- 1Department of Geography, Environmental Change Research Centre, University College London, London, UK
- 2Department of Biology, University of Regina, Regina, SK, Canada
- 3Lake Group, Department of Bioscience, Aarhus University, Silkeborg, Denmark
- 4Section for Ecoinformatics and Biodiversity, Department of Bioscience, Aarhus University, Aarhus, Denmark
- 5The National Trust, Bury St. Edmunds, UK
- 6School of Geography, University of Nottingham, Nottingham, UK
- 7School of Geography, University of Nottingham Malaysia Campus, Semenyih, Malaysia
- 8School of Geography, Archaeology and Palaeoecology, Queen's University Belfast, Belfast, UK
- 9Institute of Environmental Change and Society, University of Regina, Regina, SK, Canada
Eutrophication is the most pressing threat to highly calcareous (marl) lakes in Europe. Despite their unique chemistry and biology, comprehensive studies into their unimpacted conditions and eutrophication responses are underrepresented in conservation literature. A multi-indicator palaeolimnological study spanning ca. 1260–2009 was undertaken at Cunswick Tarn (UK), a small, presently eutrophic marl lake, in order to capture centennial timescales of impact. Specific aims were to (1) establish temporal patterns of change (gradual/abrupt) across biological groups, thereby testing theories of resistance of marl lake benthic communities to enrichment, and (2) compare the core record of reference condition with prevailing descriptions of high ecological status. Analyses of sediment calcium (Ca), phosphorus (P), pigments, diatoms, testate amoebae, cladocerans, and macrofossils, revealed three abrupt changes in ecosystem structure. The first (1900s), with biomass increases in charophytes and other benthic nutrient-poor indicators, supported ideas of resistance to eutrophication in Chara lakes. The second transition (1930s), from charophyte to angiosperm dominance, occurred alongside reductions in macrophyte cover, increases in eutrophic indicators, and a breakdown in marling, in support of ideas of threshold responses to enrichment. Core P increased consistently into the 1990s when rapid transitions into pelagic shallow lake ecology occurred and Cunswick Tarn became biologically unidentifiable as a marl lake. The moderate total P at which these changes occurred suggests high sensitivity of marl lakes to eutrophication. Further, the early record challenges ideas of correlation between ecological condition, charophyte biomass and sediment Ca. Instead, low benthic production, macrophyte cover, and Ca sedimentation, was inferred. Management measures must focus on reducing external nutrient and sediment loads at early stages of impact in order to preserve marl lakes.
Introduction
Globally, freshwater habitats are among the most anthropogenically impacted (Geist, 2011). Increasing population density along with technological advances in food production have increased hydrological modification, landscape homogenization, as well as nutrient loading and sediment losses from terrestrial to aquatic habitats especially over the twentieth century (Stoate et al., 2001; Geist, 2011). The effects on freshwater ecosystems include eutrophication (Hasler, 1947; Ulén et al., 2007), reductions in biodiversity (Baastrup-Spohr et al., 2013), and diminished recreational value (Pretty et al., 2003; Priskin, 2008). The widespread alteration of fresh waters especially in lowland areas where population densities are highest, has likely changed how freshwater habitats are perceived following increasingly few incidences of human encounters with truly natural habitats. Therefore, cultural perceptions of what constitutes natural, undisturbed conditions, may have become biased (i.e., shifting baseline syndrome) (Papworth et al., 2009). In turn, where records of pre-disturbance conditions is lacking, restoration can often be misguided when based on increasingly old and scattered anecdotal evidence (Tibby et al., 2008).
In terms of freshwater biology, it is desirable to characterize lake ecosystems prior to significant human impact, a prerequisite for drafting international legislation and guidelines on environmental protection and restoration (European Commission, 2000; Lake, 2001). Science and legislation, in turn, need to shape the decisions and actions of monitoring agencies, site managers, and owners of private land, in order to have a tangible positive impact on the environment. It is difficult to ascertain pre-impact conditions without historical information, yet in Europe especially, human impact extends beyond the beginning of the Industrial Revolution to Medieval, Roman, and prehistoric times, for which ecological literature is scarce. Consequently, knowledge of the natural state of the environment requires additional methods such as palaeolimnology (Brenner et al., 1993; Bennion et al., 2011). Palaeolimnological methods for tracking changes in ecological structure and function in response to multiple drivers such as eutrophication (Sayer et al., 2010; Davidson and Jeppesen, 2013; Bennion et al., 2015), climate (Last and Smol, 2001; Leng et al., 2006), hydrology, and chemistry (Smol et al., 2001) have developed substantially over the previous decades, allowing for increasingly confident ecosystem reconstructions.
In the UK, most of the highly calcareous (marl) lakes lie in heavily agricultural areas where human impact has a long history (Pentecost, 2009). Marl lakes in their natural state are highly alkaline, carbonate-rich lakes that precipitate calcite and contain distinct macrophyte communities dominated by Characeae and Potamogetonaceae (Pentecost, 2009; Wiik et al., 2013). Further, owing to their geochemistry, the water is naturally of a translucent blue-green color of high transparency (Spence, 1982). Owing to the immobilization of phosphorus (P) via coprecipitation with calcite, which occurs with the highest intensity during summer months, marl lakes have widely been held resistant to eutrophication (Wiik et al., 2013). However, the immobilization of P may be restricted temporally (e.g., dissociation in winter) and spatially (e.g., dissociation in hypolimnion/sediment) (Wiik et al., 2013), and P may be recycled in the sediment via uptake by macrophytes. Therefore, substantial changes in the benthos may occur despite low limnetic P. Indeed, even minor increases in limnetic nutrient availability have been linked to substantial changes in macrophyte communities (Krause and King, 1994; Free et al., 2007) and extirpations of key biodiversity species at a European scale (Stewart, 2001; Blaženèič et al., 2006). In lakes, moderately clear water combined with dense aquatic flora is frequently considered near-pristine conditions, yet may not reflect truly unimpacted ecology. This may be especially true for marl lakes should further empirical support for sensitivity of their benthic communities arise.
Cunswick Tarn in northern England, despite being listed as a marl lake (Pentecost, 2009), currently contains no marl lake indicator species, does not undergo visible marl precipitation in the summer months, and has brown and turbid rather than clear blue-green, water color. It would not, therefore, be biologically categorized as a marl lake (Wiik et al., 2014) and has been classed as a (naturally) eutrophic water body in legal documentation for Sites of Special Scientific Interest (SSSI) (SD 49/2). Sufficient historical records do not currently exist to characterize the unimpacted “reference status” ecology (European Commission, 2000) of the site. In order to elaborate on concepts of marl lake ecology and -reference status, a multi-indicator palaeolimnological approach was undertaken with specific aims to (1) establish the centennial-scale temporal patterns of change (gradual/abrupt) across biological groups, thereby testing theories of resistance of marl lake benthic communities to enrichment, (2) test whether ecological change was limited to one or two biological groups or whether it was apparent at an ecosystem level (physical–chemical function; multiple biological groups at all observed trophic levels; synchronicity across responses), and (3) compare the core record evidence of reference condition with prevailing descriptions of high ecological status for marl lakes.
Given the steep shelving of the margins of Cunswick Tarn, and the sensitivity of charophytes to light penetration through the water column (Middelboe and Markager, 1997), eutrophication effects were expected to be reflected by noticeable and relatively rapid changes in the charophyte community (as abundance of fossil charophyte oospores; Zhao et al., 2006). These changes were expected to be associated with upcore increases in remains of tall-growing or floating-leaved angiosperms (Blindow, 1992; Ayres et al., 2008; Sayer et al., 2010) as well as of groups of algae and cladocerans that reflect increased nutrient availability and functional change toward increasingly pelagic shallow lake ecology (Vadeboncoeur et al., 2003; Davidson and Jeppesen, 2013). Finally, assuming that marl lakes have distinct biological communities compared with other lake types, and are sensitive to eutrophication, both calcium (Ca) and P were expected to be significant correlates of biological community composition.
Methods
Study Site
Cunswick Tarn (SD489937) is a small (0.8 ha), fishless, relatively shallow (Zmax = 6.4 m; Zmean = 3.7 m) kettlehole lake in Cumbria, UK (Figure 1). The limestone catchment comprises improved pasture to the north and west, and woodland along a sheltering hillside to the east. A low-gradient outflow lies in the southwest corner (Figure 1). The main inflow lies at the southeast corner, in addition to which small, low-nutrient and carbonate-rich streams issue from springs in the east (Figure 1). Water high in P seeps from woodland and pastures to the north, and a drain pipe from the north pasture feeds directly into the lake. Further, grain for duck rearing has been deposited at the northern lake margins where soil erosion also occurs. The Tarn is currently eutrophic with mean annual total phosphorus (TP) of 56 μg L−1. Phytoplankton blooms occur in summer months and anoxia develops below 2.5–3 m. Macrophytes are abundant to approximately 4 m depth and dominated by two species (Nuphar lutea, Elodea canadensis) (Wiik et al., 2014).
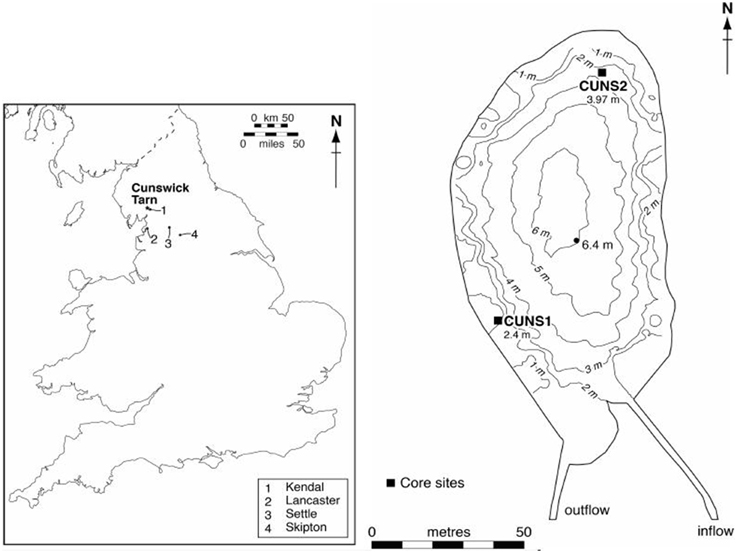
Figure 1. Cunswick Tarn location and bathymetry, showing the core collection sites and water depths.
Core Collection
Two littoral sediment cores were taken from Cunswick Tarn (Figure 1). CUNS1 (74 cm) was taken in January 2008 at a depth of 2.4 m from the southwestern margins using a “fat” Livingstone piston corer (internal diameter 71 mm). CUNS2 (94 cm) was taken in October 2009 from the northern margins at a water depth of 4 m using a “Big Ben” piston corer (internal diameter 140 mm) (Patmore et al., 2014). Most palaeolimnological analyses were undertaken on CUNS2, but analysis of macrofossils and loss-on-ignition (LOI) in CUNS1 confirmed a lakewide signal. The cores were extruded at 1 cm intervals, and sediment samples for pigment analysis (CUNS2) were placed in separate bags and frozen.
Core Chronology and Lithostratigraphy
Core CUNS2 was dated by a combination of 210Pb and 14C accelerator mass spectrometry (AMS) methods. Freeze-dried sediment samples were analyzed for 210Pb, 226Ra, 137Cs, and 241Am by direct gamma assay in the Bloomsbury Environmental Isotope Facility at University College London (UK) (Appleby et al., 1986). The chronology of CUNS2 followed the CRS model (Appleby, 2001), adjusted to a well-resolved 137Cs peak marking 1963. AMS on terrestrial macrofossils was undertaken at the Natural Environment Research Council Radiocarbon Facility (East Kilbride, UK) and the Keck C cycle AMS Lab (University of California, Irvine) (Table 1). For samples under 100 μg C, a correction based on small NIST Oxalic acid II was used to account for the effect of old carbon. Further, data were corrected for modern carbon contamination following Santos et al. (2007). The full chronology of CUNS2 was modeled with the Bayesian-based Bacon package (Blaauw and Christen, 2013) of R version 3.1.2 (R Development Core Team, 2010) using 17 210Pb, and 3 14C samples (Table 1).
Carbonate and organic carbon content of the cores were quantified by LOI following Dean (1974). P and Ca in CUNS2 were measured by X-ray fluorescence (XRF) (Boyle, 2002) at 1 cm intervals for the uppermost 10 cm, and 2 cm thereafter. In order to track hydrological changes in the Tarn, core CUNS2 carbonate δ18O and δ13C were measured at 5 cm intervals at the Natural Environment Research Council (NERC) Isotope Geosciences Laboratory (Keyworth, UK). Samples were disaggregated in 5% sodium hypochlorite solution (10% chlorox) for 24 h followed by washing and sieving at 85 μm. The residue was filtered, washed, dried (40°C) and ground in agate. The isolated material was reacted with anhydrous phosphoric acid in vacuo overnight at a constant 25°C to liberate CO2 for measurement. δ18O and δ13C are reported as per mil (‰) deviations of the isotopic ratios (18O/16O, δ13C/δ12C) calculated to the Vienna Pee Dee Belemnite scale using a within-run laboratory standard calibrated against National Bureau of Standards standards. The patterns in δ18O, and between δ18O and δ13C, were used to determine changes between open and closed conditions in the lake following Leng and Marshall (2004) and Leng et al. (2006), with consideration to disequilibrium values following Chara stem and oospore calcification (Andrews et al., 2004).
Biological Groups
Analysis of chlorophyll and carotenoid pigments followed Moorhouse et al. (2014) and was undertaken at all levels of CUNS2. Diatom analysis of CUNS2 followed Battarbee et al. (2001) and was undertaken at 2 cm intervals to a depth of 45 cm, beyond which preservation was poor. Where a strong preference for epiphytic or benthic substrates was absent, percentage abundance by habitat was calculated with an equal weighting for both habitats. Identification was carried out with reference to Krammer and Lange-Bertalot (1986, 1988, 1991, 2004). All identifications were to species level excepting Gomphonema pumilum and Gomphonema angustum (see Kelly et al., 2008) which were aggregated as Gomphonema angustum (agg.).
Testate amoebae were analyzed roughly at 2–3 cm intervals throughout the core. Subsamples of 2 cm3 were wet sieved through a 250 μm mesh to remove course organic flocs and a 35 μm mesh to remove clay and fine silt. The resulting residues were split into eight aliquots using a wet- splitter (Scott and Hermelin, 1993) and, in accordance with Patterson and Fishbein (1989), were quantified to >150 specimens per sample where abundance was permitting. Species and strains were identified according to lacustrine testate amoeba keys (Medioli and Scott, 1983; Kumar and Dalby, 1998) and published scanning electron micrographs (Roe et al., 2010; Patterson et al., 2012). Due to the significant amount of morphological variability in lacustrine testate amoebae (Medioli and Scott, 1983), a strain-based nomenclature was adopted in accordance with other lacustrine researchers (Escobar et al., 2008; Kihlman and Kauppila, 2012; Patterson et al., 2012), in order to include these “ecophenotypes.”
Subfossil cladocerans were analyzed at 3 cm intervals for CUNS2 following Korhola and Rautio (2000). Due to high sediment carbonate concentrations, samples were treated with 10% HCl prior to 10% KOH. All samples were sieved into 150 and 45 μm size fractions and counted following Davidson et al. (2007) to a minimum count of 300 individuals of the most abundant species, or the entire large size fraction where counts were low. Post-abdominal claws other than those of Daphnia pulex were aggregated to Ceriodaphnia and Daphnia spp. Morphological plasticity of Chydorus remains was interpreted as variability within Chydorus sphaericus sensu lato. Bosmina longirostris was the only species of Bosmina confirmed in the core, however the abundance of fragmented and non-diagnostic remains led to aggregation to Bosmina sp(p). Identification was undertaken using Frey (1965), Flößner (2000), and Szeroczyńska and Sarmaja-Korjonen (2007).
Macrofossil analysis followed Sayer et al. (2010) and was undertaken at every 6 cm of CUNS2, and for 16 levels of CUNS1. Macrofossils were identified using a dedicated reference collection of plant and animal parts and relevant publications held at UCL Geography. Where species-level detail was not attainable, remains were aggregated. These included Daphnia hyalina agg. ephippia (UK species other than D. magna and D. pulex), Potamogeton pusillus + berchtoldii leaf tips (P. pusillus and P. berchtoldii) and Nymphaeaceae trichosclereids, i.e., leaf cells (Nymphaea alba and N. lutea). Molluscs were mostly identified to family or genus level. Owing to morphological plasticity, charophyte oospores were aggregated as Chara spp. for numerical purposes. However, morphotypes were also tentatively identified using reference collection material and an oospore key (Haas, 1994). Uncalcified and calcified oospores were counted separately.
Historical presence of fish in Cunswick Tarn was determined by identification of chaoborid larval mandibles using the cladoceran method. Chaoborus obscuripes and Chaoborus crystallinus are non-migratory species associated with fishless lakes (Luoto and Nevalainen, 2009; Palm et al., 2012; Tolonen et al., 2012) and therefore their consistent presence in the core record was interpreted as a proxy for fishlessness. Mandibles were identified using Aitken (1954), Uutala (1990), Živić and Marković (2006), and Palm et al. (2011). Chaoborus obscuripes and Chaoborus crystallinus were not separated and are referred to as C. obsuripes/crystallinus.
Historical Evidence
Diagrammatic reconstructions of the macrophyte communities and colonization depths in Cunswick Tarn for the present, the mid-1900s, and the late 1800s/early 1900s were constructed based on (1) the CUNS1 and CUNS2 macrofossil records; (2) historical macrophyte presence and spatial distribution data collated from records held by UCL and Natural England, and other field naturalists; (3) macrophyte community composition and zonation in other marl lakes (Jupp et al., 1974; Spence et al., 1984; Pentecost, 2009; Hilt et al., 2010) or lakes with similar species composition (Spence, 1967, 1982).
Statistical Analysis
All data analysis was performed with R version 3.1.2 (R Development Core Team, 2010) using packages analogue (Simpson, 2007; Simpson and Oksanen, 2011), vegan (Oksanen et al., 2011), and mvpart (De'ath, 2002). Statistical analysis was only undertaken on CUNS2 data owing to the low resolution of the chronology for CUNS1.
Diatom and testate amoeba data were transformed into percentage abundances and taxa with <5% abundance and less than five occurrences in the core profile were omitted. They were also square-root transformed. Pigment and macrofossil data were (log10+1), and XRF data (logln)-transformed and normalized with respect to organic matter, respectively. Cladoceran data were transformed into percentage data for principal curves and multivariate regression trees (MRT), and square-root transformed for all analyses. Finally, pigment data were further standardized to abundances between 0 and 1 to reduce the statistical effects of “abundant” pigments (low degradation, high preservation).
Principal Curves
Principal curves (PC) are a multivariate ordination-based method particularly suitable for data sets with a single dominant gradient (Hastie and Stuetzle, 1989; De'ath, 1999) which applied to Cunswick Tarn. For CUNS2 data, PCA axis 1 scores were used for all data sets as a starting point for PCs. The complexity of the smoothing splines fitted to each species was allowed to vary, and the spline degrees of freedom were chosen using the GCV criterion following Simpson and Birks (2012).
Multivariate Regression Trees
Multivariate regression trees (MRT; De'ath, 2002) are a form of constrained cluster method that allow for multiple constraining variables on a response data set. The response data set can be a species abundance table or a dissimilarity matrix, clustered by age to add chronological constraint on temporal series (Simpson and Birks, 2012). MRT was applied to geochemical (P, Ca) and biological data (pigments, diatoms, testate amoebae, cladocerans, macrofossils) for all analyzed levels using age (in years AD) as a constraining parameter in order to determine the timing of major shifts in geochemistry and ecological responses (see Simpson and Birks, 2012; p. 261), and to test for synchronicity in change across indicators (ecosystem change). Analysing each response separately allowed for a high number of data points and therefore confidence in grouping. The number of data points was n = 94 (pigments), n = 54 (P, Ca), n = 39 (testate amoebae), n = 32 (cladocerans), n = 24 (diatoms), and n = 16 (macrofossils).
A previously developed cladoceran-based model capable of semi-quantitative inference of macrophyte and fish abundance, employing MRT (Davidson et al., 2010a) was also applied to the cladoceran data set (n = 32) in order to estimate past plant abundance [late summer plant volume infestation (PVI); Davidson et al., 2010b]. The number of Daphnia spp. ephippia (counted only at macrofossil resolution) for missing levels was modeled based on the relationship between ephippia (macrofossils) and post-abdominal claws (cladocerans).
Redundancy Analysis
Relationships between P, Ca, and biological data sets were tested using redundancy analysis (RDA). Owing to the varying resolution of the data, RDAs were fitted to matching observations, giving subsets of n = 54 (pigments), n = 18 (testate amoeba, cladocerans), and n = 15 (macrofossils). Analysis of the diatom data set was omitted due to the low number of matching observations. To allow for temporal ordering of the data, significance of variance explained was tested using ANOVA with cyclic permutations. The lowest attainable p-value of such permutations depends on the number of observations tested (1/n) and therefore significance was unattainable for most data sets at a 0.05 level (e.g., 1/18 = 0.056). Therefore, although too liberal, permutation tests were also performed without restrictions.
Results
Core Chronology and Lithostratigraphy
Core CUNS2 spanned a period of approximately 1260–2009 AD (date of core collection) with highest age uncertainties in the middle section of the core (46.5–75.5 cm; Figure 2). The gap in measured ages was unavoidable given the constraints of the time spans of 210Pb (to ca. 150 years from present) and radiocarbon dating (minimum age several hundreds of years). Sedimentation rates (SRs), core carbonate (Figure 3), and Ca (Supp. 1 in Supplementary Material) followed a similar pattern, the latter two of which were considered equivalent owing to deposition as calcite/marl (CaCO3) and their high correlation (Spearman's rho = 0.97, p <0.001).
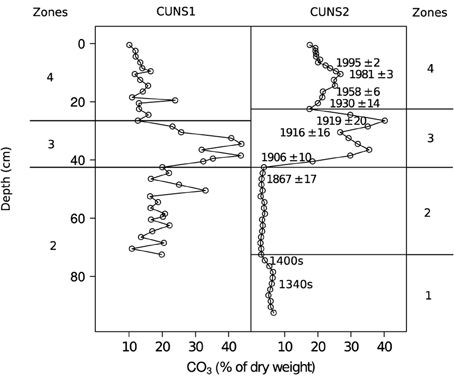
Figure 3. Carbonate concentrations in cores CUNS1 and CUNS2, shown with calendar years obtained by 210Pb-dating of CUNS2 with errors (±), and approximate calendar years based on radiocarbon dates (see Table 1 for details).
Four carbonate zones were apparent in CUNS2 (Figure 3) and were used as Zones for all Diagrams in Supplementary Materials, and as sections for presentation of biological data results. Zone 1 encompassed the base of the core (92.5 cm; 1250s) to the early 1400s, characterized by relatively low carbonate content (<10%), low variability, and low SR (ca. 0.12 cm year−1). Carbonate and SR were at their lowest values in Zone 2 (ca. 5% and 0.07 cm year−1, respectively), which encompassed the 1400s (72.5 cm) to the 1890s (42.5 cm). Zone 3 (42.5–22.5 cm) encompassed substantial increases in carbonate and SR (to ca. 1 cm year−1), with two carbonate peaks of 36% and 40% (1920s) followed by a rapid decline (ca. 10 years). Slightly lower carbonate (ca. 20–30%) and SRs (ca. 0.2–0.5 cm year−1) occurred in Zone 4 from the 1930s (22.5 cm) to 2009 (core top).
CUNS1 followed a similar carbonate pattern to CUNS2, allowing cross-correlation to estimate the chronology of CUNS1 (Figure 3). A CUNS1 parallel for CUNS2 Zone 1 was not appropriate owing to the brevity of the CUNS1 sequence and differences in carbonate concentration in this core section. The zone limits for CUNS1, therefore, are Zone 2: core base to 42.5 cm (base to ca. 1890s), Zone 3: 42.5–26.5 cm (1890s–1930s), and Zone 4: 26.5 cm to top (1930s–2008) (Figure 3). Higher carbonate concentrations than in CUNS2 were found in Zone 2 (ca. 20% vs. ca. 5%), and similar carbonate peaks (>40%) occurred in Zone 3.
CUNS2 carbonate δ18O could not be measured for most of Zone 2 owing to insufficient carbonate (data not shown). Therefore, only 14 samples were analyzed, five of which were in Zones 1 and 2 and had highly variable δ18O out of equilibrium with rainfall (−3 to −6‰), indicating a hydrologically closed system (Leng and Marshall, 2004; Leng et al., 2006). The uppermost nine samples ranged between −6 and −7‰ and were in equilibrium with rainfall, indicating that Cunswick Tarn became a hydrologically open system between the 1890s and 1900.
CUNS2 P concentrations were expressed relative to core organic matter to correct for dilution by calcite, and are therefore only interpretable as relative values within the core sequence (Supp. 1 in Supplementary Material). Ranged between 0 and 1 for simplicity, the lowest concentrations occurred before the 1530s (0.5–0.6) and between the 1530s and the 1890s (0.4–0.45). Rapid increases occurred in the early 1900s coincident with increases in Ca, with concentrations between 0.55 and 0.8. From the 1930s to 2009, concentrations increased consistently from 0.7 to 1 (Supp. 1 in Supplementary Material).
Zone 1: ca. 1250–1400 (CUNS2 Only; No Diatom Data)
Pigments of algae, higher plants, and cyanobacteria were low in Zone 1 (Supp. 2 in Supplementary Material). Compared with Zone 2, slightly higher concentrations of diatom (diatoxanthin), cyanobacteria (zeaxanthin), and cryptophyte (alloxanthin) pigments occurred throughout. The notable difference between the zones was the concentration of a purple sulfur bacterial pigment (okenone) which was highest at the base of the core and declined to levels below detection at the upper limit of Zone 1 (Supp. 2 in Supplementary Material).
The testate amoeba community (Supp. 3 in Supplementary Material) in this zone included moderate abundances of Difflugia oblonga (Ehrenberg, 1832) strain “oblonga” (ca. 15–30%), often associated with organic rich substrates (Kihlman and Kauppila, 2012), and Centropyxis aculeata (Ehrenberg, 1832) strain “aculeata” (ca. 10–25%). Lesser proportions consisted of Arcella vulgaris (Ehrenberg, 1830) (ca. <10%), Centropyxis constricta (Ehrenberg, 1843) “aerophila” and the oligotrophic indicator Cyclopyxis kahli (Deflandre, 1929) (Schönborn, 1967; Wall et al., 2010). The eutrophic indicator species Cucurbitella tricuspis (Carter, 1856) (Medioli and Scott, 1983) was also present in small amounts (<5%).
Remains of pelagic cladocerans at the base of the core were relatively abundant, and declined slightly toward the upper end of the zone (e.g., Bosmina longirostris/spp. 42% to <10%) (Supp. 4 in Supplementary Material). Other filter-feeding and/or pelagic species included D. pulex, Daphnia hyalina agg. and Simocephalus spp. Plant- and mud-associated species included Alonella exigua, Alonella nana, Eurycercus lamellatus, Graptoleberis testudinaria, Alona affinis, and Leydigia leydigi. Species exclusive to Zone 1 included Pleuroxus truncatus, Chydorus piger, and Alona rustica.
Plant macrofossils included terrestrial Juncus spp. seeds, fragments of Nymphaea alba seeds, and uncalcified oospores of Chara hispida agg., Chara contraria agg., and Nitella cf. flexilis (Supp. 5 in Supplementary Material). Oblong Plumatella sp. statoblasts were particularly abundant in Zone 1 compared to the rest of the core (Supp. 6 in Supplementary Material). Mollusca were largely absent with the exception of Sphaeriidae.
Zone 2: ca. 1400–1890
Okenone remained below detection limits throughout Zone 2 and overall pigment concentrations were lower compared to other zones (Supp. 2 in Supplementary Material). Benthic species (e.g., Pseudostaurosira brevistriata ca. 50–60%) dominated the CUNS2 diatom record at the latter end of the Zone (three samples counted), forming 60–80% of the community (Supp. 7 in Supplementary Material). Periphytic taxa formed 10–30% of the community and included Gomphonema angustum and Amphora pediculus, while planktonic species accounted for <5% (Figure 4). Cyclotella and Fragilaria compositions indicated pre-eutrophication conditions of high light penetration (Moss, 1979; Padisak and Reynolds, 1998; Meriläinen et al., 2000).
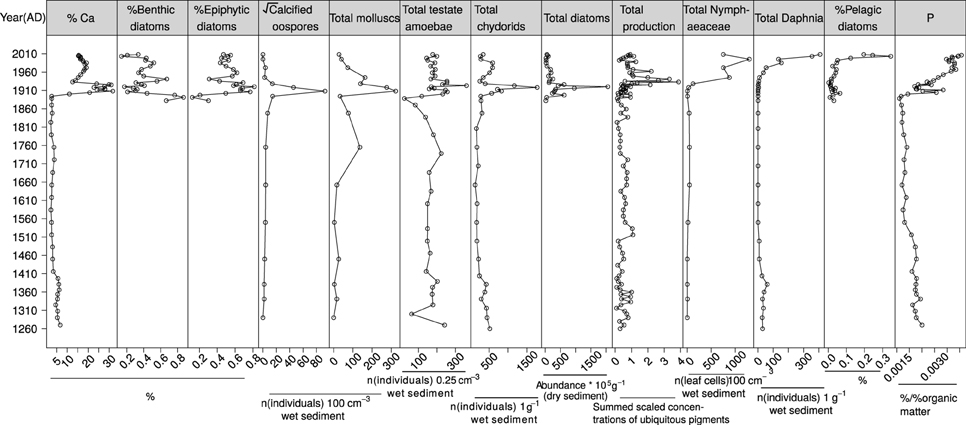
Figure 4. Summary plot of CUNS2 Ca, P, and key absolute and relative abundance changes of biological groups against time.
Changes among the dominant testate amoeba taxa (Supp. 3 in Supplementary Material) included gradual increases in Cucurbitella tricuspis (to ca. 23%), before rapidly declining in the mid nineteenth century, and decreases in Difflugia oblonga “oblonga.” Centropyxis constricta “aerophila” and Arcella vulgaris increased in abundance, while Cyclopyxis kahli remained in lesser proportions.
Diversity and total abundance of cladocerans were at their lowest in Zone 2, and pelagic taxa were rare (Supp. 4 in Supplementary Material, Figure 4). The community was dominated by benthic taxa (e.g., Tremel et al., 2000; Thienpont et al., 2015) including Chydorus sphaericus, Alona guttata/rectangula, and Alona quadrangularis. In contrast, in the macrofossil record of CUNS1 (Supp. 8 in Supplementary Material), planktonic Ceriodaphnia spp. ephippia were abundant (n >1000 × 100 ml−1) at the beginning of Zone 2 (ca. 1400–1500s), followed by consistent decreases to the upper end of the zone (n < 100 × 100 ml−1).
In CUNS2 Zone 2, there were fewer plant macrofossils of both terrestrial and aquatic origin compared with Zone 1 (Supp. 5 in Supplementary Material). Whilst species composition was similar, Charophyta differed as Nitella spp. were absent and C. hispida agg. dominated. In CUNS1, there were high abundances of calcified Chara aspera oospores in Zone 2, which was not recorded in CUNS2 (Supp. 9 in Supplementary Material, Figure 4). One other submerged macrophyte species, Potamogeton praelongus (CUNS1), occurred in this Zone. Floating-leaved macrophyte remains included Nymphaeaceae trichosclereids (leaf cells), occurring in CUNS1 and CUNS2 at slightly higher abundance than in Zone 1, and increasing substantially toward the end of Zone 2 in CUNS1 (Supps 5, 9 in Supplementary Material). Only N. alba seed remains were recorded, although N. lutea occurred in the Tarn in the early 1800s (Baker, 1885). Potamogeton natans seeds occurred throughout this Zone (CUNS1). Mollusc remains were more diverse and abundant in Zone 2 compared with Zone 1 (Supp. 6 in Supplementary Material, Figure 4). In CUNS2, the Sphaeriidae dominated the record (maximum of n = 50–100 × 100 ml−1) whereas in CUNS1 several different taxa became more abundant toward the latter part of the Zone (Bithynia leachi/tentaculata, Planorbis cf. albus, Valvata cf. macrostoma) (Supp. 8 in Supplementary Material).
Zone 3: ca. 1890–1930
The CUNS2 Zone 3 pigment assemblage remained largely similar to Zone 2 with low biomarker concentrations of cyanobacteria (zeaxanthin, canthaxanthin) and cryptophytes (alloxanthin), and purple sulfur bacteria (okenone) below the limit of detection (Supp. 2 in Supplementary Material). Consistent increases toward the top of the Zone occurred in chlorophyte (lutein, pheophytin b) and diatom (diatoxanthin) pigments. Increases in biomarkers of all algae (chl a, ß-carotene) occurred abruptly at the end of the sequence around the 1920s. In the CUNS1 and CUNS2 macrofossil record, Gloeotrichia cf. pisum, a nitrogen-fixing colonial cyanobacterium, increased approximately three-fold in abundance throughout the zone (Supps. 4, 9 in Supplementary Material). The proportion of periphytic diatoms in the CUNS2 record increased from ca. 40 to 70%, dominated by G. angustum and A. pediculus. (Supp. 7 in Supplementary Material). Throughout Zone 3, diatoms were more abundant than in the other zones (Figure 4). Persistence of the nutrient-sensitive Cymbella microcephala suggested relatively low nutrient concentrations (Schneider et al., 2000) (Supp. 7 in Supplementary Material).
The most substantial and rapid changes in the testate amoeba community occurred in this zone with peaks (at ca. 50%) in Arcella vulgaris and Centropyxis aculeata “aculeata,” taxa often associated with low oxygen environments (Roe et al., 2010; Drljepan et al., 2014). The proportions of Difflugia oblonga “oblonga” and Cucurbitella tricuspis (to ca. <5%), Cyclopyxis kahli and C. constricta “aerophila” decreased further. While species diversity was at a minimum in this zone, maximum absolute concentrations increased almost 10-fold (Figure 4).
Cladoceran abundances were highest in Zone 3 (Figure 4). Benthic taxa remained dominant, however community composition changed toward a dominance of strictly plant-associated species: Pleuroxus laevis and A. harpae became more abundant than A. quadrangularis and Alonella excisa, and Alonella exigua became absent (Supp. 4 in Supplementary Material). Ephippia of pelagic cladoceran taxa were largely absent or rare in CUNS1 and CUNS2 (Supps. 4, 8 in Supplementary Material).
Two macrophyte phases within Zone 3 were identified in the cores (Supps. 5, 9 in Supplementary Material). Oospores attained maximum abundances in the first phase (CUNS1 n = 2698 ml−1 and CUNS2 n = 8707 × 100 ml−1; Supps. 5, 9 in Supplementary Material; Figure 4) which corresponded to high abundances of encrusted stem remains. Six different oospore morphotypes were identified to C. aspera, C. hispida agg., Chara cf. vulgaris and Chara cf. globularis. Historical records further identified Chara curta in the Tarn at the end of the 1890s (Stewart, 2001), and Chara aculeolata, Chara desmacantha [syn C. curta] (1890s), and Chara fragilis [syn. Chara globularis] (1902) (Wilson, 1938). Nymphaeaceae were less abundant compared with Zone 2. In the latter phase (from ca. 1910s) oospores remained numerous, but declined with respect to the earlier phase (CUNS1 < 400 ml−1, CUNS2 ca. 200–1600 ml−1). Remains of floating-leaved species (P. natans, Nymphaeaceae incl. N. lutea) increased and narrow-leaved Potamogeton species (Potamogeton pusillus/berchtoldii, Potamogeton obtusifolius) appeared in the record for the first time. Maximum abundances of molluscs occurred in the early part of Zone 3 (Supps. 6, 8 in Supplementary Material; Figure 4).
Zone 4: ca. 1930–2000s
Diatom, cyanobacteria, and cryptophyte pigment concentrations in CUNS2 increased substantially from the 1930s to maximum abundances in the 2000s (Supp. 2 in Supplementary Material), indicative of increasing eutrophication (Leavitt, 1993; McGowan et al., 2005). In contrast, biomarkers of all algae (chl a, ß-carotene) peaked around the 1930s followed by declines to concentrations similar to the early 1900s (Figure 4). In the diatom record, nutrient-sensitive species were succeeded by the more ubiquitous Amphora pediculus (Kwandrans et al., 1998; Garcia-Rodriguez et al., 2007; Stenger-Kovács et al., 2007) (Supp. 7 in Supplementary Material). Okenone reappeared in the pigment record and increased from the 1980s, coinciding with a decrease in G. cf. pisum (Supps. 2, 5, 9 in Supplementary Material) and an increase in the proportion of planktonic diatoms such as Aulacoseira spp. and Stephanodiscus hantzchii indicative of eutrophication (Reynolds et al., 2002; Negro and de Hoyos, 2005) from ca. 5% to ca. 30% (Supp. 7 in Supplementary Material; Figure 4).
Remains of pelagic cladocerans, Daphnia spp. in particular, increased consistently from the 1930s to the 2000s in the macrofossil (CUNS1, CUNS2) and cladoceran records (CUNS2) (Supps. 4, 8 in Supplementary Material). Bosmina spp., which had been absent from the record after the 1400s, were found in the most recent sediment. Five plant-associated cladoceran species characteristic of Zone 3 declined in abundance in contrast to increases in ubiquitous species (Alonella nana), filter feeders (Sida crystallina) and sediment-associated species (Leydigia leydigi) (Supp. 4 in Supplementary Material).
A substantial increase occurred in the relative abundance of Difflugia oblonga “oblonga” in the 1930s (Supp. 3 in Supplementary Material), corresponding with increases in core organic matter. In contrast, relative abundances of Arcella vulgaris and Centropyxis aculeata “aculeata” decreased. Some recovery in the uppermost part of the core was evident for the aforementioned species with modest increases also in Cucurbitella tricuspis (to ca. 9%) in the 1990s. Species diversity and concentrations (Figure 4) returned to levels similar to those in Zones 1 and 2.
In the 1930s, P. natans seeds disappeared from the core record, and charophyte remains declined substantially (Supps. 5, 9 in Supplementary Material). In CUNS1, oospore numbers fell two-three orders of magnitude from maximal abundances in Zones 2 and 3, while CUNS2 numbers fell to levels comparable with Zone 1. Nymphaeaceae trichosclereids increased to >3 times their previous abundance (CUNS1, CUNS2). Linear-leaved Potamogeton remains persisted in the core record (CUNS2). Of the Nymphaeaceae, only seeds of N. lutea were found. The Tarn was at the time (1936) described as “very rich in aquatic plants” (Wilson, 1938). The first core record of Myriophyllum spicatum (CUNS1) in the 1950s coincided with descriptions of Cunswick Tarn as an “excellent calcareous tarn with Cladium mariscus” (NC SSSI citation), however, concerns were raised regarding the spreading of Phragmites [australis] which dominated the east side (Lewis, 1954).
The abundance of mollusc remains decreased markedly following the 1940s and Bithynia spp. became the dominant taxon until the 1980s after which numbers declined to Zone 2 levels (Supps 6, 8 in Supplementary Material).
Constrained Analyses
Ca and P were correlated with between 10 and 37% of the CUNS2 biological data (Table 2). Relationships were strongest for P, and for pigments and cladocerans for which the cyclic permutation test was either significant (pigments) or took the smallest p-value possible given the number of samples (cladocerans). Owing to the possibility of Type II errors with relatively low numbers of data points, the significance of all relationships (pigments, testate amoebae, cladocerans, macrofossils) under unconstrained permutation tests suggests that P and Ca were strongly associated with biological change. Macrofossils were the only biological group explained more by Ca (26%) than P (20%) (Table 2), however, it is likely that bimodality, which could not be removed, negatively influenced the strength of the test with Ca.
Principal Curves and Multivariate Regression Trees
Chronological MRT splits are indicated as the midpoint between samples either side of a split and therefore are not, for data with differing resolutions, an exact reflection of the temporal relationship across groups. While P and Ca data occurred at identical depths, the splits for pigments (median age gap 6 years) will necessarily be more precise than those for macrofossils (median age gap 40 years). In order to remain unambiguous about split locations given the exact dates put into the model, dating errors were not incorporated into split estimates.
Four significant MRT zones were identified for P (Figure 5). For Ca, three or four significant zones were identified by MRT depending on the data transformation used (bimodality in the density distribution of Ca-values interfered with the method). In order to cohere with P and biological data groupings, and to delineate the decline in Ca content in the uppermost section of the core (Figure 3), four zones were selected. Relatively small changes occurred in P and Ca concentrations between 1200s and the late 1800s within which one split was found at ca. 1400 (Ca) (Zone 1/2) and 1530 (P). Changes were more synchronous in the latter end of the sequence where splits were identified at 1896 (Ca), 1902 (P), 1930 (Ca), and 1935 (P). The first split marks an increase in the concentration of both elements, and the second a decrease in Ca and continued increase in P.
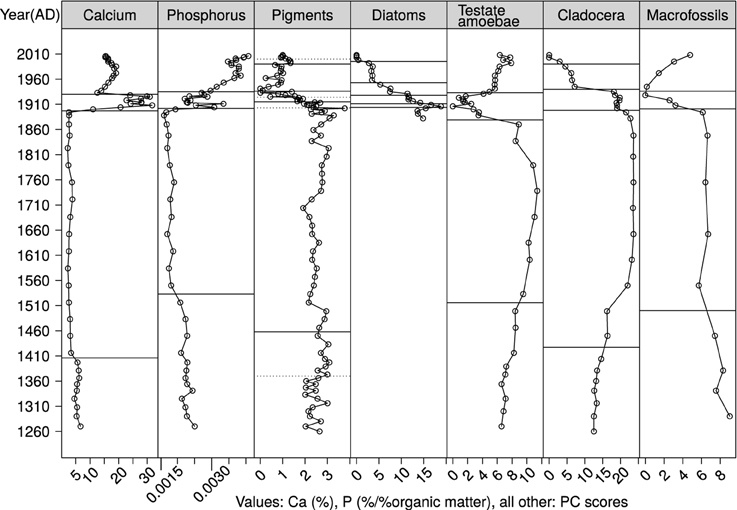
Figure 5. Ca and P, and PCs of biological groups against time. Horizontal lines indicate MRT-derived splits, those dashed to aid inspection indicate least importance.
Principal curves identified modest changes in biological communities between the 1200s and the late 1800s. MRTs located shifts of cladocerans and pigments around 1427, and 1370 and 1458, respectively, followed by macrofossils around 1500, and testate amoebae around 1516 (Figure 5).
Substantial community change occurred between the 1890s and the 2000s during which splits in biological groups showed clear synchronicity. Split 2 as per P (1902) and Ca (1896) (Zone 2/3), where both elements increased, coincided with splits in pigments (1903), diatoms (1900), cladocerans (1898) and macrofossils (1901). Split 3, where Ca declined (1930) (Zone 3/4) and P continued to increase (1935) coincided with splits in pigments (1936), diatoms (1933), testate amoebae (1933), and macrofossils (1936), with a slight lag in cladocerans (1940).
The highest number of significant splits occurred in primary producers (pigments n = 8, diatoms n = 5), which also changed significantly in the most recent years (pigments: 2000, diatoms: 1995), coincident with the most recent split in cladocerans (1990) (Figure 5). The highest frequency of splits occurred between the 1900s and 1930s. The most important pigment splits are indicated by solid lines (Figure 5).
Fish Abundance and the Cladocera-Macrophyte-Fish Model
Absence of fish throughout the core record was indicated by the presence of C. obscuripes/crystallinus, and the absence of fish scales and fish leech cocoons (Piscicola geometra) (Odgaard and Rasmussen, 2001). Zooplanktivorous fish densities indicated by the cladoceran-based MRT model (Davidson et al., 2010a) were low (<0.001 ZF density m−2) for most data points with the highest density (ca. 0.5) indicated for the lowermost sample in the cladoceran record with high numbers of Bosmina spp (Figure 6). These values are low compared with the range of zooplanktivorous fish used to construct the model (0–3.26) (Davidson et al., 2010a) and support the idea that fish were absent from the lake particularly through Zones 2–4. Primary producer and cladoceran community changes were therefore assumed to be independent of top-down effects from fish.
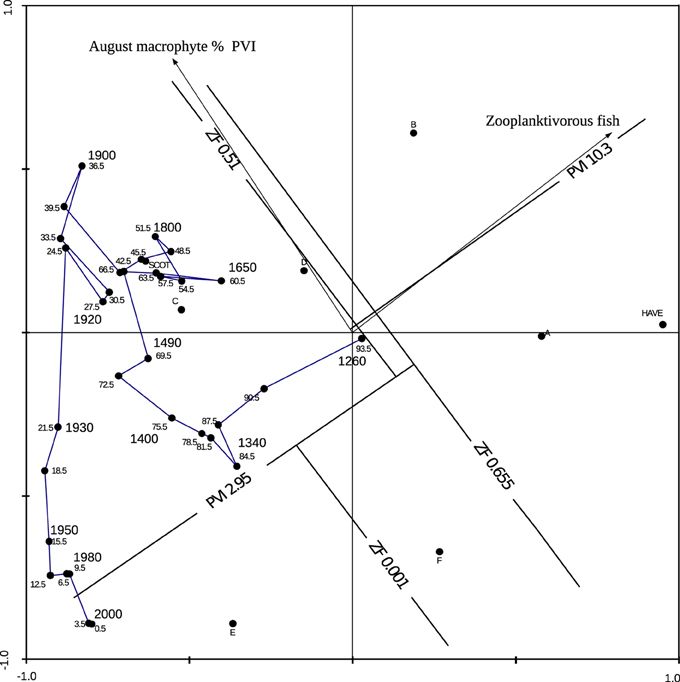
Figure 6. Cladoceran-inferred August plant volume infestation and zooplanktivorous fish abundance of CUNS2 sample depths (cm).
Reconstructed August PVI was low (<10%) prior to the 1400s (Zone 1) and following the 1950s (Zone 4). Very low PVI (<3%) was indicated for the uppermost two levels (>2000 AD). The MRT model is semi-quantitative and strictly speaking it is only the changing of the MRT end group that strongly supports the idea of changes in macrophyte abundance (Davidson et al., 2010b). However, the direction of movement in the two-dimensional space of the biplot can also be used to infer past dynamics in macrophyte abundance. This suggests an increase in macrophyte abundance after 1890, which peaked between 1900 and 1915, followed by a consistent decline of plant abundance to the present day where plant abundance is rather low (Figure 6).
Discussion
Cunswick Tarn proved particularly suited for detailing bottom-up effects of eutrophication owing to the absence of fish and their top-down influence throughout the core record. With reference to the original research questions, the high correlation of both core P (15–37%) and Ca (13–27%) with all biological data sets (Table 2) supported expectations of eutrophication (P) and marling (Ca) as correlates of biological community structure at the whole ecosystem level (Figure 5). The two main changes were (1) an abrupt shift between two different benthic phases in the early 1900s (low Chara + organic sediment to high Chara + marl sediment), and (2) the erosion of benthic primary production pathways as the Tarn became increasingly eutrophic in the mid-late 1900s. While the latter followed initial hypotheses and is widely documented (Bumby, 1977; Carbiener et al., 1990; Vadeboncoeur et al., 2003), the former is a previously undocumented eutrophication-driven switch in marl deposition with important consequences to lake management targets and marl lake reference conditions.
Drivers of Ecosystem Change
Hydrology
The first substantial changes in Ca, P, and ecosystem structure occurred following lake drainage in the 1890s, corresponding to a period of intensifying land management in the area following land enclosures and attempts at drainage of local lakes (Otley, 1830; Walker, 1955; Cousins, 2013). Both lake shallowing and eutrophication can have similar ecological effects and their synchrony in the core record render strict eutrophication responses difficult to identify. For example, increases in calcified Chara oospore and stem abundance (Supps. 5, 9 in Supplementary Material), as well as of epiphytic diatoms and macrophyte-associated molluscs (Figure 4) may reflect improved light conditions. Higher light availability expands benthic photosynthetic habitat (Leavitt, 1993), and also modulates the optimal depth of calcite deposition (Pukacz et al., 2014), which may confound simplistic responses to nutrients.
Shallowing is supported in the oospore record of CUNS2 by a shift from a dominance of the Chara hispida group to the more shore-line associated, lower-growing Chara aspera. However, evidence for a predominant effect of eutrophication following an initial pulse of lake shallowing is supported by a few factors. Firstly, the Ca and oospore increase also occurred in CUNS1, the location of which was 1.5 m shallower than that of CUNS2 (Figure 1). Secondly, CUNS1 reflected littoral conditions (high carbonate content and Chara aspera oospores) throughout the record and therefore did not undergo charophyte community shifts, suggesting that increased nutrient availability was in part responsible for the change. Thirdly, the change in Ca and charophytes was a continuous rather than a stepwise transition, suggesting underlying eutrophication responses independent of a simple water level change. Indeed, the positive relationship between Ca and P in the early 1900s strongly suggest a eutrophication effect on Chara biomass and marl deposition.
Internal Drivers: Marl Lake Ecology and Ca-P Cycling
The reasons behind notions of self-sustaining, eutrophication-buffering properties of charophytes include the maintenance of clear water via nutrient uptake from the water column, coprecipitation of Ca with P, and sediment stabilization owing to dense growth (Otsuki and Wetzel, 1972; Kufel and Kufel, 2002; Robertson et al., 2007). This so-called inertia to external pressure, and therefore internal driving mechanisms, underlie ideas of occasional abrupt and rapid ecological changes following extended periods of eutrophication (Scheffer et al., 1993; Scheffer and van Nes, 2007). While punctuated change has been largely absent in other palaeolimnological studies of (formerly) Chara-dominated lakes (Davidson et al., 2005; Ayres et al., 2008; Sayer et al., 2010), elements of rapid change driven by internal mechanisms could be inferred in the Cunswick Tarn record. These events corresponded to key changes in community composition within a marl lake framework, namely changes in Chara abundance, transitions from Chara to angiosperm dominance, and finally transitions from benthic to pelagic ecosystem structure (Figure 7).
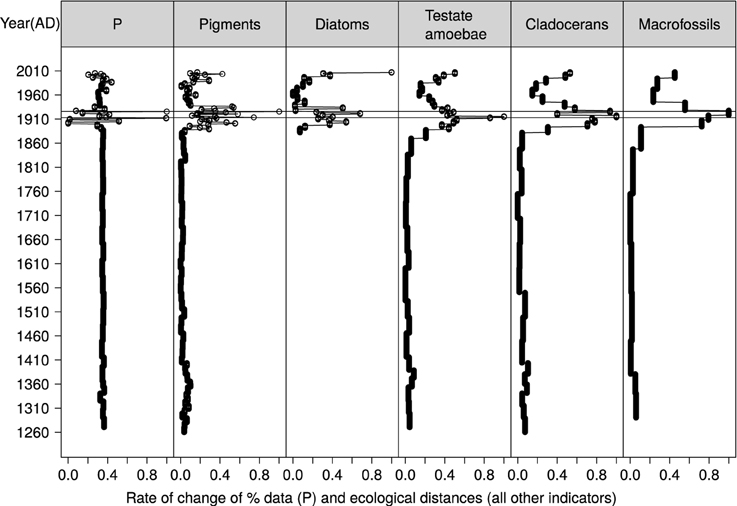
Figure 7. Rate of change in P and ecological distances between consecutive samples of biological groups, ranged between 0 and 1.
The first major ecological shift in the Cunswick record occurred in the 1890s with sub-decadal changes across all biological groups. The positive relationship between Ca, P, and Chara biomass in Cunswick Tarn, mirrored by increased cladoceran-inferred PVI (Figure 6), support ideas of self-stabilizing mechanisms especially in the early stages of eutrophication via increased nutrient uptake in the benthos and prevention of phytoplankton increases. Importantly, the structuring role of charophytes was also apparent in the close association of charophyte biomass with abundance responses in other benthic primary producers and consumers (Figure 4). Also importantly, these numerical changes were synchronous with strictly qualitative ecosystem change as seen in the multivariate analyses of cladocerans, diatoms, and testate amoebae (Figure 5), all of which were transformed to remove effects of total abundance. Indirect effects of high macrophyte biomass, such as local anoxia at the sediment level (Lindholm et al., 2008), may have contributed to the increase in Arcella vulgaris and Centropyxis aculeata “aculeata” during this time (Roe et al., 2010; Drljepan et al., 2014).
The importance of plant biomass responses as buffers to eutrophication were further supported by the lack of association between P and Ca in CUNS2, which argues against significant chemical defenses against eutrophication via coprecipitation (see also Hobbs et al., 2005; Hutorowicz and Dziedzic, 2008). Further, the second abrupt change in the core record in the 1930s (Figure 7) corresponded to the breaking down of marling, substantial declines in macrophyte biomass (Figure 6), particularly that of charophytes (Figure 4), and increases in eutrophic indicator taxa, suggesting that high charophyte densities were the main factor in maintaining clear water and ecology reflective of nutrient-poor conditions.
The third marked point of change in the pigments, diatoms, testate amoebae, and cladocera occurred in the 1990s (Figures 5, 7). Increases in eutrophic indicators such as Cucurbitella tricuspis (Medioli and Scott, 1983) (Supp. 3 in Supplementary Material), and planktonic diatoms (Supp. 7 in Supplementary Material), cryptophytes (Supp. 2 in Supplementary Material) and Daphnia spp. (Figure 4) (e.g., Bos and Cumming, 2003; Davidson et al., 2007) together with Nymphaeaceae, combined with associated declines in concentrations of ubiquitous pigments, all support the idea of a larger change in ecosystem structure toward pelagic shallow lake ecology (McGowan et al., 2005). Reciprocally, major declines in cladoceran-inferred PVI (Figure 6) and reductions in macrophyte species richness from 9 to 4 between the 1980s and the early 2000s (Wiik et al., 2014) support the idea of a substantial decline in benthic production.
Given the modest concentrations of TP in the lake currently (56 μg L−1), and increasing P concentrations toward the core top, the decoupling between marling and P loading following the 1930s likely occurred at very low TP concentrations and opened up a “slippery slope” toward the loss of marl lake characteristics in Cunswick Tarn. The buffering capacity of charophytes may function over lower ranges of impact than previously thought. Indeed, sensitivity of charophytes to eutrophication is supported by studies of other marl lakes through time (Krause and King, 1994; Krolikowska, 1997; Hargeby et al., 2007), and also via space-for-time substitution (Kornijów et al., 2002; Free et al., 2007).
Interestingly, there are several marl lakes which have sustained high abundances of precipitating Chara meadows, also to considerable water depth (ca. 4 m), despite high nutrient loading and limnetic TP concentrations. These include several lakes in Poland with TP between 40 and 220 μg L−1 (Pełechaty et al., 2004, 2013). There are also charophyte-dominated clear marl lakes with intermediate and variable TP, including Polish Lakes Jasne (TP 30–50) and Słone (TP 10–74) (Peczuła et al., 2014; Pukacz et al., 2014). More detailed studies would be required to establish whether the variability is random, or driven by some other factor such as water hardness, sediment structure, or other spectral characteristics of the water. For example, a TP-independent negative relationship has been found between charophyte abundance and dissolved organic carbon (DOC) (Kłosowski et al., 2006). The relationship does not, however, preclude the existence of marl lakes (such as Cunswick Tarn) in peaty, wetland catchments (Pentecost, 2000; Jones et al., 2011; Peczuła et al., 2014).
Nitrogen
Proxies for biological nitrogen availability were not measured in the sediment record and therefore the ecological impact of changing major nutrient stoichiometry could not be evaluated. However, atmospheric nitrogen deposition, especially in the northwest of England, has been high over the twentieth century, particularly since the 1950s (Pitcairn and Fowler, 1995), which in part reflects increases in nitrogen-rich fertilizer application (Fowler et al., 2005). In Cunswick Tarn, increases in fossil nitrogen-fixing Gloeotrichia colonies occurred in both cores around the 1900s and peaked in the 1920s–1930s (Supps. 5, 9 in Supplementary Material), suggestive of modest eutrophication (Laugaste and Lessok, 2004) under conditions of nitrogen limitation (Vis et al., 2008; Hudon et al., 2009).
Concentrations of Gloeotrichia colonies soon after declined to pre-1900 levels while Cocconeis placentula, a diatom with potential affinity to nitrogen-rich habitats (Werner, 1977; Lebkuecher et al., 2014), increased in abundance. Further, total nitrogen has been shown to display significant controls over testate amoebae populations (Patterson et al., 2012; Ju et al., 2014) in addition to P (Roe et al., 2010), and increasing N availability could be contributing to the 1920s shift in community composition toward a difflugid-dominated assemblage. The transition in algal and testate amoeba indicators corresponded with shifts toward angiosperm dominance and reduced macrophyte biomass in the lake, which may indicate a combined effect of nitrogen and phosphorus in shaping the biological eutrophication responses of Cunswick Tarn.
Climate
The Medieval Climate Anomaly (MCA) was a relatively warm period in the UK, and occurred ca. AD 880–1350 (Guiot et al., 2010; Dong et al., 2012). The MCA was coincident with Zone 1 in the CUNS2 core record (ca. 1260–1400), during which planktonic-dominated foodwebs in Cunswick Tarn were indicated by low macrophyte PVI (Figure 6), an abundance of pelagic cladocerans, and relatively high concentrations of cyanobacterial pigments (Supps. 2, 4 in Supplementary Material; Figure 4). The presence of considerable concentrations of purple sulfur bacteria pigments further indicated hypolimnetic anoxia at this time. However, high total production was unlikely given low core P and Ca concentrations, the presence of cladoceran oligotrophic indicators Alonella exigua (Whiteside, 1970; De Eyto et al., 2003) and Chydorus piger (Whiteside, 1970; Bos and Cumming, 2003), the oligotrophic indicator testate amoeba Cyclopyxis kahli (Schönborn, 1967; Wall et al., 2010), and the low concentration of Nymphaeaceae trichosclereids.
Warmer climate can promote primary production, thereby effecting carbonate precipitation (Mullins, 1998; Mirosław-Grabowska and Niska, 2007), and favoring high relative abundances of plankton (Jeppesen et al., 2012) as well as high thermal stability, strong stratification, and hypolomnetic anoxia (Viner, 1985; Foley et al., 2012). While the aforementioned were inferred in the “MCA” core record, it is likely that climate was a subsidiary rather than dominant driver of the observed changes. Firstly, interannual temperature variability was considerable through the MCA and the Little Ice Age in the UK (Cage and Austin, 2010; Guiot et al., 2010), implying that a more noisy signal should have been detected in the CUNS2 record if the biology was driven solely by temperature patterns. Secondly, numerical analyses of the biological data sets against variably smoothed April–September temperature reconstructions (Guiot et al., 2010) combined with Central England temperature time series (HadCET) revealed no detectable impact of temperature (data not shown). It remains possible that (1) climate effects were non-linear and (2) climatic conditions interacted with more discrete change within the catchment over the period between zones 1 and 2.
Land Clearance and DOC
Cunswick Tarn lies in a shallow depression in the landscape and would naturally have been surrounded by alder (Alnus glutinosa) carr habitat with shading willows in the margin. Under such conditions it is strongly possible that the lake margins would have been heavily shaded, resulting in reduced macrophyte development and periodic local oxygen depletion. Coppicing or marginal clearing for agriculture would have opened up the lake margins to light and increased benthic primary production and water column oxygenation, as suggested by the increase in inferred macrophyte PVI in Zone 2 (Figure 6) and the disappearance of okenone (Supp. 2 in in Supplementary Material). While human impact in the catchment was likely, given the long history of the Cunswick Hall estate (Nicholson, 1861), there are unfortunately no detailed records to support the hypothesis.
Some support for shading and DOC as drivers of ecological change can be found in the more recent history of the lake during which okenone has reappeared in the core record. The period coincides with growth of willows (Salix spp.) at the lake margins, bank erosion at the north end of the lake which has resulted in high DOC and suspended sediment loads, and strong chemical stratification with hypolimnetic anoxia in summer months.
Implications for Management
Most change in the geochemistry and biology of Cunswick Tarn occurred in the late 1800s when larger-scale land improvement occurred in the catchment, consistent with European agriculture and population trends (Battarbee et al., 2011). Indeed, based on these trends, palaeolimnology-based reference status for lakes under the European Union Water Framework Directive (WFD: European Commission, 2000) is typically derived from the pre-1850s (Bennion et al., 2011). Marl lakes fall under the WFD but are also a designated habitat under the EU Habitats Directive (European Commission, 1992), hence being required to achieve both Good ecological status and Favorable conservation status, respectively. Sites with such dual designation are additionally considered “protected areas” under WFD and are consequently given earlier deadlines for achieving their target objectives.
In order to comply with the targets of river basin management plans as part of the WFD, lake condition assessments, and extensive cross-validation of ecological metrics have been undertaken to ensure that effective measures are taken on correctly and consistently classified sites across Europe (Cardoso et al., 2007; Carvalho et al., 2009; Hering et al., 2013). However, concerns have been raised over the effectiveness of macrophyte and chlorophyll a metrics for assessing very shallow (Zmean < 3 m) and shallow (Zmean 3-15 m) marl lakes (Wiik et al., 2014), with Cunswick Tarn data in particular indicating shifting perceptions of good ecological status, and therefore assessment bias, for marl lakes. Further, this improved understanding of the pre-impact character of Cunswick Tarn has implications for how it is described, protected and restored under national nature conservation designations (SSSI); the Tarn is currently identified as a naturally eutrophic water body, which is in strong disagreement with the palaeolimnological data.
Implications for Marl Lake Conservation Assessment and Reference Status
The uncertainties regarding drivers of biological communities in the lowermost core record (Zones 1 and 2) hinder firm conclusions as to the reference status of Cunswick Tarn. Zone 1, where high DOC loads and/or warmer climate were potentially inferred, depicts the Tarn as an oligotrophic marl lake, with carbonate-precipitating Chara aspera and low densities of Nymphaeaceae (especially N. alba) in the shallow waters. Abundant organic matter deposition created oxygen gradients in the deeper water, where Nitella flexilis agg. grew in low densities. The relatively high volume of open water allowed the development of planktonic foodwebs, with Daphnia and chaoborids in the deeper regions and Bosmina longirostris in the sublittoral zone (Figure 8). Zone 2, in contrast, portrayed oxygenated sediments, higher macrophyte PVI, and foodwebs based on benthic production with planktonic taxa largely absent.
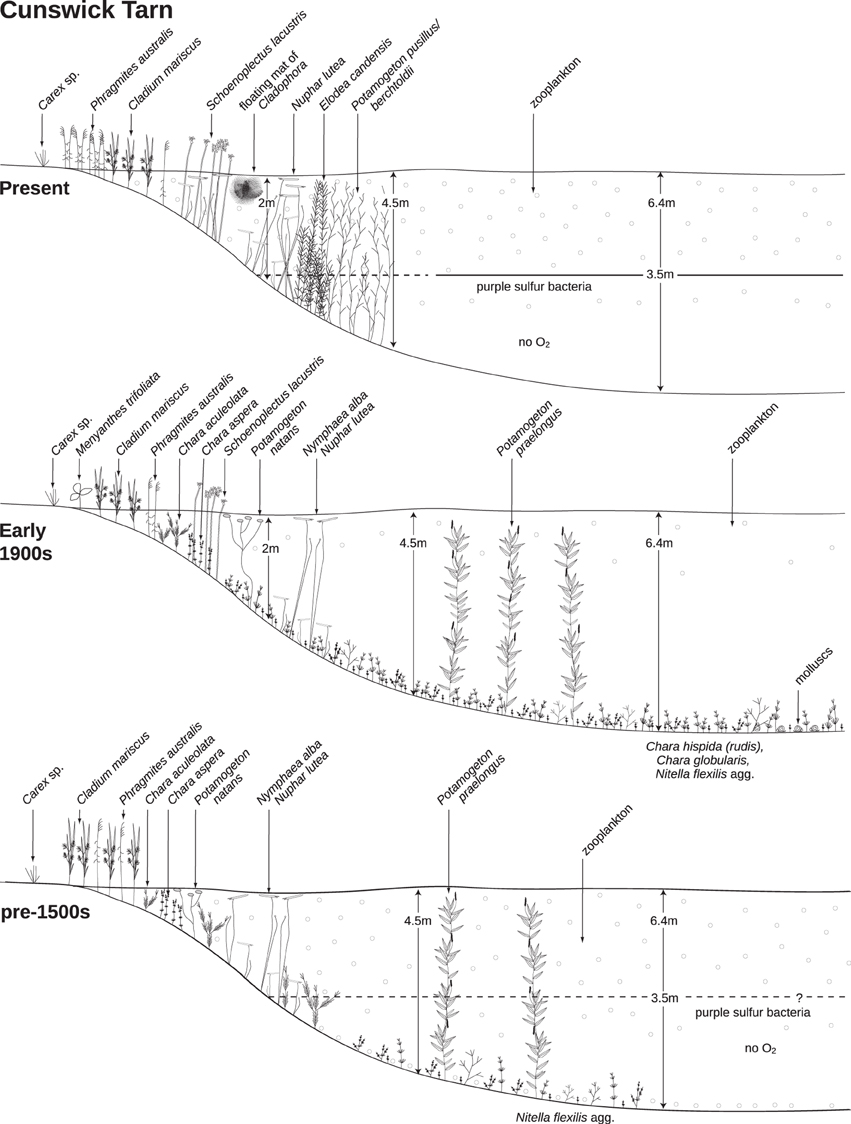
Figure 8. Cross sections of macrophyte cover, showing the currently dominant community, and historical communities based on available historical data.
Given the differences between zones 1 and 2, it is important to focus on their similarities to set guidelines for near-pristine status. For example, core carbonate content in both zones was low (<10%) in the deeper water, with low inferred abundances of charophytes and other submerged macrophytes. Higher but still moderate levels of charophytes and carbonate (<20%) occurred in the shallower margins (Figure 3; Supp. 9 in Supplementary Material) suggestive of charophyte-dominated marl benches (Jones et al., 2011). The relatively low levels of calcite deposition likely reflected the localized nature of marling in oligotrophic conditions, with calcite supersaturation occurring primarily in the epilimnion and the littoral compared with dissolution in the deeper water (Gonsiorczyk et al., 1997; Ekdahl et al., 2004). Near-pristine conditions in Cunswick Tarn were therefore remarkably “non-marly.”
In contrast to prevailing associations of dense charophyte meadows, and high production in the benthos, with high ecological quality, the Cunswick Tarn record has shown these characteristics to be an early response to eutrophication (Figure 8). The observation is not completely unexpected, considering that biomass responses to nutrient loading as a self-stabilizing method in Chara lakes inherently imply that these characteristics are a symptom of eutrophication. Indeed, particularly dense (and in some cases tall) growth of charophytes has been observed in lakes subject to considerable nutrient loading (Pełechaty et al., 2004; Haycock and Hinton, 2010) in comparison with the more scarce and modest growth of charophytes in lakes of lower impact (Fuller, 1741; Praeger, 1906; Spence, 1982), which was also indicated in the early record of Cunswick Tarn.
Management and Restoration Priorities
Cunswick Tarn is currently a marl lake only by geology, and is therefore considerably impacted. It is highly unlikely that conditions similar to the thirteenth to eighteenth centuries can be attained given the high load of P in the sediment, low flushing rates, and therefore high potential for internal loading in the lake. However, reductions of external nutrient loads are potentially the most effective means of restoring shallow lakes (Jeppesen et al., 2007), especially lakes such as Cunswick Tarn where fish manipulation is irrelevant. Unless nutrient availability for phytoplankton is restricted, charophytes will not be able to recolonize the Tarn. The most likely sources of nutrients to the Tarn are in the north where a drain pipe leads directly into the lake, and high nutrient concentrations have been measured in the inflow (December TP 44 μg L−1). Streams in the east woodland are likely to contribute less (December TP 14–20 μg L−1). Until 2015, the feeding of artificially high populations of ducks in the lake margins was a further major nutrient source. Given the potential for hydrology to be complex in limestone catchments, an inclusive and larger scale approach to determining nutrient sources may be important.
Given the lags in macrophyte re-establishment in deeper water owing to the high light requirements of germlings and species with low growth forms (Wang et al., 2014), restoration of lake margins is especially important in sites with considerable water depth gradients (Hilt et al., 2010). In this respect, the erosion occurring at the north end of Cunswick Tarn, exacerbated by the duck feeding, is particularly damaging and should be prevented. High external DOC and suspended sediment loads lead to anoxic sediments unfavorable for charophytes (Smith, 2003; Sederias and Colman, 2009) and maintain conditions suitable for reeds and Nymphaeaceae which are currently dominant.
Tree clearance would further open up light-flooded marginal habitat for charophyte re-establishment, however, this kind of management could also be seen as interference with natural succession of trees in the lake surrounds. It is therefore a judgment call of whether moderate interference promoting biodiversity (e.g., Sayer et al., 2012, see also Flöder and Sommer, 1999) is to be preferred over gradual natural successions following neglect (e.g. Biggs et al., 2005) (with the caveat that “natural” succession in this context does not account for grazers prior to major human influence). Considering the large-scale, centennial-scale human alteration of the natural landscape of the UK, including the eradication of top predators, and the high global conservation priority of preventing further biodiversity losses (European Parliament, 2012), coppicing around the lake would be strongly advisable.
Conclusion
Biological community shifts in Cunswick Tarn demonstrated synchronicity in response to gradual eutrophication pressure. Drainage of the lake and land improvement in the lake surrounds in the 1890s led to sub-decadal responses in multiple biological groups (microalgae, macrophytes, molluscs, testate amoebae, cladocerans) and substantial increases in carbonate precipitation. Further abrupt ecosystem shifts occurred in the 1920s, 1930s, and finally the 1990s when the lake changed into its current condition of low macrophyte diversity, high pelagic production, reduced macrophyte colonization depth and predominance of nutrient-tolerant micro- and macrophyte taxa. The patterns of rapid ecosystem-wide change, partly decoupled from the change in core P, supported ideas of abrupt responses to eutrophication as well as the importance of internal dynamics such as the self-sustaining capacity of charophyte beds, in shaping ecological lake structure.
Variance in marl lake biological communities correlated with both Ca and P as expected for marl lake communities responding to external nutrient load. Somewhat surprisingly, the period of least impact in the Tarn was characterized by very low carbonate precipitation in the deeper water, with marling restricted to the shallower littoral zone where charophyte meadows occurred. It is likely that pristine marl lakes do not attain sufficient levels of photosynthesis for intensive, whole-lake scale authigenic carbonate precipitation, and that macrophyte cover could be quite low.
The detection of two periods of accelerated change over intermediate core P concentrations support hypotheses of high eutrophication sensitivity of benthic communities in marl lakes, especially considering the modest concentration of TP (ca. 56 μg L−1) in Cunswick Tarn currently. Importantly, the association of high benthic biomass and calcite deposition with high ecological condition may be biased, reflecting a shift in perception due to the scarcity of truly high quality examples of marl lakes. The complete loss of marl lake biology in Cunswick Tarn argues strongly for restoration interventions at early stages of eutrophication in order to preserve characteristic marl lake communities. Further, if similar lake type transformations have occurred in other (formerly) marl lakes, perceptions of their geographical distribution and scarcity may be misguided.
Author Contributions
The majority of this publication is the direct result of a PhD by EW, which was supervised and guided by HB, CS, SC, and TD. EW undertook all palaeolimnological analyses, taught variously by HB, CS, SMG, and TD, excepting testate amoebae which are part of a PhD by SP, and diatoms which were part of an undergraduate dissertation by LS. All fieldwork was undertaken by EW and a combination of HB, CS, TD, and LS among others. GS offered crucial guidance during statistical analysis of the data during EW's PhD, and also contributed to the design and execution of statistical methods particular to this publication. EW wrote the manuscript, and all other authors contributed essentially to the interpretation and wording of components falling within their expertise.
Conflict of Interest Statement
The authors declare that the research was conducted in the absence of any commercial or financial relationships that could be construed as a potential conflict of interest.
Acknowledgments
This study was funded by a NERC and Natural England Ph.D. studentship (NE/F013086/1) to EW. Funding of sediment isotope analyses was awarded to EW by the NERC Isotope Geosciences Facilities (IP-1220-1110) (stable isotopes) and the NERC Radiocarbon Facility (NRCF010001; 1685.0313) (radiocarbon dating) with the help of Dr. Xiaomei Xu at the Keck C cycle AMS Lab, University of California, Irvine. We would also like to thank Dr. Charlotte Bryant at the NERC labs for her commitment, help, and investment in the radiocarbon project. Further, many thanks go to the gamekeeper Andy Dixon for providing access to Cunswick Tarn, to field experts Ian Patmore and Jorge Salgado for crucial help with coring and equipment maintenance, and macrophyte identification, respectively. Thanks also to John Birks and staff at various Natural England offices for finding and sharing old survey data of the site, and to John Boyle for his expert advice on interpreting XRF data. Thanks also to Teresa Needham and Graham Morris (University of Nottingham) for assistance with pigment analysis, and Handong Yang (UCL) for 210Pb dating. Miles Irving and Cath D'Alton at the UCL Drawing Office constructed macrophyte colonization and site location diagrams. Finally, thanks to Magdalena Toporowska for introductions to Polish marl lake literature, and the reviewers for their constructive and positive suggestions for manuscript improvement.
Supplementary Material
The Supplementary Material for this article can be found online at: http://journal.frontiersin.org/article/10.3389/fevo.2015.00082
References
Aitken, T. H. G. (1954). The culicidae of sardinia and corsica (diptera). Bull. Entomol. Res. 45, 437–494. doi: 10.1017/S0007485300029564
Andrews, J. E., Coletta, P., Pentecost, A., Riding, R., Dennis, S., Dennis, P. F., et al. (2004). Equilibrium and disequilibrium stable isotope effects in modern charophyte calcites: implications for palaeoenvironmental studies. Palaeogeogr. Palaeoclimatol. Palaeoecol. 204, 101–114. doi: 10.1016/S0031-0182(03)00725-9
Appleby, P. G. (2001). “Chronostratigraphic techniques in recent sediments,” in Tracking Environmental Change Using Lake Sediments, eds J. Smol, H. Birks, and W. Last (Dordrecht: Kluwer Academic Publishers), 171–203.
Appleby, P. G., Nolan, P. J., Gifford, D. W., Godfrey, M. J., Oldfield, F., and Battarbee, R. W. (1986). 210Pb dating by low background gamma counting. Hydrobiologia 143, 21–27. doi: 10.1007/BF00026640
Ayres, K. R., Sayer, C. D., Skeate, E. R., and Perrow, M. R. (2008). Palaeolimnology as a tool to inform shallow lake management: an example from Upton Great Broad, Norfolk. Biodivers. Conserv. 17, 2153–2168. doi: 10.1007/s10531-007-9223-1
Baastrup-Spohr, L., Iversen, L. L., Dahl-Nielsen, J., and Sand-Jensen, K. (2013). Seventy years of changes in the abundance of Danish charophytes. Freshw. Biol. 58, 1682–1693. doi: 10.1111/fwb.12159
Battarbee, R., Jones, V., Flower, R. J., Cameron, N. G., Bennion, H., Carvalho, L., et al. (2001). “Diatoms,” in Tracking Environmental Change Using Lake Sediments, eds J. Smol, H. Birks, and W. Last (Dordrecht: Kluwer Academic Publishers), 155–202.
Battarbee, R. W., Morley, D., Bennion, H., Simpson, G. L., Hughes, M., and Bauere, V. (2011). A palaeolimnological meta-database for assessing the ecological status of lakes. J. Paleolimnol. 45, 405–414. doi: 10.1007/s10933-010-9417-5
Bennion, H., Battarbee, R. W., Sayer, C. D., Simpson, G. L., and Davidson, T. A. (2011). Defining reference conditions and restoration targets for lake ecosystems using palaeolimnology: a synthesis. J. Paleolimnol. 45, 533–544. doi: 10.1007/s10933-010-9419-3
Bennion, H., Davidson, T. A., Sayer, C. D., Simpson, G. L., Rose, N. L., and Sadler, J. P. (2015). Harnessing the potential of the multi-indicator palaeoecological approach: an assessment of the nature and causes of ecological change in a eutrophic shallow lake. Freshw. Biol. doi: 10.1111/fwb.12579.
Biggs, J., Williams, P., Whitfield, M., Nicolet, P., and Weatherby, A. (2005). 15 years of pond assessment in Britain: results and lessons learned from the work of pond conservation. Aquat. Conserv. 15, 693–714. doi: 10.1002/aqc.745
Blaauw, M., and Christen, J. (2013). Bacon Manual - V 2.2. Available online at: http://chrono.qub.ac.uk/
Blaženèič, J., Stevanović, B., Blaženčić, Z., and Stevanović, V. (2006). Red data list of charophytes in the Balkans. Biodivers. Conserv. 15, 3445–3457. doi: 10.1007/s10531-005-2008-5
Bos, D. G., and Cumming, B. F. (2003). Sedimentary Cladoceran remains and their relationship to nutrients and other limnological variables in 53 lakes from British Columbia, Canada. Can. J. Fish. Aquat. Sci. 60, 1177–1189. doi: 10.1139/f03-097
Boyle, J. F. (2002). “Inorganic geochemical methods in palaeolimnology,” in Tracking Environmental Change Using Lake Sediments, eds W. M. Last and J. P. Smol (Dordrecht: Springer), 83–141.
Blindow, I. (1992). Decline of charophytes during eutrophication: comparison with angiosperms. Freshw. Biol. 28, 9–14.
Brenner, M., Whitmore, T. J., Flannery, M. S., and Binford, M. W. (1993). Paleolimnological methods for defining target conditions in lake restoration: Florida case studies. Lake Reserv. Manag. 7, 209–217. doi: 10.1080/07438149309354272
Bumby, M. J. (1977). Changes in submerged macrophytes in Green Lake, Wisconsin, from 1921 to 1971. Trans. Wiscons Acad. Sci. Arts Lett. LXV, 120–151.
Cage, A. G., and Austin, W. E. N. (2010). Marine climate variability during the last millennium: the Loch Sunart record, Scotland, UK. Quat. Sci. Rev. 29, 1633–1647. doi: 10.1016/j.quascirev.2010.01.014
Carbiener, R., Trémolières, M., Mercier, J., and Ortscheit, A. (1990). Aquatic macrophyte communities as bioindicators of eutrophication in calcareous oligosaprobe stream waters (Upper Rhine plain, Alsace). Vegetatio 86, 71–88. doi: 10.1007/BF00045135
Cardoso, A. C., Solimini, A., Premazzi, G., Carvalho, L., Lyche Solheim, A., and Rekolainen, S. (2007). Phosphorus reference concentrations in European lakes. Hydrobiologia 584, 3–12. doi: 10.1007/s10750-007-0584-y
Carvalho, L., Solimini, A. G., Phillips, G., Pietiläinen, O. P., Moe, J., Cardoso, A. C., et al. (2009). Site-specific chlorophyll reference conditions for lakes in Northern and Western Europe. Hydrobiologia 633, 59–66. doi: 10.1007/s10750-009-9876-8
Cousins, T. (2013). “Draft parish/township histories: Skelsmergh,” in Victoria County History of Cumbria Project, 1–35.
Davidson, T. A., and Jeppesen, E. (2013). The role of palaeolimnology in assessing eutrophication and its impact on lakes. J. Paleolimnol. 49, 391–410. doi: 10.1007/s10933-012-9651-0
Davidson, T. A., Sayer, C. D., Bennion, H. E., David, C., Rose, N., and Wade, M. P. (2005). A 250 year comparison of historical, macrofossil and pollen records of aquatic plants in a shallow lake. Freshw. Biol. 50, 1671–1686. doi: 10.1111/j.1365-2427.2005.01414.x
Davidson, T. A., Sayer, C. D., Langdon, P. G., Burgess, A., and Jackson, M. (2010a). Inferring past zooplanktivorous fish and macrophyte density in a shallow lake: application of a new regression tree model. Freshw. Biol. 55, 584–599. doi: 10.1111/j.1365-2427.2009.02391.x
Davidson, T. A., Sayer, C. D., Perrow, M. R., Bramm, M., and Jeppesen, E. (2007). Are the controls of species composition similar for contemporary and sub-fossil cladoceran assemblages? A study of 39 shallow lakes of contrasting trophic status. J. Palaelimnol. 38, 117–134. doi: 10.1007/s10933-006-9066-x
Davidson, T. A., Sayer, C. D., Perrow, M. R., Bramm, M., and Jeppesen, E. (2010b). The simultaneous inference of zooplanktivorous fish and macrophyte density from sub-fossil cladoceran assemblages: a multivariate regression tree approach. Freshw. Biol. 55, 546–564. doi: 10.1111/j.1365-2427.2008.02124.x
De'ath, G. (1999). Principal curves: a new technique for indirect and direct gradient analysis. Ecology 80, 2237–2253.
De'ath, G. (2002). Multivariate regression trees: a new technique for modeling species–environment relationships. Ecology 83, 1105–1117. doi: 10.2307/3071917
Dean, W. E. (1974). Determination of carbonate and organic-matter in calcareous sediments and sedimentary-rocks by loss on ignition - comparison with other methods. J. Sediment. Petrol. 44, 242–248.
De Eyto, E., Irvine, K., Garcia-Criado, F., Gyllström, M., Jeppesen, E., Kornijow, R., et al. (2003). The distribution of chydorids (Branchiopoda, Anomopoda) in European shallow lakes and its application to ecological quality monitoring. Archiv. Hydrobiol. 156, 181–202. doi: 10.1127/0003-9136/2003/0156-0181
Dong, X., Bennion, H., Battarbee, R. W., and Sayer, C. D. (2012). A multiproxy palaeolimnological study of climate and nutrient impacts on Esthwaite Water, England over the past 1200 years. Holocene 22, 107–118. doi: 10.1177/0959683611409780
Drljepan, M., McCarthy, F. M., and Hubeny, J. B. (2014). Natural and cultural eutrophication of Sluice Pond, Massachusetts, USA, recorded by algal and protozoan microfossils. Holocene 24, 1731–1742. doi: 10.1177/0959683614551227
Ekdahl, E. J., Teranes, J., Guilderson, T., Turton, C. L., McAndrews, J. H., Wittkop, C., et al. (2004). Prehistorical record of cultural eutrophication from Crawford Lake, Canada. Geology 32, 745–748. doi: 10.1130/G20496.1
Escobar, J., Brenner, M., Whitmore, T. J., Kenney, W. F., and Curtis, J. H. (2008). Ecology of Testate amoebae (thecamoebians) in subtropical Florida lakes. J. Paleolimnol. 40, 715–731. doi: 10.1007/s10933-008-9195-5
European Commission. (2000). Directive 2000/60/EC of the European parliament and of the council of 23 October 2000 establishing a framework for community action in the field of water policy. Off. J. Eur. Communities L327, 1–72.
European Commission. (1992). Council Directive 92/43/EEC of 21 May 1992 on the conservation of natural habitats and of wild fauna and flora. Off. J. Eur. Communities L206, 7–72.
European Parliament. (2012). Our Life Insurance, Our Natural Capital: An EU Biodiversity Strategy to 2020. European Parliament resolution of 20 April 2012 (2011/2307(INI)).
Flöder, S., and Sommer, U. (1999). Diversity in planktonic communities: an experimental test of the intermediate disturbance hypothesis. Limnol. Oceanogr. 44, 1114–1119. doi: 10.4319/lo.1999.44.4.1114
Foley, B., Jones, I. D., Maberly, S. C., and Rippey, B. (2012). Long-term changes in oxygen depletion in a small temperate lake: effects of climate change and eutrophication. Freshw. Biol. 57, 278–289. doi: 10.1111/j.1365-2427.2011.02662.x
Fowler, D., O'Donoghue, M., Muller, J. B. A., Smith, R. I., Dragosits, U., Skiba, U., et al. (2005). A chronology of nitrogen deposition in the UK between 1900 and 2000. Water Air Soil Pollut. Focus 4, 9–23. doi: 10.1007/s11267-004-3009-1
Free, G., Little, R., Tierney, D., Donnelly, K., and Caroni, R. (2007). A Reference Based Typology and Ecological Assessment System for Irish Lakes: Preliminary Investigations. University College Dublin; National University of Ireland; Trinity College Dublin, Galway.
Frey, D. G. (1965). Differentiation of Alona costata Sars from two related species (Cladocera, Chydoridae). Crustaceana 8, 159–173. doi: 10.1163/156854065X00695
Fuller, J. (1741). Esq., A description of a large lake called Malholm Tarn, near Skipton in Craven, in the County of Yorkshire. Philos. Trans. R. Soc. Lond. (1683-1775) 41, 612–614.
Garcia-Rodriguez, F., Bate, G. C., Smailes, P., Adams, J. B., and Metzeltin, D. (2007). Multivariate analysis of the dominant and sub-dominant epipelic diatoms and water quality data from South African rivers. Water SA 33, 653–658.
Geist, J. (2011). Integrative freshwater ecology and biodiversity conservation. Ecol. Indic. 11, 1507–1516. doi: 10.1016/j.ecolind.2011.04.002
Gonsiorczyk, T., Casper, P., and Koschel, R. (1997). Variations of phosphorus release from sediments in stratified lakes. Water Air Soil Pollut. 99, 427–434.
Guiot, J., Corona, C., and ESCARSEL members (2010). European 1400 Year Spring-Summer Temperature Reconstructions. IGBP PAGES/World Data Center for Paleoclimatology - Data Contribution Series 2010–047.
Haas, J. N. (1994). First identification key for charophyte oospores from Central Europe. Eur. J. Phycol. 29, 227–235. doi: 10.1080/09670269400650681
Hargeby, A., Blindow, I., and Andersson, G. (2007). Long-term patterns of shifts between clear and turbid states in Lake Krankesjön and Lake Tåkern. Ecosystems 10, 28–35. doi: 10.1007/s10021-006-9008-5
Hasler, A. D. (1947). Eutrophication of lakes by domestic drainage. Ecology 28, 383–395. doi: 10.2307/1931228
Hastie, T., and Stuetzle, W. (1989). Principal curves. J. Am. Stat. Assoc. 84, 502–516. doi: 10.1080/01621459.1989.10478797
Haycock, B., and Hinton, G. (2010). “Monitoring Stoneworts Chara spp. at Bosherston Lakes,” in Conservation Monitoring in Freshwater Habitats: A Practical Guide and Case Studies, eds C. Hurford, M. Schneider, and I. Cowx (Dordrecht: Springer), 277–290. doi: 10.1007/978-1-4020-9278-7_25
Hering, D., Borja, A., Carvalho, L., and Feld, C. K. (2013). Assessment and recovery of European water bodies: key messages from the WISER project. Hydrobiologia 704, 1–9. doi: 10.1007/s10750-012-1438-9
Hilt, S., Henschke, I., Rücker, J., and Nixdorf, B. (2010). Can submerged macrophytes influence turbidity and trophic state in deep lakes? Suggestions from a case study. J. Environ. Qual. 39, 725–733. doi: 10.2134/jeq2009.0122
Hobbs, W., Irvine, K., and Donohue, I. (2005). Using sediments to assess the resistance of a calcareous lake to diffuse nutrient loading. Archiv. Hydrobiol. 164, 109–125. doi: 10.1127/0003-9136/2005/0164-0109
Hudon, C., Cattaneo, A., and Gagnon, P. (2009). Epiphytic cyanobacterium Gloeotrichia pisum as an indicator of nitrogen depletion. Aquat. Microbial. Ecol. 57, 191–202. doi: 10.3354/ame01344
Hutorowicz, A., and Dziedzic, J. (2008). Long-term changes in macrophyte vegetation after reduction of fish stock in a shallow lake. Aquat. Bot. 88, 265–272. doi: 10.1016/j.aquabot.2007.11.002
Jeppesen, E., Meerhoff, M. S. M., Lauridsen, T. L., and Jensen, J. P. (2007). Shallow lake restoration by nutrient loading reduction—some recent findings and challenges ahead. Hydrobiologia 584, 239–252. doi: 10.1007/s10750-007-0596-7
Jeppesen, E., Mehner, T., Winfield, I. J., Kangur, K., Sarvala, J., Gerdeaux, D., et al. (2012). Impacts of climate warming on the long-term dynamics of key fish species in 24 European lakes. Hydrobiologia 694, 1–39. doi: 10.1007/s10750-012-1182-1
Jones, R., Marshall, J., Fisher, E., Hatton, J., Patrick, C., Anderson, K., et al. (2011). Controls on lake level in the early to mid holocene, hawes water, lancashire, UK. Holocene 21, 1061–1072. doi: 10.1177/0959683611400455
Ju, L., Yang, J., Liu, L., and Wilkinson, D. M. (2014). Diversity and distribution of freshwater testate amoebae (protozoa) along latitudinal and trophic gradients in China. Microb. Ecol. 68, 657–670. doi: 10.1007/s00248-014-0442-1
Jupp, B. P., Spence, D. H. N., and Britton, R. H. (1974). The distribution and production of submerged macrophytes in Loch Leven, Kinross. Proc. R. Soc. Edinburgh B Biol. 74, 195–208. doi: 10.1017/S0080455X0001239X
Kelly, M., Juggins, S., Guthrie, R., Pritchard, S., Jamieson, J., Rippey, B., et al. (2008). Assessment of ecological status in UK rivers using diatoms. Freshw. Biol. 53, 403–422. doi: 10.1111/j.1365-2427.2007.01903.x
Kihlman, S., and Kauppila, T. (2012). Effects of mining on testate amoebae in a Finnish lake. J. Paleolimnol. 47, 1–15. doi: 10.1007/s10933-011-9541-x
Kłosowski, S., Tomaszewicz, G. H., and Tomaszewicz, H. (2006). The expansion and decline of charophyte communities in lakes within the Sejny Lake District (north-eastern Poland) and changes in water chemistry. Limnol. Ecol. Manag. Inland Waters 36, 234–240. doi: 10.1016/j.limno.2006.07.001
Korhola, A., and Rautio, M. (2000). “Cladocera and other branchiopod crustaceans,” in Tracking Environmental Change Using Lake Sediments, eds J. Smol, H. Birks, and W. Last (Dordrecht: Kluwer Academic Publishers), 5–41.
Kornijów, R., Peczula, W., Lorens, B., Ligeza, S., Rechulicz, J., and Kowalczyk-Pecka, D. (2002). Shallow Polesie lakes from the view point of the alternative stable states theory. Acta Agrophysica 68, 61–72.
Krammer, K., and Lange-Bertalot, H. (1986). “Bacillariophyecae, 1. Teil: Naviculaceae (Band 2/1),” in Süßwasserflora von Mitteleuropa, eds H. Ettl, J. Gerloff, H. Heynig, and D. Mollenhauer (Stuttgart: Gustav Fischer).
Krammer, K., and Lange-Bertalot, H. (1988). “Bacillariophyceae, 2. Teil: Bacillariaceae, Epithemiaceae, Surirellaceae (Band 2/2),” in Süßwasserflora von Mitteleuropa, eds H. Ettl, J. Gerloff, H. Heynig, and D. Mollenhauer (Stuttgart: Gustav Fischer).
Krammer, K., and Lange-Bertalot, H. (1991). “Bacillariophyecae, 3. Teil: Centrales, Fragilariaceae, Eunotiaceae (Band 2/3),” in Süßwasserflora von Mitteleuropa, eds H. Ettl, J. Gerloff, H. Heynig, and D. Mollenhauer (Stuttgart: Gustav Fischer).
Krammer, K., and Lange-Bertalot, H. (2004). “Bacillariophyecae, 4. Teil: Achnanthaceae, Kritische Erganzungen zu Achnanthes s.l, Navicula s.str., Gomphonema (Band 2/4),” in Süßwasserflora von Mitteleuropa, eds H. Ettl, J. Gerloff, H. Heynig, and D. Mollenhauer (Stuttgart: Gustav Fischer).
Krause, W., and King, J. J. (1994). The ecological status of Lough Corrib, Ireland, as indicated by physiographic factors, water chemistry and macrophytic flora. Plant Ecol. 2, 149–161. doi: 10.1007/BF00033395
Krolikowska, J. (1997). Eutrophication processes in a shallow, macrophyte-dominated lake - species differentiation, biomass and the distribution of submerged macrophytes in Lake Łuknajno (Poland). Hydrobiologia 342–343, 411–416. doi: 10.1023/A:1017055827120
Kufel, L., and Kufel, I. (2002). Chara beds acting as nutrient sinks in shallow lakes - a review. Aquat. Bot. 72, 249–260. doi: 10.1016/S0304-3770(01)00204-2
Kumar, A., and Dalby, A. P. (1998). Identification key for Holocene lacustrine arcellacean (thecamoebian) taxa. Palaeontol. Electronica 1, 4A.
Kwandrans, J., Eloranta, P., Kawecka, B., and Wojtan, K. (1998). Use of benthic diatom communities to evaluate water quality in rivers of southern Poland. J. Appl. Phycol. 10, 193–201. doi: 10.1023/A:1008087114256
Lake, P. (2001). On the maturing of restoration: linking ecological research and restoration. Ecol. Manag. Restor. 2, 110–115. doi: 10.1046/j.1442-8903.2001.00074.x
Last, W. M., and Smol, J. P. (eds.). (2001). Tracking Environmental Change Using Lake Sediments, 1st Edn. Dordrecht: Springer. doi: 10.1007/0-306-47669-x
Laugaste, R., and Lessok, K. (2004). Planktonic algae and epiphyton of the littoral in lake Peipsi, Estonia. Limnol. Ecol. Manag. Inland Waters 34, 90–97. doi: 10.1016/S0075-9511(04)80026-4
Leavitt, P. R. (1993). A review of factors that regulate carotenoid and chlorophyll deposition and fossil pigment abundance. J. Paleolimnol. 9, 109–127. doi: 10.1007/BF00677513
Lebkuecher, J., Craft, J., Hankenson, R., Johnson, J., and Martin, J. (2014). Impacts of nonpoint-source pollution on periphyton characteristics in the west fork of the Red River in north-central Tennessee. Phytoneuron 95, 1–7.
Leng, M. J., Lamb, A. L., Marshall, J. D., Wolfe, B. E., Jones, M. D., Holmes, J. A., et al. (2006). “Isotopes in lake sediments,” in Isotopes in Palaeoenvironmental Research, ed M. J. Leng (Dordrecht: Springer), 147–184. doi: 10.1007/1-4020-2504-1_04
Leng, M. J., and Marshall, J. D. (2004). Palaeoclimate interpretation of stable isotope data from lake sediment archives. Quat. Sci. Rev. 23, 811–831. doi: 10.1016/j.quascirev.2003.06.012
Lindholm, T., Rönnholm, E., and Häggqvist, K. (2008). Changes due to invasion of Myriophyllum sibiricum in a shallow lake in Åland, SW Finland. Aquat. Invasions 3, 10–13. doi: 10.3391/ai.2008.3.1.3
Luoto, T. P., and Nevalainen, L. (2009). “Larval chaoborid mandibles in surface sediments of small shallow lakes in Finland: implications for palaeolimnology,” in Palaeolimnological Proxies as Tools of Environmental Reconstruction in Fresh Water Developments in Hydrobiology, eds K. Buczkó, J. Korponai, J. Padisák, S. W. Starratt, and H. J. Dumont (Dordrecht: Springer), 185–195.
McGowan, S., Leavitt, P. R., Hall, R. I., Anderson, N. J., Jeppesen, E., and Odgaard, B. V. (2005). Controls of algal abundance and community composition during ecosystem state change. Ecology 86, 2200–2211. doi: 10.1890/04-1029
Medioli, F., and Scott, D. (1983). Holocene Arcellacea Thecamoebians from Eastern Canada. Washington, DC: Cushman Foundation Special Publication.
Meriläinen, J. J., Hynynen, J., Palomäki, A., Reinikainen, P., Teppo, A., and Granberg, K. (2000). Importance of diffuse nutrient loading and lake level changes to the eutrophication of an originally oligotrophic boreal lake: a palaeolimnological diatom and chironomid analysis. J. Paleolimnol. 24, 251–270. doi: 10.1023/A:1008111117718
Middelboe, A. L., and Markager, S. (1997). Depth limits and minimum light requirements of freshwater macrophytes. Freshw. Biol. 37, 553–568. doi: 10.1046/j.1365-2427.1997.00183.x
Mirosław-Grabowska, J., and Niska, M. (2007). Isotope and Cladocera data and interpretation from the Eemian optimum and postoptimum deposits, Kaliska palaeolake (Central Poland). Quat. Int. 175, 155–167. doi: 10.1016/j.quaint.2007.03.018
Moorhouse, H. L., McGowan, S., Jones, M. D., Barker, P., Leavitt, P. R., Brayshaw, S. A., et al. (2014). Contrasting effects of nutrients and climate on algal communities in two lakes in the Windermere catchment since the late 19th century. Freshw. Biol. 59, 2605–2620. doi: 10.1111/fwb.12457
Moss, B. (1979). Algal and other fossil evidence for major changes in Strumpshaw Broad, Norfolk, England in the last two centuries. Br. Phycol. J. 14, 263–283. doi: 10.1080/00071617900650301
Mullins, H. T. (1998). Environmental change controls of lacustrine carbonate, Cayuga Lake, New York. Geology 26, 443–446.
Negro, A. I., and de Hoyos, C. (2005). Relationships between diatoms and the environment in Spanish reservoirs. Limnetica 24, 133–144.
Odgaard, B. V., and Rasmussen, P. (2001). The occurrence of egg-cocoons of the leech Piscicola geometra (L.) in recent lake sediments and their relationship with remains of submerged macrophytes. Archiv. Hydrobiol. 152, 671–686.
Oksanen, J., Blanchet, F. G., Kindt, R., Legendre, P., Minchin, P. R., O'Hara, R. B., et al. (2011). vegan: Community Ecology Package. Available online at: http://R-Forge.R-project.org/projects/vegan/
Otley, J. (1830). A Concise Description of the English Lakes, and Adjacent Mountains: with General Directions to Tourists. Keswick: J. Otley.
Otsuki, A., and Wetzel, R. G. (1972). Coprecipitation of phosphate with carbonates in a marl lake. Limnol. Oceanogr. 17, 763–767. doi: 10.4319/lo.1972.17.5.0763
Padisak, J., and Reynolds, C. (1998). Selection of phytoplankton associations in Lake Balaton, Hungary, in response to eutrophication and restoration measures, with special reference to the cyanoprokaryotes. Hydrobiologia 384, 41–53. doi: 10.1023/A:1003255529403
Palm, F., El-Daoushy, F., and Svensson, J.-E. (2011). Fragmented subfossil Chaoborus mandibles reveal periods of cyprinid presence in lake histories. J. Palaeolimnol. 45, 101–111. doi: 10.1007/s10933-010-9483-8
Palm, F., El-Daoushy, F., and Svensson, J.-E. (2012). Development of subfossil Daphnia and Chaoborus assemblages in relation to progressive acidification and fish community alterations in SW Sweden. Hydrobiologia 684, 83–95. doi: 10.1007/s10750-011-0971-2
Papworth, S., Rist, J., Coad, L., and Milner-Gulland, E. (2009). Evidence for shifting baseline syndrome in conservation. Conserv. Lett. 2, 93–100. doi: 10.1111/j.1755-263x.2009.00049.x
Patmore, I. R., Sayer, C. D., Goldsmith, B., Davidson, T. A., Rawcliffe, R., and Salgado, J. (2014). Big Ben: a new wide-bore piston corer for multi-proxy palaeolimnology. J. Palaeolimnol. 51, 79–86. doi: 10.1007/s10933-013-9756-0
Patterson, R. T., and Fishbein, E. (1989). Re-examination of the statistical methods used to determine the number of point counts needed for micropaleontological quantitative research. J. Paleontol. 63, 245–248.
Patterson, R. T., Roe, H. M., and Swindles, G. T. (2012). Development of an Arcellacea (testate lobose amoebae) based transfer function for sedimentary Phosphorus in lakes. Palaeogeogr. Palaeoclimatol. Palaeoecol. 348–349, 32–44. doi: 10.1016/j.palaeo.2012.05.028
Peczuła, W., Suchora, M., and Toporowska, M. (2014). Ecological and trophic status of two small hardwater lakes of the Lublin Upland with agricultural catchments. Teka Komisji Ochrony i Kształtowania Œrodowiska Przyrodniczego 11, 146–155.
Pełechaty, M., Pukacz, A., Apolinarska, K., Pełechata, A., and Siepak, M. (2013). The significance of Chara vegetation in the precipitation of lacustrine calcium carbonate. Sedimentology 60, 1017–1035. doi: 10.1111/sed.12020
Pełechaty, M., Pukacz, A., and Pełechata, A. (2004). Diversity of micro- and macrophyte communities in the context of the habitat conditions of a meromictic lake on Lubuskie Lakeland. Limnol. Rev. 4, 209–214.
Pentecost, A. (2000). Some observations on the erosion of Tarn Moss by the waters of Malham Tarn. Field Stud. 9, 569–581.
Pentecost, A. (2009). The marl lakes of the British Isles. Freshw. Rev. 2, 167–197. doi: 10.1608/FRJ-2.2.4
Pitcairn, C. E. R., and Fowler, D. (1995). Deposition of fixed atmospheric nitrogen and foliar nitrogen content of bryophytes and Calluna vulgaris (L.) Hull. Environ. Pollut. 88, 193–205. doi: 10.1016/0269-7491(95)91444-P
Pretty, J. N., Mason, C. F., Nedwell, D. B., Hine, R. E., Leaf, S., and Dils, R. (2003). Environmental costs of freshwater eutrophication in England and Wales. Environ. Sci. Technol. 37, 201–208. doi: 10.1021/es020793k
Pukacz, A., Pełechaty, M., and Frankowski, M. (2014). Carbon dynamics in a hardwater lake: effect of charophyte biomass on carbonate deposition. Polish J. Ecol. 62, 695–705.
R Development Core Team. (2010). R: A Language and Environment for Statistical Computing. Vienna, Austria: R Foundation for Statistical Computing. Available online at: http://www.R-project.org/
Reynolds, C., Huszar, V., Kruk, C., Flores, N. L., and Melo, S. (2002). Towards a functional classification of the freshwater phytoplankton. J. Plankton Res. 24, 417–428. doi: 10.1093/plankt/24.5.417
Robertson, D. M., Garn, H. S., and Rose, W. J. (2007). Response of calcareous Nagawicka Lake, Wisconsin, to changes in phosphorus loading. Lake Reserv. Manag. 23, 298–312. doi: 10.1080/07438140709354018
Roe, H. M., Patterson, R. T., and Swindles, G. T. (2010). Controls on the contemporary distribution of lake thecamoebians (Testate amoebae) within the greater Toronto area and their potential as water quality indicators. J. Paleolimnol. 43, 955–975. doi: 10.1007/s10933-009-9380-1
Santos, G., Southon, J., Griffin, S., Beaupre, S., and Druffel, E. (2007). Ultra small-mass AMS 14C sample preparation and analyses at KCCAMS/UCI Facility. Nucl. Instrum. Methods Phys. Res. B 259, 293–302. doi: 10.1016/j.nimb.2007.01.172
Sayer, C., Andrews, K., Shilland, E., Edmonds, N., Edmonds-Brown, R., Patmore, I., et al. (2012). The role of pond management for biodiversity conservation in an agricultural landscape. Aquat. Conserv. 22, 626–638. doi: 10.1002/aqc.2254
Sayer, C., Burgess, A., Kari, K., Davidson, T., Peglar, S., Yang, H., et al. (2010). Long-term dynamics of submerged macrophytes and algae in a small and shallow, eutrophic lake: implications for the stability of macrophyte-dominance. Freshw. Biol. 55, 565–583. doi: 10.1111/j.1365-2427.2009.02353.x
Scheffer, M., Hosper, S. H., Meijer, M.-L., Moss, B., and Jeppesen, E. (1993). Alternative equilibria in shallow lakes. Trends Ecol. Evol. 8, 275–279. doi: 10.1016/0169-5347(93)90254-M
Scheffer, M., and van Nes, E. H. (2007). Shallow lakes theory revisited: various alternative regimes driven by climate, nutrients, depth and lake size. Hydrobiologia 584, 455–466. doi: 10.1007/s10750-007-0616-7
Schneider, S., Schranz, C., and Melzer, A. (2000). Indicating the trophic state of running waters by submersed macrophytes and epilithic diatoms: exemplary implementation of a new classification of taxa into trophic classes. Limnol. Ecol. Manag. Inland Waters 30, 1–8. doi: 10.1016/S0075-9511(00)80033-X
Schönborn, W. (1967). Taxozönotik der beschalten süsswasser-rhizopoden: eine raumstrukturanalytische untersuchung über lebensraumerweiterung und evolution bei der mikrofauna. Limnologica 5, 159–207.
Scott, D. B., and Hermelin, J. O. R. (1993). A device for precision splitting of micropaleontological samples in liquid suspension. J. Paleontol. 67, 151–154.
Sederias, J., and Colman, B. (2009). Inhibition of Chara vulgaris oospore germination by sulfidic sediments. Aquat. Bot. 91, 273–278. doi: 10.1016/j.aquabot.2009.07.006
Simpson, G. (2007). Analogue methods in palaeoecology: using the analogue package. J. Stat. Softw. 22, 1–29.
Simpson, G. L., and Birks, H. J. B. (2012). “Statistical learning in palaeolimnology,” in Tracking Environmental Change Using Lake Sediments, eds H. J. B. Birks, A. L. Lotter, S. Juggins, and J. P. Smol (Dordrecht: Springer), 249–327. doi: 10.1007/978-94-007-2745-8_9
Simpson, G., and Oksanen, J. (2011). Analogue: Analogue Matching and Modern Analogue Technique Transfer Function Models. (R package Version 0.7-6). Available online at: http://cran.r-project.org/package=analogue
Smith, D. C. (2003). The Population Biology of Charophytes in the Context of Shallow Lake Restoration. Ph.D. Thesis, University of East Anglia UK.
Smol, J. P., Birks, H. J. B., and Last, W. M. (eds.). (2001). Tracking Environmental Change Using Lake Sediments: Zoological Indicators. Dordrecht: Springer.
Spence, D. H. N. (1967). Factors controlling the distribution of freshwater macrophytes with particular reference to the lochs of Scotland. J. Ecol. 55, 147–170. doi: 10.2307/2257723
Spence, D. H. N. (1982). The zonation of plants in freshwater lakes. Adv. Ecol. Res. 12, 37–124. doi: 10.1016/S0065-2504(08)60077-X
Spence, D. H. N., Barclay, A. M., and Allen, E. D. (1984). Limnology and macrophyte vegetation of a deep, clear limestone lake, Loch Borralie. Trans. Bot. Soc. Edinburgh 44, 187–204. doi: 10.1080/03746608408685387
Stenger-Kovács, C., Buczkó, K., Hajnal, E., and Padisák, J. (2007). Epiphytic, littoral diatoms as bioindicators of shallow lake trophic status: Trophic Diatom Index for Lakes (TDIL) developed in Hungary. Hydrobiologia 589, 141–154. doi: 10.1007/s10750-007-0729-z
Stewart, N. (2001). Review of the Status of BAP Stonewort Species. Joint Nature Conservation Committee (Plantlife).
Stoate, C., Boatman, N. D., Borralho, R. J., Carvalho, C. R., de Snoo, G. R., and Eden, P. (2001). Ecological impacts of arable intensification in Europe. J. Environ. Manage. 63, 3379–3365. doi: 10.1006/jema.2001.0473
Szeroczyńska, K., and Sarmaja-Korjonen, K. (2007). Atlas of Subfossil Cladocera from Central and Northern Europe. Świecie: Friends of the Lower Vistula Society.
Thienpont, J. R., Korosi, J. B., Cheng, E. S., Deasley, K., Pisaric, M. F. J., and Smol, J. P. (2015). Recent climate warming favours more specialized cladoceran taxa in western Canadian Arctic lakes. J. Biogeogr. 42, 1553–1565. doi: 10.1111/jbi.12519
Tibby, J., Lane, M. B., and Gell, P. A. (2008). Local knowledge and environmental management: a cautionary tale from Lake Ainsworth, New South Wales, Australia. Environ. Conserv. 34, 334–341. doi: 10.1017/S037689290700433X
Tolonen, K. T., Brodersen, K. P., Kleisborg, T. A., Holmgren, K., Dahlberg, M., Hamerlik, L., et al. (2012). Phantom midge-based models for inferring past fish abundances. J. Palaeolimnol. 47, 531–547. doi: 10.1007/s10933-012-9579-4
Tremel, B., Frey, S. E. L., Yan, N. D., Somers, K. M., and Pawson, T. W. (2000). Habitat specificity of littoral Chydoridae (Crustacea, Branchiopoda, Anomopoda) in Plastic Lake, Ontario, Canada. Hydrobiologia 432, 195–205. doi: 10.1023/A:1004023003179
Ulén, B., Bechmann, M., Følster, J., Jarvie, H. P., and Tunney, H. (2007). Agriculture as a phosphorus source for eutrophication in the north-west European countries, Norway, Sweden, United Kingdom and Ireland: a review. Soil Use Manage. 23, 5–15. doi: 10.1111/j.1475-2743.2007.00115.x
Uutala, A. J. (1990). Chaoborus (Diptera: Chaoboridae) mandibles - paleolimnological indicators of the historical status of fish populations in acid-sensitive lakes. J. Palaeolimnol. 4, 139–151. doi: 10.1007/BF00226321
Vadeboncoeur, Y., Jeppesen, E., Zanden, M. J. V., Schierup, H.-H., Christoffersen, K., and Lodge, D. M. (2003). From Greenland to green lakes: cultural eutrophication and the loss of benthic pathways in lakes. Limnol. Oceanogr. 48, 1408–1418. doi: 10.4319/lo.2003.48.4.1408
Viner, A. B. (1985). Thermal stability and phytoplankton distribution. Hydrobiologia 125, 47–69. doi: 10.1007/BF00045925
Vis, C., Cattaneo, A., and Hudon, C. (2008). Shift from chlorophytes to cyanobacteria in benthic macroalgae along a gradient of nitrate depletion. J. Phycol. 44, 38–44. doi: 10.1111/j.1529-8817.2007.00429.x
Walker, D. (1955). Studies in the post-glacial history of British Vegetation XIV. Skelsmergh Tarn and Kentmere, Westmorland. New Phytol. 54, 222–254. doi: 10.1111/j.1469-8137.1955.tb06176.x
Wall, A. A. J., Magny, M., Mitchell, E. A. D., Vannière, B., and Gilbert, D. (2010). Response of testate amoeba assemblages to environmental and climatic changes during the Lateglacial-Holocene transition at Lake Lautrey (Jura Mountains, eastern France). J. Quat. Sci. 25, 945–956. doi: 10.1002/jqs.1377
Wang, H.-J., Wang, H.-Z., Liang, X.-M., and Wu, S.-K. (2014). Total phosphorus thresholds for regime shifts are nearly equal in subtropical and temperate shallow lakes with moderate depths and areas. Freshw. Biol. 59, 1659–1671. doi: 10.1111/fwb.12372
Werner, D. (1977). “Ecology of freshwater diatoms – diatom communities,” The Biology of Diatoms, eds J. H. Burnett, H. Baker, H. Beevers, and F. R. Whatley (Berkeley; Los Angeles: University of California Press), 284–332.
Whiteside, M. C. (1970). Danish chydorid Cladocera: modern ecology and core studies. Ecol. Monogr. 40, 79–118. doi: 10.2307/1942442
Wiik, E., Bennion, H., Sayer, C. D., and Clarke, S. J. (2014). Assessing the status of marl lakes under the European Union Water Framework Directive – insights from contemporary and palaeolimnological studies of three English lakes. Fundam. Appl. Limnol. 185, 121–138. doi: 10.1127/fal/2014/0648
Wiik, E., Bennion, H., Sayer, C. D., and Willby, N. J. (2013). Chemical and biological responses of marl lakes to eutrophication. Freshw. Rev. 6, 35–62. doi: 10.1608/FRJ-6.2.630
Wilson, A. (ed.). (1938). The Flora of Westmorland - An Account of the Flowering Plants, Ferns and Their Allies, Mosses, Hepatics and Lichens So Far Known to Occur in the County. Arbroath: T. Buncle & Company.
Živić, I., and Marković, Z. (2006). First finding of larvae of Chaoborus crystallinus (Diptera, Chaoboridae) in Serbia. Arch. Biol. Sci. Belgrade 58, 23–24. doi: 10.2298/ABS0603001Z
Keywords: marl lake, chara, palaeolimnology, eutrophication, lake management, ecological quality, cladocera, macrofossils
Citation: Wiik E, Bennion H, Sayer CD, Davidson TA, Clarke SJ, McGowan S, Prentice S, Simpson GL and Stone L (2015) The coming and going of a marl lake: multi-indicator palaeolimnology reveals abrupt ecological change and alternative views of reference conditions. Front. Ecol. Evol. 3:82. doi: 10.3389/fevo.2015.00082
Received: 01 May 2015; Accepted: 07 July 2015;
Published: 12 August 2015.
Edited by:
Isabelle Larocque-Tobler, The L.A.K.E.S. Institute, SwitzerlandReviewed by:
Mariusz Lamentowicz, Adam Mickiewicz University in Poznan, PolandKrystyna Magdalena Saunders, University of Bern, Switzerland
Copyright © 2015 Wiik, Bennion, Sayer, Davidson, Clarke, McGowan, Prentice, Simpson and Stone. This is an open-access article distributed under the terms of the Creative Commons Attribution License (CC BY). The use, distribution or reproduction in other forums is permitted, provided the original author(s) or licensor are credited and that the original publication in this journal is cited, in accordance with accepted academic practice. No use, distribution or reproduction is permitted which does not comply with these terms.
*Correspondence: Emma Wiik, Department of Biology, University of Regina, Laboratory Building, 3737 Wascana Parkway, Regina, SK S4S0A2, Canada,ZW1tYS53aWlrQHVyZWdpbmEuY2E=