- 1Department of Ocean Sciences, Memorial University of Newfoundland, St. John’s, NL, Canada
- 2Department of Ecology and Evolutionary Biology, University of Toronto, Toronto, ON, Canada
- 3World Resources Institute, San Francisco, CA, United States
Plastic pollution and climate change are two major environmental issues of this century, with implications for ecosystem health, the economy, and humankind. Plastics have the potential to affect the climate in multiple ways, yet we lack a thorough understanding of what data we have on this phenomenon and where the knowledge gaps are. Here, we conducted a systematic review to assemble knowledge and answer the question: How do plastics impact climate through three major mechanisms–emissions of greenhouse gases across the plastics lifecycle, interference with Earth’s carbon sinks, and interference with Earth’s radiation budget? We searched through all 14 databases in Web of Science for relevant articles, and amended this pool with articles from manual reference searching and expert elicitations. Using rigorous inclusion and exclusion criteria, including the exclusion of non-peer reviewed studies to minimize risk of bias, we ultimately selected 143 articles for our review - 36 lifecycle greenhouse gas emissions papers, 83 carbon sink papers, and 24 radiation budget papers. Based on current available data, we found that the plastics lifecycle can emit up to two gigatonnes of carbon dioxide equivalents per year, with the most emissions being produced at the primary production and product manufacture stages. From existing carbon sink studies, we identified more instances of plastics negatively affecting carbon sequestration than vice versa. From the radiation budget papers, we found that radiative impacts are predominantly cooling in nature. The body of evidence is incomplete and more research is needed to confirm these findings and fill in existing knowledge gaps. Future work should prioritize quantifying greenhouse gas emissions from the transportation, consumption, and unmanaged waste stages of the plastics lifecycle. We need more studies that examine the impact of plastics on coastal blue carbon ecosystems and marine carbon sequestration endpoints, and more studies examining the impact of plastics on direct radiative forcing via aerosols, cloud properties, and the albedo/melting rate of surfaces and ice/snow. Immediate action is required to decarbonize the plastics lifecycle, and full accounting of the climate impact of plastics is needed in emissions scenarios, inventories, and climate models across geographies and sectors.
BOX 1 | Glossary of terms. Definitions of key terminology used in this study are found here, some of which have been adapted from other sources (GRID-Arendal, 2024; Villarrubia-Gómez et al., 2024).
Albedo: Percentage of incident radiation that is reflected from a surface.
Biological carbon pump (BCP): The mechanism by which the ocean stores carbon on the order of centuries; the BCP encompasses the mixing, gravitational, and migrant pumps which transport carbon to the deep ocean.
Carbon sink: A place of storage of carbon, for instance the ocean or soils, which slow the rise of carbon dioxide (CO2) in the atmosphere.
Chemical recycling: The process by which plastic waste is broken down into its molecular constituents using chemical reactions, often employing high temperatures and harsh solvents. Chemical, or “advanced” recycling, depolymerizes plastics into monomers or other substances. The products derived from chemical recycling, including gases and liquid feedstocks, form feedstock for the production of new polymers, chemicals, or fuels.
Conventional plastics: Refers to a variety of synthetic polymers which are produced from the polymerization of petrochemical feedstocks. They often contain additives including plasticizers, flame retardants, pigments, and other petrochemicals.
Coastal blue carbon ecosystems: Coastal ecosystems, in particular salt marshes, seagrasses, and mangroves, which account for a relatively small area globally but play a major role in carbon sequestration.
Earth’s radiation budget: The balance of incoming radiation towards the Earth’s surface and outgoing radiation away from the Earth’s surface, which helps maintain the surface temperature of the Earth.
Incineration: The burning of waste material at high temperatures in a controlled environment.
Landfilling: The disposal of waste material in a dedicated location, usually lined with barriers to prevent contamination of the surrounding landscape.
Macroplastics: Plastic particles accumulating in the environment which are greater than 5 mm in diameter.
Marine ecosystems: Refers to diverse habitats found in the global ocean and its biota.
Mechanical recycling: The shredding, grinding, washing, drying, and re-pelletizing of plastic waste for conversion into secondary raw materials, without significant alteration to its chemical structure.
Microplastics: Plastic particles accumulating in the environment which are 1 μm–5 mm in diameter.
Monomer: Molecules which form the building blocks of plastics. They are linked together repeatedly to form long polymer chains.
Nanoplastics: Plastic particles accumulating in the environment which are less than 1 μm in diameter.
Open burning: The burning of waste material outdoors, resulting in the direct release of greenhouse gases, volatile organic compounds, and toxic compounds into the atmosphere and surroundings.
Open dumping: The disposal of waste in areas that are not designed to handle them, including on land or in water, with consequences including air, soil, and water pollution.
Plastic debris: Pieces of plastic that have infiltrated into the environment.
Plastic pollution: Pollution refers to the introduction of plastics and the chemicals in them into the ecosphere, i.e., into the system of living and non-living components, including humans.
Plastic waste: Waste is the unwanted or unusable plastic material that remains after its intended use.
Polymer: Long chains of monomers linked together by chemical bonds, which can be natural or synthetic. Plastics are examples of synthetic polymers.
Terrestrial ecosystems: Refers to land-based ecosystems, excluding coastal blue carbon ecosystems.
1 Introduction
Plastic pollution and climate change are two of the most pressing global environmental issues of the 21st century, and are pushing humanity towards the limits of our planet’s safe operating space (Rockström et al., 2009; United Nations, 2015; Villarrubia-Gómez et al., 2024; Villarrubia-Gómez et al., 2018). Both of these issues need to be addressed in order to achieve the United Nations Sustainable Development Goals (UN SDGs) of Climate Action (SDG 13) and Life Under Water (SDG 14), among others (SDG 3 “Good Health and Wellbeing”, SDG 6 “Clean Water and Sanitation”, SDG 12 “Responsible Consumption and Production”, and SDG 15 “Life on Land”) (United Nations, 2015). However, the way in which these issues interact with and potentially exacerbate one another is poorly understood. To date there is no rigorous synthesis of knowledge on how plastics affect climate change, and via which mechanisms. A thorough understanding of how plastics affect climate change is crucial to effectively mitigate both issues (Ford et al., 2022; Zhu, 2021a).
Conventional plastic polymers are produced from the polymerization of petrochemical feedstocks (Center for International Environmental Law, 2019; Zhu, 2021a). They often contain additives including plasticizers, flame retardants, and pigments (Rochman et al., 2019). Plastic is a wonder material used in our everyday lives, playing a vital role in the technology, medical, and transportation industries, among others (PlasticsEurope, 2017). However, plastics leak into the environment throughout their lifecycle, and especially after their useful phase of life; over time, these plastics break down into smaller and smaller pieces, often with negative consequences for ecosystems and biota (Geyer et al., 2017; Rochman et al., 2019; Thompson et al., 2004) and people (Leslie et al., 2022; Ragusa et al., 2021; Vethaak and Leslie, 2016; Wright and Kelly, 2017). Plastic particles less than 5 mm in diameter are referred as to microplastics (Thompson et al., 2004), and particles less than 1 μm are referred to as nanoplastics (Koelmans et al., 2015).
Plastics affect the climate in numerous ways. There are three major mechanisms through which plastics impact climate, or “impact categories”: 1) greenhouse gas (GHG) emissions throughout the plastics lifecycle, 2) impacts to carbon cycling, and 3) impacts to the Earth’s radiation budget. GHG emissions exist at every single step of the plastics lifecycle, due to the energy needed to fuel these lifecycle processes such as fossil fuel extraction, and plastic and related petrochemical manufacture and production, as well as to emissions of GHGs as a result of plastic degradation or burning (Zheng and Suh, 2019). By 2050, it is anticipated that GHG emissions from the plastics lifecycle can consume up to 13% of Earth’s remaining carbon budget based on existing estimates (Center for International Environmental Law, 1989). Black carbon is also an emission of interest from the plastics lifecycle, produced from the burning or incineration of plastics–it is an aerosol that has a warming effect on the planet (Intergovernmental Panel on Climate Change, 2021). Upon incorporation into ice/snow, it can reduce their albedo and increase their melting rate (Hadley and Kirchstetter, 2012). However, knowledge about emissions across the lifecycle has not been synthesized to date across peer-reviewed literature. This precludes an understanding of how much we know about the impact of plastic on climate across its lifecycle, which steps are the most polluting, and where the knowledge gaps are.
Plastics may also impact carbon cycling, in particular Earth’s ability to sequester carbon. There has been increasing concern around how plastic debris may impact the ability of terrestrial and marine ecosystems to photosynthesize, affect soil carbon stores, and affect the biological carbon pump (BCP) (Galgani and Loiselle, 2021; Shen et al., 2020; Zhao et al., 2023). As a result, studies in this area have been increasing over the years (Cole et al., 2016; Hou et al., 2023; Meng et al., 2023; Zhao et al., 2023). There have been numerous studies published that discuss the potential impact of plastic pollution on Earth’s natural carbon sinks, however many of these studies do not provide evidence of impact, i.e., they only speculate on the potential effects. A more thorough analysis is required to disentangle speculation from true effect.
The third major category of impacts is how plastics may interfere with Earth’s radiation budget. As more and more plastic pollution enters the environment and breaks up into smaller pieces, scientists are detecting increasing concentrations of plastic aerosols, which are micro- and nano-plastic particles suspended in the atmosphere [e.g., (Allen et al., 2022; Rosso et al., 2023; Trainic et al., 2020)] as well as higher abundances of micro- and nano-plastic particles incorporated into snow, ice, and clouds [e.g., (Crosta et al., 2022; Wang et al., 2023; Zhang et al., 2022)]. These particles may influence how the Earth interacts with incoming ultraviolet (UV) radiation and outgoing longwave radiation, affecting the ability of the Earth to maintain its current temperature. It is necessary to assemble what we know about the impact of plastics on Earth’s radiation budget and identify future steps for research in order to gain a comprehensive understanding of how plastics are impacting Earth’s climate.
Here, we conducted a systematic review to determine the current state of knowledge on how plastics, and their inherent pollution, impact climate change, identify knowledge gaps, and provide next steps to inform future research. We also discuss necessary policy recommendations to provide for full accounting and reduce the impact of plastics on the Earth’s climate.
2 Materials and methods
2.1 Identifying relevant studies
We followed the guidelines of the Preferred Reporting Items for Systematic Reviews and Meta-Analyses 2020 (PRISMA) (Page et al., 2021) in our identification and selection of relevant studies. The PRISMA flowchart is shown in Figure 1.
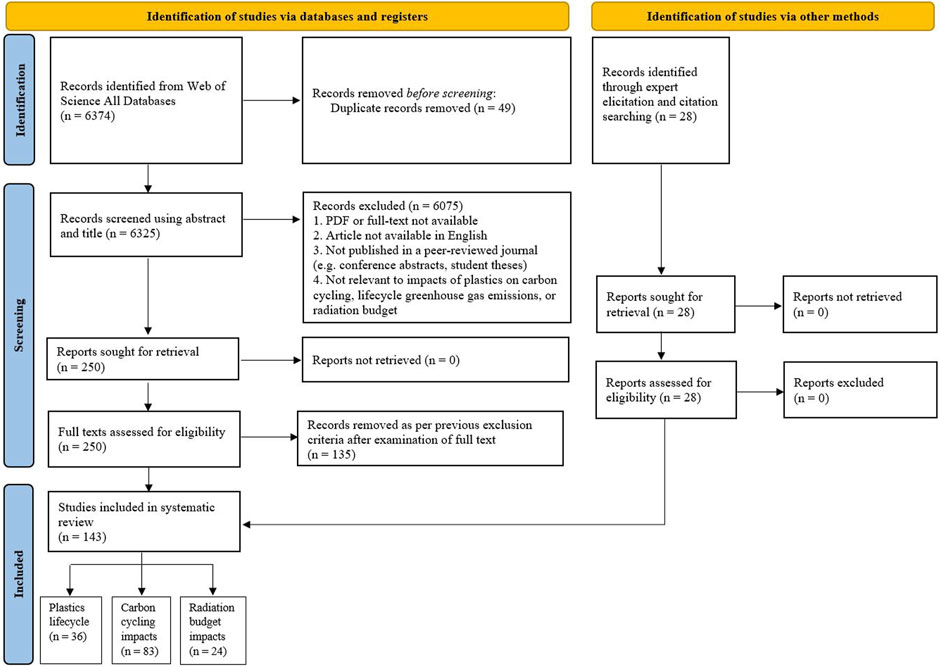
Figure 1. PRISMA flowchart showing the systematic process used to identify relevant studies for this review. The search query used to find relevant articles can be found in Supplementary Text S1.
We searched Web of Science All Databases on 29 August 2023, using a search query (Supplementary Text S1) to find relevant studies for the period of 1 January 1900 to 29 August 2023 using keywords relevant to GHG emissions from the plastics lifecycle, the impact of plastics on carbon sinks, and the impact of plastics on Earth’s radiation budget. Multiple methods were used to retrieve articles. We searched through all 14 databases within Web of Science across all editions. We also identified relevant studies by manually searching through the reference lists of articles and via expert elicitation (we expand on this further below). A total of 6,374 studies were identified from the initial search on Web of Science All Databases (Figure 1). After removing duplicates, 6,325 studies remained (Figure 1). Two independent reviewers (the co-authors) filtered through the studies using abstract and title; we removed studies where the full-text was not available, studies were not written in English, the material was non-peer reviewed, and where studies were not relevant to the impacts of plastics on climate via one of the three mechanisms (lifecycle GHG emissions, impacts to carbon cycling, or impacts to the Earth’s radiation budget) (Figure 1).
For lifecycle GHG emissions studies, we included studies that report at least one GHG emissions or GHG emissions intensity estimate pertaining to the plastics lifecycle. For carbon cycling studies, we included studies where the impact of plastics on at least one endpoint relevant to carbon cycling was measured or speculated. For radiative studies, we included studies where the impact of plastic on a radiative forcing (RF) mechanism was measured or speculated. We excluded studies that quantified plastic in an environmental matrix (soil, water, biota) without discussing impact. For lifecycle studies and carbon cycling studies that measured soil respiration as an endpoint, we only included studies that focused on carbon dioxide (CO2) or methane (CH4), the two greenhouse gases that account for the greatest amount of anthropogenic warming to date (Intergovernmental Panel on Climate Change, 2021), as well as any studies that report emissions of black carbon from open burning. For carbon cycling studies we focused on terrestrial and marine ecosystems, and excluded studies on freshwater ecosystems owing to their relatively smaller capacity for carbon sequestration. We focused on studies where at least one of the plastics tested was a conventional polymer (i.e., we excluded studies that only used bioplastics–please see Supplementary Text S2 for more information). We were left with 250 studies after filtering using abstract and title. The remaining 250 studies were assessed again for relevance against the same exclusion criteria, but this time by examining the full text.
We also elicited relevant articles from experts and manually searched through reference lists to identify relevant studies, as a quality assurance/quality control step, to ensure that the collection of articles informing our evidence base is comprehensive. After incorporating 28 records identified via expert elicitation and by searching through the reference lists of included studies, we were left with 143 studies for our systematic review: 36 lifecycle papers, 83 carbon cycling papers, and 24 radiation papers (Figure 1; Supplementary Table S1 contains all the papers we considered in our review).
We screened our final selection of studies for quality. We checked that all lifecycle GHG emissions, carbon cycling, and radiative studies described their methodology in a reproducible way. We ensured that all carbon cycling and radiative studies have a control (for experimental studies) and have replicates (for field sampling and experimental studies).
2.2 Extracting relevant information
We extracted relevant information from studies for data analysis. Details can be found in Supplementary Text S3.
2.3 Data analysis and presentation
R 3.4.3 was used to generate all barplots. Adobe Illustrator 2024 was used to generate summary figures (Figures 2–4). Figures 2–4 summarize the amount of attention being paid to the climate effects of plastics by lifecycle stage (Figure 2), ecosystem (Figure 3), and radiative forcing mechanism (Figure 4). Figures 3, 4 shed light on how much speculation of effect exists relative to the actual testing of effects. Ultimately, these figures show what data exists currently, and where the knowledge gaps are.
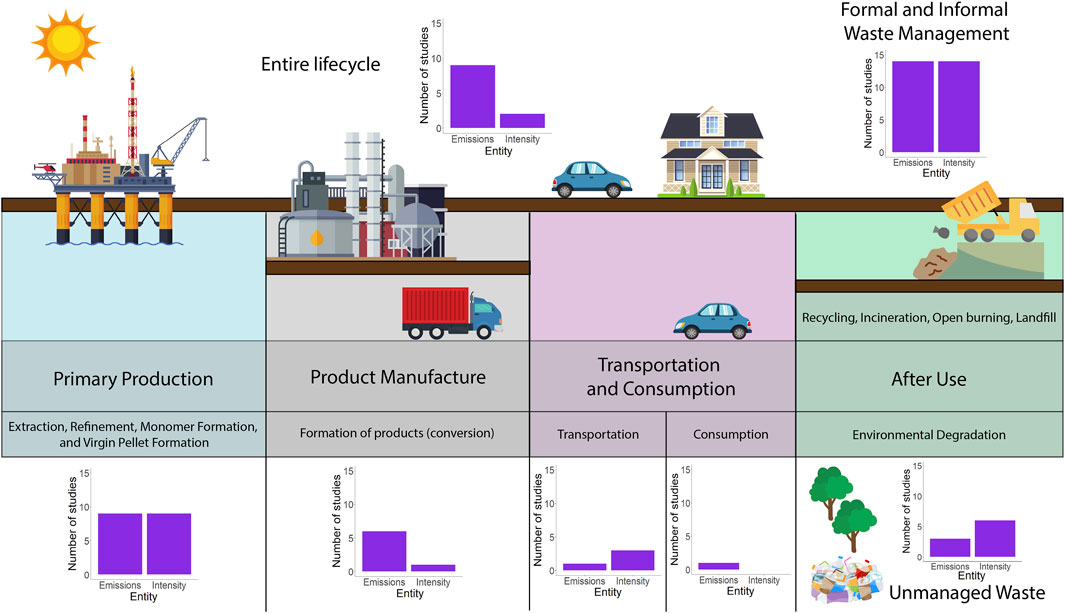
Figure 2. Synthesis of existing knowledge on GHG emissions from the plastics lifecycle. The plastics lifecycle is broken down into the primary production, product manufacture, transportation and consumption, and after use stages (waste management and unmanaged waste). Barplots show the number of studies that reported absolute GHG emissions and/or emissions intensities for specific stages of the plastics lifecycle. Entity refers to GHG emissions or GHG emissions intensity.
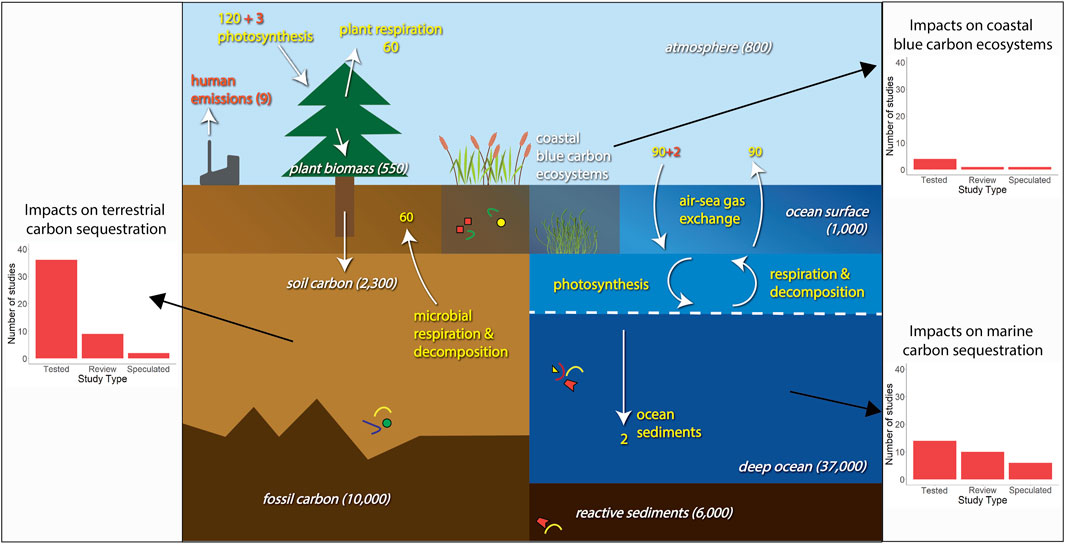
Figure 3. Synthesis of existing knowledge on the impacts of plastics on carbon sequestration by Earth’s natural carbon sinks. Carbon sinks are shown in white text with units of gigatons carbon, carbon fluxes are shown in yellow text with units of gigatons carbon per year, and anthropogenic interference on carbon fluxes is shown in red text. The number of studies that tested for an effect, number of reviews, and number of studies that speculated an effect are grouped by ecosystem–terrestrial, coastal blue carbon, and marine–and shown in barplots. The y-axis in each barplot shows number of studies, and the x-axis shows study type. Plastic particles are shown as colorful shapes and lines.
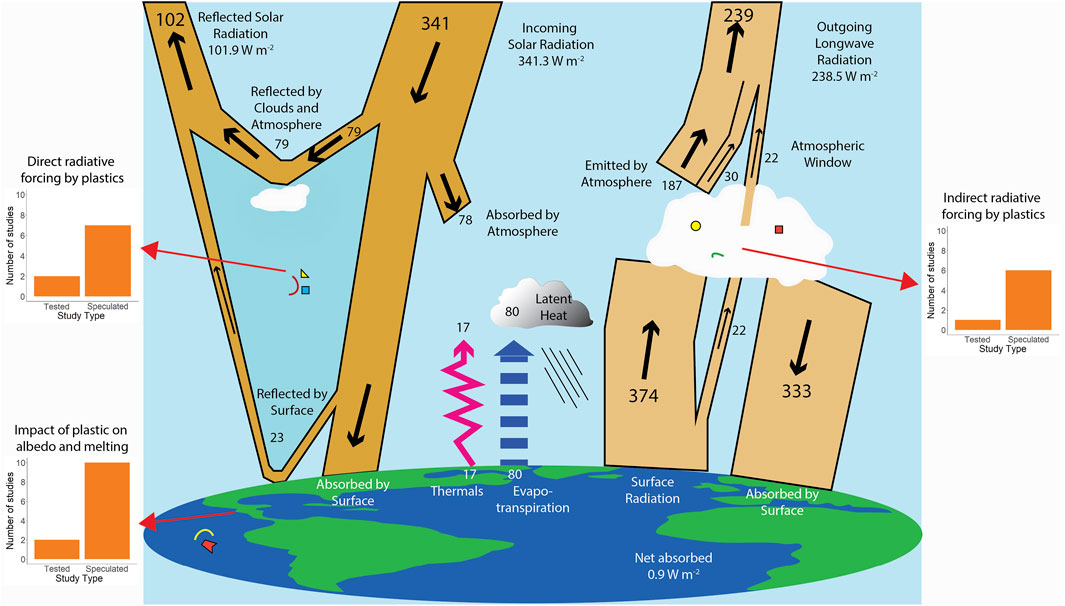
Figure 4. Synthesis of existing knowledge on the impacts of plastics on Earth’s radiation budget. All numbers in this diagram have units of watts per square meter–including black arrows, pink arrow, blue arrow, and white text. The number of studies that tested for an effect, and number of studies that speculated an effect are grouped by endpoint–direct RF, indirect RF, and change in albedo of surfaces/melting rate of snow or ice–and shown in barplots. The y-axis of each barplot shows number of studies, and the x-axis shows study type. Plastic particles are shown as colorful shapes and lines.
3 Results
A summary of publication trends can be found in Supplementary Text S4 and Supplementary Figure S1.
3.1 Study characteristics and synthesis of current knowledge
3.1.1 GHG emissions from the plastics lifecycle
All 36 studies in the category of GHG emissions from the plastics lifecycle are primary articles [please see Supplementary Data Material for all study characteristics (Zhu, 2025)]. A total of 14 studies reported absolute GHG emissions, and 26 studies reported GHG emissions intensities from one or more stages of the plastics lifecycle. Nine studies were global in scale, two were continental, 23 were national, and four had unspecified geographies. GHG emissions were reported in units of either carbon dioxide (CO2) or carbon dioxide equivalents (CO2e). Units of CO2e are used to standardize the global warming potential of other greenhouse gases to that of CO2. Emissions intensities were reported in units of CO2e/unit plastic or kWh/unit plastic, which can be used to derive CO2e. We focused on the CO2e emissions intensities for all subsequent analyses. The amount of data available on GHG emissions and emissions intensities is summarized by plastics lifecycle stage in Figure 2. One study quantified black carbon emissions from the open burning of plastics (Cruz et al., 2022).
There exist nine GHG emissions quantifications and two emissions intensity estimates for the entire plastics lifecycle (Figure 2). There are both nine emissions estimates as well as nine emissions intensity estimates for the primary production stage; six emissions estimates and one emissions intensity estimate for the product manufacture stage, one emissions estimate and three emissions intensity estimates for the transportation stage; one emissions estimate for the consumption stage; fourteen estimates for both emissions as well as emissions intensities for the waste management stage; and finally, three emissions estimates and six emissions intensity estimates for the unmanaged waste stage (Figure 2).
Ranges of annual GHG emissions and GHG emissions intensities reported in the literature are summarized by plastics lifecycle stage in Table 1. All GHG emissions and emissions intensity estimates are non-polymer specific unless otherwise noted. We combined polymer-specific and non-polymer specific GHG data in our tables because our goal was to assess how much information exists at each stage of the plastics lifecycle across geographies, not how GHG emissions and GHG emissions intensity estimates differ between polymer types. However, we still discuss the extent of known polymer-specific GHG data (for the timeframe included in this study) later in this section.
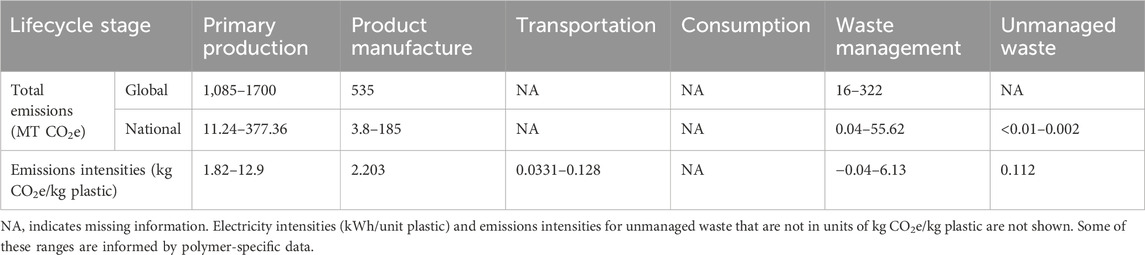
Table 1. Summary of reported annual GHG emissions and GHG emissions intensity values for each stage of the plastics lifecycle from the scientific literature. Ranges of reported ranges are shown.
All studies reported non-polymer specific GHG emissions estimates except for Chu et al. (2022a) which reported GHG emissions specific to the PET lifecycle in China. Globally, GHG emissions at the primary production stage of the plastics lifecycle span 1,085–1700 million tonnes (MT) CO2e (Table 1). An estimated 535 MT CO2e of emissions are associated with the product manufacture stage, and 16–322 MT CO2e of emissions are associated with the waste management stage (Table 1). At the national scale, emissions from the primary production stage span 11.24–377.36 MT CO2e, emissions from the product manufacture stage span 3.8–185 MT CO2e (185 MT CO2e pertains to PET), emissions from the waste management stage span 0.04–55.62 MT CO2e, and emissions from unmanaged waste span <0.01–0.002 MT CO2e (Table 1). There is no data on GHG emissions for the transportation or consumption stages nationally or globally, and no data on emissions for the unmanaged waste stage at the global level (Table 1). Polymer-specific GHG emissions intensity estimates for the plastics lifecycle were reported by 7 out of 36 lifecycle GHG studies (An et al., 2022; Bora et al., 2020; Chung et al., 2003; Drewniok et al., 2023; Goga et al., 2023; Poovarodom et al., 2015; Zappitelli et al., 2021) - all other GHG emissions intensity estimates are non-polymer specific. Emissions intensities span 1.82 (LLDPE) - 12.9 (LDPE) kg CO2e/kg plastic for the primary production stage, 2.203 kg CO2e/kg plastic for the product manufacture stage, −0.04-6.13 (6.13 kg CO2e/kg plastic pertains to Polyamide 6) kg CO2e/kg plastic for the waste management stage, and 0.112 kg CO2e/kg plastic for unmanaged waste (Table 1). GHG emissions intensities for the consumption stage are currently unavailable (Table 1).
Taking a deeper dive into emissions and intensities associated with specific waste management strategies, Table 2 summarizes the ranges of these values across the literature. Mechanical recycling refers to the reprocessing of plastic material into secondary raw materials via physical means, such as grinding and shedding. Forms of chemical recycling explored in studies include coke oven, pyrolysis, gasification, and gasification-pyrolysis. Incineration and landfill refer to the burning and disposal of waste materials in controlled facilities, while open burning and open dumping are counterparts to the above two processes but performed in an uncontrolled manner.
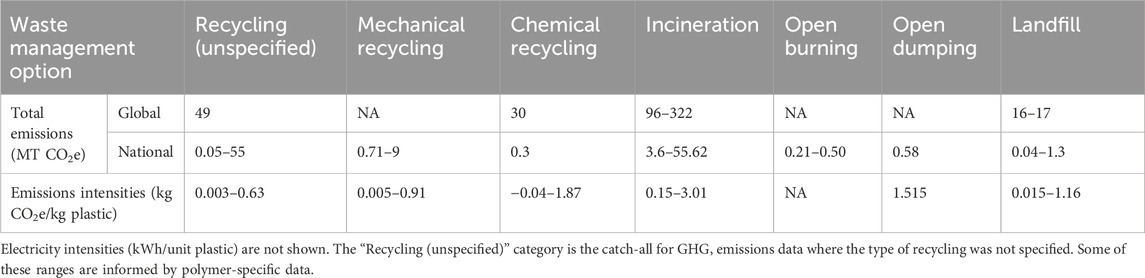
Table 2. Summary of reported annual GHG emissions and GHG emissions intensity values for different waste management strategies for the waste management stage of the plastics lifecycle. Ranges of reported values are shown.
Globally, recycling (type unspecified) is associated with emissions of 49 MT CO2e, chemical recycling with emissions of 30 MT CO2e, incineration with emissions of 96–322 MT CO2e, and landfilling with emissions of 16–17 MT CO2e (Table 2). Nationally, recycling (unspecified) is associated with emissions of 0.05–55 MT CO2e, mechanical recycling with emissions of 0.71–9 MT CO2e (9 MT CO2e pertains to PET), chemical recycling with emissions of 0.3 MT CO2e for PET, incineration with emissions of 3.6–55.62 MT CO2e, open burning with emissions of 0.21–0.50 MT CO2e, open dumping with emissions of 0.58 MT CO2e, and landfilling with emissions of 0.04–1.3 MT CO2e (1.3 MT CO2e pertains to PET) (Table 2). Emissions intensities span 0.003–0.63 kg CO2e/kg plastic for recycling (unspecified), 0.005–0.91 kg CO2e/kg plastic for mechanical recycling, −0.04-1.87 kg CO2e/kg plastic for chemical recycling, 0.15–3.01 kg CO2e/kg plastic for incineration (0.15 kg CO2e/kg plastic pertains to PP), 1.515 kg CO2e/kg plastic for open dumping, and 0.015–1.16 kg CO2e/kg plastic for landfilling (1.16 kg CO2e/kg plastic pertains to PP) (Table 2). Our review did not find any GHG emissions estimates associated with open burning or open dumping at the global scale, or GHG emissions intensities associated with the open burning of plastic waste (Table 2).
PS had the greatest polymer-specific GHG emissions intensity and HDPE the lowest at the primary production stage, out of HDPE, LDPE, PP, PET, PVC, PS, and other plastics, based on a 2023 study conducted in South Africa (Goga et al., 2023). A 2022 study conducted in China found that PVC had the greatest polymer-specific GHG emissions intensity and PE the lowest at the primary production stage, out of PVC, PP, and PE (An et al., 2022). Interestingly, the majority of polymer-specific GHG emissions intensity estimates to date have been reported for the primary production, product conversion, and unmanaged waste (environmental degradation) stages of the plastics lifecycle. Only one study has reported polymer-specific GHG emissions intensities for end-of-life treatment strategies of waste polypropylene (Bora et al., 2020). This makes sense as different polymers are often lumped and treated together as mixed plastic waste [e.g., (Demetrious and Crossin, 2019; Gradus et al., 2017; Jang et al., 2020; Shan et al., 2023)]. However, this precludes an understanding of the carbon footprint of different polymer types in the waste management system.
Global GHG emissions from the plastics lifecycle vary depending on the source of the emissions intensity data used in calculations and the geographic scale of the data (Cabernard et al., 2021; Zheng and Suh, 2019). Cabernard et al. (2021) divided the world into 49 regions and estimated the GHG emissions from 163 industrial sectors per region, while Zheng and Suh (2019) estimated GHG emissions for the entire world as a single region. GHG emissions across the plastics lifecycle as well as across waste management strategies exhibit greater variation at the national scale than the global scale (Table 1, 2). This is due to the fact that GHG emissions are often estimated via GHG emissions intensities, which are influenced by the differences in energy mix of the individual countries (Fruergaard et al., 2009). GHG emissions and emissions intensities also vary depending on the year in which studies were conducted.
3.1.2 Impact of plastics on carbon cycling
We identified 83 studies pertaining to the impact of plastics on carbon sequestration by Earth’s natural carbon sinks. A detailed breakdown of all the characteristics of the selected studies can be found in the Supplementary Data Material (Zhu, 2025). A total of 47 studies focused on impacts on terrestrial ecosystems, 6 studies focused on coastal blue carbon ecosystems, and 30 studies focused on marine ecosystems. Fifty-four studies tested the impact of plastics on carbon sequestration, 20 studies were reviews, and 9 studies speculated an impact (Figure 3). Studies that tested the impact of plastics on carbon sequestration deployed an experimentation (n = 51), modelling (n = 2), or field sampling approach (n = 1). Studies that speculated an impact on carbon cycling did not provide evidence to support their claim that an impact exists, for instance by conducting an experiment.
For terrestrial and coastal blue carbon ecosystem studies, soil sampling depths ranged from 5 cm (Chen et al., 2022) to 30 cm (Elbasiouny et al., 2023). The most frequently tested soil types were sandy soil (n = 12) and clay soil (n = 4). Experiment lengths ranged from 7 days to 10 years, with a median length of 53 days. Plastics used in experiments ranged from <200 nm in diameter (Pang et al., 2023) to objects as large as 25 cm in length (Menicagli et al., 2023). Microplastic morphologies used in experiments include fragments, films, spheres, tire wear particles, and microplastic powder purchased from manufacturers; the top three morphologies used in experiments were sphere (n = 14), film (n = 10), and fiber (n = 8). Studies deployed a variety of conventional plastic polymer types in experiments; the top three polymers used in experiments were PE (n = 23), PP (n = 9), and polyester (n = 6). Polymers were added to soil in pristine or aged forms, or in combination with co-stressors such as heavy metal mixtures (Mai et al., 2023), flame retardants (Han et al., 2023), and carbon nanotubes (Wang et al., 2022); a total of 19 of 39 studies investigated the effect of plastics on soil with a co-stressor. Experimental studies deployed soil microplastic concentrations ranging from 0.0005% w/w polystyrene spheres (Xu et al., 2021) to 75% w/w tire wear particles (Šourková et al., 2021).
For marine ecosystem studies, length of the experiment or simulation ranged from 3 hours (Bermúdez et al., 2021) to multiple centuries (Kvale et al., 2021). Plastics used in the experiments ranged from 100 nm to 7 mm. Microplastic morphologies used in experiments only consist of spheres (n = 9) or fragments (n = 2), apart from unspecified particle morphologies. Material types deployed in experiments only include the conventional plastic polymers of PS (n = 7), PE (n = 5), and PMMA (n = 1), apart from unspecified polymer types. These polymers were used on their own or in conjunction with co-stressors (e.g., UV filters, plastic additives, UV-B radiation, ocean warming); seven out of 15 studies tested the effects of plastics on marine endpoints in conjunction with one or more co-stressors. Concentrations were reported in a variety of units, including mg/L (range 0.01–100 mg/L), % w/v solution (2.5% w/v), items/mL (range 430–106 items/mL), and % v/v solution (range 0.0053%–0.053% v/v).
A total of 12 climate-relevant endpoints were commonly investigated or speculated by authors (for primary articles or studies that tested an effect). For terrestrial and coastal blue carbon ecosystem studies, these endpoints include: shift in microbial community, shift towards carbon sequestering strains, change in soil properties, carbon fixation via plants (or photosynthesis capacity), soil carbon stores, integrity/health of soil biota, and soil respiration. If the addition of plastic to soil increases the abundance of carbon sequestering strains of bacteria, which would help to release carbon from plastics and contribute to soil organic carbon, this would impose a cooling effect on the climate; a shift away from carbon sequestering strains or a negative effect on abundance of carbon sequestering strains indicates carbon mineralization or the loss of carbon from soil as carbon dioxide, resulting in a warming effect on climate. If the addition of plastics to soil hinders the ability of plants to fix carbon, then a warming effect results; vice versa, if plastics enhance carbon fixation by plants, this would result in a cooling effect. Plastics decreasing soil carbon stores (negative effect) results in a warming effect on climate; vice versa, a cooling effect. If soil biota are harmed, this may have implications for how much carbon soils are capable of storing, which would also exacerbate climate change–a negative effect on the health/integrity of soil biota results in a warming effect; vice versa, a cooling effect. Plastics increasing soil respiration rates (positive effect) results in a warming effect; vice versa, a cooling effect.
For marine studies, relevant endpoints reported in the literature include: functioning of the BCP, health/integrity of marine bacteria, health/integrity of phytoplankton, health/integrity of zooplankton, and ocean carbon uptake capacity. The BCP is a major mechanism by which carbon is permanently stored in the deep ocean; if this mechanism is disrupted, this may impact the drawdown of atmospheric carbon dioxide into the ocean and ultimately how much carbon the ocean can sequester–a negative impact on the BCP results in a warming effect on climate; vice versa, a cooling effect. For this endpoint, researchers often investigated the impact of plastic on fecal pellet or marine snow sinking rate. In addition, the health of marine organisms (including phytoplankton, zooplankton, and marine bacteria) is crucial to maintaining the ocean’s carbon cycle–a negative impact on the health/integrity of marine organisms results in a warming effect; vice versa, a cooling effect. Finally, some researchers investigated the impact of marine plastic on the drawdown of atmospheric carbon dioxide into the ocean–a negative effect on drawdown translates into a warming effect on climate; an increase in carbon dioxide drawdown would constitute a cooling effect. For each endpoint, we summarized how many times an effect was found or not found, and if applicable, the direction of effect for that endpoint (Table 3). Treatments where no effect was found tested for an effect, but the result was statistically insignificant. Treatments where an unclear effect was found generated results that were difficult to interpret, or where the resulting impact on climate change was unclear.
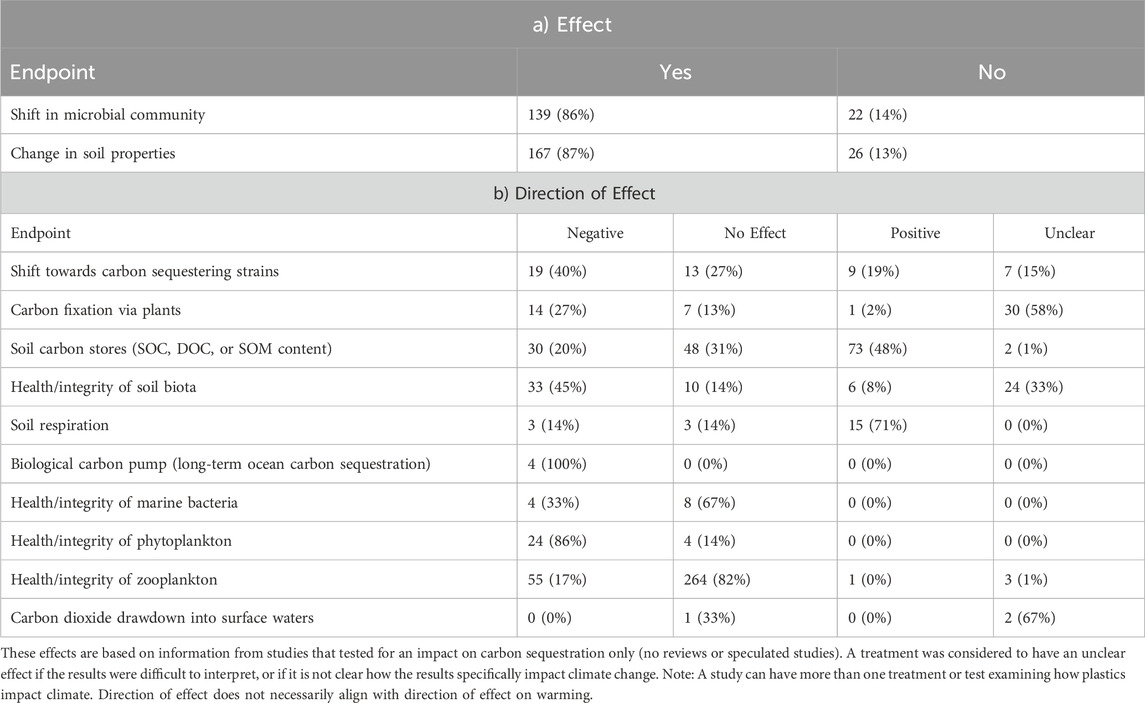
Table 3. a) Summary of the number of treatments where an effect was found, and where an effect was not found for the endpoints of “shift in microbial community” and “change in soil properties”. b) Summary of number of treatments where a negative effect, no effect, positive effect, or unclear effect was found for the listed endpoints. Percentages are shown in parentheses.
Overwhelmingly, experimental soil (terrestrial and coastal blue carbon) studies found that plastics induced a shift in microbial community (86% of treatments) and changed soil properties (87% of treatments). Apart from unclear results, forty percent (40%) of treatments found that there was a shift away from carbon sequestering strains upon administration of plastic in some form to the soil relative to the control, followed by 27% that found no significant effect (Table 3). Twenty-seven percent (27%) of treatments found a negative effect of plastic on carbon fixation capacity of plants relative to the control, followed by 13% that found no significant effect (Table 3). Nearly fifty percent (48%) of treatments found that the addition of plastics to soil increased soil carbon stores in the form of dissolved organic carbon (DOC), soil organic carbon (SOC), or soil organic matter (SOM), followed by 31% that found no significant effect (Table 3). Forty-seven (45%) of treatments found that addition of plastics to soil exhibited a negative effect on the health/integrity of soil biota relative to the control, followed by 14% that found no significant effect (Table 3). The majority of treatments (71%) that tested the impact of plastics on soil respiration found that addition of plastics to soil increased soil respiration rates relative to the control; studies that found plastic decreased soil respiration rates and studies that found no significant effect were tied at 14% (Table 3).
In terms of climate-relevant endpoints for carbon cycling studies on marine ecosystems, apart from unclear results: 100% of treatments found that plastics negatively impacted the functioning of the BCP (Table 3). The majority (67%) of treatments that examined the effect of plastics on marine bacteria found that plastic exerted no significant effect on their health/integrity, followed by 33% that found a negative effect (Table 3). Eighty-six (86%) of treatments found that plastic negatively impacted phytoplankton, followed by 14% of treatments that did not find a significant effect; these results are reversed in the case of zooplankton – 82% of treatments did not find an impact on zooplankton health/integrity, followed by 17% of treatments that found a negative impact (Table 3). Studies to date have not found that plastic influences carbon dioxide drawdown into the ocean appreciably in either direction (Table 3).
3.1.3 Impact of plastics on Earth’s radiation budget
Out of the 24 studies pertaining to the impact of plastics on Earth’s radiation budget, 11 studies focused on the direct RF of plastics, seven focused on indirect RF, and 11 focused on impact to albedo or melting rate. A detailed breakdown of all characteristics of the selected studies can be found in the Supplementary Data Material (Zhu, 2025). Five studies carried out primary research to test for an impact on a radiative endpoint, while 19 studies speculated the potential of plastics to have an impact on one or more radiative endpoints (Figure 4). Studies that tested for an effect either deployed an experimentation approach (n = 4) or a modelling approach (n = 1).
For the five studies that tested the impact of plastics on direct RF via aerosols, indirect RF via interactions with clouds, or surface albedo/melting rate changes, the sizes of plastic particles deployed in experiments or simulations range from nanoplastics (<1 μm) (Ganguly and Ariya, 2019) to large plastic sheets for albedo experiments (Fan et al., 2014). Colors of plastics deployed in experiments or simulations include non-pigmented, white, and a mixture of blue, yellow, purple, and translucent (Fan et al., 2014; Geilfus et al., 2019; Revell et al., 2021). Fragments (n = 2), fibers (n = 1), and spheres (n = 1) as well as macroplastics (n = 1) have been deployed in aerosol, ice nucleating particle, and albedo experiments or models apart from unspecified morphologies (Fan et al., 2014; Ganguly and Ariya, 2019; Geilfus et al., 2019; Revell et al., 2021; Yang and Ma, 2023). Conventional polymers of PE (n = 3), PP (n = 2), polyester (n = 1), and a mixture of PP, polyester, and PVC (n = 1) have been used in radiative experiments apart from unspecified polymers (Ganguly and Ariya, 2019; Geilfus et al., 2019; Revell et al., 2021; Yang and Ma, 2023).
Three climate-relevant endpoints were investigated or speculated by authors–direct RF via aerosolized plastics, indirect RF via changes to cloud properties, and impact on albedo or melting rate of ice/snow. For each endpoint, we summarized how many times an effect was found or not found, and the direction of effect for studies that tested the impact of plastics on climate (Table 4). A decrease in direct radiative forcing (negative effect) results in a cooling effect on climate; vice versa, a warming effect. A decrease in indirect radiative forcing (negative effect) results in a cooling effect on climate; vice versa, a warming effect. An increase in albedo (positive effect) results in a cooling effect on climate - and vice versa, a warming effect–as albedo represents the percentage of incident light that is reflected by a given surface.
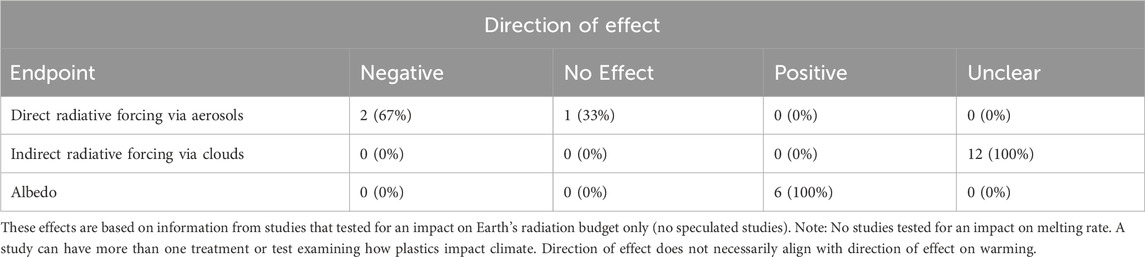
Table 4. Summary of the number of treatments where a negative effect, no effect, positive effect, or unclear effect was found for the listed endpoints. Percentages are shown in parentheses.
Apart from unclear results, there are two instances where studies found that aerosolized plastics decreased direct radiative forcing (cooling effect), and one instance where no significant effect was found (Table 4). To date, no study has found a negative, no effect, or positive effect of plastic on climate through its interaction with clouds (Table 4). All six tests (100%) which investigated the impact of plastics on albedo found that plastics increased the albedo of surfaces or of snow/ice upon incorporation into its bulk–in other words, a negative effect on warming (Table 4). In the case of albedo, studies did not apply a test of significance to their results; they simply reported the percent albedo change observed.
4 Discussion
4.1 Synthesis of current knowledge
4.1.1 GHG emissions from the plastics lifecycle
Current data suggest that most GHG emissions arise during primary plastic production, followed by the product manufacture stage, both at the national and global scales (Table 1). The same trend is reflected in the GHG emissions intensities; however, the emissions intensities associated with waste management can also be quite high depending on which strategy is used (Table 1). These results suggest that in order to decarbonize the plastics lifecycle, the primary production and product manufacture stages need to be prioritized. Ultimately reducing consumption and production of plastics is the most reliable pathway to reducing plastic pollution, waste, and GHG emissions (Bergmann et al., 2022; Borrelle et al., 2020; Zheng and Suh, 2019; Zink and Geyer, 2017). Another strategy for cutting the amount of virgin pellets produced each year is to incentivize the reuse of plastic products, while considering the caveats of reuse presented in Zink and Geyer (to avoid circular economy rebound) (Zink and Geyer, 2017). In addition, more research is needed to understand GHG emissions from the consumption phase of plastic products (e.g., plastic windows and doors) to ensure the reuse of plastic products does not exacerbate climate change (or human health impacts) as well.
The waste management strategy associated with the highest GHG emissions both nationally and globally is incineration, while landfilling is associated with the fewest GHG emissions (Table 2). Incineration has the highest upper-end of emissions intensity ranges, followed by chemical recycling (Table 2). Open dumping also has a high upper-end for its emissions intensity range, perhaps due to the direct release of any GHGs produced into the atmosphere, in contrast to more controlled settings such as advanced landfills. Comparatively, mechanical and certain chemical recycling options have comparatively low lower-end GHG emissions intensities. These reported values suggest that mechanical and certain forms of chemical recycling help to mitigate GHG emissions from the waste management stage of the plastics lifecycle relative to other waste management options (van der Hulst et al., 2022). Some of the reported GHG emissions from chemical recycling can be smaller than those of other waste management strategies, or even completely offset, if GHG emissions savings from the avoidance of virgin pellet production are factored into the calculation (van der Hulst et al., 2022). This was found to be the case for a plastic-to-olefin conversion process commercialized by Synova and Technip Energies (van der Hulst et al., 2022). While recycling of various types may offer some benefits from a GHG standpoint, these benefits need to be weighed against their disadvantages which include contribution of micro- and nano-plastics to the environment during the recycling process, the climate and health impacts associated with these plastic particles, the impacts of toxic chemicals used or produced during the recycling of plastics (Singla, 2022), and associated environmental justice impacts (Bora et al., 2020). Mechanical recycling only delays the inevitable transformation of plastic into waste (Bora et al., 2020; Smith et al., 2022). In addition, most chemical recycling processes create products that are ultimately burned, such as the production of fuels rather than recycled plastics (Singla, 2022).
Although several studies state that they included GHG emissions associated with the transportation and consumption stages in their overall lifecycle emissions estimate (Ding et al., 2023; Goga et al., 2022), the disaggregated emission values were not provided (Table 1). The lack of quantifications of GHG emissions associated with the transportation, consumption, and unmanaged waste stages of the plastics lifecycle are major knowledge gaps for this impact category. For the consumption stage, we lack measurements of GHG emissions from plastic products while in use, including packaging, clothing, and infrastructure; many plastics emit GHGs on an ongoing basis regardless of whether they have been discarded or not, when exposed even once to ambient solar radiation (Royer et al., 2018). For the unmanaged plastic waste stage, we need more estimates of GHG emissions from unmanaged large plastic items, as well as the microplastics that fragment from these larger items. We lack polymer-specific GHG emissions both nationally and globally, as well as polymer-specific GHG emissions intensity estimates for specific waste management strategies. More studies are needed which quantify emissions of black carbon from the open burning of plastic waste to add to the sole study of black carbon emissions from open burning in Guatemala (Cruz et al., 2022). We also need more studies of GHG emissions from the plastics lifecycle at the national scale - information on GHG emissions from the plastics lifecycle are missing for the vast majority of countries (studies have only been done on 14 of 195 countries), excluding those which are continental or global in nature, or with unspecified geographies (Supplementary Figure S2).
4.1.2 Impact of plastics on carbon cycling
The marine ecosystem category had the greatest amount of speculation with respect to the impact of plastics on carbon cycling, and the coastal blue carbon ecosystem category had the least number of studies done to date (Figure 3). The terrestrial ecosystem category had the highest number of studies that tested for an effect on a climate-relevant endpoint by plastics, with 37 studies testing an effect on one or more endpoints compared to only 4 for coastal blue carbon ecosystems and 14 for marine ecosystems (Figure 3) Across all endpoints, the health/integrity of zooplankton endpoint is the most tested endpoint with 323 treatments, followed by the soil carbon content endpoint with 153 treatments. There are mixed results for almost all endpoints, with tests finding both positive and negative impacts on abundance of carbon sequestering strains, carbon fixation capability of plants, soil carbon stores, health/integrity of soil biota, soil respiration, and health/integrity of zooplankton (Table 3). Nonetheless, there is overwhelming evidence that the addition of plastics to soil in turn alters microbial community structures and soil properties, which has implications for climate (Table 3). Plastic debris mostly affect soil biota for the worse. In one instance, the addition to soil of decabromodiphenyl, a flame retardant used in plastics, led to an increase in neurotoxic biomarkers and irreversible tissue damage in earthworms (Eisenia fetida) (Han et al., 2023). The addition of polyethylene and polypropylene spheres in conjunction with the flame retardant exacerbated earthworm biotoxicity (Han et al., 2023).
The addition of plastics to soil increased soil respiration rates in the majority cases, which has a warming effect on climate. The DOC, SOC, and SOM content of soil increased with addition of plastic, suggesting that plastics may help to increase soil carbon stores (and mitigate additional warming), but this finding should be interpreted with caution–first, SOM encompasses more than simply soil organic carbon. Secondly, this finding may be influenced by the degradation process of plastic which leaches carbon into soil and whether researchers corrected for this additional carbon source. At present, this correction is not being done (Kim et al., 2021; Meng et al., 2022; 2023; Pang et al., 2023; Rillig, 2018). Thus, the true ability of plastics to increase the carbon sequestration capacity of soil is uncertain. To rigorously determine the true impact of plastic on soil organic carbon content, studies would need to control for the carbon in plastic and carbon loss from plastic when measuring soil carbon content (Kim et al., 2021; Rillig, 2018).
Excluding non-significant results or results with no effect, two endpoints only have effects in one direction: health/integrity of marine bacteria (warming) and health/integrity of phytoplankton (warming). In addition, studies thus far have only found negative impacts by plastics (warming) on the BCP, but we are limited to a sample size of four studies. Kvale et al. (2023) ran model simulations showing that a decrease in fecal pellet sinking velocities due to microplastics resulted in a reduction in ocean carbon uptake by roughly 4.4 Pg C between 1950 and 2,100. Wieczorek et al., (2019) and Cole et al., (2016) both found that the incorporation of microplastics (between 30 μm and 500 μm) into fecal pellets in the laboratory reduced their settling velocities. Thus far, studies have found that marine microplastics either have no effect or an unclear effect on carbon dioxide drawdown into surface waters. Romera-Castillo et al. (2018) observed that 7 mm PE fragments leach DOC into the ocean, thereby stimulating microbial activity in the ocean. However, it is unclear whether this would lead to an increased drawdown of atmospheric carbon dioxide. Kvale et al. (Kvale et al., 2021) found no effect on atmospheric carbon dioxide drawdown in their model simulating the impact of plastics on zooplankton grazing.
The direction of effect of the majority of tests indicates a tendency to worsen climate change, for the endpoints of: shift towards carbon sequestering strains, health/integrity of soil biota, soil respiration, BCP, and health/integrity of phytoplankton (Table 3). The remaining endpoints were dominated by non-significant or unclear results (carbon fixation via plants, health/integrity of marine bacteria, health/integrity of zooplankton) (Table 3). Summing results together across all endpoints with a direction of effect, there have been 198 warming effects (accelerate climate change) and 94 cooling effects (decelerate climate change) detected. We have found that plastics more frequently have negative effects on carbon sequestration (contributes to additional warming) than positive ones.
The least tested climate-relevant endpoints are the BCP, health/integrity of marine bacteria, and carbon dioxide drawdown endpoints for the carbon sequestration impact category (Table 3). Future studies focusing on these endpoints should be prioritized, as well as endpoints where most of the test results are unclear or insignificant (carbon fixation via plants, health/integrity of marine zooplankton) (Table 3). Our understanding of how microplastics change and affect plant roots, leaves, biomass, photosynthesis, fruits, and hormones is in large part lacking, and more plant species need to be analyzed (Kaur et al., 2022; Li et al., 2024; Mbachu et al., 2021; Palansooriya et al., 2022; Qiang et al., 2023; Rillig et al., 2021). Experiments will contribute knowledge to the effects of plastics on climate, and improve modelling efforts by informing model parameters (Kvale et al., 2023). Few studies had experimental designs longer than a year, or which were evaluated in the field, instead of in a controlled environment. We need more field experiments as well as experiments that are longer in duration so that our understanding of the impacts of plastics is not biased by the results of short-term studies.
Pellet and foam morphologies have not been used in experiments investigating the impact of plastics on terrestrial carbon sequestration; however, these morphologies are present in nature. Pellet spills from industry are commonplace (Tsui et al., 2020), and foam particles may be shed through the breakdown of foam products including polystyrene food containers and insulation (Gao et al., 2023). For marine studies, experiments deploying fiber morphologies are missing in addition to pellet and foam, even though fibers are the most abundant type of microplastic in the environment by count (Cole et al., 2016; Ross et al., 2021; Zhu et al., 2021; Zhu et al., 2019). More studies deploying a variety of microplastic morphologies as well as mixtures of morphologies–in particular aged plastics - are critical to accurately evaluate the impact of plastic pollution on carbon sequestration. Furthermore, to improve harmonization of data, either a consistent unit of microplastic concentration should be used across studies, or studies should report microplastic concentrations in both units of mass (e.g., 1% w/w) as well as units of count (e.g., particles/kg dry weight).
4.1.3 Impact of plastics on Earth’s radiation budget
Two studies found a negative impact on surface/ice albedo by plastics, while one study found that plastic exerts either no effect or a negative effect on direct RF via aerosols (Table 4). The role of plastics in indirect RF via interaction with clouds is the least understood subcategory within radiative impacts (Table 4). One study found that polypropylene and polyethylene particles can act as ice nucleating particles across all their treatments (Ganguly and Ariya, 2019); however, clouds play different roles in climate depending on their altitude (Intergovernmental Panel on Climate Change, 2021). Therefore, this result is not enough to determine whether the interaction of plastics with clouds has a warming or cooling effect on the planet (Table 1). At present, the data suggests that the interaction of plastics with radiation primarily seeks to cool the planet. This aligns with the net effect of aerosols on Earth’s climate, which is also a cooling effect (Intergovernmental Panel on Climate Change, 2021).
There are several instances of speculation across all three subcategories of impacts within the radiation category (Figure 4). In particular, the impact of plastics on cloud properties is the most poorly understood subcategory of impacts within the radiation impact category. Compared to the body of knowledge surrounding GHG emissions across the plastics lifecycle and impacts of plastics on carbon sequestration by Earth’s natural carbon sinks, the radiative impacts of plastics are the least understood category of impacts. The majority of studies are field studies or secondary articles that hypothesized an impact without testing for an effect (19 of 24 studies).
At present, the magnitude of direct radiative forcing of plastics is negligible relative to the net human-induced RF of 2.3 (1.1–3.3) W/m2 for the period of 1750–2011, in fact being three to four orders of magnitude smaller (Intergovernmental Panel on Climate Change, 2021; Revell et al., 2021). However, future work should focus on sampling aerosolized plastics and plastics in clouds over a greater spatial area, and over a longer timeframe, in order for more RF estimates to be made. At the moment, studies typically collect samples of plastic aerosols collected in a fixed location for a snapshot in time. The two studies examining the impact of plastics on the albedo of surfaces both found that the presence of white-colored or a mixture of different colors of plastics (blue, yellow, purple, and translucent) increases albedo (Fan et al., 2014; Geilfus et al., 2019). A 0.01 increase in albedo roughly translates into a −2.9 to −1.3 W/m2 change in radiative forcing of the surface, on the same order of magnitude as the net human-induced RF for 1750–2011 (Intergovernmental Panel on Climate Change, 2021; Xu et al., 2015). The change in albedo observed was >0.01, indicating the potential for plastic to influence the radiative forcing of surfaces/ice depending on how much of our planet is covered in plastics, and how much plastics have infiltrated into ice. The incorporation of microplastics into sea ice may create impurities that increase the diffuse reflection of light from ice and increase albedo (Geilfus et al., 2019). To support the findings of these studies, more RF calculations are needed, in particular taking into consideration mixtures of different plastic morphologies, sizes, and colors to represent the concoction of microplastics we see in nature. Overall, we need more studies to be conducted in the radiative category that test an effect, including more experimental studies examining how plastics affect the albedo and melting rate of ice/snow matrices, how plastic alters the albedo of surfaces such as grassland and soil, how plastic affects the number and size of cloud condensation nuclei relative to a control, and what RF is associated with aerosols generated from the burning of plastic waste to corroborate existing studies and better understand how plastics are altering the Earth’s radiation budget.
4.2 Summary and outlook, including future research to fill knowledge gaps
The current body of evidence shows that plastics are impacting our climate in a multitude of ways. Up to two gigatonnes of GHG emissions (1%–2% of Earth’s carbon budget under a 1.5°C target) are estimated to come from the plastics lifecycle each year based on current available information, which will exacerbate climate change. The infiltration of plastic particles into soil, water, and biota are affecting the sequestration of carbon by natural sinks, with more warming effects than cooling effects reported at present. On the contrary, the majority of directional effects from the interaction of plastics with radiation are cooling in nature. However, this body of evidence is incomplete, with numerous knowledge gaps, unclear results, and instances where researchers did not detect a significant effect. Moving forward, more research is needed to fill existing knowledge gaps in order to gain a comprehensive understanding of the role that plastics play in climate change.
For the lifecycle category, more studies on GHG emissions are needed across all stages of the plastics lifecycle, in particular at the transportation, consumption, and unmanaged waste stages. We need to continue quantifying estimates of GHG emissions and GHG emissions intensities across the plastics lifecycle for specific polymers. Furthermore, more estimates of emissions of black carbon from the open burning of plastics are needed, as well as studies conducted at the national level.
For the carbon cycling category of impacts, more studies focusing on the climate impacts of plastics on coastal blue carbon ecosystems are needed. Calculations of effect sizes are necessary for all endpoints in order to explore the effect of explanatory variables (concentration, morphology, polymer type, particle size) on the resulting effect. A pooled estimate of the percentage of SOC loss from soils globally is critical, as this number directly informs how much of Earth’s remaining carbon budget plastics consume through its impact on the terrestrial carbon sink. Marine endpoints are understudied and require more testing by deploying microplastic morphologies of all types, but in particular foam, pellet, and fiber morphologies. In addition to experiments involving a single plastic polymer of a certain size and morphology, researchers should deploy a diverse mixture of various types of plastic particles in soil, ecotoxicology, and ice/snow albedo experiments, that are representative of what is observed in nature, and at environmentally-relevant concentrations to accurately determine how plastic is impacting the climate. Field experiments beyond the lab are necessary to verify that what we are seeing in the lab occurs in nature (Goddijn-Murphy et al., 2023).
In terms of the radiative impacts of plastics, more calculations of entities that directly inform how plastics contribute to global temperature rise (i.e., albedo, radiative forcing estimates) are needed. Finally, the field is in need of more studies that examine how micro- and nano-plastics affect cloud properties including lifetime and brightness, and how much plastic and what types are getting into clouds of different kinds, at different altitudes. Increasing the geographic scale of plastic aerosol, cloud, and ice sampling will help in modelling and RF calculations, as well as with quantifying the magnitude of effects over time.
Compared with the impact of plastics on climate through its lifecycle GHG emissions, there is more speculation around its carbon cycling and radiative impacts. While speculated effects are not supported by evidence, this does show that there is more attention being given to the role that plastics may play in exacerbating climate change.
4.3 Policy solutions presented by studies
While the body of evidence with respect to the impact of plastics on climate is incomplete, it is clear that there is already damage being done by plastics to ecosystems, biota, and humans and that plastics are impacting our climate. The impact of plastics on climate needs to be mitigated, and policy solutions have been presented by studies across all three categories of impacts. A reduction in our dependence on virgin pellet production will help reduce GHG emissions from the plastics lifecycle, as will shifting the plastics supply chain to a cleaner energy grid and employing other decarbonization processes (Astrup et al., 2009; Cabernard et al., 2021; Fruergaard et al., 2009; Goga et al., 2023). Policies can even target stages of the plastics lifecycle before virgin pellet production, e.g., disincentivize the extraction of fossil fuels and the expansion and construction of existing and new plastics and related petrochemical facilities. Waste management approaches need to be chosen wisely considering their potential to emit GHGs, but also considering their health effects–for instance, while certain forms of chemical recycling may have the potential to reduce GHG emissions, they pose a myriad of risks to ecosystems (Bora et al., 2020). And while open burning is a relatively convenient waste management option, it is extremely detrimental to the surrounding environment and the health of the people who live in the vicinity (Wu et al., 2021). Landfilling minimizes GHG emissions both nationally and globally, but not all landfills are designed and constructed adequately, landfill space is getting scarce, and ultimately we need to reduce our dependence on plastic material and stop generating so much waste of all kinds in the first place (Borrelle et al., 2017; Ellen MacArthur Foundation, 2017).
Although GHG emissions inventories of the Intergovernmental Panel on Climate Change (IPCC), the world’s foremost authority on climate change, consider GHG emissions from the oil and gas industry, it is not clear which stages of the plastics lifecycle are being taken into consideration when deriving these emissions estimates (Intergovernmental Panel on Climate Change, 2021). The most recent IPCC report does not mention plastics or plastic pollution, and thus at present, the impact of plastics on Earth’s natural carbon sinks and Earth’s radiation budget are not being considered in IPCC scenarios (Intergovernmental Panel on Climate Change, 2021). GHG emissions scenarios and policymaking need to recognize the interactions between plastics and climate, in order to achieve effective mitigation on both fronts (Intergovernmental Panel on Climate Change, 2021; Zhu, 2021b).
Further policies can be implemented to address the spreading, concentration, and mitigation of microplastics. In soils, for instance, a framework that connects measured soil concentrations of plastics to management actions by comparing concentrations against toxicity thresholds can be developed for microplastic concentrations in soil, the way it has been done for microplastic in water (Mehinto et al., 2022). Characterizing the sources and pathways of aerosolized microplastics and aerosols produced from the burning of plastic waste is essential, and limiting exposure is critical as they can pose respiratory health risks similar to those posed by PM2.5 and PM10 (Rosso et al., 2023).
Interestingly, researchers who investigated the impacts of plastics on zooplankton health warn against replacing plastic with a regrettable substitution, without fully understanding the impacts of the alternative options (Beiras et al., 2018). On this note, numerous studies suggest the replacement of conventional plastics with bioplastics as a way of limiting toxicity to biota, as well as reducing GHG emissions since their emissions are offset by the carbon sequestered by the crops during growth (for bio-based plastics) (Goga et al., 2023; Maity and Pramanick, 2020). However, some studies have found that bioplastics (see section on Bioplastics for definition) can be just as toxic as conventional polymers (Zimmermann et al., 2020). In addition, the carbon neutrality benefits of bio-based plastics (a type of bioplastic) may be cut short by the emissions associated with land use change, to grow the crops to manufacture them in the first place (Ford et al., 2022; Piemonte and Gironi, 2012). The viability of bioplastics as a method of reducing GHG emissions from the plastics lifecycle without unintended consequences requires further investigation.
4.4 Limitations of this review
We set a cut-off date of 29 August 2023 for study inclusion so that we could begin data analysis. To minimize risk of bias, we excluded non-peer reviewed literature from this review including books, book chapters, and reports. Many of these resources contain information on the impact of plastics on climate - in particular information on GHG emissions from the plastics lifecycle; however, they were not subject to an assessment of validity and quality by scientists [e.g., (Bauer et al., 2022; Bylsma et al., 2023; Cullen et al., 2022; Gaia, 2022; Geyer, 2020; Gordon et al., 2022; GRID-Arendal, 2024; Hamilton et al., 2019; International Energy Agency, 2018; Karali et al., 2024; O’Farrell and Pickin, 2023; Rubio-Domingo et al., 2022; Vallette, 2021)]. Having read through all of these reports, it was confirmed that many of them are based on peer-reviewed literature that we had already included in our study [e.g., (Rubio-Domingo et al., 2022)], or it is unclear how they arrived at their GHG emissions estimates [e.g., (Center for International Environmental Law, 2019)].
We narrowed the scope of our review due to time and resource limitations. Specifically, we only focused on the GHGs of carbon dioxide and methane. We do note that certain plastics, depending on feedstocks and environmental conditions, can emit other GHGs as well, such as ethylene (Royer et al., 2018) and nitrous oxide emissions from adipic acid production to make nylon (Environmental Protection Agency, 1990). We only focused on marine and terrestrial carbon sinks, and excluded freshwater ecosystem studies, as freshwater ecosystems do not constitute prominent carbon sinks in comparison to other ecosystems. We did not examine the impact of (intact) plastic mulch used in agriculture - which are large sheets placed over crops to retain moisture–on climate. We also did not include studies on GHG emissions from plastic composting, industrial or residential, as this pertains to bioplastics waste management strategies. In addition, there are other ways in which plastics may exacerbate the consequences of climate–for instance, plastic waste may clog storm drains and aggravate the flooding of urban areas, as well as have social and environmental justice impacts (MacAfee and Löhr, 2024). These interactions were not investigated in this review, as we solely focused on the role that plastics play in causing climate change, not in aggravating its ensuing consequences.
Furthermore, there are limitations to the body of evidence we collected as each experiment was conducted under different conditions, using different types of plastics, and using different concentrations of plastics. As a result, we chose to focus on direction of effect, as opposed to magnitude of effect. Some of the plastic concentrations used in experiments can be many times greater than what is environmentally-relevant [e.g., (Cole et al., 2016; Galgani et al., 2023; Geilfus et al., 2019)]. Thus, there may be a risk of bias if results are used to interpret how plastics are impacting the climate at present. However, high concentrations are useful for demonstrating what type of effect would exist (if one were to exist), and can inform us of effects that would occur under future scenarios of plastic contamination.
5 Conclusion
We conducted a comprehensive systematic review to answer the question of how plastics are impacting our climate. We performed a qualitative and quantitative synthesis of the existing data to identify the current state of knowledge regarding the impact of plastics on climate across three major impact categories - GHG emissions from the plastics lifecycle, impacts to natural carbon sinks, and impacts to Earth’s radiation budget. We disentangled demonstration of effects from speculation and highlighted general directions of effect within the three impact categories, to inform what we know and do not know about the impact of plastic pollution on climate change. Emissions of GHGs from the plastics lifecycle will exacerbate climate change, with the pellet production and product manufacture stages emitting the most GHGs across all lifecycle stages given the data that exists to date. Incineration produces the highest GHG emissions both at the national and global scale, while landfilling accounts for the least GHG emissions out of all the waste management strategies assessed to date. Our analyses on carbon cycling endpoints identified more accounts of warming impacts than cooling impacts, while we found that reported impacts of plastics on radiative endpoints have a predominantly cooling effect.
We found that the lifecycle GHG category has the most evidence of impact on climate, while radiative impacts are the least understood category of impacts. GHG emissions from the transportation, consumption, and unmanaged waste stages of the plastics lifecycle are the least understood out of all the stages. For the carbon cycling impacts, the climate-relevant endpoints with the most data on effects are the microbial community and soil properties endpoints, while the endpoints with the least data pertain to the impact of plastics on the functioning of the carbon pump, carbon dioxide drawdown into surface waters, and health/integrity of marine bacteria. The impact of plastics on clouds is the most poorly understood radiative impact subcategory. We also presented future directions for research to fill in existing knowledge gaps.
To summarize, our suggested future research directions include:
• More quantifications of GHG emissions at all stages of the plastics lifecycle, both nationally and globally, and in particular at the transportation, use/consumption, and unmanaged waste stages
• More estimates of polymer-specific GHG emissions and GHG emissions intensities across the plastics lifecycle both nationally and globally, and for specific waste management strategies, including littering and open dumping
• The production of more experimental and field data on the impact of plastics on understudied carbon cycling endpoints, including its impact on the BCP, the health/integrity of marine bacteria, and carbon dioxide drawdown into surface waters
• The production of more experimental and field data on the impact of plastics on carbon cycling endpoints with conflicting results, in particular carbon fixation via plants and health/integrity of marine zooplankton
• More studies focusing on the climate effects of plastics on coastal blue carbon ecosystems, which necessarily involves an assessment of the impact of plastics on the soil properties, microbial communities, biota, and soil carbon stores of mangrove, seagrass, and salt marsh ecosystems
• Calculation of effect sizes across all carbon cycling endpoints
• Correction for the carbon in plastics from experimental studies that examine the impact of plastics on soil carbon stores
• Calculation of a global mean percent change in soil carbon stores due to the infiltration of plastics into terrestrial ecosystems, to estimate how much of Earth’s remaining carbon budget would be used up via the impact of plastics on terrestrial carbon sinks
• Greater spatial and temporal sampling of plastics in clouds of different altitudes and in the atmosphere, and recognizing that plastic aerosols have become a fraction of existing PM2.5 and PM10 pollution
• Calculations of direct and indirect radiative forcing due to the presence of plastic debris in clouds and in the atmosphere
• Calculation of a mean global average temperature change arising from the radiative impacts of plastics
• More experimental, field, and modelling studies investigating the potential impacts of bioplastics on climate
Policy needs to be implemented to actively address the multitude of negative impacts we are already seeing from plastics. Policies should consider the full lifecycle of plastics, since every step of the plastics lifecycle exacerbates climate change as well as other planetary challenges (Villarrubia-Gómez et al., 2024). Emerging technology and innovation will likely play a role in solutions (Mohanty et al., 2024; Samal and Das, 2024; 2023). For instance, technological innovation will be unavoidable if we want to repair our flawed recycling system. However, the adoption of new technologies for reducing the climate impacts of plastics should always be accompanied by rigorous environmental impact assessments, to ensure that its adoption to ameliorate the plastic pollution problem does not exacerbate other environmental, justice, and human health challenges.
In summary, policy implementations that are needed to address the impact of plastics on climate include:
• Mitigation of the climate impacts of plastics across its entire lifecycle, and policy frameworks that reinforce the need for lifecycle approaches
• Policies which seek to reduce our dependence on plastics, especially virgin plastics, as early on in the plastics lifecycle as possible including at the fossil fuel extraction stage; these policies may include curbing the expansion of existing plastics and petrochemical plants and ending the construction of new ones; ending the use of non-essential plastics; incentivizing and mandating reduction, reuse, and refill systems; and promoting green chemistry and alternatives to harmful plastics
• Decarbonization of the plastics lifecycle including through a shift toward cleaner energy grids
• Prioritizing waste management strategies for plastics with low climate impacts, including landfilling and recycling, while considering their benefits and shortcomings holistically–recycling can become a more appealing option if major technological innovations and standardization of materials and designs are implemented that reduce recycling’s current downsides, including the generation of micro and nanoplastics that end up in air, water, soil, flora, and fauna, including human bodies
• Monitoring frameworks and programs that fill in the gaps in our understanding of the sources, transport, distribution, and fate of plastics in terrestrial and aquatic ecosystems, and in the atmosphere, and link them to management actions
• Policies that encourage, incentivize, and enforce such management actions, such as the regulation of local point sources of plastic debris
• Full accounting of the climate impacts of plastics in IPCC reports–including production of an IPCC Special Report on the climate impacts of plastics; IPCC GHG emissions scenarios, GHG emissions inventories, climate models, and consideration/incorporation of the climate impacts of plastics in local, national, international, and entity climate policies
The body of evidence of the impacts of plastics on climate is growing, but incomplete. We hope researchers will continue to build this body of evidence in future work, in order to fully understand how these two issues interact with one another, and ultimately enable a reasonable estimate of the cumulative effect of plastics on global average temperature rise. Doing so will allow us to address each issue more effectively, thereby helping to ensure the sustainability of our wildlife, our planet, and ourselves.
Data availability statement
The datasets presented in this study can be found in online repositories. The names of the repository/repositories and accession number(s) can be found below: https://figshare.com/s/524b8d2c5bba504a2fec.
Author contributions
XZ: Conceptualization, Data curation, Formal Analysis, Investigation, Methodology, Project administration, Software, Supervision, Writing – original draft, Writing – review and editing. JK: Formal Analysis, Investigation, Writing – original draft, Writing – review and editing. HK: Conceptualization, Funding acquisition, Project administration, Writing – original draft, Writing – review and editing.
Funding
The author(s) declare that financial support was received for the research and/or publication of this article. XZ acknowledges funding from the Vanier Canada Graduate Scholarship and the Banting Postdoctoral Fellowship. Research and production of this paper was undertaken as part of the Plastics and Climate Project, which also provided financial and in-kind support (www.PlasticsAndClimate.com).
Acknowledgments
The authors and the Plastics and Climate Project wish to thank the following people for reviewing the draft manuscript and providing input: Dr. John Doherty, Cecilia Diedrich, Dr. Lisa Erdle, Dr. Dan Lashof, Dr. Kara Lavender Law, Cindy Matuch, Molly Morse, Dr. Chelsea Rochman, Dr. Usha Pillai, Ieva Rucevska, and Natalia Skripnikova.
Conflict of interest
The authors declare that the research was conducted in the absence of any commercial or financial relationships that could be construed as a potential conflict of interest.
Generative AI statement
The author(s) declare that no Generative AI was used in the creation of this manuscript.
Publisher’s note
All claims expressed in this article are solely those of the authors and do not necessarily represent those of their affiliated organizations, or those of the publisher, the editors and the reviewers. Any product that may be evaluated in this article, or claim that may be made by its manufacturer, is not guaranteed or endorsed by the publisher.
Supplementary material
The Supplementary Material for this article can be found online at: https://www.frontiersin.org/articles/10.3389/fenvs.2025.1563488/full#supplementary-material
Abbreviations
LLDPE, Linear low-density polyethylene; LDPE, Low-density polyethylene; PE, Polyethylene; PMMA; Polymethyl methacrylate; PP, Polypropylene; PS, Polystyrene; PVC, Polyvinyl chloride.
References
Allen, D., Allen, S., Abbasi, S., Baker, A., Bergmann, M., Brahney, J., et al. (2022). Microplastics and nanoplastics in the marine-atmosphere environment. Nat. Rev. Earth Environ. 3, 393–405. doi:10.1038/s43017-022-00292-x
An, J., Wu, F., Wang, D., and You, J. (2022). Estimated material metabolism and life cycle greenhouse gas emission of major plastics in China: a commercial sector-scale perspective. Resour. Conserv. Recycl 180, 106161. doi:10.1016/j.resconrec.2022.106161
Astrup, T., Fruergaard, T., and Christensen, T. H. (2009). Recycling of plastic: accounting of greenhouse gases and global warming contributions. Waste Manag. Res. 27, 763–772. doi:10.1177/0734242X09345868
Bauer, F., Kulionis, V., Oberschelp, C., Pfister, S., Tilsted, J. P., Finkill, G. D., et al. (2022). Petrochemicals and climate change: tracing globally growing emissions and key blind spots in a fossil-based industry, IMES/EESS report.
Beiras, R., Bellas, J., Cachot, J., Cormier, B., Cousin, X., Engwall, M., et al. (2018). Ingestion and contact with polyethylene microplastics does not cause acute toxicity on marine zooplankton. J. Hazard Mater 360, 452–460. doi:10.1016/j.jhazmat.2018.07.101
Bergmann, M., Almroth, B. C., Brander, S. M., Dey, T., Green, D. S., Gundogdu, S., et al. (2022). A global plastic treaty must cap production. Science 376, 469–470. doi:10.1126/science.abq0082
Bermúdez, J. R., Metian, M., Oberhänsli, F., Taylor, A., and Swarzenski, P. W. (2021). Preferential grazing and repackaging of small polyethylene microplastic particles (≤5 μm) by the ciliate Sterkiella sp. Mar. Environ. Res. 166, 105260. doi:10.1016/j.marenvres.2021.105260
Bora, R. R., Wang, R., and You, F. (2020). Waste polypropylene plastic recycling toward climate change mitigation and circular economy: energy, environmental, and technoeconomic perspectives. ACS Sustain Chem. Eng. 8, 16350–16363. doi:10.1021/acssuschemeng.0c06311
Borrelle, S. B., Ringma, J., Lavender Law, K., Monnahan, C. C., Lebreton, L., McGivern, A., et al. (2020). Predicted growth in plastic waste exceeds efforts to mitigate plastic pollution. Science 369, 1515–1518. doi:10.1126/SCIENCE.ABA3656
Borrelle, S. B., Rochman, C. M., Liboiron, M., Bond, A. L., Lusher, A., Bradshaw, H., et al. (2017). Opinion: why we need an international agreement on marine plastic pollution. Proc. Natl. Acad. Sci. 114, 9994–9997. doi:10.1073/PNAS.1714450114
Bylsma, S., Conway, T., and Fallurin, J. (2023). Addressing plastics’ climate risk. Available online at: https://rmi.org/insight/we-can-cut-petrochemicals-use-today/.
Cabernard, L., Pfister, S., Oberschelp, C., and Hellweg, S. (2021). Growing environmental footprint of plastics driven by coal combustion. Nat. Sustain. 2021, 139–148. doi:10.1038/s41893-021-00807-2
Center for International Environmental Law (1989). Fossil fuels and plastic. Available online at: https://www.ciel.org/issue/fossil-fuels-plastic/(Accessed June 29, 20).
Center for International Environmental Law (2019). Plastic and climate, the hidden costs of a plastic planet. Available online at: https://www.ciel.org/wp-content/uploads/2019/05/Plastic-and-Climate-FINAL-2019.pdf.
Chen, M. M., Nie, F. H., Qamar, A., Zhu, D. hua, Hu, Y., Zhang, M., et al. (2022). Effects of microplastics on microbial community in zhanjiang mangrove sediments. Bull. Environ. Contam. Toxicol. 108, 867–877. doi:10.1007/s00128-021-03429-8
Chung, J.-C., Park, C.-H., Lee, J.-W., and Kim, Y.-J. (2003). Development of institutional system for efficient recycling waste plastic. J. Korea Org. Resour. Recycl. Assoc. 11 (1), 138–148.
Cole, M., Lindeque, P. K., Fileman, E., Clark, J., Lewis, C., Halsband, C., et al. (2016). Microplastics alter the properties and sinking rates of zooplankton faecal pellets. Environ. Sci. Technol. 50, 3239–3246. doi:10.1021/acs.est.5b05905
Crosta, A., De Felice, B., Antonioli, D., Chiarcos, R., Perin, E., Ortenzi, M. A., et al. (2022). Microplastic contamination of supraglacial debris differs among glaciers with different anthropic pressures. Sci. Total Environ. 851, 158301. doi:10.1016/j.scitotenv.2022.158301
Cruz, M. B., Saikawa, E., Hengstermann, M., Ramirez, A., McCracken, J. P., and Thompson, L. M. (2022). Plastic waste generation and emissions from the domestic open burning of plastic waste in Guatemala. Environ. Sci. Atmos. 3, 156–167. doi:10.1039/d2ea00082b
Cullen, J. M., Meng, F., Allen, D., Christopher, P., Hamlin, C., Hamlin, P., et al. (2022). C-THRU: year 1 report carbon clarity in the global petrochemical supply chain, 3 PAGE 2.
Demetrious, A., and Crossin, E. (2019). Life cycle assessment of paper and plastic packaging waste in landfill, incineration, and gasification-pyrolysis. J. Mater Cycles Waste Manag. 21, 850–860. doi:10.1007/s10163-019-00842-4
Ding, H., Liao, S., Tu, D., Hua, P., and Zhang, J. (2023). Trade drives leakage of life-cycle carbon dioxide emissions from plastics in China over 2010–2021. J. Clean. Prod. 417, 137994. doi:10.1016/j.jclepro.2023.137994
Drewniok, M. P., Gao, Y., Cullen, J. M., and Cabrera Serrenho, A. (2023). What to do about plastics? Lessons from a study of United Kingdom plastics flows. Environ. Sci. Technol. 57, 4513–4521. doi:10.1021/acs.est.3c00263
Elbasiouny, H., Mostafa, A. A., Zedan, A., Elbltagy, H. M., Dawoud, S. F. M., Elbanna, B. A., et al. (2023). Potential effect of biochar on soil properties, microbial activity and Vicia faba properties affected by microplastics contamination. Agronomy 13, 149. doi:10.3390/agronomy13010149
Ellen MacArthur Foundation (2017). The new plastics economy: rethinking the future of plastics. Available online at: https://www.ellenmacarthurfoundation.org/the-new-plastics-economy-rethinking-the-future-of-plastics.
Environmental Protection Agency (1990). Inventory of U.S. Greenhouse gas emissions and sinks: 1990-2022. Available online at: https://www.epa.gov/system/files/documents/2024-04/us-ghg-inventory-2024-main-text_04-18-2024.pdf.
Fan, X., Chen, H., Xia, X., and Yu, Y. (2014). Increase in surface albedo caused by agricultural plastic film. Atmos. Sci. Lett. 16, 291–296. doi:10.1002/ASL2.556
Ford, H. V., Jones, N. H., Davies, A. J., Godley, B. J., Jambeck, J. R., Napper, I. E., et al. (2022). The fundamental links between climate change and marine plastic pollution. Sci. Total Environ. 806, 150392. doi:10.1016/j.scitotenv.2021.150392
Fruergaard, T., Astrup, T., and Ekvall, T. (2009). Energy use and recovery in waste management and implications for accounting of greenhouse gases and global warming contributions. Waste Manag. Res. 27, 724–737. doi:10.1177/0734242X09345276
Gaia (2022). Zero waste to zero emissions. Available online at: https://www.no-burn.org/zerowaste-zero-emissions/.
Galgani, L., and Loiselle, S. A. (2021). Plastic pollution impacts on marine carbon biogeochemistry. Environ. Pollut. 268, 115598. doi:10.1016/j.envpol.2020.115598
Galgani, L., Tzempelikou, E., Kalantzi, I., Tsiola, A., Tsapakis, M., Pitta, P., et al. (2023). Marine plastics alter the organic matter composition of the air-sea boundary layer, with influences on CO2 exchange: a large-scale analysis method to explore future ocean scenarios. Sci. Total Environ. 857, 159624. doi:10.1016/j.scitotenv.2022.159624
Ganguly, M., and Ariya, P. A. (2019). Ice nucleation of model nanoplastics and microplastics: a novel synthetic protocol and the influence of particle capping at diverse atmospheric environments. ACS Earth Space Chem. 3, 1729–1739. doi:10.1021/acsearthspacechem.9b00132
Gao, G. H. Y., Helm, P., Baker, S., and Rochman, C. M. (2023). Bromine content differentiates between construction and packaging foams as sources of plastic and microplastic pollution. ACS ES&T Water 3, 876–884. doi:10.1021/acsestwater.2c00628
Geilfus, N. X., Munson, K. M., Sousa, J., Germanov, Y., Bhugaloo, S., Babb, D., et al. (2019). Distribution and impacts of microplastic incorporation within sea ice. Mar. Pollut. Bull. 145, 463–473. doi:10.1016/j.marpolbul.2019.06.029
Geyer, R. (2020). “A brief history of plastics,” in Mare plasticum - the plastic sea, 31–47. Available online at: https://link.springer.com/chapter/10.1007/978-3-030-38945-1_2.
Geyer, R., Jambeck, J. R., and Law, K. L. (2017). Production, use, and fate of all plastics ever made. Sci. Adv. 3, e1700782. doi:10.1126/sciadv.1700782
Goddijn-Murphy, L., Woolf, D. K., Pereira, R., Marandino, C. A., Callaghan, A. H., and Piskozub, J. (2023). The links between marine plastic litter and the air-sea flux of greenhouse gases. Front. Mar. Sci. 10. doi:10.3389/fmars.2023.1180761
Goga, T., Harding, K., Russo, V., and von Blottnitz, H. (2022). What material flow analysis and life cycle assessment reveal about plastic polymer production and recycling in South Africa. S Afr. J. Sci. 118. doi:10.17159/sajs.2022/12522
Goga, T., Harding, K., Russo, V., and von Blottnitz, H. (2023). A lifecycle-based evaluation of greenhouse gas emissions from the plastics industry in South Africa. S Afr. J. Sci. 119, 2–6. doi:10.17159/sajs.2023/13842
Gordon, D., Bylsma, S., and Kirk, T. (2022). Emissions out the gate. Available online at: https://rmi.org/insight/emissions-out-the-gate.
Gradus, R. H. J. M., Nillesen, P. H. L., Dijkgraaf, E., and van Koppen, R. J. (2017). A cost-effectiveness analysis for incineration or recycling of Dutch household plastic waste. Ecol. Econ. 135, 22–28. doi:10.1016/j.ecolecon.2016.12.021
GRID-Arendal (2024). Climate impacts of plastics. Available online at: https://www.grida.no/publications/1023.
Hadley, O. L., and Kirchstetter, T. W. (2012). Black-carbon reduction of snow albedo. Nat. Clim. Chang. 2, 437–440. doi:10.1038/nclimate1433
Hamilton, L. A., Feit, S., Muffett, C., Kelso, M., Rubright, S. M., Bernhardt, C., et al. (2019). Plastic and climate: the hidden costs of a plastic planet.
Han, Y., Fu, M., Wu, J., Zhou, S., Qiao, Z., Peng, C., et al. (2023). Polylactic acid microplastics induce higher biotoxicity of decabromodiphenyl ethane on earthworms (Eisenia fetida) compared to polyethylene and polypropylene microplastics. Sci. Total Environ. 862, 160909. doi:10.1016/j.scitotenv.2022.160909
Hou, X., Mu, L., Hu, X., and Guo, S. (2023). Warming and microplastic pollution shape the carbon and nitrogen cycles of algae. J. Hazard Mater 447, 130775. doi:10.1016/j.jhazmat.2023.130775
Intergovernmental Panel on Climate Change (2021). Clim. Change 2021 Phys. Sci. Basis. Available online at: https://www.ipcc.ch/report/ar6/wg1/downloads/report/IPCC_AR6WGI_FullReport.pdf.
International Energy Agency (2018). The future of petrochemicals. Available online at: https://www.iea.org/reports/the-future-of-petrochemicals.
Jang, Y. C., Lee, G., Kwon, Y., Lim, J. hong, and Jeong, J. h. (2020). Recycling and management practices of plastic packaging waste towards a circular economy in South Korea. Resour. Conserv. Recycl 158, 104798. doi:10.1016/j.resconrec.2020.104798
Karali, N., Khanna, N., and Shah, N. (2024). Climate impact of primary plastic production. Available online at: https://energyanalysis.lbl.gov/publications/climate-impact-primary-plastic.
Kaur, P., Singh, K., and Singh, B. (2022). Microplastics in soil: impacts and microbial diversity and degradation. Pedosphere 32, 49–60. doi:10.1016/S1002-0160(21)60060-7
Kim, S. W., Liang, Y., Lozano, Y. M., and Rillig, M. C. (2021). Microplastics reduce the negative effects of litter-derived plant secondary metabolites on nematodes in soil. Front. Environ. Sci. 9. doi:10.3389/fenvs.2021.790560
Koelmans, A. A., Besseling, E., and Shim, W. J. (2015). Nanoplastics in the aquatic environment. Critical review. Crit. Rev. Mar. Anthropog. Litter, 325–340. doi:10.1007/978-3-319-16510-3_12
Kvale, K., Hunt, C., James, A., and Koeve, W. (2023). Regionally disparate ecological responses to microplastic slowing of faecal pellets yields coherent carbon cycle response. Front. Mar. Sci. 10. doi:10.3389/fmars.2023.1111838
Kvale, K., Prowe, A. E. F., Chien, C. T., Landolfi, A., and Oschlies, A. (2021). Zooplankton grazing of microplastic can accelerate global loss of ocean oxygen. Nat. Commun. 12, 2358. doi:10.1038/s41467-021-22554-w
Leslie, H. A., van Velzen, M. J. M., Brandsma, S. H., Vethaak, A. D., Garcia-Vallejo, J. J., and Lamoree, M. H. (2022). Discovery and quantification of plastic particle pollution in human blood. Environ. Int. 163, 107199. doi:10.1016/j.envint.2022.107199
Li, M., Ma, Q., Su, T., Wang, Z., and Tong, H. (2024). Effect of polycaprolactone microplastics on soil microbial communities and plant growth. J. Polym. Environ. 32, 1039–1045. doi:10.1007/s10924-023-03028-0
MacAfee, E. A., and Löhr, A. J. (2024). Multi-scalar interactions between mismanaged plastic waste and urban flooding in an era of climate change and rapid urbanization. Wiley Interdiscip. Rev. Water 11. doi:10.1002/wat2.1708
Mai, H., Thien, N. D., Dung, N. T., and Valentin, C. (2023). Impacts of microplastics and heavy metals on the earthworm Eisenia fetida and on soil organic carbon, nitrogen, and phosphorus. Environ. Sci. Pollut. Res. 30, 64576–64588. doi:10.1007/s11356-023-27002-4
Maity, S., and Pramanick, K. (2020). Perspectives and challenges of micro/nanoplastics-induced toxicity with special reference to phytotoxicity. Glob. Chang. Biol. 26, 3241–3250. doi:10.1111/gcb.15074
Mbachu, O., Jenkins, G., Kaparaju, P., and Pratt, C. (2021). The rise of artificial soil carbon inputs: reviewing microplastic pollution effects in the soil environment. Sci. Total Environ. 780, 146569. doi:10.1016/j.scitotenv.2021.146569
Mehinto, A. C., Coffin, S., Koelmans, A. A., Brander, S. M., Wagner, M., Thornton Hampton, L. M., et al. (2022). Risk-based management framework for microplastics in aquatic ecosystems. Microplastics Nanoplastics 2, 17–10. doi:10.1186/S43591-022-00033-3
Meng, F., Yang, X., Riksen, M., and Geissen, V. (2022). Effect of different polymers of microplastics on soil organic carbon and nitrogen – a mesocosm experiment. Environ. Res. 204, 111938. doi:10.1016/j.envres.2021.111938
Meng, Q., Diao, T., Yan, L., and Sun, Y. (2023). Effects of single and combined contamination of microplastics and cadmium on soil organic carbon and microbial community structural: a comparison with different types of soil. Appl. Soil Ecol. 183, 104763. doi:10.1016/j.apsoil.2022.104763
Menicagli, V., Balestri, E., Giommoni, F., Vannini, C., and Lardicci, C. (2023). Plastic litter changes the rhizosphere bacterial community of coastal dune plants. Sci. Total Environ. 880, 163293. doi:10.1016/j.scitotenv.2023.163293
Mohanty, C., Samal, A., and Das, N. (2024). Concurrent photoreforming of polyethylene into commercial chemicals and hydrogen generation utilizing g-C3N4/Co3O4 Z-scheme heterostructure: a waste-to-wealth concept. Int. J. Hydrogen Energy 61, 84–93. doi:10.1016/j.ijhydene.2024.02.266
O’Farrell, K., and Pickin, J. (2023). Carbon emissions assessment of Australian plastics consumption - Project report. Available online at: https://assets.wwf.org.au/image/upload/Blue_Environment_Carbon_emissions_of_Australian_plastics_Full_report?_a=ATO2Bfg0.
Page, M. J., McKenzie, J. E., Bossuyt, P. M., Boutron, I., Hoffmann, T. C., Mulrow, C. D., et al. (2021). The PRISMA 2020 statement: an updated guideline for reporting systematic reviews. BMJ 372, n71. doi:10.1136/bmj.n71
Palansooriya, K. N., Shi, L., Sarkar, B., Parikh, S. J., Sang, M. K., Lee, S. R., et al. (2022). Effect of LDPE microplastics on chemical properties and microbial communities in soil. Soil Use Manag. 38, 1481–1492. doi:10.1111/sum.12808
Pang, X., Chen, C., Sun, J., Zhan, H., Xiao, Y., Cai, J., et al. (2023). Effects of complex pollution by microplastics and heavy metals on soil physicochemical properties and microbial communities under alternate wetting and drying conditions. J. Hazard Mater 458, 131989. doi:10.1016/j.jhazmat.2023.131989
Piemonte, V., and Gironi, F. (2012). Bioplastics and GHGs saving: the land use change (LUC) emissions issue. Energy Sources, Part A Recovery, Util. Environ. Eff. 34, 1995–2003. doi:10.1080/15567036.2010.497797
PlasticsEurope (2017). Plastics - the facts 2017. Available online at: https://plasticseurope.org/wp-content/uploads/2021/10/2017-Plastics-the-facts.pdf.
Poovarodom, N., Ponnak, C., and Manatphrom, N. (2015). Impact of production and conversion processes on the carbon footprint of flexible plastic films. Packag. Technol. Sci. 28, 519–528. doi:10.1002/pts.2118
Qiang, L., Hu, H., Li, G., Xu, J., Cheng, J., Wang, J., et al. (2023). Plastic mulching, and occurrence, incorporation, degradation, and impacts of polyethylene microplastics in agroecosystems. Ecotoxicol. Environ. Saf. 263, 115274. doi:10.1016/j.ecoenv.2023.115274
Ragusa, A., Svelato, A., Santacroce, C., Catalano, P., Notarstefano, V., Carnevali, O., et al. (2021). Plasticenta: first evidence of microplastics in human placenta. Environ. Int. 146, 106274. doi:10.1016/j.envint.2020.106274
Revell, L. E., Kuma, P., Ru, E. C., Somerville, W. R. C., and Gaw, S. (2021). Direct radiative effects of airborne microplastics. Nature 598, 462–467. doi:10.1038/s41586-021-03864-x
Rillig, M. C. (2018). Microplastic disguising as soil carbon storage. Environ. Sci. Technol. 52, 6079–6080. doi:10.1021/acs.est.8b02338
Rillig, M. C., Leifheit, E., and Lehmann, J. (2021). Microplastic effects on carbon cycling processes in soils. PLoS Biol. 19, e3001130. doi:10.1371/JOURNAL.PBIO.3001130
Rochman, C. M., Brookson, C., Bikker, J., Djuric, N., Earn, A., Bucci, K., et al. (2019). Rethinking microplastics as a diverse contaminant suite. Environ. Toxicol. Chem. 38, 703–711. doi:10.1002/etc.4371
Rockström, J., Steffen, W., Noone, K., Persson, Å., Chapin, F. S., Lambin, E., et al. (2009). Planetary boundaries: exploring the safe operating space for humanity. Ecol. Soc. 14, art32. doi:10.5751/es-03180-140232
Romera-Castillo, C., Pinto, M., Langer, T. M., Álvarez-Salgado, X. A., and Herndl, G. J. (2018). Dissolved organic carbon leaching from plastics stimulates microbial activity in the ocean. Nat. Commun. 9, 1430–1437. doi:10.1038/s41467-018-03798-5
Ross, P. S., Chastain, S., Vassilenko, E., Etemadifar, A., Zimmermann, S., Quesnel, S. A., et al. (2021). Pervasive distribution of polyester fibres in the Arctic Ocean is driven by Atlantic inputs. Nat. Commun. 12, 106. doi:10.1038/s41467-020-20347-1
Rosso, B., Corami, F., Barbante, C., and Gambaro, A. (2023). Quantification and identification of airborne small microplastics (100 μm) and other microlitter components in atmospheric aerosol via a novel elutriation and oleo-extraction method. Environ. Pollut. 318, 120889. doi:10.1016/j.envpol.2022.120889
Royer, S.-J., Ferrón, S., Wilson, S. T., and Karl, D. M. (2018). Production of methane and ethylene from plastic in the environment. PLoS One 13, e0200574. doi:10.1371/journal.pone.0200574
Rubio-Domingo, G., Halevi, A., Cannon, C., Greene, S., and Johnson, M. (2022). Making plastics emissions transparent. Available online at: https://ccsi.columbia.edu/sites/default/files/content/COMET-making-plastics-emissions-transparent.pdf.
Samal, A., and Das, N. (2023). Mini-review on remediation of plastic pollution through photoreforming: progress, possibilities, and challenges. Environ. Sci. Pollut. Res. 30, 83138–83152. doi:10.1007/s11356-023-28253-x
Samal, A., and Das, N. (2024). “Shining light on the future: photoreforming (pr) as a solution to plastic trash: a brief review,” in Plastic degradation and conversion by photocatalysis (volume 2): from waste to wealth. Editors A. Kumar, S. Kumar, and L. Singh, 223–248.
Shan, C., Pandyaswargo, A. H., and Onoda, H. (2023). Environmental impact of plastic recycling in terms of energy consumption: a comparison of Japan’s mechanical and chemical recycling technologies. Energies (Basel) 16, 2199. doi:10.3390/en16052199
Shen, M., Ye, S., Zeng, G., Zhang, Y., Xing, L., Tang, W., et al. (2020). Can microplastics pose a threat to ocean carbon sequestration? Mar. Pollut. Bull. 150, 110712. doi:10.1016/j.marpolbul.2019.110712
Singla, V. (2022). Recycling lies. Available online at: https://www.nrdc.org/sites/default/files/chemical-recycling-greenwashing-incineration-ib.pdf.
Smith, R. L., Takkellapati, S., and Riegerix, R. C. (2022). Recycling of plastics in the United States: plastic material flows and polyethylene terephthalate (PET) recycling processes. ACS Sustain Chem. Eng. 10, 2084–2096. doi:10.1021/acssuschemeng.1c06845
Šourková, M., Adamcová, D., and Vaverková, M. D. (2021). The influence of microplastics from ground tyres on the acute, subchronical toxicity and microbial respiration of soil. Environ. - MDPI 8, 128. doi:10.3390/environments8110128
Thompson, R. C., Olsen, Y., Mitchell, R. P., Davis, A., Rowland, S. J., John, A. W. G., et al. (2004). Lost at sea: where is all the plastic? Science 304, 838–839. doi:10.1126/science.1094559
Trainic, M., Flores, J. M., Pinkas, I., Pedrotti, M. L., Lombard, F., Bourdin, G., et al. (2020). Airborne microplastic particles detected in the remote marine atmosphere. Commun. Earth Environ. 1, 64. doi:10.1038/s43247-020-00061-y
Tsui, N., Helm, P., Hruska, J., and Rochman, C. M. (2020). Kicking pellet emissions to the curb. Integr. Environ. Assess. Manag. 16, 788–790. doi:10.1002/IEAM.4294
United Nations (2015). The 17 goals. Sustain. Dev. Available online at: https://sdgs.un.org/goals.
van der Hulst, M. K., Ottenbros, A. B., van der Drift, B., Ferjan, Š., van Harmelen, T., Schwarz, A. E., et al. (2022). Greenhouse gas benefits from direct chemical recycling of mixed plastic waste. Resour. Conserv. Recycl 186, 106582. doi:10.1016/j.resconrec.2022.106582
Vethaak, A. D., and Leslie, H. A. (2016). Plastic debris is a human health issue. Environ. Sci. Technol. 50, 6825–6826. doi:10.1021/ACS.EST.6B02569
Villarrubia-Gómez, P., Carney Almroth, B., Eriksen, M., Ryberg, M., and Cornell, S. E. (2024). Plastics pollution exacerbates the impacts of all planetary boundaries. One Earth. doi:10.1016/j.oneear.2024.10.017
Villarrubia-Gómez, P., Cornell, S. E., and Fabres, J. (2018). Marine plastic pollution as a planetary boundary threat – the drifting piece in the sustainability puzzle. Mar. Policy 96, 213–220. doi:10.1016/j.marpol.2017.11.035
Wang, Q., Feng, X., Liu, Y., Cui, W., Sun, Y., Zhang, S., et al. (2022). Effects of microplastics and carbon nanotubes on soil geochemical properties and bacterial communities. J. Hazard Mater 433, 128826. doi:10.1016/j.jhazmat.2022.128826
Wang, Y., Okochi, H., Tani, Y., Hayami, H., Minami, Y., Katsumi, N., et al. (2023). Airborne hydrophilic microplastics in cloud water at high altitudes and their role in cloud formation. Environ. Chem. Lett. 21, 3055–3062. doi:10.1007/s10311-023-01626-x
Wieczorek, A. M., Croot, P. L., Lombard, F., Sheahan, J. N., and Doyle, T. K. (2019). Microplastic ingestion by gelatinous zooplankton may lower efficiency of the biological pump. Environ. Sci. Technol. 53, 5387–5395. doi:10.1021/acs.est.8b07174
Wright, S. L., and Kelly, F. J. (2017). Plastic and human health: a micro issue? Environ. Sci. Technol. 51, 6634–6647. doi:10.1021/acs.est.7b00423
Wu, D., Li, Q., Shang, X., Liang, Y., Ding, X., Sun, H., et al. (2021). Commodity plastic burning as a source of inhaled toxic aerosols. J. Hazard Mater 416, 125820. doi:10.1016/j.jhazmat.2021.125820
Xu, M., Du, W., Ai, F., Xu, F., Zhu, J., Yin, Y., et al. (2021). Polystyrene microplastics alleviate the effects of sulfamethazine on soil microbial communities at different CO2 concentrations. J. Hazard Mater 413, 125286. doi:10.1016/j.jhazmat.2021.125286
Xu, X., Gregory, J., and Kirchain, R. (2015). The impact of changes to surface albedo on radiative forcing. MIT CSHub.
Yang, L., and Ma, C. (2023). Toward a better understanding of microalgal photosynthesis in medium polluted with microplastics: a study of the radiative properties of microplastic particles. Front. Bioeng. Biotechnol. 11, 1193033. doi:10.3389/fbioe.2023.1193033
Zappitelli, J., Smith, E., Padgett, K., Bilec, M. M., Babbitt, C. W., and Khanna, V. (2021). Quantifying energy and greenhouse gas emissions embodied in global primary plastic trade network. ACS Sustain Chem. Eng. 9, 14927–14936. doi:10.1021/acssuschemeng.1c05236
Zhang, Y. L., Kang, S. C., and Gao, T. G. (2022). Microplastics have light-absorbing ability to enhance cryospheric melting. Adv. Clim. Change Res. 13, 455–458. doi:10.1016/j.accre.2022.06.005
Zhao, S., Mincer, T. J., Lebreton, L., and Egger, M. (2023). Pelagic microplastics in the North Pacific Subtropical Gyre: a prevalent anthropogenic component of the particulate organic carbon pool. PNAS Nexus 2, pgad070. doi:10.1093/pnasnexus/pgad070
Zheng, J., and Suh, S. (2019). Strategies to reduce the global carbon footprint of plastics. Nat. Clim. Chang. 9, 374–378. doi:10.1038/s41558-019-0459-z
Zhu, X. (2021a). The plastic cycle – an unknown branch of the carbon cycle. Front. Mar. Sci. 7, 609243. doi:10.3389/fmars.2020.609243
Zhu, X. (2021b). Plastic is part of the carbon cycle and needs to be included in climate calculations. Conversat. Available online at: https://theconversation.com/plastic-is-part-of-the-carbon-cycle-and-needs-to-be-included-in-climate-calculations-154730.
Zhu, X. (2025). Data files for systematic review as part of the Plastics and Climate Project. Figshare. Available online at: https://figshare.com/s/524b8d2c5bba504a2fec.
Zhu, X., Munno, K., Grbic, J., Werbowski, L. M., Bikker, J., Ho, A., et al. (2021). Holistic assessment of microplastics and other anthropogenic microdebris in an urban bay sheds light on their sources and fate. Environ. Sci. & Technol. Water 1, 1401–1410. doi:10.1021/acsestwater.0c00292
Zhu, X., Nguyen, B., You, J. B., Karakolis, E., Sinton, D., and Rochman, C. (2019). Identification of microfibers in the environment using multiple lines of evidence. Environ. Sci. Technol. 53, 11877–11887. doi:10.1021/acs.est.9b05262
Zimmermann, L., Dombrowski, A., Völker, C., and Wagner, M. (2020). Are bioplastics and plant-based materials safer than conventional plastics? in vitro toxicity and chemical composition. Environ. Int. 145, 106066. doi:10.1016/j.envint.2020.106066
Keywords: plastics, climate, climate change, lifecycle, greenhouse gas emissions, carbon cycling, radiative forcing, plastic pollution
Citation: Zhu X, Konik J and Kaufman H (2025) The knowns and unknowns in our understanding of how plastics impact climate change: a systematic review. Front. Environ. Sci. 13:1563488. doi: 10.3389/fenvs.2025.1563488
Received: 20 January 2025; Accepted: 17 March 2025;
Published: 08 April 2025.
Edited by:
Marco Schiavon, University of Padua, ItalyCopyright © 2025 Zhu, Konik and Kaufman. This is an open-access article distributed under the terms of the Creative Commons Attribution License (CC BY). The use, distribution or reproduction in other forums is permitted, provided the original author(s) and the copyright owner(s) are credited and that the original publication in this journal is cited, in accordance with accepted academic practice. No use, distribution or reproduction is permitted which does not comply with these terms.
*Correspondence: Xia Zhu, YWxpY2Uuemh1QG11bi5jYQ==