- Department of Psychology, Emory University, Atlanta, GA, United States
Despite the prevalence of large group-living in the animal kingdom, we know surprisingly little about how the brain facilitates grouping behavior, particularly in mammals. In this brief communication, I provide an update on advancements in the study of the neural mechanisms underlying mammalian grouping behavior. I discuss the benefits of using non-traditional organisms in the laboratory and provide examples of how using non-standard, large housing and testing apparatuses produces more ethologically-relevant behavioral datasets. Further, with advancements in computer vision-based automated tracking and increasing availability of wireless neural recording and manipulation tools, scientists can now generate unprecedented neurobehavioral datasets from multiple interacting animals. Together, recent advancements in behavioral and neural approaches hold great promise for expanding our understanding of how the brain modulates complex, mammalian grouping behaviors.
Introduction
Our understanding of how animals behave in groups has largely been garnered from studies conducted in the field. Such field studies have arguably revealed the most about collective behaviors. Collective behavior is commonly defined as the emergence of cohesive movements in groups of animals in the apparent absence of centralized control where individuals in the group act based on limited local information (i.e., physical movements or pheromonal cues from other group members), which flows through the group producing collective patterns (Couzin and Krause, 2003; Giardina, 2008). Examples of well-studied collective behaviors include migration (Shellard and Mayor, 2020; Aikens et al., 2022) and coordinated motion of flocks of birds and schools of fish (Couzin et al., 2002; Dieck Kattas et al., 2012; Jolles et al., 2017; Ribeiro et al., 2022). However, grouping behavior can be broadly defined as any type of behavior that occurs in groups and can focus not only on the group as a whole unit but also on individuals within the group (Krause and Ruxton, 2002; Majolo and Huang, 2022). Behaviors that occur in groups may also occur in non-group environments, such as foraging, which can occur alone or with others, or huddling which can occur in dyadic or large-group interactions (Gobrogge and Wang, 2015; Ding et al., 2020; Zhao et al., 2023). Importantly, though, the social context of being in a group may be quite distinct from that of the social context of being with just one other individual. Variation in social context can in turn influence the consequences of and/or motivations for particular actions, and thus may be regulated via different mechanisms in the brain. Indeed, just as a social context can elicit neural responses distinct from a non-social context (Gonzalez Abreu et al., 2022; Rodriguez-Santiago et al., 2022), so too can interactions with or exposure to a singular individual compared to a group of individuals (Rose et al., 2021).
We know a fair amount about the neural mechanisms underlying flocking behavior in birds (Goodson et al., 2012; Stevenson et al., 2020), and a few recent studies have begun to examine the neural mechanisms underlying grouping behavior in fish and insects (Tang et al., 2020; Messina et al., 2022; Homberg and Pfeiffer, 2023). However, we know little about how the brain modulates mammalian grouping behavior, likely due to issues concerning the feasibility of keeping large groups of non-human mammals in a laboratory setting. In this brief communication, I provide an update on advancements in the study of the neural mechanisms underlying mammalian grouping behavior ranging from the use of non-traditional organisms in the lab to the availability of new technology that is helping to propel this area of study forward.
Expansion of group-living mammals used in laboratory studies
Wild Norway rats (Rattus norvegicus) live in large colonies of roughly 150 individuals, with colonies typically consisting of several subgroups (Calhoun, 1962b; Calhoun, 1979; De Boer et al., 2016; Schweinfurth, 2020). Although the behavioral ecology of wild Norway rats includes large group-living, only a few studies in the past group-housed rats to explore aspects of grouping behavior (Calhoun, 1962a; Calhoun, 1962b; Blanchard et al., 1984a; Blanchard and Blanchard, 1989), and in recent decades domesticated rat strains used for neuroscience studies (i.e., Listar Hooded, Lewis, Fischer 344, Wistar, Sprague Dawley, Long Evans) are conventionally housed in dyads and sometimes alone (Makowska and Weary, 2016a; Skinner et al., 2019; Ratuski and Weary, 2022). Depending on the strain of domesticated rat, one sex may exhibit intense aggression toward same-sex conspecifics (Blanchard et al., 1984b; Kondrakiewicz et al., 2018), and thus examination of mixed-sex grouping behavior is often not feasible. Even in wild Norway rats, subgroups within colonies that are comprised of mixed-sex individuals typically contain only one male (Schweinfurth, 2020). This aside, while domesticated or wild rats could be used for studying same-sex grouping behavior, in the last ~30 years, rats have rarely been used for such studies possibly due to size-related constraints on housing.
Wild mice (Mus musculus) live in small family-groups, with males exhibiting strong territoriality but females being able to live peacefully with a few other adult females (Crowcroft, 1955; Anderson, 1961; Butler, 1980; Chambers et al., 2000). With the commercial availability of a variety of strains of laboratory mice, wild mice are not commonly used in social neuroscience studies. Laboratory mice often exhibit far more intense aggression than laboratory rats, particularly toward novel conspecifics (Vanoortmerssen, 1971; Baumans, 2004). Additionally, there is high amount of inter-strain variability in aggressiveness (Guillot and Chapouthier, 1996; Kondrakiewicz et al., 2018). However, some research groups successfully house same-sex groups of mice to examine dominance hierarchies. For example, ICR mice (Harlan Laboratories, Jerusalem, Israel) have been housed in groups of 16 adult males (Shemesh et al., 2014) and CD1 mice (Charles River, United States) have been housed in groups of 30 adult males (Williamson et al., 2016). Such studies in all-male groups of varying strains of laboratory mice can shed light on how the brain modulates social network status and dominance (So et al., 2015; Williamson et al., 2016), however, how behavior in these contexts may relate to natural behavior of wild mice, which are highly territorial and do not congregate in groups of 16+ males, is unclear. Although all-male mouse groups are used for studies of social networks and dominance hierarchies, some researchers have formed all-female groups (n = ~8 mice per group) of C57/BL6 mice to examine the influence of social isolation vs. group housing on cortical developmental and decision-making; interestingly, the authors stated in their paper that they were unable to test males because they cannot be housed together due to intense aggression (Hinton et al., 2019). Together, the study of grouping behavior in laboratory mice is likely only feasible in some strains and in only one sex.
Importantly, many scientists are not restricted to using laboratory rats and mice for social neuroscience experiments. An example of a non-traditional rodent that is used for studying the neural mechanisms of female grouping behavior is the meadow vole (Microtus pennsylvanicus). Adult meadow voles are solitary during the reproductive season (spring/summer) but aggregate in groups in the winter, with females forming multiple attachments to same-sex conspecifics that are both kin and non-kin (Beery et al., 2009). By manipulating photoperiod in the lab to mimic winter day length, studies in female meadow voles have elucidated neuroendocrinological underpinning of same-sex peer bonds (Beery, 2019). Use of this species also allows for the study of neural/behavioral flexibility given the seasonal shifts of meadow vole grouping.
An animal that is often featured on natural history television shows for their fantastic grouping behaviors including migration, foraging, food sharing, complex communication, and impressive memory for social and nonsocial acoustics, is the bat. A few research groups around the world use Egyptian fruit bats (Rousettus aegyptiacus) to examine the neural mechanisms of grouping behavior. Wild Egyptian fruit bats can be found in colonies ranging from 50 to thousands of individuals comprised of mixed-sex and mixed genetic relation (Kolodny et al., 2019; Bachorec et al., 2020). Studies using Egyptian fruit bats have identified social place cells in the hippocampus (Omer et al., 2018), and demonstrated that the hippocampus and cortex represent details of social interactions, including identity, context, shared spatial locations, and interaction history in freely interacting bat groups (Rose et al., 2021; Forli and Yartsev, 2023). While neuroscientists using Egyptian fruit bats are arguably producing the most [neuro]scientifically elegant datasets about grouping behavior, obtaining bats and maintaining breeding colonies can be difficult depending on regional and university restrictions.
Naked mole-rats (Heterocephalus glaber) are an excellent model for studying mechanisms of grouping behavior in the lab. This unusual, eusocial rodent lives entirely subterranean and has a proclivity for living in large numbers – numbers so large that naked mole rats have evolved a unique physiology that is capable of tolerating extremely high levels of carbon dioxide and ammonia and low levels of oxygen (Larson and Park, 2009; Lavinka et al., 2009; Edrey et al., 2011). Naked mole-rats are small (28-57 grams) and can be housed in groups of ~50 in the lab (Ragland et al., 2022). Neuroscience studies examining grouping behaviors in naked mole-rats have examined the neuroendocrine regulation of pubertal suppression in colonies (Peragine et al., 2017; Faykoo-Martinez et al., 2021) and shown that the brain differentially responds to conspecifics dependent on the subcaste of the individual (Hathaway et al., 2016). A recent study demonstrated that there is cultural transmission of vocal dialect in naked mole-rats (Barker et al., 2021), and ongoing studies by this research group are examining neural circuitry of vocal communication using in vivo calcium imaging and electrophysiology.
The introduction of wild-derived, communally breeding rodents to the lab provides an exciting avenue for studying the neural mechanisms of mammalian grouping behavior in both sexes. Communal breeders are characterized by the shared care of offspring, typically by multiple females, but in some species by multiple females and males (Weidt et al., 2014; Riehl, 2021; Ma et al., 2022); depending on the species, shared care of offspring occurs between kin and non-kin individuals (Weidt et al., 2014; Tuckova et al., 2016). Common degus (Octodon degus) are a medium-sized rodent (170-300g) endemic to Chile that live in groups of 1-5 males and 1-8 females in the wild (Ebensperger et al., 2004; Ebensperger et al., 2011). Field studies suggest that social groups are unstable with high turnover of group members (Ebensperger et al., 2009). Degus have been successfully maintained in laboratories for studies examining stress endocrinology and circadian rhythms (Hummer et al., 2007; Bauer et al., 2016; Bauer et al., 2019), as well as for studies examining aspects of social motivation and social learning in dyads (Lidhar et al., 2017; Lidhar et al., 2021). Degus have been proposed as a useful model for social neuroscience research (Colonnello et al., 2011), however, to our knowledge very few studies have used degus for such purposes, and it appears that degus in laboratory research are currently primarily being used as a model for Alzheimer’s disease (Tan et al., 2022). Given that there is a rich behavioral ecology on this species thanks to years of field studies, particularly about grouping behaviors (Ebensperger and Wallem, 2002; Wey et al., 2013), this lab-amenable rodent holds promise for studying how the brain facilitates various aspects of group-living.
In my lab, we use spiny mice (Acomys cahirinus), which are a communally breeding rodent native to Africa, the Middle East, and southern Asia (Nowak, 1999; Deacon, 2009; Frynta et al., 2011). Spiny mice (roughly 1/3 the size of a degu) readily breed in the lab and can be housed in adult groups of ~30 adult spiny mice (Haughton et al., 2016), allowing for the study of various grouping behaviors. Initial studies from my lab show that spiny mice exhibit a preference to affiliate with large over small groups of conspecifics, are highly affiliative, and exhibit little aggression in multiple social contexts (Fricker et al., 2021; Gonzalez Abreu et al., 2022). This high degree of sociality was also demonstrated in a study that found that established spiny mouse breeding groups will not only display low levels of aggression to unrelated newcomers, but also accept them into the group, which is uncommon in rodents (Cizkova et al., 2011). Adult females indiscriminately care for young pups, regardless of genetic relation (Tuckova et al., 2016); in our lab, we have also observed indiscriminate paternal care among adolescent and adult males (Kelly et al. unpub obs). Spiny mice are therefore ideally suited for the study of the neural mechanisms underlying various mammalian grouping behaviors. However, this species has only recently been used for neuroscience studies. Thus far, my lab has characterized basic social behaviors (Fricker et al., 2021), mapped social neural circuitry (Kelly and Seifert, 2021; Powell et al., 2022), and developed/validated the use of state-of-the-art technology (i.e., chemogenetic technology, functional genetic approaches, and fiber photometry) in spiny mice. We have shown that the hypothalamus may gate social reward in nonreproductive contexts via influences on reward circuitry (Gonzalez Abreu et al., 2022), found that the lateral septum differentially processes kin from non-kin, and identified neural circuits that facilitate the preference to affiliate with large groups (Kelly et al. unpub obs).
The ability to study affiliation in a group context in a species that naturally lives in successful, complex large groups without the need for pharmacological-induction or lab-induction (i.e., training or conditioning) can generate insight into how brains naturally evolved to facilitate large group-living. If we are to truly understand the evolution of social behavior, we need to consider the fact that various aspects of social behavior evolved independently many times. Thus, we cannot assume that relevant mechanisms have evolved similarly in all species. To build a solid foundation on which we study grouping behavior and relevant underlying mechanisms, it is important to examine a diversity of species within and across taxa. Doing so will ultimately help us determine the fundamental neural principles associated with the organization of particular social behaviors and how they evolved in relation to the behavioral ecology of an organism.
Room to breathe: Moving beyond small testing apparatuses and housing
Perhaps the most famous laboratory experiments on rodent grouping behavior were conducted by John B. Calhoun, an ethologist at the United States National Institutes of Mental Health in the mid 1900s. Calhoun created a room-sized pen to examine the growth and stability of a wild Norway rat population, which grew to 200 individuals but eventually stabilized at 150 individuals after roughly 2 years (Calhoun, 1962b). He later conducted another large group experiment in domesticated Norway rats using a converted barn that led to his findings of a “behavioral sink” in which rats voluntarily overcrowded in an area, despite negative outcomes, due to initial involuntary overcrowding (Calhoun, 1962a). These experiments resulted in high mortality rates and likely would not be considered ethical to conduct today. Yet, Calhoun’s use of large spaces was innovative and allowed for the study of naturalistic grouping behavior. As I write this, eyeing the neighbor’s barn down the road, I begrudgingly must admit that it is simply not feasible for most scientists to convert a barn into a behavioral testing room. Further, when the goal is to examine neural mechanisms of grouping behavior, there are limits on how many animals we can track and/or manipulate. However, we can certainly move beyond conventional “shoebox” rodent housing and use/create larger housing apparatuses as well as examine social interactions in large arenas rather than small cages.
Several labs have been very thoughtful and creative in designing housing and testing apparatuses for rodents. The visible burrow system, developed by Martha McClintock and Norman Adler to study sociosexual behavior (Mcclintock and Adler, 1978) and later popularized by Robert and Caroline Blanchard for studying social stress (Blanchard and Blanchard, 1989; Blanchard et al., 1995), has made a resurgence in the literature and has been used to examine group social stress, dominance, and social hierarchies in mice (Bove et al., 2018) and rats (Buwalda et al., 2017; Melhorn et al., 2017). Visible burrow systems vary in design, but are typically single-floored, large chambers that contain multiple compartments connected by tubes. With transparent Plexiglas covers/lids, top-down video recording can allow researchers to track animals and their locations. Because there are multiple compartments for animals to occupy, for rats and mice, males and females can be safely housed together to mimic a natural burrow system. Visible burrow systems can be made in-house with relative ease but are also available for purchase through companies such as Conduct Science (Stokie, Illinois, USA).
Custom vivariums made of metal and Plexiglas that comprise multiple floors connected by ladder systems have also been used for housing groups of mice to examine social networks (So et al., 2015). Such vivariums can be designed to fit on racks that typically hold rodent cages, and thus can be more easily accommodated in animal housing facilities and cleaned via industrial cage wash systems (a requirement for some institutions). Affordable alternatives are Critter Nation large cages available for purchase from companies such as MidWest Homes for Pets (Muncie, Indiana, USA). Critter Nation cages for small animals include wire cages with 2 levels (90cm L X 60cm W X 99cm H) or 4 levels (90cm L X 60cm W X 160cm H) on frames with wheels. Critter Nation cages have been successfully used to house rats in laboratories (Makowska and Weary, 2016a). Although multi-floored housing may mimic more naturalistic environments for rodents, it does not allow for top-down video recording and thus is not condusive for experiments that require continuous behavioral tracking.
The use of large arenas for housing or behavioral testing allows for top-down behavioral video recording amenable for behavioral tracking via computer vision. One research group housed groups of 16 male ICR mice in a complex cage, similar to those described above, during adolescence, and at adulthood moved groups of 4 mice into large arenas (70cm L X 50cm W X 50cm H) for behavioral testing and continuous video recording for 4 days (Shemesh et al., 2014). For testing spiny mice in group interaction tests, we use a large arena (120cm L X 120cm W X 60cm H) that contains transparent tubes and shelters so that all individuals can be continuously tracked (Figure 1). Large arenas, accompanied by enrichment available at pet stores, are an economical option for testing grouping behaviors and can be easily built to a size appropriate for various small mammals.
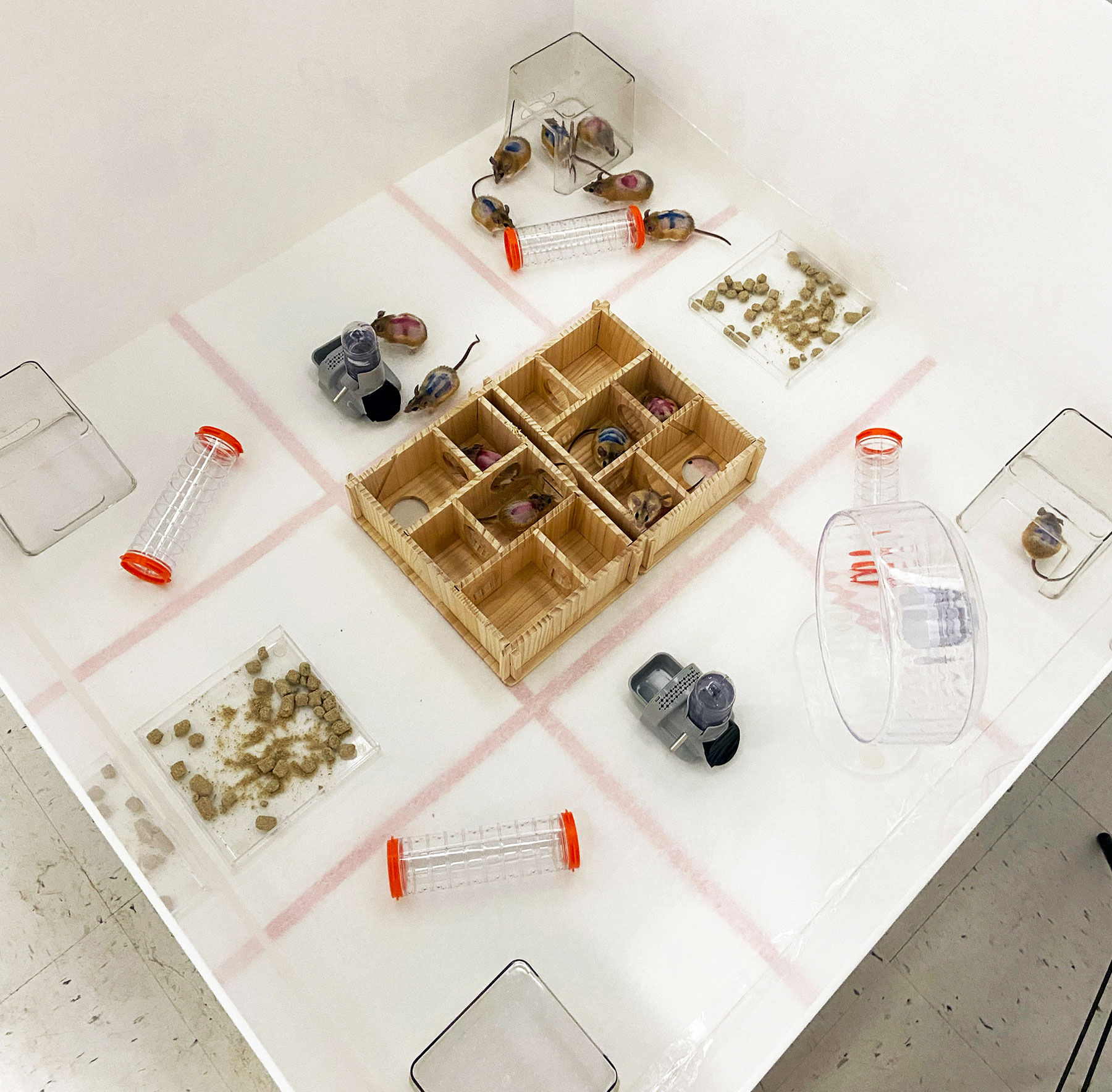
Figure 1 An example of a group interaction test conducted in a large Plexiglas arena (120cm L X 120cm W X 60cm H). 14 adult spiny mice with individual color markings on their backs interact amidst enrichment including transparent shelters and tubes, a running wheel, rodent mazes, and ad libitum food and water. A large space gives individuals the option to group or remain solitary (e.g., see mouse alone under shelter on the right side of the chamber). This setup enables top-down video recording amenable to computer vision-based automated tracking.
When animals are given space, their behavior typically changes from what we observe when they are tested or housed in small apparatuses. Rats and mice housed in visible burrow systems exhibit changes in circulating cortisol levels, circadian rhythmicity, neural plasticity, and feeding and drinking (Mckittrick et al., 2000; Melhorn et al., 2010; Lee et al., 2018). Rats housed in large Critter Nation cages exhibit differences in anticipatory behavior compared to rats housed in standard shoebox cages (Makowska and Weary, 2016a). Additionally, housing rats in large cages allows them to exhibit more naturalistic behaviors such as standing upright (Makowska and Weary, 2016b) and can have protective and neurorehabilitative effects (Kentner, 2015). Even with just two animals, when given a larger arena for interacting with another conspecific, researchers can more readily detect nuanced behaviors such as social vigilance (Williams et al., 2020). In spiny mice, we observe virtually no aggression when up to a dozen novel conspecifics are placed in a medium-size chamber (60cm L X 30cm W X 40cm H) with a single transparent shelter for an hour. However, when placed in a large arena (120cm L X 120cm W X 60cm H; see Figure 1), we observe chasing behavior within the first 10 min of group interactions (Kelly et al. unpub obs). While this seems counterintuitive – after all, with more space, spiny mice could avoid conspecifics if desired – it is possible that using a more semi-naturalistic environment with multiple resources to compete over elicits a behavioral repertoire that simply cannot emerge in simplistic environments. By using large cages/vivariums/arenas we can more feasibly house and test groups of animals together and provide them with space and resources akin to semi-naturalistic environments that are likely to produce ethologically-relevant behaviors in the laboratory.
Computer vision and continuous tracking
An exciting technological advancement in the field of animal behavior is the enhancement of animal tracking software. Novel machine learning systems such as TRex (Walter and Couzin, 2021) and SLEAP [Social LEAP Estimates Animal Poses; (Pereira et al., 2022)] are capable of tracking large groups of animals to determine the locations of multiple individuals in space. These systems are publicly available in relatively user-friendly formats that require minimal knowledge of coding. Further, more nuanced information about the types of behavior animals are engaging in (i.e., investigation, huddling, following, biting, allogrooming, etc.) can be acquired with additional computational frameworks. SimBA (Simple Behavioral Analysis; (Nilsson et al., 2020)) was created to study complex social behavior in freely moving animals. This open-source package allows for supervised machine learning and has been successfully used to identify complex social behavior during interactions between one or two rodents in a manner that yields high resolution and accuracies that out-perform human observers. To my knowledge, supervised machine learning predictive classifiers of group rodent social behavior have yet to be generated, but the tools are available for such analyses. Regardless, the ability to continuously track multiple animals to obtain location coordinates and distinct visual fields for specific individuals [see (Walter and Couzin, 2021)] can allow for rapid processing of countless hours of data needed to quantify social networks of groups. Animal tracking has recently been used to record small groups of mice over long periods of time and showed that group behavior arises from individuality, pairwise, and higher-order interactions between group members (Shemesh et al., 2014). Another group that used a custom computer vision-based automatic tracking system quantified 36 weeks-worth of continuous behavioral recordings in groups of 7 rats to examine shifts in group structure over time (Nagy et al., 2023). Such studies can generate unprecedented behavioral datasets that represent both individuals within a large group as well as the collective behavior of the group as a whole. Using such methods will not only save countless hours of manual labor scoring behavioral videos of group interactions but will also produce behavioral datasets that are more consistent and contain less bias than those collected by human observers. Notably, although computer vision and continuous tracking methods are exciting and promising, there are currently limitations to effectively using this technology. For example, humans are still needed for model tuning to avoid switching the identities of individuals in videos (Han et al., 2022; Pereira et al., 2022; Chen et al., 2023); identity swapping can occur with only two rodents, and this problem amplifies with a group of rodents. Using a combination of tools such as computer vision and RFID-based systems can help to overcome these complications (Fong et al., 2023) as the technology gradually advances.
Wireless neural recording and manipulation
Although wired neural recording and manipulation technologies (i.e., electrophysiology and optogenetics) have been widely available for well over a decade (particularly for electrophysiology) (Bullock, 1959; Boyden, 2011), in the field of animal behavior, a long-standing problem with using such tools has been the need to have subjects connected to cables for recording or manipulation. Not only does being tethered to cables alter an animal’s movements, but trying to observe more than two rodents freely interacting typically results in the non-focal animal, and sometimes the subject themselves, playing with or chewing on the cables. Further, if trying to record from more than one animal at a time, cables simply get tangled together. Thankfully, in recent years, wireless versions of these tools have become commercially available, even in a variation where both wireless electrophysiology and optogenetics are in the same multimodal platform (Bilodeau et al., 2021). Studies in Egyptian fruit bats have used wireless electrophysiology to determine that in a small group setting (group size = 4) vocal interactions elicit stable correlated neural activity across the brains of all group members, and that single neuron activity in the frontal cortex exhibits selectivity for the calls of specific individuals (Rose et al., 2021). Another study in small sibling groups of transgenic mice (group size = 4) used wireless optogenetics to stimulate activation of oxytocin neurons in the paraventricular nucleus of the hypothalamus and found that stimulation of oxytocin release dynamically increased both pro- and anti-social behaviors (Anpilov et al., 2020). Wireless optogenetic devices are available in head-mounted and back-mounted devices, expanding the options for use in non-traditional rodents that vary in size compared to a domesticated rat or mouse (Yang et al., 2021). In addition to wireless electrophysiology and optogenetics, photometry approaches are becoming more widely incorporated into behavioral neuroscience studies, including in laboratories that use non-traditional rodents (Murphy et al., 2023). Fiber photometry is a user-friendly and flexible method that detects changes in fluorescence as a proxy marker for rapid molecular changes in the brain (Gunaydin et al., 2014). This technology has recently become available in a wireless version from the company Amuza Inc. (San Diego, California, USA). The efficacy of wireless fiber photometry has been demonstrated in freely behaving individual mice (Cardenas et al., 2021), and can be scaled to record simultaneously from 8 individuals that each have their own headstage and receiver, and thus have unique transmission channels. Such advancements in recording technology enable the real-time examination of neural activity in a manner in which animals are unencumbered by cables as they freely interact in group settings.
Chemogenetic technology and functional genetic approaches are also amenable for neuromanipulative studies examining grouping behavior, albeit with less temporal resolution. Such tools typically involve a one-time viral injection into a brain region(s) of choice and do not require an implant, thus likely resulting in more naturalistic behavior from animals. Chemogenetic tools such as Designer Receptors Exclusively Activated by Designer Drugs (DREADDs) enable manipulation of neuronal and non-neuronal signal transduction in freely moving animals (Roth, 2016) and have been successfully used in non-traditional models including voles (Li et al., 2021) and spiny mice (Kelly et al. unpub obs). DREADDs can be used to determine how individual brain regions or neural circuits contribute to any behavior of interest. Further, gene-editing approaches such as CRISPR/Cas9 strategies can allow for targeted knockdown or overexpression of specific cell types in distinct brain regions. An adeno-associated virus (AAV) based strategy to deliver CRISPR/Cas9 components to adult brain tissue to reduce oxytocin receptor protein levels in vivo was recently validated in prairie voles, golden hamsters, spiny mice, house mice, California mice, and Norway rats (Boender et al., 2023). Such tools can be used to determine the contributions of specific genes to behavior. Because chemogenetic and functional genetic approaches do not require animals to be tethered to cables to manipulate the brain, these tools can readily be used to examine neural mechanisms underlying grouping behaviors.
Conclusions
Due to an expansion of non-traditional species used in laboratory settings, in recent years more options have become available for examining how the brain facilitates a variety of grouping behaviors. By designing larger housing and testing chambers we can create semi-naturalistic environments in the laboratory, allowing groups of animals to exhibit more ethologically-relevant behaviors. Further, with advancements in computer vision-based automated tracking, scientists can now generate unprecedented behavioral datasets obtained from long-term studies of multiple interacting animals. Lastly, the increasing availability of wireless neural recording and manipulation tools allows for testing animals in group settings in a manner in which they can exhibit more naturalistic behaviors due to a lack of cables connected to head implants. Together, these approaches hold great promise for expanding our understanding of how the brain modulates complex, mammalian grouping behaviors.
Data availability statement
The original contributions presented in the study are included in the article/supplementary material. Further inquiries can be directed to the corresponding author.
Author contributions
AK: Conceptualization, Funding acquisition, Writing – original draft, Writing – review & editing.
Funding
The author declare financial support was received for the research, authorship, and/or publication of this article. This work was supported by the National Science Foundation (IOS-2310626 to AK).
Conflict of interest
The author declares that the research was conducted in the absence of any commercial or financial relationships that could be construed as a potential conflict of interest.
The author AK declared that they were an editorial board member of Frontiers, at the time of submission. This had no impact on the peer review process and the final decision.
Publisher’s note
All claims expressed in this article are solely those of the authors and do not necessarily represent those of their affiliated organizations, or those of the publisher, the editors and the reviewers. Any product that may be evaluated in this article, or claim that may be made by its manufacturer, is not guaranteed or endorsed by the publisher.
References
Aikens E. O., Bontekoe I. D., Blumenstiel L., Schlicksupp A., Flack A. (2022). Viewing animal migration through a social lens. Trends Ecol. Evol. 37, 985–996. doi: 10.1016/j.tree.2022.06.008
Anderson P. K. (1961). Density, social structure, and nonsocial environment in mouse-house populations and the implications for regulation of numbers. Trans. New York Acad. Sci. 23, 447–451.
Anpilov S., Shemesh Y., Eren N., Harony-Nicolas H., Benjamin A., Dine J., et al. (2020). Wireless optogenetic stimulation of oxytocin neurons in a semi-natural setup dynamically elevates both pro-social and agonistic behaviors. Neuron 107, 644–655.e647. doi: 10.1016/j.neuron.2020.05.028
Bachorec E., Horacek I., Hulva P., Konecny A., Lucan R. K., Jedlicka P., et al. (2020). Spatial networks differ when food supply changes: Foraging strategy of Egyptian fruit bats. PloS One 15, e0229110. doi: 10.1371/journal.pone.0229110
Barker A. J., Veviurko G., Bennett N. C., Hart D. W., Mograby L., Lewin G. R. (2021). Cultural transmission of vocal dialect in the naked mole-rat. Science 371, 503–507. doi: 10.1126/science.abc6588
Bauer C. M., Correa L. A., Ebensperger L. A., Romero L. M. (2019). Stress, sleep, and sex: A review of endocrinological research in Octodon degus. Gen. Comp. Endocrinol. 273, 11–19. doi: 10.1016/j.ygcen.2018.03.014
Bauer C. M., Ebensperger L. A., Leon C., Ramirez-Estrada J., Hayes L. D., Romero L. M. (2016). Postnatal development of the degu (Octodon degus) endocrine stress response is affected by maternal care. J. Exp. Zool A Ecol. Genet. Physiol. 325, 304–317. doi: 10.1002/jez.2018
Baumans V. (2004). Preference for social contact vs environmental enrichment in male laboratory mice. Lab. Anim. 38, 178–188.
Beery A. K. (2019). Frank Beach award winner: Neuroendocrinology of group living. Horm Behav. 107, 67–75. doi: 10.1016/j.yhbeh.2018.11.002
Beery A. K., Routman D. M., Zucker I. (2009). Same-sex social behavior in meadow voles: Multiple and rapid formation of attachments. Physiol. Behav. 97, 52–57. doi: 10.1016/j.physbeh.2009.01.020
Bilodeau G., Gagnon-Turcotte G., Gagnon L. L., Keramidis I., Timofeev I., De Koninck Y., et al. (2021). A wireless electro-optic platform for multimodal electrophysiology and optogenetics in freely moving rodents. Front. Neurosci. 15. doi: 10.3389/fnins.2021.718478
Blanchard R. J., Blanchard D. C. (1989). Antipredator defensive behaviors in a visible burrow system. J. Comp. Psychol. 103, 70–82. doi: 10.1037/0735-7036.103.1.70
Blanchard R. J., Flannelly K. J., Layng M., Blanchard D. C. (1984b). The effects of age and strain on aggression in male rats. Physiol. Behav. 33, 857–861. doi: 10.1016/0031-9384(84)90219-1
Blanchard D. C., Fukunaga-Stinson C., Takahashi L. K., Flannelly K. J., Blanchard R. J. (1984a). Dominance and aggression in social groups of male and female rats. Behav. Processes 9, 31–48. doi: 10.1016/0376-6357(84)90006-8
Blanchard D. C., Spencer R. L., Weiss S. M., Blanchard R. J., Mcewen B., Sakai R. R. (1995). Visible burrow system as a model of chronic social stress: behavioral and neuroendocrine correlates. Psychoneuroendocrinol. 20, 117–134. doi: 10.1016/0306-4530(94)e0045-b
Boender A. J., Boon M., Albers H. E., Eck S. R., Fricker B. A., Kelly A. M., et al. (2023). An AAV-CRISPR/Cas9 strategy for gene editing across divergent rodent species: Targeting neural oxytocin receptors as a proof of concept. Sci. Adv. 9, eadf4950. doi: 10.1126/sciadv.adf4950
Bove M., Ike K., Eldering A., Buwalda B., De Boer S. F., Morgese M. G., et al. (2018). The Visible Burrow System: A behavioral paradigm to assess sociability and social withdrawal in BTBR and C57BL/6J mice strains. Behav. Brain Res. 344, 9–19. doi: 10.1016/j.bbr.2018.02.003
Boyden E. S. (2011). A history of optogenetics: the development of tools for controlling brain circuits with light. F1000 Biol. Rep. 3, 11. doi: 10.3410/B3-11
Bullock T. H. (1959). Neuron doctrine and electrophysiology. Science 129, 997–1002. doi: 10.1126/science.129.3355.997
Butler R. G. (1980). Population size, social behaviour, and dispersal in house mice: a quantitative investigation. Anim Behav. 28, 78–85.
Buwalda B., Koolhaas J. M., De Boer S. F. (2017). Trait aggressiveness does not predict social dominance of rats in the Visible Burrow System. Physiol. Behav. 178, 134–143. doi: 10.1016/j.physbeh.2017.01.008
Calhoun J. B. (1962a). “A behavioral sink,” in Roots of Behavior. Ed. Biss E. L. (New York: Harper).
Calhoun J. B. (1979). The Ecology and Sociobiology of the Norway Rat Bethesda (U.S. Dept. of Health, Education, and Welfare, Public Health Service). doi: 10.5962/bhl.title.112283
Cardenas A., Papadogiannis A., Dimitrov E. (2021). The role of medial prefrontal cortex projections to locus ceruleus in mediating the sex differences in behavior in mice with inflammatory pain. FASEB J. 35, e21747. doi: 10.1096/fj.202100319RR
Chambers L. K., Singleton G. R., Krebs C. J. (2000). Movements and social organization of wild house mice (Mus domesticus) in the wheatlands of northwestern Victoria, Australia. J. Mammal 81, 59–69.
Chen Z., Zhang R., Fang H. S., Zhang Y. E., Bal A., Zhou H., et al. (2023). AlphaTracker: a multi-animal tracking and behavioral analysis tool. Front. Behav. Neurosci. 17. doi: 10.3389/fnbeh.2023.1111908
Cizkova B., Sumbera R., Frynta D. (2011). A new member or an intruder: how do Sinai spiny mouse (Acomys dimidiatus) families respond to a male newcomer? Behaviour 148, 889–908.
Colonnello V., Iacobucci P., Fuchs T., Newberry R. C., Panksepp J. (2011). Octodon degus. A useful animal model for social-affective neuroscience research: basic description of separation distress, social attachments and play. Neurosci. Biobehav. Rev. 35, 1854–1863. doi: 10.1016/j.neubiorev.2011.03.014
Couzin I. D., Krause J. (2003). Self-organization and collective behavior in vertebrates. Adv. Stud. Behav. 32, 10–1016.
Couzin I. D., Krause J., James R., Ruxton G. D., Franks N. R. (2002). Collective memory and spatial sorting in animal groups. J. Theor. Biol. 218, 1–11. doi: 10.1006/jtbi.2002.3065
Crowcroft P. (1955). Territoriality in wild house mice, Mus musculus. J. Mammal 36, 299–301. doi: 10.2307/1375908
Deacon R. M. (2009). Burrowing: a sensitive behavioural assay, tested in 5 species of laboratory rodents. Behav. Brain Res. 200, 128–133.
De Boer S. F., Buwalda B., Koolhaas J. M. (2016). “Concepts, Cognition, Emotion, and Behavior: Handbook of Stress,” in Aggressive behavior and social stress, Concepts, Cognition, Emotion, and Behavior: Handbook of Stress (Elsevier Publishing Group).
Dieck Kattas G., Xu X. K., Small M. (2012). Dynamical modeling of collective behavior from pigeon flight data: flock cohesion and dispersion. PloS Comput. Biol. 8, e1002449. doi: 10.1371/journal.pcbi.1002449
Ding S. S., Muhle L. S., Brown A. E. X., Schumacher L. J., Endres R. G. (2020). Comparison of solitary and collective foraging strategies of Caenorhabditis elegans in patchy food distributions. Philos. Trans. R Soc. Lond B Biol. Sci. 375, 20190382. doi: 10.1098/rstb.2019.0382
Ebensperger L. A., Chesh A. S., Castro R. A., Tolhuysen L. O., Quirici V., Burger J. R., et al. (2009). Instability rules social groups in the communal breeder rodent Octodon degus. Ethology 115, 540–554.
Ebensperger L. A., Chesh A. S., Castro R. A., Tolhuysen L. O., Quirici V., Burger J. R., et al. (2011). Burrow limitations and group living in the communally rearing rodent, Octodon degus. J. Mammal 92, 21–30. doi: 10.1644/09-MAMM-S-383.1
Ebensperger L. A., Hurtado M. J., Soto-Gamboa M., Lacey E. A., Chang A. T. (2004). Communal nesting and kinship in degus (Octodon degus). Naturwissenschaften 91, 391–395.
Ebensperger L. A., Wallem P. K. (2002). Grouping increases the ability of the social rodent, Octodon degus, to detect predators when using exposed microhabitats. Oikos 98, 491–497.
Edrey Y. H., Park T. J., Kang H., Biney A., Buffenstein R. (2011). Endocrine function and neurobiology of the longest-living rodent, the naked mole-rat. Exp. Gerontol 46, 116–123. doi: 10.1016/j.exger.2010.09.005
Faykoo-Martinez M., Kalinowski L. M., Holmes M. M. (2021). Neuroendocrine regulation of pubertal suppression in the naked mole-rat: What we know and what comes next. Mol. Cell Endocrinol. 534, 111360. doi: 10.1016/j.mce.2021.111360
Fong T., Hu H., Gupta P., Jury B., Murphy T. H. (2023). PyMouseTracks: Flexible computer vision and RFID-based system for multiple mouse tracking and behavioral assessment. eNeuro 10(5). doi: 10.1523/ENEURO.0127-22.2023
Forli A., Yartsev M. M. (2023). Hippocampal representation during collective spatial behaviour in bats. Nature 621, 796–803. doi: 10.1038/s41586-023-06478-7
Fricker B. A., Seifert A. W., Kelly A. M. (2021). Characterization of social behavior in the spiny mouse, Acomys cahirinus. Ethology 00:1–15. doi: 10.1111/eth.13234
Frynta D., Frankova M., Cizkova B. (2011). Social and life history correlates of litter size in captive colonies of precocial spiny mice (Acomys). Acta Theriol 56, 289–295. doi: 10.1007/s13364-011-0024-2
Giardina I. (2008). Collective behavior in animal groups: theoretical models and empirical studies. HFSP J. 2, 205–219. doi: 10.2976/1.2961038
Gobrogge K., Wang Z. (2015). Neuropeptidergic regulation of pair-bonding and stress buffering: Lessons from voles. Horm Behav. 76, 91–105. doi: 10.1016/j.yhbeh.2015.08.010
Gonzalez Abreu J. A., Rosenberg A. E., Fricker B. A., Wallace K. J., Seifert A. W., Kelly A. M. (2022). Species-typical group size differentially influences social reward neural circuitry during nonreproductive social interactions. iScience 25 (5), 104230. doi: 10.1016/j.isci.2022.104230
Goodson J. L., Wilson L. C., Schrock S. E. (2012). To flock or fight: neurochemical signatures of divergent life histories in sparrows. Proc. Natl. Acad. Sci. U.S.A. 109 Suppl 1, 10685–10692. doi: 10.1073/pnas.1203394109
Guillot P., Chapouthier G. (1996). Intermale aggression and dark/light preference in ten inbred mouse strains. Behav. Brain Res. 77.
Gunaydin L. A., Grosenick L., Finkelstein J. C., Kauvar I. V., Fenno L. E., Adhikari A., et al. (2014). Natural neural projection dynamics underlying social behavior. Cell 157, 1535–1551. doi: 10.1016/j.cell.2014.05.017
Han Y., Huang K., Chen K., Pan H., Ju F., Long Y., et al. (2022). MouseVenue3D: A markerless three-dimension behavioral tracking system for matching two-photon brain imaging in free-moving mice. Neurosci. Bull. 38, 303–317. doi: 10.1007/s12264-021-00778-6
Hathaway G. A., Faykoo-Martinez M., Peragine D. E., Mooney S. J., Holmes M. M. (2016). Subcaste differences in neural activation suggest a prosocial role for oxytocin in eusocial naked mole-rats. Horm Behav. 79, 1–7. doi: 10.1016/j.yhbeh.2015.12.001
Haughton C. L., Gawriluk T. R., Seifert A. W. (2016). The biology and husbandry of the African Spiny Mouse (Acomys cahirinus) and the research uses of a laboratory colony. J. Am. Assoc. Lab. Anim. Sci. 55, 9–17.
Hinton E. A., Li D. C., Allen A. G., Gourley S. L. (2019). Social isolation in adolescence disrupts cortical development and goal-dependent decision-making in adulthood, despite social reintegration. eNeuro 6. doi: 10.1523/ENEURO.0318-19.2019
Homberg U., Pfeiffer K. (2023). Correction to: Unraveling the neural basis of spatial orientation in arthropods. J. Comp. Physiol. A Neuroethology sensory neural Behav. Physiol. 209, 465. doi: 10.1007/s00359-023-01655-5
Hummer D. L., Jechura T. J., Mahoney M. M., Lee T. M. (2007). Gonadal hormone effects on entrained and free-running circadian activity rhythms in the developing diurnal rodent Octodon degus. Am. J. Physiol. Regulatory Integr. Comp. Physiol. 292, R586–R597. doi: 10.1152/ajpregu.00043.2006
Jolles J. W., Boogert N. J., Sridhar V. H., Couzin I. D., Manica A. (2017). Consistent individual differences drive collective behavior and group functioning of schooling fish. Curr. Biol. 27, 2862–2868.e2867. doi: 10.1016/j.cub.2017.08.004
Kelly A. M., Seifert A. W. (2021). Distribution of vasopressin and oxytocin neurons in the basal forebrain and midbrain of spiny mice (Acomys cahirinus). Neuroscience 468, 16–28.
Kentner A. C. (2015). Neuroprotection and recovery from early-life adversity: considerations for environmental enrichment. Neural Regener. Res. 10, 1545–1547. doi: 10.4103/1673-5374.165315
Kolodny O., Weinberg M., Reshef L., Harten L., Hefetz A., Gophna U., et al. (2019). Coordinated change at the colony level in fruit bat fur microbiomes through time. Nat. Ecol. Evol. 3, 116–124. doi: 10.1038/s41559-018-0731-z
Kondrakiewicz K., Kostecki M., Szdzinska W., Knapska E. (2018). Ecological validity of social interaction tests in rats and mice. Genes Brain Behav. 18, e12525. doi: 10.1111/gbb.12525
Larson J., Park T. J. (2009). Extreme hypoxia tolerance of naked mole-rat brain. Neuroreport 20, 1634–1637. doi: 10.1097/WNR.0b013e32833370cf
Lavinka P. C., Brand A., Landau V. J., Wirtshafter D., Park T. J. (2009). Extreme tolerance to ammonia fumes in African naked mole-rats: animals that naturally lack neuropeptides from trigeminal chemosensory nerve fibers. J. Comp. Physiol. A Neuroethology sensory neural Behav. Physiol. 195, 419–427. doi: 10.1007/s00359-009-0420-0
Lee W., Yang E., Curley J. P. (2018). Foraging dynamics are associated with social status and context in mouse social hierarchies. PeerJ 6, e5617. doi: 10.7717/peerj.5617
Li L., Zhang L. Z., He Z. X., Ma H., Zhang Y. T., Xun Y. F., et al. (2021). Dorsal raphe nucleus to anterior cingulate cortex 5-HTergic neural circuit modulates consolation and sociability. Elife 10. doi: 10.7554/eLife.67638
Lidhar N. K., Insel N., Dong J. Y., Takehara-Nishiuchi K. (2017). Observational fear learning in degus is correlated with temporal vocalization patterns. Behav. Brain Res. 332, 362–371. doi: 10.1016/j.bbr.2017.06.011
Lidhar N. K., Thakur A., David A. J., Takehara-Nishiuchi K., Insel N. (2021). Multiple dimensions of social motivation in adult female degus. PloS One 16, e0250219. doi: 10.1371/journal.pone.0250219
Ma L., Versteegh M. A., Hammers M., Komdeur J. (2022). Sex-specific influence of communal breeding experience on parenting performance and fitness in a burying beetle. R Soc. Open Sci. 9, 211179. doi: 10.1098/rsos.211179
Makowska I. J., Weary D. M. (2016a). Differences in Anticipatory Behaviour between Rats (Rattus norvegicus) Housed in Standard versus Semi-Naturalistic Laboratory Environments. PloS One 11, e0147595. doi: 10.1371/journal.pone.0147595
Makowska I. J., Weary D. M. (2016b). The importance of burrowing, climbing and standing upright for laboratory rats. R Soc. Open Sci. 3, 160136. doi: 10.1098/rsos.160136
Mcclintock M. K., Adler N. T. (1978). The role of the female during copulation in wild and domestic Norway rats (Rattus norvegicus). Behaviour 67, 67–96.
Mckittrick C. R., Magarinos A. M., Blanchard D. C., Blanchard R. J., Mcewen B. S., Sakai R. R. (2000). Chronic social stress reduces dendritic arbors in CA3 of hippocampus and decreases binding to serotonin transporter sites. Synapse 36, 85–94. doi: 10.1002/(SICI)1098-2396(200005)36:2<85::AID-SYN1>3.0.CO;2-Y
Melhorn S. J., Elfers C. T., Scott K. A., Sakai R. R. (2017). A closer look at the subordinate population within the visible burrow system. Physiol. Behav. 178, 110–116. doi: 10.1016/j.physbeh.2017.01.039
Melhorn S. J., Krause E. G., Scott K. A., Mooney M. R., Johnson J. D., Woods S. C., et al. (2010). Meal patterns and hypothalamic NPY expression during chronic social stress and recovery. Am. J. Physiol. Regulatory Integr. Comp. Physiol. 299, R813–R822. doi: 10.1152/ajpregu.00820.2009
Messina A., Potrich D., Perrino M., Sheardown E., Miletto Petrazzini M. E., Luu P., et al. (2022). Quantity as a fish views it: behavior and neurobiology. Front. Neuroanat 16.
Murphy K. Z., Haile E., Mctigue A., Pierce A. F., Donaldson Z. R. (2023). PhAT: A flexible open-source GUI-driven toolkit for photometry analysis. bioRxiv. doi: 10.1101/2023.03.14.532489
Nagy M., Davidson J. D., Vasarhelyi G., Abel D., Kubinyi E., Hady A. E. (2023). and Vicsek, T. Social structure and individuals’ behavior shaped by group composition in long-term tracking of rats. bioRxiv. doi: 10.1101/2023.03.18.533183
Nilsson S., Goodwin N. L., Choong J. J., Hwang S., Wright H. R., Norville Z. C., et al. (2020). Simple Behavioral Analysis (SimBA) - an open source toolkit for computer classification of complex social behaviors in experimental animals. bioRxiv, 049452. doi: 10.1101/2020.04.19.049452.
Omer D. B., Maimon S. R., Las L., Ulanovsky N. (2018). Social place-cells in the bat hippocampus. Science 359, 218–224. doi: 10.1126/science.aao3474
Peragine D. E., Pokarowski M., Mendoza-Viveros L., Swift-Gallant A., Cheng H. M., Bentley G. E., et al. (2017). RFamide-related peptide-3 (RFRP-3) suppresses sexual maturation in a eusocial mammal. Proc. Natl. Acad. Sci. U.S.A. 114, 1207–1212. doi: 10.1073/pnas.1616913114
Pereira T. D., Tabris N., Matsliah A., Turner D. M., Li J., Ravindranath S., et al. (2022). SLEAP: A deep learning system for multi-animal pose tracking. Nat. Methods 19, 486–495. doi: 10.1038/s41592-022-01426-1
Powell J. M., Inoue K., Wallace K. J., Seifert A. W., Young L. J., Kelly A. M. (2022). Distribution of vasopressin 1a and oxytocin receptor protein and mRNA in the basal forebrain and midbrain of the spiny mouse (Acomys cahirinus). Brain Struct. Funct 228 (2), 413–431. doi: 10.1007/s00429-022-02581-z
Ragland N. H., Compo N. R., Wiltshire N., Shepard A., Troutman S., Kissil J. L., et al. (2022). Housing and husbandry alternatives for naked mole rat colonies used in research settings. J. Am. Assoc. Lab. Anim. Sci. 61, 412–418. doi: 10.30802/AALAS-JAALAS-22-000035
Ratuski A. S., Weary D. M. (2022). Environmental enrichment for rats and mice housed in laboratories: A metareview. Anim. (Basel) 12. doi: 10.3390/ani12040414
Ribeiro H. V., Acre M. R., Faulkner J. D., Da Cunha L. R., Lawson K. M., Wamboldt J. J., et al. (2022). Effects of shady environments on fish collective behavior. Sci. Rep. 12, 17873. doi: 10.1038/s41598-022-22515-3
Riehl C. (2021). Evolutionary origins of cooperative and communal breeding: Lessons from the crotophagine cuckoos. Ethology 127, 827–836.
Rodriguez-Santiago M., Jordan A., Hofmann H. A. (2022). Neural activity patterns differ between learning contexts in a social fish. Proc. Biol. Sci. 289, 20220135. doi: 10.1098/rspb.2022.0135
Rose M. C., Styr B., Schmid T. A., Elie J. E., Yartsev M. M. (2021). Cortical representation of group social communication in bats. Science 374, eaba9584. doi: 10.1126/science.aba9584
Roth B. L. (2016). DREADDs for neuroscientists. Neuron 89, 683–694. doi: 10.1016/j.neuron.2016.01.040
Schweinfurth M. K. (2020). The social life of Norway rats (Rattus norvegicus). Elife 9. doi: 10.7554/eLife.54020
Shellard A., Mayor R. (2020). Rules of collective migration: from the wildebeest to the neural crest. Philos. Trans. R Soc. Lond B Biol. Sci. 375, 20190387. doi: 10.1098/rstb.2019.0387
Shemesh Y., Sztainberg Y., Forkosh O., Shlapobersky T., Chen A., Schneidman E. (2014). Correction: High-order social interactions in groups of mice. Elife 3, e03602. doi: 10.7554/eLife.03602
Skinner M., Ceuppens P., White P., Prior H. (2019). Social-housing and use of double-decker cages in rat telemetry studies. J. Pharmacol. Toxicol. Methods 96, 87–94. doi: 10.1016/j.vascn.2019.02.005
So N., Franks B., Lim S., Curley J. P. (2015). A social network approach reveals associations between mouse social dominance and brain gene expression. PloS One 10, e0134509. doi: 10.1371/journal.pone.0134509
Stevenson S. A., Piepenburg A., Spool J. A., Angyal C. S., Hahn A. H., Zhao C., et al. (2020). Endogenous opioids facilitate intrinsically-rewarded birdsong. Sci. Rep. 10, 11083. doi: 10.1038/s41598-020-67684-1
Tan Z., Garduno B. M., Aburto P. F., Chen L., Ha N., Cogram P., et al. (2022). Cognitively impaired aged Octodon degus recapitulate major neuropathological features of sporadic Alzheimer’s disease. Acta Neuropathol. Commun. 10, 182. doi: 10.1186/s40478-022-01481-x
Tang W., Davidson J. D., Zhang G., Conen K. E., Fang J., Serluca F., et al. (2020). Genetic control of collective behavior in zebrafish. iScience 23, 100942. doi: 10.1016/j.isci.2020.100942
Tuckova V., Sumbera R., Cizkova B. (2016). Alloparental behaviour in Sinai spiny mice (Acomys dimidiatus): a case of misdirected parental care? Behav. Ecol. Sociobiol 70, 437–447. doi: 10.1007/s00265-016-2065-7
Vanoortmerssen G. A. (1971). Biological significance, genetics and evolutionary origin of variability in behavior within and between inbred strains of mice (Mus Musculus). Behaviour 38, 1–91.
Walter T., Couzin I. D. (2021). TRex, a fast multi-animal tracking system with markerless identification, and 2D estimation of posture and visual fields. Elife 10. doi: 10.7554/eLife.64000
Weidt A., Lindholm A. K., Konig B. (2014). Communal nursing in wild house mice is not a by-product of group living: females choose. Naturwissenschaften 101, 73–76. doi: 10.1007/s00114-013-1130-6
Wey T. W., Burger J. R., Ebensperger L. A., Hayes L. D. (2013). Reproductive correlates of social network variation in plurally breeding degus (Octodon degus). Anim. Behav. 85, 1407–1414. doi: 10.1016/j.anbehav.2013.03.035
Williams A. V., Duque-Wilckens N., Ramos-Maciel S., Campi K. L., Bhela S. K., Xu C. K., et al. (2020). Social approach and social vigilance are differentially regulated by oxytocin receptors in the nucleus accumbens. Neuropsychopharmacology 45, 1423–1430. doi: 10.1038/s41386-020-0657-4
Williamson C. M., Franks B., Curley J. P. (2016). Mouse social network dynamics and community structure are associated with plasticity-related brain gene expression. Front. Behav. Neurosci. 10. doi: 10.3389/fnbeh.2016.00152
Yang Y., Wu M., Vazquez-Guardado A., Wegener A. J., Grajales-Reyes J. G., Deng Y., et al. (2021). Wireless multilateral devices for optogenetic studies of individual and social behaviors. Nat. Neurosci. 24, 1035–1045. doi: 10.1038/s41593-021-00849-x
Keywords: grouping behavior, sociality, neural mechanisms, rodent, mammal, group dynamics
Citation: Kelly AM (2023) Advancements in the study of neural mechanisms underlying mammalian grouping behaviour. Front. Ethol. 2:1273613. doi: 10.3389/fetho.2023.1273613
Received: 06 August 2023; Accepted: 26 September 2023;
Published: 09 October 2023.
Edited by:
Todd M. Freeberg, The University of Tennessee, United StatesReviewed by:
Cristiano Azevedo, Universidade Federal de Ouro Preto, BrazilAnnaliese K. Beery, University of California, Berkeley, United States
Kalynn M. Schulz, The University of Tennessee, United States
Copyright © 2023 Kelly. This is an open-access article distributed under the terms of the Creative Commons Attribution License (CC BY). The use, distribution or reproduction in other forums is permitted, provided the original author(s) and the copyright owner(s) are credited and that the original publication in this journal is cited, in accordance with accepted academic practice. No use, distribution or reproduction is permitted which does not comply with these terms.
*Correspondence: Aubrey M. Kelly, YXVicmV5LmtlbGx5QGVtb3J5LmVkdQ==; YXVicmV5LmtlbGx5QGVtb3J5LmVkdQ==