- 1Department of Plant and Soil Science, University of Vermont, Burlington, VT, United States
- 2Department of Microbiology and Immunology, Harvard Medical School, Boston, MA, United States
- 3Department of Applied Ecology, North Carolina State University, Raleigh, NC, United States
- 4Laboratory of Evolutionary Entomology, Institute of Biology, University of Neuchâtel, Neuchâtel, Switzerland
- 5Laboratorio Nacional de Genómica para la Biodiversidad, Centro de Investigación y de Estudios Avanzados, Irapuato, Mexico
Crop domestication has been embraced as a model system to study the genetics of plant evolution. Yet, the role of the environment, including biotic forces such as microbial and insect communities, in contributing to crop phenotypes under domestication and diversification has been poorly explored. In particular, there has been limited progress in understanding how human selection, agricultural cultivation (soil disturbance, fertilization, and irrigation), and biotic forces act as selective pressures on crop phenotypes. For example, geographically-structured pathogenic, pestiferous, and mutualistic interactions with crop plants have likely given rise to landraces that interact differently with local microbial and insect communities. In order to understand the adaptive role of crop traits, we argue that more studies should be conducted in the geographic centers of origin to test hypotheses on how abiotic, biotic, and human selective forces have shaped the phenotypes of domesticated plants during crop domestication and subsequent diversification into landraces. In these centers of origin, locally endemic species associated with wild ancestors have likely contributed to the selection on plant phenotypes. We address a range of questions that can only be studied in the geographic center of crop origin, placing emphasis on Mesoamerican polyculture systems, and highlight the significance of in situ studies for increasing the sustainability of modern agricultural systems.
Introduction
The domestication of crop plants has fundamentally altered the relationship between humans and their environment (Larson et al., 2014). While the genetics of crops domestication has been widely studied for some common plant species (Darwin, 1868; Evans, 1993; Smartt and Simmonds, 1995; Ladizinsky, 1998; Hancock, 2012), the role of ecological interaction within centers of origin in contributing crop phenotypic diversity has been overlooked (Chen et al., 2015; Perez-Jaramillo et al., 2016). Prior to domestication, wild ancestors of crop plants evolved in association with a broad assemblage of microbes and insects, with which they engaged in a range of pathogenic, predatory, commensal, and mutualistic interactions (Chen et al., 2013; Huang et al., 2016; Perez-Jaramillo et al., 2016). These ecological interactions were almost certainly altered by domestication and when early domesticates were introduced to new locations with unique climates, distinct local biodiversity, and different cultural methods of farming.
The majority of domestication events occurred in specific geographic regions, often within the native range of their wild ancestors (Vavilov, 1926, 1951; Meyer et al., 2012). Despite this, most studies rarely consider whether all interacting species are endemic in the center of origin. We found that only 1.6% of 1,532 studies comparing insect responses on wild and crop plants specifically accounted for biogeographical history (Chen et al., 2015). Geographically-explicit hypotheses are needed to understand in situ crop diversification for two reasons. First, human-mediated migration of crops to new regions within centers of origin influenced the genetic structuring of crop populations. Second, domesticated cultivars experienced novel selective pressures imposed by new environments and the cultural preferences of different indigenous peoples (Figure 1; Brush, 1995; Hugo et al., 2003).
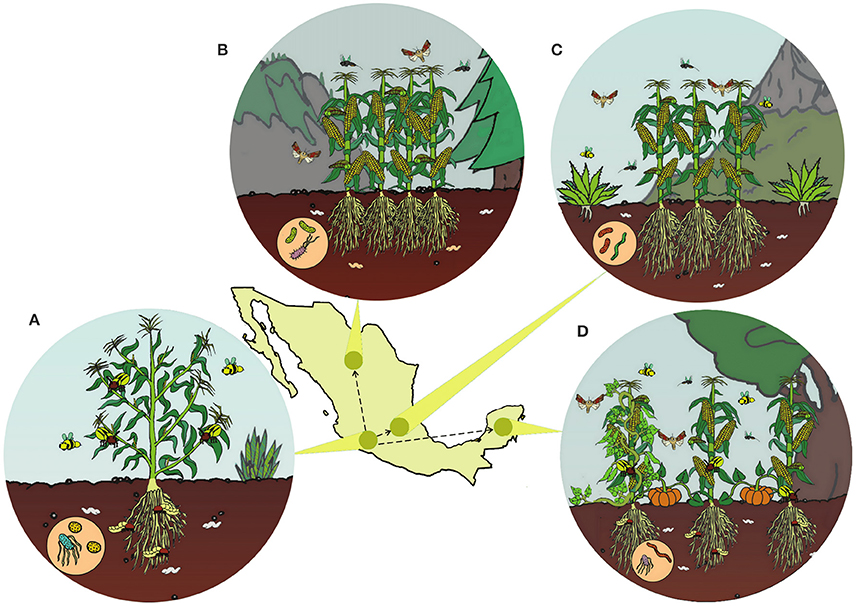
Figure 1. Following the domestication of maize in Western Mexico (A), maize was brought to new geographic regions (hypothetical routes shown by arrows). In the tropics (D), highland (B), and altiplano (C) environments, maize likely diversified as it experienced new climatic conditions, soils, cropping systems, biodiversity, and human preferences.
In situ field studies documenting variation of ecological interactions are important to determine the extent to which landrace phenotypes respond to local adaptation and artificial selection. After initial crop domestication, early landraces were brought to new environments that were often different from the ecological conditions experienced by their wild progenitors (Hufford et al., 2012). In these new environments, landraces interacted with new species, different cropping system, and new abiotic conditions (Figure 1). Also, different groups of farmers may cultivate the same crop in different polyculture systems (Casas et al., 1996, 2007; Hugo et al., 2003), which is the simultaneous cultivation of multiple crops. As a result, landraces emerge over thousands of years due to natural selection exerted by local abiotic conditions, local insect, and microbial communities, as well as human selection on traits related to ease of cultivation, aesthetics, taste, and cultural preferences (Perales et al., 2005; Brush and Perales, 2007; Casas et al., 2007; Aguirre-Dugua et al., 2013).
Here, we discuss the factors that contribute to phenotypic variation in crops and landraces. We examine the role of human selection and niche construction activities on crop phenotypic plasticity, a major factor in local adaptation (Piperno, 2017). We conceptually address two questions that could deepen our understanding of crop evolution and local adaptation: (1) To what extent are crop plants locally adapted? and (2) What are the relative roles of human selection, human-mediated migration, the local abiotic environment, endemic biotic communities, and cultivation practices in the diversification of crops into landraces? We describe polyculture systems in Mesoamerica as a suitable model in which these questions could be pursued. Ultimately, whether plants can maintain historic beneficial interactions with associated species—or form new ones in their introduced regions—have important implications for the future sustainability of agriculture.
Genetic and Environmental Contributions to Crop Phenotypic Variation
We focus on landraces as a natural experimental system to understand how natural and human selective forces have shaped the diversity of phenotypic traits of domesticated crop plants during local adaptation. In order to detect local adaptation, it is important to characterize the extent of variation and fitness of phenotypic traits in response to local selective pressures (Kawecki and Ebert, 2004). Variation in landrace phenotypes is affected by their genotype, local environmental variation, and plant phenotypic plasticity. Equation 1 describes how the components of phenotypic variation can be partitioned (Pigliucci, 2001):
Where Vp denotes the total phenotypic variation found for a population or subspecies, VG is the total genetic variation, VE is the total environmental variation, and VGxE is the genotype × environment interaction. We limit our treatment of Vp and VG because they have been discussed elsewhere (Olsen and Wendel, 2013; Piperno, 2017). In contrast, there has been a minimal effort to understand the contributions of VE and VGxE to crop domestication and diversification. Table 1 provides a set of examples illustrating the range of plant adaptations to abiotic, biotic, and human selection.
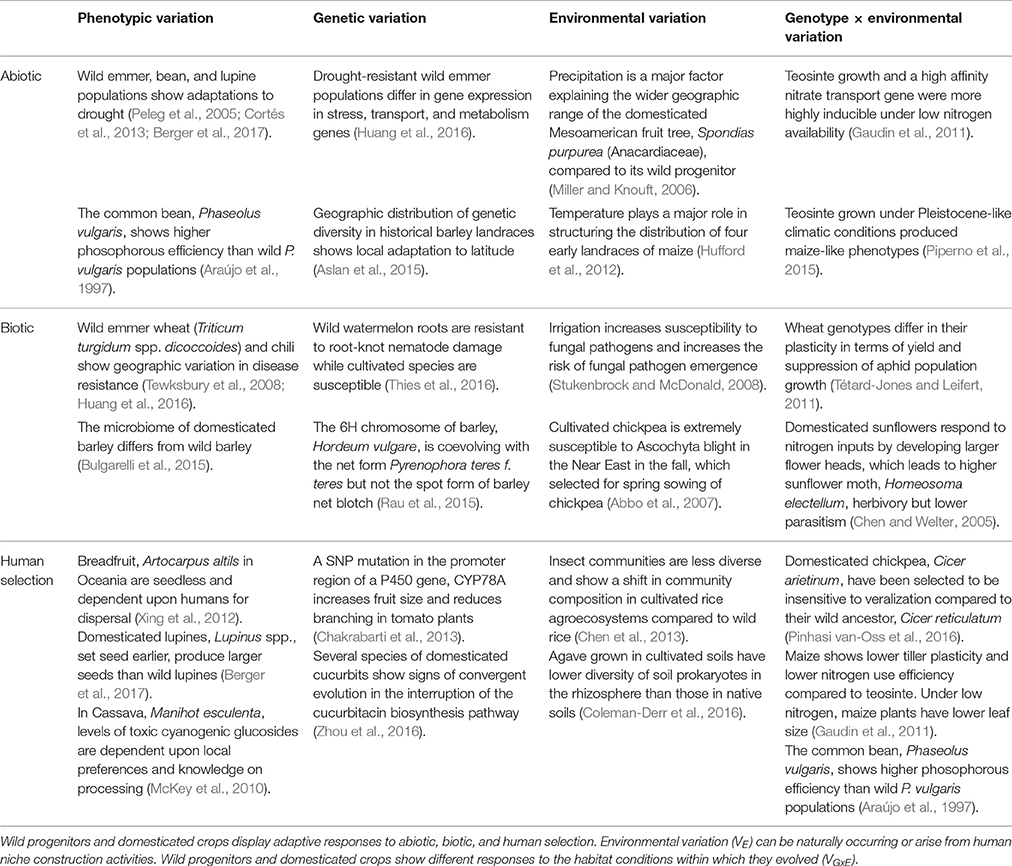
Table 1. Phenotypic variation (Vp) in wild progenitors and landraces can be attributed to genetic variation (VG), environmental variation (VE), and variation attributed to genotype by environment interactions (VGxE).
Total genetic variation (VG) is influenced by evolutionary forces including selection, genetic drift, inbreeding, and gene flow (Hartl and Clark, 2006), which likely differ between wild progenitor and crop populations. Domesticated crops have reduced effective population sizes and are under intense selection for a suite of traits favored by humans (Doebley et al., 2006; Moreno et al., 2006). Cultural factors can also structure landrace populations and dispersal patterns, as people in different ethnolinguistic groups are less likely to trade seeds (Orozco-Ramírez et al., 2016).
The contribution of the environment to phenotypic variation (VE) strongly differs between wild progenitors and domesticated crops grown in human constructed agricultural niches. In natural systems, VE is affected by local abiotic conditions such as climate, precipitation, soil type, and nutrient availability. Domesticated crops are found in a range of cultivation systems, where the purpose of cultivation is to lessen the unpredictability of the local environment in order to favor uniform plant growth and yield. Cultivation has been shown to strongly affect plant-associated microbial and insect communities (Berg, 2009; Berendsen et al., 2012; Chen et al., 2013). Surprisingly, the majority of the insect and microbial species associated with a given crop in its center of origin remain undescribed (Chen et al., 2015; Coleman-Derr et al., 2016). Given that plant-biotic interactions can influence plant phenotypes (Henning et al., 2016), human niche activities can indirectly influence crop phenotypes by influencing the diversity and community structure of biotic assemblages.
Genotype by environment interactions (VGxE) describe phenotypic plasticity, or how genotypes responds to a range of environmental conditions. Crops respond to agricultural conditions differently from their wild ancestors in growth (Table 1). Also, plasticity itself may have been constrained by domestication. Wild progenitors may have retained a greater plasticity to unpredictable environments, whereas domesticated may have lost phenotypic plasticity. Growing plants in different environments can provide important insight on the origin of important traits associated with domestication. For instance, teosinte grown under historic Pleistocene conditions (atmospheric CO2 and temperature) displayed maize-like phenotypes such as reduced tillering, uniform seed maturation, and bract-less seeds (Piperno et al., 2015). Therefore, hypotheses that account for environmental variation and the selective environment can provide important insight on how crop species adapted to divergent environmental conditions.
Crop responses to the environment can be heritable, independent of changes in the DNA sequence, which contributes another dimension to VGxE. An emerging frontier is to understand the role of transgenerational epigenetics in crop adaptation to local environmental conditions (Piperno, 2017). Epigenetics studies can reveal how the environment can shape heritable gene expression (and thus the phenotype) without changing the underlying DNA sequence (Jablonka and Raz, 2009; Laland et al., 2014). Epigenetic changes in DNA methylation can regulate gene expression patterns during stress, which can influence the ability of crop varieties to respond to local environments (Ferreira et al., 2015). Therefore, in situ studies are critically needed to test the relative roles of genetics, epigenetics, and the environment in contributing to crop diversification.
Mesoamerica As A Field Laboratory for In situ Studies
Centers of domestication offer field locations to understand how local environments and human cultural influences contributed to crop domestication and diversification into landraces. The legendary Russian botanist Nikolai Vavilov delineated eight geographic regions as “centers of domestication” where multiple crop species were domesticated (Vavilov, 1926, 1951). One such center is Mesoamerica, which is the region of origin for maize, beans, squash, peppers, avocado, vanilla, and thousands of non-commercialized plant species (Casas et al., 2007). Crop domestication in Mesoamerica has received far less attention than in other centers of origin (Casas et al., 2007). Mesoamerica is particularly well-suited for field studies on crop domestication and diversification for several reasons: (1) It hosts a dazzling array of landrace varieties that are often cultivated sympatrically with their wild ancestors, (2) It has an archeological record confirming a long history of interactions between humans and many species of crop plants, and (3) Indigenous peoples continue oral and cultural traditions associated with the cultivation of these plants (Gepts, 2004; Staller et al., 2006; Piperno et al., 2007).
We focus on Mexico, the largest country within Mesoamerica, where much of the phenotypic and genotypic variation underlying local adaptation to environments has not been characterized. Many suspected centers of domestication and regions with wild relatives remain poorly explored for most crop plants. These underexplored regions that are historically relevant for the study of crop diversification include: the Balsas River Valley (Piperno et al., 2007), the Gulf Coast (Kraft et al., 2014), and the Tehuacan Valley for species other than maize (Vallejo et al., 2016). Needless to say, there are considerable opportunities to examine the selective forces that produced the extant array of landraces for native Mesoamerican crop plant species.
In Mesoamerica, traditional agroecosystems have been maintained cohesively for hundreds to thousands of years by indigenous peoples. Different cropping systems dominate in different climatic regions (Figure 1). In the Yucatan Peninsula, home gardens are highly diverse polyculture systems, and include crops such as avocado, annona, and papaya (Moreno-Calles et al., 2016). The inland and coastal regions are dominated by agroforestry systems paired with ornamental and woody species (Moreno-Calles et al., 2016). The oldest Mesoamerican polyculture systems continue to be maintained in Tlaxcala (Gonzalez-Jacome, 2016). In cold and dry highland environments, prehispanic terraces for water management still exist in Oaxaca and the Tehuacan Valley (Donkin, 1979). In arid environments, drought-tolerant plants including cactus are cultivated in polyculture with chili pepper and other crops (Moreno-Calles et al., 2012, 2016). Slash and burn agriculture is the most widespread form of cultivation in the tropical deciduous and temperate forests, where crops are rotated after the plant cover is burned every few years. Slash and burn systems can be quite diverse, with at least 57 tree species from 33 plant families and many more herbaceous species (Moreno-Calles et al., 2016).
One of the most dominant cropping system in slash and burn agriculture is the milpa (Figure 1D), which is, at its most basic, the joint cultivation of maize, beans, squash (Zizumbo-Villarreal and Colunga-GarcíaMarín, 2010). Maize serves as a trellis for beans that fix nitrogen, while squash suppresses weeds. Although, the wild progenitors of maize, beans, and squash are native to separate regions in Mexico (Smith, 1997; Gepts, 2004; Piperno et al., 2007), these core milpa crops have been cultivated together for thousands of years. It is highly possible that associated microbes and insects have adapted to the milpa. Perhaps it should not be a surprise that the most devastating contemporary native insect pests of maize can all be collected from squash flowers (Metcalf and Lampman, 1989), and that several closely related species of leaf beetles in the genus Diabrotica damage maize, beans, and squash (Clark et al., 2001; Vidal et al., 2005; Eben and Espinosa de Los Monteros, 2013). There are many other unexplored questions on crops and biotic interactions in milpa systems such as: (1) To what extent have microbiomes, pathogens, mycorrhizae, herbivores, natural enemies, and pollinators adapted to polyculture and landrace traits? (2) Does intercropping select landraces to develop complementary use for light, nutrients, and water? and (3) How has the milpa shaped landrace phenotypes?
The present Mesoamerican landscape is also a gradient of ecological contexts where one could study whether genes underlie phenotypes that are adaptive to local abiotic and biotic conditions. Reciprocal common garden studies with maize have found that highland landraces show higher fitness and seed quality in highland conditions, while lowland landraces have higher fitness in mid-altitude locations (Mercer et al., 2008). Traits such as pigmentation, stem hair, plant height, and flowering time have been shown to be adaptive to altitude, but completely different genes underlie local adaptation to highland conditions in Mesoamerica and South America (Mercer et al., 2008). Field sampling of Mexican teosinte populations helped to clarify that maize adaptation to the Mexican highlands resulted due to introgression from wild teosinte (Hufford et al., 2013). Understanding the genomic basis of local adaptation in crops relies on multiple in situ localities, where the ecological history can be reconstructed by testing for genomic regions under divergence (Pyhäjärvi et al., 2013), and the responses of candidate genes can be observed in local environments (Doust et al., 2014; Piperno, 2017). Traditional Mesoamerican agroecosystems are living biological and ethnographic systems that are suitable for studying how human-created niches in agroecosystems interact with local biotic and abiotic environments to shape landrace phenotypes. These in situ systems provide an important reference for examining how crop plants adapted as they have diversified within centers of origin.
Implications of In situ Studies for Sustainable Agriculture
Sustainable agriculture aims to reduce reliance on pesticides and fertilizers by utilizing biodiversity to provide ecological services that provision nutrients, protect crops, and enhance yields (Altieri, 1999). For the world's most important crops, the majority of production occurs outside their centers of origin (Khoury et al., 2016). Oftentimes, crops are grown in marginal environments, where they experience low nutrient availability, excess or limited water availability, temperature extremes, or pest outbreaks (Table 1). Under climate change, these pressures are predicted to intensify (Hatfield et al., 2010). Perhaps because centers of origin tend to be the geographic source for the major diseases (Leppik, 1970) and insect pests of crops (Chen, 2016), they are also the source for genes for resistance (Harlan, 1976; Hijmans et al., 2003; Zhang et al., 2017), insect natural enemies (van den Bosch, 1971; van Driesche et al., 2008), and microbes (Philippot et al., 2013; Perez-Jaramillo et al., 2016) that help plants to resist pests and tolerate abiotic stress.
In situ studies can also provide insight on whether human selection for crop yield is fundamentally at odds with traits that mediate beneficial plant-biotic interactions. First, crop domestication appears to have promoted pests more frequently than beneficial species, especially for economically-important traits such as fruit size and seed size (Chen et al., 2015). However, we do not know if direct trade-offs between yield and pest resistance exist, and whether this relationship may vary with different landraces and environments within centers of origin. The diversity and community structure of microbes and insects associated with wild ancestors and landraces have been inadequately described (Chen et al., 2013; Perez-Jaramillo et al., 2016), and geographic variation in patterns of biodiversity within centers of origin remain unexplored.
Second, plant genotypes vary in their ability to form positive relationships with beneficial species (Table 1; Smith and Goodman, 1999; Chen and Welter, 2005; Tamiru et al., 2011). Determining the relative roles of plant genetic diversity, microbial associates, and plant gene × environment interactions in conferring resistance to biotic and abiotic stresses (Philippot et al., 2013) would help elucidate whether breeding, microbial inoculation strategies, or natural enemy introductions would better support crop production in the diverse environments where crops are grown. Finally, in situ studies provide insight on the ecological function of crop genes and metabolites within their natural environment, which are oftentimes only explored in environments far from centers of origin. For examples, teosinte and some maize varieties emit the sesquiterpene (E)-β-caryophyllene, which attracts entomopathogenic nematodes (Rasmann et al., 2005) and parasitoids (Kollner et al., 2008) in Europe. However, the role of this compound in landraces is not known, especially in Mesoamerica, where a diverse assemblage of species may be adapted to respond to plant signals. In situ studies can help resolve whether landrace varieties produce the compound, natural enemies are attracted to it, and whether breeding for (E)-β-caryophyllene would increase natural enemy attraction and enhance yield in the diverse worldwide locations where maize is now grown.
Conclusions
In situ ecological studies are an essential, but almost completely unexplored line of inquiry for evolutionary ecologists to understand the selective forces that contribute to local adaptation of landrace varieties. As one of the major centers of crop origin, Mesoamerica is an ideal location for in situ studies, because wild progenitors can be found growing sympatrically with domesticated landrace varieties cultivated in traditional polyculture systems. For many crops and cultivation systems, the unique combination of local abiotic, biotic, and cultural selective forces that shaped variation in crop phenotypes during domestication and diversification continue to coexist. We advocate that geographically-explicit studies will yield new insight into how selection from humans and the local environment contribute to landrace diversification and local adaptation. Such knowledge is immediately applicable toward understanding the capacity of crop plants to respond to the biotic and abiotic conditions over the vast geographic ranges where they are now grown, and to identify sources of germplasm that might have adaptive traits for crops in their introduced ranges.
Author Contributions
YC, LS, and AC conceived the Perspective. YC, AC, LS, and BB drafted the work and revised the content.
Funding
We thank our funders for their support: USDA National Institute of Food and Agriculture grant no. #VT-H02301MS to YC, NSF postdoctoral fellowship DBI-1202736 to LS, Swiss National Science Foundation, project No. 31003A_162860 to BB, and CONACyT Problemas Nacionales #247730 to AC.
Conflict of Interest Statement
The authors declare that the research was conducted in the absence of any commercial or financial relationships that could be construed as a potential conflict of interest.
Acknowledgments
We thank Nicolas Marguler for the design of Figure 1.
References
Abbo, S., Frenkel, O., Sherman, A., and Shtienberg, D. (2007). The sympatric Ascochyta pathosystems of Near Eastern legumes, a key for better understanding of pathogen biology. Eur. J. Plant Pathol. 119, 111–118. doi: 10.1007/s10658-007-9116-x
Aguirre-Dugua, X., Pérez-Negrón, E., and Casas, A. (2013). Phenotypic differentiation between wild and domesticated varieties of Crescentia cujete L. and culturally relevant uses of their fruits as bowls in the Yucatan Peninsula, Mexico. J. Ethnobiol. Ethnomed. 9:76. doi: 10.1186/1746-4269-9-76
Altieri, M. A. (1999). The ecological role of biodiversity in agroecosystems. Agric. Ecosyst. Environ. 74, 19–31. doi: 10.1016/S0167-8809(99)00028-6
Araújo, A. P., Teixeira, M. G., and Almeida, D. L. (1997). Phosphorus efficiency of wild and cultivated genotypes of common bean (Phaseolus vulgaris) under biological nitrogen fixation. Soil Biol. Biochem. 29, 951–957. doi: 10.1016/S0038-0717(96)00217-9
Aslan, S., Forsberg, N. E. G., Hagenblad, J., and Leino, M. W. (2015). Molecular genotyping of historical barley landraces reveals novel candidate regions for local adaption. Crop Sci. 55, 2766–2776. doi: 10.2135/cropsci2015.02.0119
Berendsen, R. L., Pieterse, C. M., and Bakker, P. A. (2012). The rhizosphere microbiome and plant health. Trends Plant Sci. 17, 478–486. doi: 10.1016/j.tplants.2012.04.001
Berg, G. (2009). Plant-microbe interactions promoting plant growth and health: perspectives for controlled use of microorganisms in agriculture. Appl. Microbiol. Biotechnol. 84, 11–18. doi: 10.1007/s00253-009-2092-7
Berger, J. D., Shrestha, D., and Ludwig, C. (2017). Reproductive strategies in Mediterranean legumes: trade-offs between phenology, seed size and vigor within and between wild and domesticated Lupinus species collected along aridity gradients. Front. Plant Sci. 8:548. doi: 10.3389/fpls.2017.00548
Brush, S. B. (1995). In situ conservation of landraces in centers of crop diversity. Crop Sci. 35, 346–354. doi: 10.2135/cropsci1995.0011183X003500020009x
Brush, S. B., and Perales, H. R. (2007). A maize landscape: ethnicity and agro-biodiversity in Chiapas Mexico. Agric. Ecosyst. Environ. 121, 211–221. doi: 10.1016/j.agee.2006.12.018
Bulgarelli, D., Garrido-Oter, R., Munch, P. C., Weiman, A., Droge, J., Pan, Y., et al. (2015). Structure and function of the bacterial root microbiota in wild and domesticated barley. Cell Host Microbe 17, 392–403. doi: 10.1016/j.chom.2015.01.011
Casas, A., Otero-Arnaiz, A., Perez-Negron, E., and Valiente-Banuet, A. (2007). In situ management and domestication of plants in Mesoamerica. Ann. Bot. 100, 1101–1115. doi: 10.1093/aob/mcm126
Casas, A., del Vázquez, M. C., Viveros, J. L., and Caballero, J. (1996). Plant management among the Nahua and the Mixtec in the Balsas River Basin, Mexico: an ethnobotanical approach to the study of plant domestication. Hum. Ecol. 24, 455–478. doi: 10.1007/BF02168862
Chakrabarti, M., Zhang, N., Sauvage, C., Muños, S., Blanca, J., Cañizares, J., et al. (2013). A cytochrome P450 regulates a domestication trait in cultivated tomato. Proc. Natl. Acad. Sci. U.S.A. 110, 17125–17130. doi: 10.1073/pnas.1307313110
Chen, Y. H. (2016). Crop domestication, global human-mediated migration, and the unresolved role of geography in pest control. Elem. Sci. Anthr. 4:106. doi: 10.12952/journal.elementa.000106
Chen, Y. H., Gols, R., and Benrey, B. (2015). Crop domestication and naturally selected species interactions. Annu. Rev. Entomol. 60, 35–58. doi: 10.1146/annurev-ento-010814-020601
Chen, Y. H., Langellotto, G. A., Barrion, A. T., and Cuong, N. L. (2013). Cultivation of domesticated rice alters arthropod biodiversity and community composition. Ann. Entomol. Soc. Am. 106, 100–110. doi: 10.1603/AN12082
Chen, Y. H., and Welter, S. C. (2005). Crop domestication disrupts a native tritrophic interaction associated with the sunflower, Helianthus annuus (Asterales: Asteraceae). Ecol. Entomol. 30, 673–683. doi: 10.1111/j.0307-6946.2005.00737.x
Clark, T. L., Meinke, L. J., and Foster, J. E. (2001). Molecular phylogeny of Diabrotica beetles (Coleoptera: Chrysomelidae) inferred from analysis of combined mitochondrial and nuclear DNA sequences. Insect Mol. Biol. 10, 303–314. doi: 10.1046/j.0962-1075.2001.00269.x
Coleman-Derr, D., Desgarennes, D., Fonseca-Garcia, C., Gross, S., Clingenpeel, S., Woyke, T., et al. (2016). Biogeography and cultivation affect microbiome composition in the drought-adapted plant Subgenus Agave. New Phytol. 209, 798–811. doi: 10.1111/nph.13697
Cortés, A. J., Monserrate, F. A., Ramírez-Villegas, J., Madri-án, S., and Blair, M. W. (2013). Drought tolerance in wild plant populations: the case of common beans (Phaseolus vulgaris L.). PLoS ONE 8:e62898. doi: 10.1371/journal.pone.0062898
Darwin, C. (1868). The Variation of Animals and Plants under Domestication, Vol. 1, 2. London: John Murray.
Doebley, J. F., Gaut, B. S., and Smith, B. D. (2006). The molecular genetics of crop domestication. Cell 127, 1309–1321. doi: 10.1016/j.cell.2006.12.006
Donkin, R. A. (1979). Agricultural Terracing in the Aboriginal New World. Flagstaff, AZ: The Wenner-Gren Foundation for Anthropological Research, The University of Arizona.
Doust, A. N., Lukens, L., Olsen, K. M., Mauro-Herrera, M., Meyer, A., and Rogers, K. (2014). Beyond the single gene: how epistasis and gene-by-environment effects influence crop domestication. Proc. Natl. Acad. Sci. U.S.A. 111, 6178–6183. doi: 10.1073/pnas.1308940110
Eben, A., and Espinosa de Los Monteros, A. (2013). Tempo and mode of evolutionary radiation in Diabroticina beetles (genera Acalymma, Cerotoma, and Diabrotica). Zookeys 207–321. doi: 10.3897/zookeys.332.5220
Ferreira, L. J., Azevedo, V., Maroco, J., Oliveira, M. M., and Santo, A. P. (2015). Salt tolerant and sensitive rice varieties display differential methylome flexibility under salt stress. PLoS ONE 10:e0124060. doi: 10.1371/journal.pone.0124060
Gaudin, A. C. M., McClymont, S. A., and Raizada, M. N. (2011). The nitrogen adaptation strategy of the wild teosinte ancestor of modern maize, Zea mays subsp. parviglumis. Crop Sci. 51, 2780–2795. doi: 10.2135/cropsci2010.12.0686
Gepts, P. (2004). Crop domestication as a long-term selection experiment. Plant Breed. Rev. 24, 1–44. doi: 10.1002/9780470650288.ch1
Gonzalez-Jacome, A. (2016). “Sistemas agrícolas en orografías complejas: las terrazas de Tlaxcala,” in Etnoagroforestería en México, eds A. I. Moreno-Calles, A. Casas, V. M. Toledo, and M. Vallejo-Ramos (Mexico: UNAM), 111–146.
Hancock, J. F. (2012). Plant Evolution and the Origin of Crop Species. Wallingford, CT; Oxfordshire: CABI.
Harlan, J. R. (1976). Diseases as a factor in plant evolution. Annu. Rev. Phytopathol. 14, 31–51. doi: 10.1146/annurev.py.14.090176.000335
Hartl, D. L., and Clark, A. G. (2006). Principles of Population Genetics, 4th Edn. Sunderland, MA: Sinauer Associates.
Hatfield, J. L., Boote, K. J., Kimball, B. A., Ziska, L. H., Izaurralde, R. C., Ortf, D., et al. (2010). Climate impacts on agriculture: implications for crop production. Agron. J. 103, 351–370. doi: 10.2134/agronj2010.0303
Henning, J. A., Weston, D. J., Pelletier, D. A., Timm, C. M., Jawdy, S. S., and Classen, A. T. (2016). Root bacterial endophytes alter plant phenotype, but not physiology. PeerJ 4:e2606. doi: 10.7717/peerj.2606
Hijmans, R. J., Jacobs, M., Bamberg, J. B., and Spooner, D. M. (2003). Frost tolerance in wild potato species: assessing the predictivity of taxonomic, geographic, and ecological factors. Euphytica 130, 47–59. doi: 10.1023/A:1022344327669
Huang, L., Raats, D., Sela, H., Klymiuk, V., Lidzbarsky, G., Feng, L., et al. (2016). Evolution and adaptation of wild emmer wheat populations to biotic and abiotic stresses. Annu. Rev. Phytopathol. 54, 279–301. doi: 10.1146/annurev-phyto-080614-120254
Hufford, M. B., Lubinksy, P., Pyhäjärvi, T., Devengenzo, M. T., Ellstrand, N. C., and Ross-Ibarra, J. (2013). The genomic signature of crop-wild introgression in maize. PLoS Genet. 9:e1003477. doi: 10.1371/journal.pgen.1003477
Hufford, M. B., Martínez-Meyer, E., Gaut, B. S., Eguiarte, L. E., Tenaillon, M. I., Cienfuegos, E., et al. (2012). Inferences from the historical distribution of wild and domesticated maize provide ecological and evolutionary insight. PLoS ONE 7:e47659. doi: 10.1371/journal.pone.0047659
Hugo, P. R., Brush, S. B., and Qualset, C. O. (2003). Dynamic management of maize landraces in Central Mexico. Econ. Bot. 57, 21–34. doi: 10.1663/0013-0001(2003)057[0021:DMOMLI]2.0.CO;2
Jablonka, E., and Raz, G. (2009). Transgenerational epigenetic inheritance: prevalence, mechanisms, and implications for the study of heredity and evolution. Q. Rev. Biol. 84, 131–176. doi: 10.1086/598822
Kawecki, T. J., and Ebert, D. (2004). Conceptual issues in local adaptation. Ecol. Lett. 7, 1225–1241. doi: 10.1111/j.1461-0248.2004.00684.x
Khoury, C. K., Achicanoy, H. A., Bjorkman, A. D., Navarro-Racines, C., Guarino, L., Flores-Palacios, X., et al. (2016). Origins of food crops connect countries worldwide. Proc. R. Soc. B 283, 468–474. doi: 10.1098/rspb.2016.0792
Kollner, T. G., Held, M., Lenk, C., Hiltpold, I., Turlings, T. C. J., Gershenzon, J., et al. (2008). A maize (E)-β-caryophyllene synthase implicated in indirect defense responses against herbivores is not expressed in most American maize varieties. Plant Cell 20, 482–494. doi: 10.1105/tpc.107.051672
Kraft, K. H., Brown, C. H., Nabhan, G. P., Luedeling, E., Luna Ruiz, J. D. J., Coppens d'Eeckenbrugge, G., et al. (2014). Multiple lines of evidence for the origin of domesticated chili pepper, Capsicum annuum, in Mexico. Proc. Natl. Acad. Sci. U.S.A. 111, 6165–6170. doi: 10.1073/pnas.1308933111
Ladizinsky, G. (1998). Plant Evolution under Domestication. Dordrecht; New York, NY: Kluwer Academic Publishers.
Laland, K., Uller, T., Feldman, M., Sterelny, K., Müller, G. B., Moczek, A., et al. (2014). Does evolutionary theory need a rethink? Nature 514, 161–164. doi: 10.1038/514161a
Larson, G., Piperno, D. R., Allaby, R. G., Purugganan, M. D., Andersson, L., Arroyo-Kalin, M., et al. (2014). Current perspectives and the future of domestication studies. Proc. Natl. Acad. Sci. U.S.A. 111, 6139–6146. doi: 10.1073/pnas.1323964111
Leppik, E. E. (1970). Gene centers of plants as sources of disease resistance. Annu. Rev. Phytopathol. 8, 323–344. doi: 10.1146/annurev.py.08.090170.001543
McKey, D., Cavagnaro, T. R., Cliff, J., and Gleadow, R. (2010). Chemical ecology in coupled human and natural systems: people, manioc, multitrophic interactions and global change. Chemoecology 20, 109–133. doi: 10.1007/s00049-010-0047-1
Mercer, K., Martínez-Vásquez, Á., and Perales, H. R. (2008). Asymmetrical local adaptation of maize landraces along an altitudinal gradient. Evol. Appl. 1, 489–500. doi: 10.1111/j.1752-4571.2008.00038.x
Metcalf, R. L., and Lampman, R. L. (1989). The chemical ecology of Diabroticites and Cucurbitaceae. Experientia 45, 240–247. doi: 10.1007/BF01951810
Meyer, R. S., DuVal, A. E., and Jensen, H. R. (2012). Patterns and processes in crop domestication: an historical review and quantitative analysis of 203 global food crops. New Phytol. 196, 29–48. doi: 10.1111/j.1469-8137.2012.04253.x
Miller, A. J., and Knouft, J. H. (2006). GIS-based characterization of the geographic distributions of wild and cultivated populations of the Mesoamerican fruit tree Spondias purpurea (Anacardiaceae). Am. J. Bot. 93, 1757–1767. doi: 10.3732/ajb.93.12.1757
Moreno, L. L., Tuxill, J., Moo, E. Y., Reyes, L. A., Alejo, J. C., and Jarvis, D. I. (2006). Traditional maize storage methods of Mayan farmers in Yucatan, Mexico: implications for seed selection and crop diversity. Biodivers. Conserv. 15, 1771–1795. doi: 10.1007/s10531-004-6679-0
Moreno-Calles, A., Casas, A., García-Frapolli, E., and Torres-García, I. (2012). Traditional agroforestry systems of multi-crop “milpa” and “chichipera” cactus forest in the arid Tehuacan Valley, Mexico: their management and role in people's subsistence. Agrofor. Syst. 84, 207–226. doi: 10.1007/s10457-011-9460-x
Moreno-Calles, A. I., Casas, A., Rivero-Romero, A. D., Romero-Bautista, Y. A., Rangel-Landa, S., Fisher-Ortíz, R. A., et al. (2016). Ethnoagroforestry: integration of biocultural diversity for food sovereignty in Mexico. J. Ethnobiol. Ethnomed. 12, 54. doi: 10.1186/s13002-016-0127-6
Olsen, K. M., and Wendel, J. F. (2013). A bountiful harvest: genomic insights into crop domestication phenotypes. Annu. Rev. Plant Biol. 64, 47–70. doi: 10.1146/annurev-arplant-050312-120048
Orozco-Ramírez, Q., Ross-Ibarra, J., Santacruz-Varela, A., and Brush, S. (2016). Maize diversity associated with social origin and environmental variation in Southern Mexico. Heredity 116, 477–484. doi: 10.1038/hdy.2016.10
Peleg, Z., Fahima, T., Abbo, S., Krugman, T., Nevo, E., Yakir, D., et al. (2005). Genetic diversity for drought resistance in wild emmer wheat and its ecogeographical associations. Plant Cell Environ. 28, 176–191. doi: 10.1111/j.1365-3040.2005.01259.x
Perales, H. R., Benz, B. F., and Brush, S. B. (2005). Maize diversity and ethnolinguistic diversity in Chiapas, Mexico. Proc. Natl. Acad. Sci. U.S.A. 102, 949–954. doi: 10.1073/pnas.0408701102
Perez-Jaramillo, J. E., Mendes, R., and Raaijmakers, J. M. (2016). Impact of plant domestication on rhizosphere microbiome assembly and functions. Plant Mol. Biol. 90, 635–644. doi: 10.1007/s11103-015-0337-7
Philippot, L., Raaijmakers, J. M., Lemanceau, P., and van der Putten, W. H. (2013). Going back to the roots: the microbial ecology of the rhizosphere. Nat. Rev. Microbiol. 11, 789–799. doi: 10.1038/nrmicro3109
Pigliucci, M. (2001). Phenotypic Plasticity: Beyond Nature and Nurture. Baltimore, MD: Johns Hopkins University Press.
Pinhasi van-Oss, R., Sherman, A., Zhang, H.-B., Vandemark, G., Coyne, C., and Abbo, S. (2016). Vernalization response of domesticated × wild chickpea progeny is subject to strong genotype by environment interaction. Plant Breed. 135, 102–110. doi: 10.1111/pbr.12325
Piperno, D. R. (2017). Assessing elements of an extended evolutionary synthesis for plant domestication and agricultural origin research. Proc. Natl. Acad. Sci. U.S.A. 114, 6429–6437. doi: 10.1073/pnas.1703658114
Piperno, D. R., Holst, I., Winter, K., and McMillan, O. (2015). Teosinte before domestication: experimental study of growth and phenotypic variability in Late Pleistocene and early Holocene environments. Quat. Int. 363, 65–77. doi: 10.1016/j.quaint.2013.12.049
Piperno, D. R., Moreno, J. E., Iriarte, J., Holst, I., Lachniet, M., Jones, J. G., et al. (2007). Late Pleistocene and Holocene environmental history of the Iguala Valley, central Balsas Watershed of Mexico. Proc. Natl. Acad. Sci. U.S.A. 104, 11874–11881. doi: 10.1073/pnas.0703442104
Pyhäjärvi, T., Hufford, M. B., Mezmouk, S., and Ross-Ibarra, J. (2013). Complex patterns of local adaptation in teosinte. Genome Biol. Evol. 5, 1594–1609. doi: 10.1093/gbe/evt109
Rasmann, S., Köllner, T. G., Degenhardt, J., Hiltpold, I., Toepfer, S., Kuhlmann, U., et al. (2005). Recruitment of entomopathogenic nematodes by insect-damaged maize roots. Nature 434, 732–737. doi: 10.1038/nature03451
Rau, D., Rodriguez, M., Leonarda Murgia, M., Balmas, V., Bitocchi, E., Bellucci, E., et al. (2015). Co-evolution in a landrace meta-population: two closely related pathogens interacting with the same host can lead to different adaptive outcomes. Sci. Rep. 5:12834. doi: 10.1038/srep12834
Smartt, J., and Simmonds, N. W. (1995). Evolution of Crop Plants. New York, NY: John Wiley & Sons, Inc.
Smith, B. D. (1997). The initial domestication of Cucurbita pepo in the Americas 10,000 years ago. Science 276, 932–934. doi: 10.1126/science.276.5314.932
Smith, K. P., and Goodman, R. M. (1999). Host variation for interactions with beneficial plant-associated microbes. Annu. Rev. Phytopathol. 37, 473–491. doi: 10.1146/annurev.phyto.37.1.473
Staller, J., Tykot, R., and Benz, B. (2006). Histories of Maize Multidisciplinary Approaches to the Prehistory, Linguistics, Biogeography, Domestication, and Evolution of Maize. New York, NY: Elsevier.
Stukenbrock, E. H., and McDonald, B. A. (2008). The origins of plant pathogens in agro-ecosystems. Annu. Rev. Phytopathol. 46, 75–100. doi: 10.1146/annurev.phyto.010708.154114
Tamiru, A., Bruce, T. J., Woodcock, C. M., Caulfield, J. C., Midega, C. A., Ogol, C. K., et al. (2011). Maize landraces recruit egg and larval parasitoids in response to egg deposition by a herbivore. Ecol. Lett. 14, 1075–1083. doi: 10.1111/j.1461-0248.2011.01674.x
Tétard-Jones, C., and Leifert, C. (2011). Plasticity of yield components of winter wheat in response to cereal aphids. NJAS Wageningen J. Life Sci. 58, 139–143. doi: 10.1016/j.njas.2011.01.003
Tewksbury, J. J., Reagan, K. M., Machnicki, N. J., Carlo, T. A., Haak, D. C., Pe-aloza, A. L. C., et al. (2008). Evolutionary ecology of pungency in wild chilies. Proc. Natl. Acad. Sci. U.S.A. 105, 11808–11811. doi: 10.1073/pnas.0802691105
Thies, J. A., Ariss, J. J., Kousik, C. S., Hassell, R. L., and Levi, A. (2016). Resistance to southern root-knot nematode (Meloidogyne incognita) in wild watermelon (Citrullus lanatus var. citroides). J. Nematol. 48, 14–19. doi: 10.21307/jofnem-2017-004
Vallejo, M., Casas, A., Moreno-Calles, A. I., and Blancas, J. (2016). “Los sistemas agroforestales del Valle de Tehuacán: una perspectiva regional,” in Etnoagroforestería en México, eds A. I. Moreno-Calles, A. Casas, V. M. Toledo, and M. Vallejo-Ramos (Mexico, DF: UNAM), 193–218.
van den Bosch, R. (1971). Biological control of insects. Annu. Rev. Ecol. Syst. 2, 45–66. doi: 10.1146/annurev.es.02.110171.000401
van Driesche, R., Hoddle, M., and Center, T. (2008). Control of Pests and Weeds by Natural Enemies. Hoboken, NJ: Wiley-Blackwell.
Vavilov, N. I. (1926). Studies on the origin of cultivated plants. Bull. Appl. Bot. Plant Breed. 16, 139–245.
Vavilov, N. I. (1951). The origin, variation, immunity and breeding of cultivated plants. Chron. Bot. 13, 1–366. doi: 10.1097/00010694-195112000-00018
Vidal, S., Kuhlmann, U., and Edwards, C. R. (eds). (2005). Western Corn Rootworm: Ecology and Management. Wallingford, CT: CABI.
Xing, X., Koch, A. M., Jones, A. M. P., Ragone, D., Murch, S., and Hart, M. M. (2012). Mutualism breakdown in breadfruit domestication. Proc. R. Soc. London B Biol. Sci. 279, 1122–1130. doi: 10.1098/rspb.2011.1550
Zhang, H., Mittal, N., Leamy, L. J., Barazani, O., and Song, B. H. (2017). Back into the wild? Apply untapped genetic diversity of wild relatives for crop improvement. Evol. Appl. 10, 5–24. doi: 10.1111/eva.12434
Zhou, Y., Ma, Y., Zeng, J., Duan, L., Xue, X., Wang, H., et al. (2016). Convergence and divergence of bitterness biosynthesis and regulation in Cucurbitaceae. Nat. Plants 2:16183. doi: 10.1038/nplants.2016.183
Keywords: crop domestication, agroecology, evolutionary ecology, biogeography, epigenetics, human culture, insects, microbes
Citation: Chen YH, Shapiro LR, Benrey B and Cibrián-Jaramillo A (2017) Back to the Origin: In Situ Studies Are Needed to Understand Selection during Crop Diversification. Front. Ecol. Evol. 5:125. doi: 10.3389/fevo.2017.00125
Received: 24 June 2017; Accepted: 25 September 2017;
Published: 18 October 2017.
Edited by:
Alejandro Casas, Instituto de Investigaciones en Ecosistemas y Sustentabilidad, Universidad Nacional Autónoma de México, MexicoReviewed by:
Shabir Hussain Wani, Michigan State University, United StatesMukhtar Ahmed, Pir Mehr Ali Shah Arid Agriculture University, Pakistan
Copyright © 2017 Chen, Shapiro, Benrey and Cibrián-Jaramillo. This is an open-access article distributed under the terms of the Creative Commons Attribution License (CC BY). The use, distribution or reproduction in other forums is permitted, provided the original author(s) or licensor are credited and that the original publication in this journal is cited, in accordance with accepted academic practice. No use, distribution or reproduction is permitted which does not comply with these terms.
*Correspondence: Angélica Cibrián-Jaramillo, YW5nZWxpY2EuY2licmlhbkBnbWFpbC5jb20=