- 1Planning of Landscape and Urban Systems, Department of Civil, Environmental and Geomatic Engineering, ETH Zurich, Zurich, Switzerland
- 2Institute for Transport Planning and Systems, Department of Civil, Environmental and Geomatic Engineering, ETH Zurich, Zurich, Switzerland
- 3Department of Ecology and Evolution, University of Lausanne, Lausanne, Switzerland
- 4Institute of Earth Surface Dynamics, University of Lausanne, Lausanne, Switzerland
- 5Biodiversity and Conservation Biology, WSL Swiss Federal Research Institute, Birmensdorf, Switzerland
- 6Institute of Integrative Biology, Department of Environmental Systems Science, ETH Zurich, Zurich, Switzerland
Worldwide, the expansion of settlement and transport infrastructure is one of the most important proximate as well as ultimate causes of biodiversity loss. As much as every modern human society depends on a network of settlements that is well-connected by transport infrastructure (i.e., settlement network), animal and plant species depend on networks of habitats between which they can move (i.e., habitat networks). However, changes to a settlement network in a region often threaten the integrity of the region's habitat networks. Determining plans and policy to prevent these threats is made difficult by the numerous interactions and feedbacks that exist between and within the settlement and habitat networks. Mathematical models of coupled settlement and habitat networks can help us understand the dynamics of this social-ecological system. Yet, few attempts have been made to develop such mathematical models. In this paper, we promote the development of models of coupled settlement and habitat networks for biodiversity conservation. First, we present a conceptual framework of key variables that are ideally considered when operationalizing the coupling of settlement and habitat networks. In this framework, we first describe important network-internal interactions by differentiating between the structural (i.e., relating to purely physical conditions determining the suitability of a location for living or movement) and functional (i.e., relating to the actual presence, abundance or movement of people or other organisms) properties of either network. We then describe the main one-way influences that a settlement network can exert on the habitat networks and vice versa. Second, we give several recommendations for the mathematical modeling of coupled settlement and habitat networks and present several existing modeling approaches (e.g., habitat network models and land-use transport interaction models) that could be used for this purpose. Lastly, we elaborate on potential applications of models of coupled settlement and habitat networks in the development of complex network theory, in the assessment of system resilience and in conservation, transport and urban planning. The development of coupled settlement and habitat network models is important to gain a better system-level understanding of biodiversity conservation under a rapidly urbanizing and growing human population.
Introduction
Due to human activities, the past century has seen highly elevated rates of species extinctions at the global scale (Ceballos et al., 2015) and strong reductions in species richness at the local scale (Newbold et al., 2015). A large proportion of this biodiversity loss is attributed to land-use change (Millennium Ecosystem Assessment, 2005; Newbold et al., 2015). Important land-use related threats to biodiversity are, amongst others, the development of settlements and transport infrastructure (Salafsky et al., 2008), which has led to the destruction, degradation or fragmentation of natural habitats across the world (e.g., Salafsky et al., 2008; Reed et al., 2012; McDonald et al., 2013; Bennie et al., 2016). The severity of the threat of settlements and transport infrastructure to the survival of many plant and animal species will further increase with a strongly growing and urbanizing global population (Güneralp et al., 2013; United Nations, 2015). It is predicted that a total of 6.3 billion people (i.e., two-thirds of the global population) will live in urban areas by 2050 (United Nations, 2015), the area of settlements will nearly triple between 2000 and 2030 (Seto et al., 2012) and the combined length of roads and railways will increase by 60% between 2010 and 2050 (Dulac, 2013). Given the fact that many urban centers occur in areas with a high biodiversity (Luck, 2007; Luck and Smallbone, 2010), it is a major challenge to prevent these ever increasing rates of urbanization from having a devastating effect on the world's biodiversity. It is therefore of vital importance that we increase our understanding of how the long-term development of settlements and transport infrastructure can be conducted in a way that minimizes the impact on biodiversity.
The development of settlements and transport infrastructure are two strongly interrelated processes (Wegener, 2014). Settlements connected by transport infrastructure (e.g., roads and railways) form intricate spatial networks (i.e., settlement networks), in which changes to either the settlements or the transport infrastructure can bring about changes in other parts of the network (Badoe and Miller, 2000; Levinson, 2008; Israel and Cohen-Blankshtain, 2010). In a similar way, animal and plant populations connected via movement, dispersal or migration (henceforth referred to as movement) can also form large spatial networks (i.e., habitat networks). Also in habitat networks, changes to any part of the network can have profound effects on other parts of the network or on the network as a whole (Galpern et al., 2011). Just as much as modern human societies have become socially and economically dependent on well-connected settlement networks (Axhausen, 2007; Lakshmanan, 2011), many plant and animal species are dependent on well-connected habitat networks for their survival (Crooks and Sanjayan, 2006). However, expansion of a settlement network often threatens the integrity of habitat networks for plants and animals. Urban sprawl and associated land-use changes can destroy or degrade habitats as well as reduce the movement of species between habitats (Salafsky et al., 2008; Pickett et al., 2011; Van Strien et al., 2014). Also transportation infrastructure and traffic can be detrimental to both habitat suitability and connectivity (Forman et al., 2003; Seiler, 2003; Salafsky et al., 2008; Holderegger and Di Giulio, 2010). Paradoxically, human society also depends on biodiversity as driver for the functioning of a healthy ecosystems (Hector and Bagchi, 2007; MacDougall et al., 2013) and for the provision of many ecosystem services (e.g., clean water, crops, water regulation; Isbell et al., 2011; Mace et al., 2012). Due to this mutual dependency, coupled settlement and habitat networks should be regarded as a complex social-ecological system (Berkes and Folke, 2000). Yet, they have not been treated as such in science or practice.
Coupled social-ecological systems often “exhibit non-linear dynamics with thresholds, reciprocal feedback loops, time lags, resilience, heterogeneity, and surprises” (Liu et al., 2007, p. 1513). Ignoring such complexities when managing social-ecological systems can result in ineffective or even counterproductive policies or actions (Levin et al., 2013). Models of social-ecological systems can help us reveal such complexities and ultimately find better ways to conserve biodiversity (Luck, 2007; Milner-Gulland, 2012). The dynamics and processes that exist within either settlement or habitat networks are fairly well studied. Studies on the evolution of or processes in settlement networks have been performed in the field of human geography and inspired by concepts like central place theory (Christaller, 1933) or complex systems theory (Prigogine and Stengers, 1984). Likewise, the interest in habitat networks in ecology originated from research areas like island biogeography (MacArthur and Wilson, 1967), population genetics (Wright, 1943) or metapopulation ecology (Levins, 1969). Although there is thus a long history of studies aiming to reveal interactions in either settlement or habitat networks, few studies have regarded these networks as a coupled system.
To parameterize the relationships that exist between settlement and habitat networks, one can draw upon knowledge and approaches from several fields of research. In the fields of transport and urban planning, studies have assessed how to mitigate the negative impacts of transport and urban development on ecology or environment (e.g., Williams, 2005; Beatley, 2011; Ercoskun, 2012; Nuissl and Siedentop, 2012). The ecological effects of settlements, roads and traffic have been extensively studied in the research fields of urban ecology (Gaston, 2010; Forman, 2014) and road ecology (Forman et al., 2003; Van der Ree et al., 2015; Bennett, 2017). However, within the fields of road and urban ecology, focus has mainly been on specific types of interactions at a local scale. In an overview of trends in road ecology, Van der Ree et al. (2011, p. 1) concludes that “the current situation, with numerous small-scale projects being undertaken independently of each other, cannot provide the information required to quantify and mitigate the negative effects of roads and traffic on higher levels.” In the book Urban Ecology, McKinney (2010, p. 304) summarizes that “[…] conservation planning must extend far beyond the very local scales at which most urban ecology is carried out. We must begin to focus much more attention on the many impacts of cities on distant areas at all spatial scales […].” It thus seems crucial that knowledge of these local scale projects is aggregated and integrated in models of coupled settlement and habitat networks in order to study the dynamics in these networks at larger scales and in a more holistic, system-focussed approach (Ramalho and Hobbs, 2012). Yet, very few studies have actually endeavored to model coupled settlement and habitat networks in a dynamic way.
In order to aid scientists in setting up models of coupled settlement and habitat networks, we present a conceptual framework of such models and discuss several ways in which such models can be implemented and applied. It can be a challenging task to define and parameterize coupled networks representing social-ecological systems (Janssen et al., 2006). In our conceptual framework, we therefore present an overview of key variables that were found to be important for the dynamics in coupled settlement and habitat networks and are thus ideally considered when developing a model of this social-ecological system. Furthermore, from our assessment of key variables, we distil four general recommendations for implementing integrated models of coupled settlement and habitat networks. We also present several existing mathematical modeling approaches that can form the basis of a coupled network model. Lastly, we discuss several ways in which such models can contribute to biodiversity conservation. We see potential applications in the development of complex network theory, in the assessment of system resilience and in conservation, transport and urban planning.
Network Definitions
Many social-ecological systems can be represented as networks, but the determination of the network's structure and components can present a challenge (Janssen et al., 2006). Therefore, in order to focus our discussion, we need to define settlement and habitat networks in more detail. Networks (or graphs) consist of nodes (or vertices) and edges (or links), which, in the case of spatial networks, “are constrained by some geometry and are usually embedded in a two- or three-dimensional space, and this has important effects on their topological properties and consequently on the processes which take place on them” (Barthélemy, 2011, p. 3). An edge in a network indicates a relationship between nodes. Properties can be ascribed to nodes and edges by assigning weights, expressing for instance area, population sizes or movement rates.
As the relationship between biodiversity and human activities is scale-dependent (Pautasso, 2007), we will focus on settlement or habitat networks at a regional or national scale that encompass tens or hundreds of settlements. The interactions between the networks can, however, be at a local scale. In this article, the term network does not only refer to the abstract, mathematical representations of nodes and edges, but we use the term in a more encompassing way to indicate the connectedness and relatedness of settlements or habitats in a region.
Settlement Networks
The nodes and edges in networks of areas where people live can be defined in several ways, mainly depending on the level of spatial aggregation of the networks (e.g., Buhl et al., 2006; De Montis et al., 2007; Xie and Levinson, 2011). Fine-scale networks encompassing one or a few settlements, usually consist of nodes that represent road intersections and edges that represent the road segments connecting them (referred to as street or road networks; e.g., Buhl et al., 2006; Lämmer et al., 2006). At a regional or national scale, networks encompass many settlements and are usually more aggregated: a node represents a whole settlement and the edges between nodes represent main roads connecting settlements (e.g., Mollanejad and Zhang, 2014) or the flows of people between settlements (e.g., De Montis et al., 2007). For such aggregated networks, nodes need to be identified by delineating settlements, which is not an easy task given the fact that settlements usually do not follow administrative boundaries (Antrop, 2000). Settlements can be delineated making use of, for example, census data, remote sensing or population data, but also with fine-scale street network data (Zhou, 2015). It should be noted, however, that also at national scales, fine-scale road networks have been used to model traffic flows (e.g., Meister et al., 2010). Settlement networks may also be referred to as urban networks (Antrop, 2000). However, as the term “urban” could suggest a focus on cities, we here prefer the more comprehensive term settlement, which includes smaller villages or towns. As human mobility along roads (including highways) is by far the dominant mode of transport (Dulac, 2013), we will focus our discussion on roads, but many of our findings can also be applied to other forms of terrestrial transport (e.g., railways).
Habitat Networks
There are several ways in which nodes and edges can be defined in networks of animal or plant habitats (Urban et al., 2009; Galpern et al., 2011; Rayfield et al., 2011). In general, the nodes represent habitat patches for a certain (group of) species. The delineation of habitats in a habitat network is not always straightforward, especially not for species that are habitat generalist or those that inhabit landscapes where transitions between environmental conditions are gradual and not abrupt (Galpern et al., 2011). Habitat suitability modeling can be employed for habitat patch delineation (e.g., Ramirez-Reyes et al., 2016). The edges in habitat networks represent the (potential) movement of organisms between the habitats (Galpern et al., 2011). In contrast to human movement, which mainly takes place along transport infrastructure such as roads or railways, the movement of most animals is less bound to distinct landscape features. Furthermore, animal movement is notoriously difficult to measure (Kool et al., 2013). For these two reasons, the definition of edges and their weights (expressing for example movement rates) can present a challenge in habitat networks. Computer models can help determine potential movement routes through the landscape (e.g., least-cost paths or current flow maps; Adriaensen et al., 2003; McRae, 2006). These routes are often derived from resistance surfaces, which are raster maps of the study area showing the hypothesized cost of movement through each cell (Zeller et al., 2012; Cushman et al., 2014). Alternatively, transects can be used to quantify the landscape between habitat patches (e.g., straight-line or least-cost transects; Scolozzi and Geneletti, 2012; Van Strien et al., 2012, 2014). Empirical data on animal movement is either collected by tracking the movement of individual animals (e.g., mark-recapture studies, radio tracking, GPS sensors) or with genetic methods (Kool et al., 2013). Note that for the vast majority of the world's regions there is one settlement network, but many habitat networks, since species differ in their habitat or movement characteristics (e.g., Concepción et al., 2015).
Conceptual Framework for Models of Coupled Settlement and Habitat Networks
Our conceptual framework summarizes the main network-internal interactions that can take place in either the settlement or habitat networks (sections Interactions Within Settlement Networks and Interactions Within Habitat Networks, respectively) as well as the key one-way influences of settlement networks on habitat networks (section Influences of a Settlement Network on Habitat Networks) and those of habitat networks on settlement networks (section Influences of Habitat Networks on a Settlement Network). A dynamic feedback mechanism between settlement and habitat networks can only exist if such influences exist in both directions (Figure 1). These interactions and influences are important to capture the complexity of the dynamics within this social-ecological system.
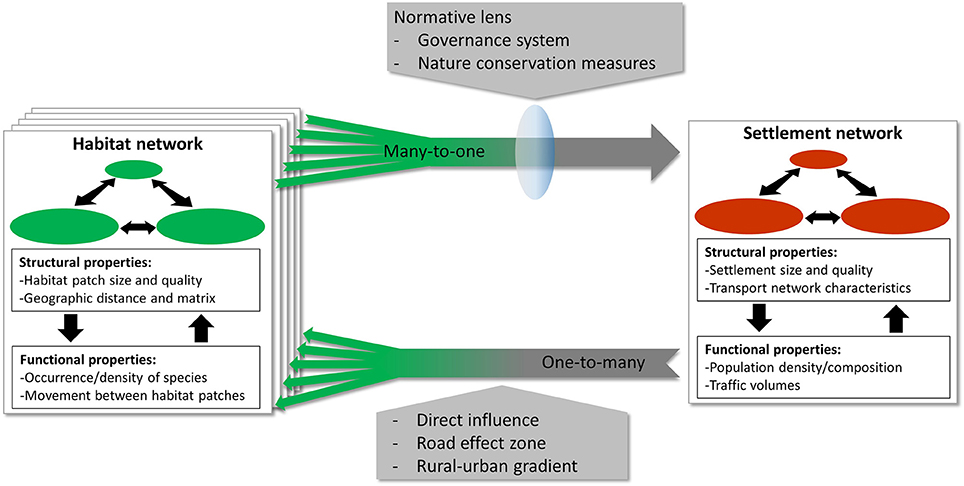
Figure 1. Conceptual framework of models of coupled settlement and habitat networks. The different interactions and influences within and between settlement and habitat networks are shown. Both types of network are embedded in the same landscape. In general there is one settlement network and multiple species-specific habitat networks. Changes in the settlement network often influence several habitat networks (i.e., one-to-many relationship), whereas habitat networks usually only influence the settlement network if change takes place in multiple networks (i.e., many-to-one relationship). Network-internal interactions are between structural and functional properties of the networks. Changes to the structural or functional properties of settlement network can have a direct influence on habitat networks, but also an indirect influence via a range of effects that radiate out from roads (i.e., road-effect zone) or from settlement centers (i.e., urban-rural gradient). The influence of the habitat networks on the settlement network is rarely direct, but usually only arises once nature conservation measures are installed, which are a result of the implemented governance system and influenced by the normative lens through which society views biodiversity and its conservation.
To aid our description of the network-internal interactions, we differentiate between the structural and functional properties of both the settlement and habitat networks (Newman, 2003; Figure 1), which has also been used to differentiate between different types of habitat connectivity (Taylor et al., 2006). The structural properties of the networks are characterized by the purely physical conditions that determine whether an area is inhabitable for humans or other organisms (i.e., structural properties of the nodes) or suitable to move through (i.e., structural properties of the edges). For settlement networks, buildings and roads are examples of structural properties of nodes and edges respectively. The structural properties of a habitat network for an amphibian could be, for instance, water bodies (nodes) that are at a certain distance from one another (edges). These structural properties do not reflect the actual use of the networks by humans or other organisms. In contrast, the functional properties of networks are determined by variables expressing the actual presence or abundance (i.e., functional properties of nodes) or movement (i.e., functional properties of edges) of people or other organisms in the networks. The structural and functional properties of the network interact with each other.
Interactions Within Settlement Networks
Human artifacts, such as buildings and roads, form the basis of the structural properties of settlement networks. In modern times, these artifacts basically form the prerequisites for people to inhabit a certain region. Their quality and quantity will thus determine the functional properties of the network, i.e., the number of people that live in a certain settlement or that travel between settlements (Figure 1). Due to the capacity of humans to alter their surrounding for their own benefit, there are strong spatial and temporal interactions between the structural and functional properties in settlement networks (Wegener and Fürst, 1999; Badoe and Miller, 2000). Changes to road or settlement structure can lead to demographic or traffic flows changes, which in turn can trigger settlement or road development, and so forth (Axhausen et al., 2011; Xie and Levinson, 2011; Wegener, 2014). Such an effect was, for instance, reported for the state of Minnesota, USA by Iacono and Levinson (2016, p. 216), who “find evidence of feedbacks between population changes and the growth of local [road] networks.” Also for the railway development in the city of London, UK, during the period 1871-2007, Levinson (2008, p. 19) found that there was a positive feedback between the development of the rail network and population growth in certain neighborhoods: “train service led to a suburbanization of countryside and increased population of new developments, which attracted more railways.” Several studies have shown that the establishment of new transport infrastructure, be it roads or railways, can spark growth in the settlements along these infrastructures as well as changes in the locations of organizations and businesses throughout the region (e.g., Kreibich, 1978; Chi, 2010; Israel and Cohen-Blankshtain, 2010). Job opportunities play an important role in the relocation of people. For instance, one of the main drivers of the widespread phenomenon of rural abandonment is the decrease of job opportunities in agricultural areas and increasing opportunities in and around larger settlements (Rey Benayas et al., 2007). These are only some of the many examples of the interactions that can take place within settlement networks.
Settlement network expansion is hardly limited by those natural factors that limit population growth and spread in other species (e.g., resource availability, competition with other species, climate, topography and diseases). Therefore, to prevent their resource use from surpassing sustainable levels (Ostrom, 2009), human societies have implemented systems of governance (i.e., “all processes of governing, whether undertaken by a government, market, or network, whether over a family, tribe, formal or informal organization, or territory, and whether through laws, norms, power, or language”; Bevir, 2012, p. 1). In settlement networks, governance systems can regulate, for instance, traffic or land allocation. Empirical evidence indicates that traffic can be regulated with a range of policy actions, such as land-use planning, pricing of roads or of parking, increasing the attractiveness of public transport or awareness campaigns (Graham-Rowe et al., 2011; Salon et al., 2012). As changes in mobility patterns can have consequences for location choices of households, companies and organization, these policy measures may cause unforeseen effects on patterns of built-up land-use in the future (Goodwin, 1998), but can also be used as an effective measure to steer settlement development (Nuissl and Siedentop, 2012). Other governance systems to regulate settlement development can be based on planning (e.g., zoning, density controls or transport planning), management (e.g., information campaigns or forums) or market forces (e.g., development taxes, subsidies or tradable permit schemes; Nuissl and Siedentop, 2012).
Interactions Within Habitat Networks
In order to describe the interactions within habitat networks, it is important to define the functional and structural properties of habitat networks. For the assessment of habitat connectivity, a distinction between structural and functional properties is commonly made. Taylor et al. (2006, p. 30) write that “structural connectivity […] describes only physical relationships among habitat patches such as habitat corridors or inter-patch distances. […] Functional connectivity, on the other hand, increases when some change in the landscape structure (including but not limited to changes in structural connectivity) increases the degree of movement or flow of organisms through the landscape.” Here we apply this classification to both the edges (that express habitat connectivity) and the nodes in habitat networks. Especially important structural properties of habitat patches (i.e., nodes) are their size and their quality (Pascual-Hortal and Saura, 2006; Prevedello and Vieira, 2010; Hodgson et al., 2011). Influential structural properties of the edges in habitat networks are their geographic distance and the landscape between habitat patches (the latter is commonly referred to as matrix; Hodgson et al., 2011). Functional properties of the nodes in a habitat network are for instance the presence or abundance of certain species in habitat patches, whereas the existence or frequencies of movement among patches are functional properties of the edges.
Functional properties of habitat networks result from behavioral responses of species to their surroundings, which are generally specific to a (group of) species (Concepción et al., 2015). Therefore, separate habitat networks need to be parameterized for each (group of) species. Empirical studies have shown that changes to habitats and their surroundings can affect species richness (Fahrig, 2003) as well as the abundance and occurrence of species in habitats (Prugh et al., 2008; Thornton et al., 2011). Habitat quality affects, for instance, patch occupancy (e.g., Thomas et al., 2001; Fleishman et al., 2002). Geographic distance as well as matrix composition and configuration between habitat patches have effects on the movement probability of organisms between patches (Holderegger and Wagner, 2008). Therefore, changes to the landscape matrix among habitat patches can have an effect on a patch's emigration and immigration rates (e.g., Todd et al., 2009). Also genetic processes can be influenced by habitat patch size and isolation (i.e., a measure calculated from a patch's geographic distance to other patches; e.g., Holmes et al., 2013). Through biological interactions between species, certain structural landscape changes also exert indirect effects on species. This is, for instance, shown in a study by Todd et al. (2008) where habitat disturbance led to an increase in fire ants, which in turn led to a decrease of their prey (i.e., amphibians) in these habitats. Also mathematical models of ecological processes in habitat networks frequently use the above structural properties and species characteristics as explanatory variables. For instance, metapopulation dynamics can be assessed from patch isolation, patch size and a species' migration range (Hanski, 1998). Several habitat connectivity indices are calculated from habitat size, habitat quality and the probability a species moves between habitat patches (which can be a function of matrix quality and interpatch distance; Saura and Pascual-Hortal, 2007).
The relative importance of the structural properties of habitat networks (i.e., habitat size, quality, isolation and matrix quality) on ecological processes is a subject of debate. From a quantitative review of 104 studies, Prevedello and Vieira (2010, p. 1205) concluded that “overall, the type of matrix is important, but patch size and isolation are the main determinants of ecological parameters in landscapes.” In another review, Hodgson et al. (2011, p. 148) found that “variations in habitat area and quality have bigger effects [on population viability] than variations in spatial arrangement of habitats or properties of the intervening land.” With respect to biodiversity, Fahrig (2013, p. 1655) posed that “the number of species in a patch is a function of both the size of the patch […], and the area of habitat in the landscape surrounding the patch […].” In a reaction to the latter publication, Hanski (2015) argues that the number of species is not only a function of habitat amount, but also of habitat fragmentation. Despite these mixed findings, patch size is the one variable that is consistently mentioned as being an important determinant for ecological processes.
Functional properties of networks (e.g., the presence or abundance of a species) can also influence the structural properties of habitat networks (Figure 1). Although the ability of plant and animal species to alter their surroundings is generally small compared to that of humans, the influence of organisms on their environment should not be neglected (Wright and Jones, 2006). So-called “ecosystem engineers are organisms that directly or indirectly modulate the availability of resources to other species, by causing physical state changes in biotic or abiotic materials. In so doing they modify, maintain and create habitats” (Jones et al., 1994, p. 373). Ecosystem engineers are represented in many organismic groups from small organisms, such as ants or phytoplankton, to large ones, such as beavers, elephants and forest trees (Jones et al., 1994).
Influences of a Settlement Network on Habitat Networks
The influences of the processes taking place in settlement networks on those in habitat networks are manifold and studied in the research fields of road ecology and urban ecology. For comprehensive overviews of the findings in these fields, we refer to Forman et al. (2003), Hansen et al. (2005), Gaston (2010), Luck and Smallbone (2010), Forman (2014), Van der Ree et al. (2015) and Bennett (2017). The influence of a settlement network on habitat networks can be characterized as a “one-to-many” relationship (Figure 1), as changes in a settlement network will usually affect multiple species. For instance, in most cases road construction or changes to traffic flows will not only affect a certain species, but a range of species that are sensitive to such changes. Likewise, the conversion of forest patches to residential areas will affect all species inhabiting the respective patch to a certain degree.
Many changes to settlements will affect the structural properties of habitat networks. A direct effect is caused by the sealing of land that is used for constructing buildings and other urban structures, which renders the land uninhabitable for most species, thereby destroying habitat patches, reducing their size or fragmenting them (McKinney, 2002). However, within settlements the intensity of these effects is not homogenous in space and usually follows a gradient from densely built inner cities to relatively sparsely built peri-urban areas (i.e., commonly refered to as the “rural-urban gradient”; McDonnell and Hahs, 2008). For example, land-use change radiating out from settlement centers can produce a habitat-loss gradient, which is “a gradient of natural habitat loss that steepens from rural areas toward the urban center” (McKinney, 2002, p. 884). The shape of this rural-urban gradient strongly depends on the type of outward expansion that a city experienced in the past (Forman, 2014). A settlement's influence can also reach beyond its boundaries. Habitat quality surrounding settlements can be affected by, for instance, changes in nutrient and hydrological cycles and water quality, disturbance from domestic animals like dogs and cats, and disturbance from recreational activities by humans (Hansen et al., 2005). Land-use change in and around settlements can also replace natural land cover with land-uses that are less permeable for species (Bierwagen, 2007), thereby changing the structural properties of a habitat network's edges (i.e., inter-patch movement is a function of, among others, the edge's structural properties).
As with settlements, roads and traffic can also have profound effects on the structural properties of habitat networks. Apart from the habitat destruction directly caused by road construction (Seiler, 2003; Coffin, 2007), influences of roads on habitat networks usually radiate into the surrounding landscape, creating a “road-effect zone” (Forman, 2000; Coffin, 2007; Ibisch et al., 2016). Not only the habitat size, but also the habitat quality can be affected both at the location of roads and in their surrounding (Coffin, 2007). Factors like traffic noise, pollutants, light and invasive species can reduce habitat quality in the landscape surrounding a road (Spellerberg, 1998; Seiler, 2003; Hulme, 2009). Additionally, road construction often facilitates access of humans into the surrounding natural habitats, which can cause a range of disturbances, such as outdoor sports (Trombulak and Frissell, 2000). Roads and traffic can also change the structural properties of edges in a habitat network, by changing the permeability of the matrix between habitat patches (Jaeger et al., 2005; Holderegger and Di Giulio, 2010).
Changes to the settlement network can also directly affect the functional properties of habitat networks. In many cases, processes leading to habitat loss or degradation are also accompanied by the destruction or removal of plants and animals from the area. Worldwide, settlement expansion, for example, triggers the spread of non-native species and changes biotic interactions (McKinney, 2002; Hansen et al., 2005). Similar effects can also be found in the surroundings of new roads (Trombulak and Frissell, 2000). Avoidance of roads or traffic and mortality by cars can reduce species' movement between habitat patches or populations (Jaeger et al., 2005; Holderegger and Di Giulio, 2010). Mitigation structures, like wildlife over- or underpasses and fencing, have proven to be effective to reduce roadkill (Rytwinski et al., 2016), but conclusive evidence for positive effects on population connectivity at the landscape scale is rarely documented (Van der Ree et al., 2007; Corlatti et al., 2009).
Not all changes to the habitat networks resulting from changes in settlement networks are negative for biodiversity. The reaction of species to settlements ranges from species that adapt to such human surroundings to species that are very sensitive to any disturbance caused by humans (McKinney, 2002, 2008; Hansen et al., 2005; Reed et al., 2012; Concepción et al., 2015). Whereas urban core areas are generally found to be species poor (McKinney, 2002; Reed et al., 2012), suburbs can actually be relatively species rich (McKinney, 2008). However, the relatively high abundance of alien species in suburban areas can also explain—at least partially—the relatively high biodiversity in these areas. In Central Europe, the share of alien species in total species richness increases with city size (Pyšek, 1998). Also for roads, there are species that benefit from their existence as well as those that are deterred by them (Fahrig and Rytwinski, 2009; Benítez-López et al., 2010). Especially road verges present suitable habitats or movement corridors for certain species (Seiler, 2003). Road construction can thus also enhance the number of habitats or habitat connectivity for some species.
From this myriad of influences that settlement networks may have on habitat networks, there are two key factors that determine the severity of these effects at a local scale: the geographical location of settlements and roads relative to the habitats, and the density of people in settlements or using roads (Luck, 2007; Pautasso, 2007; Charry and Jones, 2009). The locations of roads and highways are necessary to determine those habitats that are influenced by road or settlement development. However, the magnitude of these influences is probably determined in large part by the intensity with which urban and peri-urban areas and roads are used by humans. Charry and Jones (2009, p. 159) argued that “road location and traffic volume are the two most important factors to assess when evaluating a road's potential impacts [on wildlife].” In addition, Luck (2007) stated that complex interactions of social, economic and demographic variables influence species diversity in and around settlements, but that human population density is likely a key driver of all these interactions. Although a positive correlation between human population density and species richness has repeatedly been found in studies at large spatial scales and with a large spatial grain, at regional scales and with a smaller grain (i.e., study area less than 10,000 km2 and grain lower than 1 km2) this correlation is generally negative (Pautasso, 2007). However, also at national scales McKee et al. (2013, p. 776) shows “that human population density is a key ultimate cause, and probably in many places a proximate cause, of species of mammals and birds becoming threatened with extinction.” The suburban peak in biodiversity is probably due to the tolerable human disturbance and the large diversity of habitats and niches found in suburban areas (McKinney, 2002). Increasing population and building density (i.e., urban densification; see below) in suburban areas could thus reduce the number of species in these suburban habitats. In several studies, human population density surrounding nature reserves was found to be positively correlated with the number of extinctions of mammal species in these reserves (Brashares et al., 2001; Parks and Harcourt, 2002).
Influences of Habitat Networks on a Settlement Network
There are several potential influences of plants or animals on humans, but we here specifically focus on those aspects of habitat networks that, if changed, can bring about changes to the structural or functional properties of the settlement network (e.g., settlement sizes, population density, traffic flows, attractiveness of settlements or mobility). The influence of habitat networks on a settlement network will usually result from the combined effect of all habitat networks in an area (i.e., a “many-to-one” relationship between habitat networks and a settlement network; Figure 1). We have classified two kinds of effects of habitat networks on settlement networks: direct influences and influences resulting from the implemented governance system.
The direct effects of habitat networks on settlement networks are relatively limited. Some human communities in developing countries can be - to some degree - directly dependent on certain undomesticated species or groups of species for their livelihood (Díaz et al., 2006; CBD, 2010). Disappearance of these species thus threatens the existence of these communities, which could force them to relocate to other areas. In developed countries, however, livelihoods of most people do no longer directly depend on the existence of wildlife. Another direct effect between habitat networks and settlement networks could come from pathogens. Historically, settlement abandonments following disease outbreaks have been reported (McLeman, 2011), such as with the plague that was spread by fleas on rats throughout Europe (Hirschfeld, 2006). In modern times, the effect of such outbreaks will probably be less devastating, but can nevertheless have an impact on the habitability of certain regions for humans (e.g., Sachs and Malaney, 2002). The above two examples (i.e., dependence on wildlife for livelihood and changes in habitability due to disease) also reflect the few cases where there is a “one-to-one” relationship between habitat and settlement networks. Another potential direct effect of habitat networks on settlement networks is urban sprawl as one of the drivers for urban sprawl is the desire of many city inhabitants to move to the countryside (i.e., country-living desire; Bhatta, 2010). However, there is little evidence that desire is directly caused by a higher biodiversity or the occurrence of certain species in the countryside. Instead, the country-living desire is better explained by factors like green surroundings, spaciousness and high numbers of recreational opportunities in the countryside (Fernandez et al., 2005; Tobias et al., 2016). Vehicle-wildlife collisions are probably one of the few examples in which mobility of humans is directly affected by the mobility of animals. Although such collisions can cause human fatalities and significant economic damage (Huijser et al., 2009), to our knowledge there are no reports of significant reductions in traffic volumes following vehicle-wildlife collisions, unless mitigation measures are being taken (see below).
The majority of effects that habitat networks can have on a settlement network will be indirect via governance systems focussed at protecting nature and the environment. The way a governance system is shaped, depends on the “normative lens” through which decision makers and society view a certain problem and define their desired system states (Figure 1). Existing norms, principles and values “underpin all decisions since they inspire those who govern how to think and make judgments about how the world works and how to act in particular situations” (Kooiman and Jentoft, 2009, p. 818). In social-ecological systems, the norms and values about the relationship of humans with nature have effects on governance systems (Kooiman and Jentoft, 2009). Different environmental ethics, such as anthropocentrism, biocentrism or ecocentrism, are anticipated to result in different environmental policy measures (Stenmark, 2002).
There is a large variety of nature conservation measures that can be taken to improve or maintain the components of habitat networks (i.e., increasing the number and size of habitats or increasing their quality and their connectivity; Salafsky et al., 2008). These measures can affect settlement networks in several, sometimes unexpected, ways. For example, the establishment of a conservation area could increase the prices of surrounding residential areas (e.g., Lutzenhiser and Netusil, 2001). However, this effect may be less strong if accessibility to the open space is not guaranteed (Geoghegan, 2002). Restricting accessibility to open spaces can be a potential conservation measure to reduce human disturbance in these areas (Newsome et al., 2013). To compensate or off-set the potential damage people are causing by developing housing in natural surroundings, economic conservation measures can be implemented, such as cap-and-trade, subsidy or tax systems and payments for ecosystem services (Pirard, 2012; Barrett et al., 2013). In order to preserve natural habitats under a growing human population, one of the most often proposed solutions is urban densification (i.e., increasing the density of buildings in existing settlements; Jabareen, 2006; Gaigné et al., 2012; Tobias et al., 2016). Also to mitigate the negative effects that roads and traffic can have on habitat networks there exist a range of potential conservation measures (Keller et al., 2003), some of which have effects on traffic flows in the settlement networks. By reducing vehicle speeds or closing roads during certain hours of the day (i.e., traffic calming), road avoidance by animals or the number of wildlife-vehicle collisions can be reduced, but at the same time traffic volumes on other roads are increased (Van Langevelde and Jaarsma, 2009). Conservation measures can thus be seen as the proximate causes of many changes in settlement networks as a reaction to changes in habitat networks.
Implementing Models of Coupled Habitat and Settlement Networks
Several authors have developed models that can be considered predecessors of models that couple habitat and settlement networks (e.g., Jaeger et al., 2006; Jaeger, 2007; Van Langevelde and Jaarsma, 2009; Rhodes et al., 2014; Van Strien and Grêt-Regamey, 2016). For instance, Jaeger (2007) simulated connectivity in landscapes with different road configurations. Similarly, Rhodes et al. (2014) modeled Koala movement and mortality in a real landscape and derived how mortality would change when increases in traffic volume were either assigned to existing roads or divided over new roads. In these studies, traffic volumes were used as a proximate variable for the investigated ecological process. However, changes in traffic flows were not modeled dynamically. In contrast, Van Langevelde and Jaarsma (2009) modeled the effect of the size of traffic calmed areas (i.e., areas in which the number of cars is reduced) on animal population persistence. In their study, traffic flows were dynamically modeled based on the size of the traffic calmed area. Yet, human population density was equal throughout the simulated landscapes. Recently, Van Strien and Grêt-Regamey (2016) simulated habitat connectivity in landscapes in which both the configuration of the road network and that of the settlements were varied. Traffic flows were calculated from human population sizes in the settlements and the travel times between settlements. The above studies showed that there are significant effects of settlement and road configuration on ecological processes taking place in habitat networks. Nevertheless, they do not adhere to all the recommendations for models of coupled settlement and habitat networks that we have outlined below.
Recommendations for Models of Coupled Settlement and Habitat Networks
Based on the description of the conceptual framework in the previous sections and its depiction in Figure 1, we have formulated four recommendations for the modeling of coupled habitat and settlement networks.
(1) Both networks should be spatially embedded. The settlement and habitat networks are by definition spatial networks, but the interactions between the networks are also of a spatial nature. For instance, the expansion of a settlement will mainly have an effect on the habitats surrounding this settlement. Similarly, increases in traffic will mainly have an influence on the quality of habitats within a road's effect zone. For many ecological processes taking place in habitat networks, habitat patch size is an important determinant (see section Interactions Within Habitat Networks). For these reasons, it is important that both networks are embedded in a spatial plane (or landscape) in which the effects that the networks have on each other can be simulated. The competition for space between the two networks can be made spatially explicit, by altering the locations and geometries of settlements (e.g., area), roads, habitats and other landscape elements over time. These locations and geometries are important variables in defining the structural properties of the settlement and habitat networks.
(2) Multiple habitat networks should be modeled for a variety of species. The “many-to-one” influence of habitat networks on settlement networks (Figure 1) makes it necessary to develop habitat networks for multiple species in order to get an accurate representation of biodiversity. Ideally, habitat networks are constructed for every species in the study region, but this is an extremely laborious task. It is therefore advisable to select (groups of) focal species so that the range of possible responses to and influences on settlement networks is covered (Van Teeffelen et al., 2012). For instance, focal species can be selected from different dispersal guilds that contain species with similar movement characteristics and habitat requirements (Lechner et al., 2017). Focal species can also be selected based on their functional traits (De Bello et al., 2010), which describe the role that a group of species play in ecosystem functioning or in the provision of ecosystem services (Díaz et al., 2007; Luck et al., 2009). In order to ensure multifunctional ecosystems, it is important that as many functional traits as possible are represented in an ecosystem and thus included in the modeling.
(3) Influences between the networks should be in both directions: i.e., from settlement to habitat networks and vice versa. Only if both networks react to changes in the other network, can a dynamic feedback mechanism be simulated. Abrupt critical transitions especially occur in systems with positive feedbacks (Angeli et al., 2004; Scheffer et al., 2012). Such feedbacks can also take place through interactions within one of the networks. Therefore, even a one-way influence from one network can trigger an abrupt transition in the other network. Nevertheless, if influences between the networks are not considered in both ways, the effects of this transition will stay limited to the network in which it takes place and a full picture of its effects in the social-ecological system cannot be obtained. In order to incorporate the influence of habitat networks on settlement networks in models, it is advisable that the latter is capable of reacting to spatial or aspatial nature conservation measures, which we have identified as the main influence of habitat networks on a settlement network (see section Influences of Habitat Networks on a Settlement Network).
(4) Both the structural and functional properties of networks should be modeled. In both networks, changes to the structural properties usually have an effect on their functional properties (section Interactions Within Settlement Networks and Interactions Within Habitat Networks). Especially in settlement networks, this effect is also vice versa (section Interactions Within Settlement Networks). In many cases, changes to functional properties in one network can trigger changes to structural properties in the other network. For example, we have identified human population density in the settlement network (i.e., a functional property) as one of the most influential drivers of changes in habitat network (see section Influences of a Settlement Network on Habitat Networks). However, the resulting changes in the habitat network are often to its structural properties (e.g., decreases in habitat quality due to an increase in traffic). Likewise, following reductions in the occurrences of species (i.e., a functional property) in multiple habitat networks (i.e., biodiversity loss), nature conservation measures can be implemented, which can have an impact on both the structural and functional properties of the settlement network (see section Influences of Habitat Networks on a Settlement Network).
Modeling Settlement Networks
Following the above recommendations, both the structural and functional properties of settlement networks as well as their interactions are ideally included in the model of the settlement network. Furthermore, the model should be spatially explicit at a local scale and predict local human population density and traffic flows as key variables. Particularly useful for this purpose are land-use transport interaction (LUTI) models, “which explicitly model the two-way interaction between land use and transport to forecast the likely impacts of land-use policies […] and of transport policies […]” (Wegener, 2014, p. 742). Although many different LUTI models have been developed based on various theoretical assumptions and which are applicable to various spatial scales and aggregation levels (Wegener, 2014; Acheampong and Silva, 2015), many of them aim to simulate the interactions between the choices people make regarding transport and the locations where they reside, work and go to for other activities such as recreation (e.g., see the conceptual model of a land-use-transport system in Acheampong and Silva, 2015). Wherever attractiveness or demand increases, construction of new buildings or roads will take place (e.g., see the land-use transport feedback cycle in Wegener and Fürst, 1999), provided that this is permitted under the implemented land-use or transport regulations. By changing the regulations, LUTI models can also be used to forecast the effect of policy measures on functional properties of a settlement network. This has, for instance, been shown by Bodenmann et al. (2014), who used a Swiss-wide LUTI model to predict how population sizes in municipalities may change due to changes in land-use regulations or changes in the transport infrastructure. In a similar way, LUTI models could also be used to forecast the effects of alternative environmental governance systems. A further advantage of LUTI models, is that many of them are agent-based models (Wegener, 2014; Acheampong and Silva, 2015), which are considered particularly useful when modeling social-ecological systems, as they allow simulating the influence of the decisions of individuals on a system's dynamics (Milner-Gulland, 2012). From several points of view, LUTI models are thus suitable for modeling the interactions in settlement networks.
A drawback of many current LUTI models is that “land-use” in these models usually refers to the built environment and that they are thus not capable of simulating changes in land-use and human influences that radiate from settlements and roads. Combining LUTI models with land-use models that consider a broader spectrum of land-uses (e.g., Verburg and Overmars, 2009) is a potential approach to simulate such gradients. Especially spatially-explicit models that link human population density to land-use change (e.g., Verburg et al., 1999) can prove useful for this purpose. To our knowledge, no models have been developed that specifically model the effects of roads or traffic on surrounding habitats.
Modeling Habitat Networks
As with the settlement networks, the habitat network models should capture the interactions between the structural and functional properties of the networks. Agent-based models can also be used to simulate habitat usage and movement of individual animals throughout a landscape (Tang and Bennett, 2010). For example, Rhodes et al. (2014) used an agent-based model to simulate koala movement through a landscape and assess how the survivability of Koalas was affected by traffic. Although such agent-based models are capable of capturing complex behaviors of organisms in realistic and heterogeneous landscapes (Wallentin, 2017), they are usually very data hungry and laborious to program and parameterize. The latter becomes problematic when one has to setup models for multiple species (see section Recommendations for Models of Coupled Settlement and Habitat Networks). An alternative to agent-based models of habitat networks are aggregated network-based models (Urban et al., 2009; Galpern et al., 2011). In these models, discrete patches of habitat need to be delineated to form the nodes in the habitat network, which is a simplification of the heterogeneous landscapes that can be used in agent-based models. The edges in the network can be weighted based on, for instance, the permeability of the matrix or on species characteristics (e.g., dispersal kernels). From these network-based models a variety of network measures can be calculated that express the importance of nodes or edges in the network or the connectedness of the network as a whole (Rayfield et al., 2011). These network measures can be used to predict the occurrence of species in habitat patches (e.g., Pereira et al., 2011) or the diversity in certain groups of species (e.g., for amphibians; Ribeiro et al., 2011). Both agent-based and network-based models require information on habitat and movement characteristics of (groups of) species. Although it is laborious to collect such data in the field for multiple species, trait databases that compile such data for certain taxa are increasingly being developed (e.g., for amphibeans; Trochet et al., 2014).
Both agent-based and network-based models can thus capture the effect of the structural properties of habitat networks on its functional properties. However, the opposite effect (i.e., functional on structural properties) remains difficult to model. In the relatively young field of study of ecosystem engineers, some models have been developed, but most are mainly conceptual and not operative (Wright and Jones, 2006; Hastings et al., 2007). The limited number of general models of ecosystem engineers currently reduces the possibilities to capture the feedback of functional on structural habitat network properties.
Coupling of Settlement and Habitat Networks
The actual coupling of the spatially-explicit settlement and habitat network models can be achieved by ensuring that output variables from the settlement network model function as the input variables for the habitat network model and vice versa. Edge weights in habitat networks can include the presence of transportation infrastructure (Pereira et al., 2011) or traffic intensity on roads (Scolozzi and Geneletti, 2012). For instance, in Van Strien and Grêt-Regamey (2016), traffic volumes were output of the settlement network model. These traffic volumes were subsequently input variables for the habitat network model and used to calculate habitat connectivity for several animal species. Modeling the effect of landscape and human mobility changes on habitat connectivity can be operationalized with resistance surfaces (Zeller et al., 2012; Cushman et al., 2014). Although these surfaces are usually static, they can also be modeled in a dynamic way so that they react to changes in the settlement network (e.g., Van Strien and Grêt-Regamey, 2016). The influence of habitat networks on settlement networks can be implemented in a similar way: output of the habitat network models (e.g., the loss of a species or a reduction in biodiversity) can trigger certain nature conservation measures, which are simulated by adjusting input variables of the settlement network model (e.g., density of housing or capacity of certain roads). By iteratively performing this information exchange between the settlement network and habitat network models, the dynamics of this social-ecological system can be simulated over time.
A challenge in simulating such time series are the differences in temporal scales of the processes taking place in the networks. For example, settlement development takes place at a much slower pace than changes to human travel behavior (Wegener and Fürst, 1999; Levinson, 2008). This means that a conservation measure like traffic calming will have an immediate effect on the traffic volumes in and around the traffic calmed areas, but that the resulting changes to settlement sizes or human population densities will only become visible over a longer time period. Such temporal lags should be considered when determining the period over which the dynamics in coupled settlement and habitat networks are simulated.
Applications of Coupled Settlement and Habitat Network Models for Biodiversity Conservation
Once a model of coupled settlement and habitat networks has been developed, we envisage several potential applications of such models to benefit biodiversity conservation. Our overview of potential applications of coupled settlement and habitat network models is not exhaustive, but is illustrative of the breadth or research topics in which such models can be applied.
Complex Networks Theory
Models of coupled settlement and habitat networks can draw from and contribute to the rapidly increasing fundamental research on complex networks. Many complex networks are represented as coupled networks (also called multiplex, multi-layered or interdependent networks or network of networks; Gao et al., 2014). In coupled networks, Buldyrev et al. (2010) have shown that small changes in one network can bring about a cascade of changes in both networks leading to abrupt fragmentation of the coupled system. Coupled spatial networks, such as coupled settlement and habitat networks, appear especially sensitive to small changes in either of the networks (Bashan et al., 2013). Such abrupt changes usually only take place beyond certain thresholds. These thresholds are influenced by properties of the coupled spatial networks, such as the fraction of interdependent nodes (Bashan et al., 2013) or the maximum geographic distance between nodes (Danziger et al., 2014). Identification of such thresholds and associated network properties is thus very important for vulnerability assessments of coupled networks, such as coupled settlement and habitat networks. However, knowledge of many theoretical studies on coupled spatial networks is not directly transferable to coupled settlement and habitat networks, as the nature of the dependencies between the coupled networks is different. In many studies, the networks are coupled by dependencies between spatially coinciding nodes in either networks (e.g., Buldyrev et al., 2010; Bashan et al., 2013; Danziger et al., 2014). In coupled settlement and habitat networks, the nodes are usually not spatially coinciding (i.e., settlements and habitats are spatially separated) and the dependencies between the networks are not only between nodes, but also between edges (e.g., roads intersecting animal movement paths) and between nodes and edges (e.g., habitat quality affected by traffic; Van Strien and Grêt-Regamey, 2016). Models of coupled settlement and habitat networks, in which these dependencies are incorporated, could thus lead to new insights in the behavior of coupled spatial networks and could potentially be used to assess the threat of rapid biodiversity loss in coupled settlement and habitat networks.
Resilience of Coupled Settlement and Habitat Networks
Analysis of the temporal dynamics in coupled settlement and habitat networks can also be used to estimate the resilience of this social-ecological system and all of its components (including biodiversity; Folke, 2006). The concept of system resilience was coined by Holling (1973, p. 17), who applied it to ecological systems and defined it as “a measure of the ability of these systems to absorb changes of state variables, driving variables, and parameters, and still persist.” Later, the concept was introduced to social-ecological systems (a history of the concept is described in Folke, 2006). Nowadays there are many definitions of resilience as well as approaches to assess the resilience of a system (Hosseini et al., 2016). Resilience has been the focus of studies on habitat networks (e.g., Uden et al., 2014) as well as on settlement networks (e.g., Ip and Wang, 2011) and recently has also been introduced to complex network theory (e.g., Gao et al., 2016). Models of coupled settlement and habitat networks can potentially be used to determine how the system recovers after sudden exogenous or endogenous disruptions and thereby assess system resilience.
Models of coupled settlement and habitat networks can also be used to aid practitioners in adapting their management strategies or transforming their system to ensure the resilience in real settlement and habitat networks (Levin et al., 2013). Rapidly changing environment and land-use has led to the realization that habitat networks should not be managed as static entities, but as dynamic systems that are constantly changing (Van Teeffelen et al., 2012). For this reason, “adaptive governance” is often propagated (Chaffin et al., 2014). For adaptive governance, a broad range of management options should be kept open to be able to react to unexpected events and changing conditions. By analysing different socio-economic, environmental or demographic development scenarios, models of coupled settlement and habitat networks can help identifying management options that could become necessary in the future. These analyses can give insights into the overall resilience of the system of coupled settlement and habitat networks and shed light on the long-term survivability of specific species or biodiversity in general.
Conservation, Transport, and Urban Planning
Models of coupled settlement and habitat networks can also be used to aid conservation, transport and urban planning. In habitat networks, the importance of nodes or edges for network connectivity can be assessed (Galpern et al., 2011). Comparable analyses can also be performed in settlement networks (e.g., Jenelius and Mattsson, 2015). These analyses are usually performed with node or edge removal experiments. Although such experiments can be applied for the analysis of resilience in networks (see section Resilience of Coupled Settlement and Habitat Networks), they can also serve a more applied goal: to prioritize spatial planning. For instance, Pereira et al. (2017) constructed habitat networks for 20 bird species in North-East Spain and ranked habitat patches based on their importance for network connectivity in order to prioritize nature conservation actions. With models of coupled settlement and habitat networks, the importance of nodes or edges in either network can be assessed based on current or forecasted changes in the other network. For example, by assessing which edges in habitat networks are most affected by current or future traffic on intersecting roads, optimal locations for roadkill mitigation measures can be identified (Loro et al., 2015; Mimet et al., 2016; Rytwinski et al., 2016). Especially when such mitigation measures can have an effect on traffic flows, such as traffic calming (Van Langevelde and Jaarsma, 2009), is it important to not only assess the effects of the measure on the habitat network but also its effect on the settlement network. Another example of a conservation and urban planning measure that can potentially have effects on both the settlement as well as the habitat networks is urban densification, which is promoted for reducing the urban land consumption and commute distances (Gaigné et al., 2012). However, on the long term urban densification can bring about significant changes in the settlement network, which can have unforeseen negative environmental effects (Gaigné et al., 2012). Models of coupled settlement and habitat networks could help identifying these negative effects and adapt conservation, transport and urban planning accordingly.
Conclusions
In this article, we present coupled settlement and habitat networks as a social-ecological system and promote the development of models of such coupled networks to generate new insights and effective policy for biodiversity conservation. In our conceptual framework of models of coupled settlement and habitat networks, we have described the network-internal interactions as well as the influences between the networks. In summary, there are strong two-way interactions between the structural properties of a settlement network and its functional properties (Figure 1). Whereas, in habitat networks, structural properties mainly influence their functional properties (Figure 1). Through ecosystem engineer species, a habitat network's functional properties in some cases can also influence its structural properties. Changes to settlement networks can have direct as well as indirect influences on the structural and functional properties of habitat networks (Figure 1). For both settlements and roads, these influences are not limited to only the location of a settlement or road, but also radiate out into their surrounding landscape. Direct influences of habitat networks on settlement networks are usually limited. More prevalent are indirect influence caused by nature conservation measures that are implemented to maintain or enhance species or biodiversity. The governance system to which these conservation measures belong, is influenced by the normative lens through which society views human-nature relationships (Figure 1).
Due to these numerous network-internal interactions in settlement or habitat networks and the between-network influences, it is difficult to assess whether changes to any part of either network can result in positive or negative feedbacks leading to complex system behavior. Coupling models of settlement networks and habitat networks in a dynamic way, can reveal such complexities that cannot be found when only parts of the social-ecological system are studied. These models do not have to be built from scratch, but can be based upon existing models of settlement or habitat networks, of which we have presented some in this article (e.g., habitat network models or land-use transport interaction models). Nevertheless, new data may have to be collected to parameterize some of the relationships between or within the networks. We see several potential applications of models of coupled settlement and habitat networks for biodiversity conservation, ranging from fundamental research on desirable network properties to more applied assessments of weaknesses in existing settlement or habitat networks for conservation planning purposes. We envisage that integrated models of coupled settlement and habitat networks can contribute to a world in which both human well-being is ensured and biodiversity is maintained.
Author Contributions
The conceptual model presented in this manuscript is the result of a series of discussions and meetings that took place as part of the CHECNET (Coupling human and ecological networks for sustainable landscape and transport planning) project. All authors are part of this project, have participated in the meetings and discussions and have contributed to the formulation of the conceptual model. MvS wrote a first draft of the manuscript, which was reviewed by KA, ID, AG, AG-R, AK-M, DO-R, and RH.
Funding
This study was part of the CHECNET project financed by the Swiss National Science Foundation (Grant nr. CR30I3_159250).
Conflict of Interest Statement
The authors declare that the research was conducted in the absence of any commercial or financial relationships that could be construed as a potential conflict of interest.
Acknowledgments
We thank two reviewers for their helpful comments on earlier versions of the manuscript.
References
Acheampong, R. A., and Silva, E. (2015). Land use–transport interaction modeling: a review of the literature and future research directions. J. Transp. Land Use 8, 11–38. doi: 10.5198/jtlu.2015.806
Adriaensen, F., Chardon, J. P., De Blust, G., Swinnen, E., Villalba, S., Gulinck, H., et al. (2003). The application of “least-cost” modelling as a functional landscape model. Landsc. Urban Plan. 64, 233–247. doi: 10.1016/S0169-2046(02)00242-6
Angeli, D., Ferrell, J. E., and Sontag, E. D. (2004). Detection of multistability, bifurcations, and hysteresis in a large class of biological positive-feedback systems. Proc. Natl. Acad. Sci. U.S.A. 101, 1822–1827. doi: 10.1073/pnas.0308265100
Antrop, M. (2000). Changing patterns in the urbanized countryside of Western Europe. Landsc. Ecol. 15, 257–270. doi: 10.1023/A:1008151109252
Axhausen, K. W. (2007). Activity spaces, biographies, social networks and their welfare gains and externalities: some hypotheses and empirical results. Mobilities 2, 15–36. doi: 10.1080/17450100601106203
Axhausen, K. W., Froelich, P., and Tschopp, M. (2011). Changes in Swiss accessibility since 1850. Res. Transp. Econ. 31, 72–80. doi: 10.1016/j.retrec.2010.11.010
Badoe, D. A., and Miller, E. J. (2000). Transportation–land-use interaction: empirical findings in North America, and their implications for modeling. Transp. Res. Part D 5, 235–263. doi: 10.1016/S1361-9209(99)00036-X
Barrett, C. B., Bulte, E. H., Ferraro, P., and Wunder, S. (2013). “Economic instruments for nature conservation,” in Key Topics in Conservation Biology, Vol 2, eds D. W. Macdonald and K. J. Willis (Chichester: Wiley), 59–73.
Bashan, A., Berezin, Y., Buldyrev, S. V., and Havlin, S. (2013). The extreme vulnerability of interdependent spatially embedded networks. Nat. Phys. 9, 667–672. doi: 10.1038/nphys2727
Beatley, T. (2011). Biophilic Cities: Integrating Nature Into Urban Design and Planning. Washington, DC: Island Press.
Benítez-López, A., Alkemade, R., and Verweij, P. A. (2010). The impacts of roads and other infrastructure on mammal and bird populations: a meta-analysis. Biol. Conserv. 143, 1307–1316. doi: 10.1016/j.biocon.2010.02.009
Bennett, V. J. (2017). Effects of road density and pattern on the conservation of species and biodiversity. Curr. Landsc. Ecol. Rep. 2, 1–11. doi: 10.1007/s40823-017-0020-6
Bennie, J., Davies, T. W., Cruse, D., and Gaston, K. J. (2016). Ecological effects of artificial light at night on wild plants. J. Ecol. 104, 611–620. doi: 10.1111/1365-2745.12551
Berkes, F., and Folke, C. (2000). “Linking social and ecological systems for resilience and sustainability,” in Linking Social and Ecological Systems: Management Practices and Social Mechanisms for Building Resilience, eds F. Berkes and C. Folke (Cambridge: Cambridge University Press), 1–25.
Bierwagen, B. G. (2007). Connectivity in urbanizing landscapes: the importance of habitat configuration, urban area size, and dispersal. Urban Ecosyst. 10, 29–42. doi: 10.1007/s11252-006-0011-6
Bodenmann, B. R., Sanchez, B., Bode, J., Zeiler, A., Kuliowsky, M., Furtak, P., et al. (2014). “FaLC land-use and transport interaction model for Switzerland: first results,” in Proceedings of the 13th Swiss Transport Research Conference (Ascona: STRC), 1–29.
Brashares, J. S., Arcese, P., and Sam, M. K. (2001). Human demography and reserve size predict wildlife extinction in West Africa. Proc. R. Soc. Lond. Ser B 268, 2473–2478. doi: 10.1098/rspb.2001.1815
Buhl, J., Gautrais, J., Reeves, N., Solé, R. V., Valverde, S., Kuntz, P., et al. (2006). Topological patterns in street networks of self-organized urban settlements. Eur. Phys. J. B 49, 513–522. doi: 10.1140/epjb/e2006-00085-1
Buldyrev, S. V., Parshani, R., Paul, G., Stanley, H. E., and Havlin, S. (2010). Catastrophic cascade of failures in interdependent networks. Nature 464, 1025–1028. doi: 10.1038/nature,08932
CBD (2010). Linking Biodiversity Conservation and Poverty Alleviation: A State of Knowledge Review. Montreal: Secretariat of the Convention on Biological Diversity.
Ceballos, G., Ehrlich, P. R., Barnosky, A. D., García, A., Pringle, R. M., and Palmer, T. M. (2015). Accelerated modern human–induced species losses: entering the sixth mass extinction. Sci. Adv. 1:e1400253. doi: 10.1126/sciadv.1400253
Chaffin, B. C., Gosnell, H., and Cosens, B. A. (2014). A decade of adaptive governance scholarship: synthesis and future directions. Ecol. Soc. 19:56. doi: 10.5751/ES-06824-190356
Charry, B., and Jones, J. (2009). “Traffic volume as a primary road characteristic impacting wildlife: a tool for land use and transportation planning,” in Proceedings of the 2009 International Conference on Ecology and Transportation, eds P. J. Wagner, D. Nelson, and E. Murray (Raleigh, NC: Center for Transportation and the Environment, North Carolina State University), 159–172.
Chi, G. (2010). The impacts of highway expansion on population change: an integrated spatial approach. Rural Sociol. 75, 58–89. doi: 10.1111/j.1549-0831.2009.00003.x
Coffin, A. W. (2007). From roadkill to road ecology: a review of the ecological effects of roads. J. Transp. Geogr. 15, 396–406. doi: 10.1016/j.jtrangeo.2006.11.006
Concepción, E. D., Moretti, M., Altermatt, F., Nobis, M. P., and Obrist, M. K. (2015). Impacts of urbanisation on biodiversity: the role of species mobility, degree of specialisation and spatial scale. Oikos 124, 1571–1582. doi: 10.1111/oik.02166
Corlatti, L., Hackländer, K., and Frey-Roos, F. (2009). Ability of wildlife overpasses to provide connectivity and prevent genetic isolation. Conserv. Biol. 23, 548–556. doi: 10.1111/j.1523-1739.2008.01162.x
Crooks, K. R., and Sanjayan, M. (2006). “Connectivity conservation: maintaining connections for nature,” in Connectivity Conservation, eds K. R. Crooks and M. Sanjayan (New York, NY: Cambridge University Press), 1–20.
Cushman, S., Lewis, J., and Landguth, E. (2014). Why did the bear cross the road? Comparing the performance of multiple resistance surfaces and connectivity modeling methods. Diversity 6:844. doi: 10.3390/d6040844
Danziger, M. M., Bashan, A., Berezin, Y., and Havlin, S. (2014). Percolation and cascade dynamics of spatial networks with partial dependency. J. Complex Netw. 2, 460–474. doi: 10.1093/comnet/cnu020
De Bello, F., Lavorel, S., Díaz, S., Harrington, R., Cornelissen, J. H. C., Bardgett, R. D., et al. (2010). Towards an assessment of multiple ecosystem processes and services via functional traits. Biodivers. Conserv. 19, 2873–2893. doi: 10.1007/s10531-010-9850-9
De Montis, A., Barthélemy, M., Chessa, A., and Vespignani, A. (2007). The structure of interurban traffic: a weighted network analysis. Environ. Plan. B 34, 905–924. doi: 10.1068/b32128
Díaz, S., Fargione, J., Chapin, F. S. III, and Tilman, D. (2006). Biodiversity loss threatens human well-being. PLoS Biol. 4:e277. doi: 10.1371/journal.pbio.0040277
Díaz, S., Lavorel, S., de Bello, F., Quétier, F., Grigulis, K., and Robson, T. M. (2007). Incorporating plant functional diversity effects in ecosystem service assessments. Proc. Natl. Acad. Sci. U.S.A. 104, 20684–20689. doi: 10.1073/pnas.0704716104
Dulac, J. (2013). Global Land Transport Infrastructure Requirements - Estimating Road and Railway Infrastructure Capacity and Costs to 2050. Paris: International Energy Agency.
Ercoskun, O. Y. (2012). Green and Ecological Technologies for Urban Planning: Creating Smart Cities. Hershey, PA: Information Science Reference.
Fahrig, L. (2003). Effects of habitat fragmentation on biodiversity. Ann. Rev. Ecol. Evol. Syst. 34, 487–515. doi: 10.1146/annurev.ecolsys.34.011802.132419
Fahrig, L. (2013). Rethinking patch size and isolation effects: the habitat amount hypothesis. J. Biogeogr. 40, 1649–1663. doi: 10.1111/jbi.12130
Fahrig, L., and Rytwinski, T. (2009). Effects of roads on animal abundance: an empirical review and synthesis. Ecol. Soc. 14:21. doi: 10.5751/ES-02815-140121
Fernandez, L. E., Brown, D. G., Marans, R. W., and Nassauer, J. I. (2005). Characterizing location preferences in an exurban population: implications for agent-based modeling. Environ. Plan. B 32, 799–820. doi: 10.1068/b3071
Fleishman, E., Ray, C., Sjögren-Gulve, P., Boggs, C. L., and Murphy, D. D. (2002). Assessing the roles of patch quality, area, and isolation in predicting metapopulation dynamics. Conserv. Biol. 16, 706–716. doi: 10.1046/j.1523-1739.2002.00539.x
Folke, C. (2006). Resilience: the emergence of a perspective for social–ecological systems analyses. Glob. Environ. Change 16, 253–267. doi: 10.1016/j.gloenvcha.2006.04.002
Forman, R. T. T. (2000). Estimate of the area affected ecologically by the road system in the United States. Conserv. Biol. 14, 31–35. doi: 10.1046/j.1523-1739.2000.99299.x
Forman, R. T. T., Sperling, D., Bissonette, J. A., Clevenger, A. P., Cutshall, C. D., Dale, V. H., et al. (2003). Road Ecology. Science and Solutions. Washington, DC: Island Press.
Gaigné, C., Riou, S., and Thisse, J.-F. (2012). Are compact cities environmentally friendly? J. Urban Econ. 72, 123–136. doi: 10.1016/j.jue.2012.04.001
Galpern, P., Manseau, M., and Fall, A. (2011). Patch-based graphs of landscape connectivity: a guide to construction, analysis and application for conservation. Biol. Conserv. 144, 44–55. doi: 10.1016/j.biocon.2010.09.002
Gao, J., Barzel, B., and Barabási, A. L. (2016). Universal resilience patterns in complex networks. Nature 530, 307–312. doi: 10.1038/nature16948
Gao, J., Li, D., and Havlin, S. (2014). From a single network to a network of networks. Natl. Sci. Rev. 1, 346–356. doi: 10.1093/nsr/nwu020
Geoghegan, J. (2002). The value of open spaces in residential land use. Land Use Policy 19, 91–98. doi: 10.1016/S0264-8377(01)00040-0
Goodwin, P. (1998). “Unintended effects of transport policies,” in Transport Policy and the Environment, ed D. Banister (New York, NY: Routledge), 114–130.
Graham-Rowe, E., Skippon, S., Gardner, B., and Abraham, C. (2011). Can we reduce car use and, if so, how? A review of available evidence. Transp. Res. Part A 45, 401–418. doi: 10.1016/j.tra.2011.02.001
Güneralp, B., McDonald, R. I., Fragkias, M., Goodness, J., Marcotullio, P. J., and Seto, K. C. (2013). “Urbanization forecasts, effects on land use, biodiversity, and ecosystem services,” in Urbanization, Biodiversity and Ecosystem Services: Challenges and Opportunities: A Global Assessment, eds T. Elmqvist, M. Fragkias, J. Goodness, B. Güneralp, P. J. Marcotullio, R. I. McDonald, S. Parnell, M. Schewenius, M. Sendstad, K. C. Seto, and C. Wilkinson (Dordrecht: Springer), 437–452.
Hansen, A. J., Knight, R. L., Marzluff, J. M., Powell, S., Brown, K., Gude, P. H., et al. (2005). Effects of exurban development on biodiversity: patterns, mechanisms, and research needs. Ecol. Appl. 15, 1893–1905. doi: 10.1890/05-5221
Hanski, I. (2015). Habitat fragmentation and species richness. J. Biogeogr. 42, 989–993. doi: 10.1111/jbi.12478
Hastings, A., Byers, J. E., Crooks, J. A., Cuddington, K., Jones, C. G., Lambrinos, J. G., et al. (2007). Ecosystem engineering in space and time. Ecol. Lett. 10, 153–164. doi: 10.1111/j.1461-0248.2006.00997.x
Hector, A., and Bagchi, R. (2007). Biodiversity and ecosystem multifunctionality. Nature 448, 188–190. doi: 10.1038/nature05947
Hirschfeld, Y. (2006). The crisis of the sixth century: climatic change, natural disasters and the plague. Mediter. Archaeol. Archaeomet. 6, 19–32. Available online at: http://maajournal.com/Issues/2006/Vol06-1/Full2.pdf
Hodgson, J. A., Moilanen, A., Wintle, B. A., and Thomas, C. D. (2011). Habitat area, quality and connectivity: striking the balance for efficient conservation. J. Appl. Ecol. 48, 148–152. doi: 10.1111/j.1365-2664.2010.01919.x
Holderegger, R., and Di Giulio, M. (2010). The genetic effects of roads: a review of empirical evidence. Basic Appl. Ecol. 11, 522–531. doi: 10.1016/j.baae.2010.06.006
Holderegger, R., and Wagner, H. H. (2008). Landscape genetics. BioScience 58, 199–207. doi: 10.1641/B580306
Holling, C. S. (1973). Resilience and stability of ecological systems. Annu. Rev. Ecol. Syst. 4, 1–23. doi: 10.1146/annurev.es.04.110173.000245
Holmes, S. M., Baden, A. L., Brenneman, R. A., Engberg, S. E., Louis, E. E., and Johnson, S. E. (2013). Patch size and isolation influence genetic patterns in black-and-white ruffed lemur (Varecia variegata) populations. Conserv. Genetics 14, 615–624. doi: 10.1007/s10592-013-0455-1
Hosseini, S., Barker, K., and Ramirez-Marquez, J. E. (2016). A review of definitions and measures of system resilience. Reliabil. Engineer. Syst. Safety 145, 47–61. doi: 10.1016/j.ress.2015.08.006
Huijser, M. P., Duffield, J. W., Clevenger, A. P., Ament, R. J., and McGowen, P. T. (2009). Cost-benefit analyses of mitigation measures aimed at reducing collisions with large ungulates in the United States and Canada: a decision support tool. Ecol. Soc. 14:15. doi: 10.5751/ES-03000-140215
Hulme, P. E. (2009). Trade, transport and trouble: managing invasive species pathways in an era of globalization. J. Appl. Ecol. 46, 10–18. doi: 10.1111/j.1365-2664.2008.01600.x
Iacono, M., and Levinson, D. (2016). Mutual causality in road network growth and economic development. Transp. Policy 45, 209–217. doi: 10.1016/j.tranpol.2015.06.005
Ibisch, P. L., Hoffmann, M. T., Kreft, S., Pe'er, G., Kati, V., Biber-Freudenberger, L., et al. (2016). A global map of roadless areas and their conservation status. Science 354, 1423–1427. doi: 10.1126/science.aaf7166
Ip, W. H., and Wang, D. (2011). Resilience and friability of transportation networks: evaluation, analysis and optimization. IEEE Syst. J. 5, 189–198. doi: 10.1109/JSYST.2010.2096670
Isbell, F., Calcagno, V., Hector, A., Connolly, J., Harpole, W. S., Reich, P. B., et al. (2011). High plant diversity is needed to maintain ecosystem services. Nature 477, 199–202. doi: 10.1038/nature10282
Israel, E., and Cohen-Blankshtain, G. (2010). Testing the decentralization effects of rail systems: empirical findings from Israel. Transp. Res. Part A 44, 523–536. doi: 10.1016/j.tra.2010.03.021
Jabareen, Y. R. (2006). Sustainable urban forms: their typologies, models, and concepts. J. Plan. Educ. Res. 26, 38–52. doi: 10.1177/0739456X05285119
Jaeger, J. A. G. (2007). “Effects of the configuration of road networks on landscape connectivity,” in Proceedings of the 2007 International Conference on Ecology and Transportation, eds. C. L. Irwin, D. Nelson, and K. P. McDermott (Raleigh, NC: Center for Transportation and the Environment, North Carolina State University), 267–280.
Jaeger, J. A. G., Bowman, J., Brennan, J., Fahrig, L., Bert, D., Bouchard, J., et al. (2005). Predicting when animal populations are at risk from roads: an interactive model of road avoidance behavior. Ecol. Modell. 185, 329–348. doi: 10.1016/j.ecolmodel.2004.12.015
Jaeger, J. A. G., Fahrig, L., and Ewald, K. C. (2006). “Does the configuration of road networks influence the degree to which roads affect wildlife populations?” in Proceedings of the 2005 International Conference on Ecology and Transportation, eds C. L. Irwin, P. Garrett, and K. P. McDermott (Raleigh, NC: Center for Transportation and the Environment, North Carolina State University), 151–163.
Janssen, M. A., Bodin, Ö., Anderies, J. M., Elmqvist, T., Ernstson, H., McAllister, R. R. J., et al. (2006). Toward a network perspective of the study of resilience in social-ecological systems. Ecol. Soc. 11:15. doi: 10.5751/ES-01462-110115
Jenelius, E., and Mattsson, L.-G. (2015). Road network vulnerability analysis: conceptualization, implementation and application. Comput. Environ. Urban Syst. 49, 136–147. doi: 10.1016/j.compenvurbsys.2014.02.003
Jones, C. G., Lawton, J. H., and Shachak, M. (1994). Organisms as ecosystem engineers. Oikos 69, 373–386. doi: 10.2307/3545850
Keller, V., Bekker, G. J., Cuperus, R., Folkson, L., Rosell, C., and Trocmé, M. (2003). “Avoidance, mitigation, and compensatory measures and their maintenance,” in COST 341 - Habitat Fragmentation Due to Transportation Infrastructure: The European Review, eds M. Trocmé, S. Cahill, J. G. de Vries, H. Farrall, L. Folkeson, G. Fry, C. Hicks, and J. Peymen (Luxembourg: Publications Office of the European Union), 129–173.
Kooiman, J. A. N., and Jentoft, S. (2009). Meta-governance: values, norms and principles, and the making of hard choices. Public Adm. 87, 818–836. doi: 10.1111/j.1467-9299.2009.01780.x
Kool, J., Moilanen, A., and Treml, E. (2013). Population connectivity: recent advances and new perspectives. Landsc. Ecol. 28, 165–185. doi: 10.1007/s10980-012-9819-z
Kreibich, V. (1978). The successful transportation system and the regional planning problem: an evaluation of the Munich rapid transit system in the context of urban and regional planning policy. Transportation 7, 137–145. doi: 10.1007/BF00184636
Lakshmanan, T. R. (2011). The broader economic consequences of transport infrastructure investments. J. Trans. Geogr. 19, 1–12. doi: 10.1016/j.jtrangeo.2010.01.001
Lämmer, S., Gehlsen, B., and Helbing, D. (2006). Scaling laws in the spatial structure of urban road networks. Physica A 363, 89–95. doi: 10.1016/j.physa.2006.01.051
Lechner, A. M., Sprod, D., Carter, O., and Lefroy, E. C. (2017). Characterising landscape connectivity for conservation planning using a dispersal guild approach. Landsc. Ecol. 32, 99–113. doi: 10.1007/s10980-016-0431-5
Levin, S., Xepapadeas, T., Crépin, A.-S., Norberg, J., de Zeeuw, A., Folke, C., et al. (2013). Social-ecological systems as complex adaptive systems: modeling and policy implications. Environ. Dev. Econ. 18, 111–132. doi: 10.1017/S1355770X12000460
Levins, R. (1969). Some demographic and genetic consequences of environmental heterogeneity for biological control. Bull. Entomol. Soc. Am. 15, 237–240. doi: 10.1093/besa/15.3.237
Levinson, D. (2008). Density and dispersion: the co-development of land use and rail in London. J. Econ. Geogr. 8, 55–77. doi: 10.1093/jeg/lbm038
Liu, J., Dietz, T., Carpenter, S. R., Alberti, M., Folke, C., Moran, E., et al. (2007). Complexity of coupled human and natural systems. Science 317, 1513–1516. doi: 10.1126/science.1144004
Loro, M., Ortega, E., Arce, R. M., and Geneletti, D. (2015). Ecological connectivity analysis to reduce the barrier effect of roads. An innovative graph-theory approach to define wildlife corridors with multiple paths and without bottlenecks. Landsc. Urban Plan. 139, 149–162. doi: 10.1016/j.landurbplan.2015.03.006
Luck, G. W. (2007). A review of the relationships between human population density and biodiversity. Biol. Rev. 82, 607–645. doi: 10.1111/j.1469-185X.2007.00028.x
Luck, G. W., Harrington, R., Harrison, P. A., Kremen, C., Berry, P. M., Bugter, R., et al. (2009). Quantifying the contribution of organisms to the provision of ecosystem services. BioScience 59, 223–235. doi: 10.1525/bio.2009.59.3.7
Luck, G. W., and Smallbone, L. T. (2010). “Species diversity and urbanisation: patterns, drivers and implications,” in Urban Ecology, ed. K. J. Gaston (Cambridge: Cambridge University Press), 88–119.
Lutzenhiser, M., and Netusil, N. R. (2001). The effect of open spaces on a home's sale price. Contemp. Econ. Policy 19, 291–298. doi: 10.1093/cep/19.3.291
MacArthur, R. H., and Wilson, E. O. (1967). The Theory of Island Biogeography. Princeton: Princeton University Press.
MacDougall, A. S., McCann, K. S., Gellner, G., and Turkington, R. (2013). Diversity loss with persistent human disturbance increases vulnerability to ecosystem collapse. Nature 494, 86–89. doi: 10.1038/nature11869
Mace, G. M., Norris, K., and Fitter, A. H. (2012). Biodiversity and ecosystem services: a multilayered relationship. Trends Ecol. Evol. 27, 19–26. doi: 10.1016/j.tree.2011.08.006
McDonald, R. I., Marcotullio, P. J., and Güneralp, B. (2013). “Urbanization and global trends in biodiversity and ecosystem services,” in Urbanization, Biodiversity and Ecosystem Services: Challenges and Opportunities: A Global Assessment, eds T. Elmqvist, M. Fragkias, J. Goodness, B. Güneralp, P. J. Marcotullio, R. I. McDonald, S. Parnell, M. Schewenius, M. Sendstad, K. C. Seto, and C. Wilkinson (Dordrecht: Springer), 31–52.
McDonnell, M. J., and Hahs, A. K. (2008). The use of gradient analysis studies in advancing our understanding of the ecology of urbanizing landscapes: current status and future directions. Landsc. Ecol. 23, 1143–1155. doi: 10.1007/s10980-008-9253-4
McKee, J., Chambers, E., and Guseman, J. (2013). Human population density and growth validated as extinction threats to mammal and bird species. Hum. Ecol. 41, 773–778. doi: 10.1007/s10745-013-9586-8
McKinney, M. L. (2002). Urbanization, biodiversity, and conservation. BioScience 52, 883–890. doi: 10.1641/0006-3568(2002)052[0883:UBAC]2.0.CO;2
McKinney, M. L. (2008). Effects of urbanization on species richness: a review of plants and animals. Urban Ecosyst. 11, 161–176. doi: 10.1007/s11252-007-0045-4
McKinney, M. L. (2010). “Urban futures,” in Urban Ecology, ed K. J. Gaston (Cambridge, UK: Cambridge University Press), 287–308.
McLeman, R. A. (2011). Settlement abandonment in the context of global environmental change. Glob. Environ. Change 21, S108–S120. doi: 10.1016/j.gloenvcha.2011.08.004
McRae, B. H. (2006). Isolation by resistance. Evolution 60, 1551–1561. doi: 10.1111/j.0014-3820.2006.tb00500.x
Meister, K., Balmer, M., Ciari, F., Horni, A., Rieser, M., Waraich, R. A., et al. (2010). Large-Scale Agent-Based Travel Demand Optimization Applied to Switzerland, Including Mode Choice. Working paper. Zurich: Institute for Transport Planning and Systems; ETH Zurich.
Millennium Ecosystem Assessment (2005). Millennium Ecosystem Assessment. Washington, DC: Island Press.
Milner-Gulland, E. J. (2012). Interactions between human behaviour and ecological systems. Philos. Trans. R. Soc. B 367, 270–278. doi: 10.1098/rstb.2011.0175
Mimet, A., Clauzel, C., and Foltête, J.-C. (2016). Locating wildlife crossings for multispecies connectivity across linear infrastructures. Landsc. Ecol. 31, 1955–1973. doi: 10.1007/s10980-016-0373-y
Mollanejad, M., and Zhang, L. (2014). Incorporating spatial equity into interurban road network design. J. Transp. Geogr. 39, 156–164. doi: 10.1016/j.jtrangeo.2014.06.023
Newbold, T., Hudson, L. N., Hill, S. L., Contu, S., Lysenko, I., Senior, R. A., et al. (2015). Global effects of land use on local terrestrial biodiversity. Nature 520, 45–50. doi: 10.1038/nature14324
Newman, M. E. J. (2003). The structure and function of complex networks. Siam Rev. 45, 167–256. doi: 10.1137/S003614450342480
Newsome, D., Moore, S. A., and Kingston, R. (2013). Natural Area Tourism: Ecology, Impacts and Management. Bristol: Channel View Publications.
Nuissl, H., and Siedentop, S. (2012). “Landscape planning for minimizing land consumption,” in Encyclopedia of Sustainability Science and Technology, ed R. A. Meyers (New York, NY: Springer), 5785–5817.
Ostrom, E. (2009). A general framework for analyzing sustainability of social-ecological systems. Science 325, 419–422. doi: 10.1126/science.1172133
Parks, S. A., and Harcourt, A. H. (2002). Reserve size, local human density, and mammalian extinctions in U.S. protected areas. Conserv. Biol. 16, 800–808. doi: 10.1046/j.1523-1739.2002.00288.x
Pascual-Hortal, L., and Saura, S. (2006). Comparison and development of new graph-based landscape connectivity indices: towards the priorization of habitat patches and corridors for conservation. Landsc. Ecol. 21, 959–967. doi: 10.1007/s10980-006-0013-z
Pautasso, M. (2007). Scale dependence of the correlation between human population presence and vertebrate and plant species richness. Ecol. Lett. 10, 16–24. doi: 10.1111/j.1461-0248.2006.00993.x
Pereira, J., Saura, S., and Jordán, F. (2017). Single-node vs. multi-node centrality in landscape graph analysis: key habitat patches and their protection for 20 bird species in NE Spain. Methods Ecol. Evol. 8, 1458–1467. doi: 10.1111/2041-210X.12783
Pereira, M., Segurado, P., and Neves, N. (2011). Using spatial network structure in landscape management and planning: a case study with pond turtles. Landsc. Urban Plan. 100, 67–76. doi: 10.1016/j.landurbplan.2010.11.009
Pickett, S. T. A., Cadenasso, M. L., Grove, J. M., Boone, C. G., Groffman, P. M., Irwin, E., et al. (2011). Urban ecological systems: scientific foundations and a decade of progress. J. Environ. Manage. 92, 331–362. doi: 10.1016/j.jenvman.2010.08.022
Pirard, R. (2012). Market-based instruments for biodiversity and ecosystem services: a lexicon. Environ. Sci. Policy 19–20, 59–68. doi: 10.1016/j.envsci.2012.02.001
Prevedello, J. A., and Vieira, M. V. (2010). Does the type of matrix matter? A quantitative review of the evidence. Biodiver. Conserv. 19, 1205–1223. doi: 10.1007/s10531-009-9750-z
Prigogine, I., and Stengers, I. (1984). Order Out of Chaos: Man's New Dialogue With Nature. Toronto, ON: Bantam Books.
Prugh, L. R., Hodges, K. E., Sinclair, A. R. E., and Brashares, J. S. (2008). Effect of habitat area and isolation on fragmented animal populations. Proc. Natl. Acad. Sci. U.S.A.105, 20770–20775. doi: 10.1073/pnas.0806080105
Pyšek, P. (1998). Alien and native species in Central European urban floras: a quantitative comparison. J. Biogeogr. 25, 155–163. doi: 10.1046/j.1365-2699.1998.251177.x
Ramalho, C. E., and Hobbs, R. J. (2012). Time for a change: dynamic urban ecology. Trends Ecol. Evol. 27, 179–188. doi: 10.1016/j.tree.2011.10.008
Ramirez-Reyes, C., Bateman, B. L., and Radeloff, V. C. (2016). Effects of habitat suitability and minimum patch size thresholds on the assessment of landscape connectivity for jaguars in the Sierra Gorda, Mexico. Biol. Conserv. 204, 296–305. doi: 10.1016/j.biocon.2016.10.020
Rayfield, B., Fortin, M. J., and Fall, A. (2011). Connectivity for conservation: a framework to classify network measures. Ecology 92, 847–858. doi: 10.1890/09-2190.1
Reed, S. E., Kretser, H. E., Glennon, M. J., Pejchar, L., and Merenlender, A. M. (2012). “Faunal biodiversity at the urban–rural interface: current knowledge, research priorities, and planning strategies,” in Urban–Rural Interfaces: Linking People and Nature, eds. D. N. Laband, B. G. Lockaby, and W. C. Zipperer (Madison, WI: American Society of Agronomy, Soil Science Society of America, Crop Science Society of America, Inc.), 99–114.
Rey Benayas, J. M., Martins, A., Nicolau, J. M., and Schulz, J. J. (2007). Abandonment of agricultural land: an overview of drivers and consequences. CAB Rev. 2, 1–14. doi: 10.1079/PAVSNNR20072057
Rhodes, J. R., Lunney, D., Callaghan, J., and McAlpine, C. A. (2014). A few large roads or many small ones? How to accommodate growth in vehicle numbers to minimise impacts on wildlife. PLoS ONE 9:e91093. doi: 10.1371/journal.pone.0091093
Ribeiro, R., Carretero, M., Sillero, N., Alarcos, G., Ortiz-Santaliestra, M., Lizana, M., et al. (2011). The pond network: can structural connectivity reflect on (amphibian) biodiversity patterns? Landsc. Ecol. 26, 673–682. doi: 10.1007/s10980-011-9592-4
Rytwinski, T., Soanes, K., Jaeger, J. A., Fahrig, L., Findlay, C. S., Houlahan, J., et al. (2016). How effective is road mitigation at reducing road-kill? A meta-analysis. PLoS ONE 11:e0166941. doi: 10.1371/journal.pone.0166941
Sachs, J., and Malaney, P. (2002). The economic and social burden of malaria. Nature 415, 680–685. doi: 10.1038/415680a
Salafsky, N., Salzer, D., Stattersfield, A. J., Hilton-Taylor, C., Neugarten, R., Butchart, S. H., et al. (2008). A standard lexicon for biodiversity conservation: unified classifications of threats and actions. Conserv. Biol. 22, 897–911. doi: 10.1111/j.1523-1739.2008.00937.x
Salon, D., Boarnet, M. G., Handy, S., Spears, S., and Tal, G. (2012). How do local actions affect VMT? A critical review of the empirical evidence. Transp. Res. Part D 17, 495–508. doi: 10.1016/j.trd.2012.05.006
Saura, S., and Pascual-Hortal, L. (2007). A new habitat availability index to integrate connectivity in landscape conservation planning: comparison with existing indices and application to a case study. Landsc. Urban Plan. 83, 91–103. doi: 10.1016/j.landurbplan.2007.03.005
Scheffer, M., Carpenter, S. R., Lenton, T. M., Bascompte, J., Brock, W., Dakos, V., et al. (2012). Anticipating critical transitions. Science 338, 344–348. doi: 10.1126/science.1225244
Scolozzi, R., and Geneletti, D. (2012). Assessing habitat connectivity for land-use planning: a method integrating landscape graphs and Delphi survey. J. Environ. Plan. Manag. 55, 813–830. doi: 10.1080/09640568.2011.628823
Seiler, A. (2003). “Effects of infrastructure on nature,” in COST 341 - Habitat Fragmentation due to Transportation Infrastructure: The European Review, eds M. Trocmé, S. Cahill, J. G. de Vries, H. Farrall, L. Folkeson, G. Fry, C. Hicks, and J. Peymen (Luxembourg: Publications Office of the European Union), 31–50.
Seto, K. C., Güneralp, B., and Hutyra, L. R. (2012). Global forecasts of urban expansion to 2030 and direct impacts on biodiversity and carbon pools. Proc. Natl. Acad. Sci. U.S.A. 109, 16083–16088. doi: 10.1073/pnas.1211658109
Spellerberg, I. A. N. (1998). Ecological effects of roads and traffic: a literature review. Glob. Ecol. Biogeogr. 7, 317–333. doi: 10.1046/j.1466-822x.1998.00308.x
Tang, W., and Bennett, D. A. (2010). Agent-based modeling of animal movement: a review. Geogr. Compass 4, 682–700. doi: 10.1111/j.1749-8198.2010.00337.x
Taylor, P. D., Fahrig, L., and With, K. A. (2006). “Landscape connectivity: a return to the basics,” in Connectivity Conservation, eds K. R. Crooks and M. Sanjayan (Cambridge: Cambridge University Press), 29–43.
Thomas, J. A., Bourn, N. A., Clarke, R. T., Stewart, K. E., Simcox, D. J., Pearman, G. S., et al. (2001). The quality and isolation of habitat patches both determine where butterflies persist in fragmented landscapes. Proc. R. Soc. Lond. Ser. B 268, 1791–1796. doi: 10.1098/rspb.2001.1693
Thornton, D. H., Branch, L. C., and Sunquist, M. E. (2011). The influence of landscape, patch, and within-patch factors on species presence and abundance: a review of focal patch studies. Landsc. Ecol. 26, 7–18. doi: 10.1007/s10980-010-9549-z
Tobias, S., Ströbele, M., Nobis, M., Obrist, M., Moretti, M., Hunziker, M., et al. (2016). Siedlungs- und Landschaftsentwicklung in Agglomerationsnahen Räumen. Merkblatt für die Praxis 56. Birmensdorf: Swiss Federal Research Institute WSL.
Todd, B. D., Luhring, T. M., Rothermel, B. B., and Gibbons, J. W. (2009). Effects of forest removal on amphibian migrations: implications for habitat and landscape connectivity. J. Appl. Ecol. 46, 554–561. doi: 10.1111/j.1365-2664.2009.01645.x
Todd, B. D., Rothermel, B. B., Reed, R. N., Luhring, T. M., Schlatter, K., Trenkamp, L., et al. (2008). Habitat alteration increases invasive fire ant abundance to the detriment of amphibians and reptiles. Biol. Invasions 10, 539–546. doi: 10.1007/s10530-007-9150-9
Trochet, A., Moulherat, S., Calvez, O., Stevens, V., Clobert, J., and Schmeller, D. (2014). A database of life-history traits of European amphibians. Biodiver. Data J. 2:e4123. doi: 10.3897/BDJ.2.e4123
Trombulak, S. C., and Frissell, C. A. (2000). Review of ecological effects of roads on terrestrial and aquatic communities. Conserv. Biol. 14, 18–30. doi: 10.1046/j.1523-1739.2000.99084.x
Uden, D. R., Hellman, M. L., Angeler, D. G., and Allen, C. R. (2014). The role of reserves and anthropogenic habitats for functional connectivity and resilience of ephemeral wetlands. Ecol. Appl. 24, 1569–1582. doi: 10.1890/13-1755.1
United Nations (2015). World Urbanisation Prospects: The 2014 Revision. New York, NY: UN Department of Economic and Social Affairs, Population Division.
Urban, D. L., Minor, E. S., Treml, E. A., and Schick, R. S. (2009). Graph models of habitat mosaics. Ecol. Lett. 12, 260–273. doi: 10.1111/j.1461-0248.2008.01271.x
Van der Ree, R., Gulle, N., Holland, K., van der Grift, E., Mata, C., and Suarez, F. (2007). “Overcoming the barrier effect of roads-how effective are mitigation strategies?” in Proceedings of the 2007 International Conference on Ecology and Transportation, eds C. L. Irwin, D. Nelson, and K. P. McDermott (Raleigh, NC: Center for Transportation and the Environment, North Carolina State University), 423–431.
Van der Ree, R., Jaeger, J. A. G., van der Grift, E. A., and Clevenger, A. P. (2011). Effects of roads and traffic on wildlife populations and landscape function: road ecology is moving toward larger scales. Ecol. Soc. 16:48. doi: 10.5751/ES-03982-160148
Van der Ree, R., Smith, D. J., and Grilo, C. (eds.). (2015). Handbook of Road Ecology. Chichester: Wiley.
Van Langevelde, F., and Jaarsma, C. F. (2009). Modeling the effect of traffic calming on local animal population persistence. Ecol. Soc. 14:39. doi: 10.5751/ES-03061-140239
Van Strien, M. J., and Grêt-Regamey, A. (2016). How is habitat connectivity affected by settlement and road network configurations? Results from simulating coupled habitat and human networks. Ecol. Model. 342, 186–198. doi: 10.1016/j.ecolmodel.2016.09.025
Van Strien, M. J., Keller, D., and Holderegger, R. (2012). A new analytical approach to landscape genetic modelling: least-cost transect analysis and linear mixed models. Mol. Ecol. 21, 4010–4023. doi: 10.1111/j.1365-294X.2012.05687.x
Van Strien, M. J., Keller, D., Holderegger, R., Ghazoul, J., Kienast, F., and Bolliger, J. (2014). Landscape genetics as a tool for conservation planning: predicting the effects of landscape change on gene flow. Ecol. Appl. 24, 327–339. doi: 10.1890/13-0442.1
Van Teeffelen, A. J. A., Vos, C. C., and Opdam, P. (2012). Species in a dynamic world: consequences of habitat network dynamics on conservation planning. Biol. Conserv. 153, 239–253. doi: 10.1016/j.biocon.2012.05.001
Verburg, P. H., and Overmars, K. P. (2009). Combining top-down and bottom-up dynamics in land use modeling: exploring the future of abandoned farmlands in Europe with the Dyna-CLUE model. Landsc. Ecol. 24, 1167–1181. doi: 10.1007/s10980-009-9355-7
Verburg, P. H., Veldkamp, T., and Bouma, J. (1999). Land use change under conditions of high population pressure: the case of Java. Glob. Environ. Change 9, 303–312. doi: 10.1016/S0959-3780(99)00175-2
Wallentin, G. (2017). Spatial simulation: a spatial perspective on individual-based ecology—a review. Ecol. Modell. 350, 30–41. doi: 10.1016/j.ecolmodel.2017.01.017
Wegener, M. (2014). “Land-use transport interaction models,” in Handbook of Regional Science, eds M. M. Fischer and P. Nijkamp (Berlin: Springer), 741–758.
Wegener, M., and Fürst, F. (1999). “Land-use transport interaction: state of the art,” in Berichte Aus Dem Institut für Raumplanung 46. (Dortmund: Institute of Spatial Planning, University of Dortmund).
Williams, K. (ed.). (2005). Spatial Planning, Urban Form and Sustainable Transport. London: Routledge.
Wright, J. P., and Jones, C. G. (2006). The concept of organisms as ecosystem engineers ten years on: progress, limitations, and challenges. BioScience 56, 203–209. doi: 10.1641/0006-3568(2006)056[0203:TCOOAE]2.0.CO;2
Zeller, K., McGarigal, K., and Whiteley, A. (2012). Estimating landscape resistance to movement: a review. Landsc. Ecol. 27, 777–797. doi: 10.1007/s10980-012-9737-0
Keywords: social-ecological system, land-use transport interaction, spatial networks, habitat connectivity, land-use planning, transport planning, conservation planning
Citation: van Strien MJ, Axhausen KW, Dubernet I, Guisan A, Grêt-Regamey A, Khiali-Miab A, Ortiz-Rodríguez DO and Holderegger R (2018) Models of Coupled Settlement and Habitat Networks for Biodiversity Conservation: Conceptual Framework, Implementation and Potential Applications. Front. Ecol. Evol. 6:41. doi: 10.3389/fevo.2018.00041
Received: 04 June 2017; Accepted: 27 March 2018;
Published: 16 April 2018.
Edited by:
Rodney Van Der Ree, University of Melbourne, AustraliaReviewed by:
Melissa Renee McHale, Colorado State University, United StatesLoren B. Byrne, Roger Williams University, United States
Copyright © 2018 van Strien, Axhausen, Dubernet, Guisan, Grêt-Regamey, Khiali-Miab, Ortiz-Rodríguez and Holderegger. This is an open-access article distributed under the terms of the Creative Commons Attribution License (CC BY). The use, distribution or reproduction in other forums is permitted, provided the original author(s) and the copyright owner are credited and that the original publication in this journal is cited, in accordance with accepted academic practice. No use, distribution or reproduction is permitted which does not comply with these terms.
*Correspondence: Maarten J. van Strien, bWFhcnRlbkB2c3RyaWVuLm5s