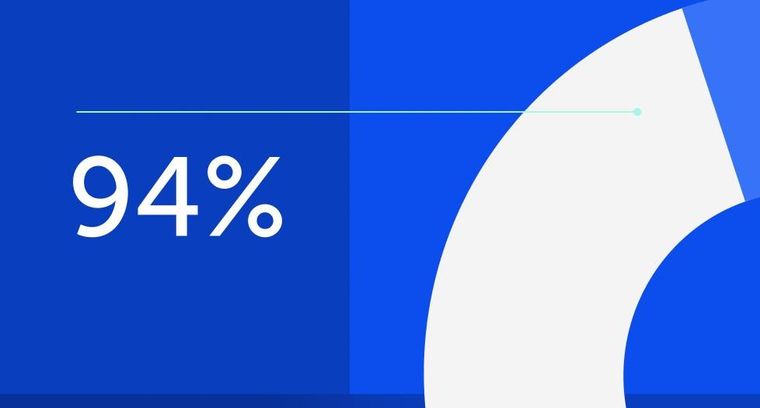
94% of researchers rate our articles as excellent or good
Learn more about the work of our research integrity team to safeguard the quality of each article we publish.
Find out more
ORIGINAL RESEARCH article
Front. Ethol., 10 October 2024
Sec. Adaptation and Evolution
Volume 3 - 2024 | https://doi.org/10.3389/fetho.2024.1464308
This article is part of the Research TopicSexual Selection and Evolutionary FitnessView all 6 articles
Although sexual selection can be a powerful evolutionary force in shaping the phenotype, sexually selected traits do not evolve in isolation of other traits or without influence from other selective pressures. Expensive tissues, such as brains, can constrain the evolution of sexually selected traits, such as testes, as can other energetically expensive processes, like the costs of locomotion. However, simple linear or binary analyses of specific traits of interest can prevent detection of important links within the integrated phenotype and obfuscate the importance of multiple selective forces. We used phylogenetically informed path analysis to determine causal links among mating system type, pace of life history, costs of locomotion, brain size, and testis size across 48 mammal species that exhibit a wide range of body sizes, life-history strategies, and types of locomotion. We found species with non-monogamous mating systems were associated with larger testes, faster life histories, and lower costs of locomotion compared to monogamous species. Having a larger brain was associated with a slower life history and, surprisingly, larger testes. In addition to highlighting the non-intuitive nature of certain causal relationships, our results also emphasize the utility of including multiple traits in studies of sexually selected traits, as well as considering the constraints imposed by linked traits and selection on those linked traits.
Sexual selection is the primary driving force behind the evolution of some of the most bizarre and extreme traits in the animal kingdom (Andersson, 1994). Although sexual selection has powerful effects on a number of key fitness-related traits, including both primary and secondary sexual traits, those phenotypic effects also depend upon a variety of other factors, including the selective context, phylogenetic placement, and ecological and phenotypic milieu of the organism in question (Cornwallis and Uller, 2010). Collectively, these factors have the potential to facilitate or mitigate the form and intensity of sexual selection operating on any given trait of interest. Classic examples of guppy coloration (Endler, 1983; Millar et al., 2006) and Túngara frog calls (Ryan et al., 1982) illustrate nicely how phenotypes are dynamic compromises that represent the net effect of all selective pressures within physical, physiological, and phylogenetic constraints. However, both of these examples are compromises due to conflicting selection on the same trait (i.e., coloration or calls).
The opportunity for sexual selection in a population is greatly influenced by the mating system in that population (Trivers, 1972; Emlen and Oring, 1977; Shuster and Wade, 2003; Gowaty, 2006; Hall et al., 2013; Henshaw et al., 2016); however, the way in which mating systems evolve can also affect a variety of traits other than those under the direct influence of sexual selection (Badyaev and Qvarnstrom, 2002; Pélabon et al., 2014). Furthermore, life-history strategies can impact mating system evolution and, in turn, the potential for sexual selection (Höglund and Sheldon, 1998; Kemp, 2002; King et al., 2013; Sowersby et al., 2022), which can result in numerous indirect phenotypic effects as a result of selection on life-history variation. Studies that do not consider this larger context risk either missing important links, or focusing overmuch on potentially spurious links that may be indirect or incidental (Hunt et al., 2009). For example, physiological or morphological traits may evolve to compensate for secondary sexual ornaments (reviewed in Husak and Swallow, 2011), but other simultaneous selection pressures may constrain or facilitate evolution of those compensatory traits, leading us to over- or under-estimate the impact of secondary sexual traits on other related phenotypes. Despite its potential importance, trait covariation was seldom considered in life-history theory until physiological traits were explicitly incorporated in recent decades (reviewed in Zera and Harshman, 2001; Ricklefs and Wikelski, 2002; Dammahan et al., 2018). Subsequent work has shown that because most traits are part of an integrated organismal phenotype bolstered by underlying patterns of genetic correlations and resource-based trade-offs (Ghalambor et al., 2003, 2004), sexually selected trait expression is influenced not only by other selective forces, but by selection on other traits, such as physiological and life-history traits, as well (Cornwallis and Uller, 2010).
Studies of phenotypic evolution tend to focus on evolutionary scenarios that explain variation in a particular trait(s) of interest. For example, there is a large literature regarding the evolutionary pressures driving variation in brain size across vertebrate species (Maklakov et al., 2011; Heldstab et al., 2022; Song et al., 2023), where the fitness benefits of evolving a large brain are thought to be countered by the incurred energetic costs, often reduced allocation to traits associated with reproduction or maintenance. A similarly large literature focusses on how mating systems, multiple mating, and sperm competition drive variation in testis size (Pitnick et al., 2006; Lüpold et al., 2020). Figure 1 illustrates some common, but not exhaustive, hypotheses concerning the evolution of testis and brain size. All of these hypotheses share the assumption that selection operates primarily on the trait of interest, with adjustments made elsewhere in the phenotype, typically to one or two ancillary traits. However, less consideration is given to the strength and direction of selection on those other traits, or to how that selection might impact the trait of interest (Figure 2A). Each of these target traits (e.g., brain, testes, etc.) is part of the integrated phenotype that evolves under numerous constraints, be they phylogenetic, energetic, quantitative genetic, or ecological. Thus, although these studies position their trait of interest as a dependent variable whose variation is to be explained by other factors, the trait of interest itself could just as reasonably be used as a predictor to explain variation in a different trait of interest in another study. We do not feel that this is an intentional omission by researchers, but just an artifact of how these hypotheses are set up to explain variation in the phenotype. Consequently, determining patterns of causality in trait evolution can be difficult to determine or even predict (Jiménez-Ortega et al., 2020). Life-history theory provides a way to approach such questions by examining the evolution of one trait in the context of investment in other traits, especially those that are energetically expensive (van Noordwijk and De Jong, 1986; Lemaítre et al., 2009; Laskowski et al., 2021).
Figure 1. Investing in a costly organ, such as a brain or testes, (solid lines) should result in decreased investment in other costly organs, and vice versa (dotted lines). Each of these hypotheses considers that selection operates primarily on the trait of interest, and adjustments are made elsewhere with less consideration of the strength and direction of selection happening on the other traits that might be affected. For example, in the expensive tissue hypothesis (Aiello and Wheeler, 1995), the benefits of evolving larger brains mean taking away from the digestive tract without regard to the strength of selection on digestion or other traits that might impose constraints on either trait. Similarly, in the expensive sexual tissue hypothesis (Pitnick et al., 2006), the benefits of evolving larger brains mean taking away from investment in testes or secondary sexual structures. The expensive brain hypothesis (Isler and Van Schaik, 2009) is more general than the expensive tissue hypothesis and predicts that resources may go toward either a large brain or toward reproduction or somatic maintenance.
Figure 2. Schematics showing how selection on multiple components of the phenotype sharing a common resource pool can obscure simple predictions about how selection impacts any one trait. (A) In an integrative life-history approach, the focus is not on a single trait, but instead assumes that selection is potentially happening on all or many traits simultaneously. Increasing or decreasing investment in any one trait can potentially alter investment in any other trait, depending on individual selection strengths and directions. (B) Strong sexual selection on an ornament may not lead to maximized ornament size (grey arrow) if there is also strong selection to have a large, expensive brain. Instead, the ornament evolves a smaller size (black arrow) as a phenotypic ‘compromise’ with brain size. (C) Strong selection for a large brain may not lead to maximized brain size (grey arrow) if there is also strong selection on locomotion and current reproduction due to life-history strategy. Instead, brain size evolves to be smaller than if selection acted on brain size alone (black arrow) as a phenotypic ‘compromise’ with locomotion, ornament size, and testis size (current reproduction).
Investment in current reproduction, growth, and brain mass are all energetically expensive traits that are rightly included in studies of mating systems and sexual selection, but the costs of locomotion, although potentially as important, are much less common. Whole-organism performance traits are quantitative traits that allow an individual to accomplish an ecologically relevant task in a dynamic way, including running, flying, and biting (Bennett and Huey, 1990; Lailvaux and Irschick, 2006). Locomotion and other whole-organism performance traits are key targets of selection (Arnold, 1983; Husak and Fox, 2008; Irschick et al., 2008) that are energetically expensive to develop, maintain, and use (Husak and Lailvaux, 2017). Locomotion in particular is an important trait (Dickinson et al., 2000), because every animal that must move during its life must deal with costs associated with locomotion (Tucker, 1970; Alexander, 2003; Pontzer, 2016). Locomotion costs are often considered to be a component of the cost of reproduction (Miles et al., 2000), and indeed the energetic costs of locomotion are related to pace-of-life across mammalian species, with slower-paced species having higher costs of transport and faster-paced species having lower costs (see also Lailvaux and Husak, 2017; Biro, 2024). Experimental manipulation of life-history traits also impacts locomotion (reviewed in Lailvaux and Husak, 2014), and experimental enhancement of locomotion through exercise training negatively impacts a variety of life-history traits (reviewed in Husak and Lailvaux, 2022). Therefore, costs of locomotion are essential to consider in studies that seek to explain variation in a sexually selected trait, any single life-history trait, or a suite of life-history traits (Lailvaux and Husak, 2014, 2017; Garland et al., 2022).
Understanding the collective selective pressures acting on a particular trait of interest is important but difficult to achieve by simple experiment. If one is interested in the evolution of an ornament under the influence of sexual selection, for example, then both the consequences to other traits of that increased investment and the intensity of selection on those other traits should be considered (Hunt et al., 2009). Figure 2 shows such an approach. Strong selection on brain size may constrain ornament size (Figure 2B), yet the upper limit of brain size might itself be constrained by investment in locomotor costs due to strong selection on locomotion (Figure 2C). Even if ample acquired resources remain following allocation to locomotion, strong selection for current reproduction might conceivably divert those resources away from brain growth and maintenance (Figure 2C). The near-endless number of potential alternative scenarios means that, when considering the evolution of a sexually selected ornament, as many fitness-related traits as possible should be considered, especially those that are relatively expensive, such as key life-history traits, brain size (Aiello and Wheeler, 1995), testis size (Pitnick et al., 2006) (Lüpold et al., 2020), and locomotor costs (Husak and Lailvaux, 2017).
In this paper, we conduct such an analysis across mammal species, which are well-suited for comparative analyses of phenotypic evolution. Mammal life-histories and mating systems have been extensively studied, and many studies regarding variation in testis (Kenagy and Trombulak, 1986; Iossa et al., 2008; Lemaítre et al., 2009) and brain size (Pérez-Barberia et al., 2007; Isler and Van Schaik, 2012; Gonzalez-Voyer et al., 2016) have been conducted on mammals. Mammals also have a wide variety of locomotor strategies, including terrestrial running/walking, swimming, and flying, with substantial data available on the costs of these types of locomotion. They have also been an important group in the development of pace of life syndrome (POLS) theory (Vasilieva, 2022), with fast POLS species favoring current reproduction over survival and tending to be smaller with shorter lifespans and larger litters, amongst other traits, whereas slow POLS species are larger and more long-lived, producing fewer offspring and commencing breeding later in life (Gaillard et al., 1989; Harvey et al., 1989; Promislow and Harvey, 1990). Additionally, variation in mammalian POLS can be captured by levels of insulin-like growth factor-I (IGF1), with faster species having higher IGF1 levels than slower species (Swanson and Dantzer, 2014). Finally, POLS is associated with costs of locomotion across species, with faster-paced species having lower costs, and slower-paced species having higher costs (Lailvaux and Husak, 2017). Thus, we expect that the energetic costs of locomotion will impinge upon other energetically expensive traits and show clear links with mating system, brain size, and testis size as well.
We used phylogenetically informed path analysis to test for links among mating system, pace of life, costs of locomotion, brain size, and testis size. We compared several potential models of causal links based on predictions from the literature. Overall, we predicted that the type of mating system would directly influence testis size and costs of locomotion, resulting in indirect effects on brain size. We predicted hypothesis 1 in Figure 3 to be the best supported model. Decades of sexual selection research suggest that mating system should have a direct effect on testis size, with monogamous species having smaller testes than non-monogamous species (Harcourt et al., 1981; Kenagy and Trombulak, 1986). Pace of life should influence mating system, with faster life histories having non-monogamous mating systems and slower life histories having monogamous mating systems (Tarka et al., 2018). The expensive brain hypothesis (Isler and Van Schaik, 2009) predicts that species with slower life histories should have larger brains than species with faster life histories, because the latter will invest more energy in current reproduction. Similarly, the expensive brain hypothesis predicts that species with lower costs of locomotion should have larger brains than species with higher costs of locomotion (Heldstab et al., 2022). Costs of locomotion should also depend on pace of life, with faster-paced species having lower costs of locomotion compared to slower-paced species (Lailvaux and Husak, 2017). The expensive sexual tissue hypothesis (Pitnick et al., 2006) predicts that investment in a large brain will result in decreased investment in testes. Similarly, if species have low costs of locomotion, then they can invest more in testes compared to species with high costs of locomotion (Pitnick et al., 2006). Finally, we predicted that mating system would predict costs of locomotion, since non-monogamous species are expected to have increased activity for territory defense, mate searching, displaying, or courting potential mates (Emlen and Oring, 1977; Daly, 1978; Husak and Fox, 2008; Lane et al., 2010; Soulsbury, 2019). However, as we point out above, the causal direction is difficult to predict for many of these links (see also Jiménez-Ortega et al., 2020), so we also tested models with reverse causality (Figure 3).
Figure 3. Hypotheses compared with phylopath. Model 1 was our a priori hypothesis, and subsequent models have different links shown with grey arrows. Model 4 is the same as Model 3 but is missing the path from ECT to brain size. Solid arrows are links in all models, and dashed/dotted arrows were not present in every model. Brain, brain mass; ECT, ecological cost of transport; IGF1, insulin-like growth factor-1, a proxy of life-history pace of life; Testes, testis mass.
It becomes obvious from following these predictions that if some causal links are true, then others cannot be. For example, non-monogamous mating systems should have increased costs of locomotion, which should in turn reduce testis size, and in turn increase brain size. However, testis size should be larger in non-monogamous compared to monogamous species. Similarly, increased costs of locomotion could also directly reduce brain size (or at least prevent its enlargement), which should allow an increase in testis size. This example, and many other possible ones, highlight the integrated nature of the phenotype, as well as how conflicting selection pressures among linked traits can make simple binary predictions more complex. Thus, any causal links detected in path analysis will depend on the species in the analysis and their unique selection regimes.
We added data on mating system, brain, and testis size to an existing database of mammal life-history traits (Swanson and Dantzer, 2014; Lailvaux and Husak, 2017) to ask how pace of life impacts brain size, testes size, and locomotor costs within the context of mating system. For each species within the mammal database used by Lailvaux and Husak (2017), we sourced data on brain size, testes size, and mating system from the literature (see supplementary references). Because phylogenetic path analysis can only handle binary dependent categorical variables, we categorized mating system as socially monogamous or non-monogamous (Mabry et al., 2013). As a measurement of the cost of locomotion, we used the ecological cost of transport (ECT), defined by Garland (1983) as the percentage of daily energetic expenditure accounted for by the energetic costs of movement. Although existing ECT data are sparse, Lailvaux and Husak (2017) showed that ECT covaries negatively with the slow-fast life-history continuum in mammals such that species with fast life-history allocate less of their daily energy budget towards locomotion compared to those on the slow end of the continuum. Our original approach was to use phylogenetic principal components to derive multivariate axes capturing variation among seven key life-history traits [body size; lifespan; basal metabolic rate; gestation period; litter size; weaning age; and age at reproductive maturity (following Swanson and Dantzer, 2014; Lailvaux and Husak, 2017)]; however, preliminary analysis using both ordinary least squares and phylogenetic generalized least squares revealed significant collinearity between the derived PC1 axis capturing the majority of the slow-fast life-history continuum and brain size. Because incorporating brain size into the phylogenetic PCA would have cost us the ability to test for independent relationships between these two variables and ECT/testes size, we use measurements of circulating insulin-like growth factor-I (IGF1) as a proxy for life-history variation in our analyses here. Dantzer and Swanson (2012); Swanson and Dantzer (2014) showed that IGF1 covaries positively with fast life-histories in a phylogenetic framework across 41 species of mammals. Thus, higher IGF1 levels correspond to faster life histories (early maturity, short lifespan, and fast reproductive rate), whereas low levels correspond to slower life histories (older age at maturity, longer lifespan, and slower reproduction). Because we found that body size is collinear with brain size as well, we did not include an explicit size variable in any of our path diagrams here as many previous studies using path analysis do; however, we note that body size is an integral component of the life-history strategies that is explicitly captured by IGF1, just as are the other major components of the slow-fast life-history continuum (Swanson and Dantzer, 2014). Consequently, variation in body size is an integral component of the variation described by circulating IGF1.
Complete datasets are necessary for phylogenetic path analysis (von Hardenberg and Gonzalez-Voyer, 2013). Our dataset here was limited primarily by the availability of testis mass data, and several other variables of interest could not be included in our analyses due to a lack of relevant data in the literature. Where mass data were reported for both testes combined, we divided that value by 2. In cases where testis size is reported as volume rather than mass, or given only by length and width dimensions, we converted those data to mass in grams following Moller (1991). Briefly, we used the formula for volume of a prolate spheroid (, where a = and b = ) to convert length and width measures to cm3, and then multiplied this value by 1.087 (as in Moller, 1991). Nonetheless, of the 72 species of mammals analyzed by Lailvaux and Husak (2017), 23 were excluded due to missing data, and one was not represented on the most recent mammal phylogeny (see below). Our final dataset for path analyses therefore comprised 48 mammal species.
We used phylogenetic path analysis (von Hardenberg and Gonzalez-Voyer, 2013) as implemented in the phylopath package (Van der Bijl, 2018) in R v. 4.4 (Team, 2024) to test alternative hypotheses regarding the relationships among mating system, ECT, testis size, brain size, and the slow-fast life-history continuum (as represented by circulating IGF1). We log-transformed all traits prior to analyses, both to meet assumptions of normality, and because allometric relationships among life-history traits tend to be log-linear. Path analysis is based on multiple regression and tests for different hypothesized causal links among variables, represented as direct acyclic graphs (DAGs) (Shipley, 2000). The benchmark for model fit is the minimum number of conditional independences (i.e., cases where conditioning on a variable renders the outcomes independent of a treatment) that must be true to not reject the causal model, and the p-values of the conditional independences for a given model combined are represented by Fisher’s C statistic, a goodness of fit measure of the model to the data. The C statistic exhibits a Chi-squared distribution with 2k degrees of freedom, where k denotes the number of conditional independences in a model. Cases where sufficient conditional dependences are not met, as indicated by a significant C statistic at the 0.05 alpha level, indicates a poor fit of the model to the data and thus a rejection of the candidate causal model (Shipley, 2000). The C statistic information criteria (CIC) allows for comparison and ranking of non-nested models, with models differing by >2 CICc units typically considered to be different (Van der Bijl, 2018). Models are also weighted by their likelihood, wi.
Phylogenetic path analysis allows such causal models to be fit to interspecific data, taking into account the evolutionary relationships among the species of interest (von Hardenberg and Gonzalez-Voyer, 2013). We used the most recent, comprehensive mammalian timetree integrating phylogenomic data (Àlvarez-Carretero et al., 2022). We fit all path models using Pagel’s ʎ.
The best-fitting models provide consistent evidence for ecological cost of transport being shaped by mating system, brain size, and pace of life (as indicated by circulating IGF1). Our results further indicate that testis size is affected not only by mating system, but by the costs of locomotion and brain size as well. Our a priori hypothesis (hypothesis 1), although exhibiting a good fit to the data (p = 0.464), was not the best fitting model. Instead, hypothesis 2, which differs from hypothesis 1 in that it reverses the causality between IGF1 and brain size, and also lacks a relationship between brain size and ECT (to avoid unacceptable directed cycles), was the best fitting model (ΔCICc = 3.3 between hypothesis 1 and hypothesis 2; Table 1; see also Supplementary Table S1). Figure 4 presents the causal pathways and coefficients of this best fit model. This indicates that brain size determines IGF1, and thus pace-of-life, and also exerts an indirect effect on testis size. Mating system exerts a negative effect on the ecological cost of transport in hypothesis 2, suggesting that mammal species following a polygamous strategy spend less energy on a day-to-day basis on locomotion compared to monogamous species. Mating system is also positively related to testis size, such that polygamous species exhibit larger testes than do monogamous species. Thus, our best path model recapitulates a classic evolutionary relationship. Furthermore, IGF1 has a positive and direct effect on mating system, such that polygamous species occupy the fast region of the pace-of-life spectrum. These results also confirm the previous findings of Lailvaux and Husak (2017), showing a negative relationship between IGF1 and ECT, such that species with heightened IGF1 that exhibit faster life histories also spend less energy on locomotion on a daily basis, likely because they cannot afford to spend more. Finally, hypothesis 2 suggests a modest positive effect of ECT on testis size. By contrast, our final two models (hypothesis 3 and hypothesis 4) each exhibited a poor fit to the data, yielding significant C statistics and ΔCICc values of 9.5 and 12 respectively in comparison to our best fit hypothesis 2 (Table 1).
Table 1. Four of the tested models ordered based on ΔCICc value, where k represents the number of parameters; q number of tested conditional independencies; the C statistic and associated p values; CICc; and corresponding weight (w).
Figure 4. Best path model from comparison of models in Figure 4. Arrows represent the hypothesized causal links and values represent standardized coefficients. Positive links are black arrows, and negative links are grey arrows. Abbreviations are as in Figure 3.
The integrated nature of organismal phenotypes renders perilous the search for associations between individual traits without taking into account that organismal context. Here we show that expensive tissues, namely the brain and testes, evolve in concert with mating system, life history, and the costs of locomotion in mammals. We confirmed several relationships in mammals, such as how non-monogamous mating systems are associated with larger testes and slow-paced life histories are associated with low costs of locomotion. However, we also found several novel associations among variables that are likely important to the evolution of mating systems and sexually selected traits. First, monogamous species spend more of their time daily moving in the environment compared to non-monogamous species. Second, non-monogamous species are associated with faster life histories compared to monogamous species. Third, having a larger brain is associated with a slower life history. Finally, contrary to our prediction from the expensive brain hypothesis, we found a positive association between brain and testis size, as well as between costs of locomotion and testis size. These results show that including multiple key variables together in a path analysis can reveal patterns that result from multiple selective pressures acting on various components of the phenotype simultaneously.
One of our main predictions was that there would be tradeoffs between locomotion costs and either testis size or brain size. Migratory bird species, for example, have smaller brains for their body size, presumably as a consequence of energetically demanding migration (Sol et al., 2010). However, our best-supported causal scenario exhibited no association between ECT and brain size in our mammal dataset; instead, we found a positive association between ECT and testis size. We expected that species expending more energy to move more would have less available to other expensive tissues, but our results suggest that mammals moving for higher proportions of their day had larger testes than those moving less. One possible explanation for this is that ECT does not capture costs of locomotion well enough, as it does not distinguish the type of locomotion used for the calculations. It is possible that low-cost locomotor strategies are used for much of the active time, thus reducing energy taken from other tissues. ECT also does not distinguish the purpose of that locomotion; if much of the movement is for foraging, then those with higher ECT could have higher resource acquisition rates than those with lower ECT. For example, the high ECT values exhibited by carnivores made them clear outliers to the general mammalian relationship between ECT and the pace of life continuum reported by Lailvaux and Husak (2017); (see also Garland, 1983). This could explain why non-monogamous species did not have higher ECT values than monogamous species; the amount of locomotion by non-monogamous species used for sexually selected purposes may be low compared to that used for foraging or other purposes.
As predicted, we found that monogamous mating systems were associated with slower life-histories. Although necessarily simplified for the purposes of the phylogenetic path analysis, the relationships between mating system and testis size and between mating system and ECT nonetheless exhibited moderate to high path coefficients (Figure 4). A further important caveat here is that we used circulating IGF1 as a proxy for the multivariate pace-of-life continuum [based on the relationship reported by Swanson and Dantzer (2014)] due to collinearity issues with brain size in particular (see Methods above). Thus, our relationship between mating system and pace-of-life is inferred based on links between a simplified variable and a proxy. It is likely that a more fine-grained analysis of mating strategies could partition these connections further; for example, sexually selected weapons and ornaments show distinct trade-offs with testes size in primates (Lüpold et al., 2019), and recent evidence suggests that pre- and post-copulatory sexual selection may impose different trade-offs on specific life-history traits as well (Chung et al., 2024). Studies that are able to parse diverse mammalian mating systems into subsets that are subject to the various modes of sexual selection would be useful for further integrating locomotor costs into the life-history/expensive tissues paradigm.
The positive association between brain and testis size is somewhat surprising, since this seems to contradict studies testing the expensive brain (Heldstab et al., 2022) and expensive sexual tissue (Pitnick et al., 2006) hypotheses. Since the pace of life history (IGF1), which also captures body size variation, was included in the path models, overall body size is not the reason for this association. The expensive sexual tissue hypothesis has been controversial, with many studies finding no tradeoff between brains and testes, including across a wide taxonomic sampling of mammals (Lemaítre et al., 2009). So, what might explain our positive relationship here? In their large comparative analysis, Lemaítre et al. (2009) found a non-significant trend for a similar positive relationship among rodents, but there was no hypothesis given to explain the trend. Kotrschal et al. (2015) found that guppies artificially selected for larger brains also had higher investment in sexual structures (but not testis mass). Did our sample detect a case where there is strong selection for big brains and large testes that are compensated for by evolutionary decreases in other parts of the phenotype? The best path model has mating system, ECT, and brain size all as causal links to testis size, but no causal links to brain size were supported. This suggests that there are variables not included in our analyses that impact brain size, which then in turn take resources away from other traits and facilitates a slower pace of life in mammals. We also note that, even though path analysis can find directional correlations, causality is still difficult to determine.
Although large brains appear to be associated with slower life histories in mammals (Deaner et al., 2003; Isler and Van Schaik, 2012) and other vertebrate lineages (Heldstab et al., 2022), the generality of this is uncertain. For example, bigger brains are associated with faster life histories in killifishes (Sowersby et al., 2021). This seeming exception is a good example of how considering selection on all life history traits may reveal different patterns; here, fast-living killifish have lower somatic maintenance costs (Blažek et al., 2013) and may be able to invest more in brain size because of the less constrained energy budget (Sowersby et al., 2021). Indeed, the direction of the link between brain size and pace of life is difficult to predict from first principles because so many coevolving traits are involved. In primates it is hypothesized that the large time and energy investment in brain growth for species with large brains leads to the evolution of longer lives and reproductive investment commensurate with that slow-paced strategy (Leigh, 2004; Heldstab et al., 2022). Indeed, González-Lagos et al. (2010) found that mammals with relatively larger brains enjoyed longer lifespans across 493 mammal species. Similarly, in a comparative study of 217 parrot species (Smeele et al., 2022), the evolution of larger brains was suggested to allow the evolution of greater longevity because of the cognitive advantages of larger brains in dealing with environmental variability or predators (see also Jiménez-Ortega et al., 2020). Perhaps within specific mammalian taxa, different selective pressures are associated with having large brains. Chambers et al. (2021) found that brain size in primates was associated with ecological factors and sociality, whereas only ecological factors, especially home range size, were found to be associated with brain size in carnivores. In pinnipeds (seals, sea lions, and walruses), sexual selection for large male body size has decoupled the evolutionary relationship between body size and brain size, resulting in relatively smaller brains in species where sexual selection is intense (Fitzpatrick et al., 2012). Finally, sociality and cognition have also been hypothesized to affect brain size in concert in several taxa (Ashton et al., 2018). When species from across all mammals are included, perhaps the multitude of direct links become muddled, which would explain why path models with the causal link between IGF1 and brain size reversed (hypotheses 1 and 2 in Figure 3) were both supported with our data.
Our results revealed both previously known and novel links among mating system type, pace of life, expensive tissues, and costs of locomotion. Although the number of species in our analysis is somewhat modest, we feel that our approach is useful for future studies, both from an analytical perspective and from a conceptually integrative perspective; more potentially important variables in an analysis will give a clearer picture of how the integrated phenotype evolves under diverse selective pressures and within phylogenetic constraints. A challenge moving forward is to have enough data on enough species. Indeed, this was our biggest challenge: finding species for which we had data for all the variables. Publicly accessible databases (Kissling et al., 2014; Gainsbury et al., 2018) and the publication of full datasets will help to alleviate this for future studies.
The original contributions presented in the study are included in the article/Supplementary Material. Further inquiries can be directed to the corresponding author.
JH: Conceptualization, Investigation, Methodology, Validation, Visualization, Writing – original draft, Writing – review & editing. MS: Conceptualization, Data curation, Validation, Writing – original draft, Writing – review & editing. SL: Conceptualization, Data curation, Formal analysis, Investigation, Methodology, Software, Validation, Visualization, Writing – original draft, Writing – review & editing.
The author(s) declare that no financial support was received for the research, authorship, and/or publication of this article.
We thank Gabriella Gardner for help with data collection.
The authors declare that the research was conducted in the absence of any commercial or financial relationships that could be construed as a potential conflict of interest.
The author(s) declared that they were an editorial board member of Frontiers, at the time of submission. This had no impact on the peer review process and the final decision.
All claims expressed in this article are solely those of the authors and do not necessarily represent those of their affiliated organizations, or those of the publisher, the editors and the reviewers. Any product that may be evaluated in this article, or claim that may be made by its manufacturer, is not guaranteed or endorsed by the publisher.
The Supplementary Material for this article can be found online at: https://www.frontiersin.org/articles/10.3389/fetho.2024.1464308/full#supplementary-material
Aiello L. C., Wheeler P. (1995). The Expensive-Tissue hypothesis: the brain and the digestive system in human and primate evolution. Curr. Anthropol. 36, 199–221.
Àlvarez-Carretero S., Tumari A. U., Battini M., Nascimento F. F., Carlisle E., Asher R. J., et al. (2022). A species-level timeline of mamal evolution integrating phylogenomic data. Nature 602, 263–267. doi: 10.1038/s41586-021-04341-1
Arnold S. J. (1983). Morphology, performance, and fitness. Am. Zoology 23, 347–361. doi: 10.1093/icb/23.2.347
Ashton B. J., Thornton A., Ridley A. R. (2018). An intraspecific appraisal of the social intelligence hypothesis. Philos. Trans. R. Soc. B 373, 20170288. doi: 10.1098/rstb.2017.0288
Badyaev A. V., Qvarnstrom A. (2002). Putting sexual traits into the context of an organism: a life-history perspective in studies of sexual selection. Auk 119, 301–310. doi: 10.1093/auk/119.2.301
Bennett A. F., Huey R. B. (1990). “Studying the evolution of physiological performance,” in Oxford Surveys in Evolutionary Biology. Eds. Futuyma D. J., Antonovics J. (Oxford University Press, Oxford), 251–284.
Biro P. A. (2024). Testing personality-pace-of-life associations via artifical selection: insights from selected lines of ranbow trout on the context-dependence of correlations. Biol. Lett. 20, 20240181. doi: 10.1098/rsbl.2024.0181
Blažek R., Polačik M., Reichard M. (2013). Rapid growth, early maturation, and short generation time in African annual fishes. Evodevo 4, 1–7. doi: 10.1186/2041-9139-4-24
Chambers H. R., Heldstab S. A., O’Hara S. J. (2021). Why big brains? A comparison of models for both primate and carnivore brain size evolution. PloS One 16, e0261185. doi: 10.1371/journal.pone.0261185
Chung M. H. J., Fox R. J., Jennions M. D. (2024). Male allocation to ejaculation and mating effort imposes different life history trade-offs. PloS Biol. 22, e3002519. doi: 10.1371/journal.pbio.3002519
Cornwallis C. K., Uller T. (2010). Towards an evolutionary ecology of sexual traits. Trends Ecol. Evol. 25, 145–152. doi: 10.1016/j.tree.2009.09.008
Dammahan M., Dingemanse N. J., Niemelä P. T., Réale D. (2018). Pace-of-life syndromes: a framework for the adaptive integration of behaviour, physiology, and life history. Behav. Ecol. Sociobiology 72, 1–8. doi: 10.1007/s00265-018-2473-y
Dantzer B., Swanson E. M. (2012). Mediation of vertebrate life histories via insulin-like growth factor-1. Biol. Rev. 87, 414–429. doi: 10.1111/j.1469-185X.2011.00204.x
Deaner R. O., Barton R. A., Schaik C. P. (2003). “Primate brains and life histories: renewing the connection,” in Primate life histories and socioecology. Eds. Kappeler P. M., Pereira M. E. (Chicago, IL USA: University of Chicago Press).
Dickinson M. H., Farley C. T., Full R. J., Koehl M. A. R., Kram R., Lehman S. (2000). How animals move: an integrative view. Science 288, 100–106. doi: 10.1126/science.288.5463.100
Emlen S. T., Oring L. W. (1977). Ecology, sexual selection, and the evolution of mating systems. Science 197, 215–223. doi: 10.1126/science.327542
Endler J. A. (1983). Natural and sexual selection on color patterns in poeciliid fishes. Environ. Biol. Fishes 9, 173–190. doi: 10.1007/BF00690861
Fitzpatrick J. L., Almbro M., Gonzalez-Voyer A., Hamada S., Pennington C., Scanlan J., et al. (2012). Sexual selection uncouples the evolution of brain and body size in pinnipeds. J. Evolutionary Biol. 25, 1321–1330. doi: 10.1111/j.1420-9101.2012.02520.x
Gaillard J. M., Pontier D., Allaine D., Lebreton J. D., Touvillez J., Clobert J. (1989). An analysis of demographic tactics in birds and mammals. Oikos 56, 59–76. doi: 10.2307/3566088
Gainsbury A. M., Tallowin O. J. S., Meiri S. (2018). An updated global dataset for diet preferences in terrestrial mammals: testing the validity of extrapolation. Mamm. Rev. 48, 160–167. doi: 10.1111/mam.2018.48.issue-3
Garland T. (1983). Scaling the ecological cost of transport to body mass in terrestrial mammals. Am. Nat. 121, 571–587. doi: 10.1086/284084
Garland T., Downs C. J., Ives A. R. (2022). Trade-offs (and constraints) in organismal biology. Physiol. Biochem. Zoology 95, 82–112. doi: 10.1086/717897
Ghalambor C. K., Reznick D. N., Walker J. A. (2004). Constraints on adaptive evolution: the functional trade-off between reproduction and fast-start swimming performance in the Trinidadian guppy (Poecilia reticulata). Am. Nat. 164, 38–50. doi: 10.1086/421412
Ghalambor C. K., Walker J. A., Reznick D. N. (2003). Multi-trait selection, adaptation, and constraints on the evolution of burst swimming performance. Integr. Comp. Biol. 43, 431–438. doi: 10.1093/icb/43.3.431
González-Lagos C., Sol D., Reader S. M. (2010). Large-brained mammals live longer. J. Evolutionary Biol. 23, 1064–1074. doi: 10.1111/j.1420-9101.2010.01976.x
Gonzalez-Voyer A., González-Suárez M., Vilà C., Revilla E. (2016). Larger brain size indirectly increases vulnerability to exinction in mammals. Evolution 70, 1364–1375. doi: 10.1111/evo.12943
Gowaty P. A. (2006). “Beyond extra-pair paternity: constraints, fitness components, and social mating systems,” in Essays in Animal Behaviour - Celebrating 50 Years of Animal Behaviour. Eds. Lucas J. R., Simmons L. W. (Elsevier Academic Press, Burlington), 221–254.
Hall M. D., Lailvaux S. P., Brooks R. C. (2013). Sex-specific evolutionary potential of pre-and postcopulatory reproductive interactions in the field cricket, Teleogryllus commodus. Evolution 67, 1831–1837. doi: 10.1111/evo.12067
Harcourt A. H., Harvey P. H., Larson S. G. (1981). Testis weight, body weight and breeding system in primates. Nature 293, 55–57. doi: 10.1038/293055a0
Harvey P. H., Read A. F., Promislow D. E. L. (1989). “Life-history variation in placental mammals: unifying data with theory.” in Oxford Surveys in Evolutionary Biology, Volume 6. eds. Harvey P. H., Partridge L. (New York: Oxford University Press), 23–32.
Heldstab S. A., Isler K., Graber S. M., Schuppli C., van Schaik C. P. (2022). The economics of brain size evolution in vertebrates. Curr. Biol. 32, R697–R708. doi: 10.1016/j.cub.2022.04.096
Henshaw J. M., Kahn A. T., Fritzsche K. (2016). A rigorous comparison of sexual selection indexes via simulations of diverse mating systems. Proc. Natl. Acad. Sci. United States America 113, E300–E308. doi: 10.1073/pnas.1518067113
Höglund J., Sheldon B. C. (1998). The cost of reproduction and sexual selection. Oikos 83, 478–483. doi: 10.2307/3546675
Hunt J., Breuker C. J., Sadowski J. A., Moore A. J. (2009). Male-male competition, female mate choice and their interaction: determining total sexual selection. J. Evolutionary Biol. 22, 13–26. doi: 10.1111/j.1420-9101.2008.01633.x
Husak J. F., Fox S. F. (2008). Sexual selection on locomotor performance. Evolutionary Ecol. Res. 10, 213–228.
Husak J. F., Lailvaux S. P. (2017). How do we measure the cost of whole-organism performance traits? Integr. Comp. Biol. 57, 333–343. doi: 10.1093/icb/icx048
Husak J. F., Lailvaux S. P. (2022). Conserved and convergent mechanisms underlying performance-life-history trade-offs. J. Exp. Biol. 225, jeb243351. doi: 10.1242/jeb.243351
Husak J. F., Swallow J. G. (2011). Compensatory traits and the evolution of male ornaments. Behaviour 148, 1–29. doi: 10.1163/000579510X541265
Iossa G., Soulsbury C. D., Baker P. J., Harris S. (2008). Sperm competition and the evolution of testes size in mammalian carnivorees. Funct. Ecol. 22, 655–662. doi: 10.1111/j.1365-2435.2008.01409.x
Irschick D. J., Meyers J. J., Husak J. F., Le Galliard J. (2008). How does selection operate on whole-organism functional performance capacities? A review and synthesis. Evolutionary Ecol. Res. 10, 177–196.
Isler K., Van Schaik C. P. (2009). The expensive brain: a framework for understanding evolutionary changes in brain size. J. Hum. Evol. 57, 392–400. doi: 10.1016/j.jhevol.2009.04.009
Isler K., Van Schaik C. P. (2012). Allomaternal care, life history and brain size evolution in mammals. J. Hum. Evol. 63, 52–63. doi: 10.1016/j.jhevol.2012.03.009
Jiménez-Ortega D., Kolm N., Immler S., Maklakov A. A., Gonzalez-Voyer A. (2020). Long life evolves in large-brained bird lineages. Evolution 74, 2617–2628. doi: 10.1111/evo.14087
Kemp D. J. (2002). Sexual selection constrained by life-history in a butterfly. Proc. R. Soc. B-Biological Sci. 269, 1341–1345. doi: 10.1098/rspb.2002.2000
Kenagy G. J., Trombulak S. C. (1986). Size and function of mammalian testes in relation to body size. J. Mammalogy 67, 1–22. doi: 10.2307/1380997
King E. D. A., Banks P. B., Brooks R. C. (2013). Sexual conflict in mammals: consequences for mating systems and life-history. Mamm. Rev. 43, 47–58. doi: 10.1111/j.1365-2907.2011.00200.x
Kissling W. D., Dalby L., Fløjgaard C., Lenoir J., Sandel B., Sandom C., et al. (2014). Establishing macrocological trait datasets: digitization, extrapolation, and validation of diet preferences in terrestrial mammals worldwide. Ecol. Evol. 4, 2913–2930. doi: 10.1002/ece3.2014.4.issue-14
Kotrschal A., Corral-Lopez A., Zajitschek S., Immler S., Maklakov A. A., Kolm N. (2015). Positive genetic correlation between brain size and sexual traits in male guppies artificially selected for brain size. J. Evolutionary Biol. 28, 841–850. doi: 10.1111/jeb.2015.28.issue-4
Lailvaux S. P., Husak J. F. (2014). The life-history of whole-organism performance. Q. Rev. Biol. 89, 285–318. doi: 10.1086/678567
Lailvaux S. P., Husak J. F. (2017). Predicting life-history trade-offs with whole-organism performance. Integr. Comp. Biol. 57, 325–332. doi: 10.1093/icb/icx073
Lailvaux S. P., Irschick D. J. (2006). A functional perspective on sexual selection: insights and future prospects. Anim. Behav. 72, 263–273. doi: 10.1016/j.anbehav.2006.02.003
Lane J. E., Boutin S., Speakman J. R., Humphries M. M. (2010). Energetic costs of male reproduction in a scramble competition mating system. J. Anim. Ecol. 79, 27–34. doi: 10.1111/j.1365-2656.2009.01592.x
Laskowski K. L., Moiron M., Niemelä P. T. (2021). Integrating behavior in life-history theory: allocation versus acquisition? Trends Ecol. Evol. 36, 132–138. doi: 10.1016/j.tree.2020.10.017
Leigh S. R. (2004). Brain growth, life history, and cognition in primate and human evolution. Am. J. Primatology 62, 139–164. doi: 10.1002/ajp.20012
Lemaítre J. F., Ramm S. A., Barton R. A., Stockley P. (2009). Sperm competition and brain size evolution in mammals. J. Evolutionary Biol. 22, 2215–2221. doi: 10.1111/j.1420-9101.2009.01837.x
Lüpold S., de Boer R. A., Evans J. P., Fitzpatrick J. L. (2020). How sperm competition shapes the evolution of testes and sperm: a meta-analysis. Philos. Trans. R. Soc. B 375, 20200064. doi: 10.1098/rstb.2020.0064
Lüpold S., Simmons L. W., Grueter C. C. (2019). Sexual ornaments but not weapons trade off against testes size in primates. Proc. R. Soc. B-Biological Sci. 286, 20182524. doi: 10.1098/rspb.2018.2542
Mabry K. E., Shelley E. L., Davis K. E., Blumstein D. T., Van Vuren D. H. (2013). Social mating system and sex-biased dispersal in mammals and birds: a phylogenetic analysis. PloS One 8, e57980. doi: 10.1371/journal.pone.0057980
Maklakov A. A., Immler S., Gonzalez-Voyer A., Ronn J., Kolm N. (2011). Brains and the city: big-brained passerine birds succeed in urban environments. Biol. Lett. 7, 730–732. doi: 10.1098/rsbl.2011.0341
Miles D. B., Sinervo B., Frankino W. A. (2000). Reproductive burden, locomotor performance, and the cost of reproduction in free ranging lizards. Evolution 54, 1386–1395. doi: 10.1111/j.0014-3820.2000.tb00570.x
Millar N. P., Reznick D. N., Kinnison M. T., Hendry A. P. (2006). Disentangling the selective factors that act on male colour in wild guppies. Oikos 113, 1–12. doi: 10.1111/j.0030-1299.2006.14038.x
Moller A. P. (1991). Sperm competition, sperm depletion, paternal care, and relative testis size in birds. Am. Nat. 137, 882–906. doi: 10.1086/285199
Pélabon C., Larsen L. K., Bolstad G. H., Viken A. A., Fleming I. A., Rosenqvist G. (2014). The effects of sexual selection on life-history traits: an experimental study on guppies. J. Evolutionary Biol. 27, 406–414. doi: 10.1111/jeb.12309
Pérez-Barberia F. J., Shultz S., Dunbar R. I. M. (2007). Evidence for coevolution of sociality and relative brain size in three orders of mammals. Evolution 61, 2811–2821. doi: 10.1111/j.1558-5646.2007.00229.x
Pitnick S., Jones K. E., Wilkinson G. S. (2006). Mating system and brain size in bats. Proc. R. Soc. B-Biological Sci. 273, 719–724. doi: 10.1098/rspb.2005.3367
Pontzer H. (2016). A unified therory for the energy costs of legged locomotion. Biol. Lett. 12, 20150935. doi: 10.1098/rsbl.2015.0935
Promislow D. E. L., Harvey P. H. (1990). Living fast and dying young: a comparative analysis of life-history variation among mammals. J. Zoology 220, 417–437. doi: 10.1111/j.1469-7998.1990.tb04316.x
Ricklefs R. E., Wikelski M. (2002). The physiology/life-history nexus. Trends Ecol. Evol. 17, 462–468. doi: 10.1016/S0169-5347(02)02578-8
Ryan M. J., Tuttle M. D., Rand A. S. (1982). Bat predation and sexual advertisement in a neotropical anuran. Am. Nat. 119, 136–139. doi: 10.1086/283899
Shipley B. (2000). Cause and Correlation in Biology: A User’s Guide to Path Analysis, Structural Equations, and Causal Inference. (Cambridge, U.K: Cambridge University Press).
Shuster S. M., Wade M. J. (2003). Mating Systems and Strategies (Princeton: Princeton University Press).
Smeele S. Q., Conde D. A., Baudisch A., Brusland S., Iwaniuk A., Staerk J., et al. (2022). Coevolution of relative brain size and life expectancy in parrots. Proc. R. Soc. B-Biological Sci. 289, 20212397. doi: 10.1098/rspb.2021.2397
Sol D., Garcia N., Iwaniuk A., Davis K., Meade A., Boyle A., et al. (2010). Evolutionary divergence in brain size between migratory and resident birds. PloS One 5, e9617. doi: 10.1371/journal.pone.0009617
Song Z., Griesser M., Schuppli C., van Schaik C. P. (2023). Does the expensive brain hypothesis apply to amphibians and reptiles? BMC Ecol. Evol. 23, 77. doi: 10.1186/s12862-023-02188-w
Soulsbury C. D. (2019). Income and capital breeding in males: energetic and physiological limitations on male mating strategies. J. Exp. Biol. 222, jeb184895. doi: 10.1242/jeb.184895
Sowersby W., Eckerström-Liedholm S., Kotrschal A., Näslund J., Rowiński P. K., Gonzalez-Voyer A., et al. (2021). Fast life-histories are associated with larger brain size in killifishes. Evolution 75, 2286–2298. doi: 10.1111/evo.14310
Sowersby W., Eckerström-Liedholm S., Rowiński P. K., Balogh J., Eiler S., Upstone J. D., et al. (2022). The relative effects of pace of life-history and habitat characteristics on the evolution of sexual ornaments: a comparative reassessment. Evolution 73, 114–127. doi: 10.1111/evo.14358
Swanson E. M., Dantzer B. (2014). Insulin-like growth factor-1 is associated with life-history variation across Mammalia. Proc. R. Soc. B-Biological Sci. 281, 7. doi: 10.1098/rspb.2013.2458
Tarka M., Guenther A., Niemelä P. T., Nakagawa S., Noble D. W. A. (2018). Sex differences in life history, behavior, and physiology along a slow-fast continuum: a meta-analysis. Behav. Ecol. Sociobiology 72, 132. doi: 10.1007/s00265-018-2534-2
Team R. C. (2024). “R: A language and environment for statistical computing,” (R Foundation for Statistical Computing, Vienna, Austria). Available at: https://www.R-project.org/.
Trivers R. L. (1972). “Parental investment and sexual selection,” in Sexual Selection and the Descent of Man. Ed. Campbell B. (Aldine, Chicago), 136–179.
Tucker V. A. (1970). Energetic cost of locomotion in animals. Comp. Biochem. Physiol. 24, 841–846. doi: 10.1016/0010-406X(70)91006-6
Van der Bijl W. (2018). phylopath: Easy phylogenetic path analysis in R. PeerJ 6, e4718. doi: 10.7717/peerj.4718
van Noordwijk A. J., De Jong G. (1986). Acquisition and allocation of resources: their influence on variation in life history tactics. Am. Nat. 128, 137–142. doi: 10.1086/284547
Vasilieva N. A. (2022). Pace-of-life syndrome (POLS): evolution of a concept. Biol. Bull. 47, 750–762. doi: 10.1134/S1062359022070238
von Hardenberg A., Gonzalez-Voyer A. (2013). Disentangling evolutionary cause-effect relationships within a phylogenetic confirmatory path analysis. Evolution 67, 378–387. doi: 10.1111/j.1558-5646.2012.01790.x
Keywords: life history, locomotion, performance, sexual selection, tradeoff
Citation: Husak JF, Sorlin MV and Lailvaux SP (2024) Counting the costs of expensive tissues: mating system, brain size, and IGF-1 affect the ecological costs of transport in mammals. Front. Ethol. 3:1464308. doi: 10.3389/fetho.2024.1464308
Received: 13 July 2024; Accepted: 25 September 2024;
Published: 10 October 2024.
Edited by:
Susanne R.K. Zajitschek, Liverpool John Moores University, United KingdomReviewed by:
Arnaud Badiane, UMR5242 Institut de Génomique Fonctionnelle de Lyon (IGFL), FranceCopyright © 2024 Husak, Sorlin and Lailvaux. This is an open-access article distributed under the terms of the Creative Commons Attribution License (CC BY). The use, distribution or reproduction in other forums is permitted, provided the original author(s) and the copyright owner(s) are credited and that the original publication in this journal is cited, in accordance with accepted academic practice. No use, distribution or reproduction is permitted which does not comply with these terms.
*Correspondence: Jerry F. Husak, amVycnkuaHVzYWtAc3R0aG9tYXMuZWR1
Disclaimer: All claims expressed in this article are solely those of the authors and do not necessarily represent those of their affiliated organizations, or those of the publisher, the editors and the reviewers. Any product that may be evaluated in this article or claim that may be made by its manufacturer is not guaranteed or endorsed by the publisher.
Research integrity at Frontiers
Learn more about the work of our research integrity team to safeguard the quality of each article we publish.