- 1Department of Biological Sciences, University of Tampa, Tampa, FL, USA
- 2Department of Biological Sciences, University of Maryland Baltimore Count, Baltimore, MD, USA
The underwater environment places unique constraints on the vision of cetaceans compared to their terrestrial mammalian counterparts. Water absorbs and filters light affecting both the intensity and spectral distribution of light available for vision. Therefore, the aquatic environment restricts the spectral distribution of photons and limits the distance at which objects may be observed. The cetacean eye possesses numerous anatomical, cellular and molecular adaptations to the underwater light environment that increase photon capture in a light-limited environment. These adaptations include a powerful spherical lens, a unique corneal design allowing for acute vision in both air and water, as well as a blue reflective optic tapetum. There are also molecular adaptations that influence the spectral sensitivity of both the rod and cone visual pigments. The spectral sensitivities of cetacean retinae have been a focus of attention over the last two decades. While most terrestrial mammals have dichromatic color vision based on two classes of cone photoreceptors, all cetaceans lack cone based color vision. For example, the Delphinidae (dolphins), Phocoenidae (porpoises), and some members of Ziphidae (beaked whales) possess single rod and cone photoreceptor classes. Recently, rod monochromats were identified in Ziphidae, Physeteroidea, and almost all of the mysticete (baleen) whales. The absorbance spectra of cetacean rod visual pigments are spectrally tuned to the available radiance spectra at foraging depths with an inverse relationship observed between the wavelength of maximum sensitivity of the rod pigment and depth. This also holds true for the spectral tuning of long-wavelength sensitive cone visual pigments in cetaceans. Cetacean melanopsins, the visual pigment expressed in a small subset of ganglion cells in mammalian retinae, have only just recently been examined in the cetacean rod monochromats. Genetic analyses coupled with molecular modeling predict that cetacean melanopsins possess nearly identical absorption spectra compared to their terrestrial mammalian counterparts. However, it appears that the melanopsins from the cetacean rod monochromats may possess a mechanism that inhibits relatively rapid deactivation of the light-activated melanopsin. This mechanism would result in prolonged pupil constriction resulting in a very useful mechanism in the prevention of photobleaching of rod pigments under photopic conditions.
Visual pigments are light absorbing molecules comprised of a light-sensitive chromophore covalently attached to an opsin protein. In vertebrates, chromophores consist of an aldehyde of either vitamin A1 or A2 (retinal), are bound to opsin through a protonated Schiff base (PSB) linkage, and lie within an amino acid-lined chromophore binding pocket (Figure 1). Although, a protonated chromophore attached to an amine via a Schiff's base has an absorbance maximum (λmax) well within the visible spectrum (around 440 nm; Figure 2), wavelength modulation of chromophore absorbance spanning the visible region of the spectrum relies almost exclusively on the amino acids lining the chromophore binding pocket and their dipolar electrostatic interactions with the chromophore (Kochendoerfer et al., 1999). Simply put, amino acid side chains that localize the Schiff base proton at the Schiff base result in blue-shifting of the visual pigment, while side chains that delocalize the Schiff base proton result in red-shifting of the visual pigment. Although, one may expect to see an endless number of amino acid substitutions within the retinal binding pocket to account for the diversity of vertebrate rod and cone photoreceptor sensitivities, in fact many amino acid substitutions have been strongly conserved amongst the vertebrate rod and cone photoreceptor classes (see Yokoyama, 2008 for review). As an example, extreme blue-shifting of a visual pigment, as is found in vertebrates possessing ultraviolet (UV) cone visual pigments, results from the deprotonation of the Schiff base resulting in a pigment similar in its absorbance to that of 11-cis retinal free in solution (λmax ~ 380 nm; Figure 2). Deprotonation of the Schiff base in these pigments relies on a key amino acid substitution in both mammals and birds, the former possessing the substitution Tyr86Phe, with the latter possessing the substitution Ser84Cys (Wilkie et al., 2000; Yokoyama et al., 2000; Shi et al., 2001; Cowing et al., 2002; Fasick et al., 2002). Although, cetaceans lack a functional SWS cone, these marine mammals nevertheless have acquired several amino acid substitutions in both their rod and LWS cone visual pigments that influence the absorbance spectra of these pigments when compared to corresponding pigments from terrestrial mammals.
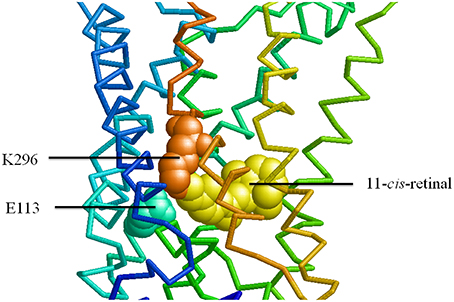
Figure 1. Proximal positions of chromophore, Schiff base linkage, and counterion in rhodopsin. Bovine rhodopsin (Protein Data Bank accession: 1F88; Palczewski et al., 2000) was modeled using RasWin to highlight positions E113 (counterion), K296 (site of Schiff base linkage) and retinal.
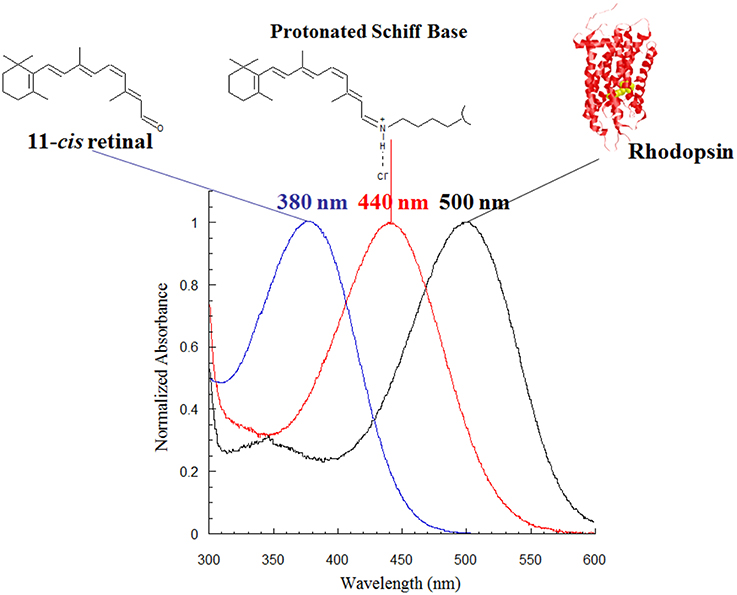
Figure 2. Absorbance spectra of the visual pigment chromophore, 11-cis retinal, in three states: free in solution (left); protonated in the presence of chloride ions (middle); surrounded by the bovine RH1 opsin protein (right).
Cetacean Visual Pigments
Although, the first published spectral sensitivity measurement of a cetacean retina was made by Dartnall on the humpback whale (maximum sensitivity of 492 nm; Dartnall, 1962), the first comprehensive study of cetacean retinal sensitivity and the supporting visual pigments was conducted by McFarland. He examined 10 species, including both odontocetes (toothed whales) and mysticetes (baleen whales), using a method of partial bleaching to estimate the absorption maxima and homogeneity of visual pigments in the extracts (McFarland, 1971). From this early work, McFarland reported blue-shifted absorption maxima (relative to ungulates) for all species ranging from 497 nm in gray whale to 481 nm in beaked whale. Although, only two mysticete species were examined (gray and humpback whales) both possessed retinal absorbance values greater than 490 nm, while all of the odontocete species studied possessed retinal absorbance values less than 490 nm. In regards to cetaceans, McFarland was the first to report the correlation between diving (foraging) depth and spectral sensitivity of the retinal visual pigments, e.g., the deep-diving pelagic species will have correspondingly more blue-shifted visual pigments compared to shallow-diving species. As McFarland states, selective forces most likely resulted in a balance being struck between the maximization of spectral sensitivity and the degree in which contrast cues may be utilized in visually guided tasks.
Several years after McFarland's work, a series of behavioral studies were conducted on bottlenose dolphin (Tursiops truncatus) in order to test spectral discrimination and determine if the dolphin possesses color vision, as well as define the spectral sensitivity of the dolphin retina under both scotopic and photopic light levels (Madsen, 1976). From these studies, Madsen, along with her graduate adviser Louis Herman, was able to demonstrate that the dolphin lacked the ability to discriminate colors and was indeed color-blind (Madsen, 1976). The rod and cone photoreceptor sensitivities were deduced by Madsen by having the dolphin perform light illumination discrimination tasks under either scotopic or photopic light levels where rod and cone photoreceptors typically function, respectively. Interestingly, the dolphin's retinal sensitivity was quite similar under both illumination conditions with scotopic and photopic retinal sensitivity maxima found at 495 and 500 nm, respectively. Both of these values are red-shifted relative to the retinal absorbance maximum observed by McFarland (1971) for the bottlenose dolphin (486 nm).
More recent studies of cetacean visual pigments address the number of different retinal photoreceptor classes as well as the molecular mechanisms of spectral tuning of both the rod and cone photoreceptors. Below we review a series of papers from several laboratories that have contributed to our current understanding of the nature of the retinae of cetaceans as well as other marine mammals.
Fasick et al. used molecular techniques to confirm the absence of color vision in the bottlenose dolphin as well as to describe the molecular mechanism underlying the spectral tuning of both the rod and cone photoreceptors from several cetacean species (Fasick et al., 1998; Fasick and Robinson, 1998, 2000). Their approach involved screening a dolphin retinal bacteriophage cDNA library with radiolabeled human homologs of the rod opsin, as well as the short-wavelength (SWS) and long-wavelength sensitive (LWS) cone opsins, open reading frames to determine the number of different photoreceptor classes in the dolphin retina. This approach resulted in the cloning, sequencing and expression of both the rod and LWS cone visual pigments (λmax of 488 nm, and 524 nm respectively), but failed to isolate SWS cone opsin cDNA (Fasick et al., 1998).
Sequence comparisons between the dolphin and bovine rod opsin sequences revealed three likely amino acid substitutions involved in the complete wavelength modulation between the two pigments. The positions of these substitutions were 83, 292, and 299 all of which fell within the transmembrane region of the proposed opsin structure, with positions 292 and 299 positioned approximately one helical turn away from Lys296, the site of the protonated Schiff base linkage with the chromophore (Fasick et al., 1998). A series of bovine mutants were constructed and expressed to determine the degree of wavelength modulation made by individual amino acid substitutions at these positions as well as the combination of all three substitutions together (see Table 1). The single bovine mutant Asp-to-Asn at position 83 resulted in a 4 nm blue-shift relative to wildtype bovine rhodopsin (λmax = 499 nm), while the individual Ala-to-Ser mutations in bovine rhodopsin at positions 292 and 299 resulted in a 10 nm blue-shift and a 2 nm red-shift, respectively. When the three amino acid substitutions were included together in a single mutant construct, the triple-mutant resulted in a λmax = 489 nm resulting in a 10 nm blue-shift relative to bovine rhodopsin (Fasick and Robinson, 1998). A subsequent study observed a 12 nm blue-shift in bottlenose dolphin rhodopsin relative to bovine rhodopsin which is in better agreement with the original values from McFarland (1971) and Bischoff et al. (2012). Variations of these three substitutions at positions 83, 292, and 299 are found in a variety of cetacean rhodopsins and appear to correspond with foraging depth (Figure 3). Deep-diving odontocetes such as the sperm whale and the beaked whales possess the combination of Asn83, Ser292, and Ala299 with resulting absorbance maxima around 479 nm (Bischoff et al., 2012). By contrast, as outlined in Figure 4, the more shallow diving and coastal Balaenidae whales possess the combination of Asn83, Ala292, and Ser299 with resulting absorbance maxima around 493 nm, while the more oceanic Balaenopteridae possess the combination of Asn83, Ser292, and Ser299 with resulting absorbance maxima around 484 nm similar to the delphinidae rhodopsins (Bischoff et al., 2012). Curiously, the pygmy right whale possesses the same amino acid substitutions at positions 83, 292, and 299 as the sperm and beaked whales, Asn, Ser, Ala, respectively, suggesting the possibility that this baleen whale species is adapted to sensing blue spectral light found at great depths. Very little is known of the foraging ecology of this rare species of whale, but it would be of great interest to explore this hypothesis further.
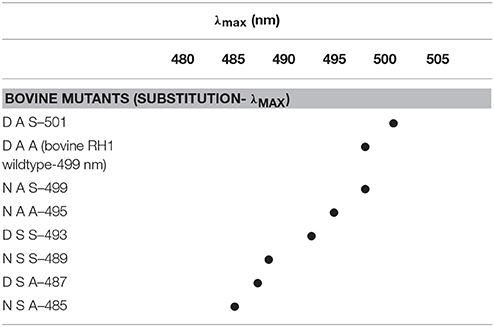
Table 1. Comparison of substitutions at positions 83, 292, and 299 in bovine opsin mutants with corresponding absorbance maxima (λmax; from Fasick and Robinson, 2000).
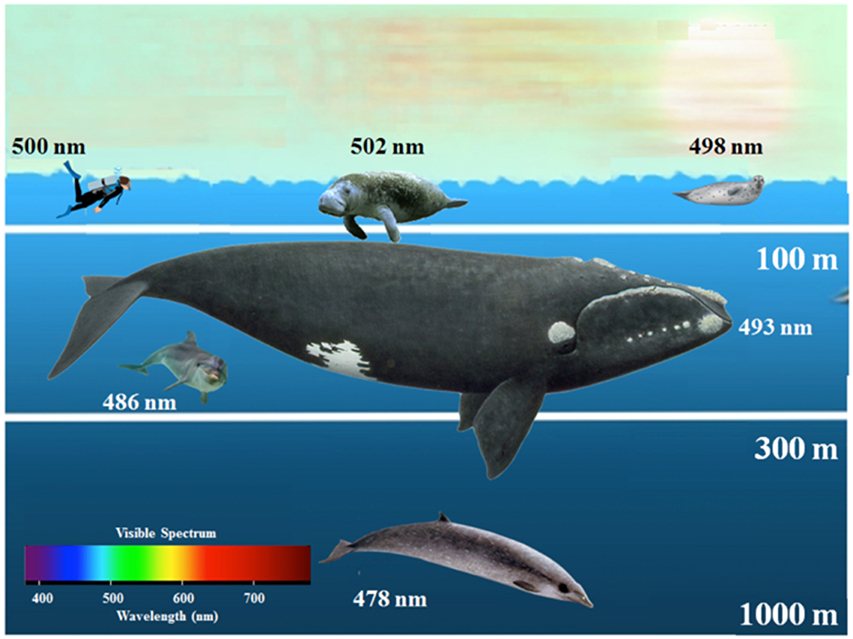
Figure 3. Marine mammal rhodopsin absorbance maxima relative to foraging depth. Diagrammed marine organisms possess the following amino acids at positions 83, 292, and 299: from left to right are: human: D-A-A, manatee; D-A-S, harp seal; D-A-A, from top to bottom are; bowhead whale; N-A-S, bottlenose dolphin; N-S-S, beaked whale; N-S-A. Figure adapted from Fasick (2009).
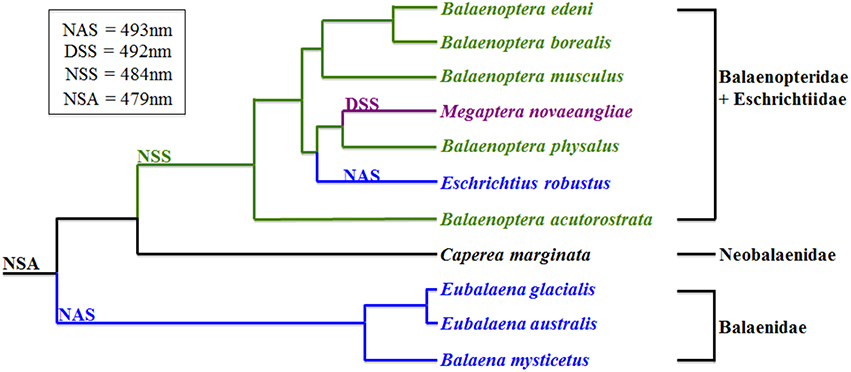
Figure 4. Amino acid substitutions at positions 83, 292, and 299 in 11 mysticete rod opsins. Figure adapted from Bischoff et al. (2012). Phylogenetic tree adapted from McGowen et al. (2009).
To address the status of the dolphin SWS cone opsin, Fasick et al. used genomic DNA (gDNA) and PCR amplified the entire dolphin SWS cone opsin gene, subsequently aligning the sequence with the homologous bovine SWS cone opsin gene (Fasick et al., 1998). The sequence analysis of the dolphin SWS cone opsin gene revealed two important features: first, the deduced amino acid sequence resulted in a premature stop codon in exon1; and second, intron 4 was absent with exons 4 and 5 being uninterrupted in the dolphin gene (Fasick et al., 1998). This finding of a dolphin SWS cone opsin pseudogene confirmed the conclusion of Fasick et al. that the bottlenose dolphin lacks any cone photoreceptor form of color vision and the earlier behavioral observations of Madsen and Herman (Madsen, 1976). It is important to note that Fasick et al. failed to isolate retinal cDNA during their initial screening of the retinal cDNA phage library, strongly suggesting the absence of the dolphin SWS cone photoreceptors altogether. This finding turns out to be important as seen in the subsequent discussion of rod monochromacy, and is supported by experimental evidence discussed below.
Two independent groups followed these studies and showed that the scope of SWS cone photoreceptor inactivation was characteristic of the entire cetacean infraorder. Peichl et al. utilized visual pigment-specific antibodies to demonstrate an absence of SWS cone photoreceptors in the retinae of six dolphin species and one porpoise species, as well as in five species of marine carnivore (eared and earless seals; Peichl et al., 2001). The authors suggest that the loss of the SWS cone class from these two distant mammalian orders is due to convergent evolution resulting in an adaptive advantage for this trait (Peichl et al., 2001). These results were confirmed by Levenson and Dizon who examined the SWS cone opsin genes from 16 cetacean species that represented 12 of the 14 extant mysticete and odontocete families (Levenson and Dizon, 2003). Levenson and Dizon also examined SWS cone opsin coding sequences that were PCR amplified from cetacean gDNA and observed, for all species examined, one or more mutations indicating that these sequences were indeed SWS cone opsin pseudogenes.
Although, much work has been done examining the nature of cetacean color vision, or lack thereof, with studies of the SWS cone opsin pseudogenes, very little work has focused on the cetacean LWS cone opsins. The bottlenose dolphin LWS cone visual pigment possesses an absorption maximum (λmax) of 524 nm that is significantly blue-shifted relative to the LWS cone pigments from terrestrial mammals (e.g., bovine LWS cone λmax = 555 nm; Fasick et al., 1998). Likewise, pilot whale (λmax = 531 nm) and harbor porpoise (λmax = 522 nm) share this trait of a blue-shifted LWS cone visual pigment (Newman and Robinson, 2005). Like cetacean rod opsins, cetacean LWS cone opsins have an alanine to serine substitution at position 292 (using the bovine rhodopsin numbering system). Although, positions 83 and 299 do not appear to play a role in wavelength modulation in these LWS cone pigments, all functional cetacean LWS opsins studied to date possess a serine at position 292 replacing an alanine most commonly found in their terrestrial mammalian counterparts.
In 2013 Meredith et al. published a landmark paper reporting that many cetacean species lacked functional LWS cone opsins. These species have an exclusively rod-only retina in the absence of functional expression of both the SWS and LWS cone opsins making them rod monochromats. Why would this occur in species that are often active during daytime hours and frequently observed foraging in the upper regions of the water column, even at the surface as is the case with the North Atlantic right whale? The following section sheds some light on the nature of rod monochromacy in terms of evolution, adaptation, as well as the role that cone photoreceptors may continue to play in rod monochromat whales.
Cetacean Rod Monochromats
McFarland first postulated that cetaceans may possess predominantly, if not exclusively, a pure rod retina (McFarland, 1971). As Meredith et al. have shown, this is the case for most of the mysticete species, as well as with Physeteroidea and the Mesoplodont beaked whales, with retinae being exclusively rod-based due to both the SWS and LWS opsin pseudogenes (Meredith et al., 2013). Furthermore, the mutations in the LWS cone opsin gene in Cetacea is an example of convergent evolution as mutations resulting in the cetacean pseudogenes arose at least five different times (Meredith et al., 2013). Based on genomic DNA sequence analyses, the authors placed the timing of rod opsin blue-shifts prior to the formation of the SWS pseudogene with both occurring early in the history of Cetacea (see Meredith et al., 2013 for details on divergence times and appearance of SWS pseudogenes).
Interestingly, the pygmy right whale retains a functional LWS cone opsin gene based on genomic evidence (Meredith et al., 2013). As discussed above, the pygmy right whale's rod visual pigment possesses the “deep-diving” set of amino acid substitutions at positions 83, 292, and 299 and a predicted absorbance maximum around 480 nm. These are the same amino acid substitutions found in both the sperm and beaked whales, all of which are deep-divers. Furthermore, many of the Delphinoidea and Ziphiidae species examined by Meredith et al., and which possess functional LWS cones, routinely dive deeper than some of the mysticete species such as right, gray, and minke whales, all of which lack functional LWS cones (e.g., Tyack et al., 2006; Parks et al., 2012). Although the premise that a rod-dominated retina is advantageous in dim light conditions is certainly reasonable, arguments can be made to counter this premise when considering the deep-diving behaviors of some oceanic Delphinoidea and Ziphiidae species, and possibly Neobalaenidae, as well as the common shallow-diving behaviors of several of the Balaenidae, Eschrichtiidae, and Balaenopteridae species.
Although it is clearly evident that many cetacean species lack a functional LWS visual pigment, this does not necessarily preclude the anatomical formation of cone photoreceptors, or more correctly, non-photosensitive cone cells (NPCCs). A recent study by Schweikert et al. proposed that cone somata and pedicles may have been conserved in rod monochromats to maintain an alternative rod photoreceptor signaling pathway in the retinae (Schweikert et al., 2016). Preliminary supporting evidence for the presence of NPCCs was first observed in molecular experiments with successful PCR amplification of the LWS cone opsin pseudogene from North Atlantic right whale retinal cDNA. As diagrammed in Figure 5, sequence analysis revealed several deleterious mutations in the LWS opsin coding sequence, including the excision of exon 4 with exons 3 and 5 being spliced together and deletion mutations in the core promoter region while other regions in the gene remained intact such as the polyadenylation sequence. Likewise, Emerling and Springer provided evidence of inactivating mutations in several cone phototransduction genes from both minke whale and giant sperm whale (Emerling and Springer, 2015). Further support of the pseudogenization of the LWS, as well as SWS, cone opsin gene resulted from immunohistochemical experiments where anti-opsin immunofluorescence demonstrated the total loss of cone opsin expression in the retina of the closely related species B. mysticetus (Schweikert et al., 2016). Taken together, both the genetic and immunohistochemical evidence suggested a loss of functional visual pigment expression, but maintenance of the LWS NPCC, at least in Balaenidae. Direct evidence of cone maintenance resulted from imaging of cone soma and putative cone pedicles by light and electron microscopy, and of rod and cone bipolar cell types by immunofluorescence microscopy in the bowhead whale retina (Schweikert et al., 2016). Schweikert et al. proposed three distinct rod signaling pathways that may increase the signaling speed and sensitivity range of rod-based vision in at least the balaenid whales: (1) rods synapse on rod bipolar cells (scotopic sensitivity); (2) cone soma are synaptic intermediates in a coupling pathway between rod and ON cone bipolar cells (intermediate mesopic-light sensitivity); and (3) rods synapse directly on OFF cone bipolar cells (supporting visual processes such as center-surround receptive fields). Although, Schweikert et al. examined only two balaenid species; it would not be surprising if other cetacean rod monochromats shared a similar retinal design.
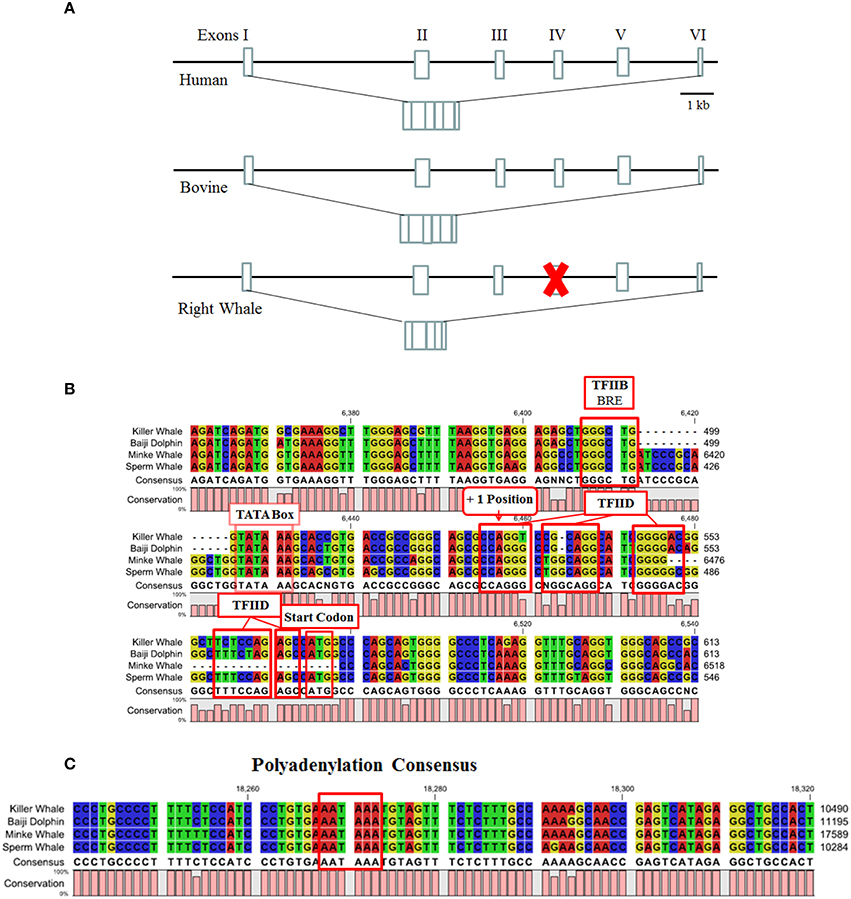
Figure 5. (A) Structure of the North Atlantic right whale LWS opsin pseudogene. Coding regions of exons are represented by boxes. The human and bovine LWS opsin genes are used for comparison. Figure is based after (Nathans et al., 1986). (B) Core promoter element of cetacean LWS opsin genes. Highlighted are the core promoter elements for transcription factors IIB (BRE) and IID, including the TATA binding element for the TATA binding protein (TBP), and start codon. (C) Consensus cetacean LWS opsin gene polyadenylation sequence.
This then raises the question of how these rod monochromats are capable of vision during bright-light daytime levels at or near the surface of the water without bleaching the rod photoreceptors? One answer to this question may be found in the pupillary light response (PLR) that is involved in constricting or dilating the pupil. The PLR is regulated by input from a subset of retinal ganglion cells that project to the olivary pretectal nucleus. These ganglion cells not only receive indirect synaptic input from classical rod and cone photoreceptors but also function as photoreceptors themselves. These ganglion cells known as intrinsically photosensitive ganglion cells (iPRGCs) express the pigment OPN4, or melanopsin. Despite the ability of rods and cones to drive the PLR, melanopsin is critical for normal PLR function in allowing maximal pupil constriction in bright and sustained light intensities (Lucas et al., 2003; Xue et al., 2011). In animals lacking cones, as is the case with the rod monochromatic whales, iPRGCs and melanopsin appear critical in the regulation of the PLR. A prolonged PLR under bright illumination might ensure the viability of rod-based vision.
Cetacean Melanopsins (OPN4): Wavelength Modulation and Inactivation
In the mammalian retina, a small subset of retinal ganglion cells express the photopigment melanopsin and represent a third class of photoreceptors that predominantly mediate non-image forming light functions. In terrestrial mammals, these intrinsically photosensitive retinal ganglion cells (ipRGCs) provide photic information for a number of light-dependent processes, including circadian photoentrainment, pupil constriction, suppression of pineal melatonin, and direct regulation of sleep, mood, and learning (Altimus et al., 2008; Lupi et al., 2008; Tsai et al., 2009; Schmidt et al., 2011a,b; LeGates et al., 2012; Pickard and Sollars, 2012). Recently, it was discovered that ipRGCs are also involved in image forming vision where they contribute to setting contrast sensitivity (Schmidt et al., 2014). ipRGCs differ from the classical rod and cone photoreceptors in both physiology, and the light-activated biochemical cascade. Melanopsin-expressing ipRGCs were identified only 15 years ago (Provencio et al., 1998, 2000). Since then, they have been the subject of intense research primarily in terrestrial dichromatic mammals, such as rodents, with significant advances having been made in elucidating the anatomy and functions of ipRGCs, as well as the rod/cone input to these cells (Lucas et al., 2012; Pickard and Sollars, 2012). However, there is a major gap in our knowledge of the role of ipRGCs in mammals that have adapted to dim light environments and are rod monochromats, such as the cetaceans.
The first vertebrates appeared approximately 500 mya and the vertebrate visual system evolved into both image and non-image forming vision (Gerkema et al., 2013). The hypothesized nocturnal bottleneck in mammalian evolution influenced the evolution of therian mammalian vision with the loss of at least 3 classes of opsins (SWS2, Rh2, and OPN4x) (Gerkema et al., 2013; Jacobs, 2013). Therefore, the typical mammal has two cone visual pigments (LWS and SWS1); one rod visual pigment (Rh1) and one melanopsin visual pigment (OPN4m). The radiation of mammals into specialized environmental niches has resulted in further adaptations of the mammalian visual system. For instance, the evolution of cone trichromacy occurred in old world primates (Jacobs, 2008; Hofmann and Palczewski, 2015). Other mammals occupy dim light environments and are either cone monochromats or rod monochromats (Meredith et al., 2013; Emerling and Springer, 2015).
Melanopsin exhibits a higher sequence homology to visual pigments from rhabdomeric photoreceptors (Provencio et al., 1998, 2000) than mammalian cone and rod visual pigments (Figure 6). Unlike the cetacean rod visual pigments which are spectrally tuned to various underwater photic environments, all melanopsin pigments characterized to date have a fairly uniform absorption maximum centered around 480 nm. Amino acid sequence alignments of cetacean and terrestrial melanopsins reveal very few non-conserved amino acid substitutions that would result in any significant divergence away from this typical absorption maximum (Figures 7, 8). However, there is divergence between the cetacean and terrestrial mammalian melanopsins at the carboxyl tail, specifically at the sites of phosphorylation that are involved in pigment inactivation (Fasick and Samuels, 2015).
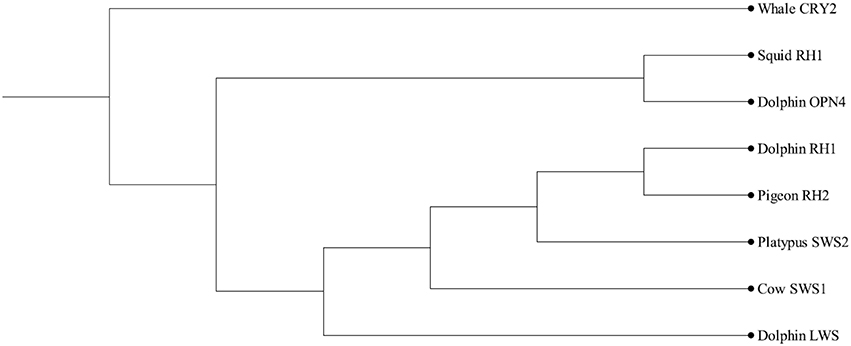
Figure 6. Dendrogram of opsins and melanopsin. CRY, cryptochrome (outgroup); RH, rhodopsin (Squid rhabdomeric opsin, dolphin rod opsin, pigeon cone opsin); OPN4, melanopsin; SWS, short wavelength-sensitive; LWS, long wavelength-sensitive. After (Sexton et al., 2012).
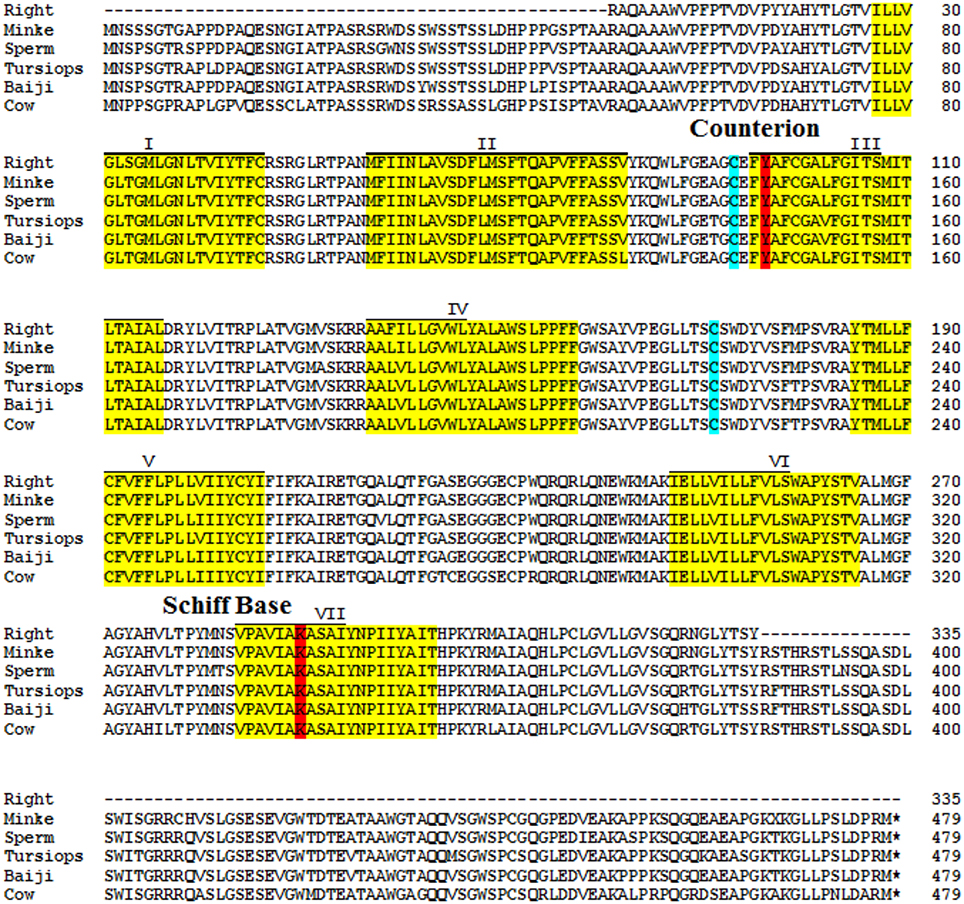
Figure 7. Amino acid alignment of cetacean melanopsins. Transmembrane regions boxed in yellow, Schiff base and Schiff base counterion boxed in red, disulfide bridge cysteines boxed in teal. Figure adapted from Fasick and Samuels (2015).
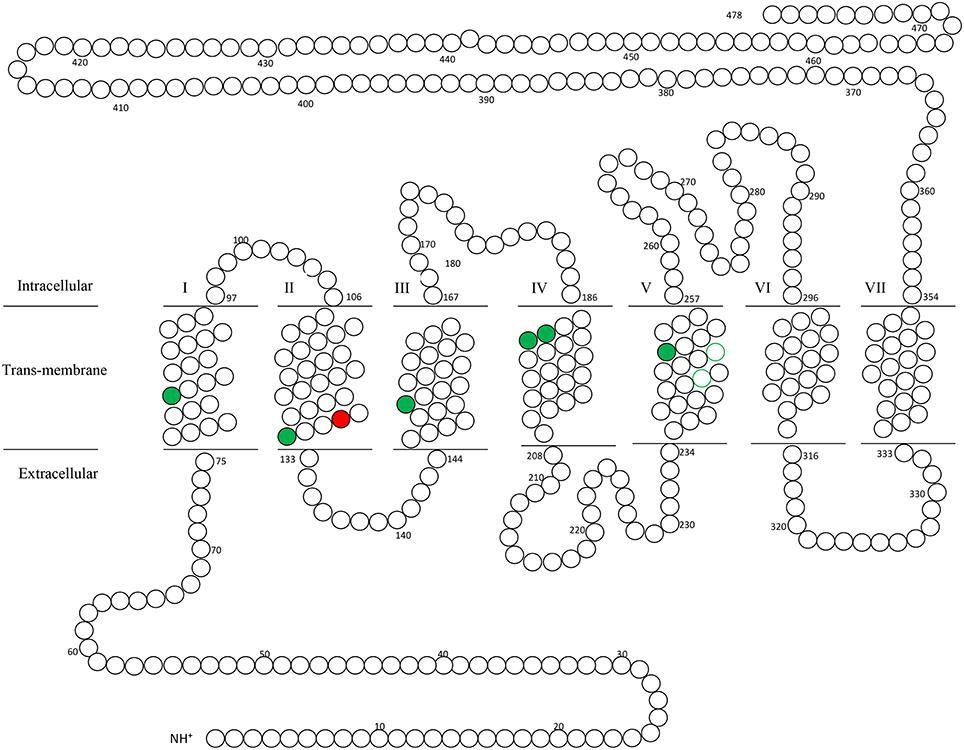
Figure 8. Secondary structure of cetacean melanopsin. Conserved amino acid substitutions between North Atlantic right whale, minke whale, sperm whale, bottlenose dolphin and baiji dolphin are labeled green, while the single nonconserved substitution occurring in baiji dolphin (A129T) is labeled red. Figure adapted from Fasick and Samuels (2015).
The biochemical kinetics of melanopsin activation and deactivation is also in marked contrast to that of both the vertebrate rod and cone visual pigments. As first described by Berson et al. (2002), the OPN4 light response is extremely slow when compared to that of either rods or cones with deactivation being even slower. The “sluggish” light activation of melanopsin postulated by Blasic et al. (2012) is intuitive to this retinal pigment class based on its expression in retinal ganglion cells. Unlike the rod and cone photoreceptors that evolved membranous stacks, as in rods, or invaginations, as is the case in cones, which dramatically increase the membranous surfaces in which visual opsins can integrate, the retinal ganglion cells, by contrast, are composed of a single outer plasma membrane in which integration of melanopsins occurs. This reduction in membrane surface to a single membrane limits the number of melanopsin molecules expressed in each cell. Along with the fact that melanopsin is expressed in only ~2% of retinal ganglion cells, we begin to appreciate the large number of photons that must strike the retinal surface in order to activate these cells.
Although, significant advances have been made in elucidating the anatomy and functions of ipRGCs as well as the rod/cone input to these cells (Schmidt et al., 2011b; Sexton et al., 2012) our knowledge of the mechanisms underlying the deactivation of ipRGC light responses and the behavioral consequences remain rudimentary. The light response in rods and cones terminates by phosphorylation of the C-terminus of rod and cone opsins by a G-protein receptor kinase (GRK), followed by the binding of visual arrestin. Mouse melanopsin, which has been the subject of much research, has a C-terminus that is 4 times longer than that of rod and cone opsins, containing 171 amino acids with 38 potential phosphorylation sites. Previous investigations have found that in a heterologous expression system the C-terminus phosphorylation of mouse melanopsin regulates the shutoff of the light response (Blasic et al., 2012, 2014). Importantly, this phosphorylation event has significant behavioral consequences as the light-induced pupil constriction is dramatically prolonged in mice expressing a phosphorylation-deficient melanopsin (Somasunda, personel communication). It can be hypothesized then that melanopsin expressed in the retina of cetacean rod monochromats will have a compliment of amino acids in the melanopsin C-terminus that result in slow deactivation kinectics (see Figure 9). This slow deactivation of melanopsin will allow these animals to maintain prolonged pupil constriction when they are actually exposed to bright lights thus protecting the rod photoreceptors from photobleaching.
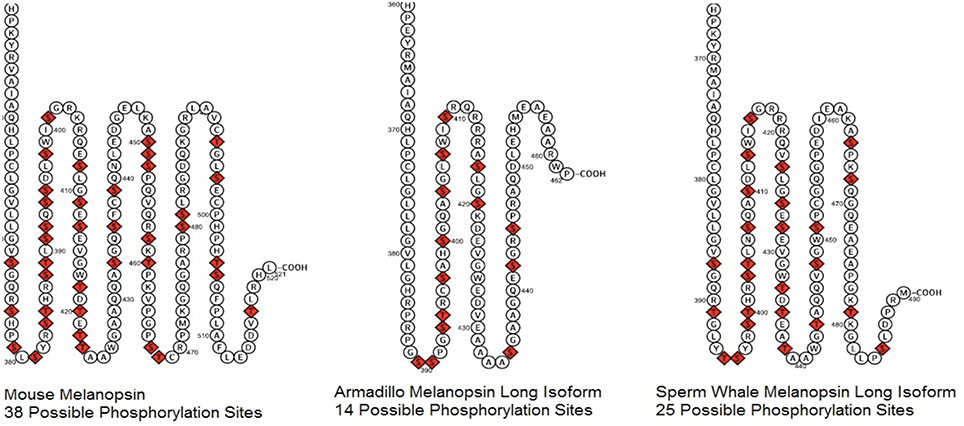
Figure 9. Two dimensional diagram of Melanopsin C-terminus from mouse, armadillo, and sperm whale. Possible phosphorylation sites, serines, and threonines, are indicated in red.
Currently, we know nothing of the role of melanopsin and ipRGCs in cetaceans. Future studies should examine the C-termini of the cetacean melanopsins, specifically the number of phosphorylation sites that presumably are involved with deactivation. It is feasible to hypothesize that the rod monochromat cetacean melanopsin pigments will have slower deactivation kinetics when compared to melanopsins from mammalian rod/cone dichromats. Slower deactivation of these pigments would provide prolonged afferent signaling from ipRGCs to physiologically (and behaviorally) relevant brain nuclei in cetacean rod monochromats.
Summary
Understanding the evolution of vision is a key question in both evolutionary biology and vision science. A combination of morphological, genetic, molecular, functional and physiological data is bringing a new level of insight into a field of inquiry that dates back to Darwin (Lamb, 2013; Nilsson, 2013). In the era of genomics, the genetic sequence of the molecules involved in phototransduction, especially visual pigments, have contributed significant insight into this problem. The typical terrestrial mammal has two cone visual pigments (LWS and SWS1); one rod visual pigment (Rh1) and one melanopsin visual pigment (OPN4m). The radiation of mammals into specialized environmental niches such as the ocean has resulted in further adaptations of the mammalian visual system. Cetaceans are an example of an evolving visual system that has adapted to the visual pressures of an aquatic environment and foraging at various depths.
Author Contributions
All authors listed, have made substantial, direct and intellectual contribution to the work, and approved it for publication.
Conflict of Interest Statement
The authors declare that the research was conducted in the absence of any commercial or financial relationships that could be construed as a potential conflict of interest.
Acknowledgments
Funding to JF was provided by the Bycatch Reduction Engineering Program, Department of Commerce/NOAA-NMFS-FHQ-2012-2003362. Funding to PR was provided by the National Science Foundation (IOS0721608) and the National Eye Institute (R01EY019053). We would like to thank Courtland Samuels and Juan Valdez for their assistance with figure construction.
References
Altimus, C. M., Guler, A. D., Villa, K. L., McNeill, D. S., Legates, T. A., and Hattar, S. (2008). Rods-cones and melanopsin detect light and dark to modulate sleep independent of image formation. Proc. Natl. Acad. Sci. U.S.A. 105, 19998–20003. doi: 10.1073/pnas.0808312105
Berson, D. M., Dunn, F. A., and Takao, M. (2002). Phototransduction by retinal ganglion cells that set the circadian clock. Science 295, 1070–1073. doi: 10.1126/science.1067262
Bischoff, N., Nickle, B., Cronin, T. W., Velasquez, S., and Fasick, J. I. (2012). Deep-sea and pelagic rod visual pigments identified in the mysticete whales. Vis. Neurosci. 29, 95–103. doi: 10.1017/S0952523812000107
Blasic, J. R. Jr., Lane Brown, R., and Robinson, P. R. (2012). Light-dependent phosphorylation of the carboxy tail of mouse melanopsin. Cell. Mol. Life Sci. 69, 1551–1562. doi: 10.1007/s00018-011-0891-3
Blasic, J. R. Jr., Matos-Cruz, V., Ujla, D., Cameron, E. G., Hattar, S., Halpern, M. E., et al. (2014). Identification of critical phosphorylation sites on the carboxy tail of melanopsin. Biochemistry 53, 2644–2649. doi: 10.1021/bi401724r
Cowing, J. A., Poopalasundaram, S., Wilkie, S. E., Robinson, P. R., and Bowmaker, J. K. (2002). The molecular mechanism for the spectral shifts between vertebrate ultraviolet-and violet-sensitive cone visual pigments. Biochem. J. 367, 129–135. doi: 10.1042/bj20020483
Dartnall, H. (1962). The identity and distribution of visual pigments in the animal kingdom. Eye 2, 367–426.
Emerling, C. A., and Springer, M. S. (2015). Genomic evidence for rod monochromacy in sloths and armadillos suggests early subterranean history for Xenarthra. Proc. Biol. Sci. 282:20142192. doi: 10.1098/rspb.2014.2192
Fasick, J. I. (2009). Visual processes in dolphins and whales: investigations of dolphin and whale retinal pigments. Soundings 34, 14–17.
Fasick, J. I., Applebury, M. L., and Oprian, D. D. (2002). Spectral tuning in the mammalian short-wavelength sensitive cone pigments. Biochemistry 41, 6860–6865. doi: 10.1021/bi0200413
Fasick, J. I., Cronin, T. W., Hunt, D. M., and Robinson, P. R. (1998). The visual pigments of the bottlenose dolphin (Tursiops truncatus). Vis. Neurosci. 15, 643–651. doi: 10.1017/S0952523898154056
Fasick, J. I., and Robinson, P. R. (1998). Mechanism of spectral tuning in the dolphin visual pigments. Biochemistry 37, 433–438. doi: 10.1021/bi972500j
Fasick, J. I., and Robinson, P. R. (2000). Spectral-tuning mechanisms of marine mammal rhodopsins and correlations with foraging depth. Vis. Neurosci. 17, 781–788. doi: 10.1017/S095252380017511X
Fasick, J. I., and Samuels, C. R. (2015). “Marine Mammal Melanopsins (OPN4): molecular characterization and wavelength modulation,” in 21st Biennial of the Society for Marine Mammalogy (San Francisco, CA).
Gerkema, M. P., Davies, W. I., Foster, R. G., Menaker, M., and Hut, R. A. (2013). The nocturnal bottleneck and the evolution of activity patterns in mammals. Proc. R. Soc. Lond. B Biol. Sci. 280:20130508. doi: 10.1098/rspb.2013.0508
Hofmann, L., and Palczewski, K. (2015). Advances in understanding the molecular basis of the first steps in color vision. Prog. Retin. Eye Res. 49, 46–66. doi: 10.1016/j.preteyeres.2015.07.004
Jacobs, G. H. (2008). Primate color vision: a comparative perspective. Vis. Neurosci. 25, 619–633. doi: 10.1017/S0952523808080760
Jacobs, G. H. (2013). Losses of functional opsin genes, short-wavelength cone photopigments, and color vision–a significant trend in the evolution of mammalian vision. Vis. Neurosci. 30, 39–53. doi: 10.1017/S0952523812000429
Kochendoerfer, G. G., Lin, S. W., Sakmar, T. P., and Mathies, R. A. (1999). How color visual pigments are tuned. Trends Biochem. Sci. 24, 300–305. doi: 10.1016/S0968-0004(99)01432-2
Lamb, T. D. (2013). Evolution of phototransduction, vertebrate photoreceptors and retina. Prog. Retin. Eye Res. 36, 52–119. doi: 10.1016/j.preteyeres.2013.06.001
LeGates, T. A., Altimus, C. M., Wang, H., Lee, H. K., Yang, S., Zhao, H., et al. (2012). Aberrant light directly impairs mood and learning through melanopsin-expressing neurons. Nature 491, 594–598. doi: 10.1038/nature11673
Levenson, D., and Dizon, A. (2003). Genetic evidence for the ancestral loss of short-wavelength-sensitive cone pigments in mysticete and odontocete cetaceans. Proc. R. Soc. Lond. B Biol. Sci. 270, 673–679. doi: 10.1098/rspb.2002.2278
Lucas, R., Hattar, S., Takao, M., Berson, D., Foster, R., and Yau, K.-W. (2003). Diminished pupillary light reflex at high irradiances in melanopsin-knockout mice. Science 299, 245–247. doi: 10.1126/science.1077293
Lucas, R. J., Lall, G. S., Allen, A. E., and Brown, T. M. (2012). How rod, cone, and melanopsin photoreceptors come together to enlighten the mammalian circadian clock. Prog. Brain Res. 199, 1–18. doi: 10.1016/B978-0-444-59427-3.00001-0
Lupi, D., Oster, H., Thompson, S., and Foster, R. G. (2008). The acute light-induction of sleep is mediated by OPN4-based photoreception. Nat. Neurosci. 11, 1068–1073. doi: 10.1038/nn.2179
Madsen, C. (1976). Tests for Color Discrimination and Spectral Sensitivity in the Bottlenosed Dolphin, Tursiops Truncatus. Ph.D. thesis, University of Hawaii, Manoa.
McFarland, W. N. (1971). Cetacean visual pigments. Vis. Res. 11, 1065–IN1062. doi: 10.1016/0042-6989(71)90113-1
McGowen, M. R., Spaulding, M., and Gatesy, J. (2009). Divergence date estimation and a comprehensive molecular tree of extant cetaceans. Mol. Phylogenet. Evol. 53, 891–906 doi: 10.1016/j.ympev.2009.08.018
Meredith, R. W., Gatesy, J., Emerling, C. A., York, V. M., and Springer, M. S. (2013). Rod monochromacy and the coevolution of cetacean retinal opsins. PLoS Genet. 9:e1003432. doi: 10.1371/journal.pgen.1003432
Nathans, J., Thomas, D., and Hogness, D. S. (1986). Molecular genetics of human color vision: the genes encoding blue, green, and red pigments. Science 232, 193–202. doi: 10.1126/science.2937147
Newman, L. A., and Robinson, P. R. (2005). Cone visual pigments of aquatic mammals. Vis. Neurosci. 22, 873–879. doi: 10.1017/S0952523805226159
Nilsson, D.-E. (2013). Eye evolution and its functional basis. Vis. Neurosci. 30, 5–20. doi: 10.1017/S0952523813000035
Palczewski, K., Kumasaka, T., Hori, T., Behnke, C. A., Motoshima, H., Fox, B. A., et al. (2000). Crystal structure of rhodopsin: AG protein-coupled receptor. Science 289, 739–745. doi: 10.1126/science.289.5480.739
Parks, S. E., Warren, J. D., Stamieszkin, K., Mayo, C. A., and Wiley, D. (2012). Dangerous dining: surface foraging of North Atlantic right whales increases risk of vessel collisions. Biol. Lett. 8, 57–60. doi: 10.1098/rsbl.2011.0578
Peichl, L., Behrmann, G., and KroÈger, R. H. (2001). For whales and seals the ocean is not blue: a visual pigment loss in marine mammals. Eur. J. Neurosci. 13, 1520–1528. doi: 10.1046/j.0953-816x.2001.01533.x
Pickard, G. E., and Sollars, P. J. (2012). Intrinsically photosensitive retinal ganglion cells. Rev. Physiol. Biochem. Pharmacol. 162, 59–90. doi: 10.1007/112_2011_4
Provencio, I., Jiang, G., De Grip, W. J., Hayes, W. P., and Rollag, M. D. (1998). Melanopsin: an opsin in melanophores, brain, and eye. Proc. Natl. Acad. Sci. U.S.A. 95, 340–345. doi: 10.1073/pnas.95.1.340
Provencio, I., Rodriguez, I. R., Jiang, G., Hayes, W. P., Moreira, E. F., and Rollag, M. D. (2000). A novel human opsin in the inner retina. J. Neurosci. 20, 600–605.
Schmidt, T. M., Alam, N. M., Chen, S., Kofuji, P., Li, W., Prusky, G. T., et al. (2014). A role for melanopsin in alpha retinal ganglion cells and contrast detection. Neuron 82, 781–788. doi: 10.1016/j.neuron.2014.03.022
Schmidt, T. M., Chen, S. K., and Hattar, S. (2011a). Intrinsically photosensitive retinal ganglion cells: many subtypes, diverse functions. Trends Neurosci. 34, 572–580. doi: 10.1016/j.tins.2011.07.001
Schmidt, T. M., Do, M. T., Dacey, D., Lucas, R., Hattar, S., and Matynia, A. (2011b). Melanopsin-positive intrinsically photosensitive retinal ganglion cells: from form to function. J. Neurosci. 31, 16094–16101. doi: 10.1523/JNEUROSCI.4132-11.2011
Schweikert, L. E., Fasick, J. I., and Grace, M. S. (2016). Evolutionary loss of cone photoreception in balaenid whales reveals circuit stability in the mammalian retina. J. Comp. Neurosci. doi: 10.1002/cne.23996. [Epub ahead of print].
Sexton, T., Buhr, E., and Van Gelder, R. N. (2012). Melanopsin and mechanisms of non-visual ocular photoreception. J. Biol. Chem. 287, 1649–1656. doi: 10.1074/jbc.R111.301226
Shi, Y., Radlwimmer, F. B., and Yokoyama, S. (2001). Molecular genetics and the evolution of ultraviolet vision in vertebrates. Proc. Natl. Acad. Sci. U.S.A. 98, 11731–11736. doi: 10.1073/pnas.201257398
Tsai, J. W., Hannibal, J., Hagiwara, G., Colas, D., Ruppert, E., Ruby, N. F., et al. (2009). Melanopsin as a sleep modulator: circadian gating of the direct effects of light on sleep and altered sleep homeostasis in Opn4(−∕−) mice. PLoS Biol. 7:e1000125. doi: 10.1371/journal.pbio.1000125
Tyack, P. L., Johnson, M., Soto, N. A., Sturlese, A., and Madsen, P. T. (2006). Extreme diving of beaked whales. J. Exp. Biol. 209, 4238–4253. doi: 10.1242/jeb.02505
Wilkie, S. E., Robinson, P. R., Cronin, T. W., Poopalasundaram, S., Bowmaker, J. K., and Hunt, D. M. (2000). Spectral tuning of avian violet-and ultraviolet-sensitive visual pigments. Biochemistry 39, 7895–7901. doi: 10.1021/bi992776m
Xue, T., Do, M., Riccio, A., Jiang, Z., Hsieh, J., Wang, H., et al. (2011). Melanopsin signalling in mammalian iris and retina. Nature 479, 67–73. doi: 10.1038/nature10567
Yokoyama, S. (2008). Evolution of dim-light and color vision pigments. Annu. Rev. Genomics Hum. Genet. 9, 259–282. doi: 10.1146/annurev.genom.9.081307.164228
Keywords: cetaceans, visual pigment opsin, melanopsin retinal ganglion cells, aquatic organisms, adaptation, physiological
Citation: Fasick JI and Robinson PR (2016) Adaptations of Cetacean Retinal Pigments to Aquatic Environments. Front. Ecol. Evol. 4:70. doi: 10.3389/fevo.2016.00070
Received: 01 April 2016; Accepted: 01 June 2016;
Published: 23 June 2016.
Edited by:
Wayne Iwan Lee Davies, University of Western Australia, AustraliaReviewed by:
Robert William Meredith, Montclair State University, USAChristopher Allan Emerling, University of California, Berkeley, USA
Copyright © 2016 Fasick and Robinson. This is an open-access article distributed under the terms of the Creative Commons Attribution License (CC BY). The use, distribution or reproduction in other forums is permitted, provided the original author(s) or licensor are credited and that the original publication in this journal is cited, in accordance with accepted academic practice. No use, distribution or reproduction is permitted which does not comply with these terms.
*Correspondence: Phyllis R. Robinson, cHJvYmluc29AdW1iYy5lZHU=