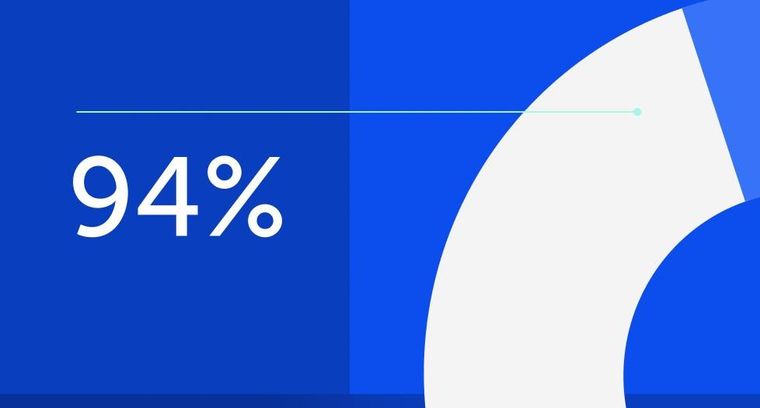
94% of researchers rate our articles as excellent or good
Learn more about the work of our research integrity team to safeguard the quality of each article we publish.
Find out more
REVIEW article
Front. Environ. Sci., 10 April 2025
Sec. Interdisciplinary Climate Studies
Volume 13 - 2025 | https://doi.org/10.3389/fenvs.2025.1525574
This article is part of the Research TopicClimate Change Impacts on Arctic Ecosystems and Associated Climate FeedbacksView all 9 articles
Arctic landscapes occupy a nexus of environmental change processes, globally significant soil carbon stores, wildlife populations, and subsistence-based human societies. In response to rapid climate warming, tundra ecosystems are experiencing widespread changes to vegetation and underlying permafrost, coupled with an array of ecological disturbances that are expected to intensify in the future. Declines in the extent of the cryosphere on land (permafrost and seasonal snow) and offshore (sea-ice) raise the question of whether and for how long warmer portions of the Low Arctic will fit established concepts of “what is Arctic,” given the influence the cryosphere has historically had on tundra ecosystem structure and function. The era of spaceborne observation of circumpolar tundra greenness, in the form of the Normalized Difference Vegetation Index (NDVI), has entered its fifth decade and provides foundational information concerning ecosystem conditions and responses to climatic trends, variability, ecological disturbance, and successional processes. Here we review the evolving story of Arctic greening, and synthesize long-term spaceborne records of NDVI, climatic data, field observations, and the knowledge base of Arctic residents to place the last four decades of Arctic environmental change in context, and establish expectations and research priorities for the coming decade. Greenness dynamics display high spatio-temporal variability, reflecting complex interactions of climatic warming and variability, landscape history, ecological disturbance, and other factors. Nonetheless, long-term increases in NDVI—commonly known as “the greening of the Arctic”—remain prominent across large areas in all available long-term spaceborne datasets and align with long-term shifts in vegetation structure documented in disparate Arctic regions. Common shifts reported from the Low Arctic, such as shrubification, generally portend declines in floristic diversity, and shifts in fauna that favor boreal forest species. Despite lingering uncertainties regarding trend attribution and sources of interannual variability, the sequence of record-high circumpolar tundra greenness values observed since 2020 provides strong evidence that Arctic tundra ecosystems have entered a state without historic precedent on timescales approaching a millennium.
Arctic tundra ecosystems are experiencing a cascade of interwoven changes to vegetation, permafrost, disturbance regimes, hydrological processes, biogeochemical cycling, and wildlife populations that are linked to a rapidly warming climate (Druckenmiller et al., 2024). The Arctic lies at the forefront of global climate warming due to the strong feedbacks that connect atmospheric processes with declining seasonal snow and ice cover, collectively termed “Arctic amplification” (Serreze and Barry, 2011; Rantanen et al., 2022). Few parts of the Arctic have been spared from profound changes in environmental conditions on land and at sea. In turn, these changes feed back to the global carbon cycle and climate, and impact the Arctic’s biodiversity and culturally rich subsistence-based societies.
The Arctic tundra biome spans a broad bioclimatic gradient that includes exceptionally cold, maritime climate regimes in the High Arctic (e.g., mean July air temperature +1.0°C at Krenkel, Franz Josef Land, Russia), to continental Low Arctic environments near the forest-tundra ecotone (e.g., +13.3°C at Bethel, Alaska) (Walker et al., 2005a; Raynolds et al., 2019) (Figure 1). Accompanying this gradient are marked differences in the spatial extent and seasonality of the cryosphere. Permafrost is continuous throughout most of the biome (Brown et al., 2001), but areas rich in ground-ice are experiencing dramatic changes even in the coldest parts of the Arctic and permafrost is becoming increasingly fragmented in southerly locations (Farquharson et al., 2019; Farquharson et al., 2022). Offshore, the extent and duration of seasonal sea ice vary substantially, and high interannual variability in sea-ice extent has become the norm in recent years, with mounting impacts to marine ecosystems (Baker et al., 2020) and coastal communities (Overbeck et al., 2020; Irrgang et al., 2022). Collectively, the responses of tundra ecosystems along bioclimatic gradients provide clues to predict what the future may hold in a warmer, less frozen Arctic.
Figure 1. Ground and aerial perspectives of heterogeneous Arctic landscapes. Photo locations are shown in the central map: (A) Low Arctic tundra in patterned ground landscape, northwestern Siberia; (B) High Arctic tundra with discontinuous vegetation, Franz Josef Land, Russia; (C) mosaic of vegetation and bedrock in recently deglaciated landscape, southwestern Greenland; (D) polygonal tundra with thermokarst pond, North Slope, Alaska; (E) moist tussock tundra, northwestern Alaska; (F) tundra interspersed with waterbodies, Seward Peninsula, Alaska; (G) recently burned tundra with unburned tall shrubs, Andreafsky Hills, Alaska; (H) active-layer detachment, Kolyma River drainage, northeastern Siberia. Extents of the Low and High Arctic regions follow (Raynolds et al., 2019). Photos by (A, C–G) G. V. Frost, (B) D.A. Walker, and (H) L. T. Berner.
Superimposed on large-scale changes to the Arctic climate and cryosphere are complex landscape-scale factors, such as tundra wildfires and other disturbances, hydrologic changes, long-term increases in shrub abundance, and shifts in the distribution and abundance of animals (Jones et al., 2011; Tape et al., 2016; Fauchald et al., 2017; Sae-Lim et al., 2019; Steketee et al., 2022; Webb et al., 2022). These factors can amplify, dampen, or mask the influence of large-scale climatic and cryospheric drivers of tundra ecosystem productivity, or “greenness,” which has been monitored by satellites since 1982 using the Normalized Difference Vegetation Index (NDVI). NDVI is a foundational, if imperfect, vital sign of the terrestrial Arctic. Decades of spaceborne NDVI observations permit investigation of climatic drivers that are subject to high interannual variability and lag effects, as well as landscape disturbances and extreme weather events. Extensive areas of strong increase and localized decline in greenness are evident in multiple satellite datasets (Park et al., 2016; Phoenix and Bjerke, 2016; Potter and Alexander, 2020; Frost et al., 2024), reflecting interactions of climate change and variability, soils, disturbance, and long-term shifts in vegetation structure (Walker et al., 2009; Melvin, 2019; Myers-Smith et al., 2020; Phoenix et al., 2025).
Since the first reports of “Arctic greening” in the late 1990s and early 2000s (Myneni et al., 1997; Zhou et al., 2001; Jia et al., 2003), the long-term satellite record, available data products, and scientific knowledge concerning Arctic environmental change have expanded substantially (Beamish et al., 2020). However, key knowledge gaps and uncertainties remain concerning the historical and future state of tundra ecosystems and their connection to the spatiotemporal variability in greening trends, which serve as a proxy for vegetation change. Here we review the evolving phenomenon of Arctic greening, and synthesize a broad, multi-disciplinary information base that includes long-term spaceborne records of NDVI, climate and sea-ice data, field observations, and the knowledge of Arctic researchers and residents to put the last four decades of Arctic environmental change in context, and address scientific questions, knowledge gaps, and critical information needs for the living Arctic over the coming decades.
Vegetation greenness is an integrative measure of leaf chlorophyll content, leaf area, species composition, and canopy cover and structure. It is thus an essential indicator of tundra ecosystem conditions and responses in a time of rapid environmental change. Numerous spectral vegetation indices exist, all of which exploit the distinctive way in which vegetation absorbs and reflects incoming solar radiation in the visible and near-infrared wavelengths, respectively (Tucker, 1979). Spectral indices apply ratios of reflectance values among bands to help mitigate the effects of sensor calibration, sun-sensor geometry, and topography (Zeng et al., 2022). Here we focus on NDVI because of the long period of record that legacy spaceborne sensors provide (Pinzon and Tucker, 2014). Other spectral vegetation indices include the three-band Enhanced Vegetation Index (EVI; (Huete et al., 2002), other two-band indices such as the Enhanced Vegetation Index 2 (EVI2) (Jiang et al., 2008; Rocha and Shaver, 2009), and derivations of NDVI such as the Near Infrared Reflectance of Vegetation (NIRv) (Badgley et al., 2017), but these lack the period of record of NDVI and their advantages primarily apply in high biomass forest ecosystems.
Maximum NDVI (MaxNDVI) is the annual maximum NDVI value observed during the period of peak phytomass in midsummer, typically in late July and early August for the Arctic. MaxNDVI is a strong indicator of aboveground biomass in tundra (Walker et al., 2003; Raynolds et al., 2012), making it particularly responsive to disturbance, successional processes, and long-term changes in vegetation structure. MaxNDVI has a long history of use, and the application of maximum-value compositing is fundamental to the development of operational NDVI products across temporal scales.
We prepared time series of MaxNDVI through 2023 for the tundra domain of the Circumpolar Arctic Vegetation Map (Raynolds et al., 2019) from four sensors.
1. Advanced Very High Resolution Radiometer (AVHRR), 1982–2023, in the form of the Global Inventory Modeling and Mapping Studies 3 g V1.2 dataset (GIMMS-3g+) with a spatial resolution of 1/12° (∼8 km) (Pinzon and Tucker, 2014; Pinzon et al., 2023).
2. Moderate Resolution Imaging Spectroradiometer (MODIS), 2000–2023, combining 16-day NDVI products from the Terra (MOD13A1, v. 6.1) and Aqua (MYD13A1, v. 6.1) satellites (Didan, 2021a; Didan, 2021b) with a spatial resolution of 500 m, hereafter referred to as MCD13A1. Pixels with a SummaryQA value ≥3 (indicating cloudy or otherwise compromised observations) were masked.
3. Landsat Collection 2 data, 1984–2023, derived from the Thematic Mapper, Enhanced Thematic Mapper, and Operational Land Imager sensors at a spatial resolution of 30 m. We use the approach of Berner et al. (2020) and Berner et al. (2023) to randomly for Landsat’s coarse (non-daily) temporal resolution. Time series plots are based on annual mean MaxNDVI values at sample pixels with ≥16 observations; observations were sparse in the early part of the record for Alaska and eastern Eurasia, so circumpolar trend analysis is only possible after 2000.
4. Visible Infrared Imaging Radiometer Suite (VIIRS), 2012–2023, using the 16-day VNP13A1 product from the Suomi National Polar-orbiting Partnership (S-NPP) satellite (Didan and Barreto, 2018) with a spatial resolution of 500 m.
All data were masked to exclude permanent ice and water. Spatial trend maps depict linear decadal trends with a significance filter of p < 0.1, based on the Pearson’s correlation coefficient (Figure 2). Further details on MODIS and Landsat time series construction are given in Supplementary Material.
Figure 2. Circumpolar MaxNDVI trends from (A) GIMMS-3g+ (1982–2023), (B) MODIS (2000–2023), and Landsat for (C) 1984–2023 (areas with sufficient data density only) and (D) 2000–2023. VIIRS trends are not shown due to the short period of record. Trends are shown in muted colors for the boreal forest biome south to 60° N (GIMMS-3g+ and MODIS only). All plots show the mean August 2023 sea-ice extent and the treeline is indicated by a thin black line.
Time-integrated NDVI (TI-NDVI) is the sum of maximum NDVI values within set compositing periods during May–September, calculated for datasets with daily temporal resolution (AVHRR and MODIS only); this metric is impractical to calculate from Landsat due to that system’s coarse temporal resolution. TI-NDVI has been used since the mid-1980s (Justice et al., 1985; Tucker et al., 1985) and incorporates phenological variations throughout the growing season; therefore, it better represents gross primary production (Tucker and Sellers, 1986) and is typically better correlated with climate variables than MaxNDVI (Bhatt et al., 2010; Bhatt et al., 2021). However, because snow can occur in Arctic environments in any month, variations in TI-NDVI can be expected to reflect interannual variability in seasonal snow cover, primarily in the “shoulder” months of May and September.
We performed trend analysis of TI-NDVI using the spatial and statistical significance filters described above for MaxNDVI (Figure 3). For MODIS, we combined the Terra and Aqua monthly 500 m VI composites (MOD13A3 and MYD13A3 v6.1) to mitigate the imprecise overlap of the twice monthly GIMMS-3g+ and 16-day MODIS compositing periods; we then doubled the resultant values for comparability with GIMMS-3g+. We excluded the 2001 MODIS TI-NDVI value because MODIS Terra was non-functional for part of that summer and MODIS Aqua had not yet launched.
Figure 3. Circumpolar TI-NDVI trends from (A) GIMMS-3g+ (1982–2023) and (B) MODIS (2000–2023). Trends are shown in muted colors for the boreal forest biome south to 60° N. All plots show the mean August 2023 sea-ice extent and the treeline is indicated by a thin black line.
The first reports of Arctic greening emerged in the late 1990s based on the first decade of AVHRR observations (Myneni et al., 1997). Although the earliest reports considered a wide latitudinal band extending well south of the Arctic tundra biome, the modern GIMMS-3g + record shows a series of record or near record-high circumpolar mean MaxNDVI and TI-NDVI values for the Arctic tundra biome beginning in 1987 (Figure 4). This sequence was broken in 1992, concurrent with transient Arctic cooling following the eruption of Mt. Pinatubo the previous year (Lucht et al., 2002); the 1992 record low greenness remains one of the most prominent examples of interannual variability in the GIMMS-3g + record to date. However, four consecutive record-high MaxNDVI values were set during 1997–2000, further strengthening the positive trends reported in early studies (Zhou et al., 2001; Jia et al., 2003). TI-NDVI displayed similar patterns during this period, albeit with a somewhat weaker trend.
Figure 4. Time series of circumpolar mean (A) MaxNDVI for GIMMS-3g+, MODIS, Landsat, and VIIRS; and (B) TI-NDVI for GIMMS-3g+ and MODIS. Linear trendlines for 2000–2023 are shown as solid lines; trendlines outside this period (GIMMS-3g+ and VIIRS only) are shown as dashed lines. Landsat MaxNDVI prior to 2000 is not shown due to large geographic gaps, and the 2001 MODIS TI-NDVI value is excluded because of data gaps that year.
In the ensuing years, causal links were drawn connecting the concurrent trends of Arctic greening, increasing land surface temperatures, and declining spring and summer sea-ice extent (Comiso, 2003; Comiso and Nishio, 2008; Bhatt et al., 2010). Shrub expansion quickly emerged as a likely ecological explanation for Arctic greening (Sturm et al., 2001), consistent with predictions from earlier experimental warming studies (Chapin et al., 1995; Michelsen et al., 1996). Beginning in 2006, the U.S. National Oceanic and Atmospheric Administration’s Arctic Report Card provided annual synopses of Arctic NDVI trends stemming from the International Polar Year “Greening of the Arctic” initiative. Arctic greening became a prominent theme in several international scientific efforts during the 2010s, including Arctic Council initiatives such as the Circumpolar Arctic Flora and Fauna (CAFF) Arctic Biodiversity Assessment (Meltofte, 2013), and the Arctic Monitoring and Assessment Programme (AMAP) Snow, Water, Ice, and Permafrost in the Arctic (SWIPA) report (AMAP, 2017).
By the early 2000s, Arctic greening appeared to enter a period of hiatus after the strong positive trend of preceding decades. This hiatus was particularly evident in the GIMMS-3g + TI-NDVI record (Bhatt et al., 2013). Ecologically, a cessation of greening could occur from (1) Arctic vegetation no longer responding to a warming climate, (2) greening being balanced out by the opposite process of “browning” (i.e., declining biomass and productivity), or (3) a combination of these (Phoenix and Bjerke, 2016). If continued long term, a greening hiatus would have considerable implications for Arctic vegetation change, biogeochemical cycling, surface energy balance and permafrost degradation.
Today, the scenario of vegetation not responding may seem unlikely in light of the magnitude of Arctic warming (Rantanen et al., 2022), but a fact often overlooked is that for much of the satellite record, most of the Arctic showed no statistically significant greenness trend; for example, 51% no change versus 39% greening during 1982–2010 (Xu et al., 2013), and 58% no change versus 37% greening during 1985–2016 (Berner et al., 2020) in the GIMMS-3g+ and Landsat records, respectively. While attribution uncertainty and the inherent limitations of NDVI play a role, if about half of the Arctic shows no spectral trend, could mechanisms favoring stability operate more widely to result in a greening hiatus? A key issue is that stability in Arctic ecosystems has been understudied, with literature overwhelmingly focused on greening rather than browning or stability (Callaghan et al., 2021). While this focus on change is understandable, it may bias our understanding of the precursors and causes of stability.
The alternative idea that browning could offset greening, leading to no net change also has some merit. First, while only about 5% of the Arctic exhibits a browning trend (Xu et al., 2013; Berner et al., 2020), many causes of browning are “pulse” disturbance events that are spatially and temporally discrete (Foster et al., 2022), from which vegetation may recover in just a few years (Racine, 1981; Bokhorst et al., 2012; Olofsson et al., 2012; Bret-Harte et al., 2013) and therefore will not be reflected in long-term trend analyses. Nonetheless, these acute browning events could still disrupt the greening signal. Increases in surface water due to thawing permafrost can decrease NDVI values in areas that are otherwise greening (Raynolds and Walker, 2016). In addition, increases in disturbances such as tundra fires (Hu et al., 2015; Zhu et al., 2023), permafrost thaw (Lewkowicz and Way, 2019; Turetsky et al., 2020; Kokelj et al., 2023), climatic extremes (Vikhamar-Schuler et al., 2016; Rietze et al., 2024), and outbreaks of defoliating insects (Vindstad et al., 2022) could directly connect climate warming with browning events and a more muddled greening signal (Phoenix et al., 2025). For instance, multiple disturbance events came together to result in record low productivity in the Nordic Arctic in 2012 (Bjerke et al., 2014).
Regional browning has also been observed from multi-year “press” mechanisms that halted or reversed regional greening for extended periods (Phoenix and Bjerke, 2016). Examples include periods of greater and longer snow cover reducing springtime greenness in Arctic Alaska (Bieniek et al., 2015), and reduced summer warmth over the Eurasian Arctic (Bhatt et al., 2013). Therefore, despite considerable Arctic warming, the 2000s and early 2010s demonstrated that a greening hiatus is ecologically possible. As we now understand, however, Arctic greening did not stop, and while interannual variability is a key feature of Arctic climate change, a greening hiatus has not become the new normal. The stability and browning processes mentioned above are now best described as sources of complexity that operate against the backdrop of the overall Arctic greening trend (Myers-Smith et al., 2020).
2020 was a benchmark year for Arctic tundra greenness, as record-high circumpolar mean MaxNDVI values were set in all four satellite records (Figure 4), and for TI-NDVI in the MODIS record. These records were concurrent with record-high summer warmth over Arctic lands (see Climatic Drivers of Greening, below). In the years that followed, GIMMS-3g+, MODIS, and Landsat continued to observe a series of record or near-record-high values. For example, the top 3 MaxNDVI values in the 42-year GIMMS-3g + record, 4 of the top 5 values in the 24-year MODIS record, and the top 2 MaxNDVI values in the 24-year circumpolar Landsat record were all recorded during 2020–2023. Interestingly, both GIMMS-3g+ and VIIRS recorded large declines in MaxNDVI from 2020 to 2021, but interannual variability was much lower in the MODIS and Landsat records. On balance, the frequency of exceptionally high greenness values in multiple satellite records provides strong evidence that Arctic peak season live phytomass continues to increase and the greening hiatus has ended.
Long-term records for TI-NDVI present a more confusing picture. While the full 42-year GIMMS-3g + record indicates an overall increasing trend in TI-NDVI of similar magnitude to that recorded by MODIS from 2000 onward, trends over the period of concurrent observation are very different, with GIMMS-3g + indicating weak declines and MODIS indicating strong increases (Figure 4). Although patterns of interannual variability have been qualitatively similar throughout the period of concurrent observations, variability has been much higher in the GIMMS-3g + record, particularly over the last 10 years. Nonetheless, both records indicate that circumpolar TI-NDVI has reached unprecedented highs in the past several years. Like MaxNDVI, the top 2 and top 3 TI-NDVI values in GIMMS-3g+ and MODIS, respectively, were recorded during 2020–2023. Browning and stability remain important, but greening continues, and in light of continued Arctic warming, this seems unlikely to change.
Long-term spaceborne records of tundra greenness and Arctic surface conditions on land and at sea indicate numerous overarching linkages among tundra greenness, sea-ice, precipitation, cloudiness, and other components of the climate system (Raynolds et al., 2008; Bhatt et al., 2017). Spring sea-ice trends and interannual (Bhatt et al., 2010; Dutrieux et al., 2012) to multi-decadal variability (Hendricks et al., 2023; Polyakov et al., 2023) shape the growing season for Arctic tundra, ∼80% of which lies within 100 km of the coast (Walker et al., 2005b). Extended periods of open water are largely responsible for warming air temperatures along the coasts of the Arctic Ocean that, in turn, promote warmer growing seasons onshore that favor increased greenness (Figure 5). Open water (May–August average cover) and summer Warmth Index (SWI, the sum of average monthly temperatures above freezing) have increased steadily since 1982 and circumpolar mean values have reached record or near-record highs during 2020–2023, concurrent with exceptionally high MaxNDVI and TI-NDVI. These correspondences indicate that, in the big picture, the sensitivity of tundra ecosystems to sea-ice conditions and growing season temperature remains strong. Nonetheless, the correlation between sea-ice variability, NDVI, and field-based metrics of plant productivity has decreased over the last decade as sea ice has retreated farther from the coast, (Buchwal et al., 2020; Bhatt et al., 2021), suggesting that other drivers are becoming more important. The influence of sea ice on vegetation also varies regionally, as it is shaped by local oceanic circulation and landscape-scale drivers (Dutrieux et al., 2012; Yu et al., 2021).
Figure 5. Climatology (A) and trend (B) of average May–August Open Water (% cover) based on passive microwave sea-ice observations, and climatology (C) and trend (D) of onshore summer Warmth Index based on ERA5 2 m-air temperature, 1982–2023.
From the climate driver perspective, changes in the hydrological cycle are expected to play an increasing role in tundra greenness dynamics, with precipitation and cloudiness increasing (Liu et al., 2012). As temperature increases and the permafrost active layer thickens, the net result could be increased moisture limitation on plant growth (Haynes et al., 2018). While data on snow and precipitation are less reliable than for sea ice and air temperature, new reanalysis datasets such as the European Centre for Medium-Range Weather Forecasts Reanalysis 5 (ERA5) and GlobSnow (Luojus et al., 2021) provide new opportunities to investigate the changing Arctic hydrological cycle. The last day of snow (snowoff, defined as the last day of the last period of ≥7 days with any snow during 1 January–30 June) is climatologically earlier in North America, but the trend towards earlier snowoff is generally stronger in Eurasia (Figures 6A,B). Climatologically, northwesternmost North America and the Barents and Kara Sea coasts of western Eurasia receive the most summer precipitation, while the eastern North American Arctic receives the least (Figure 6C). Arctic summer precipitation trends are very patchy and generally weak, with increasing interannual variability over the last decade (Figure 7). Where present, trends are mostly positive except in southern Greenland and portions of northeastern Siberia (Figure 6D). As a result, impacts of the changing hydrological cycle are highly variable and regional analysis is more likely to reveal relationships with vegetation.
Figure 6. Climatology (A) and trend (B) of snow-off day, defined as the last day of the last 7-day period with any snow between 1 January and 30 June, and climatology (C) and trend (D) of June–August total precipitation, based on ERA5 reanalysis and climatologies; trends are for 1982–2023.
Figure 7. Time series of average (A) Open Water (%), (B) summer Warmth Index (˚C month), (C) snow-off day (Julian Day), and (D) total June–August precipitation (mm), 1982–2023. Plots are based on microwave sea-ice observations and ERA5 reanalysis data.
Although climatic variability and trends are key overarching drivers of Arctic greenness, NDVI dynamics are strongly influenced by the local circumstances of soils, geomorphology, glacial history, permafrost and snow conditions, disturbance history, and other factors (Figure 8). Therefore, regional perspectives are needed to connect spectral trends with salient drivers and manifestations of change.
Figure 8. Conceptual diagram of interactions among Arctic vegetation, climate, and landscape characteristics and their influence on spaceborne NDVI. Colored arrows indicate key feedbacks that tend to intensify future warming, sea-ice loss, or disturbance (orange); increase tundra productivity (green), or decrease tundra productivity (purple).
Alaska’s North Slope has stood out as a circumpolar “hotspot” of greening virtually throughout the period of spaceborne NDVI monitoring (Jia et al., 2003), yet the eastern Bering Sea sector in southwestern Alaska is one of the most prominent areas of browning in GIMMS-3g + MaxNDVI and TI-NDVI records (Frost et al., 2021). Relationships between MaxNDVI and summer warmth variations have been different in these regions since 1982 (Supplementary Figure 1). In northern Alaska, greenness and SWI share strong increasing trends, but their patterns of interannual variability differ greatly; in southwestern Alaska, trends are much weaker but interannual variability is similar. These contrasts suggest that their responses to climate change are being modified by regional and landscape-scale factors in different ways. Alaska in general, and northern Alaska in particular possesses a rich history of field-based research due to favorable logistics and the presence of long established field stations (Metcalfe et al., 2018), making it an important region for identifying the ecological manifestations, drivers, and impacts of spectral trends.
Seminal studies of Arctic shrubification—increases in the cover, height, and density of woody plants—were focused on Alaska’s North Slope, where retrospective photo comparisons revealed widespread increases in shrub abundance since the mid-20th century (Sturm et al., 2001; Tape et al., 2006). Shrubification soon emerged as one of the most universally recognized mechanisms for greening trends in Alaska and the Low Arctic generally (Myers-Smith et al., 2011), but landscape-scale studies have demonstrated complex patterns of stability and change, with most shrub increase concentrated on or near floodplains (Naito and Cairns, 2011; Naito and Cairns, 2015; Tape et al., 2012; Liljedahl et al., 2020) and in historical tundra fire scars (Jones et al., 2013; Klupar et al., 2021). Findings from western Alaska provide similar conclusions, except that shrubification has also been prominent on hillslopes (Racine et al., 2004; Narita et al., 2015; Salmon et al., 2019; Schore et al., 2023). Retrospective studies of shrubification in Alaska and elsewhere have generally emphasized tall (>∼1.5 m) shrubs such as Siberian alder (Alnus viridis); however, while tall shrublands are conspicuous in tundra landscapes, their overall spatial extent is limited. In contrast, shrub-tussock tundra is far more extensive in Arctic Alaska, but the lower stature and density of shrubs in this community make it much more difficult to demonstrate cover changes. While field studies indicate that NDVI is more sensitive to changes in the leaf area of shrubs than other plant functional types in tussock tundra (Jespersen et al., 2023), and remote sensing time series strongly point toward widespread increases in deciduous shrub abundance on the North Slope (Macander et al., 2022), repeat-monitoring at long-term plots provides mixed indications of phytomass increase and the plant functional types responsible (Jorgenson J. C. et al., 2015; Pattison et al., 2015; Hobbie et al., 2017). Nonetheless, reports of increasing shrub abundance are virtually ubiquitous in discussions with elders and other indigenous residents in Arctic Alaska (Rearden and Fienup-Riordan, 2014; Fienup-Riordan et al., 2021), and provide a compelling line of evidence that extends the observational period associated with remote sensing-based studies.
Repeated ground measurements of NDVI near Utqiaġvik provide uniquely detailed, albeit local, information that implicated changes in herbaceous plant cover at this colder, coastal location (Huemmrich et al., 2023). Transect-based NDVI measurements from circa 2001 and 2022 demonstrated greening linked to increased leaf area in wet sedge tundra, while portions of the transect that exhibited browning had increased standing dead leaf cover in moist graminoid communities. Studies applying handheld NDVI have provided mixed signals concerning the effects of early snowmelt timing and growing season length on tundra productivity, with both positive (Arndt et al., 2019) and negative (Gamon et al., 2013) effects reported.
Ice-wedge degradation has been widely documented in Arctic Alaska in polygonal ground associated with ice-rich permafrost (Jorgenson et al., 2006; Jorgenson et al., 2022; Kanevskiy et al., 2017; Frost et al., 2018a; Jones et al., 2024), where thaw settlement, ponding, and alteration of hydrological pathways produces spatially variable patterns of vegetation mortality, succession, and changes in surface water. Available information indicates this process generally decreases productivity (Lara et al., 2018), though this depends on local circumstances and the time period considered (Raynolds and Walker, 2016); ice-wedge degradation is occurring in many Arctic regions (Liljedahl et al., 2016) and the long-term implications are likely to vary widely (see Current Research Gaps, below). Lake drainage has also been a powerful driver of local greening (Jones et al., 2011; Jones et al., 2020; Nitze et al., 2018), particularly in northwestern Alaska (Nitze et al., 2020), as vegetation colonization and successional changes occur rapidly on newly exposed lacustrine sediments (Chen et al., 2021d). Concurrent beaver expansion in northwestern Alaska promoted pond formation and permafrost degradation (Tape et al., 2018; Tape et al., 2022; Jones et al., 2021; see Long-term Disturbance Effects, below).
The Canadian Arctic encompasses diverse tundra ecosystems ranging from productive tall shrublands north of treeline to partially vegetated barrens in the northern polar desert. In contrast to Alaska and Eurasia, the Canadian Arctic predominantly consists of young (c. 7,000–14,000 years old), postglacial landscapes that were glaciated during the Pleistocene (Margold et al., 2018). MaxNDVI and TI-NDVI trends show considerable variability; MaxNDVI is closely correlated with summer temperature in the Low Arctic, but not in the High Arctic (Supplementary Figure 2). Numerous regional analyses have linked greening patterns with terrain factors including soil moisture, elevation, and surficial geology, and suggest that climate-driven shrub expansion is mediated by the availability of nutrients and mesic soil conditions (Campbell et al., 2021; Chen A. et al., 2021; Seider et al., 2022). Analyses of plot-scale repeat photographs and dendrochronology also point to soil moisture as a key determinant of change (Myers-Smith et al., 2015; Cameron and Lantz, 2016; Bjorkman et al., 2018). Several studies also suggest that spatial heterogeneity is linked to differences in the growth strategies among dominant species (Chen A. et al., 2021; Larking et al., 2021).
Northern Nunavik exhibits some of the strongest greening trends across all sensors; here, repeat photography and field surveys highlight shrubification, especially dwarf birch (Betula glandulosa) (Ropars and Boudreau, 2012; Tremblay et al., 2012). Other regional study areas in continental Canada show more variable greenness trends among sensors; however, retrospective image analysis and fieldwork also document increase in dwarf birch (Fraser et al., 2016; Moffat et al., 2016; Andruko et al., 2020; Davis et al., 2021), Siberian alder (Lantz et al., 2013; Cameron and Lantz, 2016; Travers-Smith and Lantz, 2020), willows (Salix spp.) (Myers-Smith et al., 2019), and evergreen shrubs such as Labrador tea (Rhododendron tomentosum) (Moffat et al., 2016). Field studies suggest that most shrubification has been associated with recruitment (Lantz et al., 2010; Ropars and Boudreau, 2012; Tremblay et al., 2012; Davis et al., 2021), but Bonta et al. (2023) documented change involving vertical expansion and infilling of existing shrub canopies.
NDVI trends vary strongly among all satellite records in the Canadian High Arctic, and few fine-scale investigations exist to help resolve uncertainties (see Current Research Gaps, below). The field studies that do exist highlight a wide range of vegetation responses. These include stability (Bjorkman et al., 2020; Schaefer, 2023); declines in prostrate deciduous shrubs (e.g., Dryas integrifolia) and select forbs (e.g., Bistorta vivipara and Saxifraga oppositifolia) (Schaefer, 2023); and increases in graminoids (Hill and Henry, 2011; Campbell et al., 2021; Schaefer, 2023), forbs (Hill and Henry, 2011; Campbell et al., 2021), shrubs (Hudson and Henry, 2010; Hill and Henry, 2011; Bjorkman et al., 2020; Campbell et al., 2021; Schaefer, 2023), and bryophytes (Hudson and Henry, 2009).
Greenland is the world’s largest island, with a vegetated perimeter predominantly consisting of young (<16,000 years old) postglacial landscapes that encircle the Greenland Ice Sheet (Lecavalier et al., 2014). Strong warming has occurred across Greenland’s wide summer climate gradient (Mayewski et al., 2014), but MaxNDVI and SWI have been poorly correlated during 1982–2023 (Supplementary Figure 3). Overall, Greenland exhibits greening trends for GIMMS-3g + MaxNDVI since 1982, but trends have been more modest in MODIS and strongly negative in Landsat since 2000. However, studies documenting land cover responses to climate change and ice loss from the Greenland Ice Sheet and surrounding glaciers strongly indicate that vegetation cover has increased in proglacial and periglacial areas since the 1980s. Grimes et al. (2024) determined proglacial land cover changes island-wide using Landsat data over the last three decades, reporting ∼28,000 km2 loss in ice cover, a doubling of vegetation cover, a fourfold increase in wetland cover, more meltwater, less bare bedrock, and increased cover of fine, unconsolidated sediment. These changes were strongly correlated with an increased number of growing degree-days, especially >6°C, since the 1980s; however, the absolute rise in temperature had a minimal direct association with these land cover changes. As in other Arctic regions, warming summer temperatures have been linked to increases in shrub abundance, albeit with substantial time lags (Büntgen et al., 2015). In southwestern Greenland, observed shrub increases appear to be partially linked to changes in human land-use and grazing intensity (Jørgensen et al., 2013). Moth outbreaks have also temporarily reduced plant productivity in southern Greenland (Lund et al., 2017). Long-term field studies at Zackenberg, northeast Greenland have revealed variable, species-specific changes in plant cover despite strong summer warming (Hobbie et al., 2017); the observed changes, coupled with interannual variability in plant phenology, appear to be connected to local soil moisture rather than summer temperature (Westergaard-Nielsen et al., 2017).
Svalbard also consists primarily of postglacial landscapes and includes extensive areas of High Arctic tundra in close proximity to ice-covered land. The instrumental record at Svalbard Airport indicates temperatures have increased 0.32°C decade−1 since 1898, about 3.5 times the global average; after 1991 warming accelerated to 1.7 °C decade−1, over twice the average for the Arctic and seven times the global average (Nordli et al., 2020). Warming over the northern Barents Sea has been even faster due to dramatic sea-ice decline; Isaksen et al. (2022) reported temperature increases of up to 2.7 °C decade−1 in northeastern Svalbard. Variations in MaxNDVI have been strongly correlated with increasing summer warmth (Karlsen et al., 2024) (Supplementary Figure 3). In central Svalbard, AVHRR data revealed that MaxNDVI increased 29% during 1986–2015, concurrent with a 59% increase in mean summer temperature (Vickers et al., 2016). However, high interannual variability in greenness in eastern Svalbard is linked to high variability in the timing of sea-ice breakup (Macias-Fauria et al., 2017; Karlsen et al., 2024).
Field measurements of greenness correlate well with MODIS data in Svalbard (Parmentier et al., 2021; Tømmervik and Nilsen, 2023). Regional analysis of MODIS interpolated to daily timescales showed strong correlations with field-based phenological observations, annual productivity, and growing degree days (Karlsen et al., 2018; Karlsen et al., 2022; Karlsen et al., 2024; Karlsen, 2023). Interannual variability in greenness was high, with record-high and intermediate values in 2020 and 2022, respectively, and very low values in 2019 and 2021 (Tømmervik and Nilsen, 2023; Tømmervik et al., 2023).
Historical field plots resurveyed in central Svalbard after up to 85 years revealed increases in graminoids and forbs, consistent with warmer temperatures (Kapfer and Grytnes, 2017). A similar study from central Spitsbergen did not reveal any changes since 1936–1937 that were attributable to climate change (Prach et al., 2010); however, the original surveys were undertaken during an anomalously warm period (Overland and Wang, 2005) and the resurvey was undertaken in 2008, before contemporary warming had started to accelerate.
The northwest Siberian Arctic stands out as a circumpolar greening hotspot in all records and long-term GIMMS-3G + MaxNDVI variations have been well correlated with increasing summer warmth in the region (Supplementary Figure 4). At the landscape scale, trend variability has been connected to differences in substrates, which vary in texture, nutrient status, moisture holding capacity, and ground-ice content; sandy uplands tend to support tundra with lower biomass and weak NDVI trends, whereas strong greening trends tend to overlap clayey marine deposits (Walker et al., 2009; Frost et al., 2014; Tassone et al., 2024).
Regional studies again highlight shrubification as a key form of greening. At the level of individual organisms, tundra willow radial growth has been strongly related to summer temperature and NDVI (Forbes et al., 2010). At the landscape scale, shrubification appears to be more closely connected to upland landscapes than in Alaska, but shares similar connections to ecological disturbances. Cryogenic landslides (i.e., active-layer detachments and retrogressive thaw slumps) are common and have been closely studied on the Yamal Peninsula; after initial loss of biomass, these disturbances become focal points of shrubification and greening (Verdonen et al., 2020). In the southernmost Yamal, upland landscapes support widespread mosaics of sorted and non-sorted circles (i.e., frost boils) that provide similar opportunities for rapid alder expansion (Frost et al., 2013) and have been directly linked to pixel-level Landsat greening trends (Frost et al., 2014). All of these disturbance features facilitate shrub recruitment by enhancing nutrient availability (Ukraintseva and Leibman, 2007), snow cover (Leibman et al., 2015), and soil temperatures (Frost et al., 2018b; Loranty et al., 2018a). Indigenous Nenets reindeer herders further corroborate shrubification, reporting increases in the height, density, and abundance of willows in recent decades, with the tallest shrubs now exceeding the antler height of standing reindeer (Forbes and Stammler, 2009). Shrub increase has had some negative impacts on reindeer herding, as animals can disappear from sight and subsequently be lost during migration.
Thermokarst lakes are widespread in northwestern Siberia and seminal studies of lake drainage in permafrost regions documented this process using Landsat data (Smith et al., 2005). On the Yamal Peninsula, there has been a 5.5% net loss in lake area, with most loss occurring in southerly areas with discontinuous permafrost (Nitze et al., 2018). Plant succession is generally very rapid on newly exposed lacustrine sediments; NDVI can reach values comparable to surrounding tundra within a few years after lake drainage (Liu et al., 2023; Von Baeckmann et al., 2024). Young lake basins tend to be floristically diverse, and lush wetlands are regarded by herders as attractive but hazardous grazing areas for reindeer (Laptander et al., 2024).
Reindeer herding has been practiced on the Yamal for centuries, and the distribution and abundance of reindeer has been linked to regional NDVI patterns (Yu et al., 2011). Regional analysis of Sentinel-1/2 and MODIS data suggest that at current densities, reindeer have substantial impacts on ecosystem structure and can potentially counteract shrubification and other climate-induced vegetation shifts (Spiegel et al., 2023). Nonetheless, reindeer browsing appears to have had little impact on tall shrubs on cryogenic landslides, (Skarin et al., 2020), possibly because animals select for young shoots and low shrubs, rather than older, tall shrubs which tend to be less palatable (Thompson and Barboza, 2014).
Oil and gas development has increased dramatically since the 1970s (Kumpula et al., 2011) and the region’s industrial footprint is among the highest in the Arctic (Bartsch et al., 2021). Industrialization strongly impacts local greenness, first as extensive off-road vehicle tracks and structures during the exploration phase, followed by proliferation of roads, railways, buildings, and pipeline networks. Although these are relatively small linear features within a vast matrix of tundra, cumulative impacts extend beyond the infrastructure footprint, with impacts to reindeer habitat and movements (Kumpula et al., 2011; Kumpula et al., 2012; Yu et al., 2015).
The northeast Siberian Arctic, extending from the Lena River Delta eastward to the Chukchi Peninsula, encompasses a variety of terrain, surficial materials, and climate conditions (Stonevicius et al., 2018), including the Lena River Delta, the Arctic’s largest delta. Greenness trends are very mixed (Figure 4) with no net regional trend and no clear relationships between MaxNDVI and summer warmth (Supplementary Figure 4). Northeastern Siberia has received comparatively little scientific attention (Virkkala et al., 2019), but notably, the Yana-Indigirka Lowland in the central part of the region is one of the Arctic’s most prominent browning hotpots (Figure 2). The region is underlain by continuous permafrost, including extensive ice-rich, late-Pleistocene Yedoma and Holocene alas (drained pond basin) deposits (Veremeeva et al., 2021; Strauss et al., 2022). These landscapes have recently experienced extensive thermokarst and thermal erosion (Nitze et al., 2018; Veremeeva et al., 2021), which create landforms such as baydzherakhs (residual thermokarst mounds) and alases that support highly productive vegetation. Northeastern Siberia has experienced several extreme climatic events that have likely influenced greenness both directly and indirectly in recent years. Severe heat waves occurred in spring and summer 2019–2021, with associated drought conditions and reduced land surface cooling by vegetation (Rietze et al., 2024), increases in wildfire activity, burned area and atmospheric aerosols, permafrost destabilization, and pest outbreaks (Overland and Wang, 2021; Kharuk et al., 2022; Scholten et al., 2022; Talucci et al., 2022).
The Lena River Delta includes a wide variety of surficial materials and landscape settings, and several regional studies have shown corresponding patterns of variability in greenness trends. Overall, the Lena Delta appears to show increases in MODIS MaxNDVI, concurrent with rising summer temperatures and high variability in snowmelt timing. Early snowmelt was not clearly related to midsummer NDVI, but high June temperatures were (Heim et al., 2022). NDVI trends show strong links to fluvial processes, lake dynamics and permafrost disturbances (Nitze and Grosse, 2016), and floodplain areas in particular support highly dynamic disturbance and successional regimes (Lisovski et al., 2023). Overall, lake drainage events have predominated (Nitze et al., 2018). Landsat data for 2003–2022 also show greening on remnant Yedoma deposits, as well as on central and northern parts of the Lena Delta, but no trend in the eastern delta (Nitze et al., 2024).
The browning hotspot formed by the Yana-Indigirka Lowland is distinctive. Regional analysis of MODIS NDVI indicates an episode of browning during 2010–2020. However, annual drone-based observations after 2014 suggest that this hotspot may reflect exceptionally high interannual variability in moisture and surface water extent resulting from extreme weather events, rather than a long-term trend. The subregion is rich in floodplains and drained lake basins; surface water is typically extensive, but high recent variability in surface water appears to be linked to NDVI variability (Magnússon et al., 2021a). Landsat time series showed lower NDVI in dry summers with little rainfall, but also in summers following extreme snowfall, suggesting that high variability in precipitation may help explain regional browning (Magnússon et al., 2023). Extremely high snowfall in 2016–2018 resulted in large spring floods that plausibly explain widespread browning, including tree mortality at the forest-tundra ecotone (Tei et al., 2020; Magnússon et al., 2021b; Nogovitcyn et al., 2023). Marshes of the Indigirka Delta show browning likely caused by coastal flooding, while browning to the west was attributable to wildfires (Talucci et al., 2022; Nitze et al., 2024). Field studies have also documented shrub mortality and increased aquatic vegetation after ice-wedge degradation (Lashchinskiy et al., 2020; Magnússon et al., 2021), highlighting the influence of soil moisture changes (Ohta et al., 2014; Magnússon et al., 2023).
Further east, the Kolyma River watershed experienced greening across ∼20% of its extent from 1982 to 2010; greening was most common in shrublands at higher elevations, where annual productivity was positively correlated with summer air temperatures (Berner et al., 2013). However, more recent time series analyses have shown no substantial trend (MODIS) or even browning (Landsat). These patterns could be partly explained by recent thaw lake expansion; while lake area changed little during 1999–2013, it increased sharply following high precipitation during 2013–2018 (Veremeeva et al., 2021). Similarly, some large floodplains exhibited browning in recent years, likely caused by flooding due to high precipitation.
Chukotka has shown positive MaxNDVI trends across sensors (Figure 2), especially on the Chukchi Peninsula. In central Chukotka, changes in plant aboveground biomass during c. 2002–2017 were modeled by linking field measurements with Landsat satellite data. Plant aboveground biomass widely increased near treeline, particularly with expansion of larch trees and deciduous shrubs. However, few changes were evident in tundra (Shevtsova et al., 2021). Ecosystem models suggest further larch forest densification and expansion into tundra under a warming climate, resulting in loss of tundra-associated biodiversity (Kruse and Herzschuh, 2022; Kruse et al., 2023). However, identified mismatches between NDVI and larch tree density (Loranty et al., 2018b) suggest that relations between larch expansion and NDVI dynamics may be more complex near the treeline. Historical satellite images show increases in both shrub and aquatic vegetation in coastal regions of eastern Chukotka (Lin et al., 2012), and tall shrub expansion in upland landscapes (Frost and Epstein, 2014).
Retrospective assessment of the now 43-year spaceborne record of tundra greenness provides confidence that the “greening of the Arctic” continues, but many questions persist concerning the last several decades of environmental change and the trajectory of the future Arctic that are relevant to societal efforts to anticipate and adapt to these changes.
There is wide consensus that the Arctic greening trend is quite variable across spatial and temporal scales and many areas show little to no significant change (Berner et al., 2020; Myers-Smith et al., 2020; Callaghan et al., 2021). Nonetheless, the long-term record of Arctic tundra greenness provides compelling evidence that in the big picture, “the greening of the Arctic” is an ongoing phenomenon, with the most unequivocal evidence emerging from MaxNDVI trends across the continental Low Arctic. While long-established connections between Arctic greening and concurrent climatic warming and sea-ice decline endure (Berner et al., 2020; Hendricks et al., 2023), they appear to be waning in some regions (Keenan and Riley, 2018). The greatest uncertainties generally concern trend attribution, scaling issues, drivers of regional variation, the strength of seasonality and phenological change over the full growing season, the role of “pulse” events, and the extent to which spectral metrics in general and NDVI in particular can be used to address emerging science questions.
Some sources of NDVI variability and trends are clear—for example, spatially discrete disturbances such as wildfire interact with predominant trends both through initial vegetation mortality and then by the successional processes that follow. However, interactions among disturbances, topography, and warming can create complex long-term patterns (Rocha et al., 2018; Chen et al., 2021b; Gaglioti et al., 2021). Many other sources of variability remain difficult to parse, and can be obscured by inherent technical limitations of spaceborne monitoring (e.g., low spatial resolution and revisit frequency) and the NDVI metric itself (Huang et al., 2021). Although NDVI’s simplicity and long period of record make it integral to Arctic environmental monitoring, it can also oversimplify the complexity of ecosystem dynamics and cannot capture the full scope of changes due to its nonlinear relationship with biomass and resultant saturation effects (Blok et al., 2011; Fraser et al., 2014; Huemmrich et al., 2021). While continued investment and continuity in legacy sensors is crucial, many argue for moving towards more ecologically meaningful metrics, such as solar induced fluorescence (SIF), which is directly linked to leaf chlorophyll concentrations and can potentially serve as an improved proxy for ecosystem gross primary productivity (Cheng, 2024). In addition, the development of hyperspectral sensors and LiDAR capabilities is helping to disentangle heterogenous Arctic ecosystems and explore ecosystem dimensions such as functional traits, biodiversity, and structure (Bjorkman et al., 2018; Thomas et al., 2020; Nelson et al., 2022).
Spatial and temporal scaling issues also continue to limit understanding (Melvin, 2019; Myers-Smith et al., 2020; Nelson et al., 2022). Coarse spatial-scale data cannot capture nuanced changes occurring at smaller scales, while high spatial resolution sensors have traditionally suffered from a lack of temporal resolution that limits time series analysis. New very high resolution commercial systems, such as Planet, offer increased temporal and spatial resolution but lack the long-term record of previous sensors and can be cost-prohibitive. In addition, radiometric and other technical differences among legacy sensors introduce inconsistency among records that can never be fully resolved. For example, the GIMMS-3g + record is invaluable for its long period of record, but pixel-level global area coverage data products are derived from systematic sampling of the original observations. Collectively, these issues call for intercomparison of long-term greenness datasets (Beck et al., 2011; Guay et al., 2014; Liu et al., 2024) to “stress test” hypotheses and conclusions based on one dataset by way of corroboration with others, particularly 30-m resolution Landsat data (Berner et al., 2020; Liu et al., 2021). Improved integration of field-based datasets is also badly needed, particularly outside of traditionally well studied areas (Melvin, 2019). At present, this challenge is greatly exacerbated by barriers to access and collaboration in the Eurasian Arctic arising from the Russian invasion of Ukraine. Even before the escalation of Russia’s invasion in early 2022, the Russian Arctic was greatly underrepresented in English literature. While the Russian scientific community remains active, the curtailment of cross-border collaboration has undermined efforts to integrate Russian science into circumarctic perspectives of Arctic ecosystem conditions and change (Büntgen and Rees, 2023; Zaika and Lagutina, 2023; López-Blanco et al., 2024; Rees and Büntgen, 2024; Schuur et al., 2024). From the perspective of Arctic greening, these circumstances underscore the critical importance of spaceborne remote sensing and the continuity of long-term circumpolar NDVI datasets. Nonetheless, the cessation of field studies complicates the interpretation of underlying drivers and ecosystem impacts of spectral trends, particularly in regions with idiosyncratic trends such as the Indigirka-Yana Lowland of northeastern Siberia (see Section 3.6, above).
There is a sense of surprise regarding some aspects of Arctic change, including the strength and longevity of greening trends, as well as local declines in productivity, the impact of disturbances and extreme events, and the apparent resistance to change in some regions (Phoenix and Bjerke, 2016; Callaghan et al., 2021; Foster et al., 2022). At landscape scales, localized areas with strong trends are often evident that can be attributed to specific processes with high confidence. However, these hotspots are typically interspersed with more subtle trends that are widely distributed across the broader matrix of tundra landscapes that produce the major patterns evident in circumpolar portrayals. Resolution of these uncertainties generally lies at the intersection of coarse-scale time series, high-resolution data, and field observations. Here we highlight several prominent knowledge gaps.
Disturbances such as wildfire, permafrost degradation, and extreme weather events have become increasingly prominent, influencing local to regional greenness directly through mortality, defoliation, or stress (Lara et al., 2017; Sundqvist et al., 2019; Post et al., 2021) or indirectly through the modification of surface properties and vegetation succession (Frost et al., 2020; Verdonen et al., 2020; Chen et al., 2021c). Disturbance impacts on spectral trends are widely acknowledged, and different forms of disturbance can have contrasting short- and long-term impacts (Foster et al., 2022; Phoenix et al., 2025). However, the extent to which “today’s browning portends tomorrow’s greening” in disturbed environments remains an active area of research (Gaglioti et al., 2021; Chen and Lantz, 2024; Chen et al., 2024). Given that many disturbances are expected to intensify, understanding how these will interact with climate-driven greening over the long term is crucial.
Tundra wildfires have emerged as a prominent form of disturbance, particularly in northwestern North America and northeastern Siberia. Combustion of vegetation and organic soil drives abrupt browning of the landscape, but this is followed by intense greening as post-fire succession unfolds (Narita et al., 2015; Chen et al., 2021d), typically resulting in a greener landscape 20–50 years postfire (Rocha et al., 2012; Chen et al., 2021b) and in some cases after more than a century (Jones et al., 2013). The long-term trajectory of postfire vegetation succession determines the magnitude of greening as graminoid-dominated tundra shifts to shrub tundra (Rupp et al., 2000; Racine et al., 2004). Similarly, varied disturbances caused by permafrost degradation initially decrease greenness, but can increase it over long timescales as nutrients, light, and other resources become available to colonizing plants (Lantz et al., 2009; Verdonen et al., 2020). Thermokarst and thermoerosional processes can also trigger catastrophic lake drainage (Nitze et al., 2018; Lara et al., 2021; Jones et al., 2022), in which newly exposed lacustrine sediments support greener landscapes than the surrounding terrain within years (Lantz, 2017; Chen et al., 2021d), an effect that can persist for decades after drainage (Lara et al., 2018; Chen et al., 2023). However, the longevity of this effect appears to be variable across regions (Von Baeckmann et al., 2024).
Biotic disturbances such as herbivory, trampling, and defoliation events can strongly affect local to regional-scale NDVI (Soininen et al., 2021). For example, muskoxen and caribou/reindeer generally decrease greenness by reducing the abundance of deciduous shrubs and overall plant density (Bernes et al., 2015; Sundqvist et al., 2019; Lindén et al., 2021; Spiegel et al., 2023). Small mammals can also influence greenness; Olofsson et al. (2012) linked interannual declines in NDVI with intense defoliation events within experimental plots in northern Sweden. Similar patterns have been observed in northern Alaska, with anomalous phenological patterns in NDVI and other productivity metrics with lemming population booms (Zhang et al., 2023). Nonetheless, links between spaceborne NDVI and rodent herbivory is difficult to detect due to the high spatiotemporal variability (Siewert and Olofsson, 2020) of microtine rodent boom-bust population dynamics (Pitelka and Batzli, 2007).
Human disturbances also influence regional NDVI trends. For example, at least 465 km2 have been directly affected by infrastructure construction since 2000 along Arctic coastlines, evident as NDVI decline in Landsat time series (Bartsch et al., 2021). Although the initial effects of infrastructure development are obvious, cumulative impacts can affect areas well beyond the footprint of infrastructure and require decades to play out (Raynolds et al., 2014). In addition, disused roads, mining activities, and other infrastructure can support greening (Cameron et al., 2024). With an increasing rate of almost 5% per year, human industrial activity in the Arctic is becoming increasingly important. Finally, atmospheric nitrogen deposition from industrial sources outside the Arctic could play an increasingly significant, but little explored role in altering Arctic nitrogen cycles (Choudhary et al., 2016; Liu et al., 2018).
Rain-on-snow (ROS) and other extreme weather events are known to impact regional NDVI trends, yet the timing, footprint, and long-term impacts of these events remain difficult to predict (Serreze et al., 2021). For example, the effects of ROS vary depending if the snowpack is above or below the vegetation canopy. Although ROS is expected to become more frequent, no clear trends in the frequency of mid-winter events are evident from satellite data in the last two decades. Nonetheless, there have been several high impact events in northwestern Siberia, Alaska, and Svalbard (Bjerke et al., 2017; Bartsch et al., 2023). Delayed sea-ice formation in early winter has been suggested to be linked to severe events documented for the Yamal region (Forbes et al., 2016). However, the progression of individual ROS events there has differed (Bartsch et al., 2023) and impacts in NDVI not reported.
The challenges inherent to remote sensing of Arctic ecosystems are widely recognized (Beamish et al., 2020). Nowhere are these challenges more acute than in the High Arctic, where the growing season is shortest, cloud frequency is high (Karlsen et al., 2018), and observations are highly subject to subpixel effects of late-lying snow and surface water (Cooper et al., 2019). NDVI trends recorded by GIMMS-3g+ and MODIS are very dissimilar (Figures 2, 3) and field observations are scarce outside of a few well-studied areas; as a result, there is lingering uncertainty regarding what is really happening in these remote ecosystems. These uncertainties are particularly troubling considering the amplified importance of incremental changes in temperature in these cold ecosystems, and the pace and severity of the resultant changes to permafrost landforms and surface hydrology (Farquharson et al., 2019).
Vegetation tends to be highly discontinuous in High Arctic ecosystems; although NDVI should be highly sensitive to incremental changes in vegetation cover under these circumstances, time series on barren surfaces are often incoherent, and surface reflectance is disproportionately affected by seasonal snow and surface water rather than by the vegetation itself. On balance, available plot-based monitoring studies in the Canadian Arctic Archipelago, Greenland, and Svalbard (see regional highlights sections) call GIMMS-3g + -based long-term browning trends into question and favor the greening signal recorded by MODIS, with long-term increases in graminoid cover being the most common change recorded. Landsat time series for the Antarctic Peninsula, which experiences a climate regime similar to the High Arctic, unequivocally demonstrate recent increases in vegetation cover concurrent with warming (Roland et al., 2024).
Unlike MaxNDVI, trends in TI-NDVI observed by long-term GIMMS-3g+ and MODIS generally do not agree in magnitude, sign, or regional pattern over the 23-year period of concurrent observation (Figure 3) (Frost et al., 2021). However, qualitative patterns of interannual variability are similar (Figure 4), suggesting that the overall negative trend in GIMMS-3g + TI-NDVI may be an artifact of high noise in this record. Circumpolar mean TI-NDVI values in 2018, 2019, and 2021 are the lowest in the GIMMS-3g + record since the eruption of Mt. Pinatubo, yet are interspersed with some of the highest TI-NDVI values on record. Although increasing climatic variability is a prominent component of Arctic change (Christensen et al., 2021), such extreme interannual fluctuations in circumpolar TI-NDVI are difficult to reconcile.
One possible explanation is that the TI-NDVI metric is highly sensitive to “shoulder season” observations, which are periods when greenness can change very rapidly due to snowmelt, greenup, senescence, and snow-on. In addition, the latter part of the snowfree season (late August–September) coincides with increasing cloudiness and low sun angles in much of the Arctic, complicating trend analysis in the late season regardless of the sensor used (Swanson, 2017). GIMMS-3g+ in particular could be prone to low bias during these periods due to the fact that there is only one sensor. In contrast, MODIS TI-NDVI is derived from two sensors, which provides more opportunities for observation during favorable sky and ground conditions and favors higher values using a maximum-value compositing approach (Bayle et al., 2024). Additional context for TI-NDVI could be gained by comparing patterns of interannual variability using detrended time series from GIMMS-3g+ and MODIS.
Permafrost is warming and degrading across the Arctic (Biskaborn et al., 2019; Miner et al., 2022; Schuur et al., 2022; 2023; See et al., 2024) with diverse and contrasting effects on plant growth, soil hydrology, surface water, and NDVI (Foster et al., 2022; Heijmans et al., 2022). Deepening of the active layer can improve access to nitrogen and other nutrients, increasing plant productivity (Salmon et al., 2016; Blume-Werry et al., 2019; Heijmans et al., 2022), particularly of deep-rooting taxa with high root plasticity (e.g., tussocks and other graminoids) (Wang et al., 2017). In addition, permafrost disturbances in the Low Arctic often favor recruitment of nitrogen-fixing alder shrubs, which strongly impact local soil nutrient regime (Hiltbrunner et al., 2014). However, deeper active layers may also lower the water table and dry the rooting zone (Heijmans et al., 2022). Collectively, these factors make it difficult to disentangle the effects of active layer deepening on NDVI and plant growth from concurrent air temperature dynamics.
The spatial scale of permafrost degradation has implications for remote sensing of permafrost-mediated NDVI dynamics. Landscape-scale permafrost processes such as thaw lake initiation, expansion and decline, thaw slumping or active layer detachments may be identified in long-term NDVI records and monitored for subsequent development and recovery (Rodenhizer et al., 2024). Local changes such as ice-wedge degradation and formation of smaller thaw ponds or thermo-erosional gullies produce obvious vegetation effects in the field (e.g., “drowning” tussocks and shrubs) that can also be resolved in VHR imagery (Jorgenson M. T. et al., 2015; Magnússon et al., 2021) but not in Sentinel-2 or Landsat imagery. Occurrence of ground-validated, small-scale processes of thaw pond formation and expansion may even be uncorrelated to NDVI dynamics at a spatial resolution larger than a few meters (Magnússon et al., 2023). Hence, linking NDVI records to gradual permafrost degradation processes, and to rapid changes that occur in mosaic landscapes (e.g., polygonal ground), are the most challenging.
Perennial lakes and ponds are widespread in many Arctic landscapes, particularly in coastal plain and deltaic settings. While attempts are made to mask waterbodies from NDVI time series analysis, faithful masking is difficult to perform. High variability and spurious trends may persist due to errors of omission, while important dynamics could be concealed by errors of commission, particularly in warmer Arctic regions that are experiencing systematic declines in lake area, whose drained basins are foci of rapid vegetation development (Nitze et al., 2020; Lindgren et al., 2021; Jones et al., 2022). Finally, systematic spectral trends have been identified in Arctic waterbodies (Kuhn and Butman, 2021), although reports of declining lake greenness are based on different spectral metrics and it is not clear what influence these trends may have on terrestrial NDVI.
Many Arctic regions do not exhibit any statistically significant NDVI trend (Callaghan et al., 2021). While a lack of NDVI trend does not necessarily indicate a lack of change in ground conditions, there are ample plot-based examples of tundra ecosystems that exhibit little or no change over time. Identifying the site factors that confer stability is a pressing need. Several potential factors favoring ecological stability have been proposed (Callaghan et al., 2021). Many Arctic plants have large geographic ranges and are long-lived; these characteristics would seem to require a capacity to survive strong interannual climate variability, and therefore may also favor survival in a warming climate. Second, structural or physiological limitations of cold-adapted Arctic plants may mean that some taxa simply cannot grow more in response to warming; the presence of such species can also preclude seed recruitment of fast-growing, resource acquisitive taxa, including many deciduous shrubs. In addition, many tundra plant communities may lack the capacity to respond to further warming where soil and permafrost conditions do not favor increased access to soil nutrients. Finally, changing trophic webs can also counter the response of vegetation to climate change at regional scales, such as increased herbivore populations arising from the removal of top predators such as wolves (Van Bogaert et al., 2009). Here, greening is being “masked” by browning, given that many disturbance events also increase as a result of the same warming that causes greening (Phoenix and Bjerke et al., 2016). However, only a few of these ideas have been tested and additional research is needed.
The sunsetting of the MODIS Terra and Aqua satellites makes urgent the integration of VIIRS data to maintain the continuity of this key dataset. In addition, the eighteenth and final AVHRR sensor was launched onboard the MetOp-C satellite in 2018 (Kalluri et al., 2021). Although VIIRS was purposefully designed to serve as a successor to MODIS, the VIIRS circumpolar MaxNDVI record to date is substantially different (Figure 4) and displays relatively high interannual variability that is more comparable to GIMMS-3g+. The release of fully reprocessed VIIRS data products, in the form of VIIRS version 2, is ongoing and will facilitate more robust intercomparison with contemporaneous MODIS collections. In addition, current VIIRS products are derived from a single satellite (Suomi NPP); the incorporation of observations from additional VIIRS platforms could also improve the record‘s comparability to MODIS.
The advancement and proliferation of high-resolution data collection (e.g., UAVs, airborne, and commercial satellite imagery) and big data analysis (e.g., machine learning techniques and artificial intelligence) will help to clarify the actual on-the-ground mechanisms of ecological changes in complex Arctic landscapes. Arctic plant communities typically have diverse plant functional groups such that both vascular (e.g., shrubs, forbs, graminoids) and nonvascular (bryophytes, lichens) lifeforms are prominent, and are experiencing compositional and structural changes (Elmendorf et al., 2012a; Elmendorf et al., 2012b; Bjorkman et al., 2018). Diverse plant communities can show variability in the seasonal timing of leaf emergence, peak productivity and senescence, and diversity in responses to climate drivers on small spatial scales. In tundra ecosystems, this spatio-temporal heterogeneity may be lost when monitoring changes in vegetation indices at resolutions larger than 0.5 × 0.5 m (Assmann et al., 2020). Preserving spatio-temporal dynamics, for instance through the collection of multiple moderate to high resolution satellite, drone or “phenocam” images within a season, can aid in the distinction and monitoring of different Arctic plant communities and their phenological dynamics (Beamish et al., 2017; Westergaard-Nielsen et al., 2017; Karami et al., 2018; Yang et al., 2023). Solar Induced Fluorescence (SIF) is an emerging radiance-based metric of photosynthetic activity and phenology, but the spatio-temporal coverage of SIF datasets remains small.
A key limitation of NDVI is the diminishing sensitivity of the metric as a measure of ecological change in landscapes with high biomass. This limit places bounds on spectral greening, particularly in the Low Arctic, as MaxNDVI values begin to “plateau” in shrubby areas, particularly near the forest-tundra ecotone. Here, current high-resolution spaceborne estimates of vegetation structure can be an important complement to spectral time series, by providing contemporary stratification of landscape vegetation patterns and a means to account for variation in NDVI sensitivity.
The availability of Sentinel-1 and Sentinel-2 at 10-m spatial resolution improves our capability to capture individual disturbance features and thus enhances ability of change attribution. Although data are available only since 2016, they can complement Landsat time series (Runge and Grosse, 2020; Bartsch et al., 2021). The emergence of harmonized Landsat/Sentinel-2 (HLS) data products, which provide much higher spatial resolution with improved temporal resolution, should prove extremely useful for high spatial resolution trend monitoring, especially for challenging regions such as the High Arctic. The quality of these products benefits from very high sidelap of adjacent orbital paths in the Arctic, which increases the effective observation frequency.
The high relevance of direct consequences of environmental change to human livelihoods in the Arctic necessitates improved exchange of information between natural scientists, local people, land managers, and other stakeholders (Bronen et al., 2020). There is increasing recognition of the insights that Indigenous knowledge (IK) can provide in synthesizing a broad base of information regarding Arctic environmental change (Fienup-Riordan et al., 2013; Ksenofontov et al., 2019; Bronen et al., 2020). For example, sharing of IK through the Local Environmental Observer (LEO) network (Mosites et al., 2018) has led to integrated studies (Jones et al., 2023). The shift in concerns among Indigenous communities from industrial impacts to climate-related issues in northwestern Siberia and elsewhere highlights the broader impacts of environmental changes. The involvement of elders is particularly helpful for understanding the drivers, manifestations, and consequences of Arctic greening because their frame of reference can predate the period of record for high-resolution remote sensing, and elder observations can help address uncertainties and disagreement among disparate long-term NDVI and climate datasets through their knowledge of underlying landscape conditions and change (Fienup-Riordan et al., 2021).
The concentration of record-high MaxNDVI values in multiple long-term records in recent years, coupled with continued advances in the state of knowledge of key mechanisms provides unequivocal evidence that the “greening of the Arctic” continues and in many cases represents borealization of Low Arctic ecosystems. Given what has been learned from circumpolar monitoring of Arctic tundra greenness since 1982, what are reasonable expectations for the coming decades?
Arctic greening trends in a warming climate have sparked concern that further shrubification and northward expansion of the tundra-taiga ecotone will result in considerable loss of tundra ecosystems and their associated floristic biodiversity (Henry et al., 2022; Kruse et al., 2023). Long-term vegetation compositional records from International Tundra Experiment (ITEX) monitoring sites confirm increases in vascular plant abundance (particularly shrubs and graminoids) and canopy height both under ambient and experimental warming, while bryophyte and lichen cover and diversity decrease, particularly under shrub expansion, lowering overall plant diversity (Henry et al., 2022). Such changes are more evident in warmer, mesic Low Arctic ecosystems, suggesting that Arctic greening resulting from increased cover and stature of woody species could have negative consequences for floristic diversity. In addition, apparent increases in the frequency and extent of tundra fires have particularly detrimental effects on tundra lichens (Jandt et al., 2008; Frost et al., 2020), a key component of floristic diversity and important forage for caribou (Joly et al., 2009). Shifts in Arctic climate and seasonality also directly affect plant phenology, with implications for plant-pollinator interactions and plant reproductive success, particularly in the High Arctic (Prevéy et al., 2019; Collins et al., 2021). Datasets such as ITEX, with three decades of plant community data across the tundra biome, are invaluable for the validation and interpretation of plot- and landscape-level remote sensing products of tundra greenness.
Arctic greening has wide ranging implications for higher trophic levels, including species that are important to subsistence-based Arctic societies (Wheeler et al., 2018). For example, though greening trends may signify increased browse for Arctic ungulates such as caribou and reindeer (e.g., Potravny and Elsakov, 2024), many herds have paradoxically declined as the abundance of plants with strong chemical defenses has increased (Fauchald et al., 2017). Increased canopy height and structural complexity associated with shrubification strongly modify bird habitats, favoring expansion of boreal forest species (mostly songbirds) but at the detriment of tundra-nesting species (largely shorebirds) (Boelman et al., 2015; Munro, 2017; Mizel and Swanson, 2022; Lyons et al., 2024). Greening in northern European tundra has also been linked to increased nest predation risk (Ims et al., 2019).
The Arctic has entered a new climate and cryospheric regime, where historic extremes are becoming the new normal. Although spring and summer sea-ice concentration has long been recognized as a key control of growing season temperatures and tundra greenness (Bhatt et al., 2010), sea-ice extent has approached zero in Arctic marginal seas in several recent years and is expected to decline further in the future (Overland and Wang, 2013). This trend poses two challenges for future projections of tundra vegetation dynamics. The first is simply that spring and summer sea-ice will no longer be a relevant variable for predicting tundra vegetation greenness in parts of the Arctic. Second, the loss of sea ice has profound implications for other climatic variables (e.g., cloudiness, atmospheric moisture), the interactions among them, and their influence on tundra growing conditions (Liu et al., 2012). This shift will affect vegetation dynamics and potentially lead to abrupt transitions in some areas. There is general agreement that greening will continue as an overall trend across the Arctic, but this greening will continue to be complicated by disturbances that may cause localized browning or affect NDVI trends in more complex ways.
Although the relatively subtle “background” greening trends evident across large parts of the Arctic often cannot be interpreted with confidence, other aspects of Arctic change, such as continued sea-ice decline and warming permafrost temperatures, are known with high confidence. The combination of rising atmospheric and ground temperatures portends deepening of permafrost active layer thickness that will, in turn, mobilize heretofore frozen soil nutrients that can support further increases in biomass. In addition, Arctic warming could accelerate decomposition, soil development and associated increases in plant cover and biomass (Doetterl et al., 2021). Although NDVI is inherently tied to aboveground biomass, any changes aboveground are linked proportionately to belowground biomass, and also have implications for soil temperature and biological processes (Mortier et al., 2024). Despite lingering uncertainties regarding trend attribution and sources of interannual variability, the sequence of record-high circumpolar tundra greenness values observed since 2020 provides strong evidence that aboveground live biomass in many Arctic tundra regions is without recent historic precedent. Considering the strength of relationships between tundra productivity, growing season temperature, summer sea-ice extent, and permafrost conditions, aboveground live biomass across much of the Arctic likely exceeds that of any period since well before the era of circumpolar remote sensing. Indeed, given that Arctic air temperatures have generally been colder than today for many centuries (Kaufman et al., 2009), this time period likely extends to nearly a millennium.
GF: Conceptualization, Formal Analysis, Funding acquisition, Investigation, Methodology, Project administration, Resources, Supervision, Validation, Visualization, Writing–original draft, Writing–review and editing. UB: Conceptualization, Data curation, Formal Analysis, Funding acquisition, Investigation, Methodology, Resources, Supervision, Validation, Visualization, Writing–original draft, Writing–review and editing. MM: Data curation, Formal Analysis, Funding acquisition, Investigation, Methodology, Resources, Software, Validation, Visualization, Writing–review and editing. LB: Data curation, Formal Analysis, Investigation, Methodology, Software, Validation, Visualization, Writing–original draft, Writing–review and editing. DW: Writing–original draft, Writing–review and editing, Conceptualization. MR: Writing–review and editing, Conceptualization. RM: Writing–review and editing, Writing–original draft, Visualization. AB: Writing–original draft, Writing–review and editing. JB: Writing–original draft, Writing–review and editing. HE: Writing–review and editing. BF: Writing–original draft, Writing–review and editing. SG: Writing–review and editing. EH: Writing–review and editing. SK: Writing–review and editing. TK: Writing–original draft, Writing–review and editing. TL: Writing–original draft, Writing–review and editing. ML: Writing–original draft, Writing–review and editing. EL-B: Writing–original draft, Writing–review and editing. PM: Funding acquisition, Writing–review and editing. CN: Writing–review and editing, Funding acquisition. IN: Writing–original draft, Writing–review and editing. KO: Writing–review and editing. TP: Writing–review and editing. GP: Writing–review and editing, Writing–original draft. AR: Writing–review and editing. BR: Writing–review and editing. GS-S: Writing–review and editing, Conceptualization. HT: Writing–review and editing, Writing–original draft. MV: Writing–original draft, Writing–review and editing. AV: Writing–review and editing, Writing–original draft. A-MV: Writing–review and editing. CW: Writing–review and editing.
The author(s) declare that financial support was received for the research, authorship, and/or publication of this article. This work was supported by NASA Arctic Boreal Vulnerability Experiment (ABoVE) grants 80NSSC22K1256 (GF, UB, MM, PM, CN) and 80NSSC22K1247 (LB, SG). Additional funding came from the European Union through H2020 CHARTER No. 869471 (AB, JB, BF, TK, GS-S, HT); the European Research Council synergy project Q-Arctic No. 951288 (AB); the Finnish Ministry of the Environment No. VN/9768/2022-YM-4 (TK, MV); the European Union‘s HORIZON No. 101056921 (EL-B); the German Federal Ministry for Economic Affairs and Climate Action (BMWK) research project ML4EARTH, European Space Agency (ESA) CCI+ Permafrost, National Science Foundation (NSF) No. 1927872, and google.org No. 2052107 (IN); the “Permafrost Pathways” TED Audacious Project (BR, AV); and NordForsk No. 164079 (MV).
We thank J. Pinzon at the Biospheric Sciences Laboratory, NASA Goddard Space Flight Center for providing annual updates for the GIMMS-3g + dataset. We also thank Dedi Yang and members of the NASA ABoVE Vegetation Dynamics Working Group for helpful insights on the manuscript.
Authors GF and MM were employed by Alaska Biological Research, Inc. Author AB was employed by b.geos GmbH. Author EH was employed by Global Science & Technology, Inc. Author PM was employed by ADNET Systems, Inc.
The remaining authors declare that the research was conducted in the absence of any commercial or financial relationships that could be construed as a potential conflict of interest.
The author(s) declare that no Generative AI was used in the creation of this manuscript.
All claims expressed in this article are solely those of the authors and do not necessarily represent those of their affiliated organizations, or those of the publisher, the editors and the reviewers. Any product that may be evaluated in this article, or claim that may be made by its manufacturer, is not guaranteed or endorsed by the publisher.
The Supplementary Material for this article can be found online at: https://www.frontiersin.org/articles/10.3389/fenvs.2025.1525574/full#supplementary-material
AMAP (2017). Snow, water, ice and permafrost in the arctic (SWIPA) 2017. Oslo, Norway: Arctic Monitoring and Assessment Programme.
Andruko, R., Danby, R., and Grogan, P. (2020). Recent growth and expansion of birch shrubs across a Low Arctic landscape in continental Canada: are these responses more a consequence of the severely declining caribou herd than of climate warming? Ecosystems 23, 1362–1379. doi:10.1007/s10021-019-00474-7
Arndt, K. A., Santos, M. J., Ustin, S., Davidson, S. J., Stow, D., Oechel, W. C., et al. (2019). Arctic greening associated with lengthening growing seasons in Northern Alaska. Environ. Res. Lett. 14, 125018. doi:10.1088/1748-9326/ab5e26
Assmann, J. J., Myers-Smith, I. H., Kerby, J. T., Cunliffe, A. M., and Daskalova, G. N. (2020). Drone data reveal heterogeneity in tundra greenness and phenology not captured by satellites. Environ. Res. Lett. 15, 125002. doi:10.1088/1748-9326/abbf7d
Badgley, G., Field, C. B., and Berry, J. A. (2017). Canopy near-infrared reflectance and terrestrial photosynthesis. Sci. Adv. 3, e1602244. doi:10.1126/sciadv.1602244
Baker, M. R., Kivva, K. K., Pisareva, M. N., Watson, J. T., and Selivanova, J. (2020). Shifts in the physical environment in the Pacific Arctic and implications for ecological timing and conditions. Deep Sea Res. Part II Top. Stud. Oceanogr. 177, 104802. doi:10.1016/j.dsr2.2020.104802
Bartsch, A., Bergstedt, H., Pointner, G., Muri, X., Rautiainen, K., Leppänen, L., et al. (2023). Towards long-term records of rain-on-snow events across the Arctic from satellite data. Cryosphere 17, 889–915. doi:10.5194/tc-17-889-2023
Bartsch, A., Pointner, G., Nitze, I., Efimova, A., Jakober, D., Ley, S., et al. (2021). Expanding infrastructure and growing anthropogenic impacts along Arctic coasts. Environ. Res. Lett. 16, 115013. doi:10.1088/1748-9326/ac3176
Bayle, A., Gascoin, S., Berner, L. T., and Choler, P. (2024). Landsat-based greening trends in alpine ecosystems are inflated by multidecadal increases in summer observations. Ecography 2024, e07394. doi:10.1111/ecog.07394
Beamish, A., Raynolds, M. K., Epstein, H., Frost, G. V., Macander, M. J., Bergstedt, H., et al. (2020). Recent trends and remaining challenges for optical remote sensing of Arctic tundra vegetation: a review and outlook. Remote Sens. Environ. 246, 111872. doi:10.1016/j.rse.2020.111872
Beamish, A. L., Coops, N., Chabrillat, S., and Heim, B. (2017). A phenological approach to spectral differentiation of low-arctic tundra vegetation communities, North Slope, Alaska. Remote Sens. 9, 1200. doi:10.3390/rs9111200
Beck, H. E., McVicar, T. R., van Dijk, A. I. J. M., Schellekens, J., de Jeu, R. A. M., and Bruijnzeel, L. A. (2011). Global evaluation of four AVHRR–NDVI data sets: intercomparison and assessment against Landsat imagery. Remote Sens. Environ. 115, 2547–2563. doi:10.1016/j.rse.2011.05.012
Berner, L. T., Assmann, J. J., Normand, S., and Goetz, S. J. (2023). ‘LandsatTS’: an R package to facilitate retrieval, cleaning, cross-calibration, and phenological modeling of Landsat time series data. Ecography 2023, e06768. doi:10.1111/ecog.06768
Berner, L. T., Beck, P. S. A., Bunn, A. G., and Goetz, S. J. (2013). Plant response to climate change along the forest-tundra ecotone in northeastern Siberia. Glob. Change Biol. 19, 3449–3462. doi:10.1111/gcb.12304
Berner, L. T., Massey, R., Jantz, P., Forbes, B. C., Macias-Fauria, M., Myers-Smith, I., et al. (2020). Summer warming explains widespread but not uniform greening in the Arctic tundra biome. Nat. Commun. 11, 4621. doi:10.1038/s41467-020-18479-5
Bernes, C., Bråthen, K. A., Forbes, B. C., Speed, J. D., and Moen, J. (2015). What are the impacts of reindeer/caribou (Rangifer tarandus L.) on arctic and alpine vegetation? A systematic review. Environ. Evid. 4, 4. doi:10.1186/s13750-014-0030-3
Bhatt, U., Walker, D., Raynolds, M., Bieniek, P., Epstein, H., Comiso, J., et al. (2013). Recent declines in warming and vegetation greening trends over Pan-Arctic tundra. Remote Sens. 5, 4229–4254. doi:10.3390/rs5094229
Bhatt, U. S., Walker, D. A., Raynolds, M. K., Bieniek, P. A., Epstein, H. E., Comiso, J. C., et al. (2017). Changing seasonality of panarctic tundra vegetation in relationship to climatic variables. Environ. Res. Lett. 12, 055003. doi:10.1088/1748-9326/aa6b0b
Bhatt, U. S., Walker, D. A., Raynolds, M. K., Comiso, J. C., Epstein, H. E., Jia, G., et al. (2010). Circumpolar Arctic tundra vegetation change is linked to sea ice decline. Earth Interact. 14, 1–20. doi:10.1175/2010EI315.1
Bhatt, U. S., Walker, D. A., Raynolds, M. K., Walsh, J. E., Bieniek, P. A., Cai, L., et al. (2021). Climate drivers of Arctic tundra variability and change using an indicators framework. Environ. Res. Lett. 16, 055019. doi:10.1088/1748-9326/abe676
Bieniek, P. A., Bhatt, U. S., Walker, D. A., Raynolds, M. K., Comiso, J. C., Epstein, H. E., et al. (2015). Climate drivers linked to changing seasonality of Alaska coastal tundra vegetation productivity. Earth Interact. 19, 1–29. doi:10.1175/EI-D-15-0013.1
Biskaborn, B. K., Smith, S. L., Noetzli, J., Matthes, H., Vieira, G., Streletskiy, D. A., et al. (2019). Permafrost is warming at a global scale. Nat. Commun. 10, 264. doi:10.1038/s41467-018-08240-4
Bjerke, J. W., Rune Karlsen, S., Arild Høgda, K., Malnes, E., Jepsen, J. U., Lovibond, S., et al. (2014). Record-low primary productivity and high plant damage in the Nordic Arctic Region in 2012 caused by multiple weather events and pest outbreaks. Environ. Res. Lett. 9, 084006. doi:10.1088/1748-9326/9/8/084006
Bjerke, J. W., Treharne, R., Vikhamar-Schuler, D., Karlsen, S. R., Ravolainen, V., Bokhorst, S., et al. (2017). Understanding the drivers of extensive plant damage in boreal and Arctic ecosystems: insights from field surveys in the aftermath of damage. Sci. Total Environ. 599–600, 1965–1976. doi:10.1016/j.scitotenv.2017.05.050
Bjorkman, A. D., García Criado, M., Myers-Smith, I. H., Ravolainen, V., Jónsdóttir, I. S., Westergaard, K. B., et al. (2020). Status and trends in Arctic vegetation: evidence from experimental warming and long-term monitoring. Ambio 49, 678–692. doi:10.1007/s13280-019-01161-6
Bjorkman, A. D., Myers-Smith, I. H., Elmendorf, S. C., Normand, S., Rüger, N., Beck, P. S. A., et al. (2018). Plant functional trait change across a warming tundra biome. Nature 562, 57–62. doi:10.1038/s41586-018-0563-7
Blok, D., Schaepman-Strub, G., Bartholomeus, H., Heijmans, M. M. P. D., Maximov, T. C., and Berendse, F. (2011). The response of Arctic vegetation to the summer climate: relation between shrub cover, NDVI, surface albedo and temperature. Environ. Res. Lett. 6, 035502. doi:10.1088/1748-9326/6/3/035502
Blume-Werry, G., Milbau, A., Teuber, L. M., Johansson, M., and Dorrepaal, E. (2019). Dwelling in the deep – strongly increased root growth and rooting depth enhance plant interactions with thawing permafrost soil. New Phytol. 223, 1328–1339. doi:10.1111/nph.15903
Boelman, N. T., Gough, L., Wingfield, J., Goetz, S., Asmus, A., Chmura, H. E., et al. (2015). Greater shrub dominance alters breeding habitat and food resources for migratory songbirds in Alaskan arctic tundra. Glob. Change Biol. 21, 1508–1520. doi:10.1111/gcb.12761
Bokhorst, S., Tømmervik, H., Callaghan, T. V., Phoenix, G. K., and Bjerke, J. W. (2012). Vegetation recovery following extreme winter warming events in the sub-Arctic estimated using NDVI from remote sensing and handheld passive proximal sensors. Environ. Exp. Bot. 81, 18–25. doi:10.1016/j.envexpbot.2012.02.011
Bonta, C., King, G. M., and Danby, R. K. (2023). Greening on the Bathurst caribou range in northern Canada: are erect shrubs responsible for remotely sensed trends? Arct. Sci. as-2022-0036. doi:10.1139/as-2022-0036
Bret-Harte, M. S., Mack, M. C., Shaver, G. R., Huebner, D. C., Johnston, M., Mojica, C. A., et al. (2013). The response of Arctic vegetation and soils following an unusually severe tundra fire. Philosophical Trans. R. Soc. B Biol. Sci. 368, 20120490. doi:10.1098/rstb.2012.0490
Bronen, R., Pollock, D., Overbeck, J., Stevens, D., Natali, S., and Maio, C. (2020). Usteq: integrating indigenous knowledge and social and physical sciences to coproduce knowledge and support community-based adaptation. Polar Geogr. 43, 188–205. doi:10.1080/1088937X.2019.1679271
Brown, J., Ferrians, O. J., Heginbottom, J. A., and Melnikov, E. S. (2001). Circum-arctic map of permafrost and ground ice conditions.
Buchwal, A., Sullivan, P. F., Macias-Fauria, M., Post, E., Myers-Smith, I. H., Stroeve, J. C., et al. (2020). Divergence of Arctic shrub growth associated with sea ice decline. Proc. Natl. Acad. Sci. 117, 33334–33344. doi:10.1073/pnas.2013311117
Büntgen, U., Hellmann, L., Tegel, W., Normand, S., Myers-Smith, I., Kirdyanov, A. V., et al. (2015). Temperature-induced recruitment pulses of Arctic dwarf shrub communities. J. Ecol. 103, 489–501. doi:10.1111/1365-2745.12361
Büntgen, U., and Rees, G. (2023). Global change research needs international collaboration. Sci. Total Environ. 902, 166054. doi:10.1016/j.scitotenv.2023.166054
Callaghan, T. V., Cazzolla Gatti, R., and Phoenix, G. (2021). The need to understand the stability of arctic vegetation during rapid climate change: an assessment of imbalance in the literature. Ambio 51, 1034–1044. doi:10.1007/s13280-021-01607-w
Cameron, E. A., and Lantz, T. C. (2016). Drivers of tall shrub proliferation adjacent to the dempster highway, northwest territories, Canada. Environ. Res. Lett. 11, 045006. doi:10.1088/1748-9326/11/4/045006
Cameron, E. A., Lantz, T. C., and Kokelj, S. V. (2024). Impacts of shrub removal on snow and near-surface thermal conditions in permafrost terrain adjacent to the Dempster Highway, NT, Canada. Arct. Sci. 10, 87–107. doi:10.1139/as-2022-0032
Campbell, T. K. F., Lantz, T. C., Fraser, R. H., and Hogan, D. (2021). High Arctic vegetation change mediated by hydrological conditions. Ecosystems 24, 106–121. doi:10.1007/s10021-020-00506-7
Chapin, F. S., Shaver, G. R., Giblin, A. E., Nadelhoffer, K. J., and Laundre, J. A. (1995). Responses of arctic tundra to experimental and observed changes in climate. Ecology 76, 694–711. doi:10.2307/1939337
Chen, A., and Lantz, T. C. (2024). Influence of tundra fire severity on vegetation recovery in the Northwest Territories. Arct. Sci. 10, 569–582. doi:10.1139/as-2022-0050
Chen, A., Lantz, T. C., Hermosilla, T., and Wulder, M. A. (2021a). Biophysical controls of increased tundra productivity in the western Canadian Arctic. Remote Sens. Environ. 258, 112358. doi:10.1016/j.rse.2021.112358
Chen, D., Fu, C., Jenkins, L. K., He, J., Wang, Z., Jandt, R. R., et al. (2024). Regional fire–greening positive feedback loops in Alaskan Arctic tundra. Nat. Plants 10, 1886–1891. doi:10.1038/s41477-024-01850-5
Chen, Y., Cheng, X., Liu, A., Chen, Q., and Wang, C. (2023). Tracking lake drainage events and drained lake basin vegetation dynamics across the Arctic. Nat. Commun. 14, 7359. doi:10.1038/s41467-023-43207-0
Chen, Y., Hu, F. S., and Lara, M. J. (2021b). Divergent shrub-cover responses driven by climate, wildfire, and permafrost interactions in Arctic tundra ecosystems. Glob. Change Biol. 27, 652–663. doi:10.1111/gcb.15451
Chen, Y., Lara, M. J., Jones, B. M., Frost, G. V., and Hu, F. S. (2021c). Thermokarst acceleration in Arctic tundra driven by climate change and fire disturbance. One Earth S259033222100659X, 1718–1729. doi:10.1016/j.oneear.2021.11.011
Chen, Y., Liu, A., and Cheng, X. (2021d). Vegetation grows more luxuriantly in Arctic permafrost drained lake basins. Glob. Change Biol. 27, 5865–5876. doi:10.1111/gcb.15853
Cheng, R. (2024). Solar-Induced chlorophyll fluorescence (SIF): towards a better understanding of vegetation dynamics and carbon uptake in Arctic-boreal ecosystems. Curr. Clim. Change Rep. 10, 13–32. doi:10.1007/s40641-024-00194-8
Choudhary, S., Blaud, A., Osborn, A. M., Press, M. C., and Phoenix, G. K. (2016). Nitrogen accumulation and partitioning in a High Arctic tundra ecosystem from extreme atmospheric N deposition events. Sci. Total Environ. 554 (555), 303–310. doi:10.1016/j.scitotenv.2016.02.155
Christensen, T. R., Lund, M., Skov, K., Abermann, J., López-Blanco, E., Scheller, J., et al. (2021). Multiple ecosystem effects of extreme weather events in the Arctic. Ecosystems 24, 122–136. doi:10.1007/s10021-020-00507-6
Collins, C. G., Elmendorf, S. C., Hollister, R. D., Henry, G. H. R., Clark, K., Bjorkman, A. D., et al. (2021). Experimental warming differentially affects vegetative and reproductive phenology of tundra plants. Nat. Commun. 12, 3442. doi:10.1038/s41467-021-23841-2
Comiso, J. C. (2003). Warming trends in the Arctic from clear sky satellite observations. J. Clim. 16, 3498–3510. doi:10.1175/1520-0442(2003)016<3498:WTITAF>2.0.CO;2
Comiso, J. C., and Nishio, F. (2008). Trends in the sea ice cover using enhanced and compatible AMSR-E, SSM/I, and SMMR data. J. Geophys. Res. 113. doi:10.1029/2007JC004257
Cooper, E. J., Little, C. J., Pilsbacher, A. K., and Mörsdorf, M. A. (2019). Disappearing green: shrubs decline and bryophytes increase with nine years of increased snow accumulation in the High Arctic. J. Veg. Sci. 30, 857–867. doi:10.1111/jvs.12793
Davis, E., Trant, A., Hermanutz, L., Way, R. G., Lewkowicz, A. G., Collier, L. S., et al. (2021). Plant–environment interactions in the low arctic torngat mountains of labrador. Ecosystems 24, 1038–1058. doi:10.1007/s10021-020-00577-6
Didan, K. (2021a). MODIS/Aqua vegetation indices 16-day L3. Glob. 500m Sin. Grid V061 [Data Set]. doi:10.5067/MODIS/MYD13A1.061
Didan, K. (2021b). MODIS/Terra vegetation indices 16-day L3. Glob. 500m Sin. Grid V061 [Data Set]. doi:10.5067/MODIS/MOD13A1.061
Didan, K., and Barreto, A. (2018). VIIRS/NPP vegetation indices 16-day L3 global 500m SIN grid V001. doi:10.5067/VIIRS/VNP13A1.001
Doetterl, S., Alexander, J., Fior, S., Frossard, A., Magnabosco, C., Broek, M., et al. (2021). Invited contribution for the 2022 Anniversary Edition: will accelerated soil development be a driver of Arctic Greening in the late 21st century? J. Plant Nutr. Soil Sci., 202100334. doi:10.1002/jpln.202100334
Druckenmiller, M. L., Thoman, R. L., Moon, T. A., Andreassen, L. M., Ballinger, T. J., Berner, L. T., et al. (2024). The arctic. Bull. Am. Meteorological Soc. 105, S277–S330. doi:10.1175/BAMS-D-24-0101.1
Dutrieux, L. P., Bartholomeus, H., Herold, M., and Verbesselt, J. (2012). Relationships between declining summer sea ice, increasing temperatures and changing vegetation in the Siberian Arctic tundra from MODIS time series (2000–11). Environ. Res. Lett. 7, 044028. doi:10.1088/1748-9326/7/4/044028
Elmendorf, S. C., Henry, G. H. R., Hollister, R. D., Björk, R. G., Bjorkman, A. D., Callaghan, T. V., et al. (2012a). Global assessment of experimental climate warming on tundra vegetation: heterogeneity over space and time. Ecol. Lett. 15, 164–175. doi:10.1111/j.1461-0248.2011.01716.x
Elmendorf, S. C., Henry, G. H. R., Hollister, R. D., Björk, R. G., Boulanger-Lapointe, N., Cooper, E. J., et al. (2012b). Plot-scale evidence of tundra vegetation change and links to recent summer warming. Nat. Clim. Change 2, 453–457. doi:10.1038/nclimate1465
Farquharson, L. M., Romanovsky, V. E., Cable, W. L., Walker, D. A., Kokelj, S. V., and Nicolsky, D. (2019). Climate change drives widespread and rapid thermokarst development in very cold permafrost in the Canadian High Arctic. Geophys. Res. Lett. 46, 6681–6689. doi:10.1029/2019GL082187
Farquharson, L. M., Romanovsky, V. E., Kholodov, A., and Nicolsky, D. (2022). Sub-aerial talik formation observed across the discontinuous permafrost zone of Alaska. Nat. Geosci. 15, 475–481. doi:10.1038/s41561-022-00952-z
Fauchald, P., Park, T., Tømmervik, H., Myneni, R., and Hausner, V. H. (2017). Arctic greening from warming promotes declines in caribou populations. Sci. Adv. 3, e1601365. doi:10.1126/sciadv.1601365
Fienup-Riordan, A., Brown, C., and Braem, N. M. (2013). The value of ethnography in times of change: the story of Emmonak. Deep Sea Res. Part II Top. Stud. Oceanogr. 94, 301–311. doi:10.1016/j.dsr2.2013.04.005
Fienup-Riordan, A., Frost, G. V., Nayamin-Kelly, R., Bhatt, U. S., Hendricks, A. S., John, M., et al. (2021). Yup’Ik and Cup’Ik observations of Alaska’s changing Yukon-Kuskokwim Delta: results of a knowledge exchange meeting with natural scientists. Fairbanks, AK: ABR, Inc.—Environmental Research and Services.
Forbes, B. C., Kumpula, T., Meschtyb, N., Laptander, R., Macias-Fauria, M., Zetterberg, P., et al. (2016). Sea ice, rain-on-snow and tundra reindeer nomadism in Arctic Russia. Biol. Lett. 12, 20160466. doi:10.1098/rsbl.2016.0466
Forbes, B. C., Macias-Fauria, M., and Zetterberg, P. (2010). Russian Arctic warming and ‘greening’ are closely tracked by tundra shrub willows. Glob. Change Biol. 16, 1542–1554. doi:10.1111/j.1365-2486.2009.02047.x
Forbes, B. C., and Stammler, F. (2009). Arctic climate change discourse: the contrasting politics of research agendas in the West and Russia. Polar Res. 28, 28–42. doi:10.1111/j.1751-8369.2009.00100.x
Foster, A. C., Wang, J. A., Frost, G. V., Davidson, S. J., Hoy, E., Turner, K. W., et al. (2022). Disturbances in North American boreal forest and Arctic tundra: impacts, interactions, and responses. Environ. Res. Lett. 17, 113001. doi:10.1088/1748-9326/ac98d7
Fraser, R. H., Lantz, T. C., Olthof, I., Kokelj, S. V., and Sims, R. A. (2014). Warming-induced shrub expansion and lichen decline in the western Canadian Arctic. Ecosystems 17, 1151–1168. doi:10.1007/s10021-014-9783-3
Fraser, R. H., Olthof, I., Lantz, T. C., and Schmitt, C. (2016). UAV photogrammetry for mapping vegetation in the low-Arctic. Arct. Sci. 2, 79–102. doi:10.1139/as-2016-0008
Frost, G. V., Bhatt, U. S., Macander, M. J., Hendricks, A. S., and Jorgenson, M. T. (2021). Is Alaska’s Yukon–Kuskokwim Delta greening or browning? Resolving mixed signals of tundra vegetation dynamics and drivers in the maritime Arctic. Earth Interact. 25, 76–93. doi:10.1175/EI-D-20-0025.1
Frost, G. V., Christopherson, T., Jorgenson, M. T., Liljedahl, A. K., Macander, M. J., Walker, D. A., et al. (2018a). Regional patterns and asynchronous onset of ice-wedge degradation since the mid-20th century in Arctic Alaska. Remote Sens. 10, 1312. doi:10.3390/rs10081312
Frost, G. V., and Epstein, H. E. (2014). Tall shrub and tree expansion in Siberian tundra ecotones since the 1960s. Glob. Change Biol. 20, 1264–1277. doi:10.1111/gcb.12406
Frost, G. V., Epstein, H. E., and Walker, D. A. (2014). Regional and landscape-scale variability of Landsat-observed vegetation dynamics in northwest Siberian tundra. Environ. Res. Lett. 9, 025004. doi:10.1088/1748-9326/9/2/025004
Frost, G. V., Epstein, H. E., Walker, D. A., Matyshak, G., and Ermokhina, K. (2013). Patterned-ground facilitates shrub expansion in Low Arctic tundra. Environ. Res. Lett. 8, 015035. doi:10.1088/1748-9326/8/1/015035
Frost, G. V., Epstein, H. E., Walker, D. A., Matyshak, G., and Ermokhina, K. (2018b). Seasonal and long-term changes to active-layer temperatures after tall shrubland expansion and succession in Arctic tundra. Ecosystems 21, 507–520. doi:10.1007/s10021-017-0165-5
Frost, G. V., Loehman, R. A., Saperstein, L. B., Macander, M. J., Nelson, P. R., Paradis, D. P., et al. (2020). Multi-decadal patterns of vegetation succession after tundra fire on the Yukon-Kuskokwim Delta, Alaska. Environ. Res. Lett. 15, 025003. doi:10.1088/1748-9326/ab5f49
Frost, G. V., Macander, M. J., Bhatt, U. S., Berner, L. T., Bjerke, J. W., Epstein, H. E., et al. (2024). Tundra greenness [in “state of the climate in 2023”]. Bull. Am. Meteorological Soc. 105, S305–S308. doi:10.1175/BAMS-D-24-0101.1
Gaglioti, B. V., Berner, L. T., Jones, B. M., Orndahl, K. M., Williams, A. P., Andreu-Hayles, L., et al. (2021). Tussocks enduring or shrubs greening: alternate responses to changing fire regimes in the Noatak River Valley, Alaska. J. Geophys. Res. Biogeosciences 126, e2020JG006009. doi:10.1029/2020JG006009
Gamon, J. A., Huemmrich, K. F., Stone, R. S., and Tweedie, C. E. (2013). Spatial and temporal variation in primary productivity (NDVI) of coastal Alaskan tundra: decreased vegetation growth following earlier snowmelt. Remote Sens. Environ. 129, 144–153. doi:10.1016/j.rse.2012.10.030
Grimes, M., Carrivick, J. L., Smith, M. W., and Comber, A. J. (2024). Land cover changes across Greenland dominated by a doubling of vegetation in three decades. Sci. Rep. 14, 3120. doi:10.1038/s41598-024-52124-1
Guay, K. C., Beck, P. S. A., Berner, L. T., Goetz, S. J., Baccini, A., and Buermann, W. (2014). Vegetation productivity patterns at high northern latitudes: a multi-sensor satellite data assessment. Glob. Change Biol. 20, 3147–3158. doi:10.1111/gcb.12647
Haynes, K. M., Connon, R. F., and Quinton, W. L. (2018). Permafrost thaw induced drying of wetlands at Scotty Creek, NWT, Canada. Environ. Res. Lett. 13, 114001. doi:10.1088/1748-9326/aae46c
Heijmans, M. M. P. D., Magnússon, R. Í., Lara, M. J., Frost, G. V., Myers-Smith, I. H., van Huissteden, J., et al. (2022). Tundra vegetation change and impacts on permafrost. Nat. Rev. Earth Environ. 3, 68–84. doi:10.1038/s43017-021-00233-0
Heim, B., Lisovski, S., Wieczorek, M., Morgenstern, A., Juhls, B., Shevtsova, I., et al. (2022). Spring snow cover duration and tundra greenness in the Lena Delta, Siberia: two decades of MODIS satellite time series (2001–2021). Environ. Res. Lett. 17, 085005. doi:10.1088/1748-9326/ac8066
Hendricks, A. S., Bhatt, U. S., Frost, G. V., Walker, D. A., Bieniek, P. A., Raynolds, M. K., et al. (2023). Decadal variability in spring sea ice concentration linked to summer temperature and NDVI on the Yukon–Kuskokwim Delta. Earth Interact. 27, e230002. doi:10.1175/EI-D-23-0002.1
Henry, G. H. R., Hollister, R. D., Klanderud, K., Björk, R. G., Bjorkman, A. D., Elphinstone, C., et al. (2022). The International Tundra Experiment (ITEX): 30 years of research on tundra ecosystems. Arct. Sci. 8, 550–571. doi:10.1139/as-2022-0041
Hill, G. B., and Henry, G. H. R. (2011). Responses of High Arctic wet sedge tundra to climate warming since 1980. Glob. Change Biol. 17, 276–287. doi:10.1111/j.1365-2486.2010.02244.x
Hiltbrunner, E., Aerts, R., Bühlmann, T., Huss-Danell, K., Magnusson, B., Myrold, D. D., et al. (2014). Ecological consequences of the expansion of N2-fixing plants in cold biomes. Oecologia 176, 11–24. doi:10.1007/s00442-014-2991-x
Hobbie, J. E., Shaver, G. R., Rastetter, E. B., Cherry, J. E., Goetz, S. J., Guay, K. C., et al. (2017). Ecosystem responses to climate change at a Low Arctic and a High Arctic long-term research site. Ambio 46, 160–173. doi:10.1007/s13280-016-0870-x
Hu, F. S., Higuera, P. E., Duffy, P., Chipman, M. L., Rocha, A. V., Young, A. M., et al. (2015). Arctic tundra fires: natural variability and responses to climate change. Front. Ecol. Environ. 13, 369–377. doi:10.1890/150063
Huang, S., Tang, L., Hupy, J. P., Wang, Y., and Shao, G. (2021). A commentary review on the use of normalized difference vegetation index (NDVI) in the era of popular remote sensing. J. For. Res. 32, 1–6. doi:10.1007/s11676-020-01155-1
Hudson, J. M., and Henry, G. H. R. (2009). Increased plant biomass in a High Arctic heath community from 1981 to 2008. Ecology 90, 2657–2663. doi:10.1890/09-0102.1
Hudson, J. M. G., and Henry, G. H. R. (2010). High Arctic plant community resists 15 years of experimental warming. J. Ecol. 98, 1035–1041. doi:10.1111/j.1365-2745.2010.01690.x
Huemmrich, K. F., Gamon, J., Campbell, P., Mora, M., Vargas Z, S., Almanza, B., et al. (2023). 20 years of change in tundra NDVI from coupled field and satellite observations. Environ. Res. Lett. 18, 094022. doi:10.1088/1748-9326/acee17
Huemmrich, K. F., Vargas Zesati, S., Campbell, P., and Tweedie, C. (2021). Canopy reflectance models illustrate varying NDVI responses to change in high latitude ecosystems. Ecol. Appl. 31, e02435. doi:10.1002/eap.2435
Huete, A., Didan, K., Miura, T., Rodriguez, E. P., Gao, X., and Ferreira, L. G. (2002). Overview of the radiometric and biophysical performance of the MODIS vegetation indices. Remote Sens. Environ. 83, 195–213. doi:10.1016/S0034-4257(02)00096-2
Ims, R. A., Henden, J.-A., Strømeng, M. A., Thingnes, A. V., Garmo, M. J., and Jepsen, J. U. (2019). Arctic greening and bird nest predation risk across tundra ecotones. Nat. Clim. Change 9, 607–610. doi:10.1038/s41558-019-0514-9
Irrgang, A. M., Bendixen, M., Farquharson, L. M., Baranskaya, A. V., Erikson, L. H., Gibbs, A. E., et al. (2022). Drivers, dynamics and impacts of changing Arctic coasts. Nat. Rev. Earth and Environ. 3, 39–54. doi:10.1038/s43017-021-00232-1
Isaksen, K., Nordli, Ø., Ivanov, B., Køltzow, M. A. Ø., Aaboe, S., Gjelten, H. M., et al. (2022). Exceptional warming over the Barents area. Sci. Rep. 12, 9371. doi:10.1038/s41598-022-13568-5
Jandt, R., Joly, K., Meyers, C. R., and Racine, C. (2008). Slow recovery of lichen on burned caribou winter range in Alaska tundra: potential influences of climate warming and other disturbance factors. Arct. Antarct. Alp. Res. 40, 89–95. doi:10.1657/1523-0430(06-122)[jandt]2.0.co;2
Jespersen, R. G., Anderson-Smith, M., Sullivan, P. F., Dial, R. J., and Welker, J. M. (2023). NDVI changes in the Arctic: functional significance in the moist acidic tundra of Northern Alaska. PLoS ONE 18, e0285030. doi:10.1371/journal.pone.0285030
Jia, G. J., Epstein, H. E., and Walker, D. A. (2003). Greening of arctic Alaska, 1981–2001. Geophys. Res. Lett. 30, 2067. doi:10.1029/2003GL018268
Jiang, Z., Huete, A. R., Didan, K., and Miura, T. (2008). Development of a two-band enhanced vegetation index without a blue band. Remote Sens. Environ. 112, 3833–3845. doi:10.1016/j.rse.2008.06.006
Joly, K., Jandt, R. R., and Klein, D. R. (2009). Decrease of lichens in Arctic ecosystems: the role of wildfire, caribou, reindeer, competition and climate in north-western Alaska. Polar Res. 28, 433–442. doi:10.1111/j.1751-8369.2009.00113.x
Jones, B. M., Arp, C. D., Grosse, G., Nitze, I., Lara, M. J., Whitman, M. S., et al. (2020). Identifying historical and future potential lake drainage events on the western Arctic coastal plain of Alaska. Permafr. Periglac. Process. 31, 110–127. doi:10.1002/ppp.2038
Jones, B. M., Breen, A. L., Gaglioti, B. V., Mann, D. H., Rocha, A. V., Grosse, G., et al. (2013). Identification of unrecognized tundra fire events on the north slope of Alaska. J. Geophys. Res. Biogeosciences 118, 1334–1344. doi:10.1002/jgrg.20113
Jones, B. M., Grosse, G., Arp, C. D., Jones, M. C., Walter Anthony, K. M., and Romanovsky, V. E. (2011). Modern thermokarst lake dynamics in the continuous permafrost zone, northern Seward Peninsula, Alaska. J. Geophys. Res. 116, G00M03. doi:10.1029/2011JG001666
Jones, B. M., Grosse, G., Farquharson, L. M., Roy-Léveillée, P., Veremeeva, A., Kanevskiy, M. Z., et al. (2022). Lake and drained lake basin systems in lowland permafrost regions. Nat. Rev. Earth and Environ. 3, 85–98. doi:10.1038/s43017-021-00238-9
Jones, B. M., Kanevskiy, M. Z., Shur, Y., Gaglioti, B. V., Jorgenson, M. T., Ward Jones, M. K., et al. (2024). Post-fire stabilization of thaw-affected permafrost terrain in northern Alaska. Sci. Rep. 14, 8499. doi:10.1038/s41598-024-58998-5
Jones, B. M., Schaeffer Tessier, S., Tessier, T., Brubaker, M., Brook, M., Schaeffer, J., et al. (2023). Integrating local environmental observations and remote sensing to better understand the life cycle of a thermokarst lake in Arctic Alaska. Arct. Antarct. Alp. Res. 55, 2195518. doi:10.1080/15230430.2023.2195518
Jones, B. M., Tape, K. D., Clark, J. A., Bondurant, A. C., Ward Jones, M. K., Gaglioti, B. V., et al. (2021). Multi-dimensional remote sensing analysis documents beaver-induced permafrost degradation, Seward Peninsula, Alaska. Remote Sens. 13, 4863. doi:10.3390/rs13234863
Jørgensen, R. H., Meilby, H., and Kollmann, J. (2013). Shrub expansion in SW Greenland under modest regional warming: disentangling effects of human disturbance and grazing. Arct. Antarct. Alp. Res. 45, 515–525. doi:10.1657/1938-4246-45.4.515
Jorgenson, J. C., Raynolds, M. K., Reynolds, J. H., and Benson, A.-M. (2015). Twenty-five year record of changes in plant cover on tundra of northeastern Alaska. Arct. Antarct. Alp. Res. 47, 785–806. doi:10.1657/aaar0014-097
Jorgenson, M. T., Kanevskiy, M., Shur, Y., Moskalenko, N., Brown, D. R. N., Wickland, K., et al. (2015). Role of ground ice dynamics and ecological feedbacks in recent ice wedge degradation and stabilization. J. Geophys. Res. Earth Surf. 120, 2280–2297. doi:10.1002/2015JF003602
Jorgenson, M. T., Kanevskiy, M. Z., Jorgenson, J. C., Liljedahl, A., Shur, Y., Epstein, H., et al. (2022). Rapid transformation of tundra ecosystems from ice-wedge degradation. Glob. Planet. Change 216, 103921. doi:10.1016/j.gloplacha.2022.103921
Jorgenson, M. T., Shur, Y. L., and Pullman, E. R. (2006). Abrupt increase in permafrost degradation in Arctic Alaska. Geophys. Res. Lett. 33. doi:10.1029/2005GL024960
Justice, C. O., Townshend, J. R. G., Holben, B. N., and Tucker, C. J. (1985). Analysis of the phenology of global vegetation using meteorological satellite data. Int. J. Remote Sens. 6, 1271–1318. doi:10.1080/01431168508948281
Kalluri, S., Cao, C., Heidinger, A., Ignatov, A., Key, J., and Smith, T. (2021). The advanced very high resolution radiometer: contributing to earth observations for over 40 years. Bull. Am. Meteorological Soc. 102, E351–E366. doi:10.1175/BAMS-D-20-0088.1
Kanevskiy, M., Shur, Y., Jorgenson, T., Brown, D. R. N., Moskalenko, N., Brown, J., et al. (2017). Degradation and stabilization of ice wedges: implications for assessing risk of thermokarst in northern Alaska. Geomorphology 297, 20–42. doi:10.1016/j.geomorph.2017.09.001
Kapfer, J., and Grytnes, J.-A. (2017). Large climate change, large effect? Vegetation changes over the past century in the European High Arctic. Appl. Veg. Sci. 20, 204–214. doi:10.1111/avsc.12280
Karami, M., Westergaard-Nielsen, A., Normand, S., Treier, U. A., Elberling, B., and Hansen, B. U. (2018). A phenology-based approach to the classification of Arctic tundra ecosystems in Greenland. ISPRS J. Photogrammetry Remote Sens. 146, 518–529. doi:10.1016/j.isprsjprs.2018.11.005
Karlsen, S. R., Anderson, H. B., Van Der Wal, R., and Hansen, B. B. (2018). A new NDVI measure that overcomes data sparsity in cloud-covered regions predicts annual variation in ground-based estimates of high arctic plant productivity. Environ. Res. Lett. 13, 025011. doi:10.1088/1748-9326/aa9f75
Karlsen, S. R., Elvebakk, A., Stendardi, L., Høgda, K. A., and Macias-Fauria, M. (2024). Greening of svalbard. Sci. Total Environ. 945, 174130. doi:10.1016/j.scitotenv.2024.174130
Karlsen, S. R., Elvebakk, A., Tømmervik, H., Belda, S., and Stendardi, L. (2022). Changes in onset of vegetation growth on Svalbard, 2000–2020. Remote Sens. 14, 6346. doi:10.3390/rs14246346
Kaufman, D. S., Schneider, D. P., McKay, N. P., Ammann, C. M., Bradley, R. S., Briffa, K. R., et al. (2009). Recent warming reverses long-term Arctic cooling. Science 325, 1236–1239. doi:10.1126/science.1173983
Keenan, T. F., and Riley, W. J. (2018). Greening of the land surface in the world’s cold regions consistent with recent warming. Nat. Clim. Change 8, 825–828. doi:10.1038/s41558-018-0258-y
Kharuk, V. I., Dvinskaya, M. L., Im, S. T., Golyukov, A. S., and Smith, K. T. (2022). Wildfires in the siberian arctic. Fire 5, 106. doi:10.3390/fire5040106
Klupar, I., Rocha, A. V., and Rastetter, E. B. (2021). Alleviation of nutrient co-limitation induces regime shifts in post-fire community composition and productivity in Arctic tundra. Glob. Change Biol. 27, 3324–3335. doi:10.1111/gcb.15646
Kokelj, S. V., Gingras-Hill, T., Daly, S. V., Morse, P., Wolfe, S., Rudy, A. C. A., et al. (2023). The Northwest Territories Thermokarst Mapping Collective: a northern-driven mapping collaborative toward understanding the effects of permafrost thaw. Arct. Sci. AS-2023-0009. doi:10.1139/AS-2023-0009
Kruse, S., and Herzschuh, U. (2022). Regional opportunities for tundra conservation in the next 1000 years. eLife 11, e75163. doi:10.7554/eLife.75163
Kruse, S., Shevtsova, I., Heim, B., Pestryakova, L. A., Zakharov, E. S., and Herzschuh, U. (2023). Tundra conservation challenged by forest expansion in a complex mountainous treeline ecotone as revealed by spatially explicit tree aboveground biomass modeling. Arct. Antarct. Alp. Res. 55, 2220208. doi:10.1080/15230430.2023.2220208
Ksenofontov, S., Backhaus, N., and Schaepman-Strub, G. (2019). There are new species’: indigenous knowledge of biodiversity change in Arctic Yakutia. Polar Geogr. 42, 34–57. doi:10.1080/1088937X.2018.1547326
Kuhn, C., and Butman, D. (2021). Declining greenness in Arctic-boreal lakes. Proc. Natl. Acad. Sci. U. S. A. 118, e2021219118. doi:10.1073/pnas.2021219118
Kumpula, T., Forbes, B. C., Stammler, F., and Meschtyb, N. (2012). Dynamics of a coupled system: multi-resolution remote sensing in assessing social-ecological responses during 25 Years of gas field development in arctic Russia. Remote Sens. 4, 1046–1068. doi:10.3390/rs4041046
Kumpula, T., Pajunen, A., Kaarlejärvi, E., Forbes, B. C., and Stammler, F. (2011). Land use and land cover change in Arctic Russia: ecological and social implications of industrial development. Glob. Environ. Change 21, 550–562. doi:10.1016/j.gloenvcha.2010.12.010
Lantz, T. C. (2017). Vegetation succession and environmental conditions following catastrophic lake drainage in Old Crow Flats, Yukon. Arctic 70, 177–189. doi:10.14430/arctic4646
Lantz, T. C., Gergel, S. E., and Kokelj, S. V. (2010). Spatial heterogeneity in the shrub tundra ecotone in the Mackenzie Delta region, Northwest Territories: implications for Arctic environmental change. Ecosystems 13, 194–204. doi:10.1007/s10021-009-9310-0
Lantz, T. C., Kokelj, S. V., Gergel, S. E., and Henry, G. H. R. (2009). Relative impacts of disturbance and temperature: persistent changes in microenvironment and vegetation in retrogressive thaw slumps. Glob. Change Biol. 15, 1664–1675. doi:10.1111/j.1365-2486.2009.01917.x
Lantz, T. C., Marsh, P., and Kokelj, S. V. (2013). Recent shrub proliferation in the Mackenzie Delta uplands and microclimatic implications. Ecosystems 16, 47–59. doi:10.1007/s10021-012-9595-2
Laptander, R., Stammler, F. M., Forbes, B., and Stark, S. (2024). Ways of identifying lichen and plant species by the Nenets reindeer herders in Yamal. Arct. Sci. as-2023-0046. doi:10.1139/as-2023-0046
Lara, M. J., Chen, Y., and Jones, B. M. (2021). Recent warming reverses forty-year decline in catastrophic lake drainage and hastens gradual lake drainage across northern Alaska. Environ. Res. Lett. 16, 124019. doi:10.1088/1748-9326/ac3602
Lara, M. J., Johnson, D. R., Andresen, C., Hollister, R. D., and Tweedie, C. E. (2017). Peak season carbon exchange shifts from a sink to a source following 50+ years of herbivore exclusion in an Arctic tundra ecosystem. J. Ecol. 105, 122–131. doi:10.1111/1365-2745.12654
Lara, M. J., Nitze, I., Grosse, G., Martin, P., and McGuire, A. D. (2018). Reduced arctic tundra productivity linked with landform and climate change interactions. Sci. Rep. 8, 2345. doi:10.1038/s41598-018-20692-8
Larking, T., Davis, E., Way, R., Hermanutz, L., and Trant, A. (2021). Recent greening driven by species-specific shrub growth characteristics in Nunatsiavut, Labrador, Canada. Arct. Sci. 7, 781–797. doi:10.1139/as-2020-0031
Lashchinskiy, N. N., Kartoziia, A. A., and Faguet, A. N. (2020). Permafrost degradation as a supporting factor for the biodiversity of tundra ecosystems. Contemp. Problems Ecol. 13, 401–411. doi:10.1134/S1995425520040071
Lecavalier, B. S., Milne, G. A., Simpson, M. J. R., Wake, L., Huybrechts, P., Tarasov, L., et al. (2014). A model of Greenland ice sheet deglaciation constrained by observations of relative sea level and ice extent. Quat. Sci. Rev. 102, 54–84. doi:10.1016/j.quascirev.2014.07.018
Leibman, M., Khomutov, A., Gubarkov, A., Mullanurov, D., and Dvornikov, Y. (2015). The research station “Vaskiny Dachi”. Central Yamal, West Sib. Russ. – a Rev. 25 years Permafr. Stud. Fennia 193, 3–30. doi:10.11143/45201
Lewkowicz, A. G., and Way, R. G. (2019). Extremes of summer climate trigger thousands of thermokarst landslides in a High Arctic environment. Nat. Commun. 10, 1329. doi:10.1038/s41467-019-09314-7
Liljedahl, A. K., Boike, J., Daanen, R. P., Fedorov, A. N., Frost, G. V., Grosse, G., et al. (2016). Pan-Arctic ice-wedge degradation in warming permafrost and its influence on tundra hydrology. Nat. Geosci. 9, 312–318. doi:10.1038/ngeo2674
Liljedahl, A. K., Timling, I., Frost, G. V., and Daanen, R. P. (2020). Arctic riparian shrub expansion indicates a shift from streams gaining water to those that lose flow. Commun. Earth and Environ. 1, 50. doi:10.1038/s43247-020-00050-1
Lin, D. H., Johnson, D. R., Andresen, C., and Tweedie, C. E. (2012). High spatial resolution decade-time scale land cover change at multiple locations in the Beringian Arctic (1948–2000s). Environ. Res. Lett. 7, 025502. doi:10.1088/1748-9326/7/2/025502
Lindén, E., Gough, L., and Olofsson, J. (2021). Large and small herbivores have strong effects on tundra vegetation in Scandinavia and Alaska. Ecol. Evol. 11, 12141–12152. doi:10.1002/ece3.7977
Lindgren, P. R., Farquharson, L. M., Romanovsky, V. E., and Grosse, G. (2021). Landsat-based lake distribution and changes in western Alaska permafrost regions between the 1970s and 2010s. Environ. Res. Lett. 16, 025006. doi:10.1088/1748-9326/abd270
Lisovski, S., Runge, A., Shevtsova, I., Landgraf, N., Morgenstern, A., Okoth, R. R., et al. (2023). A new habitat map of the Lena Delta in Arctic Siberia based on field and remote sensing datasets. doi:10.5194/essd-2023-36
Liu, A., Chen, Y., and Cheng, X. (2023). Effects of thermokarst lake drainage on localized vegetation greening in the Yamal–Gydan tundra ecoregion. Remote Sens. 15, 4561. doi:10.3390/rs15184561
Liu, C., Huang, H., Liu, C., Wang, X., and Wang, S. (2024). Comparative evaluation of vegetation greenness trends over circumpolar Arctic tundra using multi-sensors satellite datasets. Int. J. Digital Earth 17, 2328823. doi:10.1080/17538947.2024.2328823
Liu, C., Huang, H., and Sun, F. (2021). A pixel-based vegetation greenness trend analysis over the Russian tundra with all available Landsat data from 1984 to 2018. Remote Sens. 13, 4933. doi:10.3390/rs13234933
Liu, X.-Y., Koba, K., Koyama, L. A., Hobbie, S. E., Weiss, M. S., Inagaki, Y., et al. (2018). Nitrate is an important nitrogen source for Arctic tundra plants. Proc. Natl. Acad. Sci. U.S.A. 115, 3398–3403. doi:10.1073/pnas.1715382115
Liu, Y., Key, J. R., Liu, Z., Wang, X., and Vavrus, S. J. (2012). A cloudier Arctic expected with diminishing sea ice. Geophys. Res. Lett. 39, L05705. doi:10.1029/2012GL051251
López-Blanco, E., Topp-Jørgensen, E., Christensen, T. R., Rasch, M., Skov, H., Arndal, M. F., et al. (2024). Towards an increasingly biased view on Arctic change. Nat. Clim. Chang. 14, 152–155. doi:10.1038/s41558-023-01903-1
Loranty, M. M., Abbott, B. W., Blok, D., Douglas, T. A., Epstein, H. E., Forbes, B. C., et al. (2018a). Reviews and syntheses: changing ecosystem influences on soil thermal regimes in northern high-latitude permafrost regions. Biogeosciences Discuss., 1–56. doi:10.5194/bg-2018-201
Loranty, M. M., Davydov, S. P., Kropp, H., Alexander, H. D., Mack, M. C., Natali, S. M., et al. (2018b). Vegetation indices do not capture forest cover variation in upland Siberian larch forests. Remote Sens. 10, 1686. doi:10.3390/rs10111686
Lucht, W., Prentice, I. C., Myneni, R. B., Sitch, S., Friedlingstein, P., Cramer, W., et al. (2002). Climatic control of the high-latitude vegetation greening trend and Pinatubo effect. Science 296, 1687–1689. doi:10.1126/science.1071828
Lund, M., Raundrup, K., Westergaard-Nielsen, A., López-Blanco, E., Nymand, J., and Aastrup, P. (2017). Larval outbreaks in West Greenland: instant and subsequent effects on tundra ecosystem productivity and CO2 exchange. Ambio 46, 26–38. doi:10.1007/s13280-016-0863-9
Luojus, K., Pulliainen, J., Takala, M., Lemmetyinen, J., Mortimer, C., Derksen, C., et al. (2021). GlobSnow v3.0 Northern Hemisphere snow water equivalent dataset. Sci. Data 8, 163. doi:10.1038/s41597-021-00939-2
Lyons, J. E., Brown, S. C., Saalfeld, S. T., Johnson, J. A., Andres, B. A., Sowl, K. M., et al. (2024). Alaska’s climate sensitive Yukon–Kuskokwim Delta supports seven million Arctic-breeding shorebirds, including the majority of six North American populations. Ornithol. Appl. 126, duad066. doi:10.1093/ornithapp/duad066
Macander, M. J., Nelson, P. R., Nawrocki, T. W., Frost, G. V., Orndahl, K. M., Palm, E. C., et al. (2022). Time-series maps reveal widespread change in plant functional type cover across Arctic and boreal Alaska and Yukon. Environ. Res. Lett. 17, 054042. doi:10.1088/1748-9326/ac6965
Macias-Fauria, M., Karlsen, S. R., and Forbes, B. C. (2017). Disentangling the coupling between sea ice and tundra productivity in Svalbard. Sci. Rep. 7, 8586. doi:10.1038/s41598-017-06218-8
Magnússon, R. Í., Groten, F., Bartholomeus, H., Van Huissteden, K., and Heijmans, M. M. P. D. (2023). Tundra browning in the Indigirka Lowlands (north-eastern Siberia) explained by drought, floods and small-scale vegetation shifts. JGR Biogeosciences 128, e2022JG007330. doi:10.1029/2022JG007330
Magnússon, R. Í., Limpens, J., Kleijn, D., van Huissteden, K., Maximov, T. C., Lobry, S., et al. (2021a). Shrub decline and expansion of wetland vegetation revealed by very high resolution land cover change detection in the Siberian lowland tundra. Sci. Total Environ. 782, 146877. doi:10.1016/j.scitotenv.2021.146877
Magnússon, R. Í., Limpens, J., Kleijn, D., van Huissteden, K., Maximov, T. C., Lobry, S., et al. (2021b). Shrub decline and expansion of wetland vegetation revealed by very high resolution land cover change detection in the Siberian lowland tundra. Sci. Total Environ. 782, 146877. doi:10.1016/j.scitotenv.2021.146877
Margold, M., Stokes, C. R., and Clark, C. D. (2018). Reconciling records of ice streaming and ice margin retreat to produce a palaeogeographic reconstruction of the deglaciation of the Laurentide Ice Sheet. Quat. Sci. Rev. 189, 1–30. doi:10.1016/j.quascirev.2018.03.013
Mayewski, P. A., Sneed, S. B., Birkel, S. D., Kurbatov, A. V., and Maasch, K. A. (2014). Holocene warming marked by abrupt onset of longer summers and reduced storm frequency around Greenland. J Quat. Sci. 29, 99–104. doi:10.1002/jqs.2684
Meltofte, H. (2013). in Arctic biodiversity assessment: status and trends in arctic biodiversity. Akureyri (Iceland: The Conservation of Arctic Flora and Fauna).
Melvin, A. (2019). in Understanding northern latitude vegetation greening and browning: proceedings of a workshop (Washington, DC: The National Academies Press). doi:10.17226/25423
Metcalfe, D. B., Hermans, T. D. G., Ahlstrand, J., Becker, M., Berggren, M., Björk, R. G., et al. (2018). Patchy field sampling biases understanding of climate change impacts across the Arctic. Nat. Ecol. and Evol. 2, 1443–1448. doi:10.1038/s41559-018-0612-5
Michelsen, A., Jonasson, S., Sleep, D., Havstrm, M., and Callaghan, T. V. (1996). Shoot biomass, ?13C, nitrogen and chlorophyll responses of two arctic dwarf shrubs to in situ shading, nutrient application and warming simulating climatic change. Oecologia 105, 1–12. doi:10.1007/BF00328785
Miner, K. R., Turetsky, M. R., Malina, E., Bartsch, A., Tamminen, J., McGuire, A. D., et al. (2022). Permafrost carbon emissions in a changing Arctic. Nat. Rev. Earth and Environ. 3, 55–67. doi:10.1038/s43017-021-00230-3
Mizel, J. D., and Swanson, D. K. (2022). Hindcasts of passerine density in arctic and subarctic Alaska suggest noncomplementary responses to shrub expansion by tundra- and shrub-adapted species. Arct. Antarct. Alp. Res. 54, 25–39. doi:10.1080/15230430.2022.2034373
Moffat, N. D., Lantz, T. C., Fraser, R. H., and Olthof, I. (2016). Recent vegetation change (1980–2013) in the tundra ecosystems of the Tuktoyaktuk coastlands, NWT, Canada. Arct. Antarct. Alp. Res. 48, 581–597. doi:10.1657/AAAR0015-063
Mortier, S., Hamedpour, A., Bussmann, B., Wandji, R. P. T., Latré, S., Sigurdsson, B. D., et al. (2024). Inferring the relationship between soil temperature and the normalized difference vegetation index with machine learning. Ecol. Inf. 82, 102730. doi:10.1016/j.ecoinf.2024.102730
Mosites, E., Lujan, E., Brook, M., Brubaker, M., Roehl, D., Tcheripanoff, M., et al. (2018). Environmental observation, social media, and one health action: a description of the local environmental observer (LEO) network. One Health 6, 29–33. doi:10.1016/j.onehlt.2018.10.002
Myers-Smith, I. H., Elmendorf, S. C., Beck, P. S. A., Wilmking, M., Hallinger, M., Blok, D., et al. (2015). Climate sensitivity of shrub growth across the tundra biome. Nat. Clim. Change 5, 887–891. doi:10.1038/nclimate2697
Myers-Smith, I. H., Forbes, B. C., Wilmking, M., Hallinger, M., Lantz, T., Blok, D., et al. (2011). Shrub expansion in tundra ecosystems: dynamics, impacts and research priorities. Environ. Res. Lett. 6, 045509. doi:10.1088/1748-9326/6/4/045509
Myers-Smith, I. H., Grabowski, M. M., Thomas, H. J. D., Angers-Blondin, S., Daskalova, G. N., Bjorkman, A. D., et al. (2019). Eighteen years of ecological monitoring reveals multiple lines of evidence for tundra vegetation change. Ecol. Monogr. 89, e01351. doi:10.1002/ecm.1351
Myers-Smith, I. H., Kerby, J. T., Phoenix, G. K., Bjerke, J. W., Epstein, H. E., Assmann, J. J., et al. (2020). Complexity revealed in the greening of the Arctic. Nat. Clim. Change 10, 106–117. doi:10.1038/s41558-019-0688-1
Myneni, R. B., Keeling, C. D., Tucker, C. J., Asrar, G., and Nemani, R. R. (1997). Increased plant growth in the northern high latitudes from 1981 to 1991. Nature 386, 698–702. doi:10.1038/386698a0
Naito, A. T., and Cairns, D. M. (2011). Relationships between Arctic shrub dynamics and topographically derived hydrologic characteristics. Environ. Res. Lett. 6, 045506. doi:10.1088/1748-9326/6/4/045506
Naito, A. T., and Cairns, D. M. (2015). Patterns of shrub expansion in Alaskan arctic river corridors suggest phase transition. Ecol. Evol. 5, 87–101. doi:10.1002/ece3.1341
Narita, K., Harada, K., Saito, K., Sawada, Y., Fukuda, M., and Tsuyuzaki, S. (2015). Vegetation and permafrost thaw depth 10 years after a tundra fire in 2002, Seward Peninsula, Alaska. Arct. Antarct. Alp. Res. 47, 547–559. doi:10.1657/AAAR0013-031
Nelson, P. R., Maguire, A. J., Pierrat, Z., Orcutt, E. L., Yang, D., Serbin, S., et al. (2022). Remote sensing of tundra ecosystems using high spectral resolution reflectance: opportunities and challenges. JGR Biogeosciences 127. doi:10.1029/2021JG006697
Nitze, I., Cooley, S. W., Duguay, C. R., Jones, B. M., and Grosse, G. (2020). The catastrophic thermokarst lake drainage events of 2018 in northwestern Alaska: fast-forward into the future. Cryosphere 14, 4279–4297. doi:10.5194/tc-14-4279-2020
Nitze, I., and Grosse, G. (2016). Detection of landscape dynamics in the Arctic Lena Delta with temporally dense Landsat time-series stacks. Remote Sens. Environ. 181, 27–41. doi:10.1016/j.rse.2016.03.038
Nitze, I., Grosse, G., Jones, B. M., Romanovsky, V. E., and Boike, J. (2018). Remote sensing quantifies widespread abundance of permafrost region disturbances across the Arctic and Subarctic. Nat. Commun. 9, 5423. doi:10.1038/s41467-018-07663-3
Nitze, I., Lübker, T., and Grosse, G. (2024). Pan-Arctic visualization of landscape change (2003-2022). Arct. PASSION Permafr. Serv. doi:10.1594/PANGAEA.964814
Nogovitcyn, A., Shakhmatov, R., Morozumi, T., Tei, S., Miyamoto, Y., Shin, N., et al. (2023). Historical variation in the normalized difference vegetation index compared with soil moisture in a taiga forest ecosystem in northeastern Siberia. Biogeosciences 20, 3185–3201. doi:10.5194/bg-20-3185-2023
Nordli, Ø., Wyszyński, P., Gjelten, H. M., Isaksen, K., Łupikasza, E., Niedźwiedź, T., et al. (2020). Revisiting the extended Svalbard Airport monthly temperature series, and the compiled corresponding daily series 1898–2018. Polar Res. 39. doi:10.33265/polar.v39.3614
Ohta, T., Kotani, A., Iijima, Y., Maximov, T. C., Ito, S., Hanamura, M., et al. (2014). Effects of waterlogging on water and carbon dioxide fluxes and environmental variables in a Siberian larch forest, 1998–2011. Agric. For. Meteorology 188, 64–75. doi:10.1016/j.agrformet.2013.12.012
Olofsson, J., Tømmervik, H., and Callaghan, T. V. (2012). Vole and lemming activity observed from space. Nat. Clim. Change 2, 880–883. doi:10.1038/nclimate1537
Overbeck, J. R., Buzard, R. M., Turner, M. M., Miller, K. Y., and Glenn, R. J. (2020). Shoreline change at Alaska coastal communities. Alaska Div. Geol. and Geophys. Surv. doi:10.14509/30552
Overland, J. E., and Wang, M. (2005). The third Arctic climate pattern: 1930s and early 2000s. Geophys. Res. Lett. 32, 2005GL024254. doi:10.1029/2005GL024254
Overland, J. E., and Wang, M. (2013). When will the summer Arctic be nearly sea ice free? Geophys. Res. Lett. 40, 2097–2101. doi:10.1002/grl.50316
Overland, J. E., and Wang, M. (2021). The 2020 Siberian heat wave. Intl J. Climatol. 41. doi:10.1002/joc.6850
Park, T., Ganguly, S., Tømmervik, H., Euskirchen, E. S., Høgda, K.-A., Karlsen, S. R., et al. (2016). Changes in growing season duration and productivity of northern vegetation inferred from long-term remote sensing data. Environ. Res. Lett. 11, 084001. doi:10.1088/1748-9326/11/8/084001
Parmentier, F.-J. W., Nilsen, L., Tømmervik, H., and Cooper, E. J. (2021). A distributed time-lapse camera network to track vegetation phenology with high temporal detail and at varying scales. Earth Syst. Sci. Data 13, 3593–3606. doi:10.5194/essd-13-3593-2021
Pattison, R. R., Jorgenson, J. C., Raynolds, M. K., and Welker, J. M. (2015). Trends in NDVI and tundra community composition in the arctic of NE Alaska between 1984 and 2009. Ecosystems 18, 707–719. doi:10.1007/s10021-015-9858-9
Phoenix, G. K., and Bjerke, J. W. (2016). Arctic browning: extreme events and trends reversing arctic greening. Glob. Change Biol. 22, 2960–2962. doi:10.1111/gcb.13261
Phoenix, G. K., Bjerke, J. W., Björk, R. G., Blok, D., Bryn, A., Callaghan, T. V., et al. (2025). Browning events in Arctic ecosystems: diverse causes with common consequences. PLOS Clim. 4, e0000570. doi:10.1371/journal.pclm.0000570
Pinzon, J., and Tucker, C. (2014). A non-stationary 1981–2012 AVHRR NDVI3g time series. Remote Sens. 6, 6929–6960. doi:10.3390/rs6086929
Pinzon, J. E., Tucker, C. J., Bhatt, U. S., Frost, G. V., and Macander, M. J. (2023). Global vegetation greenness (NDVI) from AVHRR GIMMS-3g+. NASA's Open Data Portal, 1981–2022. doi:10.3334/ORNLDAAC/2187
Pitelka, F. A., and Batzli, G. O. (2007). Population cycles of lemmings near Barrow, Alaska: a historical review. Acta Theriol. 52, 323–336. doi:10.1007/BF03194229
Polyakov, I. V., Ingvaldsen, R. B., Pnyushkov, A. V., Bhatt, U. S., Francis, J. A., Janout, M., et al. (2023). Fluctuating Atlantic inflows modulate Arctic atlantification. Science 381, 972–979. doi:10.1126/science.adh5158
Post, E., Cahoon, S. M. P., Kerby, J. T., Pedersen, C., and Sullivan, P. F. (2021). Herbivory and warming interact in opposing patterns of covariation between arctic shrub species at large and local scales. Proc. Natl. Acad. Sci. U.S.A. 118, e2015158118. doi:10.1073/pnas.2015158118
Potravny, I. M., and Elsakov, V. V. (2024). Analysis of trends in the development of traditional trades of indigenous peoples in the context of climate change (using the example of the Novosibirsk Islands and the coastal Arctic regions of Yakutia). Arctic: Ecology and Economy. 14, 301–311. doi:10.25283/2223-4594-2024-2-301-311
Potter, C., and Alexander, O. (2020). Changes in vegetation phenology and productivity in Alaska over the past two decades. Remote Sens. 12, 1546. doi:10.3390/rs12101546
Prach, K., Košnar, J., Klimešová, J., and Hais, M. (2010). High Arctic vegetation after 70 years: a repeated analysis from Svalbard. Polar Biol. 33, 635–639. doi:10.1007/s00300-009-0739-6
Prevéy, J. S., Rixen, C., Rüger, N., Høye, T. T., Bjorkman, A. D., Myers-Smith, I. H., et al. (2019). Warming shortens flowering seasons of tundra plant communities. Nat. Ecol. and Evol. 3, 45–52. doi:10.1038/s41559-018-0745-6
Racine, C., Jandt, R., Meyers, C., and Dennis, J. (2004). Tundra fire and vegetation change along a hillslope on the Seward Peninsula, Alaska, USA. Arct. Antarct. Alp. Res. 36, 1–10. doi:10.1657/1523-0430(2004)036[0001:tfavca]2.0.co;2
Racine, C. H. (1981). Tundra fire effects on soils and three plant communities along a hill-slope gradient in the Seward Peninsula, Alaska. Arctic 34, 71–84. doi:10.14430/arctic2508
Rantanen, M., Karpechko, A.Yu., Lipponen, A., Nordling, K., Hyvärinen, O., Ruosteenoja, K., et al. (2022). The Arctic has warmed nearly four times faster than the globe since 1979. Commun. Earth and Environ. 3, 168. doi:10.1038/s43247-022-00498-3
Raynolds, M., Comiso, J., Walker, D., and Verbyla, D. (2008). Relationship between satellite-derived land surface temperatures, arctic vegetation types, and NDVI. Remote Sens. Environ. 112, 1884–1894. doi:10.1016/j.rse.2007.09.008
Raynolds, M. K., and Walker, D. A. (2016). Increased wetness confounds Landsat-derived NDVI trends in the central Alaska North Slope region, 1985–2011. Environ. Res. Lett. 11, 085004. doi:10.1088/1748-9326/11/8/085004
Raynolds, M. K., Walker, D. A., Ambrosius, K. J., Brown, J., Everett, K. R., Kanevskiy, M., et al. (2014). Cumulative geoecological effects of 62 years of infrastructure and climate change in ice-rich permafrost landscapes, Prudhoe Bay Oilfield, Alaska. Glob. Change Biol. 20, 1211–1224. doi:10.1111/gcb.12500
Raynolds, M. K., Walker, D. A., Balser, A., Bay, C., Campbell, M., Cherosov, M. M., et al. (2019). A raster version of the circumpolar arctic vegetation map (CAVM). Remote Sens. Environ. 232, 111297. doi:10.1016/j.rse.2019.111297
Raynolds, M. K., Walker, D. A., Epstein, H. E., Pinzon, J. E., and Tucker, C. J. (2012). A new estimate of tundra-biome phytomass from trans-Arctic field data and AVHRR NDVI. Remote Sens. Lett. 3, 403–411. doi:10.1080/01431161.2011.609188
Rearden, A., and Fienup-Riordan, A. (2014). Nunamta ellamta-llu ayuqucia: what our land and world are like. Fairbanks, AK: Alaska Native Language Center.
Rees, G., and Büntgen, U. (2024). Russian dilemma for global arctic science. Ambio 53, 1246–1250. doi:10.1007/s13280-024-02038-z
Rietze, N., Assmann, J. J., Plekhanova, E., Naegeli, K., Damm, A., Maximov, T. C., et al. (2024). Summer drought weakens land surface cooling of tundra vegetation. Environ. Res. Lett. 19, 044043. doi:10.1088/1748-9326/ad345e
Rocha, A. V., Blakely, B., Jiang, Y., Wright, K. S., and Curasi, S. R. (2018). Is arctic greening consistent with the ecology of tundra? Lessons from an ecologically informed mass balance model. Environ. Res. Lett. 13, 125007. doi:10.1088/1748-9326/aaeb50
Rocha, A. V., Loranty, M. M., Higuera, P. E., Mack, M. C., Hu, F. S., Jones, B. M., et al. (2012). The footprint of Alaskan tundra fires during the past half-century: implications for surface properties and radiative forcing. Environ. Res. Lett. 7, 044039. doi:10.1088/1748-9326/7/4/044039
Rocha, A. V., and Shaver, G. R. (2009). Advantages of a two band EVI calculated from solar and photosynthetically active radiation fluxes. Agric. For. Meteorology 149, 1560–1563. doi:10.1016/j.agrformet.2009.03.016
Rodenhizer, H., Yang, Y., Fiske, G., Potter, S., Windholz, T., Mullen, A., et al. (2024). A comparison of satellite imagery sources for automated detection of retrogressive thaw slumps. Remote Sens. 16, 2361. doi:10.3390/rs16132361
Roland, T. P., Bartlett, O. T., Charman, D. J., Anderson, K., Hodgson, D. A., Amesbury, M. J., et al. (2024). Sustained greening of the Antarctic Peninsula observed from satellites. Nat. Geosci. 17, 1121–1126. doi:10.1038/s41561-024-01564-5
Ropars, P., and Boudreau, S. (2012). Shrub expansion at the forest–tundra ecotone: spatial heterogeneity linked to local topography. Environ. Res. Lett. 7, 015501. doi:10.1088/1748-9326/7/1/015501
Runge, A., and Grosse, G. (2020). Mosaicking Landsat and sentinel-2 data to enhance LandTrendr time series analysis in northern high latitude permafrost regions. Remote Sens. 12, 2471. doi:10.3390/rs12152471
Rupp, T. S., Starfield, A. M., and Chapin, F. S. (2000). A frame-based spatially explicit model of subarctic vegetation response to climatic change: comparison with a point model. Landsc. Ecol. 15, 383–400. doi:10.1023/A:1008168418778
Sae-Lim, J., Russell, J. M., Vachula, R. S., Holmes, R. M., Mann, P. J., Schade, J. D., et al. (2019). Temperature-controlled tundra fire severity and frequency during the last millennium in the Yukon-Kuskokwim Delta, Alaska. Holocene 29, 1223–1233. doi:10.1177/0959683619838036
Salmon, V. G., Breen, A. L., Kumar, J., Lara, M. J., Thornton, P. E., Wullschleger, S. D., et al. (2019). Alder distribution and expansion across a tundra hillslope: implications for local N cycling. Front. Plant Sci. 10, 1099. doi:10.3389/fpls.2019.01099
Salmon, V. G., Soucy, P., Mauritz, M., Celis, G., Natali, S. M., Mack, M. C., et al. (2016). Nitrogen availability increases in a tundra ecosystem during five years of experimental permafrost thaw. Glob. Change Biol. 22, 1927–1941. doi:10.1111/gcb.13204
Schaefer, J. A. (2023). Increases in graminoids after three decades of change in the High Arctic. Polar Res. 42. doi:10.33265/polar.v42.9560
Scholten, R. C., Coumou, D., Luo, F., and Veraverbeke, S. (2022). Early snowmelt and polar jet dynamics co-influence recent extreme Siberian fire seasons. Science 378, 1005–1009. doi:10.1126/science.abn4419
Schore, A. I. G., Fraterrigo, J. M., Salmon, V. G., Yang, D., and Lara, M. J. (2023). Nitrogen fixing shrubs advance the pace of tall-shrub expansion in low-Arctic tundra. Commun. Earth Environ. 4, 421. doi:10.1038/s43247-023-01098-5
Schuur, E. A. G., Abbott, B. W., Commane, R., Ernakovich, J., Euskirchen, E., Hugelius, G., et al. (2022). Permafrost and climate change: carbon cycle feedbacks from the warming Arctic. Annu. Rev. Environ. Resour. 47, 343–371. doi:10.1146/annurev-environ-012220-011847
Schuur, E. A. G., Hicks Pries, C., Mauritz, M., Pegoraro, E., Rodenhizer, H., See, C., et al. (2023). Ecosystem and soil respiration radiocarbon detects old carbon release as a fingerprint of warming and permafrost destabilization with climate change. Phil. Trans. R. Soc. A 381, 20220201. doi:10.1098/rsta.2022.0201
Schuur, E. A. G., Pallandt, M., and Göckede, M. (2024). Russian collaboration loss risks permafrost carbon emissions network. Nat. Clim. Change 14, 410–411. doi:10.1038/s41558-024-02001-6
See, C. R., Virkkala, A.-M., Natali, S. M., Rogers, B. M., Mauritz, M., Biasi, C., et al. (2024). Decadal increases in carbon uptake offset by respiratory losses across northern permafrost ecosystems. Nat. Clim. Chang. 14, 853–862. doi:10.1038/s41558-024-02057-4
Seider, J. H., Lantz, T. C., Hermosilla, T., Wulder, M. A., and Wang, J. A. (2022). Biophysical determinants of shifting tundra vegetation productivity in the Beaufort Delta region of Canada. Ecosystems 25, 1435–1454. doi:10.1007/s10021-021-00725-6
Serreze, M. C., and Barry, R. G. (2011). Processes and impacts of Arctic amplification: a research synthesis. Glob. Planet. Change 77, 85–96. doi:10.1016/j.gloplacha.2011.03.004
Serreze, M. C., Gustafson, J., Barrett, A. P., Druckenmiller, M. L., Fox, S., Voveris, J., et al. (2021). Arctic rain on snow events: bridging observations to understand environmental and livelihood impacts. Environ. Res. Lett. 16, 105009. doi:10.1088/1748-9326/ac269b
Shevtsova, I., Herzschuh, U., Heim, B., Schulte, L., Stünzi, S., Pestryakova, L. A., et al. (2021). Recent above-ground biomass changes in central Chukotka (Russian Far East) using field sampling and Landsat satellite data. Biogeosciences 18, 3343–3366. doi:10.5194/bg-18-3343-2021
Siewert, M. B., and Olofsson, J. (2020). Scale-dependency of Arctic ecosystem properties revealed by UAV. Environ. Res. Lett. 15, 094030. doi:10.1088/1748-9326/aba20b
Skarin, A., Verdonen, M., Kumpula, T., Macias-Fauria, M., Alam, M., Kerby, J., et al. (2020). Reindeer use of low Arctic tundra correlates with landscape structure. Environ. Res. Lett. 15, 115012. doi:10.1088/1748-9326/abbf15
Smith, L. C., Sheng, Y., MacDonald, G. M., and Hinzman, L. D. (2005). Disappearing arctic lakes. Science 308, 1429. doi:10.1126/science.1108142
Soininen, E. M., Barrio, I. C., Bjørkås, R., Björnsdóttir, K., Ehrich, D., Hopping, K. A., et al. (2021). Location of studies and evidence of effects of herbivory on Arctic vegetation: a systematic map. Environ. Evid. 10, 25. doi:10.1186/s13750-021-00240-0
Spiegel, M. P., Volkovitskiy, A., Terekhina, A., Forbes, B. C., Park, T., and Macias-Fauria, M. (2023). Top-down regulation by a reindeer herding system limits climate-driven Arctic vegetation change at a regional scale. Earth’s Future 11, e2022EF003407. doi:10.1029/2022EF003407
Steketee, J. K., Rocha, A. V., Gough, L., Griffin, K. L., Klupar, I., An, R., et al. (2022). Small herbivores with big impacts: tundra voles (Microtus oeconomus) alter post-fire ecosystem dynamics. Ecol. n/a 103, e3689. doi:10.1002/ecy.3689
Stonevicius, E., Stankunavicius, G., and Rimkus, E. (2018). Continentality and oceanity in the mid and high latitudes of the northern hemisphere and their links to atmospheric circulation. Adv. Meteorology 2018, 1–12. doi:10.1155/2018/5746191
Strauss, J., Biasi, C., Sanders, T., Abbott, B. W., von Deimling, T. S., Voigt, C., et al. (2022). A globally relevant stock of soil nitrogen in the Yedoma permafrost domain. Nat. Commun. 13, 6074. doi:10.1038/s41467-022-33794-9
Sturm, M., Tape, K., and Racine, C. (2001). Climate change: increasing shrub abundance in the Arctic. Nature 411, 546–547. doi:10.1038/35079180
Sundqvist, M. K., Moen, J., Björk, R. G., Vowles, T., Kytöviita, M.-M., Parsons, M. A., et al. (2019). Experimental evidence of the long-term effects of reindeer on Arctic vegetation greenness and species richness at a larger landscape scale. J. Ecol. 107, 2724–2736. doi:10.1111/1365-2745.13201
Swanson, D. (2017). Trends in greenness and snow cover in Alaska’s Arctic national parks, 2000–2016. Remote Sens. 9, 514. doi:10.3390/rs9060514
Talucci, A. C., Loranty, M. M., and Alexander, H. D. (2022). Siberian taiga and tundra fire regimes from 2001–2020. Environ. Res. Lett. 17, 025001. doi:10.1088/1748-9326/ac3f07
Tape, K., Sturm, M., and Racine, C. (2006). The evidence for shrub expansion in northern Alaska and the Pan-Arctic. Glob. Change Biol. 12, 686–702. doi:10.1111/j.1365-2486.2006.01128.x
Tape, K. D., Clark, J. A., Jones, B. M., Kantner, S., Gaglioti, B. V., Grosse, G., et al. (2022). Expanding beaver pond distribution in Arctic Alaska, 1949 to 2019. Sci. Rep. 12, 7123. doi:10.1038/s41598-022-09330-6
Tape, K. D., Gustine, D. D., Ruess, R. W., Adams, L. G., and Clark, J. A. (2016). Range expansion of moose in arctic Alaska linked to warming and increased shrub habitat. PLOS ONE 11, e0152636. doi:10.1371/journal.pone.0152636
Tape, K. D., Hallinger, M., Welker, J. M., and Ruess, R. W. (2012). Landscape heterogeneity of shrub expansion in arctic Alaska. Ecosystems 15, 711–724. doi:10.1007/s10021-012-9540-4
Tape, K. D., Jones, B. M., Arp, C. D., Nitze, I., and Grosse, G. (2018). Tundra be dammed: beaver colonization of the Arctic. Glob. Change Biol. 24, 4478–4488. doi:10.1111/gcb.14332
Tassone, M. S., Epstein, H. E., Armstrong, A. H., Bhatt, U. S., Frost, G. V., Heim, B., et al. (2024). Drivers of heterogeneity in tundra vegetation productivity on the Yamal Peninsula, Siberia, Russia. Environ. Res. Ecol. 3, 015003. doi:10.1088/2752-664X/ad220f
Tei, S., Morozumi, T., Nagai, S., Takano, S., Sugimoto, A., Shingubara, R., et al. (2020). An extreme flood caused by a heavy snowfall over the Indigirka River basin in Northeastern Siberia. Hydrol. Process. 34, 522–537. doi:10.1002/hyp.13601
Thomas, H. J. D., Bjorkman, A. D., Myers-Smith, I. H., Elmendorf, S. C., Kattge, J., Diaz, S., et al. (2020). Global plant trait relationships extend to the climatic extremes of the tundra biome. Nat. Commun. 11, 1351. doi:10.1038/s41467-020-15014-4
Thompson, D. P., and Barboza, P. S. (2014). Nutritional implications of increased shrub cover for caribou (Rangifer tarandus) in the Arctic. Can. J. Zool. 92, 339–351. doi:10.1139/cjz-2013-0265
Tømmervik, H., Julitta, T., Nilsen, L., Park, T., Burkart, A., Ostapowicz, K., et al. (2023). The northernmost hyperspectral FLoX sensor dataset for monitoring of high-Arctic tundra vegetation phenology and Sun-Induced Fluorescence (SIF). Data Brief 50, 109581. doi:10.1016/j.dib.2023.109581
Tømmervik, H., and Nilsen, L. (2023). SIOS instrument #49 - hyperspectral measurements including sun-induced fluorescence (SIF) in Adventdalen. Nor. Meteorol. Inst. doi:10.21343/ZDM7-JD72
Travers-Smith, H. Z., and Lantz, T. C. (2020). Leading-edge disequilibrium in alder and spruce populations across the forest–tundra ecotone. Ecosphere 11, e03118. doi:10.1002/ecs2.3118
Tremblay, B., Lévesque, E., and Boudreau, S. (2012). Recent expansion of erect shrubs in the low arctic: evidence from eastern Nunavik. Environ. Res. Lett. 7, 035501. doi:10.1088/1748-9326/7/3/035501
Tucker, C. J. (1979). Red and photographic infrared linear combinations for monitoring vegetation. Remote Sens. Environ. 8, 127–150. doi:10.1016/0034-4257(79)90013-0
Tucker, C. J., and Sellers, P. J. (1986). Satellite remote sensing of primary production. Int. J. Remote Sens. 16, 1395–1416. doi:10.1080/01431168608948944
Tucker, C. J., Townshend, J. R. G., and Goff, T. E. (1985). African land-cover classification using satellite data. Science 227, 369–375. doi:10.1126/science.227.4685.369
Turetsky, M. R., Abbott, B. W., Jones, M. C., Anthony, K. W., Olefeldt, D., Schuur, E. A. G., et al. (2020). Carbon release through abrupt permafrost thaw. Nat. Geosci. 13, 138–143. doi:10.1038/s41561-019-0526-0
Ukraintseva, N. G., and Leibman, M. O. (2007). “The effect of cryogenic landslides (active-layer detachments) on fertility of tundra soils on Yamal Peninsula, Russia,” in Proceedings of the 1st North American landslide conference (Vail, CO, United States: Omnipress), 1605–1615.
Van Bogaert, R., Jonasson, C., De Dapper, M., and Callaghan, T. V. (2009). Competitive interaction between aspen and birch moderated by invertebrate and vertebrate herbivores and climate warming. Plant Ecol. and Divers. 2, 221–232. doi:10.1080/17550870903487456
Verdonen, M., Berner, L. T., Forbes, B. C., and Kumpula, T. (2020). Periglacial vegetation dynamics in Arctic Russia: decadal analysis of tundra regeneration on landslides with time series satellite imagery. Environ. Res. Lett. 15, 105020. doi:10.1088/1748-9326/abb500
Veremeeva, A., Nitze, I., Günther, F., Grosse, G., and Rivkina, E. (2021). Geomorphological and climatic drivers of thermokarst lake area increase trend (1999–2018) in the Kolyma Lowland yedoma region, north-eastern Siberia. Remote Sens. 13, 178. doi:10.3390/rs13020178
Vickers, H., Høgda, K. A., Solbø, S., Karlsen, S. R., Tømmervik, H., Aanes, R., et al. (2016). Changes in greening in the high Arctic: insights from a 30 year AVHRR max NDVI dataset for Svalbard. Environ. Res. Lett. 11, 105004. doi:10.1088/1748-9326/11/10/105004
Vikhamar-Schuler, D., Isaksen, K., Haugen, J. E., Tømmervik, H., Luks, B., Schuler, T. V., et al. (2016). Changes in winter warming events in the Nordic arctic region. J. Clim. 29, 6223–6244. doi:10.1175/JCLI-D-15-0763.1
Vindstad, O. P. L., Jepsen, J. U., Molvig, H., and Ims, R. A. (2022). A pioneering pest: the winter moth (Operophtera brumata) is expanding its outbreak range into Low Arctic shrub tundra. Arct. Sci. 8, 450–470. doi:10.1139/as-2021-0027
Virkkala, A.-M., Abdi, A. M., Luoto, M., and Metcalfe, D. B. (2019). Identifying multidisciplinary research gaps across Arctic terrestrial gradients. Environ. Res. Lett. 14, 124061. doi:10.1088/1748-9326/ab4291
Von Baeckmann, C., Bartsch, A., Bergstedt, H., Efimova, A., Widhalm, B., Ehrich, D., et al. (2024). Landcover succession for recently drained lakes in permafrost on the Yamal peninsula. West. Sib. doi:10.5194/egusphere-2024-699
Walker, D. A., Epstein, H. E., Jia, G. J., Balser, A., Copass, C., Edwards, E. J., et al. (2003). Phytomass, LAI, and NDVI in northern Alaska: relationships to summer warmth, soil pH, plant functional types, and extrapolation to the circumpolar Arctic. J. Geophys. Res. 108, 8169. doi:10.1029/2001JD000986
Walker, D. A., Leibman, M. O., Epstein, H. E., Forbes, B. C., Bhatt, U. S., Raynolds, M. K., et al. (2009). Spatial and temporal patterns of greenness on the Yamal Peninsula, Russia: interactions of ecological and social factors affecting the Arctic normalized difference vegetation index. Environ. Res. Lett. 4, 045004. doi:10.1088/1748-9326/4/4/045004
Walker, D. A., Raynolds, M. K., Daniëls, F. J. A., Einarsson, E., Elvebakk, A., Gould, W. A., et al. (2005a). The Circumpolar Arctic vegetation map. J. Veg. Sci. 16, 267–282. doi:10.1111/j.1654-1103.2005.tb02365.x
Walker, D. A., Raynolds, M. K., Daniëls, F. J. A., Einarsson, E., Elvebakk, A., Gould, W. A., et al. (2005b). The circumpolar Arctic vegetation map. J. Veg. Sci. 16, 267–282. doi:10.1658/1100-9233(2005)016[0267:tcavm]2.0.co;2
Wang, P., Limpens, J., Mommer, L., van Ruijven, J., Nauta, A. L., Berendse, F., et al. (2017). Above- and below-ground responses of four tundra plant functional types to deep soil heating and surface soil fertilization. J. Ecol. 105, 947–957. doi:10.1111/1365-2745.12718
Webb, E. E., Liljedahl, A. K., Cordeiro, J. A., Loranty, M. M., Witharana, C., and Lichstein, J. W. (2022). Permafrost thaw drives surface water decline across lake-rich regions of the Arctic. Nat. Clim. Change 12, 841–846. doi:10.1038/s41558-022-01455-w
Westergaard-Nielsen, A., Lund, M., Pedersen, S. H., Schmidt, N. M., Klosterman, S., Abermann, J., et al. (2017). Transitions in high-Arctic vegetation growth patterns and ecosystem productivity tracked with automated cameras from 2000 to 2013. Ambio 46, 39–52. doi:10.1007/s13280-016-0864-8
Wheeler, H. C., Høye, T. T., and Svenning, J.-C. (2018). Wildlife species benefitting from a greener Arctic are most sensitive to shrub cover at leading range edges. Glob. Change Biol. 24, 212–223. doi:10.1111/gcb.13837
Xu, L., Myneni, R. B., Chapin III, F. S., Callaghan, T. V., Pinzon, J. E., Tucker, C. J., et al. (2013). Temperature and vegetation seasonality diminishment over northern lands. Nat. Clim. Change 3, 581–586. doi:10.1038/nclimate1836
Yang, D., McMahon, A., Hantson, W., Anderson, J., and Serbin, S. P. (2023). PiCAM: a Raspberry Pi-based open-source, low-power camera system for monitoring plant phenology in Arctic environments. Methods Ecol. Evol. 14, 2974–2984. doi:10.1111/2041-210X.14231
Yu, L., Leng, G., and Python, A. (2021). Varying response of vegetation to sea ice dynamics over the Arctic. Sci. Total Environ. 799, 149378. doi:10.1016/j.scitotenv.2021.149378
Yu, Q., Epstein, H. E., Engstrom, R., Shiklomanov, N., and Strelestskiy, D. (2015). Land cover and land use changes in the oil and gas regions of Northwestern Siberia under changing climatic conditions. Environ. Res. Lett. 10, 124020. doi:10.1088/1748-9326/10/12/124020
Yu, Q., Epstein, H. E., Walker, D. A., Frost, G. V., and Forbes, B. C. (2011). Modeling dynamics of tundra plant communities on the Yamal Peninsula, Russia, in response to climate change and grazing pressure. Environ. Res. Lett. 6, 045505. doi:10.1088/1748-9326/6/4/045505
Zaika, Y. (2024). Omnia mea mecum porto*: science diplomacy realm of the Russian Arctic. Paradig. İktisadi ve İdari Araştırmalar Derg. 13, 1–10.
Zaika, Y., and Lagutina, M. (2023). Arctic science diplomacy in new geopolitical conditions: from “soft” power to “hard” dialogue? Polar Rec. 59, e23. doi:10.1017/S0032247423000141
Zeng, Y., Hao, D., Huete, A., Dechant, B., Berry, J., Chen, J. M., et al. (2022). Optical vegetation indices for monitoring terrestrial ecosystems globally. Nat. Rev. Earth and Environ. 3, 477–493. doi:10.1038/s43017-022-00298-5
Zhang, Q., Zhang, X., Lara, M. J., Li, Z., Xiao, J., Zhao, K., et al. (2023). Impacts of abiotic and biotic factors on tundra productivity near Utqiaġvik, Alaska. Environ. Res. Lett. 18, 094070. doi:10.1088/1748-9326/acf7d6
Zhou, L., Tucker, C. J., Kaufmann, R. K., Slayback, D., Shabanov, N. V., and Myneni, R. B. (2001). Variations in northern vegetation activity inferred from satellite data of vegetation index during 1981 to 1999. J. Geophys. Res. 106, 20069–20083. doi:10.1029/2000JD000115
Keywords: Arctic, tundra, normalized difference vegetation index, greening, remote sensing, climate change
Citation: Frost GV, Bhatt US, Macander MJ, Berner LT, Walker DA, Raynolds MK, Magnússon RÍ, Bartsch A, Bjerke JW, Epstein HE, Forbes BC, Goetz SJ, Hoy EE, Karlsen SR, Kumpula T, Lantz TC, Lara MJ, López-Blanco E, Montesano PM, Neigh CSR, Nitze I, Orndahl KM, Park T, Phoenix GK, Rocha AV, Rogers BM, Schaepman-Strub G, Tømmervik H, Verdonen M, Veremeeva A, Virkkala A-M and Waigl CF (2025) The changing face of the Arctic: four decades of greening and implications for tundra ecosystems. Front. Environ. Sci. 13:1525574. doi: 10.3389/fenvs.2025.1525574
Received: 09 November 2024; Accepted: 13 February 2025;
Published: 10 April 2025.
Edited by:
Mary E. Edwards, University of Southampton, United KingdomCopyright © 2025 Frost, Bhatt, Macander, Berner, Walker, Raynolds, Magnússon, Bartsch, Bjerke, Epstein, Forbes, Goetz, Hoy, Karlsen, Kumpula, Lantz, Lara, López-Blanco, Montesano, Neigh, Nitze, Orndahl, Park, Phoenix, Rocha, Rogers, Schaepman-Strub, Tømmervik, Verdonen, Veremeeva, Virkkala and Waigl. This is an open-access article distributed under the terms of the Creative Commons Attribution License (CC BY). The use, distribution or reproduction in other forums is permitted, provided the original author(s) and the copyright owner(s) are credited and that the original publication in this journal is cited, in accordance with accepted academic practice. No use, distribution or reproduction is permitted which does not comply with these terms.
*Correspondence: Gerald V. Frost, amZyb3N0QGFicmluYy5jb20=
Disclaimer: All claims expressed in this article are solely those of the authors and do not necessarily represent those of their affiliated organizations, or those of the publisher, the editors and the reviewers. Any product that may be evaluated in this article or claim that may be made by its manufacturer is not guaranteed or endorsed by the publisher.
Research integrity at Frontiers
Learn more about the work of our research integrity team to safeguard the quality of each article we publish.