610
Lehmann, J., Korstjens, A. H., and Dunbar, R. (2007). Fission–fusion social systems as a strategy for coping with ecological constraints: a primate case. Evol. Ecol. 21, 613–634. doi: 10.1007/s10682-006-9141-9
Liebl, A. L., and Martin, L. B. (2014). Living on the edge: range edge birds consume novel foods sooner than established ones. Behav. Ecol. 25, 1089–1096. doi: 10.1093/beheco/aru089
Lindshield, S., Danielson, B. J., Rothman, J. M., and Pruetz, J. D. (2017). Feeding in fear? How adult male western chimpanzees (Pan troglodytes verus) adjust to predation and Savanna habitat pressures. Am. J. Phys. Anthropol. 163, 1–17. doi: 10.1002/ajpa.23221
Lindshield, S. M. (2014). Multilevel Analysis of the Foraging Decisions of Western Chimpanzees (Pan troglodytes verus) and Resource Scarcity in a Savanna Environment at Fongoli, Senegal. Doctoral thesis, Iowa State University.
Luncz, L. V., and Boesch, C. (2015). The extent of cultural variation between adjacent chimpanzee (Pan troglodytes verus) communities; a microecological approach. Am. J. Phys. Anthropol. 156, 67–75. doi: 10.1002/ajpa.22628
Matsumoto-Oda, A., Hosaka, K., Huffman, M. A., and Kawanaka, K. (1998). Factors affecting party size in chimpanzees of the Mahale mountains. Int. J. Primatol. 19, 999–1011. doi: 10.1023/A:1020322203166
Matsuzawa, T., Humle, T., and Sugiyama, Y. (2011). The Chimpanzees of Bossou and Nimba. Tokyo: Springer Science & Business Media.
Matthews, A., and Matthews, A. (2004). Survey of gorillas (Gorilla gorilla gorilla) and chimpanzees (Pan troglodytes troglodytes) in Southwestern Cameroon. Primates 45, 15–24. doi: 10.1007/s10329-003-0058-4
McCabe, G. M., Fernández, D., and Ehardt, C. L. (2013). Ecology of reproduction in Sanje mangabeys (Cercocebus sanjei): dietary strategies and energetic condition during a high fruit period. Am. J. Primatol. 75, 1196–1208. doi: 10.1002/ajp.22182
McGrew, W., Baldwin, P., and Tutin, C. (1988). Diet of wild chimpanzees (Pan troglodytes verus) at Mt. Assirik, Senegal: I. Composition. Am. J. Primatol. 16, 213–226. doi: 10.1002/ajp.1350160304
McGrew, W. C., Baldwin, P. J., and Tutin, C. E. (1981). Chimpanzees in a hot, dry and open habitat: Mt. Assirik, Senegal, West Africa. J. Hum. Evol. 10, 227–244. doi: 10.1016/S0047-2484(81)80061-9
Mitani, J. C., and Rodman, P. S. (1979). Territoriality: the relation of ranging pattern and home range size to defendability, with an analysis of territoriality among primate species. Behav. Ecol. Sociobiol. 5, 241–251. doi: 10.1007/BF00293673
Mitani, J. C., Watts, D. P., and Lwanga, J. S. (2002). “Ecological and social correlates of chimpanzee party size and composition,” in Behavioural Diversity in Chimpanzees and Bonobos, eds C. Boesch, L. F. Marchant and G. Hohmann (Cambridge: Cambridge University Press), 102.
Moore, J. (1992). “Savanna” Chimpanzees,” in Topics in Primatology. Volume I: Human Origins, eds T. Nishida, W. C. McGrew, P. Marler, M. Pickford, and F. B. M. de Waal (Tokyo: University of Tokyo Press).
Muller, M. N., Kahlenberg, S. M., Thompson, M. E., and Wrangham, R. W. (2007). Male coercion and the costs of promiscuous mating for female chimpanzees. Proc. R. Soc. B 274, 1009–1014. doi: 10.1098/rspb.2006.0206
Muller, M. N., and Lipson, S. F. (2003). Diurnal patterns of urinary steroid excretion in wild chimpanzees. Am. J. Primatol. 60, 161–166. doi: 10.1002/ajp.10103
Muller, M. N., and Wrangham, R. W. (2004). Dominance, cortisol and stress in wild chimpanzees (Pan troglodytes schweinfurthii). Behav. Ecol. Sociobiol. 55, 332–340. doi: 10.1007/s00265-003-0713-1
N'guessan, A. K., Ortmann, S., and Boesch, C. (2009). Daily energy balance and protein gain among Pan troglodytes verus in the Taï National Park, Côte d'Ivoire. Int. J. Primatol. 30, 481–496. doi: 10.1007/s10764-009-9354-1
Paturel, J.-E., Servat, É., Kouame, B., Boyer, J.-F., Lubes, H., and Masson, J.-M. (1995). Manifestations de la sécheresse en Afrique de l'Ouest non sahélienne. Cas de a Côte d'Ivoire, du Togo et du Bénin. Sci. Changements Planétaires/Sécheresse 6, 95–102.
Piel, A. K., Strampelli, P., Greathead, E., Hernandez-Aguilar, A., Moore, J., and Stewart, F. A. (2017). The diet of open-habitat chimpanzees (Pan troglodytes schweinfurthii) in the Issa valley, western Tanzania. J. Hum. Evol. 112, 57–69. doi: 10.1016/j.jhevol.2017.08.016
Potts, K. B., Chapman, C. A., and Lwanga, J. S. (2009). Floristic heterogeneity between forested sites in Kibale National Park, Uganda: insights into the fine-scale determinants of density in a large-bodied frugivorous primate. J. Anim. Ecol. 78, 1269–1277. doi: 10.1111/j.1365-2656.2009.01578.x
Pruetz, J., and Bertolani, P. (2009). Chimpanzee (Pan troglodytes verus) behavioral responses to stresses associated with living in a savannah-mosaic environment: implications for hominin adaptations to open habitats. Paleoanthropology 2009, 252–262. doi: 10.4207/PA.2009.ART33
Pruetz, J. D. (2006). “Feeding ecology of savanna chimpanzees (Pan troglodytes verus) at Fongoli, Senegal,” in Feeding Ecology in Apes and Other Primates, eds G. Hohmann, M. Robbins, and C. Boesch (Cambridge: Cambridge University Press), 161–182.
Pruetz, J. D. (2018). Nocturnal behavior by a diurnal ape, the West African chimpanzee (Pan troglodytes verus), in a Savanna environment at Fongoli, Senegal. Am. J. Phys. Anthropol. doi: 10.1002/ajpa.23434. [Epub ahead of print].
Pruetz, J. D., and Bertolani, P. (2007). Savanna chimpanzees, Pan troglodytes verus, hunt with tools. Curr. Biol. 17, 412–417. doi: 10.1016/j.cub.2006.12.042
Pruetz, J. D., Bertolani, P., Boyer Ontl, K., Lindshield, S., Shelley, M., and Wessling, E. (2015). New evidence on the tool-assisted hunting exhibited by chimpanzees (Pan troglodytes verus) in a savannah habitat at Fongoli, Sénégal. R. Soc. Open Sci. 2:140507. doi: 10.1098/rsos.140507
Pruetz, J. D., Marchant, L. F., Arno, J., and McGrew, W. C. (2002). Survey of savanna chimpanzees (Pan troglodytes verus) in Southeastern Senegal. Am. J. Primatol. 58, 35–43. doi: 10.1002/ajp.10035
Pulliam, H. R. (2000). On the relationship between niche and distribution. Ecol. Lett. 3, 349–361. doi: 10.1046/j.1461-0248.2000.00143.x
Quinn, R. L., Lepre, C. J., Feibel, C. S., Wright, J. D., Mortlock, R. A., Harmand, S., et al. (2013). Pedogenic carbonate stable isotopic evidence for wooded habitat preference of early Pleistocene tool makers in the Turkana Basin. J. Hum. Evol. 65, 65–78. doi: 10.1016/j.jhevol.2013.04.002
R Core Team (2016). R: A Language and Environment for Statistical Computing. Vienna: R Foundation for Statistical Computing.
Reed, K. E. (1997). Early hominid evolution and ecological change through the African Plio-Pleistocene. J. Hum. Evol. 32, 289–322. doi: 10.1006/jhev.1996.0106
Samuni, L., Preis, A., Mundry, R., Deschner, T., Crockford, C., and Wittig, R. M. (2017). Oxytocin reactivity during intergroup conflict in wild chimpanzees. Proc. Natl. Acad. Sci. U.S.A. 114, 268–273. doi: 10.1073/pnas.1616812114
Schielzeth, H., and Forstmeier, W. (2009). Conclusions beyond support: overconfident estimates in mixed models. Behav. Ecol. 20, 416–420. doi: 10.1093/beheco/arn145
Servat, E., Paturel, J. B., Kouamé, H. L., Ouedraogo, M., and Masson, J. (1997). Climatic variability in humid Africa along the Gulf of Guinea–Part I: detailed analysis of the phenomenon in Cote d'Ivoire. J. Hydrol. 191, 1–15. doi: 10.1016/S0022-1694(96)03068-5
Sexton, J. P., McIntyre, P. J., Angert, A. L., and Rice, K. J. (2009). Evolution and ecology of species range limits. Annu. Rev. Ecol. Evol. S 40, 415–436. doi: 10.1146/annurev.ecolsys.110308.120317
Sherry, D. S., and Ellison, P. T. (2007). Potential applications of urinary C-peptide of insulin for comparative energetics research. Am. J. Phys. Anthropol. 133, 771–778. doi: 10.1002/ajpa.20562
Sol, D., Duncan, R. P., Blackburn, T. M., Cassey, P., and Lefebvre, L. (2005). Big brains, enhanced cognition, and response of birds to novel environments. Proc. Natl. Acad. Sci. U.S.A. 102, 5460–5465. doi: 10.1073/pnas.0408145102
Stolwijk, A., Straatman, H., and Zielhuis, G. (1999). Studying seasonality by using sine and cosine functions in regression analysis. J. Epidemiol. Commun. Health 53, 235–238. doi: 10.1136/jech.53.4.235
Suarez, A. V., Tsutsui, N. D., Holway, D. A., and Case, T. J. (1999). Behavioral and genetic differentiation between native and introduced populations of the Argentine ant. Biol. Invasions 1, 43–53. doi: 10.1023/A:1010038413690
Surbeck, M., Deschner, T., Behringer, V., and Hohmann, G. (2015). Urinary C-peptide levels in male bonobos (Pan paniscus) are related to party size and rank but not to mate competition. Horm. Behav. 71, 22–30. doi: 10.1016/j.yhbeh.2015.03.007
Surbeck, M., Deschner, T., Weltring, A., and Hohmann, G. (2012). Social correlates of variation in urinary cortisol in wild male bonobos (Pan paniscus). Horm. Behav. 62, 27–35. doi: 10.1016/j.yhbeh.2012.04.013
Suzuki, A. (1969). An ecological study of chimpanzees in a savanna woodland. Primates 10, 103–148. doi: 10.1007/BF01730979
Varner, J., and Dearing, M. D. (2014). Dietary plasticity in pikas as a strategy for atypical resource landscapes. J. Mammal. 95, 72–81. doi: 10.1644/13-MAMM-A-099.1
Varner, J., Horns, J. J., Lambert, M. S., Westberg, E., Ruff, J. S., Wolfenberger, K., et al. (2016). Plastic pikas: behavioural flexibility in low-elevation pikas (Ochotona princeps). Behav. Processes 125, 63–71. doi: 10.1016/j.beproc.2016.01.009
Watts, D. P., Potts, K. B., Lwanga, J. S., and Mitani, J. C. (2012). Diet of chimpanzees (Pan troglodytes schweinfurthii) at Ngogo, Kibale National Park, Uganda, 2. Temporal variation and fallback foods. Am. J. Primatol. 74, 130–144. doi: 10.1002/ajp.21015
Wessling, E. G., Kuehl, H. S., Mundry, R., Deschner, T., and Pruetz, J. D. (2018). The costs of living at the edge: seasonal stress in wild savanna-dwelling chimpanzees. J. Hum. Evol. doi: 10.1016/j.jhevol.2018.03.001. [Epub ahead of print].
Wheeler, P. E. (1984). The evolution of bipedality and loss of functional body hair in hominids. J. Hum. Evol. 13, 91–98. doi: 10.1016/S0047-2484(84)80079-2
Wheeler, P. E. (1991). The thermoregulatory advantages of hominid bipedalism in open equatorial environments: the contribution of increased convective heat loss and cutaneous evaporative cooling. J. Hum. Evol. 21, 107–115. doi: 10.1016/0047-2484(91)90002-D
White, T. D., Ambrose, S. H., Suwa, G., Su, D. F., DeGusta, D., Bernor, R. L., et al. (2009). Macrovertebrate paleontology and the Pliocene habitat of Ardipithecus ramidus. Science 326, 67–93. doi: 10.1126/science.1175822
Wrangham, R., Conklin, N., Chapman, C., Hunt, K., Milton, K., Rogers, E., et al. (1991). The significance of fibrous foods for Kibale Forest chimpanzees. Philos. Trans. R. Soc. Lond. B Biol. Sci. 334, 171–178.
Wrangham, R. W. (1977). “Feeding behavior of chimpanzees in Gombe National Park Tanzania,” in Primate Ecology, ed T. H Clutton-Brock (London: Academic Press), 504–538.
Wrangham, R. W., Clark, A. P., and Isabirye-Basuta, G. (1992). “Female social relationships and social organization of Kibale Forest chimpanzees.” in Topics in primatology, Vol. 1. Human origins, eds T. Nishida, W. C. McGrew, P. Marler, M. Pickford, F. B. M. de Waal (Tokyo: University of Tokyo Press), 81–98.
Keywords: cortisol, c-peptide, creatinine, range limit, seasonal, niche, margin
Citation: Wessling EG, Deschner T, Mundry R, Pruetz JD, Wittig RM and Kühl HS (2018) Seasonal Variation in Physiology Challenges the Notion of Chimpanzees (Pan troglodytes verus) as a Forest-Adapted Species. Front. Ecol. Evol. 6:60. doi: 10.3389/fevo.2018.00060
Received: 27 November 2017; Accepted: 23 April 2018;
Published: 15 May 2018.
Edited by:
Jordi Figuerola, Estación Biológica de Doñana (EBD), SpainReviewed by:
Alex Kenneth Piel, Liverpool John Moores University, United KingdomBeate Anna Apfelbeck, Wissenschaftszentrum Weihenstephan, Technische Universität München, Germany
Copyright © 2018 Wessling, Deschner, Mundry, Pruetz, Wittig and Kühl. This is an open-access article distributed under the terms of the Creative Commons Attribution License (CC BY). The use, distribution or reproduction in other forums is permitted, provided the original author(s) and the copyright owner are credited and that the original publication in this journal is cited, in accordance with accepted academic practice. No use, distribution or reproduction is permitted which does not comply with these terms.
*Correspondence: Erin G. Wessling, ZXJpbl93ZXNzbGluZ0BldmEubXBnLmRl