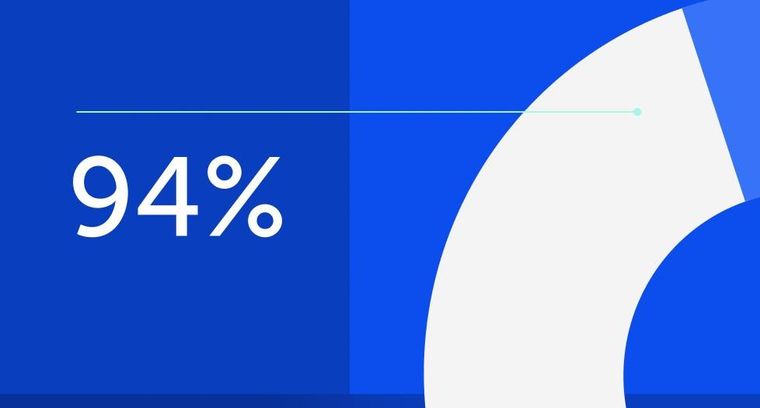
94% of researchers rate our articles as excellent or good
Learn more about the work of our research integrity team to safeguard the quality of each article we publish.
Find out more
ORIGINAL RESEARCH article
Front. Environ. Sci., 16 December 2024
Sec. Environmental Informatics and Remote Sensing
Volume 12 - 2024 | https://doi.org/10.3389/fenvs.2024.1484169
This article is part of the Research TopicRemote Sensing of the CryosphereView all 10 articles
Introduction: Dobšiná Ice Cave (Slovakia) has attracted the attention of many researchers since its discovery more than 150 years ago. Although the cave is located outside the high-mountain area, it hosts one of the largest volumes of underground perennial ice. The topographic mapping of this unique UNESCO Natural Heritage site has led to several historical surveys. In the last decades of rapid climate change, this natural formation has been subject to rapid changes that are dynamically affecting the shape of the ice body. Increased precipitation, the rise in year-round surface temperatures, and the gravity cause significant shape changes in the ice filling.
Methods: This paper describes modern technological tools to comprehensively survey and evaluate interannual changes in both the floor and wall of the underground ice body. Technologies such as digital photogrammetry, in combination with precise digital tacheometry and terrestrial laser scanning, make it possible to detect ice accumulation and loss, including the effect of sublimation due to airflow, as well as sliding movements of the ice body to the lower part of the cave. To get a comprehensive model of the ice volume, geophysical methods (microgravimetry and ground penetrating radar) have been added to determine the thickness of the floor ice in the upper parts of the cave in the last 2 years.
Results: Between 2018 and 2023, the ice volume in certain sections of the cave decreased by up to 667 m³, with notable reductions in ice thickness ranging from 0.3 to 0.9 m in areas like the Small Hall and Collapsed Dome. The study also detected dynamic changes, such as the widening of the ice tunnel by 20 cm in some sections, and a vertical ice wall in Ruffinyi’s Corridor showed localized volume losses up to 9 m3 (between 2018 and 2023). Additional geophysical methods - microgravimetry and ground penetrating radar - revealed an average ice thickness ranging from 10 to 25 m.
Discussion: The paper not only highlights the current technological possibilities but also points out the limitations of these technologies and then sets out solutions with a proposal of technological procedures for obtaining accurate geodetic and geophysical data.
The world of fragile underground ice caves offers its beauty in many countries worldwide. Their uniqueness attracts the attention of many researchers as well as the general public and they can be considered as one of the least well-known parts of the global cryosphere (Kern and Perșoiu, 2013; Perşoiu and Lauritzen, 2018). According to Ford and Williams (1989), ice caves can be defined as rock caves containing seasonal and/or perennial ice. Another definition by Perşoiu and Onac (2012) states that an ice cave is a cave formed in bedrock that contains perennial accumulations of water in its solid phase (i.e., ice and snow). Ice caves have been described in many parts of the world at altitudes where the mean annual air temperature is several degrees above 0°C (e.g., Harris, 1982; Holmlund et al., 2005). Depending on the cave morphology, this is due to the accumulation of cold air or unidirectional advection of cold air into the cave during winter (Luetscher and Jeannin, 2004). Cave ice is a special class of natural ice having various geneses, but it exists in a cavity and is located below the Earth’s surface. It is connected with the open air. Cave ice belongs to the category of subaerial - underground ice (Perşoiu and Lauritzen, 2018). The interplay of cave climate, i.e., the values, distribution, and dynamics of temperature (air and substrata), humidity, and air movement in caves, on a background of peculiar cave morphology, is responsible for the genesis, accumulation, and ice dynamics in caves. The composition and structure of cave ice provides the basis for their separation into an independent class of natural ice, intermediate between subaerial and underground (Perşoiu and Lauritzen, 2018).
Cave glaciation (perennial or temporary) is a widespread phenomenon on Earth. For example, approximately 10% of all karst cavities known in the former USSR have been subjected to cave glaciation (Perşoiu and Lauritzen, 2018). According to Mavlyudov (2018), it appears that the main areas where cave glaciation can exist are in North America and Eurasia. Due to its climate, Australia has the potential for only seasonal cave glaciation in its high mountain regions. In contrast, cave glaciation may occur in the mountains of New Zealand’s South Island. In Africa, most areas are not adapted to cave glaciation, with the exceptions being Mount Kilimanjaro and the Atlas Mountains. Cave glaciation in South America has been poorly studied, but there are suggestions of cave glaciation presence in the upper regions of the Andes Mountains. Also, there have been confirmations of ice-filled caves at high altitudes in South America. In addition to Europe being the most active region for ice caves research, many ice caves can be found in Canada (Yonge et al., 2018), Iran (Soleymani et al., 2018), Asia (Mavlyudov and Kadebskaya, 2018), Russia (Mavlyudov and Kadebskaya, 2018), and United States (Higham and Palmer, 2018).
The study of the underground cryosphere is, at present, considered to be extremely important and urgent because ice degradation processes are widely observed all over the world (Luetscher et al., 2005) and because little is known about the processes controlling the mass balance of perennial subsurface ice deposits and its evolution under changing climate conditions (Luetscher et al., 2008; Morard et al., 2010). However, cave ice serves as a local resource (for example, for tourism), its degradation may destabilize underground, and it preserves environmental information that has remained largely unexplored so far (Obleitner and Spötl, 2011). In caves, the volume and surface morphology of ice bodies result from the extent of glaciation and interannual or seasonal changes of perennial ice fill (Perşoiu and Lauritzen, 2018). Since seasonal changes cause changes in ice morphology, it is important to study not only the dynamics of spatial changes, such as the loss and gain of ice fill, but also changes in ice structure, such as changes in icing and the formation of sedimentary, metamorphosed, or sublimation ice.
Since the first discoveries of these unique natural beauties, researchers have noticed that the ice fill is subject to change over time (Șerban et al., 1948; Racoviță et al., 1987; Ohata et al., 1994; Racoviță, 1994; Fuhrmann, 2007; Tulis and Novotný, 2003; and others). Their research focuses primarily on the morphology and the dynamics of the ice body’s shape changes depending on the climatic conditions prevailing underground, but also on their age dating, genesis, and geographic location. Awareness of the world’s ice caves was relatively small until the 19th century and narrowly geographically confined to Central Europe and Russia. Ice caves in Jura and the Western Alps (now France and Switzerland) have been known for a long time, but Dobšiná Ice Cave, together with Demänovská Cave (Slovakia) and Pestera Scărisoara Cave (Romania), are among the first caves discovered in the 19th century in Central Europe outside the Alpine regions (Meyer, 2018). As stated by Meyer (2018), the history of ice cave exploration in the distant past (19th-20th century) was devoted to the unification of heterogeneous nomenclature, to the description of phenomena influencing the formation of ice in caves - depending on the airflow pattern, climatic conditions (defining the relationship between internal and external climatic conditions (Korzystka et al., 2011), microclimate, climate in front of the cave, as well as regional macroclimate), cave shapes. Many of these theories have been contradicted and disproved over time.
In the past, before electronic rangefinders become more widespread, ice level was typically measured as the distance from a fixed point (monitoring datum) on the cave wall or ceiling above the ice floor (marked by a screw or nail permanently inserted into the rock) to the surface of the ice floor (Smith, 2014). When multiple observations from a cave were available for a given year, the data offered the opportunity to model inter-annual variation in ice height. Modern geodetic (Gómez-Lende et al., 2014; Securo et al., 2022) and geophysical (Behm and Hausmann, 2008; Podsushin and Stepanov, 2008; Hausmann and Behm, 2011; Gómez-Lende et al., 2016; Securo et al., 2022) tools provide quality results for surveying ice-fillings dynamics. Behm and Hausmann (2008) examined several caves in the Northern Calcareous Alps of Austria, where ground-penetrating radar (GPR) was used to determine the thickness, volume, and internal structure of ice fillings, revealing that cave ice is heterogeneous. Hausmann and Behm (2011) examined the internal structure and formation of ice deposits in four caves in the Northern Calcareous Alps using GPR, revealing non-uniform ice with varying basal reflection signatures and banded internal reflections, likely caused by sediment layers, and suggesting temperate ice with a homogenous liquid water content of approximately 2%. Gómez-Lende et al. (2014) focused on the ice caves of the Picos de Europa (Spain), where terrestrial laser scanning (TLS) was used to monitor ice cave evolution and morphology, revealing dynamic temperature zones within the Peña Castil ice cave, distinct seasonal ice accumulation linked to snowmelt, and the identification of unique ice structures and cryospeleothems, such as radicular crystallization hoarfrost, observed for the first time in the region. In another study Gómez-Lende et al. (2016) used GPR to investigate the internal structure, stratification, and ice volume of the Peña Castil ice cave in northern Spain, revealing a minimum ice thickness of 54 m, potential vertical displacement within the ice, and banded reflections from interbedded clasts and sediment layers, demonstrating georadar’s effectiveness in providing insights into ice cave dynamics and internal features where direct visual inspection is challenging. Securo et al. (2022) explored the use of a terrestrial structure-from-motion multi-view stereo approach combined with GPR to monitor topographic changes and estimate ice mass balance in caves in the Julian Alps, offering a low-cost, efficient, and accurate method for long-term ice monitoring.
Digital tacheometry and, more recently, terrestrial laser scanners or digital photogrammetry tools allow data collection with high temporal and spatial resolution (Pukanská et al., 2023; Urban et al., 2024). These technologies have been highly proven in many research works (for instance, Hofierka et al., 2016; Pukanská et al., 2018; Šupinský et al., 2019; Pukanská et al., 2020; Bella et al., 2021). Pukanská et al. (2018) explored the modern surveying techniques for mapping underground morphological structures, emphasizing the use of TLS and digital close-range photogrammetry method of Structure-from-Motion (SfM), for accurate mapping of complex underground spaces. The study finds that while TLS provides the most accurate data, SfM offers comparable results with lower financial and time demands and better photo-texture quality, though it is limited by underground lighting conditions. In contrast, optical scanning produces less accurate results with more severe limitations, making TLS the most suitable technology for mapping, with SfM being viable under optimal lighting. Šupinský et al. (2019) used laser scanning technology to monitor the inter-seasonal dynamics of ice volume in the Silická ľadnica cave, revealing continuous changes with significant ice loss over time, and highlights the need for long-term observations to better understand the underlying mechanisms. In another work by Pukanská et al. (2020), authors mapped Ochtiná Aragonite Cave in Slovakia, known for its unique geological and mineralogical features, using TLS and digital photogrammetry, providing detailed 3D models and morphometric data that offer insights into the cave’s complex morphology and contribute to its environmental protection and tourism management. For the same cave, Bella et al. (2021), based on the same 3D data, revealed detailed depositional histories and slow sedimentation rates, with magnetostratigraphy and U-series dating tracing its development from the Early Pleistocene to the Late Pleistocene. Regarding modern surveying technologies, also mobile mapping systems can be utilized. However, they are more suitable for obtaining a general 3D structure of cave spaces and not for detailed ice surface mapping (Dušeková et al., 2024). The measurements have been used to detect bulk masses or to trace some ice masses but mainly to survey the geomorphological features of cave spaces - cartographic and topographic representation or the creation of geological maps (Petters et al., 2011; Milius and Petters, 2012; Berenguer-Sempere et al., 2014). Milius and Petters (2012) focused on the three-dimensional surveying and visualization of the Eisriesenwelt, one of the world’s largest ice caves, using 158 terrestrial laser scans and over 2,000 texture images, producing a realistic 3D model and virtual flythrough, with applications for scientific research, tourism, and cultural heritage documentation. On the other hand, Berenguer-Sempere et al. (2014) demonstrated the potential of combining TLS and thermographic techniques to create 3D thermographic models for monitoring climatological parameters in ice caves, providing a versatile and accurate method for studying ice evolution in challenging environments like the Peña Castil Ice Cave in Northern Spain.
Over time, as the technology and methodologies of topographic research have evolved, so have the knowledge and refinement of scientific results. From the early historical geodetic measurements with optical instruments and analog photogrammetry, we are now moving to technologies such as digital tacheometry and photogrammetry, TLS, or modern geophysical methods such as microgravimetry, GPR, and seismometry.
Given that Dobšiná Ice Cave is currently one of the most glaciated ice caves in the world (Perşoiu and Lauritzen, 2018; Hruby, 2022), it provides a wide range of different types of ice structures, with large areas of vertical (Ruffinyi’s Corridor, Great Curtain) and horizontal glaciation (Small and Great Hall), as well as areas with significant ice loss (Collapsed Dome), is significantly affected by climate change and precipitation activity, thus providing scope for the design of an accurate and comprehensive methodology for geodetic and geophysical measurement of morphological changes in different types of ice filling, taking into account current technological possibilities for spatial measurement, visualization, analysis and publication of data.
The Dobšiná Ice Cave (“Dobšinská ľadová jaskyňa” in Slovak) belongs to the most well-known ice cave in the world. It is located in the southern part of Slovenský raj (Slovak Paradise) National Park in the Spiš-Gemer Karst (north of the town of Dobšiná) (Figures 1A–C). Its entrance lies at 969 m a.s.l., on the right side of the Hnilec River valley, on the west slope of the Duča Plateau, 130 m above the valley bottom. The length of the cave is 1,483 m with a vertical span of 112 m. It is an inflow part of the larger genetic system of the Stratenská Cave, which is longer than 23.6 km. The whole system was formed by two underground streams - the Hnilec River and the Tiesňava Stream in the Late Pliocene (Tulis and Novotný, 1989; Novotný, 1993; Novotný and Tulis, 1996; 2005; Bella et al., 2014). The conditions for the formation of the ice filling were probably created in the Middle Pleistocene after the collapse of the ceilings (Duča sinkhole) and the breaking of the passage between Dobšinská Ice Cave and Stratenská Cave (Novotný and Tulis, 1996). At present, it is mostly filled with ice that reaches the ceiling in some places, and that divides the cavity into several parts: Small Hall, Great Hall, Collapsed Chamber, Ruffinyi’s Corridor, Ice tunnel, Ground Floor, and Hell. The ice filling of the cave is mainly made of floor and wall ice, ice stalagmites, and icefalls (Figures 1D, E). In 1995, a joint geodetic and geophysical survey of the ice volume and area was carried out, which revealed the ice volume was 110,132 m3, the surface of ice filling was 9,772 m2, the maximal thickness of floor ice reached 26.5 m, and the average thickness of floor ice was 13 m (Novotný and Tulis, 1996; see also Bella, 2006; 2018; Bella and Zelinka, 2018; Bella et al., 2020). The surface area (in the vicinity of the cave) is in moderately cool (mean annual air temperature 4, 7°C; mean air temperature in January −5,4°C, in July 14, 2°C) and very humid subregion (mean annual precipitation total 900–1,000 mm) (according to Lapin et al., 2002; Faško and Šťastný, 2002; Šťastný et al., 2002).
Figure 1. (A) Karst areas with a location of the most important ice caves in Slovakia (after Perşoiu and Lauritzen, 2018); (B) projection of the glaciated part of the cave on the surrounding digital terrain model (source of the DTM - LiDAR data: ÚGKK SR); (C) cave floorplan and cross sections with ice filling in the cave (after Tulis et al., 1999); (D) Great Hall and (E) ice tunnel in the cave.
Although the “ice hole” under the Duča had been known to shepherds and loggers for a long time, it was discovered only on 15 June 1870 by Eugene Ruffinyi and Co., (Lalkovič, 2000; Kudla, 2020). The cave was opened to the public on 8 March 1871. The first map of the Dobšiná Ice Cave was made by its discoverer Eugen Ruffínyi in 1871; later, new drawings were added. The first stereophotogrammetric surveys of the cave spaces were carried out in 1936. Another measurement was the research of spatial changes of the ice fill by geodetic and photogrammetric methods in the years 1976–1990, in which the Department of Mine Surveying and Geophysics of the Technical University of Košice (VŠT Košice) was also involved.
Dobšiná Ice Cave is a significant geomorphological and geological object of world importance. The dynamics of the evolution of the ice-filling of caves is a long-standing scientific problem (Perşoiu and Lauritzen, 2018). Since the optical properties of ice influence the interpretation of measured data, the choice of an appropriate methodology for geodetic and geophysical mapping of ground ice in the cave certainly does not have a clear answer. Therefore, the choice of surveying methodology must be considered in this context (Warren, 2019).
Research of changes in the ice surface due to the amount and composition of surface precipitation, the temperature of the internal environment, speed and direction of airflow, as well as anthropogenic factors (number of visitors, human interference), must be carried out by precise geodetic and geophysical measurements, which use detailed measurements of small surface changes and can also capture the dynamics of change over time. Geodetic methods, such as digital tacheometry, but also modern non-contact technologies (digital photogrammetry and laser scanning), as well as geophysical methods such as microgravimetry and GPR, provide an innovative way for the study of surface and volume changes of the underground ice. The systematic measurement of surface ice changes started in 2011, with the first step being the monumentation of the geodetic point field and its survey as a closed traverse, followed by its adjustment and connection to the national spatial network by GNSS measurements. Since 2011, the survey methodology has been gradually complemented by newly available technologies (Figure 2).
The acquisition of spatial data on the ice surface of horizontal and vertical parts of the ice filling and the subsequent analysis of its changes is fundamentally affected by the fact that an electromagnetic radiation (EMR) can penetrate through a variable structure of the ice. This has influenced the way in which the survey was conducted and the choice of appropriate technologies. Using our established methodology, we propose methodological procedures that eliminate the influence of factors negatively affecting the results of measurement and spatial model building (Figure 2).
When EMR passes from one environment to another, there is absorption, refraction, reflection, and transmission as the speed of propagation of EMR changes, as well as the humidity and temperature, in the different environments. The refractive index of water as a liquid is 1.333. In the solid state, it can be determined to be around 1.309. Materials with a higher refractive index are more sensitive to temperature change. The interaction of electromagnetic radiation with ice and media containing ice, as well as snow, is described by the refractive index and absorption coefficient, which are functions of the wavelength. Volume reflectivity, absorption, and transmittance are further influenced by the grain size of snow, ice, and bubbles (Warren, 2019). As stated by Persoiu and Lauritzen (2018), ice caves have different forms of glaciation: congelation, sedimentary, and sublimation ice. Congelation ice occurs as icings, ice in rocks, and sometimes as lake ice. Sedimentary and sublimation ice is more often seasonal. Pits are fine collectors of solid precipitation; therefore, the prevailing types of ice in them are sedimentary and metamorphic ice. Cave ice can originate from snow and firn (recrystallized snow, metamorphic ice), freezing of stagnant, flowing, or dripping meteoric waters (congelation ice), freezing of moisture when warmer, humid air contacts cold surfaces (ice sublimation crystals), freezing of moist sediments (ice in clastic sediments), and from frozen supercooled water emerging from microfissures on cave bedrock and others.
As a crystalline form of water, ice in a cave has a variable structure that depends on the surface and ambient temperature and the admixture of overlaying rock material. In Dobšiná Ice Cave, we can observe various structural forms of ice (Figure 3):
a) smooth, clear ice,
b) ice containing dust or impurities of overlaying rock,
c) as well as various forms of frost on the walls,
d) large ice crystals in the ice mass, which have a coarse, granular texture.
Figure 3. Various types of ice surfaces in the cave, from smooth and clear ice (A), ice with impurities (B), forms of frost (C), to large ice crystals (D).
It can be clearly stated that the ice surface is variable depending on the position of the ice mass relative to the surface (floor ice and wall ice) as well as the ambient temperature.
Figure 4 shows the penetration of the laser beam (TLS and digital tacheometry) through the ice structure, which can reach up to 0.5 m into the ice structure. Methods such as laser scanning and digital tacheometry with passive reflection (without surveying prism) are not suitable for very accurate measurements of ice surface topography, much less for detecting changes in ice-fill dynamics. Nevertheless, their use is in mapping the irregular shape of a cave’s rock surface and other geodetic activities.
Figure 4. Penetration of the laser beam (A) Leica ScanStation C10, (B) Leica RTC360, and (C, D) by Leica total station into the ice structure.
The geodetic survey started in 2011 on the initiative of the Slovak Caves Administration, and the measurements were carried out in several stages. The planimetric and altimetric measurements are carried out in the Slovak national coordinate system of the Uniform Trigonometric Cadastral Network (S-JTSK) and vertical datum Baltic Vertical Datum - After Adjustment (Bpv) from fixed points of the surveying net monumented in the ceiling parts of the cave. The basic prerequisite for accurately evaluating temporal and spatial changes in the ice fill of the cave is an underground geodetic control with the required density and accuracy.
The connection of the surface points of the Dobšinská Ice Cave network to the National Spatial Network was carried out through the Slovak Real-Time Positioning Service (SKPOS), using signals from Global Navigation Satellite Systems (GNSS) by a static method for 3 h, whereby the points of the orientation line 5,001–5,002, approximately 1,047 m apart, were determined. Underground points 5004 to 5012, monumented in the solid, unweathered parts of the cave rock ceiling by surveyor’s nails, as well as points monumented by retro-reflective targets, were determined in 2011 from the orientation line. Since 2018, the point field has been complemented in several stages (Figure 5-top), taking advantage of the drilled mine monumentation with reflective targets, with new survey marks monumented in the side walls and cave ceiling.
Figure 5. Top - geodetic point field in the cave; down (A)-(H) - various types of monumentation of the geodetic point field and photogrammetric points and their marking.
The point monumentation and marking were realized by the following methods (Figures 5A-H-down):
a) surveying nail in the cave ceilings,
b) drilled nail in the cave ceilings/wall,
c) surveying prism with reflective foil,
d) Leica retro-reflective target (2 cm × 2 cm) on an invar strip glued to the cave wall,
e) disc anchor with metal screw with leica retro-reflective target (2 cm × 2 cm),
f) disc anchor with metal screw with 12-bit coded target (2 cm × 2 cm × 3 mm) for photogrammetry,
g) A4 paper 12-bit coded target for photogrammetry,
h) 12-bit coded target (2 cm × 2 cm × 3 mm) glued to the cave wall for photogrammetry.
The geodetic network as a whole after the 2011 adjustment in the 2D cartographic plane can be characterized by a mean position error of 4.9 mm and a mean coordinate error of 3.5 mm. The mean error of the adjusted heights had a value of 1.7 mm for the height adjustment of the points. The standard error ellipses of the local geodetic network are represented by an estimate of the standard deviation of measured lengths of 1.4 mm and directions of 1.49 mgon (4.8″) for the Leica Viva TS15 motorized total station.
In order to provide original knowledge about speleoclimatic and glaciological patterns in Dobšiná Ice Cave, their seasonal and trend changes, the first two stages of detailed spatial surveying of its upper part, formed by the Small and Great halls, were carried out. A detailed 3D model of the cave entrance and its underground spaces is being built on the basis of tacheometry, TLS, and digital photogrammetry and in synergy with the existing climate monitoring system of the cave managed by the Slovak Caves Administration in Liptovský Mikuláš. In the future, it will serve as a basis for detailed scientific analyses of the state and development of the glaciation of the cave system in the environment of geographic information systems. The first measurements of the ice fill were made in 2011. However, regular and accurate measurements have been carried out from 2018 to the present. Measurements are done semi-annually, i.e., before (spring) and after (fall) the tourist season, to record the winter and summer evolution on the cave. Detailed spatial measurements of the Small Hall and the Great Hall in the cave are carried out using two total stations Leica Viva TS15 and TS50 in May and October. The accuracy of tacheometric measurements is defined by the mean positional error mp ≤ 2 mm and the mean error derived for trigonometrical measurement of heights mh ≤ 1.5 mm. The positional and vertical connection was realized to the original and complemented survey points of the underground geodetic point field in the binding national coordinate system and vertical datum. Complete inter-annual and inter-semi-annual (seasonal) measurements of the change in floor ice height will be analyzed in a separate paper.
The survey of the ice cave was realized by TLS in October 2018 (Leica ScanStation C10) and November 2023 (Leica RTC 360). Leica ScanStation C10 has a laser beam in visible green EMR with a wavelength of 532 nm. The accuracy of a single measurement in position is 6 mm, in the distance 4 mm, and angle accuracy (horizontal/vertical) is 60 µrad/60 µrad (12″/12″). The precision of the modeled surface is 2 mm. Leica RTC 360 has a laser beam in infrared EMR with a wavelength of 1,550 nm. The angular accuracy is 18″, and the distance accuracy is 1.0 mm + 10 ppm. The 3D accuracy of points is 5.3 mm for a 40 m distance.
For ScanStation C10, the spatial resolution of 30 × 30 mm of the final point cloud was achieved, with the overall spatial error of scan registration of 6 mm. Data from scanning were processed and edited in Leica Cyclone 7.3 software with export to the point cloud in LAS format.
For Leica RTC360 scanner, the spatial resolution of 3 × 3 mm for a 10 m distance was achieved, with the overall cloud-to-cloud registration error of 3 mm and georeferencing error of 4 mm. Data were processed in Cyclone Register 360 software with export to the point cloud in LAS format. Leica RTC360 was used in 2023 for the first time in some parts of the cave, to find out if a scanner with different wavelength can provide data with less noise for the ice surface.
For data from ScanStation C10, we used a triangular mesh generation method by the Poisson Surface Reconstruction algorithm (Bolitho et al., 2007) to create the 3D MESH model, where surfaces are represented as a polygonal mesh. Since the EMR penetrates the ice surface, the laser scanner survey from Leica ScanStation C10 was only used to model the overall shape of the cave itself, not to model changes in the ice surface accurately.
Due to the specific characteristics and shapes of the ice surface, or their location and accessibility, some cave parts have been mapped and monitored semi-annually by digital close-range photogrammetry method of SfM since 2018. These parts are as follows (see Figure 6):
a) vertical ice wall in Ruffinyi’s Corridor (digital tacheometry and TLS are not possible due to the size of the vertical surface, large height differences, and limited space for movement),
b) an artificial tunnel through the ice massif (digital tacheometry and TLS are not possible due to the specific ice surface in the tunnel characterized by a significant penetration of the laser beam under the ice surface),
c) a site in the part of the Great Curtain below the ice massif at the interface between the ice and the bedrock (TLS is not possible due to the site and the limited space; however, total station measurements are used to track the position of the observation points accurately).
Figure 6. Locations of the photogrammetric survey: (A) Ruffinyi’s Corridor; (B) ice tunnel; (C) under the Great Curtain.
The SfM photogrammetric method is used at all three sites. It is currently one of the most advanced photogrammetric processing techniques. It was originally based on computer vision and visual perception but has been gradually adapted to photogrammetry to derive three-dimensional structure from two-dimensional images of objects. The principle of SfM is to estimate the 3D structure of a spatial object from two-dimensional image sequences originating from a moving recording medium. The advantages of SfM are the simultaneous image matching, bundle adjustment, and reconstruction of a 3D structure on images. The principle itself, the sequence of individual processing steps, and the corresponding algorithms are generally well-known and frequently used (Seitz et al., 2006; Westoby et al., 2012; Remondino et al., 2014; Pavelka et al., 2018; Štroner et al., 2020; and others).
From the principle of SfM and image matching, it follows that the success of this method strongly depends on the structure and texture of the imaged surface. In the case of:
• flat and uniform texture,
• surfaces with high reflectivity,
• transparent surfaces,
The image matching may fail and result in too noisy data or no data at all. In ice caves, the reflectivity and transparency of the ice surface can make the SfM unusable. This is also the case with the artificial tunnel in Dobšinská Ice Cave (Figures 6, 10A). In order to be able to suppress reflections and refractions of light from the ice surface and thus use the SfM photogrammetry, we proposed to apply a cross-polarized photogrammetry (Wells et al., 2005; Edwards, 2011; Conen et al., 2018; Marčiš et al., 2016). This encompasses a polarized light source (stronger than other sources or the only source of illumination in the cave), in our case, a polarizing sheet attached directly to a DSLR camera flash, and a second polarizing filter attached to the DSLR camera lens. A prerequisite for cross-polarisation is that the polarizer on the lens must be rotated at 90° with respect to the polarizer on the flash. For more details about the cross-polarization method, see Bartoš et al. (2023).
We used a digital camera, a DSLR Pentax K-5, with the lens Pentax SMC DA 15 mm f/4 ED AL Limited, for all three parts surveyed by photogrammetry. All images are taken using a tripod, 12-s self-timer, ISO 200, aperture f/10 - f/13, and RAW format with subsequent conversion to 12-bit TIFF. Acquired images are processed in Agisoft Metashape® Professional Edition, Version 1.6.0 software (Agisoft LLC, St. Petersburg, Russia, 2019) by standard photogrammetric processing (Bartoš et al., 2023). Sample parameters of imaging and image processing for the three surveyed locations are given in Table 1.
Table 1. Parameters of imaging and image processing for all locations of the photogrammetric survey (after Bartoš et al., 2023).
Methods of applied geophysics are based on precise measurements of physical fields, which are caused or influenced by structures with anomalous physical properties in the underground. These methods are used to detect and identify various subsurface objects–from shallow to larger depths (meter to kilometer scales). Application areas come from a large interval: from geological and tectonic studies and exploration of mineral deposits to very shallow applications in the detection of archaeological objects (near surface geophysics). Based on the kind of the studied physical field, applied geophysics is divided into various methods: gravity methods (gravimetry), magnetic methods (magnetometry), geoelectrical and electromagnetic methods, seismic and seismological methods, radiometric methods, etc. (Telford et al., 1990; Milsom and Eriksen, 2011; Reynolds, 2011). Determination of the thickness of ice layers represents a quite well-posed problem formulation for several methods in near-surface geophysics because there exists a quite large contrast in selected physical properties between the ice and its rocky basement (for instance, density, electrical permittivity, and conductivity, velocity of mechanical waves). Based on the general knowledge from applied geophysics, a combination of several geophysical methods is often necessary–because various methods can support or debar outputs of them (each of the method is reacting to different physical properties of subsurface structures). From a great variety of near-surface geophysical methods, we have selected in this study the microgravity method (very precise gravimetry) and GPR, which is one of the most effective electromagnetic methods in near-surface geophysical applications.
Gravimetry, or its specific detailed version - microgravimetry, is a geophysical method to measure and interpret very small changes in gravity acceleration. In microgravimetry, gravity changes (anomalies) that are caused by shallow subsurface objects with a significant density contrast to the surroundings are particularly interesting, for instance, crypts in archaeology (Pašteka et al., 2020), mines (Bishop et al., 1997) and caves (Butler, 1984). Due to its low density compared to the surrounding rocks, the ice filling of caves can also be a subject of interest for gravimetry. In the case of ice filling, we can basically talk about two possibilities for using gravimetry. The first method is mapping the thickness of the ice fill based on the complete Bouguer anomaly (CBA) calculation and subsequent density modeling. This methodology requires the necessary corrections for the topography, as well as corrections for the cave spaces themselves.
In the calculation of CBA, state-of-the-art procedures were used with the use of Toposk software (Zahorec et al., 2017), detailed DEM5.0 based on aerial laser scanning (https://www.geoportal.sk/sk/zbgis/lls-dmr/, source: ÚGKK SR) and a spatial model of the cave derived from TLS and tachymetry. The calculated values of CBA along the profile were tentatively compared with the approximate calculation of the gravitational effect of the ice fill derived from the existing GPR measurements (Figure 13). A differential density of −1,750 kg/m3 was used for the ice, as the difference between the actual density of approx. 920 kg/m3 and the CBA correction density of 2,670 kg/m3. The two curves are very similar, which indicates that gravimetry is potentially suitable for determining the total ice volume in the cave. This will require complex areal gravity mapping and subsequent 3D density modeling in the future.
The second methodology is repeated monitoring of changes in gravity acceleration due to volume changes of the ice filling. For this purpose, test gravity measurements along the V-shaped profile were carried out at the site (Figure 7C) in 2020 and 2022. A Scintrex CG-5 gravity meter was used for the measurements. A stable base point (on rocky ground) was located inside the cave, on which measurements were taken to check the drift of the gravity meter. The reference point was located outside the cave. Measuring on ice is not easy, as the ice is easily deformed under the measuring tripod due to the weight of the gravimeter, which makes measurement very problematic. That is why we also used a special steel pad for the measurement, which better distributes the pressure on the surface of the ice (Figure 7B). Stabilization of the steel pad on the sloping surface of the ice filling is also problematic. The estimated error of the gravity measurement from the control measurements is at the level of approx. 5 µGal (5.10–8 m/s2). Measured gravity changes between 2020 and 2022 reach one order higher values (up to approximately 50 µGal), so we can discuss a reliable measurement.
Figure 7. Example from GPR (A) and gravity (B) measurements along a selected line in the Dobšiná Ice Cave (C) (used instrument: MALA GroundExplorer GX with 160 MHz antenna (A) and Scintrex CG-5 gravity meter (B)).
Electromagnetic emission (EM) can penetrate through lucent materials and environments–also through ice medium. In the case of the GPR method (familiarly georadar), electromagnetic emission is created by a transmitting antenna of the instrument to study the subsurface’s structure (Figure 7A). In the case of the GPR method, electromagnetic emission usually has frequencies that are outside the interval of visible light–these usually have a value of hundreds of MHz (typical values are from 100 to 500 MHz). Transmitted pulses of EM emission are reflected from subsurface objects, and after their return back to the surface, these are registered by a receiver antenna of the instrument. Registered reflections of EM emissions are then processed by means of special methods due to the wave character of the acquired data, and vertical time-sections (so-called radargrams) are usually the first outputs for geological or geotechnical interpretation (for instance, Milsom and Eriksen, 2011) (Figure 14A). It is also important to mention that the recorded and displayed time in these sections is the so-called two-way-time (TWT) because this is the time during the EM pulse has traveled from the emitting source to the reflecting object and then back to the registration in the instrument. In the process of recalculating the TWT values, these must then be halved. Precise positions of the surface measurements are usually obtained through terrestrial geodetic methods and/or using GNSS technology. The local position of the instrument along measuring lines is determined by an odometer wheel.
In the case of the acquired GPR data processing, usually the following steps are conducted: zero-time removal, 1D trace filtering (“dewow”), 2D filtering in spectral domain: background-removal and low-pass filtering with Butterworth filter. Important step is also the introduction of topographical corrections and eventually also migration (with topographical information). During the processing and interpretation of acquired GPR data, a very important parameter is the velocity of EM radiation in the subsurface medium–this value is influenced by the electromagnetic physical properties of soils, rocks, and ice. There exist several methods for velocities estimation, and one of them is widely used: fitting the shape of a selected diffraction wave of hyperbolic shape in the vertical time section. Typical values of the velocities for various types of ice (from freshwater ice to permafrost environments) vary in quite large intervals from 0.15 to 0.3 m/ns (for instance, Reynolds, 2011). In the case of the ice in caves, it can be expected that these values will be closer to the lower limit of this interval. For example, we can mention typical values for the ice-sheet margin in East Antarctica, where the average value equals 0.18 m/ns (Guo et al., 2022). After using estimated velocity values to the TWT data, typical depths achieved by the GPR method are first meters; in the case of lower electrical permittivity, these can reach depths of 10–20 m (but this result depends strongly upon the used frequency of the GPR antenna). The GPR method was applied before with great success for the estimation of the thickness of ice in the case of continental or mountain glaciers (Singh et al., 2012; Navarro et al., 2018; Bello et al., 2020; Guo et al., 2022 and many others) and there exist several successful worldwide GPR applications from ice caves (Colluci et al., 2014; Colluci et al., 2016; Gómez-Lende et al., 2016; Securo et al., 2022).
The ice fill is constantly changing its shape. Both external (precipitation, temperature) and internal factors (airflow velocity and direction, internal temperature, air pressure, humidity in the cave) influence the height of the floor ice in the cave during the year. The contour plots of both surfaces were made by processing the measured tacheometric data at two epochs in May 2018 and May 2023 (Figure 8).
Figure 8. Height and extent of glaciation in three parts of the cave surveyed by a digital tacheometry - Small Hall, Great Hall, and Collapsed Dome; (A) overall situation of the glaciated area in May 2023; (B) cross-sections through the glaciated part showing differences over 5 years.
TLS survey allows us to obtain a complete general point cloud and, thus, a 3D model of the glaciated part of the Dobšiná Ice Cave. Due to the penetration of the laser beam into the ice (Figure 4), not all parts of the TLS point cloud are suitable for detailed analysis. However, these data can be used for visualization and presentation to show a general overview of the cave. Processing the point clouds from both TLS and SLAM produced the resulting visualization shown in Figure 9A. The point cloud (Figure 9B) can also be viewed as freely available data on the web at http://ugkagis.fberg.tuke.sk/potree/dobsinska_ladova_jaskyna/portal.html.
Figure 9. (A) a general overview of the final 3D MESH model; (B) detail of the point cloud from laser scanning; (C) a longitudinal cross-section through the cave (source of the terrain - LiDAR data: ÚGKK SR).
A longitudinal cross-section through the trail of the guided tour in the cave and corresponding surface (classified point cloud from aerial laser scanning; ÚGKK SR) is shown in Figure 9C.
The results of digital photogrammetry confirmed the usability of the SfM method for 3D reconstruction of the ice-filling surfaces in the selected parts of the cave with sufficient accuracy. By using cross-polarized photogrammetry (Bartoš et al., 2023), we are able to suppress light reflections from the ice successfully (Figure 10). The main results of the SfM processing are dense point clouds, MESH models, and for the Ruffinyi’s Corridor also an orthophotomosaic.
Figure 10. Results of the photogrammetric survey of the ice tunnel and Ruffinyi’s Corridor; (A) image without cross-polarisation, (B) cross-polarized image; (C) resulting 3D digital model of the tunnel; (D) dense point cloud of the Ruffinyi’s Corridor in 2018.
The following Figures 10A, B shows the effect of cross-polarization (DSLR camera with a mounted flash and polarizing filter on the lens and the flash rotated at 90° with respect to each other) on the reflections caused by artificial illumination on the ice surface. Figure 10C shows the final textured 3D MESH model generated by the SfM photogrammetry from cross-polarized images, and Figure 10D shows the final dense point cloud of Ruffinyi’s Corridor. The MESH model and dense point cloud are both compact, smooth, and without significant noise or holes. The only part with lower quality is the railing of the guided tour trail in the tunnel, but this railing is not necessary to determine the tunnel’s spatial changes.
By comparing these results from individual epochs, we are able to determine changes in the ice filling over time. These results give us information about the direction and speed of the ice filling dynamics and also about changes in the volume of ice filling in these parts. Given the complexity of the issue and the amount of data, we provide a partial analysis of results for the 2018–2023 epochs only for the first two photogrammetric sites. To analyze the differences between the two epochs, we used a difference model (Figure 11) created in the open-source software CloudCompare 2.13 (GPL software, 2024) using the Multiscale Model to Model Cloud Comparison “M3C2 distance” plugin (Lague et al., 2013). This tool can be used to determine robust signed distances directly between two point clouds.
Figure 11. (A) top view on the difference model of the tunnel between epochs 2018–2023; (B) front view on the difference model of the corridor between epochs 2018–2023.
Figure 11A illustrates a difference model - i.e., spatial changes, for the ice tunnel between epochs 2018 and 2023. According to the difference model, we can say that the tunnel gradually but irregularly widens, with more significant changes in the southeast (S-E) direction. In the S-E wall, the ice surface has shifted in the range of 20 cm; while in the opposite N-W wall, the ice surface has shifted only by 5–10 cm. The total volume of the tunnel has changed by + 5 m3.
Figure 11B illustrates a difference model for the vertical ice wall in Ruffinyi’s Corridor between epochs 2018 and 2023. According to the difference model, we can say that in some parts of the wall, the ice surface is slowly retreating (green to blue color), and in some parts, the ice surface is increasing (yellow to red), in the range of 0–10 cm. The blank parts of the difference model represent parts with changes larger than 10 cm, so the color scale for the difference model and corresponding distances are easy to distinguish. The highest increase is recorded in the icefall (vertical blank part, caused by water slowly leaking from the upper parts) - more than 10 cm. The total change in the volume between 2018 and 2023 was recorded as an ice loss of 9 m3.
However, if we look at the geodetic point monumented as a disc anchor with a metal screw into the vertical wall (Figures 5, 12) in 2019, there are two apparent movements. The first is the retreat of the ice surface from 5 cm (from the SfM data–Figure 12A) to 13 cm (a visible difference by the folding meter–Figure 12B) (this displacements/inconsistency of values can also be partially caused by pushing the screw out of the ice by the ice solid itself, but there is no clear evidence of that). The second is a spatial displacement of the point by 2–3 cm per 1 year (Figure 12C). Therefore, there can be a combination of ice retreat, movement of the ice body, extrusion of the screw from the ice and its drop and tilt due to its own weight. The spatial change of this point (and the ice wall) is almost in the same direction as in the case of the ice tunnel.
Figure 12. Spatial changes of the disc anchor with metal screw monumented in the vertical ice wall between 2019–2023; (A) differences from the SfM point cloud–side view; (B) photo of the anchor in 2023 with a folding meter as a scale; (C) movement of the anchor from the total station measurement–top view; (D) general direction of the changes.
The results of repeated test gravity measurements between the years 2020 and 2022 (Figure 13) confirmed that given volume changes of the ice filling, which manifest themselves in height changes at the level of at least a few cm (or more), are reliably detectable by gravimetric methods despite the difficult measurement conditions. Gravimetry can thus be used to monitor dynamic changes in the level of the ice fill.
Figure 13. (A) Grav-1: Correlation of measured height and gravity differences between the years 2020 and 2022 at test profile points. For a better comparison, the gravity differences (in red) are shown with the opposite sign. The blue dashed line shows zero differences. (B) Grav-2: Approximate comparison of the CBA (correction density 2,670 kg/m3) and the gravitational effect of the ice fill (differential density −1,750 kg/m3) along the test gravimetric profile.
Tests of the GPR method during the years 2020–2022 in Dobšinská Ice Cave brought very interesting and promising results. Measurements have been performed by means of a MALA GroundExplorer GX instrument (with 160 MHz antenna) along the selected line in the cave. Basic processing steps have been performed with a focus on selecting the proper gain function (aiming to amplify reflection amplitudes from deeper positioned objects). As it can be seen in the processed vertical radargram from this site (Figure 14A), the ice filling is characterized by a relatively homogeneous wave pattern–besides a relatively large amount of isolated diffraction waves (with the typical hyperbolic shape), coming with great probability from isolated stones and pieces of rocks in the ice. The average velocity, estimated from these diffraction waves (Figure 14B), was set to 0.16 m/ns. In the selected vertical radargram (Figure 14A), accepting the estimated velocity of 0.16 m/ns, the depth of the base of ice varies from 10 m to approx. 25 m below the ice surface (corresponding TWT values were 125 and 320 ns). The boundary between the ice and its basement is plotted with a thick red line in Figure 14A. From repeated measurements on identical lines (in opposite directions) we could estimate an average error in the determination of the depth of the base of ice on a level of ±30 cm.
Figure 14. (A) Selected vertical radargram from the Dobšiná Ice Cave (left-hand vertical axis: two-way-time, right-hand axis: depth for the velocity 0.16 m/ns, the thick red line represents the boundary between the ice and the basement). (B) The selected part of a vertical radargram with plotted diffraction waves and estimated values of the EM wave velocity (0.16 in this case).
Different surveys of the cryosphere, and especially of ice caves, have been part of their documentation for many years. There are measurements of ice extent, ice surface characteristics, the volume of ice filling in caves, spatio-temporal dynamics of the ice bodies, and others. Analyses of the dynamics of the ice in caves have been presented in several papers (Strug and Zelinka, 2008; Perşoiu and Pazdur, 2011; Gómez-Lende and Sánchez-Fernández, 2018; Perşoiu et al., 2021). Also, the ice measurement itself has been widely reviewed and presented (Gindraux et al., 2017; Alfredsen et al., 2018; Pfeiffer et al., 2022; Hruby, 2022). Morard et al. (2010) recorded rapid changes in the Diablotins ice cave in Switzerland by monitoring the cave climate and suggested an hourly or daily monitoring interval based on a laser scanner or automatic cameras. The dynamics of ice bodies and their margins can also be monitored by data from a time-lapse camera and SfM photogrammetric processing (Mallalieu et al., 2017) or by UAS images and photogrammetric processing (Li et al., 2019). Over time, various technologies have been implemented and have been used with more or less success.
In this research, data from TLS proved to be a good and quick technique to get a general spatial overview of the cave and the extent of the glaciated part with sufficient accuracy (Figures 1, 8, 9). However, determining changes in the ice volume by TLS can be problematic due to the properties of the laser beam incident on the ice surface. For this purpose, it is more appropriate to use digital tacheometry, which can provide a spatial position of individual points on the ice surface with high accuracy and, therefore, create a planimetric and altimetric map of the ice surface for individual epochs. The comparison of tacheometric data from years 2018 and 2023 revealed a significant loss of ice area, especially in the Small Hall and Collapsed Dome parts (Figure 8). The vertical loss of the ice level over the 5 years amounts to −0.3 to −0.9 m. By comparing the amount of glaciation over this five-year period, it can be clearly stated that the ice volume has increased by 99.5 m3 in some places but decreased by up to 667 m3 in others. In 2018, the area covered by ice was 2,986 m2. Detailed inter-annual and inter-semi-annual (seasonal) measurements of the change in floor ice height will be analyzed in a separate paper.
However, the TLS results show that using a laser scanner with different wavelengths, for instance, Leica RTC360 with infrared 1,550 nm, can provide more accurate results with less penetration into the ice (Figures 4A, B).
On the other hand, digital tacheometry is suitable for use only in parts with horizontal (floor) ice, where it is possible to measure the spatial position of points using a common surveying prism. For specific parts in terms of their geometry, spatial orientation, and texture/structure of the ice, digital close-range photogrammetry would be the best option. In Dobšiná Ice Cave, we identified several specific glaciated parts suitable for the photogrammetric measurements. Due to the extensiveness of the research, we presented results for two of them. However, digital photogrammetry (the SfM method) has proven to be very suitable for parts that are hard to survey using traditional surveying techniques (and/or TLS). Moreover, we applied a new photogrammetric imaging technique using a cross-polarized illumination to properly image and model highly reflective ice surfaces (see also Bartoš et al., 2023).
In terms of geophysical methods, both of them proved to be suitable and, what is more important, complementary to TLS, tacheometry, and photogrammetry since they can provide data which are not obtainable by geodetic methods (mainly the depth of the ice body and its structure under the surface).
This means, from the point of view of speleologists, it is also important to map the total volume of ice in the cave, which is, however, a methodologically more complex and demanding task. At this stage of the study of the Dobšiná Ice Cave, we are able, on the basis of the measurements so far, to at least tentatively assess the possibility of determining the total thickness of the ice filling based on the interpretation of the CBA.
In terms of GPR, it has also been used in ice surveys for a long time. Dallimore and Davis (1992) used GPR to detect massive ground ice and map near-surface geology. GPR was also used in combination with SfM photogrammetry to describe the topography and ice thickness and determine the volume changes of ice volumes in ice cave environments (for instance, Securo et al., 2022). The usability of GPR technology to study permafrost rocks and the emergence of cryogenic processes was evaluated by Sokolov et al. (2020).
In Dobšiná Ice Cave, from repeated measurements along identical lines (acquired in both directions), the accuracy of the ice thickness could be estimated as the approximate value ± 0.2 m. This error is relatively high when compared with other geodetic methods, but the transition between ice and basement is not a clear boundary but a zone where the basement rocks are broken by the influence of ice. For this reason, the repetition of measurements along each line is important. This kind of interpretation (estimation of ice thickness or the depth of ice basement) can be performed along a system of parallel and crossing lines, and an area map of these values can be plotted–contributing to a 3D model of ice cover in selected parts of the cave.
This research demonstrates that various geodetic (digital tacheometry, laser scanning, digital photogrammetry) and geophysical methods (microgravimetry, GPR) are necessary for a comprehensive study of ice filling dynamics in an ice cave, especially in terms of long-time monitoring. Ice bodies in caves can have different characteristics for individual parts of the cave, with changes in the structure and/or texture, even in a small area. Therefore, combining these methods can give us a comprehensive view of ice filling dynamics and a suitable tool for long-term monitoring. However, we see a potential for using modern laser scanners that are available to filter reflected laser beams according to the number of the returned signal. Using such, it is possible to measure these surfaces in a comparable density and accuracy as with digital photogrammetry.
In this study, we reviewed the current methodologies for surveying the ice filling and the thickness of the underground ice, and the dynamics of its evolution in the Dobšiná Ice Cave. We evaluated the suitability of using different geodetic and geophysical methods and technologies depending on the expected results. Due to the physical properties of the laser beam and its penetration/reflection from the ice surface, as well as limiting factors in photogrammetric imaging, the use of different methodologies should be considered.
We proposed the use of a digital tacheometric method to measure 3D data on the floor ice surfaces and a photogrammetric SfM method using cross-polarized light to measure the volumetric changes of ice in specific areas of the cave. The tacheometric data showed significant ice volume changes between 2018 and 2023, with vertical decrease of ice surface ranging from 0.3 to 0.9 m in areas of the Small Hall and Collapsed Dome. The total ice volume of the floor ice decreased by up to 667 m³ in some areas, while increasing by 99.5 m³ in others. The combination of surveying technologies also allowed us to detect dynamic changes in ice structures, such as a widening of the ice tunnel by 20 cm in the southeast direction and a volume loss of 9 m³ in the vertical ice wall of Ruffinyi’s Corridor.
We found the GPR, geophysical method, and partial microgravimetry to be clearly successful in measuring the thickness of the ice. GPR measurements revealed that the ice thickness varies from 10 to 25 m, with an estimated error of ±0.2 m, providing critical data about the subsurface ice structure.
All of the technologies utilized have their accuracy limitations depending on the instruments, methods of measurement, and characteristics of the environment. However, the presented methodology can combine all of their advantages with comparable accuracy to give a general view of the behavior of this underground ice body. Nonetheless, modern ice cave research has not reached its zenith, and there is still room for improvement - depending on geodetic and geophysical measurement technology development. Since the underground ice body is characterized by its dynamic inter-annual changes, dependent on climatic developments in both the exterior and interior of the cave, as well as anthropogenic factors, there is a constant need for regular monitoring in order to preserve this unique natural phenomenon as much as possible. This research shows the high applicability and suitability of the combination of these methods and technologies to monitor spatio-temporal changes in ice caves over a long period.
The raw data supporting the conclusions of this article will be made available by the authors, without undue reservation.
KP: Conceptualization, Formal Analysis, Funding acquisition, Investigation, Methodology, Writing–original draft, Writing–review and editing. KB: Formal Analysis, Investigation, Methodology, Visualization, Writing–original draft, Writing–review and editing. JG: Conceptualization, Investigation, Methodology, Resources, Supervision, Validation, Writing–original draft. RP: Formal Analysis, Investigation, Visualization, Writing–original draft. PZ: Formal Analysis, Investigation, Visualization, Writing–original draft. JP: Formal Analysis, Investigation, Writing–original draft. PB: Conceptualization, Data curation, Investigation, Supervision, Validation, Writing–review and editing. EA: Investigation, Visualization, Writing–original draft. LD: Formal Analysis, Investigation, Resources, Visualization, Writing–original draft. DB: Software, Supervision, Writing–review and editing. ĽK: Data curation, Investigation, Writing–review and editing.
The author(s) declare that financial support was received for the research, authorship, and/or publication of this article. The study is the result of the Grant Project of the Ministry of Education of the Slovak Republic KEGA No. 003TUKE-4/2023 and VEGA No.1/0340/22 and 1/0679/25.
We would like to thank everyone who contributed to the fieldwork, mostly Ľubomír Očkaik, the manager of the Dobšiná Ice Cave. Many thanks to the reviewers and the editor for their useful comments and suggestions.
The authors declare that the research was conducted in the absence of any commercial or financial relationships that could be construed as a potential conflict of interest.
All claims expressed in this article are solely those of the authors and do not necessarily represent those of their affiliated organizations, or those of the publisher, the editors and the reviewers. Any product that may be evaluated in this article, or claim that may be made by its manufacturer, is not guaranteed or endorsed by the publisher.
The Supplementary Material for this article can be found online at: https://www.frontiersin.org/articles/10.3389/fenvs.2024.1484169/full#supplementary-material
Alfredsen, K., Haas, C., Tuhtan, J. A., and Zinke, P. (2018). Brief communication: mapping river ice using drones and structure from Motion. Cryosphere 12 (2), 627–633. doi:10.5194/tc-12-627-2018
Bartoš, K., Pukanská, K., Kseňak, Ľ., Gašinec, J., and Bella, P. (2023). Cross-polarized SFM photogrammetry for the spatial reconstruction of challenging surfaces, the case study of Dobšiná Ice Cave (Slovakia). Remote Sens. 15 (18), 4481. doi:10.3390/rs15184481
Behm, M., and Hausmann, H. (2008). “Determination of ice thicknesses in alpine caves using georadar,” in Proceedings of the 3rd International Workshop on Ice Caves, Kungur, Russia, May, 2008, 12–17.
Bella, P. (2006). Morphology of ice surface in the Dobšiná ice cave. Proc. 2nd Int. workshop Ice caves, Demänová dolina, 15–23.
Bella, P., Bosák, P., Pruner, P., Hercman, H., Pukanská, K., Bartoš, K., et al. (2021). Speleogenesis in a lens of metamorphosed limestone and ankerite: ochtiná Aragonite Cave, Slovakia. Int. J. Speleology 51 (1), 13–28. doi:10.5038/1827-806x.51.1.2397
Bella, P., Braucher, R., Holec, J., and Veselský, M. (2014). Datovanie pochovania alochtónnych fluviálnych sedimentov v hornej časti Dobšinskej ľadovej jaskyne (IV. vývojová úroveň systému Stratenskej jaskyne) pomocou kozmogénnych nuklidov Cosmogenic nuclide dating of the burial of allochthonous fluvial sediments in the upper part of the Dobšinská Ice Cave (IVth evolution level of the Stratenská cave system), Slovakia. Slov. Kras. 52 (2), 101–110. (in Slovak with English abstract).
Bella, P., Tulis, J., Zelinka, J., Papáč, V., Višňovská, Z., and Haviarová, D. (2020). Dobšiná Ice Cave (Slovakia, Central Europe) and its unique underground glacier originated in the mid-mountain position of the moderate climate zone. Aragonit 25 (1), 4–16.
Bella, P., and Zelinka, J. (2018). Ice caves in Slovakia. Ice Caves. Amsterdam, Netherlands: Elsevier, 657–689.
Bello, C., Santillan, N., Cochachin, A., Arias, S., and Suarez, W. (2020). “Ice thickness using ground penetrating radar at Znosko glacier on King George island,” in Proceedings from 2020 IEEE Latin American GRSS and ISPRS Remote Sensing Conference, 437–439.
Berenguer-Sempere, F., Gómez-Lende, M., Serrano, E., and de Sanjosé-Blasco, J. J. (2014). Orthothermographies and 3D modeling as potential tools in ice caves studies: The Peña Castil Ice Cave (Picos de Europa, Northern Spain). Int. J. Speleology 43 (1), 35–43. doi:10.5038/1827-806x.43.1.4
Bishop, I., Styles, P., Emsley, S. J., and Ferguson, N. S. (1997). “The detection of cavities using the microgravity technique: case histories from mining and karstic environments,” in Modern geophysics in engineering geology (London: Geological Society Engineering Group, Special Publication No. 12, Geological Society), 155–168.
Bolitho, M., Kazhdan, M., Burns, R., and Hoppe, H. (2007). “Multilevel streaming for out-of-core surface reconstruction,” in Proceedings of the 5th Eurographics Symposium on Geometry Processing, Barcelona, Spain, 4–6 July, 2007, 69–78.
Butler, D. K. (1984). Microgravimetric and gravity gradient techniques for detection of subsurface cavities. Geophysics 49 (7), 1084–1096. doi:10.1190/1.1441723
Colucci, R. R., Fontana, D., Forte, E., Potleca, M., and Guglielmin, M. (2016). Response of ice caves to weather extremes in the southeastern Alps, Europe. Geomorphology 261, 1–11. doi:10.1016/j.geomorph.2016.02.017
Colucci, R. R., Forte, E., and Fontana, D. (2014). “Characterization of two permanent ice cave deposits in the Southeastern Alps (Italy) by means of ground penetrating radar (Gpr),” in VI International Workshop on Ice Caves, Turin, Italy, September, 2014 (IWIC. Digital Commons @ USF Tampa Library, US), 33–39.
Conen, N., Hastedt, H., Kahmen, O., and Luhmann, T. (2018). Improving image matching by reducing surface reflections using polarising filter techniques. Int. Archives Photogrammetry, Remote Sens. Spatial Inf. Sci. XLII-2, 267–274. doi:10.5194/isprs-archives-xlii-2-267-2018
Dallimore, S. R., and Davis, J. (1992). in Ground penetrating radar investigations of massive ground ice. Editor J. A. Pilon (Canada: Geological Survey of Canada), 241. Paper no. 90-4.
Dušeková, L., Herich, P., Pukanská, K., Bartoš, K., Kseňak, Ľ., Šveda, J., et al. (2024). Comparison of non-contact measurement technologies applied on the underground glacier—the choice for long-term monitoring of ice changes in Dobšiná ice cave. Remote Sens. 16 (20), 3870. doi:10.3390/rs16203870
Edwards, N. (2011). Cross-polarisation, making it practical. J. Vis. Commun. Med. 34, 165–172. doi:10.3109/17453054.2011.635291
Faško, P., and Šťastný, P. (2002). “Mean annual precipitation totals,” in Landscape Atlas of the Slovak republic (Bratislava, Banská Bystrica: Ministry of Environment of the Slovak Republic, Slovak Environmental Agency), 99. map No. 54.
Fuhrmann, K. (2007). Monitoring the disappearance of a perennial ice deposit in Merrill Cave. J. Caves Karst Stud. 69, 256–265.
Gindraux, S., Boesch, R., and Farinotti, D. (2017). Accuracy assessment of digital surface models from unmanned aerial vehicles' imagery on glaciers. Remote Sens. 9 (2), 186. doi:10.3390/rs9020186
Gómez-Lende, M., Berenguer, F., and Serrano, E. (2014). Morphology, ice types and thermal regime in a high mountain ice cave. First studies applying terrestrial laser scanner in the Peña Castil ice cave (Picos de Europa, northern Spain). Geogr. Fis. Din. Quat. 37, 141–150. doi:10.4461/GFDQ.2014.37.13
Gómez-Lende, M., and Sánchez-Fernández, M. (2018). Cryomorphological topographies in the study of ice caves. Geosciences 8 (8), 274. doi:10.3390/geosciences8080274
Gómez-Lende, M., Serrano, E., Bordehore, L. J., and Sandoval, S. (2016). The role of GPR techniques in determining ice cave properties: Peña Castil ice cave, Picos de Europa. Earth Surf. Process. Landforms 41, 2177–2190. doi:10.1002/esp.3976
GPL software (2024). CloudCompare (version 2.13). Available at: http://www.cloudcompare.org/. Accessed on May 03, 2024.
Guo, J., Li, L., Liu, J., Fu, L., Tang, X., Wang, Y., et al. (2022). Ground-penetrating radar survey of subsurface features at the margin of ice sheet, East Antarctica. J. Appl. Geophys. 206, 104816. doi:10.1016/j.jappgeo.2022.104816
Harris, S. (1982). “Identification of permafrost zones using selected permafrost landforms,” in Proceedings, 4th Canadian conference on permafrost. Calgary, alberta, March 1981. Editor H. M. French (Ottawa, Ontario: National Research Council of Canada), 49–58.
Hausmann, H., and Behm, M. (2011). Imaging the structure of cave ice by ground-penetrating radar. Cryosphere 5 (2), 329–340. doi:10.5194/tc-5-329-2011
Higham, S. R., and Palmer, A. N. (2018). “Ice caves in the USA,” in Ice caves. Editors A. Perșoiu, and S.-E. Lauritzen (Elsevier), 705–716.
Hofierka, J., Hochmuth, Z., Kaňuk, J., Gallay, M., and Gessert, A. (2016). Mapovanie jaskyne Domica pomocou terestrického laserového skenovania. Geogr. časopis (68/1), 25–38.
Holmlund, P., Onac, B. P., Hansson, M., Holmgren, K., Mörth, M., Nyman, M., et al. (2005). Assessing the palaeoclimate potential of cave glaciers: the example of the Scǎrişoara Ice Cave (Romania). Geogr. Ann. Ser. A, Phys. Geogr. 87 (1), 193–201. doi:10.1111/j.0435-3676.2005.00252.x
Hruby, D. (2022). For an otherworldly experience, venture into Europe's Ice Caves, nationalgeographic.com. Available at: https://www.nationalgeographic.com/travel/article/for-an-otherworldly-experience-venture-into-europes-ice-caves (Accessed March 14, 2024).
Kern, Z., and Perşoiu, A. (2013). Cave ice - the imminent loss of untapped mid-latitude cryospheric palaeoenvironmental archives. Quat. Sci. Rev. 67, 1–7. doi:10.1016/j.quascirev.2013.01.008
Korzystka, M., Piasecki, J., Sawiński, T., and Zelinka, J. (2011). “Climatic system of the Dobšinská ice cave,” in 6th Congress of the International Show Caves Association, Demänovská Valley (Slovakia: SNC of Slovak Republic, Slovak Caves Administration), 85–97.
Lague, D., Brodu, N., and Leroux, J. (2013). Accurate 3D comparison of complex topography with terrestrial laser scanner: application to the rangitikei canyon (N-Z). ISPRS J. Photogrammetry Remote Sens. 82, 10–26. doi:10.1016/j.isprsjprs.2013.04.009
Lapin, M., Faško, P., Melo, M., Šťastný, P., and Tomain, J. (2002). “Climatic regions,” in Landscape Atlas of the Slovak republic (Bratislava, Banská Bystrica: Ministry of Environment of the Slovak Republic, Slovak Environmental Agency), 95.
Li, T., Zhang, B., Cheng, X., Westoby, M., Li, Z., Ma, C., et al. (2019). Resolving fine-scale surface features on Polar Sea Ice: a first assessment of UAS photogrammetry without ground control. Remote Sens. 11 (7), 784. doi:10.3390/rs11070784
Luetscher, M., and Jeannin, P. (2004). A process based classification of alpine ice caves. Theor. Appl. Karstol. 17, 5–10.
Luetscher, M., Jeannin, P. Y., and Haeberli, W. (2005). Ice caves as an indicator of winter climate evolution: a case study from the Jura Mountains. Holocene 15 (7), 982–993. doi:10.1191/0959683605hl872ra
Luetscher, M., Lismonde, B., and Jeannin, P. Y. (2008). Heat exchanges in the heterothermic zone of a karst system: monlesi cave, Swiss Jura Mountains. J. Geophys. Res. 113 (F02025), 1–13. doi:10.1029/2007jf000892
Mallalieu, J., Carrivick, J. L., Quincey, D. J., Smith, M. W., and James, W. H. M. (2017). An integrated structure-from-motion and time-lapse technique for quantifying ice-margin dynamics. J. Glaciol. 63 (242), 937–949. doi:10.1017/jog.2017.48
Marčiš, M., Barták, P., Valaška, D., Fraštia, M., and Trhan, O. (2016). Use of image based modelling for documentation of intricately shaped objects. ISPRS - Int. Archives Photogrammetry, Remote Sens. Spatial Inf. Sci. XLI-B5, 327–334. doi:10.5194/isprsarchives-xli-b5-327-2016
Mavlyudov, B. R. (2018). “Geography of cave glaciation,” in Ice caves. Editors A. Perșoiu, and S.-E. Lauritzen (Elsevier), 217.
Mavlyudov, B. R., and Kadebskaya, O. I. (2018). “Ice caves in Russia,” in Ice caves. Editors A. Perșoiu, and S.-E. Lauritzen (Elsevier), 530–606.
Milius, J., and Petters, C. (2012). Eisriesenwelt—from laser scanning to photo-realistic 3D model of the biggest ice cave onEarth. Washington, DC, USA: GI-Forum, 513–523.
Morard, S., Bochud, M., and Delaloye, R. (2010). Rapid changes of the ice mass configuration in the dynamic diablotins ice cave – fribourg prealps, Switzerland. Cryosphere 4 (4), 489–500. doi:10.5194/tc-4-489-2010
Navarro, F. J., Martín-Español, A., Lapazaran, J. J., Grabiec, M., Otero, J., Vasilenko, E. V., et al. (2018). Ice volume estimates from ground-penetrating radar surveys, wedel jarlsberg land glaciers, svalbard. Svalbard. Artic, Antartic, Alp. Res. 46 (2), 394–406. doi:10.1657/1938-4246-46.2.394
Novotný, L. (1993). Treťohorné jaskynné úrovne a zarovnané povrchy v Slovenskom raji [Tertiary cave levels and planation surfaces in the Slovak Paradise]. Slov. Kras. 31, 55–59. (in Slovak with English abstract).
Novotný, L., and Tulis, J. (1996). Výsledky najnovších výskumov v Dobšinskej ľadovej jaskyni [Results of the newest researches in the Dobšiná Ice Cave]. Slov. Kras. 34, 139–147.
Novotný, L., and Tulis, J. (2005). Kras slovenského raja [the karst of Slovak Paradise]. Správa slovenských jaskýň, slovenská speleologická spoločnosť, Liptovský mikuláš; knižné centrum. Žilina, 175. (in Slovak with English summary).
Obleitner, F., and Spötl, C. (2011). The mass and energy balance of ice within the Eisriesenwelt cave, Austria. Cryosphere 5 (1), 245–257. doi:10.5194/tc-5-245-2011
Ohata, T., Furukawa, T., and Osada, K. (1994). Glacioclimatological study of perennial ice in the Fuji ice cave, Japan. Part 2: interannual variation and relation to climate. Arct. Alp. Res. 26, 238–244. doi:10.2307/1551936
Pašteka, R., Pánisová, J., Zahorec, P., Papčo, J., Mrlina, J., Fraštia, M., et al. (2020). Microgravity method in archaeological prospection: methodical comments on selected case studies from crypt and tomb detection. Archaeol. Prospect. 27, 415–431. doi:10.1002/arp.1787
Pavelka, K., Šedina, J., and Matoušková, E. (2018). High resolution drone surveying of the Pista geoglyph in Palpa, Peru. Geosciences 8 (12), 479. doi:10.3390/geosciences8120479
Perşoiu, A., Buzjak, N., Onaca, A., Pennos, C., Sotiriadis, Y., Ionita, M., et al. (2021). Record summer rains in 2019 led to massive loss of surface and cave ice in SE Europe. Cryosphere 15 (5), 2383–2399. doi:10.5194/tc-15-2383-2021
Perşoiu, A., and Onac, B. P. (2012). “Ice in caves,” in Encyclopedia of caves. Editors W. B. White, and D. C. Culler second ed. (Elsevier), 399–404.
Perşoiu, A., and Pazdur, A. (2011). Ice genesis and its long-term mass balance and dynamics in Scărişoara Ice Cave, Romania. Cryosphere 5 (1), 45–53. doi:10.5194/tc-5-45-2011
Petters, C., Milius, J., and Buchroithner, M. E. (2011). “Terrestrial laser scanning and 3D visualisation of the largest ice cave on earth,” in Proceedings of the European LiDAR Mapping Forum, Salzburg, Austria, November, 2011, 29–30.
Pfeiffer, J., Rutzinger, M., and Spötl, C. (2022). Terrestrial Laser scanning for 3D mapping of an alpine ice cave. Photogrammetric Rec. 38, 6–21. doi:10.1111/phor.12437
Podsuhin, N., and Stepanov, Y. (2008). “Measuring of the thickness of perennial ice in Kungur ice cave by georadar,” in Proceedings of the 3rd international workshop on ice caves, kungur, Russia, 12–17 may 2008. Editors O. Kadebskaya, B. R. Mavlyudov, and M. Pyatunin, 52–55.
Pukanská, K., Bartoš, K., Bakoň, M., Papčo, J., Kubica, L., Barlák, J., et al. (2023). Multi-sensor and multi-temporal approach in monitoring of deformation zone with permanent monitoring solution and management of environmental changes: a case study of Solotvyno salt mine, Ukraine. Front. Earth Sci. 11. doi:10.3389/feart.2023.1167672
Pukanská, K., Bartoš, K., Bella, P., Gašinec, J., Blistan, P., and Kovanič, Ľ. (2020). Surveying and high-resolution topography of the Ochtiná aragonite cave based on TLS and digital photogrammetry. Appl. Sci. 10 (13), 4633. doi:10.3390/app10134633
Pukanská, K., Bartoš, K., Bella, P., Rákay, S., and Sabová, J. (2018). Comparison of non-contact surveying technologies for modelling underground morphological structures. Acta Montan. Slovaca 22 (3), 246–256.
Racoviță, G. (1994). Eléments fondamentaux dans la dynamique des spéléothèmes de glace de la grotte de Scărișoara, en relation avec la météorologie externe. Theor. Appl. Karstol. 7, 133–148.
Racoviță, G., Șerban, M., and Viehmann, I. (1987). Tendances de long terme dans la dynamique des formations de glace de la Grotte de Scărișoara (Monts du Bihor). Theor. Appl. Karstol. 3, 143–164.
Remondino, F., Spera, M. G., Nocerino, E., Menna, F., and Nex, F. (2014). State of the art in high density image matching. Photogrammetric Rec. 29, 144–166. doi:10.1111/phor.12063
Reynolds, J. M. (2011). An introduction to applied and environmental geophysics. 2nd edition. Wiley.
Securo, A., Forte, E., Martinucci, D., Pillon, S., and Colucci, R. R. (2022). Long-term mass-balance monitoring and evolution of ice in caves through structure from motion–multi-view stereo and ground-penetrating radar techniques. Prog. Phys. Geogr. Earth Environ. 46 (3), 422–440. doi:10.1177/03091333211065123
Seitz, S. M., Curless, B., Diebel, J., Scharstein, D., and Szeliski, R. (2006). “A comparison and evaluation of multi-view stereo reconstruction algorithms,” in 2006 IEEE Computer Society Conference on Computer Vision and Pattern Recognition - Volume 1 (CVPR'06).
Singh, S. K., Rathore, B. P., Bahuguna, I. M., and Ramnathan, A. L. (2012). Estimation of glacier ice thickness using Ground Penetrating Radar in the Himalayan region. Curr. Sci. 103 (1), 68–73.
Smith, K. J. (2014). “Ice cave monitoring at lava beds national monument,” in Proceedings of the sixth international workshop on ice caves. Editors L. Land, Z. Kern, V. Maggi, and S. Turri (Idaho Falls, Idako, USA: National Cave and Karst Research Institute), 88–93.
Sokolov, K., Fedorova, L., and Fedorov, M. (2020). Prospecting and evaluation of underground massive ice by ground-penetrating radar. Geosciences 10 (7), 274. doi:10.3390/geosciences10070274
Soleymani, M., Ford, D., Nakhaei, M., and Nadimi, A. (2018). “Ice caves in Iran,” in Ice caves. Editors A. Perșoiu, and S.-E. Lauritzen (Elsevier), 425–436.
Šťastný, P., Nieplová, E., and Melo, M. (2002). Mean annual air temperature. Mean january air temperature. Mean july air temperature. In landscape Atlas of the Slovak republic. Ministry of environment of the Slovak republic, bratislava. Banská Bystrica: Slovak Environmental Agency, 98–99.
Štroner, M., Urban, R., Reindl, T., Seidl, J., and Brouček, J. (2020). Evaluation of the georeferencing accuracy of a photogrammetric model using a quadrocopter with onboard GNSS RTK. Sensors 20 (8), 2318. doi:10.3390/s20082318
Strug, K., and Zelinka, J. (2008). The Demämovska Ice cave - the volume balance of the ice monolith in 2003-2007 (Slovakia). Slov. Kras. 46, 369–386.
Šupinský, J., Kaňuk, J., Hochmuth, Z., and Gallay, M. (2019). Detecting dynamics of cave floor ice with selective cloud-to-cloud approach. Cryosphere 13 (11), 2835–2851. doi:10.5194/tc-13-2835-2019
Șerban, M., Coman, D., and Givulescu, R. (1948). Découvertes récentes et observations sur la glacière naturelle dite, Gheţarul de la Scărișoara. Bull. Soc. Sci. Cluj. X, 174–210.
Telford, W. M., Geldart, L. P., and Sheriff, R. E. (1990). Applied geophysics. Cambridge University Press.
Tulis, J., and Novotný, L. (1989). Jaskynný systém Stratenskej jaskyne the cave system of Stratenská jaskyňa Cave. Osveta, Martin., 464. (in Slovak with Russian and English summary).
Tulis, J., and Novotný, L. (2003). Changes of glaciation in the Dobšinská ice cave. Aragonit 8, 7–9.
Tulis, J., Novotný, L., and Bella, P. (1999). Dobšiná ice cave – nomination for inscription on the world heritage list. Manuscript, ECS Slovakia ltd. Spišská Nová Ves – Slovak Caves Adm. Liptovský Mikuláš – Ministry Environ. Slovak Repub., 41.
Urban, R., Štroner, M., Braun, J., Suk, T., Kovanic, ľ., and Blišťan, P. (2024). Determination of accuracy and usability of a SLAM scanner GeoSLAM zeb horizon: a bridge structure case study. Appl. Sciences-Basel 14 (12), 5258. doi:10.3390/app14125258
Warren, S. G. (2019). Optical properties of ice and snow. Philosophical Trans. R. Soc. A Math. Phys. Eng. Sci. 377 (2146), 20180161. doi:10.1098/rsta.2018.0161
Wells, J., Jones, T., and Danehy, P. (2005). “Polarization and color filtering applied to enhance photogrammetric measurements of reflective surfaces,” in 46th AIAA/ASME/ASCE/AHS/ASC Structures, Structural Dynamics and Materials Conference.
Westoby, M. J., Brasington, J., Glasser, N. F., Hambrey, M. J., and Reynolds, J. M. (2012). 'structure-from-motion' photogrammetry: a low-cost, effective tool for Geoscience Applications. Geomorphology 179, 300–314. doi:10.1016/j.geomorph.2012.08.021
Yonge, C. J., Ford, D., Horne, G., Lauriol, B., and Schroeder, J. (2018). “Ice caves in Canada,” in Ice caves. Editors A. Perșoiu, and S.-E. Lauritzen (Elsevier), 285–334.
Zahorec, P., Marušiak, I., Mikuška, J., Pašteka, R., and Papčo, J. (2017). “Numerical calculation of terrain correction within the bouguer anomaly evaluation (program Toposk),” in Understanding the bouguer anomaly: a gravimetry puzzle. Editors R. Pašteka, J. Mikuška, and B. Meurers (Elsevier), chapter 5, 79–92. doi:10.1016/b978-0-12-812913-5.00004-x
Keywords: ice cave, cryomorphological topography, tacheometry, photogrammetry, laser scanning, SLAM, microgravimetry, ground penetrating radar
Citation: Pukanská K, Bartoš K, Gašinec J, Pašteka R, Zahorec P, Papčo J, Bella P, Andrássy E, Dušeková L, Bobíková D and Kseňak Ľ (2024) Methodological approaches to survey complex ice cave environments - the case of Dobšiná (Slovakia). Front. Environ. Sci. 12:1484169. doi: 10.3389/fenvs.2024.1484169
Received: 22 August 2024; Accepted: 27 November 2024;
Published: 16 December 2024.
Edited by:
Biagio Di Mauro, National Research Council (CNR), ItalyReviewed by:
Jason Polk, Western Kentucky University, United StatesCopyright © 2024 Pukanská, Bartoš, Gašinec, Pašteka, Zahorec, Papčo, Bella, Andrássy, Dušeková, Bobíková and Kseňak. This is an open-access article distributed under the terms of the Creative Commons Attribution License (CC BY). The use, distribution or reproduction in other forums is permitted, provided the original author(s) and the copyright owner(s) are credited and that the original publication in this journal is cited, in accordance with accepted academic practice. No use, distribution or reproduction is permitted which does not comply with these terms.
*Correspondence: Karol Bartoš, a2Fyb2wuYmFydG9zQHR1a2Uuc2s=
Disclaimer: All claims expressed in this article are solely those of the authors and do not necessarily represent those of their affiliated organizations, or those of the publisher, the editors and the reviewers. Any product that may be evaluated in this article or claim that may be made by its manufacturer is not guaranteed or endorsed by the publisher.
Research integrity at Frontiers
Learn more about the work of our research integrity team to safeguard the quality of each article we publish.