- 1Institut de Recherches en Sciences de la Santé, Bobo Dioulasso, Burkina Faso
- 2UMR Maladies Infectieuses et Vecteurs: Ecologie, Génétique, Evolution et Contrôle, Centre National de la Recherche Scientifique 5290, Institut de Recherche pour le Développement 224, Université Montpellier, Montpellier, France
Previous studies have shown that Plasmodium parasites can manipulate mosquito feeding behaviors such as motivation and avidity to feed on vertebrate hosts, in ways that increase the probability of parasite transmission. These studies, however, have been mainly carried out on non-natural and/or laboratory based model systems and hence may not reflect what occurs in the field. We now need to move closer to the natural setting, if we are to fully capture the ecological and evolutionary consequences of these parasite-induced behavioral changes. As part of this effort, we conducted a series of experiments to investigate the long and short-range behavioral responses to human stimuli in the mosquito Anopheles coluzzii during different stages of infection with sympatric field isolates of the human malaria parasite Plasmodium falciparum in Burkina Faso. First, we used a dual-port olfactometer designed to take advantage of the whole body odor to gauge mosquito long-range host-seeking behaviors. Second, we used a locomotor activity monitor system to assess mosquito short-range behaviors. Compared to control uninfected mosquitoes, P. falciparum infection had no significant effect neither on long-range nor on short-range behaviors both at the immature and mature stages. This study, using a natural mosquito-malaria parasite association, indicates that manipulation of vector behavior may not be a general phenomenon. We speculate that the observed contrasting phenotypes with model systems might result from coevolution of the human parasite and its natural vector. Future experiments, using other sympatric malaria mosquito populations or species are required to test this hypothesis. In conclusion, our results highlight the importance of following up discoveries in laboratory model systems with studies on natural parasite–mosquito interactions to accurately predict the epidemiological, ecological and evolutionary consequences of parasite manipulation of vector behaviors.
Introduction
Since parasitic organisms exploit patchy host resources, transmission from one host to another represents one of the most risky and challenging step of their lifecycles. In response, parasites have evolved a series of strategies that increase their probability of transmission (Poulin, 2007). Of the many potential transmission strategies, parasite manipulation of host behavior is without doubt the most appealing. For example, ants infected with Ophiocordyceps fungi leave the colony, climb into the canopy (an ideal microenvironment for parasite sporulation and dispersal to new hosts), and express a “death grip” behavior by clamping down with jaws on a leaf vein shortly before dying (Hughes et al., 2011). Crickets infected with gordian worms dive into water, allowing the aquatic parasite to exit their body and mate (Thomas et al., 2002). These are just some of the many examples of the dramatic impact parasites can have on their host's behavior (Moore, 2002). Parasite manipulation has now been documented in a large range of host-parasite associations, and studies indicate that it is a widespread phenomenon in nature with important ecological and evolutionary implications (Thomas et al., 2005; Lefèvre et al., 2009a,b; Poulin, 2010; Hughes et al., 2012).
Besides ecological and evolutionary relevance, host manipulation by parasite may also have profound significance for human health. Many manipulative parasites are responsible for devastating vector-borne diseases such as dengue fever, malaria, leishmaniasis, or sleeping sickness. These parasites can indeed induce changes in the behaviors of their insect vectors that affect the frequency of interactions between hosts and vectors (Molyneux and Jefferies, 1986; Moore, 1993; Hurd, 2003; Lefèvre et al., 2006; Lefèvre and Thomas, 2008). In particular, several vector-borne parasites seem able to induce increased biting rates in their arthropod vectors by interfering with the ingestion process: Leishmania spp. and Yersinia pestis block the foregut of sand flies and fleas, respectively (Rogers, 2012; Rebeil et al., 2013); Trypanosoma spp. obstructs phagoreceptors (Jenni et al., 1980) and modifies the salivary composition of tsetse flies (Van Den Abbeele et al., 2010); and Plasmodium spp. and Trypanosoma spp. reduce activity of the apyrase salivary protein (platelet aggregation inhibitor) in mosquitoes and kissing bugs, respectively (Añez and East, 1984; Rossignol et al., 1984). These mechanisms seem to impair the vector's ability to engorge and therefore induce them to feed several times on vertebrate hosts.
Biting rate is a key predictor of transmission intensity of vector-borne diseases (MacDonald, 1957). Specifically, the transmission potential of vector-borne parasites is strongly determined by the number of bitten vertebrate hosts: the greater the number of host bitten by infectious vectors, the more intense transmission will be. Accordingly, some studies found that increased biting rate by infectious vectors not only increase the number of bites on the same individual vertebrate host, but also the number of bites on different hosts, thereby increasing parasite transmission (Jenni et al., 1980; Rogers and Bates, 2007; Van Den Abbeele et al., 2010).
In addition, the onset of behavioral changes seems to be synchronized with the parasite development. When the parasite is not fully mature (i.e., non-transmissible stage), it changes the behavior of the vector in a way that reduces the contact rate with vertebrate hosts. Since biting is risky (e.g., host defensive behaviors can kill the vector and its parasite), reduced biting rate seems beneficial to the parasite (Schwartz and Koella, 2001). In contrast, when the parasite is mature and ready to be transmitted, it modifies the behavior of its vector in a way that increases the contact with the vertebrate host and hence the transmission. This “stage-dependant” manipulation seems to be the rule in the above cited examples (Rogers and Bates, 2007; Lefèvre and Thomas, 2008; Van Den Abbeele et al., 2010).
Over the last three decades, particular attention has been devoted to behavioral changes in malaria-infected mosquitoes (Hurd, 2003; Lefèvre and Thomas, 2008; Cator et al., 2012). Malaria is caused by protozoan parasites of the genus Plasmodium which are transmitted through the bites of infected Anopheles mosquitoes. Inside its vector, the parasite undergoes complex developmental transformations. Shortly after the ingestion of an infectious blood meal, Plasmodium male and female gametocytes fuse to form zygotes within the mosquito midgut. Zygotes then develop into motile ookinetes that penetrate the gut wall to form oocysts (non-transmissible parasite stage). There, oocysts undergo several mitotic divisions resulting in hundreds of sporozoites (transmissible parasite stage) that are released into the haemoceol about 2 weeks post-infection. At this stage, the parasites migrate to the salivary glands from which they can be injected into another human host during subsequent blood meals.
Mosquito behavioral traits altered by malaria sporozoites are multiple and include increased response to host odors (Rossignol et al., 1986; Cator et al., 2013), increased landing and biting activity (Rossignol et al., 1984, 1986; Wekesa et al., 1992; Anderson et al., 1999; Koella et al., 2002; Smallegange et al., 2013), increased number of feeds (Koella et al., 1998) and increased blood volume intake (Koella and Packer, 1996; Koella et al., 1998, 2002). The exact proximate mechanisms by which malaria parasites modify these traits remain elusive but modifications of salivary and brain proteins (Rossignol et al., 1984; Shandilya et al., 1999; Lefèvre et al., 2007) as well as changes in the responsiveness of odorant receptors (Cator et al., 2013) have been suggested.
Altogether these studies strongly support the existence of parasite manipulation of mosquito behavior. However, they have been carried out on either artificial model systems or field mosquitoes, and this can be problematic. First, using host-parasite combinations that do not occur in nature excludes the coevolutionary processes that shape parasite manipulation. Accordingly, findings on unnatural mosquito-Plasmodium associations do not always reflect natural interactions (Aguilar et al., 2005; Boëte, 2005; Cohuet et al., 2006; Dong et al., 2006; Tripet et al., 2008; Lefèvre et al., 2013; Severo and Levashina, 2014). Furthermore, the stimuli used to elicit mosquito behavioral responses in the laboratory studies cited above derived from either inappropriate - and sometimes anesthetized—hosts (e.g., human odors for mosquitoes infected with rodent Plasmodium) or from extracts adsorbed on worn pieces of cloth. Finally, sampling wild specimens can lead to some misinterpretations due to possible confounding effects. This is of particular relevance in the field of parasitology since infection can be more a consequence than a cause of host behavioral traits. For example, while the increased biting rate observed in sporozoite-infected mosquitoes can result from infection, it can also be a mere intrinsic mosquito characteristic. In other words, infected mosquitoes may exhibit increased biting rates not because of being infected but just because of an innate aggressiveness, and thus making these individuals more likely to become infected compared to less aggressive mosquitoes. Another possible confounding effect is mosquito age which is associated to infection (i.e., increased likelihood of sporozoite infection as mosquitoes become older, Koella and Packer, 1996; Koella et al., 1998; but see Wekesa et al., 1992).
As a result, there is a critical lack of data on the effects of malaria infection on its vector behavior using both controlled conditions and a natural host-parasite association. The current study aims to fill this gap using the parasite P. falciparum, responsible for causing the most severe form of human malaria, and the mosquito Anopheles coluzzii (formerly the M molecular form of An. gambiae s.s.), a major vector of P. falciparum in Africa. We experimentally challenged An. coluzzi with sympatric field isolates of P. falciparum using direct membrane feeding assays and, through a series of experiments, examined the effects of immature and mature infections on mosquito host-seeking behaviors. First, we used a dual-port olfactometer designed to take advantage of the whole human body odor (breath and skin emanations) to gauge mosquito long-range behaviors. Second, we used a locomotor activity monitor system to gauge mosquito short-range behaviors.
Materials and Methods
Mosquitoes
Three- to five-day-old laboratory-reared female of An. coluzzii were obtained from an outbred colony established in 2008 and repeatedly replenished with F1 from wild-caught mosquito females collected in Kou Valley (11°23′14″N, 4°24′42″W), 30 km from Bobo Dioulasso, south-western Burkina Faso (West Africa) and identified by PCR-RFLP (Fanello et al., 2002). Mosquitoes were maintained under standard insectary conditions (27 ± 2°C, 70 ± 5% relative humidity, 12:12 LD). Adult mosquitoes were provided with a solution of 5% glucose and water, and the larvae were breed in the laboratory with ad libitum Tetramin® food. Female mosquitoes were starved for sugar 24 h prior access to a blood meal to ensure willingness to feed.
Mosquito Infection
Experimental infections were performed as described in Alout et al. (2013) and Vantaux et al. (2014). Briefly, 3–5 days old females were fed through membranes on gametocyte (P. falciparum transmission stages)-infected blood from malaria patients in Burkina Faso. Gametocyte carriers were selected by examining thick blood smears from children aged between 5 and 11 from two villages in southwestern Burkina Faso (Dande and Soumousso, located 60 km north and 40 km southeast of Bobo-Dioulasso, respectively). As a negative control (uninfected mosquitoes), females were fed on the same blood in which gametocytes were heat-inactivated. This heat-inactivation inhibits the infection and does not affect the blood nutritive quality (Sangare et al., 2013). This was done to avoid the potential confounding effects of different blood origins on performance and behaviors of infected and control mosquitoes (Sangare et al., 2013). Parasite inactivation was performed by placing collected blood in a thermo-mixer and heating it at 43°C for 15 min and 900 rpm while the remaining infectious blood was maintained at 37°C. Three hundred μl of blood were distributed in membrane feeders maintained at 37°C by water jackets. Cups containing 80 mosquitoes were placed under the feeders to allow blood feeding through Parafilm® membranes for 2 h. Fed females were sorted out and placed in new cages (30X30X30 cm) where they had constant access to 5% glucose solution on cotton wool pads until the behavioral assays.
Long-range Behavioral Assay
We studied the olfactory responses of our mosquitoes to human odor and control outdoor air using a dual-port olfactometer (Figure 1). The dual-port olfactometer was made of glass. The set-up consisted of two odor-sources connected to two collecting boxes (L X l X h = 40X20X20 cm) linked by two glass tubes (L = 60 cm, Ø = 10 cm) to a releasing cage (L X l X h = 60X40X40 cm). Odor stimuli came from two tents (L X l X h = 250X150X150 cm) connected to the two collecting boxes of the olfactometer by air vent hoses (Scanpart®, DXL = 10X300 cm). Fans, setup at the junction of the air vent hose and the tent, drew air from the tents to the olfactometer, providing the odor laden air current against which mosquitoes were induced to fly. A mosquito net was placed at the junction of the air vent hose with the collecting boxes to restrain responding mosquitoes inside the box, and to prevent them from flying into the hoses and into the tents. The tents were located outdoors and the olfactometer inside an experimental room. The air speed in the releasing cage of the dual-port olfactometer was regulated at 15 cm/s (±2 cm/s) using a Testo 425- Compact Thermal Anemometer (Testo, Forbach, France) equipped with a hot wire probe [range: 0 to+20 m/s, accuracy: ±(0.03 m/s + 5% of mv)]. The experimental room was at ambient temperature (27.5 ± 2.5°C) and relative humidity (80 ± 10%).
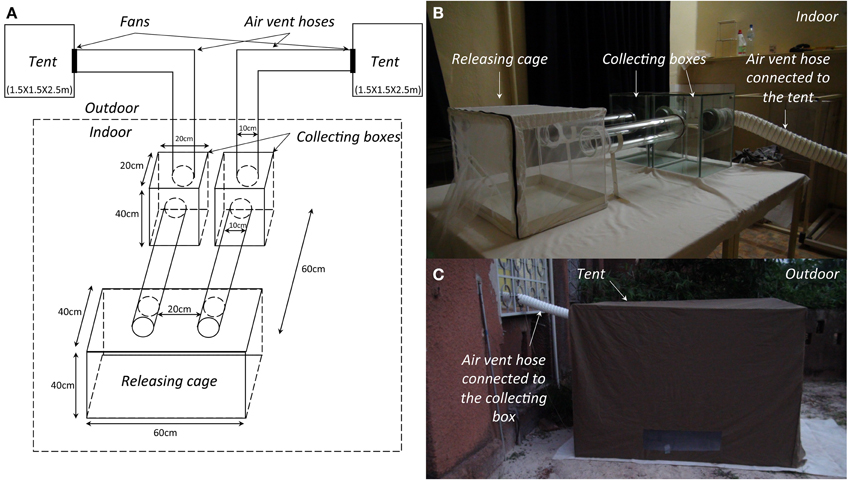
Figure 1. (A) Schematic representation of the dual-port olfactometer. (B) Photo of the indoor part of the device. (C) Photo of the outdoor part of the device.
Olfactometer assays were carried out between 6 and 8 days post-infection, corresponding to the oocyst non-transmissible parasite stage, and between 13 and 17 days post-infection, corresponding to the sporozoite transmissible parasite stage. Mosquitoes that received an infectious blood-meal (exposed to infection) and mosquitoes that received an inactivated blood-meal (unexposed) were colored in the morning of the test and had access to water only. Mosquitoes were colored with one of two different colored powders (Luminous Powder Kit, BioQuip), corresponding to their exposure status (exposed vs. unexposed). The matching between exposure status and colors was switched between each test. In the evening, about 20 exposed and 20 unexposed mosquitoes were simultaneously released into the releasing cage and allowed to respond for 30 min. During this timeframe, mosquitoes that were activated by the stimuli left the releasing cage and flew upwind into the collecting boxes from which they were retrieved. Two tests per evening were carried out between 7 and 11 p.m. Odor stimuli (human odor vs. outdoor air) were alternated between the right and left arm to avoid side effect. Different human volunteers were used as odor sources to obviate any host effect. We used a total of 15 volunteers. All volunteers who served as the human odor source were male Burkinabe between 20 and 44 years old and 50–80 kg who lived in Bobo Dioulasso. Volunteers were in good health condition. Before the test, volunteers were asked not to take a shower, apply perfume or use repellent, smoke or eat garlic and onion (Lefèvre et al., 2009c, 2010). The volunteers acting as odor sources sat on a chair inside the tent. To compare mosquito behavioral responses between immature (oocyst) and mature (sporozoite) infection phases, we used the same volunteers at both periods to reduce the background noise inherent to the high variability of mosquito response to different human hosts (Mukabana et al., 2002, 2004; Qiu et al., 2006, 2011).
Each batch of mosquitoes was tested once, so that a fresh batch of naive mosquitoes was used for each test. A total of 755 unexposed and 682 exposed mosquitoes were released. At the end of a test, the mosquitoes inside each of the two upwind collecting boxes and the releasing box were removed with an aspirator and counted. After each test, the olfactometer was washed with 70% alcohol to remove odor contaminants left from previous test. Similarly, latex gloves were worn by the experimenter to avoid contamination of the equipment. This setup allows to study mosquito long-range behavioral responses from total host emanations instead of fractions thereof, such as skin extracts adsorbed on worn stockings (e.g., Smallegange et al., 2013). Two behavioral responses were gauged: the long-range activation (proportion of mosquitoes caught in both collecting boxes out of the total number released, that is the proportion of mosquitoes engaging in take-off and up-wind flight) and attraction (proportion of mosquitoes caught in the collecting box emitting human odor out of the total number retrieved from both collecting boxes, that is the proportion of mosquitoes flying toward human volunteers' odors), both of which are part of the behavioral sequence leading a mosquito toward its host, and through which host–seeking is expressed.
Oocyst prevalence (proportion of P. falciparum-infected females) and intensity (number of oocysts in the midgut of infected females) were assessed by dissecting mosquito midguts the morning after behavioral testing (midguts were stained in a 1% mercurochrome solution and examined by light microscope). Heads and thoraces were used to determine sporozoite prevalence (proportion of infected females) by PCR assays (Morassin et al., 2002). Three groups of mosquitoes were thus obtained: (i) females that received a gametocyte-positive blood and became successfully infected (“exposed-infected” hereafter); (ii) females that received a gametocyte-positive blood and remained uninfected (“exposed-uninfected”); and (iii) females that received a heat-treated gametocytic blood (“unexposed”). Three replicates with a different gametocyte carrier each time were carried out.
Short-range Behavioral Assay I
The short-range behavior of exposed and unexposed An. coluzzii mosquitoes was studied using a locomotor activity monitor system (TriKinetics, Waltham, MA) following the procedure previously described (Lima-Camara et al., 2011; Rund et al., 2012; Figures 2A,B). Briefly, mosquitoes were placed individually in glass tubes (2.5 cmX15 cm) with an infrared beam bisecting each tube and detecting motion. As an individual interrupted an infrared beam that crossed the tube, this interruption was detected by the monitoring system and added to the tube's activity count. In the morning of the third day post-infectious blood meal, females were set-up in the tubes closed by mosquito nets. They were provided daily with a 5% glucose solution. Unlike the long-range behavioral assay for which we used total body emanations, the short-range assay used clean or worn socks as a source of stimuli. The infrared beam was placed next to the sock to allow contact between the mosquito and the socks and each time the mosquito came back and forth on the sock, infrared beam breaks were recorded (Figure 2B).
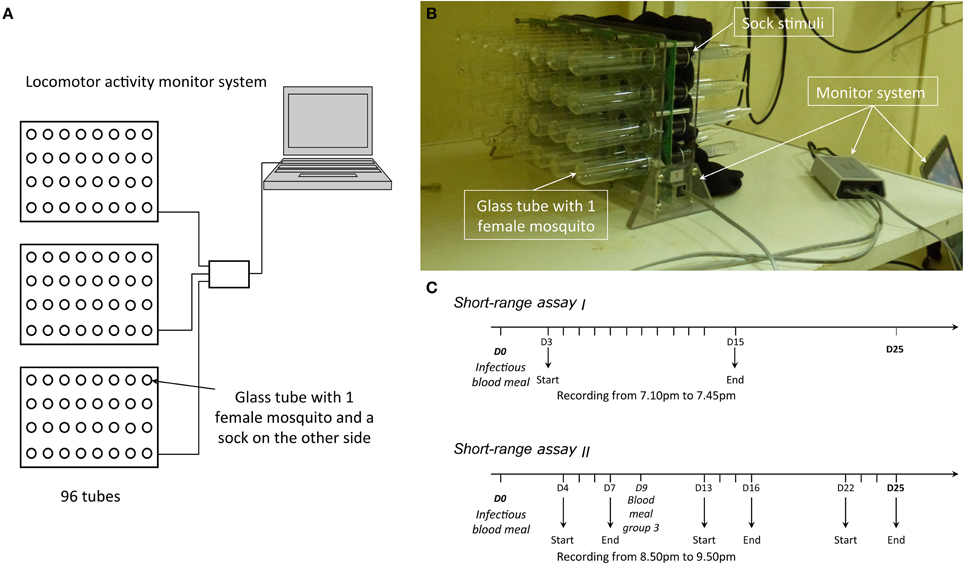
Figure 2. (A) Schematic representation of the locomotor activity monitor system. (B) Photo of the device. (C) Schematic representation of the testing periods.
Tests were conducted every evening between 7.10 and 7.45 p.m. from day 3 post-blood meal to day 15 post-blood meal except day 14 during which a power cut prevented the daily run (Figure 2C). The room was kept dark, and the temperature and relative humidity were similar to standard insectary conditions (27 ± 2°C, 70 ± 5% relative humidity, 12:12 LD). Sugar was removed at 6 pm and the mosquito nets replaced by cleaned ones. Latex gloves were worn by the experimenter to avoid contamination of the equipment. The socks were set-up and mosquitoes were left to acclimatize for 20 min prior recordings. The socks were worn for 8 h on the same day as the behavioral assay. Two pairs of socks per volunteer were used, such that they were cleaned before being re-used the second following day. All socks were cleaned with the same soap and water as well as dried in the same conditions. Three monitors were used allowing to simultaneously recording up to 96 individuals (Figures 2A,B). Stimuli (worn vs. unworn sock) as well as mosquito status (exposed vs. unexposed) were randomized on each monitor. The same mosquito individuals were followed daily; unless they died in which case they were replaced by naïve individuals kept in 30 × 30 cm cages. Four human volunteers worn the socks and a total of 265 mosquitoes were used.
Two behavioral responses were gauged: the short-range activation (proportion of mosquitoes that crossed at least once the infraread beam) and activity (number of infrared beam breaks in active individuals).
For this assay, the venous blood collected from the gametocyte-infected patients was centrifuged at 3000 rpm at 37°C for 3 min, and the serum was replaced by the same volume of European AB serum in order to limit potential effect of human transmission blocking immunity (Gouagna et al., 2004). The oocyst prevalence (number of P. falciparum infected females) and intensity (number of oocysts in the midgut of infected females) were assessed by dissecting mosquito midguts on a subset of additional (that were not used in the behavioral assay) females (n = 109), 6 days post-infectious blood meal. The midguts were stained in a 1% mercurochrome solution and examined by light microscope to detect and count the oocysts (Alout et al., 2014). Two different gametocyte carriers were used in one replicate.
Short-range Behavioral Assay II
This experiment used the same set-up as described above with some slight modifications. Mosquito activity was recorded during three periods: (i) from day 4 to day 7, (ii) from day 13 to day 16, and (iii) from day 22 to day 25 (Figure 2C). A new batch of mosquitoes was used for each period. The tests were carried out between 8.50 and 9.50 p.m. and socks were positioned at 8.25 p.m. Anopheles coluzzii mosquitoes exhibit daily nocturnal rhythms of flight activity with a peak at dusk (Jones and Gubbins, 1978; Rund et al., 2012). As short-range assay I recordings were taken soon after this dusk-related peak in flight activity possibly blurring the expected behavioral differences, we investigated whether a later recording would coincide with possible increased behavioral differences between exposed and unexposed mosquitoes. To assess the possible effect of heat-killed gametocytes on mosquito behavior, individuals were fed on three different types of blood for their first blood-meal: P. falciparum infected blood, the same heat-inactivated P. falciparum infected blood and blood from a healthy host (no P. falciparum infection) blood donor. This experiment therefore used two blood donors (one gametocyte carrier and one uninfected volunteer) and produce three groups of mosquitoes: (i) females that received a gametocyte-positive blood (“exposed” hereafter), (ii) females that received a heat-treated gametocytic blood (“unexposed”), and (iii) females that received a non-infectious blood (“unexposed healthy donor”). Finally, the batch of mosquitoes monitored from day 22 to day 25 received a second, non-infectious, blood meal from rabbit on the ninth day post-infectious blood meal (Smallegange et al., 2013). As for the odor stimuli, we used three socks-wearing volunteers and a total of 305 mosquitoes.
Oocyst prevalence (proportion of P. falciparum-infected females) and intensity (number of oocysts in the midgut of infected females) were assessed by dissecting mosquito midguts on a subset of additional females (n = 50), 7 days post-infectious blood meal. The midguts were stained in a 1% mercurochrome solution and examined by light microscope to detect and count oocysts (Alout et al., 2014). The assay was carried out on one replicate using one gametocyte carrier and one healthy blood donor and the same behavioral responses were gauged, i.e., mosquito short-range activation and activity.
Statistical Analyses
Long-range Behavioral Assay
Binomial generalized linear mixed models (“glmer” function in “lme4” package) were fitted to investigate the long-range activation (proportion of mosquitoes caught in both collecting boxes out of the total number released) and attraction (proportion of mosquitoes caught in the collecting box emitting human odor out of the total number retrieved from both collecting boxes). In these GLMMs, infection status (three levels: unexposed, exposed-infected, exposed-uninfected), time post-blood meal (two levels corresponding to the oocyst stage—6 to 8 days post-infectious blood meal—and to the sporozoite stage—13 to 17 days post-infectious blood meal), volunteer placed in the tent and the interaction between infection status and time post-blood meal were coded as fixed factors, while date and replicate were coded as random factors. Binomial GLMs were used to assess the effect of oocyst intensity on mosquito long-range activation and attraction using a data subset made of infected females at the oocyst stage only.
Short-range Behavioral Assays
A binomial GLMM (“glmer” function in “lme4” package) was used to investigate the short-range activation (proportion of females that crossed the infrared beam at least once) and a negative binomial GLMM (to account for overdispersion—“glmmadmb” function in “glmmADMB” package) was used to investigate activity (number of infrared beam breaks). In these models, odor stimuli (worn sock vs. clean sock), parasite exposure (two and three levels for assay I and II, respectively: exposed, unexposed, and unexposed healthy donor for experiment II only), time post-blood meal and their interactions were coded as fixed factors and individual mosquito was coded as a random factor to account for repeated measurements on the same individual.
For model selection, we used the stepwise removal of terms, followed by likelihood ratio tests (LRT). Term removals that significantly reduced explanatory power (P < 0.05) were retained in the minimal adequate model (Crawley, 2007). All analyses were performed in R v. 3.0.3 (R Development Core Team, 2013). Results are presented as mean ± standard error (se) and proportion ± confidence interval (CI).
Ethic Statement
Ethical approval was obtained from the Centre Muraz Institutional Ethics Committee under agreement no. A003-2012/CE-CM. The protocol conforms to the declaration of Helsinki on ethical principles for medical research involving human subjects (version 2002) and informed written consent were obtained from all volunteers.
Results
Long-range Behavioral Assay
We tested a total of 1437 females (755 unexposed females and 682 females exposed to an infectious blood-meal). Among the 682 females exposed to an infectious blood-meal, 172 out of 352 females (48.9 ± 5%) harbored parasites at the oocyst stage and significantly less, 122 out of 330 (36.9 ± 5%), harbored parasite at the sporozoite stage [X, P = 0.003; Figure S1A]. The mean oocyst intensity was 15.26 ± 2.06 (Figure S1B). Overall 495 out of 1437 An. coluzzii left the releasing cage to fly upwind into one of the two collecting boxes (long-range activation of 34.45 ± 2.5%). We found no significant effect of mosquito infection status [three levels: unexposed females, exposed-infected females, exposed-uninfected females; X, P = 0.93, Figure 3A] nor of the time post-blood meal [two-levels: days 6–8 and days 13–17; X, P = 0.29; Figure 3A], indicating that neither exposure to the parasite nor the time elapsed since the blood meal affected mosquito long-range activation. Similarly, the analysis of the subset of infected females at the oocyst stage showed no significant effect of parasite intensity on mosquito long-range activation [X, P = 0.34]. Finally, there was no infection status by time post-blood meal interaction [X, P = 0.94, Figure 3A], indicating that parasite had no effect on mosquito activation regardless of its development stage. Long-range activation was however significantly influenced by the volunteer involved [X, P = 0.007; Figure S2].
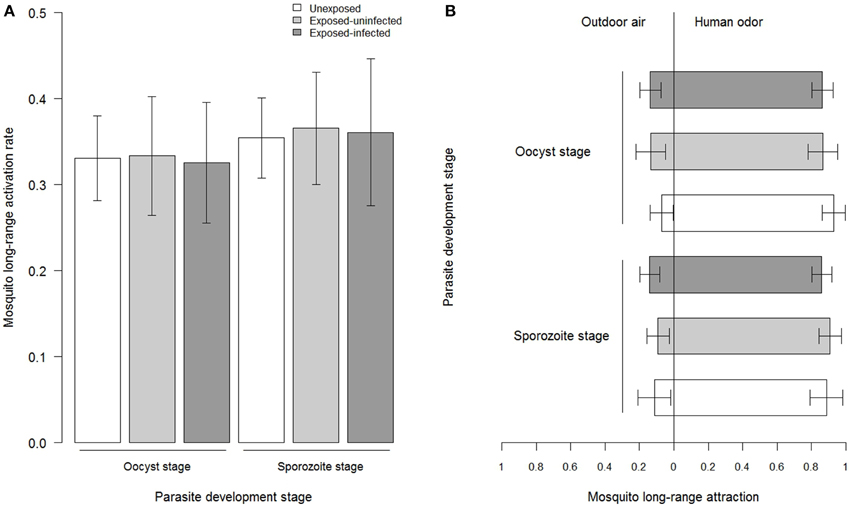
Figure 3. Mosquito long-range behavioral responses. (A) Activation rate, expressed as the proportion of mosquitoes caught in both collecting boxes out of the total number released in the releasing cage for each parasite stage and for the three mosquito's infection status. (B) Attraction, expressed as the proportion of mosquitoes caught in the collecting box emitting human odor out of the total number retrieved from both collecting boxes for each parasite stage and for the three mosquito's infection status. The oocyst stage corresponds to tests carried out from 6 to 8 days post-infectious blood-meal and the sporozoite stage corresponds to tests carried out from 13 to 17 days post-blood meal. Error bars show the 95% confidence limits.
Overall 435 out of the 495 activated mosquitoes chose the port emitting human odor (87.88 ± 3%, significant departure from a 50 to 50 distribution, z = 14.38, P < 0.0001; Figure 3B). There was no significant effect of the infection status [X, P = 0.55] nor of the time post-blood meal [X, P = 0.74] on mosquito attraction (Figure 3B). Similarly, analysis of the subset of infected females at the oocyst stage showed no influence of parasite intensity on mosquito long-range attraction [X, P = 0.87]. Finally, there was no infection status by time post-blood meal interaction [X, P = 0.6; Figure 3B], indicating that parasite had no effect on mosquito attraction toward human host regardless of its development stage. Long-range attraction was however significantly affected by the volunteer involved [X, P < 0.001; Figure S2].
Short-range Behavioral Assay I
Among the 109 additional females exposed to an infectious blood meal but not used for behavioral tests, 54 females (49.5 ± 12%) harbored parasite at the oocyst stage (Figure S3A). The mean oocyst intensity was 8.87 ± 1.16 (Figure S3B).
We tested a total of 265 mosquitoes over 12 evenings. Among the 1149 records, 346 (30.1%) were active (i.e., mosquitoes crossed the infrared beam at least once to land/probe on the socks). We did not find a significant effect of parasite exposure [X, P = 0.87] nor of odor stimuli [X, P = 0.2] on mosquito short-range activation. Activation was however affected by the time post-blood meal [X, P < 0.0001; Figure 4A]. There was no interaction between parasite exposure and time post-blood meal [X, P = 0.13], indicating that the effect of time post-blood meal on mosquito activation was consistent across exposed and unexposed mosquitoes. Similarly, there was no odor stimuli by parasite exposure interaction [X, P = 0.08], indicating that exposed mosquitoes were not more active than unexposed females when confronted to a worn sock. There was also no odor stimuli by time post-blood meal interaction [X, P = 0.15]. Finally, we did not find a significant three-way interaction between parasite exposure, time post-blood meal and odor stimuli [X, P = 0.9; Figure 4A].
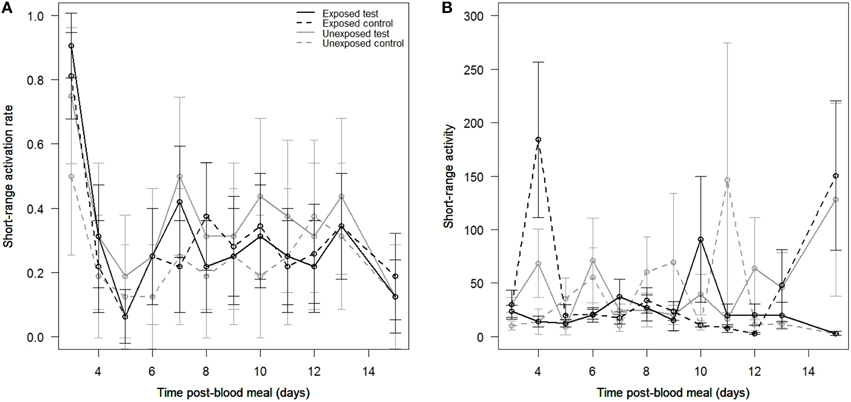
Figure 4. Mosquito short-range behavioral responses (assay I). Effects of time post-blood meal, parasite exposure, and odor stimuli on (A) the short-range activation of mosquito females, expressed as the proportion of mosquitoes that crossed at least once the infrared beam, and on (B) the short-range activity of mosquito females, expressed as the mean number of infrared beam breaks in active individuals. Control: clean sock, test: worn sock.
We found an average short-range activity of 35.4 ± 4.12 beam breaks/35 min. Mosquito activity was not significantly influenced by parasite exposure [X, P = 0.2] nor by odor stimuli [X, P = 0.73]. The time elapsed since the blood-meal had no effect on mosquito short-range activity [X, P = 0.33]. The interaction between parasite exposure and time post-blood meal did not significantly affect mosquito short-range activity [X, P = 0.48], indicating that exposed mosquitoes were not significantly more active over time compared to unexposed mosquitoes. Similarly, there was no odor stimuli by parasite exposure [X, P = 0.07], indicating that exposed mosquitoes were not more active than unexposed counterparts in the presence of a worn sock. There was no odor stimuli by time post-blood meal interaction [X, P = 1] and no three-way interaction [X, P = 0.84; Figure 4B].
Short-range Behavioral Assay II
Among the 50 females exposed to an infectious blood meal but not used in the behavioral tests and dissected 7 days later, 39 harbored parasite at the oocyst stage (78 ± 11%; Figure S3A). The mean oocyst intensity was 12.08 ± 1.83 (Figure S3B).
We tested a total of 305 mosquitoes over 12 evenings. Among the 875 records, 368 (42.06%) were active (i.e., mosquitoes crossed the infrared beam at least once to land/probe on the socks). We did not find a significant effect of blood meal type on mosquito short-range activation [X, P = 0.32, Figure 5A], indicating that exposed, unexposed and unexposed healthy donor mosquitoes had similar short-range activation. The short-range activation was not significantly affected by the time post-blood meal [X, P = 0.24] or by odor stimuli [X, P = 0.85]. There was no interaction between blood meal type and time post-blood meal [X, P = 0.53], indicating that exposed mosquitoes, unexposed and unexposed healthy donor mosquitoes displayed similar short-range activation over time. Similarly, there were no significant interactions between odor stimuli and blood meal type [X, P = 0.84] and between odor stimuli and time post-blood meal [X, P = 0.95]. Finally, there was no significant three-way interaction effect [X, P = 0.51; Figure 5A].
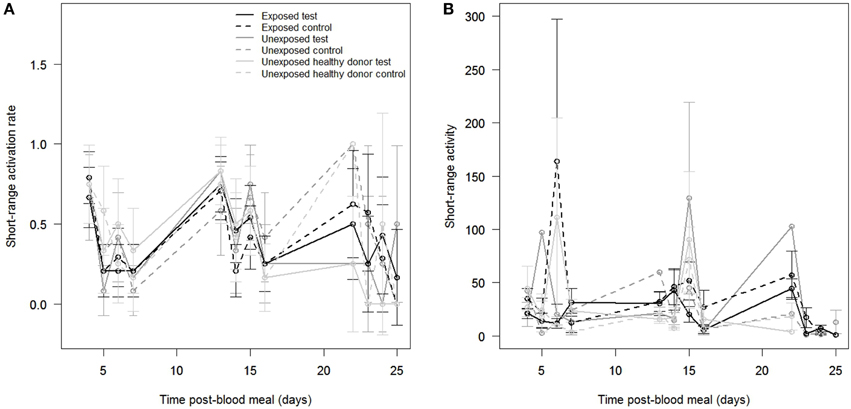
Figure 5. Mosquito short-range behavioral responses (assay II). Effects of time post-blood meal, blood meal type and odor stimuli on (A) the short-range activation of mosquito females, expressed as the proportion of mosquitoes that crossed at least once the infrared beam, and on (B) the short-range activity of mosquito females, expressed as the mean number of infrared beam breaks in active individuals. Control: clean sock, test: worn sock.
The average short-range activity was 34.7 ± 4.3 beam breaks/hour. We did not find a significant effect of blood meal type on mosquito short-range activity [X, P = 0.95; Figure 5B]. Mosquito female activity was not significantly affected by odor stimuli [X, P = 0.45] nor by the time post-blood meal [X, P = 0.52]. The interaction between blood meal type and time post-blood meal was not significant [X, P = 0.83], indicating that exposed, unexposed and unexposed healthy donor mosquitoes displayed similar short-range activity over time. Similarly, there were no significant interactions between odor stimuli and time post-blood meal [X, P = 0.89] and between odor stimuli and blood meal type exposure [X, P = 0.07]. Finally, there was no significant three-way interaction effect [X, P = 0.38; Figure 5B].
Discussion
Several laboratory and field studies have demonstrated behavioral modifications in Plasmodium-infected mosquitoes such as motivation and avidity to feed on vertebrate hosts, in ways that increase contact with vertebrate hosts and hence increase the probability of parasite transmission (Rossignol et al., 1984, 1986; Wekesa et al., 1992; Koella and Packer, 1996; Koella et al., 1998, 2002; Anderson et al., 1999; Cator et al., 2013; Smallegange et al., 2013). Because investigations on sympatric host-parasite combinations are essential to integrate local co-adaptation phenomena (Munstermann, 1994; Norris et al., 2001; Joy et al., 2008; Harris et al., 2012; Baeshen et al., 2014) as well as to validate the results found in unnatural host-parasite associations (Boëte, 2005; Cohuet et al., 2006; Tripet et al., 2008), the current study examined for the first time in controlled laboratory conditions whether natural field isolates of the deadliest human malaria parasite P. falciparum can manipulate the behavior of its vector An. coluzzii. We first used a dual-port olfactometer to investigate the activation and attraction of uninfected and infected mosquitoes in response to either outdoor air or human odors. Plasmodium falciparum infection had no significant effect on mosquito behaviors irrespective of parasite developmental stage (oocyst vs. sporozoite stages). While our device allows the study of odor-mediated behaviors and obviates short-range stimuli such as visual cues, warm, moist convective currents, and host movement, most evidence for parasite influence on mosquito behaviors come from studies using short-range devices. Therefore, it is possible that sporozoite-infected An. coluzzii presents similar behavioral responses as uninfected counterparts in the early stages of the host-seeking process, when it mostly responds to long-range odor stimuli, and then display increased responses at a shorter range, when other cues become more important. To test this possibility we used a locomotor activity monitor system (short-range behavioral assays I and II). Again, we found no effect of parasite exposure on mosquito behavior, neither during the early non-transmissible infection phase, nor during the late transmissible infection phase.
Altogether our findings contrast with the previous evidence for the existence of parasite manipulation of mosquito behavior. Several hypotheses could explain these contradictory results. First, it is possible that natural selection has not favored the evolution of manipulation in this population because uninfected wild An. coluzzii already take multiple blood meals during each gonotrophic cycle (Scott and Takken, 2012). Since feeding is costly (e.g., host defensive behavior) it is possible that, in this system, any further increases in vector feeding attempts would not significantly increase parasite fitness (as suggested for Aedes aegypti mosquitoes infected with dengue viruses, Putnam and Scott, 1995). Future studies investigating the fitness costs and benefits associated to feeding behavior of uninfected An. coluzzii in our study area would be necessary to test this hypothesis.
Second, since manipulation can be costly for the vector (e.g., higher mortality risk; Anderson et al., 2000; Schwartz and Koella, 2001), it is possible that during their long co-evolutionary history, the mosquito vector has evolved resistance (Daoust et al., 2015). In their seminal paper, May and Anderson (1979) noted that “because the generation times of most hosts are several orders of magnitude longer than those of their parasites, it is tempting to conclude that selection acts more rapidly on the parasites. However, the way parasitic infections act within host populations makes it likely that the parasites force the pace of host evolution to keep step with, or even ahead of, their own evolution.” According to this scenario, our mosquito vector is locally adapted to its parasite and is ahead in the arm race such that manipulation is not expressed. In contrast, when the parasite is artificially associated with an allopatric or unnatural vector, its ability to manipulate the mosquito behavior could be expressed.
In particular, parasite manipulation may depend on infection intensity so that behavioral manipulation can be expressed only above a certain threshold. Accordingly, unnatural mosquito-Plasmodium interactions often exhibit higher infection intensity compared to natural combinations (Cohuet et al., 2006), and higher intensities were also observed in allopatric vs. sympatric infections with field isolates of P. falciparum (Harris et al., 2012). Future experiments, increasing infection levels using, for instance, blood with exceptional amount of gametocytes, are required to address this hypothesis.
Finally, it is possible that manipulation was not expressed merely because of our designs. For example, while our experiments were carried out from 19:00 to 23:00, mosquito activity spans from dusk to dawn with a peak around midnight. This activity peak is correlated to human resting behavior and is expected to minimize defensive behaviors-related death (Lehane, 2005). Future experiments investigating host-seeking behaviors of infected and uninfected mosquitoes around this peak are required to test whether malaria parasites have evolved the ability to fine-tune their manipulation to the temporal behavior of both their mosquito vectors and vertebrate hosts.
In the long-range behavioral assay, about 88% of the activated mosquitoes were retrieved from the human collecting box regardless of their infection status. We cannot exclude the possibility that this general high level of attraction to human odor reduced the chance to detect an effect of infection. Our previous studies using the same methodology but different mosquitoes (either different species or F0 and F1 wild mosquitoes) report mosquito attraction varying from ca. 50% (Lefèvre et al., 2009c, 2010) to ca. 80% (Lefèvre et al., 2009c). Further studies using mosquito populations with varying natural level of attraction to human odors are needed to assess possible parasite manipulation of mosquito behavior.
In the short-range behavioral assays, worn socks did not induce a significant increase in mosquito activation and activity as observed in previous studies using a different device (e.g., Smallegange et al., 2013 with free flying mosquitoes in cages). At least three hypotheses can be proposed to explain this result. Firstly, with our Locomotor Activity Monitor System, mosquitoes were kept in glass tubes and this may have either constrained their movement and willingness to activate, or stressed them in some way that would make them overly active regardless of the stimulus. Secondly, as the odor stimuli were emitted in a small enclosed environment (the glass tube), mosquito odorant receptors were perhaps quickly saturated hindering behavioral responses. Thirdly, previous recordings of mosquito behavior with this system were carried out for 24 h on several days (Lima-Camara et al., 2011; Rund et al., 2012). Thus, our relatively short-recording times might have limited the threshold of detection in case of small behavioral differences between clean and worn socks.
In addition, the distinction between infected and exposed-uninfected mosquitoes was not considered in the short-range behavioral assays. Although no behavioral differences were observed among infected and exposed-uninfected mosquitoes in the long-range assay and most (~80% see above) of the mosquitoes were infected in the short-range experiment II, we cannot completely exclude the possibility that this lack of distinction obscured differences especially if the manipulation signal was subtle.
In conclusion, this study using a natural mosquito-malaria parasite association suggests that manipulation of vector behavior may not be a general phenomenon. We hypothesize that the observed contrasting phenotypes with model systems might result from coevolution of the human parasite and its natural vector. Overall, our results highlight the importance of following up discoveries in laboratory model systems with studies on natural parasite–mosquito interactions to accurately predict the epidemiological, ecological and evolutionary consequences of parasite manipulation of vector behaviors.
Conflict of Interest Statement
The authors declare that the research was conducted in the absence of any commercial or financial relationships that could be construed as a potential conflict of interest.
Acknowledgments
This study was funded by the ANR (11-PDOC-006-01). We would like to thank all children and their parents for participating in this study, the local authorities for their support, as well as all the volunteers for the dual-port olfactometer assays. We are very grateful to the IRSS staff in Burkina Faso for technical assistance. We thank Fréderic Simard and Karine Mouline for letting us use their locomotor activity monitor system. We thank the Laboratoire Mixte International LAMIVECT, Bobo Dioulasso, Burkina Faso. We are grateful to A. Wong and J. E. Crawford for their valuable comments.
Supplementary Material
The Supplementary Material for this article can be found online at: http://journal.frontiersin.org/article/10.3389/fevo.2015.00086
References
Aguilar, R., Dong, Y., Warr, E., and Dimopoulos, G. (2005). Anopheles infection responses; laboratory models versus field malaria transmission systems. Acta Trop. 3, 285–291. doi: 10.1016/j.actatropica.2005.06.005
Alout, H., Djégbé, I., Chandre, F., Djogbenou, L. S., Dabiré, R. K., Corbel, V., et al. (2014). Insecticide exposure impacts vector-parasite interactions in insecticide-resistant malaria vectors. Proc. R. Soc. Lond. 281, 1786. doi: 10.1098/rspb.2014.0389
Alout, H., Ndam, N. T., Sandeu, M. M., Djogbe, I., Chandre, F., Dabiré, R. K., et al. (2013). Insecticide resistance alleles affect vector competence of Anopheles gambiae s.s. for Plasmodium falciparum field isolates. PLoS ONE 8:e63849. doi: 10.1371/journal.pone.0063849
Anderson, R. A., Knols, B. G. J., and Koella, J. C. (2000). Plasmodium falciparum sporozoites increase feeding-associated mortality of their mosquito hosts Anopheles gambiae s.l. Parasitol 120, 329–333. doi: 10.1017/S0031182099005570
Anderson, R. A., Koella, J. C., and Hurd, H. (1999). The effect of Plasmodium yoelii nigeriensis infection on the feeding persistence of Anopheles stephensi Liston throughout the sporogonic cycle. Proc. R. Soc. Lond. 266, 1729–1733. doi: 10.1098/rspb.1999.0839
Añez, N., and East, J. S. (1984). Studies on Trypanosoma rangeli Tejera, 1920 II. Its effect on feeding behaviour of triatomine bugs. Acta Trop. 41, 93–95.
Baeshen, R., Ekechukwu, N. E., Toure, M., Paton, D., Coulibaly, M., Traore, S. F., et al. (2014). Differential effects of inbreeding and selection on male reproductive phenotype associated with the colonization and laboratory maintenance of Anopheles gambiae. Malar. J. 13, 14. doi: 10.1186/1475-2875-13-19
Boëte, C. (2005). Malaria parasites in mosquitoes: laboratory models, evolutionary temptation and the real world. Trends Parasitol. 21, 445–447. doi: 10.1016/j.pt.2005.08.012
Cator, L. J., George, J., Blanford, S., Murdock, C. C., Baker, T. C., Read, A. F., et al. (2013). ‘Manipulation’ without the parasite: altered feeding behaviour of mosquitoes is not dependent on infection with malaria parasites. Proc. R. Soc. Lond. 280:0711. doi: 10.1098/rspb.2013.0711
Cator, L. J., Lynch, P. A., Read, A. F., and Thomas, M. B. (2012). Do malaria parasites manipulate mosquitoes? Trends Parasitol. 28, 466–470. doi: 10.1016/j.pt.2012.08.004
Cohuet, A., Osta, M. A., Morlais, I., Awono-Ambene, P. H., Michel, K., Simard, F., et al. (2006). Anopheles and Plasmodium: from laboratory models to natural systems in the field. Embo. Rep. 7, 1285–1289. doi: 10.1038/sj.embor.7400831
Daoust, S. P., King, K. C., Brodeur, J., Roitberg, B., Roche, B., and Thomas, F. (2015). Making the best of a bad situation: host partial resistance and by-pass to behavioural manipulation by parasites? Trends Parasitol. doi: 10.1016/j.pt.2015.05.007. [Epub ahead of print].
Dong, Y., Aguilar, R., Xi, Z., Warr, E., Mongin, E., and Dimopoulos, G. (2006). Anopheles gambiae immune responses to human and rodent Plasmodium parasite species. PLoS Pathog. 2:e52. doi: 10.1371/journal.ppat.0020052
Fanello, C., Santolamazza, F., and della Torre, A. (2002). Simultaneous identification of species and molecular forms of the Anopheles gambiae complex by PCR-RFLP. Med. Vet. Entomol. 16, 461–464. doi: 10.1046/j.1365-2915.2002.00393.x
Gouagna, L. C., Bonnet, S., Gounoue, R., Verhave, J. P., Eling, W., Sauerwein, R., et al. (2004). Stage-specific effects of host plasma factors on the early sporogony of autologous Plasmodium falciparum isolates within Anopheles gambiae. Trop. Med. Int. Health 9, 937–948. doi: 10.1111/j.1365-3156.2004.01300.x
Harris, C., Morlais, I., Churcher, T. S., Awono-Ambene, P., Gouagna, L. C., Dabire, R. K., et al. (2012). Plasmodium falciparum produce lower infection intensities in local versus foreign Anopheles gambiae populations. PLoS ONE 7:849. doi: 10.1371/journal.pone.0030849
Hughes, D., Andersen, S., Hywel-Jones, N., Himaman, W., Billen, J., and Boomsma, J. (2011). Behavioral mechanisms and morphological symptoms of zombie ants dying from fungal infection. BMC Ecol. 11:13. doi: 10.1186/1472-6785-11-13
Hughes, D. P., Brodeur, J., and Thomas, F. (2012). Host Manipulation by Parasites. Oxford: University Press.
Hurd, H. (2003). Manipulation of medically important insect vectors by their parasites. Annu. Rev. Entomol. 48, 141–161. doi: 10.1146/annurev.ento.48.091801.112722
Jenni, L., Molyneux, D. H., Livesey, J. L., and Galun, R. (1980). Feeding behaviour of tsetse flies infected with salivarian trypanosomes. Nature 283, 383–385. doi: 10.1038/283383a0
Jones, M. D. R., and Gubbins, S. J. (1978). Changes in the circadian flight activity of the mosquito Anopheles gambiae in relation to insemination, feeding and oviposition. Physiol. Entomol. 3, 213–220. doi: 10.1111/j.1365-3032.1978.tb00151.x
Joy, D. A., Gonzalez-Ceron, L., Carlton, J. M., Gueye, A., Fay, M., McCutchan, T. F., et al. (2008). Local adaptation and vector-mediated population structure in Plasmodium vivax malaria. Mol. Biol. Evol. 25, 1245–1252. doi: 10.1093/molbev/msn073
Koella, J. C., and Packer, M. J. (1996). Malaria parasites enhance blood-feeding of their naturally infected vector Anopheles punctulatus. Parasitol 113, 105–109. doi: 10.1017/S0031182000066348
Koella, J. C., Rieu, L., and Paul, R. E. L. (2002). Stage-specific manipulation of a mosquito's host-seeking behavior by the malaria parasite Plasmodium gallinaceum. Behav. Ecol. 13, 816–820. doi: 10.1093/beheco/13.6.816
Koella, J. C., Sorensen, F. L., and Anderson, R. A. (1998). The malaria parasite, Plasmodium falciparum, increases the frequency of multiple feeding of its mosquito vector, Anopheles gambiae. Proc. R. Soc. Lond. 265, 763–768. doi: 10.1098/rspb.1998.0358
Lefèvre, T., Adamo, S. A., Biron, D. G., Missé, D., Hughes, D., and Thomas, F. (2009a). “Chapter 3 Invasion of the Body Snatchers: The Diversity and Evolution of Manipulative Strategies in Host–Parasite Interactions,” Advances in Parasitology, Vol. 68, ed P. W. Joanne (Academic Press), 45–83.
Lefèvre, T., Gouagna, L. C., Dabire, R. K., Elguero, E., Fontenille, D., Costantini, C., et al. (2009c). Evolutionary lability of odour-mediated host preference by the malaria vector Anopheles gambiae. Trop. Med. Int. Health 14, 228–236. doi: 10.1111/j.1365-3156.2009.02206.x
Lefèvre, T., Gouagna, L.-C., Dabiré, K. R., Elguero, E., Fontenille, D., Renaud, F., et al. (2010). Beer Consumption Increases Human Attractiveness to Malaria Mosquitoes. PLoS ONE 5:e9546. doi: 10.1371/journal.pone.0009546
Lefèvre, T., Koella, J. C., Renaud, F., Hurd, H., Biron, D. G., and Thomas, F. (2006). New prospects for research on manipulation of insect vectors by pathogens. PLoS Pathog. 2:72. doi: 10.1371/journal.ppat.0020072
Lefèvre, T., Lebarbenchon, C., Gauthier-Clerc, M., Missé, D., Poulin, R., and Thomas, F. (2009b). The ecological significance of manipulative parasites. Trends Ecol. Evol. 24, 41–48. doi: 10.1016/j.tree.2008.08.007
Lefèvre, T., and Thomas, F. (2008). Behind the scene, something else is pulling the strings: emphasizing parasitic manipulation in vector-borne diseases. Infect. Genet. Evol. 8, 504–519. doi: 10.1016/j.meegid.2007.05.008
Lefèvre, T., Thomas, F., Schwartz, A., Levashina, E., Blandin, S., Brizard, J. P., et al. (2007). Malaria Plasmodium agent induces alteration in the head proteome of their Anopheles mosquito host. Proteomics 7, 1908–1915. doi: 10.1002/pmic.200601021
Lefèvre, T., Vantaux, A., Dabiré, R. K., Mouline, K., and Cohuet, A. (2013). Non-genetic determinants of mosquito competence for malaria parasites. PLoS Pathog. 9:e1003365. doi: 10.1371/journal.ppat.1003365
Lehane, M. J. (2005). The Biology of Blood-Sucking in Insects, 2nd Edn. New York, NY: Cambridge University Press.
Lima-Camara, T. N., Bruno, R. V., Luz, P. M., Castro, M. G., Lourenco-de-Oliveira, R., Sorgine, M. H. F., et al. (2011). Dengue infection increases the locomotor activity of Aedes aegypti females. PLoS ONE 6:e17690. doi: 10.1371/journal.pone.0017690
May, R. M., and Anderson, R. M. (1979). Population biology of infectious diseases: Part, I. I. Nature 280, 455–461 doi: 10.1038/280455a0
Molyneux, D. H., and Jefferies, D. (1986). Feeding behaviour of pathogen-infected vectors. Parasitol. 92, 721–736. doi: 10.1017/S0031182000065574
Moore, J. (1993). Parasites and the behavior of biting flies. J. Parasitol. 79, 1–16. doi: 10.2307/3283270
Morassin, B., Fabre, R., Berry, A., and Magnaval, J. F. (2002). One year's experience with the polymerase chain reaction as a routine method for the diagnosis of imported malaria. Am. J. Trop. Med. Hyg. 66, 503–508.
Mukabana, W., Takken, W., Coe, R., and Knols, B. (2002). Host-specific cues cause differential attractiveness of Kenyan men to the African malaria vector Anopheles gambiae. Malar. J. 1:17. doi: 10.1186/1475-2875-1-17
Mukabana, W., Takken, W., Killeen, G., and Knols, B. (2004). Allomonal effect of breath contributes to differential attractiveness of humans to the African malaria vector Anopheles gambiae. Malar. J. 3:1. doi: 10.1186/1475-2875-3-1
Munstermann, L. E. (1994). Unexpected genetic consequences of colonization and inbreeding: allozyme tracking in Culcidae (Diptera). Ann. Entomol. Soc. Am. 87, 157–164. doi: 10.1093/aesa/87.2.157
Norris, D. E., Shurtleff, A. C., Toure, Y. T., and Lanzaro, G. C. (2001). Microsatellite DNA polymorphism and heterozygosity among field and laboratory populations of Anopheles gambiae s.s. (Diptera : Culicidae). J. Med. Entomol. 38, 336–340. doi: 10.1603/0022-2585-38.2.336
Poulin, R. (2007). Evolutionary Ecology of Parasites, 2nd Edn. Princeton: Princeton University Press.
Poulin, R. (2010). Parasite manipulation of host behavior: an update and frequently asked questions. Adv. Stud. Behav. 41, 151–186. doi: 10.1016/S0065-3454(10)41005-0
Putnam, J. L., and Scott, T. W. (1995). Blood-feeding behavior of dengue-2 virus-infected Aedes aegypti. Am. J. Trop. Med. Hyg. 52, 225–227.
Qiu, Y. T., Smallegange, R. C., Van Loon, J. J. A., and Takken, W. (2011). Behavioural responses of Anopheles gambiae sensu stricto to components of human breath, sweat and urine depend on mixture composition and concentration. Med. Vet. Entomol. 25, 247–255. doi: 10.1111/j.1365-2915.2010.00924.x
Qiu, Y. T., Smallegange, R. C., Van Loon, J. J. A., Ter Braak, C. J. F., and Takken, W. (2006). Interindividual variation in the attractiveness of human odours to the malaria mosquito Anopheles gambiae s. s. Med. Vet. Entomol. 20, 280–287. doi: 10.1111/j.1365-2915.2006.00627.x
R Development Core Team. (2013). R: A Language and Environment for Statistical Computing. Vienna: R Foundation for Statistical Computing.
Rebeil, R., Jarrett, C. O., Driver, J. D., Ernst, R. K., Oyston, P. C. F., and Hinnebusch, B. J. (2013). Induction of the Yersinia pestis PhoP-PhoQ regulatory system in the flea and its role in producing a transmissible infection. J. Bacteriol. 195, 1920–1930. doi: 10.1128/JB.02000-12
Rogers, M. E. (2012). The role of Leishmania proteophosphoglycans in sand fly transmission and infection of the mammalian host. Front. Microbiol. 3:223. doi: 10.3389/fmicb.2012.00223
Rogers, M. E., and Bates, P. A. (2007). Leishmania manipulation of sand fly feeding behavior results in enhanced transmission. PLoS Pathog. 3:e91. doi: 10.1371/journal.ppat.0030091
Rossignol, P. A., Ribeiro, J. M. C., and Spielman, A. (1984). Increased intradermal probing time in sporozoite-infected mosquitoes. Am. J. Trop. Med. Hyg. 33, 17–20.
Rossignol, P. A., Ribeiro, J. M. C., and Spielman, A. (1986). Increased biting rate and reduced fertility in sporozoite-infected mosquitoes. Am. J. Trop. Med. Hyg. 35, 277–279.
Rund, S. S. C., Lee, S. J., Bush, B. R., and Duffield, G. E. (2012). Strain- and sex-specific differences in daily flight activity and the circadian clock of Anopheles gambiae mosquitoes. J. Insect. Physiol. 58, 1609–1619. doi: 10.1016/j.jinsphys.2012.09.016
Sangare, I., Michalakis, Y., Yameogo, B., Dabire, R. K., Morlais, I., and Cohuet, A. (2013). Studying fitness cost of Plasmodium falciparum infection in malaria vectors: validation of an appropriate negative control. Malar. J. 12:2. doi: 10.1186/1475-2875-12-2
Schwartz, A., and Koella, J. C. (2001). Trade-offs, conflicts of interest and manipulation in Plasmodium-mosquito interactions. Trends Parasitol. 17, 189–194. doi: 10.1016/S1471-4922(00)01945-0
Scott, T. W., and Takken, W. (2012). Feeding strategies of anthropophilic mosquitoes result in increased risk of pathogen transmission. Trends Parasitol. 28, 114–121. doi: 10.1016/j.pt.2012.01.001
Severo, M. S., and Levashina, E. A. (2014). Mosquito defenses against Plasmodium parasites. Curr. Opin. Insect. Sci. 3, 30–36. doi: 10.1016/j.cois.2014.07.007
Shandilya, H., Gakhar, S. K., and Adak, T. (1999). Plasmodium infection-induced changes in salivary gland proteins of malaria vector Anopheles stephensi (Diptera:Culicidae). J. Infect. Dis. 52, 214–216.
Smallegange, R. C., van Gemert, G.-J., van de Vegte-Bolmer, M., Gezan, S., Takken, W., Sauerwein, R. W., et al. (2013). Malaria infected mosquitoes express enhanced attraction to human odor. PLoS ONE 8:e63602. doi: 10.1371/journal.pone.0063602
Thomas, F., Adamo, S., and Moore, J. (2005). Parasitic manipulation: where are we and where should we go? Behav. Process. 68, 185–199. doi: 10.1016/j.beproc.2004.06.010
Thomas, F., Schmidt-Rhaesa, A., Martin, G., Manu, C., Durand, P., and Renaud, F. (2002). Do hairworms (Nematomorpha) manipulate the water seeking behaviour of their terrestrial hosts? J. Evol. Biol. 15, 356–361. doi: 10.1046/j.1420-9101.2002.00410.x
Tripet, F., Aboagye-Antwi, F., and Hurd, H. (2008). Ecological immunology of mosquito-malaria interactions. Trend Parasitol. 24, 219–227. doi: 10.1016/j.pt.2008.02.008
Van Den Abbeele, J., Caljon, G., De Ridder, K., De Baetselier, P., and Coosemans, M. (2010). Trypanosoma brucei modifies the tsetse salivary composition, altering the fly feeding behavior that favors parasite transmission. PLoS Pathog. 6:e1000926. doi: 10.1371/journal.ppat.1000926
Vantaux, A., Dabiré, R., Cohuet, A., and Lefèvre, T. (2014). A heavy legacy: offspring of malaria-infected mosquitoes show reduced disease resistance. Malar. J. 13:442. doi: 10.1186/1475-2875-13-442
Keywords: Anopheles, Plasmodium, parasite manipulation, behavior, host-seeking, experimental infection
Citation: Vantaux A, de Sales Hien DF, Yameogo B, Dabiré KR, Thomas F, Cohuet A and Lefèvre T (2015) Host-seeking behaviors of mosquitoes experimentally infected with sympatric field isolates of the human malaria parasite Plasmodium falciparum: no evidence for host manipulation. Front. Ecol. Evol. 3:86. doi: 10.3389/fevo.2015.00086
Received: 19 April 2015; Accepted: 07 July 2015;
Published: 03 August 2015.
Edited by:
Sara Goodacre, University of Nottingham, UKReviewed by:
Alex Wong, Carleton University, CanadaJacob Evan Crawford, University of California, Berkeley, USA
Copyright © 2015 Vantaux, de Sales Hien, Yameogo, Dabiré, Thomas, Cohuet and Lefèvre. This is an open-access article distributed under the terms of the Creative Commons Attribution License (CC BY). The use, distribution or reproduction in other forums is permitted, provided the original author(s) or licensor are credited and that the original publication in this journal is cited, in accordance with accepted academic practice. No use, distribution or reproduction is permitted which does not comply with these terms.
*Correspondence: Amélie Vantaux, Malaria Molecular Epidemiology Unit, Institut Pasteur in Cambodia, 5 Boulevard Monivong, PO Box 983, Phnom Penh, Cambodia,YW1lbGllLnZhbnRhdXhAZ21haWwuY29t