- Department of Biology, Centre for Social Evolution, University of Copenhagen, Copenhagen, Denmark
The tachykinins are a family of neuropeptides that influence a range of behavioral phenotypes in both vertebrates and invertebrates; they appear to have a conserved role in the processing of stimuli, and in the control of aggression in a wide range of animals. Expression of tachykinin in a cluster of neurons was recently shown to determine the stimulus response threshold for aggressive behavior in Drosophila (1). Varying response thresholds are often implicated in division of labor within social insect colonies, so we hypothesized that Tachykinin could play a role in the organization of colony defense by affecting individual response thresholds to non-nestmate stimuli. We used quantitative-PCR in combination with behavioral assays to test for associations between the expression of Tachykinin and its receptor, and the aggressive division of labor among the castes of the leaf-cutting ant Acromyrmex echinatior, a species with multiple worker castes. After correction for differences in brain size among castes, we found that the most aggressive large worker caste had the highest Tachykinin expression levels, but that no such effect was apparent for breeding and virgin queens. To further evaluate these deviating results for the reproductive caste, we manipulated the aggression threshold of virgin-queens by removing their wings, which is known to make them express a soldier-like behavioral phenotype. Despite heightened aggression, expression levels of Tachykinin remained unaffected, suggesting that aggression levels in reproductive caste phenotypes are controlled by differential expression of other genes.
Introduction
The great success of the insect societies is often attributed to their complex colony organization (Wilson, 1971; Hölldobler and Wilson, 1990; Seeley, 1995, 2010). Within a social insect colony, the behavior of potentially millions of individuals is coordinated to produce a cohesive whole, with each individual in the colony interpreting environmental stimuli and making local decisions that sum to create a self-organized division of labor that is flexible to the varying needs of the colony (e.g., Bourke and Franks, 1995; Beshers and Fewell, 2001). However, it is essential to distinguish between two distinct kinds of division of labor: the reproductive division of labor between egg-laying queens and the unmated workers, which may be subject to reproductive conflicts (Bourke and Franks, 1995; Ratnieks et al., 2006), and the “somatic” services provided by the unmated workers, either by division of labor of different tasks or by serial partitioning of complex single tasks (Hart et al., 2002).
In later years, the study of caste has focused primarily on gene expression differences, both among behavioral caste phenotypes and among physically differentiated castes (see Toth and Robinson, 2007; Johnson and Linksvayer, 2010, for reviews) and most recently such studies are increasingly benefitting from the availability of completely sequenced reference genomes (e.g., Simola et al., 2013; Kapheim et al., 2015; Patalano et al., 2015). Since the early pioneering studies (Oster and Wilson, 1978) research on caste differentiation has also taken a number of different directions, emphasizing neurobiological differentiation (e.g., Seid and Traniello, 2005), behavioral differentiation (e.g., Johnson, 2010) and specific loci (e.g., Ben-Shahar, 2005; Nelson et al., 2007; Amdam and Page, 2010) or quantitative traits (e.g., Hughes et al., 2010) associated with caste. Another approach has emphasized the collective benefits of caste differentiation by highlighting individual variation in response thresholds for stimuli inducing specific behaviors (reviewed by Beshers and Fewell, 2001; Duarte et al., 2011).
These threshold models propose that a colony workforce consists of individuals that perform a given task when a stimulus exceeds some internal threshold (Beshers et al., 1999): individuals with a lower threshold are more likely to perform the task and end up being specialists (Myerscough and Oldroyd, 2004). For example, in Pheidole pallidula colonies the major and minor workers are differentially recruited to nest-defense and foraging in a manner that suggests threshold-like dynamics (Detrain and Pasteels, 1991, 1992). The idea of thresholds has also been used to explain why a number of derived eusocial lineages have secondarily evolved multiple queen mating, as genetic chimerism of colonies increases the diversity of available stimulus thresholds, facilitating more efficient division of labor under variable conditions for resource acquisition, disease control, and colony homeostasis (reviewed in Robinson et al., 1989; Boomsma, 2013).
The proximate mechanisms controlling division of labor are often connected to genetic or hormonal factors that alter an individual's sensitivity to stimuli or her internal motivational state to perform tasks. Division of labor should thus be directly related to systematic neurological differences among workers according to caste, consistent with the many studies of peptide titers and gene expression that have implicated genes that specifically affect neurological functions in bees and ants. For example, the manganese transporter malvolio appears to control sensitivity to sucrose and therefore foraging behavior in honeybees—with the least sensitive individuals collecting the most sucrose-rich forage (Ben-Shahar et al., 2004). Similarly, the levels of neurotransmitters, such as the biogenic amines, serotonin, dopamine and octopamine, often correlate with task in both bees and ants. Honeybee nurses show higher octopamine titers than foragers (Schulz et al., 2002). Likewise in ant workers, serotonin levels have been linked to switching to tasks performed outside the nest (Seid and Traniello, 2005), both serotonin and octopamine have been linked to aggression (Kravitz, 2000; Smith et al., 2013; Kamhi et al., 2015), and dopamine levels may be involved in processing olfactory stimuli (Boulay et al., 2000; Seid and Traniello, 2005). This suggests that substantial differences in behavior may be the result of differential expression of relatively few genes with wide-ranging effects.
The tachykinin-related proteins (TRPs) are an ancient family of neuropeptides with wide-ranging functions throughout the animal kingdom (Severini et al., 2002). They act to modulate neurotransmission, and affect many processes in the central and peripheral nervous system, as well as the gastrointestinal tract (Nässel, 1999; Maggi, 2000; Severini et al., 2002). Relatively little is known of invertebrate TRPs compared to their mammalian homologs, although they appear to have similarly broad effects. In insects, as in mammals (Pennefather et al., 2004), multiple TRPs are produced from fewer larger pro-tachykinins. Drosophila, for example, has 6 TRPs transcribed from a single Tachykinin gene (Poels et al., 2009), while 15 TRPs have been identified in the American cockroach, Periplaneta americana (Neupert et al., 2012). In locusts and Drosophila, some TRPs have been found to be involved in lipid metabolism (Nässel, 1999; Song et al., 2014), while others can trigger rhythmic firing in ganglia of the decapod gut (Nässel, 1999). TRPs have also been found in the salivary glands of a mosquito, where they act as vasodilators within the host (Champagne and Ribeiro, 1994), and similarly as a venom in the common octopus (Kanda et al., 2003).
Despite such diverse functions, TRPs appear to have a conserved role in the perception and processing of stimuli. Tachykinin expression is commonly observed in the centers of the insect brain associated with olfaction (Nässel and Homberg, 2006; Fusca et al., 2015) and higher-level information processing (Takeuchi et al., 2004), as in Drosophila where RNAi knock-down of Tachykinin impairs olfactory sensitivity and increases locomotory activity (Winther et al., 2006). Pertinently, in the honeybee brain, it has been suggested that Tachykinin is expressed at higher levels in queens and foragers than in nurses, suggesting a role as a regulator of social phenotypes (Takeuchi et al., 2003). A recent screen of 19 neuropeptides in Drosophila implicated Tachykinin as a controller of sex-specific aggression (Asahina et al., 2014; Pavlou et al., 2014), finding that higher Tachykinin expression in a subset of Tachykinin-expressing neurons increased aggression and prompted aggressive responses in scenarios where they are not normally observed, and that lower expression in the same neurons greatly reduced aggression (Asahina et al., 2014).
Here, we used quantitative-PCR to measure the expression levels of Tachykinin and its receptor Tachykinin-R99D across the morphologically distinct castes of Acromyrmex echinatior. To evaluate its potential role with respect to colony defense, we used both a comparative and an experimental approach. A. echinatior belongs to the leaf-cutting crown-group of the attine fungus-growing ants (Schultz and Brady, 2008) and lives in nutritional symbiosis with a fungus garden cultivar (Weber, 1972). Colonies of Acromyrmex ants are among the most complex insect societies, as they combine advanced fungus farming with extensive caste differentiation (Hölldobler and Wilson, 1990). The small (minor) workers mainly complete tasks within the nest, caring for brood and tending the fungus, while the large (major) workers forage and defend the colony (Camargo et al., 2007). The intermediate workers (media) are less distinct in size and may represent the upper tail end of the minor worker size distribution (Hughes et al., 2003). They appear to be intermediate in their typical spectrum of tasks, so have also been sampled here, as in a previous study (Larsen et al., 2014).
Previous behavioral work has shown that the major workers of A. echinatior respond more aggressively to foreign intruders than the smaller worker castes (Larsen et al., 2014), which was attributed to enhanced motivation in the major workers, and reduced sensitivity to non-nestmate stimuli in the less aggressive minor workers, consistent with caste-dependent differences in stimulus response thresholds. Another study showed that aggression thresholds can also be experimentally manipulated in virgin queens (gynes) by clipping their wings, which prompts a switch to a soldier-like behavioral phenotype with increased aggression and exhibition of brood retrieval behavior (Nehring et al., 2012). These soldier-like gynes are observed naturally, and are hypothesized to be gynes that miss their nuptial flights, subsequently shed their wings, and adopt the role of nursing soldiers within the colony (Nehring et al., 2012). Such gynes have not been observed in Atta, the sister genus of Acromyrmex, which possesses a morphologically specialized soldier caste (Hölldobler and Wilson, 1990). Although we could rely on previously established routines for the behavioral part of this study, we had to resolve some challenges to adequately measure the expression of brain-related genes, as the castes of A. echinatior differ greatly in body and brain size—in fact, a study comparing 40 myrmicine ant species showed that A. echinatior had both the largest and the smallest brains within this comparative sample (Seid et al., 2011). As we know of no confirmed neuron-specific housekeeping genes for A. echinatior, differential expression of brain-related genes will primarily reflect differences in relative brain size among castes, so statistical adjustments were required to account for this confounding variable.
Methods
Sample Collection, Dissections, and Behavioral Trials
Twelve founding-queens were collected in May of 2014 and 2015 in Gamboa, Panama along with their nascent fungus garden, and housed in 5 cm petri dishes with a small amount of damp cotton wool to maintain humidity. In three cases where the natural fungus was missed upon collection queens were offered a replacement fungus-garden from a mature colony that was quickly adopted as expected (Poulsen et al., 2009). After acclimatizing for at least 24 h, an allospecific A. octospinosus major worker from a sympatric colony was introduced and host behavior observed for the next 3 min. After removing the intruder, the resident queens were left for 12 h to ensure as much as possible that the trials themselves did not directly influence gene expression. Queens were then frozen for 5 min at −80°C and decapitated, after which the heads were stored in RNAlater. One trial was aborted because the queen was obviously not in good health. In both years, queens were collected before noon, and the behavioral trials conducted in the early afternoon.
Similar behavioral trials were conducted with lab colonies using 20 ants for each of the four castes (gynes, major, media, and minor workers) across two colonies (Ae226 and Ae263) that had been kept under controlled conditions in Copenhagen since 2003 and 2006, respectively, at ca. 25°C, 75% RH and on a diet of bramble leaves, apple and dry rice. Worker castes were differentiated according to head width (majors >2.0 mm, 1.6 mm < media <2.0 mm, minors < 1.2 mm; Larsen et al., 2014). Half of the hosts were subjected to intrusion by a nestmate control and the other half by an allospecific A. octospinosus major worker. Each A. echinatior individual was confronted with a with a major worker from a paired A. octospinosus colony (Ao604 and Ao273, respectively, collected in 2012 and 2006). Five additional individuals of each caste, with the exception of the minor caste where 10 individuals were taken, were collected from the two A. echinatior colonies. Additional ants were collected from a further four colonies: Ae160 (originally collected in 2002), Ae322 (2007), Ae331 (2007), and Ae372 (2008). The ants were flash frozen in liquid nitrogen, after which the heads were removed and stored at −80°C. Workers from each colony were collected concurrently, but each colony was collected on different days.
Video recordings of aggression were randomized and scored using JWatcher (Blumstein and Daniel, 2007) without knowledge of the intruder's identity. As in Larsen et al. (2014) and Nehring et al. (2012) behaviors were scored as 0 for antennation, 1 for the mandibles being opened, and 2 for biting. The aggression indices were then calculated as in Guerrieri et al. (2009) by multiplying the score of each behavior by the proportion of trial time spent conducting the behavior. The total score was then used as an aggression index, ranging from 0 (completely peaceful interactions) to 2 (continuous biting).
For brain dissection, individuals were selected at random from two colonies (Ae226 and Ae263, also used above) and chilled at 5°C for 15 min; the heads were then removed, weighed, submerged in 96% ethanol for 1–2 s, dried on lens paper, and dissected in PBS buffer. Following the protocol of Seid et al. (2011), isolated brains were transferred to a microbalance by pipette, placed on a small piece of parafilm, after which the buffer was removed using a piece of finely twisted KimTech wipe, and the brain was weighed within 4 s. Seid et al. (2011) found weight-loss during sample preparation to vary between approximately 0.9 and 2.4%, and there was no obvious correlation with brain size, so weight-loss was deemed not to have adversely affected our measurements.
We selected a further three colonies (Ae150, Ae356, and Ae394) that had also been housed under laboratory conditions since 2002, 2008, and 2009, removed all reproductive individuals, discarded the males, and chilled the gynes at 5°C for 15 min. We then removed the wings from one third of the gynes, a middle leg from another third, and left the remainder unharmed. The thorax of each gyne was marked with a spot of paint, using a different color for each treatment. Gynes were left to recover for 30 min before being returned to their colony. After 1 week within the colony to ensure the behavioral switch, aggression trials were conducted as in Nehring et al. (2012). A gyne was placed in a circular arena of 5 cm diameter with fluon-coated walls and a floor lined with filter paper that had spent at least 24 h in the gyne's colony. She was then left for 5 min to acclimatize before a large worker from either the natal nest (control) or from an A. octospinosus colony was introduced, and the gyne's behavior was recorded for the next 3 min. Again each A. echinatior colony was paired with a specific A. octospinosus colony (Ae150-Ao512, Ae394-Ao615, and Ae356-Ao615) and aggression indices were calculated as above without prior knowledge of the intruder's identity.
Another subset of the treated gynes was tested for their tendency to retrieve brood, as an indicator of a shift to a “soldier/nurse phenotype”: each gyne was placed in an 8.5 cm diameter petri dish, along with a small amount of fungus from the gyne's nest and 5–8 minor workers, and left for 30 min to acclimatize before a large larva (>4 mm) was placed in the dish some distance away from the fungus garden. Large larvae were used to prevent retrieval by the minor workers. The position of the larvae was recorded after 2 h, and the trial was considered successful if the larva had been moved to the fungus garden. This was repeated for all three colonies, and an additional 20 trials were conducted without a gyne present, to ensure that the small workers were indeed unable to retrieve the larva (correct in all cases). After the trials, the gynes were flash frozen in liquid nitrogen and their heads were stored at −80°C.
RNA Extraction and Gene Expression Quantification
RNA was extracted from individual heads for the founding queens, and from pools of individuals according to caste and colony for the gynes and worker castes (five individuals for the larger gynes, major and media workers and 10 for the minor workers), assuming that variation among individuals within castes would be minor relative to variation among castes. We also pooled five gyne heads from the brood-retrieval trials according to treatment and colony. To ensure that the gene expression changes reflected behavioral shifts, treatment gynes were not selected randomly: only individuals who successfully retrieved a larva were used for the wing-mutilated treatment, and only gynes that failed to retrieve a larva were used for the unmanipulated and leg-mutilated treatments.
All RNA was extracted using the RNEasy kit (QIAGEN) following the manufacturer's protocol. Extraction success was evaluated using a Nanodrop spectrophotometer and RNA integrity was checked using a 2% agarose gel. To obtain cDNA for qPCR, 500 ng of RNA was reverse transcribed in a 10 μl reaction containing 5.25 μl RNA sample, 0.5 μl SuperScript III (Invitrogen), 2 μl 5X first strand buffer, 1 μl dNTP, 1 μl DTT, 0.125 μl RNASin, and 0.125 μl Primer Qt (CCA GTG AGC AGA GTG ACG AGG ACT CGA GCT CAA GCT TTT TTT TTT TTT TTT TVN). RNA was heated to 65°C for 3 min before mixing the reagents. The thermal protocol consisted of 42°C for 60 mins, 50°C for 10 mins, and 70°C for 15 min. Following reverse transcription, the cDNA was treated with RNase H at 37°C for 20 min to remove any residual RNA before a 10-fold dilution in TE buffer.
Four primer pairs were designed using the A. echinatior genome (Nygaard et al., 2011): [F: CAA TGA GTT TTC AAG GGA TG, R:TCT ATT GCT CCT TCC TTG AT] for Tachykinin (Tac); [F:GTT GCA TGA ATA CTA GAT TCC, R:TAC CAT TCC GCG ATA TTC TG] for Tachykinin-receptor 99D (TacR99D); [F:CCC ACA GTT ATT GCC AAA TCG, R:CCA CTG GGA CAA GTT TTG ATG] for the housekeeping gene Elongation factor 1-β (EF1β); and [F:TCC CCA AGT TGA CGG TAT G, R:CCC TGC ATT AAG ACT GTA CG] for the housekeeping gene Ribosomal Protein L18 (RPL18). EF1β and RPL18 were chosen as they have previously been shown to be stably expressed across castes and tissues in another myrmicine ant, Solenopsis invicta (Cheng et al., 2013). The primers were designed to span an intron, as these are spliced from the mRNA after transcription, preventing erroneous amplification of genomic-DNA and ensuring more accurate quantification of the mRNA.
The efficacy of the designed primers and the success of the reverse-transcription were determined using PCR, and subsequent agarose gel electrophoresis. Reactions consisted of 10 μl RedTaq polymerase, 1 μl each of the two primers (10 μM), 7 μl H2O, and 1 μl of the cDNA template. The thermocycler protocol was 94°C for 5 min, 30 cycles of 94°C for 20 s, 55°C for 30 s, and 72°C for 1 min, followed by a final elongation step of 72°C for 10 min.
Quantitative-PCR reactions were conducted on the Strategene Mx3005P system using SYBR-Green dye. Each reaction totaled 20 μl: 10 μl System Ex Taq, 0.4 μl of both the forward and reverse primers, 0.4 μl ROX reference dye, and 2 μl cDNA template, and 6.8 μl water. The same reaction cycle was used in all cases: 95°C for 2 min, followed by 40 cycles of 95°C for 30 s, 55°C for 30 s and 72°C for 30 s. The reactions were followed by a dissociation curve and any reactions with spurious peaks were removed from analysis. All reactions were conducted in triplicate.
A common fluorescence threshold of 0.0121 dRN was used across all reactions to standardize the threshold cycle values (Ct). Efficiencies for each gene were calculated for each plate, thereby accounting for systematic differences among both runs and genes, by fitting a curve to the exponential portion of the fluorescence curve (Guescini et al., 2008) with the package “qPCR” in R 3.2.2. The geometric means of efficiencies, by gene and plate, were then used to correct for differences in efficiency using [efficiency]−Ct. Unsuccessful reactions were identified as kinetic outliers and removed from the calculations (Bar et al., 2003). The ReadQPCR and NormQPCR packages were then used to combine technical replicates and to calculate ΔCt values relative to the geometric mean of the two housekeeping genes (Perkins et al., 2012). The validity of EF1β and RPL18 as housekeeping genes was verified using the geNorm algorithm (Vandesompele et al., 2002). Although only two candidate house-keeping genes were tested, they were deemed appropriate as they were more stable with respect to one another than the recommended stability cut-off (Vandesompele et al., 2002), (maximum M = 0.099, recommended-threshold = 0.15).
Results
Using a general linear mixed model, with caste of the host and identity of the opponent (nestmate or allospecific) as fixed explanatory variables and host-colony as a random factor, all castes were found to be more aggressive toward non-nestmates than nestmates (Tukey Kramer, p-value always ≤ 0.015) (Figure 1). Host caste significantly affected aggression when compared to a similar model without the host caste variable (parametric bootstrap test, 500 simulations, p = 0.002): the major and media workers were found to be most aggressive toward non-nestmates while the gynes, minor workers and founding queens did not significantly differ in their responses. The same results were obtained with a non-parametric Kruskal-Wallis test (p < 0.0001). Likewise, the major and media workers were found to be the most aggressive toward non-nestmates, while the gynes, minor workers and founding queens did not significantly differ in their response (pairwise Wilcoxon test, p-value cut-off = 0.05). Also here, the same results were obtained when a non-parametric Kruskal-Wallis test was used (p < 0.0001).
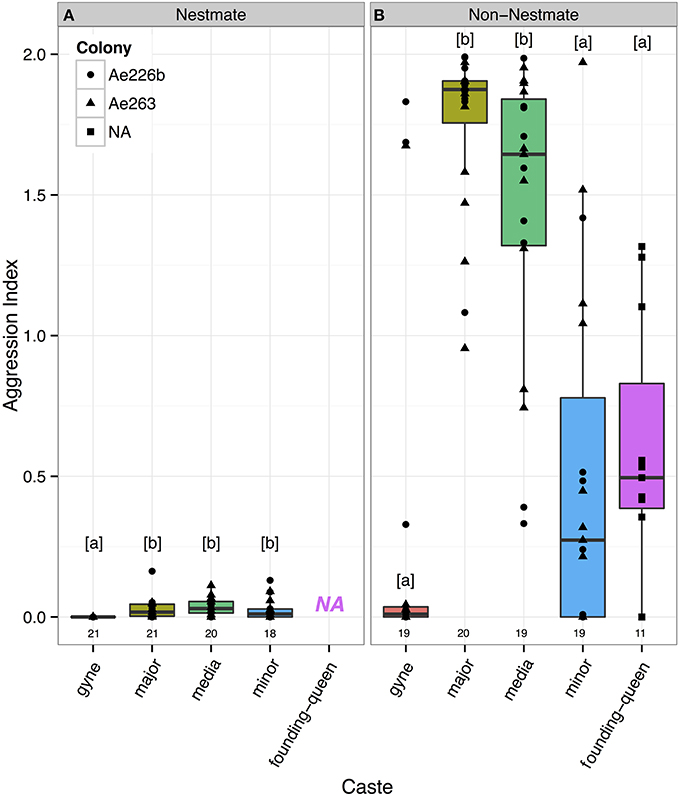
Figure 1. Aggression indices for three worker castes (major, media and minor) and two life-stages of the reproductive caste (gynes and founding-queens). All castes attacked non-nestmates (B) more than nestmates (A), with the major and media workers being most aggressive. Letters indicate significantly different pairs (Tukey-Kramer, p < 0.05) and numbers underneath boxes are sample sizes. There are no data for founding-queens challenged with nestmates (NA) because they do not yet have nestmates.
The relative expression of Tachykinin and TacR99D across the four castes is shown in Figure S1. Data were log2 transformed for analysis to normalize distributions. The relative expression levels of both Tachykinin and TacR99D differed significantly among castes (ANOVA, Tac: [F(4, 23) = 42.71, p < 0.0001], TacR99D: [F(4, 25) = 10.97, p < 0.0001]. A Tukey-Kramer pairwise comparison indicated that Tachykinin expression in founding-queens was significantly lower than in all other castes, while expression in gynes also differed from expression in the media and minor workers (Figure S1A) (p < 0.05). For TacR99D, the minor workers had higher expression than the gynes, major workers and founding-queens, while the founding-queens also differed from the major workers (Figure S1B). Variation in relative expression of Tachykinin and TacR99D was lowest among founding queens for which heads were not pooled and considerably higher in minor workers, suggesting that our assumption that gene expression variation within castes would be small relative to variation across castes was correct because variation was obviously not negatively correlated with the number of individuals pooled per sample (Figure S1), as one would expect when individual differences average out.
In order to explore whether castes exhibited differences in expression of Tachykinin and TacR99D independent of the differences in relative brain size, we investigated the relationship between their mutual expression levels (Figure 2) by fitting lines with a standardized major axis (SMA) model II regression (Warton et al., 2006, 2012; Taskinen and Warton, 2011). The slopes were not significantly different, with a common slope of 1.114 ± 0.291 ( = 0.1973, p = 0.99), but the intercepts differed significantly (W4 = 9.626, p = 0.047). The major workers had the highest intercept (0.364 ± 0.71) and the gynes the lowest (0.073 ± 0.664) after analyses based on log-transformed values. When the data were separated according to caste, the correlation between Tachykinin and TacR99D became non-significant within all castes except the gynes (p = 0.01), although Tachykinin expression increased proportionally with increasing TacR99D levels across all castes (p < 0.0001, R2 = 0.72). That the major workers have the highest intercept suggests they have higher levels of Tachykinin relative to TacR99D than we might expect based on the relationship observed in the other castes. Although a pairwise comparison did not find any pairs that significantly differed, the two larger worker castes (majors and media) appeared to be different from the founding-queens, differences that tended toward significance (p = 0.074 and p = 0.063, respectively).
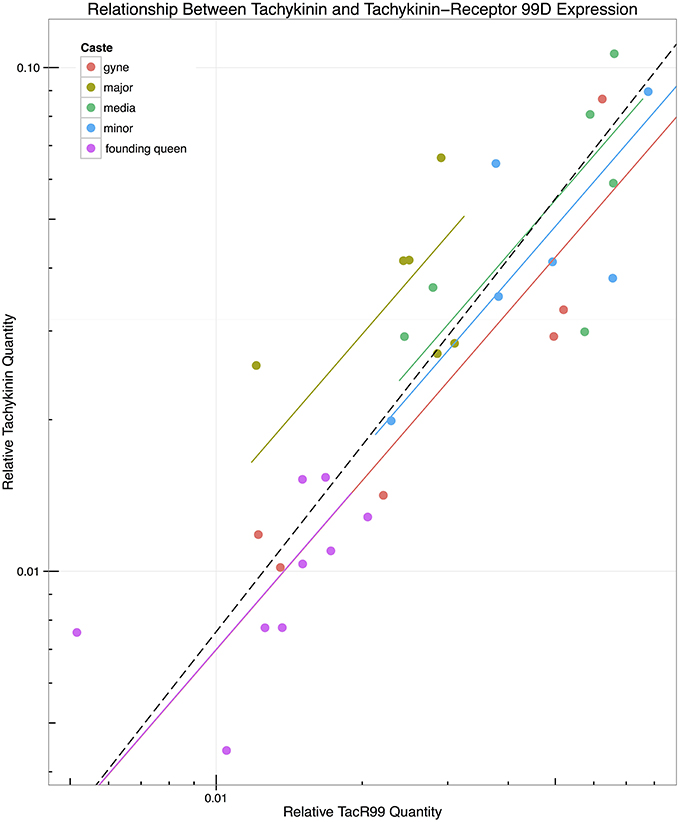
Figure 2. Relationship between expression of Tachykinin and Tachykinin-Receptor 99D. Lines were fitted using standardized major axis regression on log transformed data, showing that slopes were not significantly different (Likelihood ratio, p = 0.99), but that intercepts differed overall (Wald Statistic, p = 0.047). However, no pairs of intercepts were significantly different at p < 0.05 even though the intercepts of founding-queens and media (p = 0.063) and major (p = 0.0742) workers tended toward significance.
Absolute brain sizes decreased toward the smaller castes, but relative brain sizes increased (Figure 3) when using SMA regression lines (Warton et al., 2006, 2012; Taskinen and Warton, 2011) (p < 0.0001, R2 = 0.93). Comparing Figure 3 to Figure S1, it is notable that the expression levels of both genes in the minor workers were lower than one would expect from their relative brain size; the minor workers have significantly larger brains than the other castes (Figure 3B), but do not differ in expression levels of either gene (Figure S1).
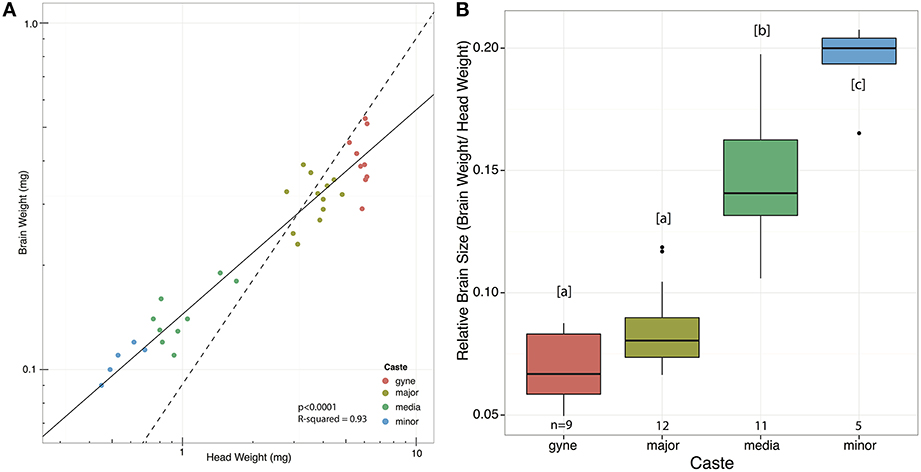
Figure 3. Relationship between brain weight and head weight across castes of A. echinatior. (A) Standardized major axis regression on double log transformed data (p < 0.0001, R2 = 0.93, log10(Brain) = 0.59log10(Head)−0.84) showed that brain weight is negatively allometric, i.e., ants with larger heads have relatively smaller brains. The dashed isometry line has been added to facilitate comparison. (B) Relative brain sizes across worker castes plotted directly and with letters indicating significantly different pairs (Tukey-Kramer, p < 0.005).
An analysis of aggression among the different gyne treatments (Figure S3A), similar to the linear model constructed for the caste differences above but with treatment replacing caste as a fixed explanatory variable, showed that treatment groups differed in aggression toward allospecifics (parametric bootstrap test, 500 simulations, p = 0.006), whereas confrontations with nestmate control workers did not produce significant differences (p > 0.05). Only the wingless gynes responded differently to the identity of the intruder (Tukey Kramer, p = 0.0001), and were found to be significantly more aggressive to non-nestmates than both the leg-mutilated (Tukey Kramer, p = 0.049) and the unmutilated gynes (p = 0.0007).
A final linear mixed model was constructed for the brood retrieval, with treatment as a fixed variable and the gyne's colony as a random variable, using a binomial error distribution. Treatment was indeed found to affect whether a gyne retrieved a larva to the fungus garden (Figure S3B; parametric bootstrap test, 500 simulations, p = 0.002), consistent with results found previously (Nehring et al., 2012). The wingless gynes were significantly more likely to move a larva than the leg-mutilated gynes (Tukey Kramer, p = 0.0004) and unmutilated gynes (p = 0.0007), but no difference was found between the leg-mutilated and unmutilated gynes (p = 0.85). In contrast, wing-removal did not have a significant effect on the expression of either Tachykinin or TacR99D (Figure S4) (paired t-tests, p > 0.05) and no consistent pattern could be discerned between the expression-levels of either gene and the two behavioral traits measured.
Discussion
Our study on the leaf-cutting ant Acromyrmex echinatior set out to test whether and to what degree Tachykinins and their receptors regulate division of labor in the expression of aggression. The inspiration for this work came from these neuropeptides having been implicated in the control of behavioral phenotypes with potential impact on social organization (Takeuchi et al., 2003), either through influencing olfaction (Nässel and Homberg, 2006; Winther et al., 2006; Fusca et al., 2015) or modulating neuronal activity in the insect brain (Takeuchi et al., 2004). Suggestive of a role for Tachykinin in division of labor, we found that its expression correlated broadly with aggression levels across the worker castes, consistent with previous evidence showing that Tachykinin controls aggression in Drosophila (Asahina et al., 2014) and appears to be differentially expressed in honeybee castes (Takeuchi et al., 2003). However, we also found that Tachykinin does not control the aggressive responses of reproductive individuals, both in direct comparisons between virgin and inseminated queens and after experimentally manipulating virgin queen phenotypes in a way that has previously been shown to enhance aggression (Nehring et al., 2012). We discuss and interpret these findings in the sections below.
Patterns of Aggression and Their Interpretation
The aggression results we obtained (Figure 1) largely agree with previous work (Larsen et al., 2014): the major workers were found to be more aggressive than the minor workers, but we did not reconfirm a difference in aggression between the major and media workers. This may be because we used experimental arenas with fungus present, making them more nest-like than the ants-only arenas used by Larsen et al. (2014) or because our sample sizes were much smaller (the trend was in the predicted direction). As found previously (Nehring et al., 2012; Larsen et al., 2014), the gynes were generally passive toward non-nestmates, with very few individuals showing any aggression.
There appears to be an increase in aggression in the founding-queens relative to the gynes, although this was not significant due to the high variance in aggression among the founding queens. Intermediate aggression of founding queens is perhaps not surprising, since they stand to lose much more than any of the other castes if they are injured in a conflict, but must defend the nest as they cannot yet rely on workers. They might, therefore, remain careful toward a large worker who is unlikely to steal their fungus garden, and we may expect higher aggression if the intruder had been another founding queen that is able to adopt a novel fungal symbiont (Poulsen et al., 2009) or steal a fungus garden from neighbors (Howe, Unpublished data). However, while gynes only showed defensive aggression after being attacked, the experimental founding-queens would often, but far from always, pursue and lunge at intruders.
Tachykinin Expression Correlates with Aggression across Worker Castes, But Not in Queens
Overall, our results are consistent with the suggestion that Tachykinin and its receptor are involved in the expression of aggressive behavior in A. echinatior leaf-cutting ants, but only in the worker castes that routinely express more or less aggression throughout their lives. The aggressive major and media workers appear to have higher Tachykinin levels than expected from the expression levels of the minor workers and the reproductive castes (Figure 2) when the relationship between the expression of the two genes is evaluated. However, there was no relationship between aggression and either direct or adjusted Tachykinin expression among the life stages of the reproductive gyne/queen caste, where both Tachykinin and TacR99D levels tended to be reduced in founding queens that expressed modest aggression toward intruding large workers (Figure 4; Figure S1). The minor workers displayed similarly low aggression levels as the founding queens (Figure 1) and had Tachykinin expression levels in between those of virgin and founding queens (Figure 4). This tentative association appears more clearly when considering the residuals of the linear models for both aggression levels and gene expression; when compared to the mean aggression levels and the expected Tachykinin:Tachykinin-Receptor 99D levels, we see that the castes with higher aggression than the mean, also tend have higher than expected Tachykinin levels (Figure S2), although this just failed to reach formal 5% significance (p = 0.055). Together, these results suggest that Tachykinin may have a role in mediating aggression in worker castes, but that it does not in either gynes or queens. The manipulation experiment confirmed the result obtained by Nehring et al. (2012) that wing-clipping both induces soldier-like aggressive behavior (Figure S3) and enhanced brood retrieval behavior (Figure S3), but the frequency of these behaviors was not associated with any aspect of Tachykinin expression (Figures S3, S4).
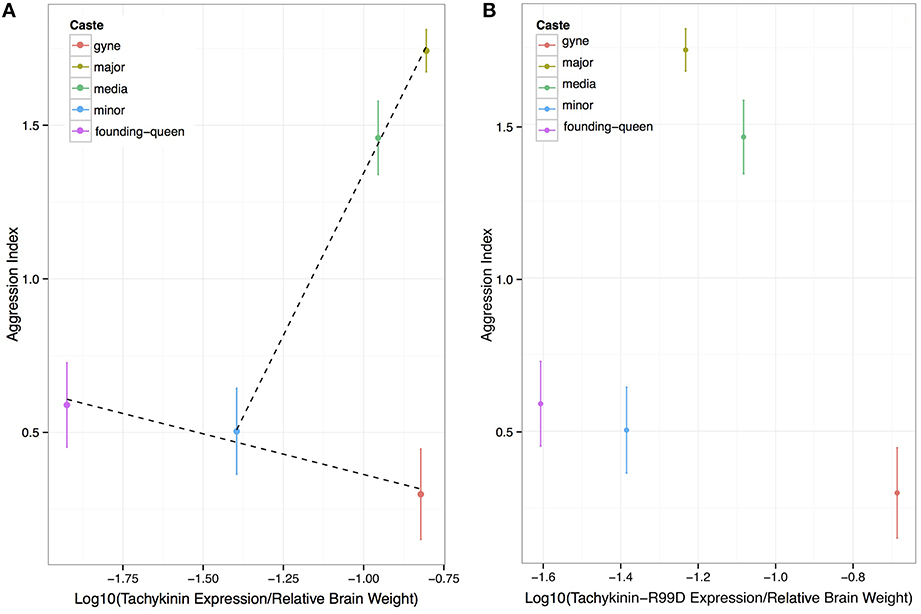
Figure 4. Aggression as a function of brain-size corrected expression levels of Tachykinin and the Tachykinin-Receptor 99D across castes and reproductive life stages. (A) Aggression increases with brain-size adjusted Tachykinin expression across the worker castes (normalized to average caste brain size), but not across life stages of queens. (B) The pattern is similar for Tac-R99D expression levels except that the major workers have a remarkably low expression level compared to the media workers.
We interpret these results to imply that aggression in the reproductive caste is mediated by other genes. At this stage we can only speculate about the identity of these genes, but genes that have been associated with aggression of social and non-social insects would appear good candidates, because it seems likely that genes regulating founding nest defense behavior are evolutionarily conserved. For example, octopamine has previously been linked with aggression in ants (Kamhi et al., 2015), bees (Robinson et al., 1999), and crickets (Stevenson et al., 2005), although octopamine titers in bees were only positively associated with aggression toward non-nestmates and in fact decreased aggression toward nestmates (Robinson et al., 1999). Octopamine also appears to be involved in switching from nursing to foraging tasks in bees (Schulz et al., 2002). As worker castes evolved independently in ants and bees and involve very different behavioral syndromes, it is perhaps not surprising that other genes were coopted. This would lead to the expectation that worker aggression in related myrmicine ants may also be mediated by Tachykinin expression, but in phylogenetically basal ants where workers are more queen-like, or in bees or wasps, worker aggression may be regulated through different means.
The Multiple Roles of Tachykinin in Social and Non-social Insects
We currently do not know how many TRPs are produced from the Tachykinin gene in Acromyrmex leaf-cutting ants, but there may be many—15 were identified in the brain of the American cockroach Periplaneta americana (Neupert et al., 2012). However, it is likely that Tachykinin also influences many other physiological and behavioral traits (Nässel, 1999), and that any differences responsible for aggression changes may be masked by changes affecting other behaviors. To explore this possibility, it would be necessary to evaluate the spatial distribution of Tachykinin-expressing neurons among the different castes, to determine whether Tachykinin has qualitatively different effects in the brains of different castes as Asahina et al. (2014) suggest may be the case for the sexes in Drosophila. However, no such differences have been observed in spatial expression patterns in the honeybee (Takeuchi et al., 2004).
Tachykinin expression is often also implicated in olfactory processing, both in direct observations (Takeuchi et al., 2003) and in manipulative experiments (Winther et al., 2006; Ignell et al., 2009). The attine ants have a huge array of olfactory glomeruli, with numbers for seven other Acromyrmex species ranging from 369 to 477, i.e., an order of magnitude more than the 43 glomeruli in Drosophila and substantially above the ca. 164 of the honey bee Apis mellifera (Kelber et al., 2009). There is therefore considerable potential for Tachykinin-mediated olfactory processes to affect other ant behaviors. In A. echinatior, major workers are better able to recognize non-nestmates by dissimilarity of cuticular chemical profiles (Larsen et al., 2014), more likely to reject a foreign strain of the symbiotic fungus (Ivens et al., 2008), and less likely to show aggression toward nestmates contaminated with waste (Waddington and Hughes, 2010) when compared to minor workers. Similarly, differently-sized worker castes of the phylogenetically related Atta leaf-cutting ants differ in their sensitivity to components of trail pheromones with the large workers showing the greatest sensitivity, and therefore greatest propensity to forage (Kleineidam et al., 2007). This difference appears to result from a trail-pheromone-sensitive macro-glomerulus that is only found in the large worker antennal lobes (Kleineidam et al., 2005; Kelber et al., 2009, 2010; Kuebler et al., 2010). Something similar may also occur in Acromyrmex where the major workers also possess macro-glomeruli (Kelber et al., 2009) that must contribute to their division of labor, as they are the only caste that forages (Kooij et al., 2014).
Tachykinin appears to also be involved in locomotion (Winther et al., 2006) and spatial orientation (Kahsai et al., 2010) in Drosophila, and is thought to be differentially expressed between forager and nurse bees (Takeuchi et al., 2004), further implicating Tachykinin in foraging behaviors. The higher levels of Tachykinin that we found in the major and media workers can in fact be interpreted as being associated with the foraging phenotypes of A. echinatior, so it will be worth exploring changes in Tachykinin expression as age-dependent polyethism unfolds. Much like honeybee workers, major workers of Acromyrmex subterraneus brunneus tend to stay in the nest while young, before switching to foraging when older (Camargo et al., 2007), a pattern in Acromyrmex that is also associated with growing extensive cuticular covers of antibiotic-producing actinomycete bacteria in the first few weeks of adult life, that are greatly reduced later in life (Poulsen et al., 2003; Andersen et al., 2013). The workers selected in our present study were chosen exclusively from outside the nest, so a different pattern might be observed with younger workers.
Along these lines, it is possible that foraging and aggression are neurologically linked, and that both behaviors potentially interact with Tachykinin. The forager gene encodes a cGMP-dependent protein kinase (PKG) known to correlate with aggression across the workers of Pheidole pallidula (Lucas and Sokolowski, 2009; Lucas et al., 2010) with higher PKG activity being observed in the brains of aggressive major workers compared to foraging minor workers (Lucas et al., 2010). Tachykinin appears able to influence cGMP levels in the cytosol of the neurons that express the receptor (Birse et al., 2006), meaning that Tachykinin could act upstream of forager in the control of foraging behavior. However, the founding-queens of Acromyrmex also provide a difficulty for this interpretation, as they forage during colony foundation (Fernández-Marín et al., 2004), perhaps suggesting again that Tachykinin expression is unlikely to be associated with behavioral changes of reproductives.
Conclusion
In sum, Tachykinin expression levels are consistent with aggression across the worker castes of the leaf-cutting ant A. echinatior, but are inconsistent with aggression in the reproductive gynes/queens. Tachykinin and its receptor may also have significant roles in other aspects of behavioral differentiation among the worker castes of A. echinatior as well as other social insects. Thus, to refine our understanding of the role that Tachykinin may have in producing division of labor phenotypes, an estimate of the number of tachykinin-related peptides across brains of Acromyrmex castes would be needed. It would also be useful to explore the spatial distribution of expression across and within castes, particularly among unmated, mated and mature queens (the latter were missing in our present sample), and between winged and wingless gynes. Finally, expression should also be explored with respect to the age-dependent sequences of typical worker behaviors (Camargo et al., 2007), similar to studies conducted in honeybees (Oldroyd and Thompson, 2006) that have so far been neglected in ants with morphological castes.
Author Contributions
JH, MS, and JB all contributed to the original hypothesis generation and experimental design. JH collected the samples, and conducted both the behavioral and the genetic analyses. JH also wrote the majority of the manuscript, with contributions and feedback from JB and MS.
Funding
This work was funded by an ERC Advanced Grant awarded to JB (323085).
Conflict of Interest Statement
The authors declare that the research was conducted in the absence of any commercial or financial relationships that could be construed as a potential conflict of interest.
Acknowledgments
We are indebted to the Smithsonian Tropical Research Institute for logistic help and hosting this research and to the Autoridad Nacional del Ambiente y el Mar for permission to sample ants in Panama and to export them to Denmark. Additionally, we are incredibly grateful to Aaron O'Dea and family for granting us access to ‘Lorenzo Bank’.
Supplementary Material
The Supplementary Material for this article can be found online at: http://journal.frontiersin.org/article/10.3389/fevo.2016.00055
Figure S1. Relative quantity of Tachykinin and Tachykinin-R99D, expressed in proportion to the housekeeping genes EF1β and RPL18. Letters indicate significantly different pairs (Tukey-Kramer, p < 0.05) and sample sizes are indicated underneath each box. Caste (workers) and life stage (founding-queens) were found to have a significant effect on expression of Tachykinin [F(4, 28) = 2.887, p = 0.044] (A), but not on Tac-R99D expression levels [F(4, 28) = 0.29, p = 0.881] (B).
Figure S2. Residuals of aggression and gene expression models. A linear regression showing the relationship between the residuals of each individual from the overall mean aggression level, and the residuals of the SMA-regression of Tac and Tac-R99D. The overall relationship was barely significant (p = 0.055, R = 0.758), but higher than expected aggression, relative to the other castes, tends to co-occur with a higher than expected expression of Tachykinin, compared to Tac-R99D.
Figure S3. Worker-like behavior of wingless virgin queens. (A) Wingless gynes showed increased aggression relative to both gynes with a leg removed, or unmanipulated gyne controls when challenged with an allospecific non-nestmate (left), but not when confronted with a nestmate intruder (right). Numbers below each column refer to the sample size. Letters indicate significantly different pairs (Tukey-Kramer test, p < 0.05). (B) Brood retrieving behavior of experimentally treated virgin queens (gynes). Wing-clipped gynes were more likely to retrieve a large larva than untreated gynes or gynes with a leg removed rather than their wings. Numbers below each bar represent sample sizes (***p < 0.001, Tukey Kramer).
Figure S4. Expression of Tachykinin (A) and Tac-R99D (B) following wing or leg removal in gynes, no treatments were found to be significant (paired t-test, p > 0.005).
References
Amdam, G. V., and Page, R. E. (2010). The developmental genetics and physiology of honeybee societies. Anim. Behav. 79, 973–980. doi: 10.1016/j.anbehav.2010.02.007
Andersen, S. B., Hansen, L. H., Sapountzis, P., Sørensen, S. J., and Boomsma, J. J. (2013). Specificity and stability of the Acromyrmex–Pseudonocardia symbiosis. Mol. Ecol. 22, 4307–4321. doi: 10.1111/mec.12380
Asahina, K., Watanabe, K., Duistermars, B. J., Hoopfer, E., González, C. R., Eyjólfsdóttir, E. A., et al. (2014). Tachykinin-expressing neurons control male-specific aggressive arousal in Drosophila. Cell 156, 221–235. doi: 10.1016/j.cell.2013.11.045
Bar, T., Ståhlberg, A., Muszta, A., and Kubista, M. (2003). Kinetic Outlier Detection (KOD) in real-time PCR. Nucleic Acids Res. 31:e105. doi: 10.1093/nar/gng106
Ben-Shahar, Y. (2005). The foraging gene, behavioral plasticity, and honeybee division of labor. J. Comp. Physiol. A 191, 987–994. doi: 10.1007/s00359-005-0025-1
Ben-Shahar, Y., Dudek, N. L., and Robinson, G. E. (2004). Phenotypic deconstruction reveals involvement of manganese transporter malvolio in honey bee division of labor. J. Exp. Biol. 207, 3281–3288. doi: 10.1242/jeb.01151
Beshers, S. N., and Fewell, J. H. (2001). Models of division of labor in social insects. Annu. Rev. Entomol. 46, 413–440. doi: 10.1146/annurev.ento.46.1.413
Beshers, S. N., Robinson, G. E., and Mittenthal, J. E. (1999). “Response thresholds and division of labor in insect colonies,” in Information Processing in Social Insects, eds C. Detrain, J. L. Deneubourg, and J. M. Pasteels (Basel: Birkhäuser Basel), 115–139. doi: 10.1007/978-3-0348-8739-7_7
Birse, R. T., Johnson, E. C., Taghert, P. H., and Nässel, D. R. (2006). Widely distributed Drosophila G-protein-coupled receptor (CG7887) is activated by endogenous tachykinin-related peptides. J. Neurobiol. 66, 33–46. doi: 10.1002/neu.20189
Blumstein, D. T., and Daniel, J. C. (2007). Quantifying Behavior the JWatcher Way. Sunderland, MA: Sinauer Associates, Inc.
Boomsma, J. J. (2013). Beyond promiscuity: mate-choice commitments in social breeding. Philos. Trans. R. Soc. B Biol. Sci. 368:20120050. doi: 10.1098/rstb.2012.0050
Boulay, R., Soroker, V., Godzinska, E. J., Hefetz, A., and Lenoir, A. (2000). Octopamine reverses the isolation-induced increase in trophallaxis in the carpenter ant Camponotus fellah. J. Exp. Biol. 203, 513–520.
Bourke, A. F., and Franks, N. R. (1995). Social Evolution in Ants. Princeton: Princeton University Press.
Camargo, R. S., Forti, L. C., Lopes, J. F. S., Andrade, A. P. P., and Ottati, A. L. T. (2007). Age polyethism in the leaf-cutting ant Acromyrmex subterraneus brunneus Forel, 1911 (Hym., Formicidae). J. Appl. Entomol. 131, 139–145. doi: 10.1111/j.1439-0418.2006.01129.x
Champagne, D. E., and Ribeiro, J. M. (1994). Sialokinin I and II: vasodilatory tachykinins from the yellow fever mosquito Aedes aegypti. Proc. Natl. Acad. Sci. U.S.A. 91, 138–142. doi: 10.1073/pnas.91.1.138
Cheng, D., Zhang, Z., He, X., and Liang, G. (2013). Validation of reference genes in solenopsis invicta in different developmental stages, castes and tissues. PLoS ONE 8:e57718. doi: 10.1371/journal.pone.0057718
Detrain, C., and Pasteels, J. M. (1991). Caste differences in behavioral thresholds as a basis for polyethism during food recruitment in the ant, pheidole-pallidula (Nyl) (Hymenoptera, Myrmicinae). J. Insect Behav. 4, 157–176. doi: 10.1007/BF01054609
Detrain, C., and Pasteels, J. M. (1992). Caste polyethism and collective defense in the ant, pheidole-pallidula - the outcome of quantitative differences in recruitment. Behav. Ecol. Sociobiol. 29, 405–412.
Duarte, A., Weissing, F. J., Pen, I., and Keller, L. (2011). An evolutionary perspective on self-organized division of labor in social insects. Annu. Rev. Ecol. Evol. Syst. 42, 91–110. doi: 10.1146/annurev-ecolsys-102710-145017
Fernández-Marín, H., Zimmerman, J., and Wcislo, W. (2004). Ecological traits and evolutionary sequence of nest establishment in fungus-growing ants (Hymenoptera, Formicidae, Attini). Biol. J. Linnean Soc. 81, 39–48. doi: 10.1111/j.1095-8312.2004.00268.x
Fusca, D., Schachtner, J., and Kloppenburg, P. (2015). Colocalization of allatotropin and tachykinin-related peptides with classical transmitters in physiologically distinct subtypes of olfactory local interneurons in the cockroach (Periplaneta americana). J. Comp. Neurol. 523, 1569–1586. doi: 10.1002/cne.23757
Guerrieri, F. J., Nehring, V., Jørgensen, C. G., Nielsen, J., Galizia, C. G., and D'Ettorre, P. (2009). Ants recognize foes and not friends. Proc. R. Soc. Lond. B Biol. Sci. 276, 2461–2468. doi: 10.1098/rspb.2008.1860
Guescini, M., Sisti, D., Rocchi, M. B., Stocchi, L., and Stocchi, V. (2008). A new real-time PCR method to overcome significant quantitative inaccuracy due to slight amplification inhibition. BMC Bioinformatics 9:326. doi: 10.1186/1471-2105-9-326
Hart, A., Anderson, C., and Ratnieks, F. (2002). Task partitioning in leafcutting ants. Acta Ethol. 5, 1–11. doi: 10.1007/s10211-002-0062-5
Hughes, W. O., Bot, A. N., and Boomsma, J. J. (2010). Caste-specific expression of genetic variation in the size of antibiotic-producing glands of leaf-cutting ants. Proc. Biol. Sci. 277, 609–615. doi: 10.1098/rspb.2009.1415
Hughes, W. O. H., Sumner, S., Van Borm, S., and Boomsma, J. J. (2003). Worker caste polymorphism has a genetic basis in Acromyrmex leaf-cutting ants. Proc. Natl. Acad. Sci. U.S.A. 100, 9394–9397. doi: 10.1073/pnas.1633701100
Ignell, R., Root, C. M., Birse, R. T., Wang, J. W., Nässel, D. R., and Winther, Å. M. (2009). Presynaptic peptidergic modulation of olfactory receptor neurons in Drosophila. Proc. Natl. Acad. Sci. U.S.A. 106, 13070–13075. doi: 10.1073/pnas.0813004106
Ivens, A. B. F., Nash, D. R., Poulsen, M., and Boomsma, J. J. (2008). Caste-specific symbiont policing by workers of Acromyrmex fungus-growing ants. Behav. Ecol. 20, 378–384. doi: 10.1093/beheco/arn150
Johnson, B. R. (2010). Division of labor in honeybees: form, function, and proximate mechanisms. Behav. Ecol. Sociobiol. 64, 305–316. doi: 10.1007/s00265-009-0874-7
Johnson, B. R., and Linksvayer, T. A. (2010). Deconstructing the superorganism: social physiology, groundplans, and sociogenomics. Q. Rev. Biol. 85, 57–79. doi: 10.1086/650290
Kahsai, L., Martin, J. R., and Winther, A. M. (2010). Neuropeptides in the Drosophila central complex in modulation of locomotor behavior. J. Exp. Biol. 213, 2256–2265. doi: 10.1242/jeb.043190
Kamhi, J. F., Nunn, K., Robson, S. K. A., and Traniello, J. F. A. (2015). Polymorphism and division of labour in a socially complex ant: neuromodulation of aggression in the Australian weaver ant, Oecophylla smaragdina. Proc. R. Soc. B. 282. doi: 10.1098/rspb.2015.0704. Available online at: http://rspb.royalsocietypublishing.org/content/282/1811/20150704.article-info
Kanda, A., Iwakoshi-Ukena, E., Takuwa-Kuroda, K., and Minakata, H. (2003). Isolation and characterization of novel tachykinins from the posterior salivary gland of the common octopus Octopus vulgaris. Peptides 24, 35–43. doi: 10.1016/S0196-9781(02)00274-7
Kapheim, K. M., Pan, H., Li, C., Salzberg, S. L., Puiu, D., Magoc, T., et al. (2015). Genomic signatures of evolutionary transitions from solitary to group living. Science 348, 1139–1143. doi: 10.1126/science.aaa4788
Kelber, C., Rössler, W., and Kleineidam, C. J. (2010). Phenotypic plasticity in number of glomeruli and sensory innervation of the antennal lobe in leaf-cutting ant workers (A. vollenweideri). Dev. Neurobiol. 70, 222–234. doi: 10.1002/dneu.20782
Kelber, C., Rössler, W., Roces, F., and Kleineidam, C. J. (2009). The antennal lobes of fungus-growing ants (Attini): neuroanatomical traits and evolutionary Trends. Brain Behav. Evol. 73, 273–284. doi: 10.1159/000230672
Kleineidam, C. J., Obermayer, M., Halbich, W., and Rössler, W. (2005). A macroglomerulus in the antennal lobe of leaf-cutting ant workers and its possible functional significance. Chem. Senses. 30, 383–392. doi: 10.1093/chemse/bji033
Kleineidam, C. J., Rössler, W., Hölldobler, B., and Roces, F. (2007). Perceptual differences in trail-following leaf-cutting ants relate to body size. J. Insect Physiol. 53, 1233–1241. doi: 10.1016/j.jinsphys.2007.06.015
Kooij, P. W., Liberti, J., Giampoudakis, K., Schiøtt, M., and Boomsma, J. J. (2014). Differences in forage-acquisition and fungal enzyme activity contribute to niche segregation in panamanian leaf-cutting ants. PLoS ONE 9:e94284. doi: 10.1371/journal.pone.0094284
Kravitz, E. A. (2000). Serotonin and aggression: insights gained from a lobster model system and speculations on the role of amine neurons in a complex behavior. J. Comp. Physiol. 186, 221–238. doi: 10.1007/s003590050423
Kuebler, L. S., Kelber, C., and Kleineidam, C. J. (2010). Distinct antennal lobe phenotypes in the leaf-cutting ant (Atta vollenweideri). J. Comp. Neurol. 518, 352–365. doi: 10.1002/cne.22217
Larsen, J., Fouks, B., Bos, N., d'Ettorre, P., and Nehring, V. (2014). Variation in nestmate recognition ability among polymorphic leaf-cutting ant workers. J. Insect Physiol. 70, 59–66. doi: 10.1016/j.jinsphys.2014.09.002
Lucas, C., Hughson, B. N., and Sokolowski, M. B. (2010). Job switching in ants: role of a kinase. Commun. Integr. Biol. 3, 6–8. doi: 10.4161/cib.3.1.9723
Lucas, C., and Sokolowski, M. B. (2009). Molecular basis for changes in behavioral state in ant social behaviors. Proc. Natl. Acad. Sci. U.S.A. 106, 6351–6356. doi: 10.1073/pnas.0809463106
Maggi, C. A. (2000). Principles of tachykininergic co-transmission in the peripheral and enteric nervous system. Regul. Pept. 93, 53–64. doi: 10.1016/S0167-0115(00)00177-4
Myerscough, M. R., and Oldroyd, B. P. (2004). Simulation models of the role of genetic variability in social insect task allocation. Insectes Soc. 51, 146–152. doi: 10.1007/s00040-003-0713-1
Nässel, D. R. (1999). Tachykinin-related peptides in invertebrates: a review. Peptides 20, 141–158. doi: 10.1016/S0196-9781(98)00142-9
Nässel, D. R., and Homberg, U. (2006). Neuropeptides in interneurons of the insect brain. Cell Tissue Res. 326, 1–24. doi: 10.1007/s00441-006-0210-8
Nehring, V., Boomsma, J. J., and d'Ettorre, P. (2012). Wingless virgin queens assume helper roles in Acromyrmex leaf-cutting ants. Curr. Biol. 22, R671–R673. doi: 10.1016/j.cub.2012.06.038
Nelson, C. M., Ihle, K. E., Fondrk, M. K., Page, R. E., and Amdam, G. V. (2007). The gene vitellogenin has multiple coordinating effects on social organization. PLoS Biol. 5:e62. doi: 10.1371/journal.pbio.0050062
Neupert, S., Fusca, D., Schachtner, J., Kloppenburg, P., and Predel, R. (2012). Toward a single-cell-based analysis of neuropeptide expression in Periplaneta americana antennal lobe neurons. J. Comp. Neurol. 520, 694–716. doi: 10.1002/cne.22745
Nygaard, S., Zhang, G., Schiøtt, M., Li, C., Wurm, Y., Hu, H., et al. (2011). The genome of the leaf-cutting ant Acromyrmex echinatior suggests key adaptations to advanced social life and fungus farming. Genome Res. 21, 1339–1348. doi: 10.1101/gr.121392.111
Oldroyd, B. P., and Thompson, G. J. (2006). Behavioural genetics of the honey bee Apis mellifera. Adv. Insect Physiol. 33, 1–49. doi: 10.1016/S0065-2806(06)33001-9
Oster, G. F., and Wilson, E. O. (1978). Caste and ecology in the social insects. Monogr. Popul. Biol. 12, 1–352.
Patalano, S., Vlasova, A., Wyatt, C., Ewels, P., Camara, F., Ferreirab, P. G., et al. (2015). Molecular signatures of plastic phenotypes in two eusocial insect species with simple societies. Proc. Natl. Acad. Sci. U.S.A. 112, 13970–13975. doi: 10.1073/pnas.1515937112
Pavlou, H. J., Neville, M. C., and Goodwin, S. F. (2014). Aggression: tachykinin is all the rage. Curr. Biol. 24, R243–R244. doi: 10.1016/j.cub.2014.02.017
Pennefather, J. N., Lecci, A., Candenas, M. L., Patak, E., Pinto, F. M., and Maggi, C. A. (2004). Tachykinins and tachykinin receptors: a growing family. Life Sci. 74, 1445–1463. doi: 10.1016/j.lfs.2003.09.039
Perkins, J. R., Dawes, J. M., McMahon, S. B., Bennett, D. L., Orengo, C., and Kohl, M. (2012). ReadqPCR and NormqPCR: R packages for the reading, quality checking and normalisation of RT-qPCR quantification cycle (Cq) data. BMC Genomics 13:296. doi: 10.1186/1471-2164-13-296
Poels, J., Birse, R. T., Nachman, R. J., Fichna, J., Janecka, A., Vanden Broeck, J., et al. (2009). Characterization and distribution of NKD, a receptor for Drosophila tachykinin-related peptide 6. Peptides 30, 545–556. doi: 10.1016/j.peptides.2008.10.012
Poulsen, M., Bot, A., Currie, C., Nielsen, M., and Boomsma, J. (2003). Within-colony transmission and the cost of a mutualistic bacterium in the leaf-cutting ant Acromyrmex octospinosus. Funct. Ecol. 17, 260–269. doi: 10.1046/j.1365-2435.2003.00726.x
Poulsen, M., Fernández-Marín, H., Currie, C. R., and Boomsma, J. J. (2009). Ephemeral windows of opportunity for horizontal transmission of fungal symbionts in leaf-cutting ants. Evolution 63, 2235–2247. doi: 10.1111/j.1558-5646.2009.00704.x
Ratnieks, F. L., Foster, K. R., and Wenseleers, T. (2006). Conflict resolution in insect societies. Annu. Rev. Entomol. 51, 581–608. doi: 10.1146/annurev.ento.51.110104.151003
Robinson, G. E., Heuser, L. M., Leconte, Y., Lenquette, F., and Hollingworth, R. M. (1999). Neurochemicals aid bee nestmate recognition. Nature 399, 534–535. doi: 10.1038/21095
Robinson, G. E., Page, R. E. Jr., Strambi, C., and Strambi, A. (1989). Hormonal and genetic control of behavioral integration in honey bee colonies. Science 246, 109–112. doi: 10.1126/science.246.4926.109
Schultz, T. R., and Brady, S. G. (2008). Major evolutionary transitions in ant agriculture. Proc. Natl. Acad. Sci. U.S.A. 105, 5435–5440. doi: 10.1073/pnas.0711024105
Schulz, D. J., Barron, A. B., and Robinson, G. E. (2002). A role for octopamine in honey bee division of labor. Brain Behav. Evol. 60, 350–359. doi: 10.1159/000067788
Seeley, T. D. (1995). The Wisdom of the Hive: the Social Physiology of Honey Bee. Cambridge, MA: Harvard University Press.
Seid, M. A., Castillo, A., and Wcislo, W. T. (2011). The allometry of brain miniaturization in ants. Brain Behav Evol. 77, 5–13. doi: 10.1159/000322530
Seid, M. A., and Traniello, J. F. A. (2005). Age-related changes in biogenic amines in individual brains of the ant Pheidole dentata. Naturwissenschaften 92, 198–201. doi: 10.1007/s00114-005-0610-8
Severini, C., Improta, G., Falconieri-Erspamer, G., Salvadori, S., and Erspamer, V. (2002). The tachykinin peptide family. Pharmacol. Rev. 54, 285–322. doi: 10.1124/pr.54.2.285
Simola, D. F., Wissler, L., Donahue, G., Waterhouse, R. M., Helmkampf, M., Roux, J., et al. (2013). Social insect genomes exhibit dramatic evolution in gene composition and regulation while preserving regulatory features linked to sociality. Genome Res. 23, 1235–1247. doi: 10.1101/gr.155408.113
Smith, A. R., Muscedere, M. L., Seid, M. A., Traniello, J. F., and Hughes, W. O. (2013). Biogenic amines are associated with worker task but not patriline in the leaf-cutting ant Acromyrmex echinatior. J. Comp. Physiol. A Neuroethol. Sens. Neural Behav. Physiol. 199, 1117–1127. doi: 10.1007/s00359-013-0854-2
Song, W., Veenstra, J. A., and Perrimon, N. (2014). Control of lipid metabolism by tachykinin in Drosophila. Cell Rep. 9, 40–47. doi: 10.1016/j.celrep.2014.08.060
Stevenson, P. A., Dyakonova, V., Rillich, J., and Schildberger, K. (2005). Octopamine and experience-dependent modulation of aggression in crickets. J. Neurosci. 25, 1431–1441. doi: 10.1523/JNEUROSCI.4258-04.2005
Takeuchi, H., Yasuda, A., Yasuda-Kamatani, Y., Kubo, T., and Nakajima, T. (2003). Identification of a tachykinin-related neuropeptide from the honeybee brain using direct MALDI-TOF MS and its gene expression in worker, queen and drone heads. Insect Mol. Biol. 12, 291–298. doi: 10.1046/j.1365-2583.2003.00414.x
Takeuchi, H., Yasuda, A., Yasuda-Kamatani, Y., Sawata, M., Matsuo, Y., Kato, A., et al. (2004). Prepro-tachykinin gene expression in the brain of the honeybee Apis mellifera. Cell Tissue Res. 316, 281–293. doi: 10.1007/s00441-004-0865-y
Taskinen, S., and Warton, D. I. (2011). Robust estimation and inference for bivariate line-fitting in allometry. Biom J. 53, 652–672. doi: 10.1002/bimj.201000018
Toth, A. L., and Robinson, G. E. (2007). Evo-devo and the evolution of social behavior. Trends Genet. 23, 334–341. doi: 10.1016/j.tig.2007.05.001
Vandesompele, J., De Preter, K., Pattyn, F., Poppe, B., Van Roy, N., De Paepe, A., et al. (2002). Accurate normalization of real-time quantitative RT-PCR data by geometric averaging of multiple internal control genes. Genome Biol. 3, research0034.1–research0034.11.
Waddington, S. J., and Hughes, W. O. H. (2010). Waste management in the leaf-cutting ant Acromyrmex echinatior: the role of worker size, age and plasticity. Behav. Ecol. Sociobiol. 64, 1219–1228. doi: 10.1007/s00265-010-0936-x
Warton, D. I., Duursma, R. A., Falster, D. S., and Taskinen, S. (2012). smatr 3- an R package for estimation and inference about allometric lines. Methods Ecol. Evol. 3, 257–259. doi: 10.1111/j.2041-210X.2011.00153.x
Warton, D. I., Wright, I. J., Falster, D. S., and Westoby, M. (2006). Bivariate line-fitting methods for allometry. Biol. Rev. Camb. Philos. Soc. 81, 259–291. doi: 10.1017/S1464793106007007
Weber, N. A. (1972). The fungus-culturing behavior of ants. Am. Zool. 12, 577–587. doi: 10.1093/icb/12.3.577
Keywords: tachykinin, aggression, division of labor, colony defense, attine ants
Citation: Howe J, Schiøtt M and Boomsma JJ (2016) Tachykinin Expression Levels Correlate with Caste-Specific Aggression in Workers of the Leaf-Cutting Ant Acromyrmex echinatior. Front. Ecol. Evol. 4:55. doi: 10.3389/fevo.2016.00055
Received: 15 January 2016; Accepted: 29 April 2016;
Published: 18 May 2016.
Edited by:
Graham J. Thompson, Western University, CanadaReviewed by:
Brian R. Johnson, University of California, Davis, USAJulia Catherine Jones, University of Sussex, UK
Copyright © 2016 Howe, Schiøtt and Boomsma. This is an open-access article distributed under the terms of the Creative Commons Attribution License (CC BY). The use, distribution or reproduction in other forums is permitted, provided the original author(s) or licensor are credited and that the original publication in this journal is cited, in accordance with accepted academic practice. No use, distribution or reproduction is permitted which does not comply with these terms.
*Correspondence: Jack Howe, amFjay5ob3dlQGJpby5rdS5kaw==