- 1School of Public Administration, Hebei University of Economics and Business, Shijiazhuang, China
- 2Hebei Collaborative Innovation Center for Urban-rural Integrated Development, Shijiazhuang, China
The comprehensive effects of nanoparticles and coexisting heavy metals on plant growth are still unclear, especially in soil medium. The single and combined effects of multiwall carbon nanotubes (MWCNTs) and cadmium (Cd) on rice (Oryza sativa L.) growth were examined in this study through a 4 months pot experiment in 2022. Rice plants were exposed to different concentrations of MWCNTs (100 and 500 mg kg−1) in the presence of 5.0 mg kg−1 Cd stress. At the tillering stage, the 500 mg kg−1 MWCNTs addition reduced plant height by 8.0% and increased soluble protein content in the leaves by 13.7%, demonstrating that a single MWCNTs had a slight negative impact on rice growth. When exposed to Cd stress, the inclusion of 500 mg kg−1 MWCNTs led to a 6.7%–9.0% decrease in bioavailable Cd level in soil, resulting in considerable reductions in Cd content in roots (23.4%–29.9%), shoots (24.5%–28.3%) and grains (28.3%–66.2%). Compared to the single Cd treatment, the O. sativa L. leaves treated with Cd and MWCNTs (500 mg kg−1) had considerably reduced levels of malondialdehyde (MDA), soluble protein, and activities of antioxidant enzymes (POD, CAT, and SOD). The findings of this study indicated that appropriate concentrations of MWCNTs application in soil could alleviate Cd-induced toxicity on rice growth.
1 Introduction
Nanomaterial products, such as nano-fertilizer and nano-pesticide, were widely used in agriculture to improve crop productivity and nutrient use efficiency (Lee et al., 2018; Rong et al., 2018; Hochella et al., 2019). Nanomaterials may enter the farmland soil during their usage and disposal (Hu et al., 2021; Priester et al., 2012; Peng et al., 2017). Carbon nanomaterials such as graphene, fullerene, and carbon nanotubes, offer diverse potential applications in agriculture and environmental pollution remediation. Carbon nanotubes (CNTs) are a typical carbon-based nanomaterial, have been widely used in the field of pollution remediation because of their large surface area, excellent electrical conductivity and the ability to strengthen the mass transfer process of pollutants. There are differences in structure, specific surface area and electrical conductivity between single-walled carbon nanotubes (SWCNTs) and multi-walled carbon nanotubes (MWCNTs). Due to the high specific surface area and active surface, MWCNTs perform well in adsorptive and catalytic degradation of organic pollutants, which is more suitable for environmental remediation. However, the potential toxicities and environmental behavior of MWCNTs in soil medium are not well understood (Cao et al., 2022).
The biotoxicity of MWCNTs on terrestrial plants has attracted great attention (Jordan et al., 2020; Wan et al., 2020; Zhai et al., 2015). Previous research has found both adverse and beneficial effects of MWCNTs on plants (Babbitt and Moore, 2018; Ruotolo et al., 2018; Cao et al., 2020; Pandey et al., 2018). In a hydroponic experiment, for example, Tiwari et al. (2014) indicated that the treatment of 60 mg L−1 MWCNTs (diameter of 6–9 nm) increased the biomass of corn. Similarly, Zhao et al. (2022) discovered that applying 25 and 50 mg kg-1 MWCNTs led to increase in the fresh weight of the alfalfa shoot. However, Hatami (2017) found that MWCNTs (diameter 5–15 nm, length 50 μm) addition (125, 250, 500, 1,000 mg L−1) to standard agar Murashige and Skoog medium had harmful impacts on the Cucurbita. pepo L. germination process and seedling growth. According to Sainao et al. (2020), the application of two distinct diameters (<8 nm and 20–30 nm) MWCNTs (1,000 mg L−1) reduced growth levels in rice seedlings, accompanied by decrease in the soluble protein contents and activities of peroxidase (POD) and superoxide dismutase (SOD) in roots, as well as the amount of chlorophyll in leaves. The effects of MWCNTs on plant growth is dependent on various factors, including the concentration, diameter, and length of MWCNTs, as well as the growth medium and species of plant (Lahiani et al., 2013; Shen et al., 2018).
MWCNTs can influence the migration, toxicity, and bioavailability of other contaminants (heavy metals, organic pollutants, pesticides, antibiotics) (Zhao et al., 2017). According to recent research, MWCNTs may be used in environmental pollutants remediation (Zhou et al., 2017; Zhang et al., 2016; Gong et al., 2019). Long et al. (2022) revealed that the application of MWCNTs (100, 500, 1,000 mg kg−1) decreased the acid-extractable Cd level in soil and immobilized Cd in contaminated soil. Song et al. (2017) reported that MWCNTs (15 g kg−1) exhibited enhanced adsorption capabilities for Cd(II) and phenanthrene, leading to a notable decrease in their concentrations in the overlying water. Shen et al. (2018) observed that MWCNTs (1, 10, 100, 1,000 mg L−1) reduced pyrene absorption in cucumber roots, and the level of inhibition decreased as the outer diameters of MWCNTs increased. The application of MWCNTs (1%, w/w) greatly enhanced the adsorption of herbicides to soil (Yao et al., 2021). However, MWCNTs substantially increased pyrene accumulation in alfalfa roots (Zhao et al., 2022). Cao et al. (2022) found that MWCNTs (0.075 wt%) markedly increased the levels of antimony (Sb) in rice roots and shoots. Furthermore, earlier research has verified that MWCNTs have a distinct impact on the phytotoxicity of coexisting pollutants on plants. Applying MWCNTs at concentrations of 0.5% and 1% notably decreased the adverse effects of quinclorac on tomatoes and enhanced plant heights and fresh weights (Zhao et al., 2022). This was confirmed by Yao et al. (2021); MWCNTs alleviated the phytotoxicity of quinclorac in soil and promoted the growth of tobacco. It has been found that MWCNTs (50, 100, 250, 500, 1,000 mg L−1) may increase the toxicity of heavy metals in plants (Sharifi et al., 2021). For instance, Wang et al. (2014) discovered that the combination of MWCNT-COOH (2.5, 5, 10 mg L−1) with Pb and Cd aggravated damage in bean seedling leaves. Furthermore, the co-exposure of MWCNTs and pyrene did not result in an observation of any notable phytotoxicity (Shen et al., 2018). MWCNTs aggravated the toxicity of coexisting pollutants in a concentration-dependent fashion (Zhao et al., 2022). In ramie seedlings, Gong et al. (2019) discovered that MWCNTs (500 mg kg-1) considerably reduced Cd-induced toxicity, but MWCNTs (5,000 mg kg-1) aggravated this toxicity. Currently, the interaction of MWCNTs with coexisting pollutants is poorly known, particularly in soil medium.
Cadmium (Cd), a prevalent pollutant in paddy fields, has garnered considerable interest owing to its exceptional mobility and biotoxicity in soil-rice systems (Zhang et al., 2019). Air pollution, sewage irrigation and fertilizer application can cause Cd pollution in farmland soil. Cd pollution could inhibit plant water metabolism, photosynthesis and respiration, etc. In addition, Cd could also affect the soil ecosystem and the availability of nutrient elements, thus affecting plant growth (Yang et al., 2022). The environmental behavior of MWCNTs with coexisted Cd in soil is poorly known. In this study, a 4 months pot experiment was conducted to evaluate the single and combined effects of MWCNTs and Cd on rice growth in soil medium. This study can provide reference data for understanding the effectiveness and environmental risk of MWCNTs application in farmland soil remediation.
2 Materials and methods
2.1 Characteristics of soil and MWCNTs
The experimental soil was collected from a paddy field located in Huizhou, Guangdong, South China (114°49′, 23°01′), with a depth ranging from 0 to 30 cm. After air-drying, soil samples were sieved (2-mm mesh). Table 1 shows the characteristics of the paddy soil.
MWCNTs with lengths varying from 0.5 to 2.0 μm and diameters between 10 and 20 nm (purity: 95 wt%, ash content <2.5 wt%) were bought from Nanjing XFNANO Materials Tech Co., Ltd. The inner diameter is 5–10 nm, tap density is 0.22 g cm-3, specific surface area >200 m2 g-1, electrical conductivity >100 s cm-1. The SEM image of the MWCNTs was show in Figure 1, and the Zeta potential distribution was shown in Supplementary Figure S1.
2.2 Experimental design
CdCl2 solution (CdCl2·5H2O, analytically pure) was added to soil in a plastic bucket and mixed thoroughly. According to the toxicity of Cd in soil and previous study (Zhang et al., 2019), the target Cd concentration in the soil was determined to be 5.0 mg kg-1. After 3 months of equilibration, the soils contaminated with Cd were air-dried and passed through a 2 mm-mesh sieve. The soils had final Cd concentrations of 5.11 ± 0.35 mg kg-1.
Two doses of MWCNTs (100 and 500 mg kg-1 soil) were set in this study according to the toxicity to the plants and remediation effect reported in previous study (Gong et al., 2019; Cao et al., 2022). To prevent nanoparticle aggregation during the addition process, a stepwise amplification strategy was used (Xu et al., 2015). One hundred milligrams of MWCNTs powder were mixed uniformly with 25 g dry weight (d.w.) of soil, then sequentially mixed with 100 and 875 g (d.w.) soil to obtain a concentration of 100 mg kg-1 of MWCNTs in soil. Five kilograms treated soil was added into a plant pot (diameter 200 mm, height 320 mm). There were a total of 6 treatments, and 3 replications were performed for each treatment. The treatments and serial numbers of the pot experiment are shown in Table 2.
The WUYOU116 test rice, commonly grown in Guangdong Province, was supplied by the Rice Research Institute, Guangdong Academy of Agricultural Science, Guangzhou, China. Seedlings were transplanted into the experimental box when the rice grown to 80 mm height, one seeding per plot. A 3 cm deionized water layer was maintained in the experimental plot. The pot experiment was carried out under natural light and heat (temperature variation: 20.1°C–35.9°C) in a greenhouse from April 5th to July 27th in 2022 at Peaking University Shenzhen Graduate School. According to the nutrient requirements of rice at different growth stages (Liu et al., 2023), 1.0 g KH2PO4 and CO(NH2)2 were dissolved in 100 mL water and added in the pot in pre-tillering stage (May 1st), flowering stage (June 10th) and heading stage (June 28th).
Plant height was measured at the tillering stage (May 5th), booting stage (June 6th), and heading stage (July 5th). Oryza sativa L. leaves were also tested for physiological and biochemical indexes at various growth stages.
The pot experiment lasted for 113 days, and rice (O. sativa L.) was harvested in July 27th. Tillering numbers were counted, and the plants were divided into three parts: roots, shoots, and ears. All plant tissues were washed with deionized water to remove the soil and dust, and then dried in a drying oven at 70°C to a constant weight. Ears were husked, and all the plant samples were pulverized using a plant grinding machine. The soil samples were obtained from various places in the pots, combined evenly to make a sample for each treatment, and thereafter left to air dry, crushed, and sifted through a 100 mesh sieve.
2.3 Measurement of Cd contents in soil and plants
The soil samples underwent digestion using a mixed-acid digestion method consisting of HCl-HNO3-HF-HClO4 (HJ1315, 2023, Ministry of Ecology and Environment, PRC). The following steps were taken: soil samples (0.2 g) were placed in a Teflon crucible and wetted with 0.2 mL deionized water. Then, 10 mL hydrochloric acid (concentration: 37%) was placed in the Teflon crucible with heating on an electric heating plate at 90–100°C. When the digestion solution had evaporated to about 3 mL, 15 mL nitric acid (concentration: 69%–70%) was added. The crucible was covered and heated until there were no visible particles in the solution. After that, the cap was removed, 10 mL hydrofluoric acid (concentration ≥40%) was added, and the temperature was set at 120°C for an hour. Finally, 5 mL perchloric acid (concentration: 70%–72%) was added. The crucible was heated at 150°C–170°C until the digestion solution became sticky and no longer flowing. After cooling, the residue was dissolved in a 5% nitric acid solution, filtered, and brought to a constant volume.
The HNO3-HClO4 mixed-acid approach was employed to digest shoot, root, and grain samples of plants (Zhang et al., 2016). The samples (2.0 g) were incorporated into a triangular flask, to which 10 mL of nitric acid (concentration: 69%–70%) was added, mixed, and left for a minimum of 8 h. Subsequently, 3 mL of perchloric acid (concentration: 70%–72%) was introduced, and the mixture was heated between 140°C and 160°C on an electric heating plate until the digestion solution became pale and mushy. The samples were filtered and diluted to a constant volume with deionized water.
Glacial acetic acid (HOAc) (0.11 mol L−1) was utilized for extraction of bioavailable Cd, 1.0 g soil sample was placed in the centrifuge tube (100 mL), and 40 mL 0.11 mol L−1 HOAc was added in the tube. Then, centrifuge tube was placed in a constant temperature shaker, shaking for 16 h in 22°C ± 5°C, with the speed of 200 r·min-1. After that, the centrifuge tube was centrifuged for 20 min at 4,000 rpm min-1, and the supernatant liquid was collected.
Inductively coupled plasma-mass spectrometry (Agilent 7,700x ICP-MS) was employed to measure the amounts of Cd in the extractants.
2.4 Measurement of chlorophyll content
Two hundred milligrams of fresh leaves were homogenized with acetone-anhydrous ethanol (2:1) extracting agent and a small amount of CaCO3 was transferred to a 25 mL volumetric bottle, and ultrasonicated (50 Hz, 200 W, 25 °C) for 10 min. Absorbances of the supernatants were read at 649 and 665 nm. The total chlorophyll content (mg g-1) was determined as follows:
2.5 Measurement of soluble protein and malondialdehyde (MDA) content
Soluble protein levels were measured using Coomassie Brilliant Blue (Bradford, 1976). Fresh leaf tissue (0.2 g) was centrifuged for 10 min at 4,000 r min-1 after being homogenized in distilled water (5 mL). Supernatants were mixed with 5 mL of Coomassie reagent, absorbances were read at 595 nm, and the soluble protein amount was assessed as:
where
The quantification of MDA was performed utilizing a colorimetric approach based on thiobarbituric acid (TBA) (Lee and Lee, 2000). After homogenizing fresh leaves (1.0 g) with trichloroacetic acid (TCA, 3 mL, 10%), the sample was centrifuged for 10 min at 4,000 rpm. After being heated for 35 min at 95°C, the mixture of supernatant (2 mL) and TBA (2 mL, 0.5%) was rapidly cooled and centrifuged. Supernatant absorbances were read at 450, 532, and 600 nm, and the content of MDA was determined using the following formula:
where
2.6 Superoxide dismutase (SOD), peroxidase (POD), and catalase (CAT) activity measurement
Two hundred milligrams of fresh leaves were homogenized in phosphate buffer (8 mL, 0.1 mmol-1, pH 7.0, with 0.1 μmol L-1 EDTA, ω = 1% (wt/vol) polyvinyl pyrrolidone) and a small amount of silica sand.
The homogenates were subjected to a 15-min centrifugation at 11,500 g for 4°C, and enzyme activities were measured in the supernatants. SOD, POD and CAT activities were analyzed using the nitrogen blue tetrazole photoreduction, guaiacol, and iodometry methods, respectively, the details for analysis were described by Chen et al. (2021).
2.7 Statistical analysis
Data were analyzed utilizing IBM SPSS 22.0 software and are represented as the mean ± standard deviation (SD). Tukey’s HSD tests were performed to determine the significance differences (P < 0.05) between individual treatments. Two-way ANOVA was performed to test the significance of Cd and MWCNTs and their interaction on plant growth and physiological indices.
3 Results
3.1 Changes in the morphological properties of plant
As shown in Table 3, Cd stress inhibited plant height, especially at the tillering stage. In comparison to the C0M0 treatment, the C5M0 treatment led to a 12.5% decrease in plant heights. MWCNTs addition also inhibited plant growth during the tillering stage; the addition of MWCNTs (500 mg kg-1) reduced plant height by 8.0%. Following Cd stress, the plant height in the treatments with MWCNT addition was slightly greater than that in the treatment without MWCNT addition. When compared to the C5M0 treatment, the C5M1 and C5M5 treatments resulted in a 7.9% and 8.5% increase in plant height, respectively. The outcomes demonstrated that the inclusion of MWCNTs could mitigate the negative impact of Cd on growth. However, during the booting and heading stages, this type of impact was not apparent.
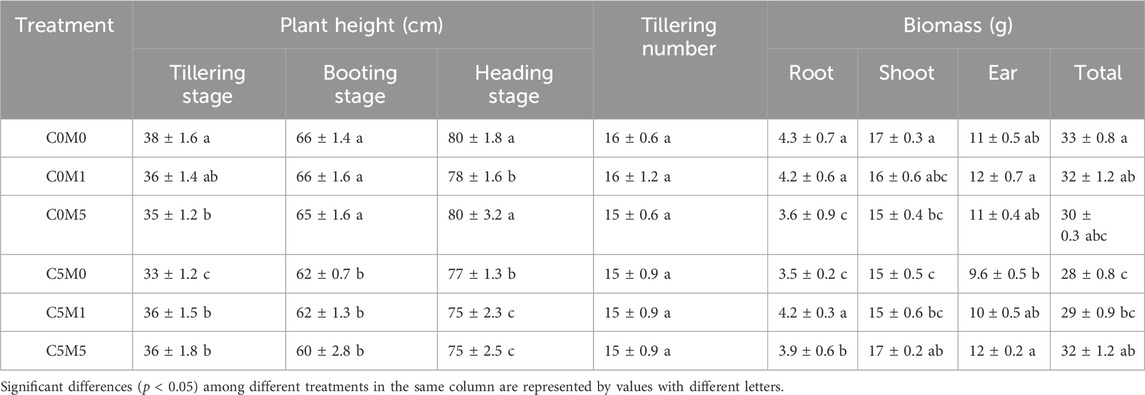
Table 3. Impact of different treatments on height, tillering numbers, and biomass of rice plants. Mean values ±S.D. are shown (n = 3).
There was no significant difference in tillering number between different doses of Cd and MWCNTs addition treatments. Cd stress significantly reduced root biomass, shoot biomass, and total biomass in the C5M0 treatments, which were 18.6%, 11.7%, and 15.2% lower than in the C0M0 treatment, respectively. A 500 mg kg-1 MWCNTs addition caused 16.3% and 11.8% reductions in root and shoot biomass, respectively, in contrast to the C0M0 treatment. However, relative to the C5M0 treatment, the C5M5 treatment resulted in an increase of 14.3% in total biomass, 11.4% in root biomass, 13.3% in shoot biomass, and 25.0% in ear biomass. Cd and MWCNTs have a interaction on the total biomass (F = 7.02, P < 0.01), root biomass (F = 45.24, P < 0.01), and shoot biomass (F = 7.77, P < 0.01) (Supplementary Table S1).
3.2 Changes in Cd content
The introduction of MWCNTs into the soil led to substantially reduced levels of bioavailable Cd (Figure 2). In particular, the C5M1 and C5M5 treatments exhibited a decrease of 0.18 and 0.33 mg kg-1 in bioavailable Cd, respectively, in contrast to the C5M0 treatment.
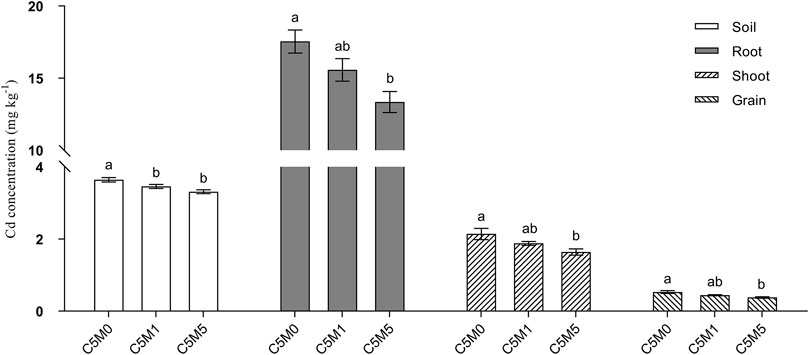
Figure 2. Content of Cd in the grain, roots, shoots, and soil. Mean values ±S.D. are shown (n = 3). Significant differences are shown by various letters above each column (p < 0.05).
A 500 mg kg-1 MWCNT addition to soil considerably reduced the tissue Cd contents (Figure 2). The addition of 100 mg kg-1 MWCNTs had a negligible impact on the variations in the amount of Cd found in plant tissues. Cd contents in roots, shoots, and grain in the C5M5 treatment decreased by 23.9%, 23.4%, and 28.3%, respectively, relative to the C5M0 treatment. Even though the introduction of MWCNTs (500 mg kg-1) greatly decreased the Cd concentration in rice grain, it was still higher than the Chinese threshold for rice grain concentration of Cd (0.2 mg kg-1) (GB2762-2017).
The total Cd content uptake by rice plant in C5M0, C5M1 and C5M5 treatment was 0.099, 0.098 and 0.083 mg. The Cd content uptake by rice in C5M5 treatment decreased by 16.16% compared to C5M0 treatment.
3.3 Changes in plant biochemical characteristics
3.3.1 Chlorophyll content
At the tillering and booting stages, leaf chlorophyll content was significantly reduced due to Cd stress. In the C5M0 treatment, the chlorophyll content dropped by 0.20 and 0.25 mg kg-1, respectively, in contrast to the C0M0 treatment (Table 4).
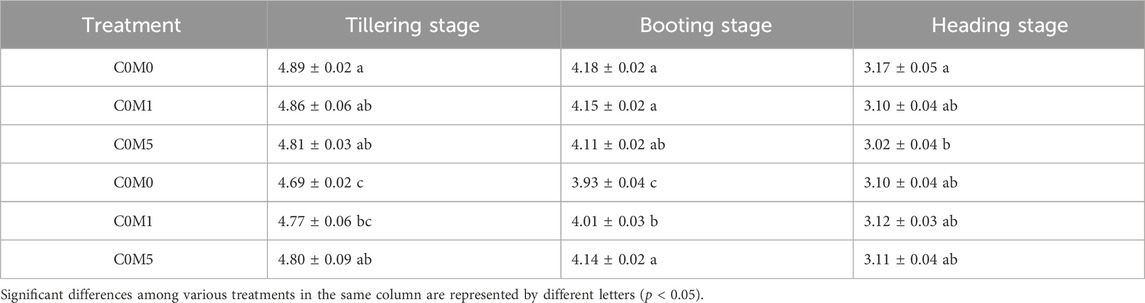
Table 4. Total chlorophyll content in Oryza sativa L. leaves (mg kg-1). Mean values ±S.D. are shown (n = 3).
C5M5 treatment resulted in a 0.11 and 0.21 mg kg-1 increase in chlorophyll contents throughout the tillering and booting stages, respectively, relative to C5M0 treatment. Cd did not affect the amount of chlorophyll in the leaf at the heading stage; however, a single 500 mg kg-1 MWCNT addition led to a noticeable drop in leaf chlorophyll content. Cd and MWCNTs have a interaction on the chlorophyll content at the tillering (F = 4.34, P < 0.05) and booting (F = 18.03, P < 0.01) stages (Supplementary Table S2).
3.3.2 Soluble protein and MDA content
As demonstrated in Figure 3A, at various growth stages, the soluble protein content rose in proportion to the Cd level in the soil. Relative to the C0M0 treatment, the soluble protein content rose by 24.5%, 36.4%, and 38.4% in the C5M0 treatment, respectively, during the tillering, booting, and heading stages. Compared with the controls, the inclusion of 500 mg kg-1 MWCNTs at the tillering stage increased the soluble protein content by 13.7%. The soluble protein concentration is drastically reduced when 500 mg kg-1 of MWCNTs are added under Cd stress conditions. At the tillering, booting, and heading stages, the soluble protein concentration dropped in the C5M5 treatment by 8.1%, 13.7%, and 15.6%, respectively, in comparison to the C5M0 group. Cd and MWCNTs have a interaction on the soluble protein content at the tillering (F = 8.23, P < 0.01) and heading (F = 6.86, P < 0.05) stages (Supplementary Table S2).
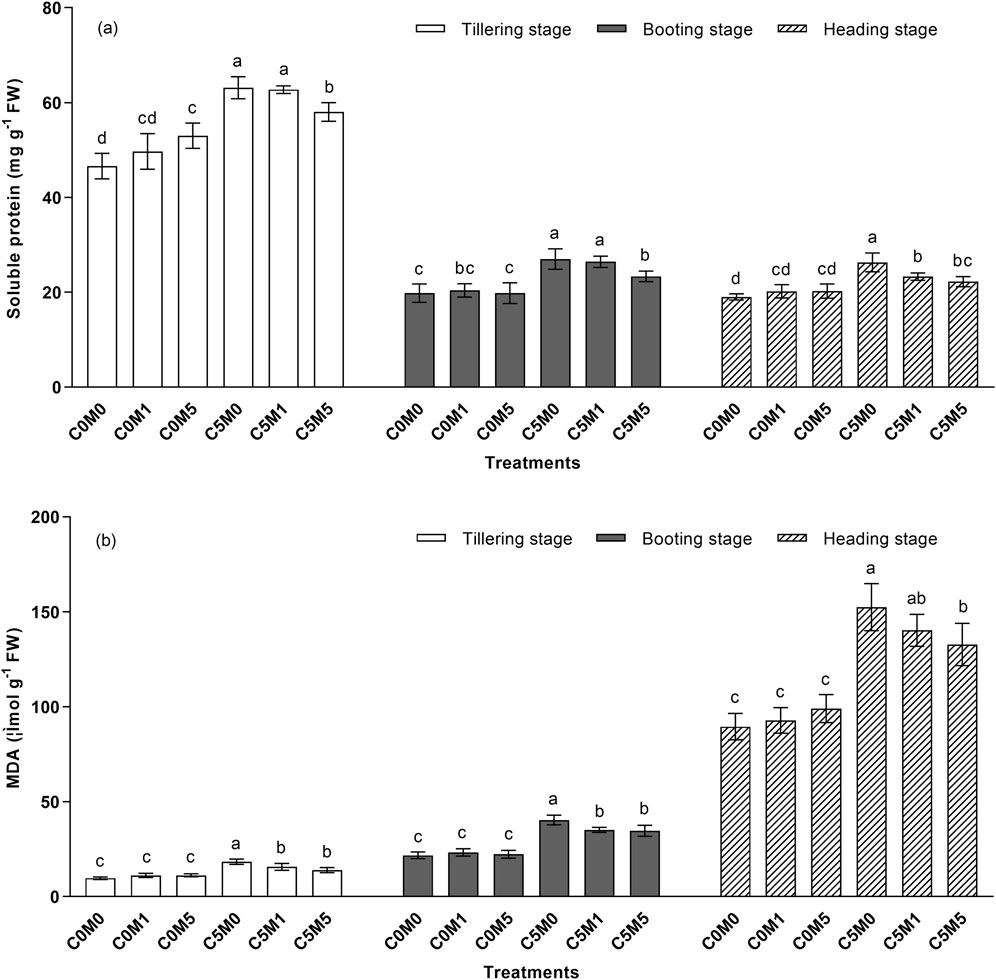
Figure 3. Alterations in the contents of soluble protein (A) and MDA (B) in Oryza sativa L. leaves. Mean values ±S.D. are shown (n = 3). Significant differences (p < 0.05) between treatment conditions at the same stage of growth are indicated by different letters above the column.
The MDA content increased significantly under Cd stress at various growth stages, as illustrated in Figure 3B. The MDA content was considerably greater in the C5M0 treatment relative to the C0M0 treatment. The inclusion of MWCNTs (100 and 500 mg kg-1) reduced Cd stress by 15.5% and 23.9%, respectively, during the tillering stage as compared to a treatment without MWCNTs. In comparison to the C5M0 treatment, the MDA content decreased by 12.9% and 14.1%, respectively, in the C5M1 and C5M5 treatments during the booting stage. C5M5 treatments resulted in a 12.9% decrease in MDA content at the heading stage when compared to C5M0 treatments. Cd and MWCNTs have a interaction on the MDA content at the tillering (F = 9.35, P < 0.01) and booting (F = 4.63, P < 0.05) stages (Supplementary Table S2).
3.3.3 Activities of antioxidant enzymes
Activities of antioxidant enzymes increased in the leaves following Cd stress. Figure 4A shows that, in comparison to the C0M0 treatment, there was a 14.3% elevation in SOD activities in the C5M0 treatment during the tillering stage. SOD activity in the heading stage was greater in the C5M0 treatment than in the C0M0 treatment, by 36.4%. The inclusion of MWCNTs (500 mg kg-1) reduced SOD activity at the heading stage by 9.1% under Cd stress conditions.
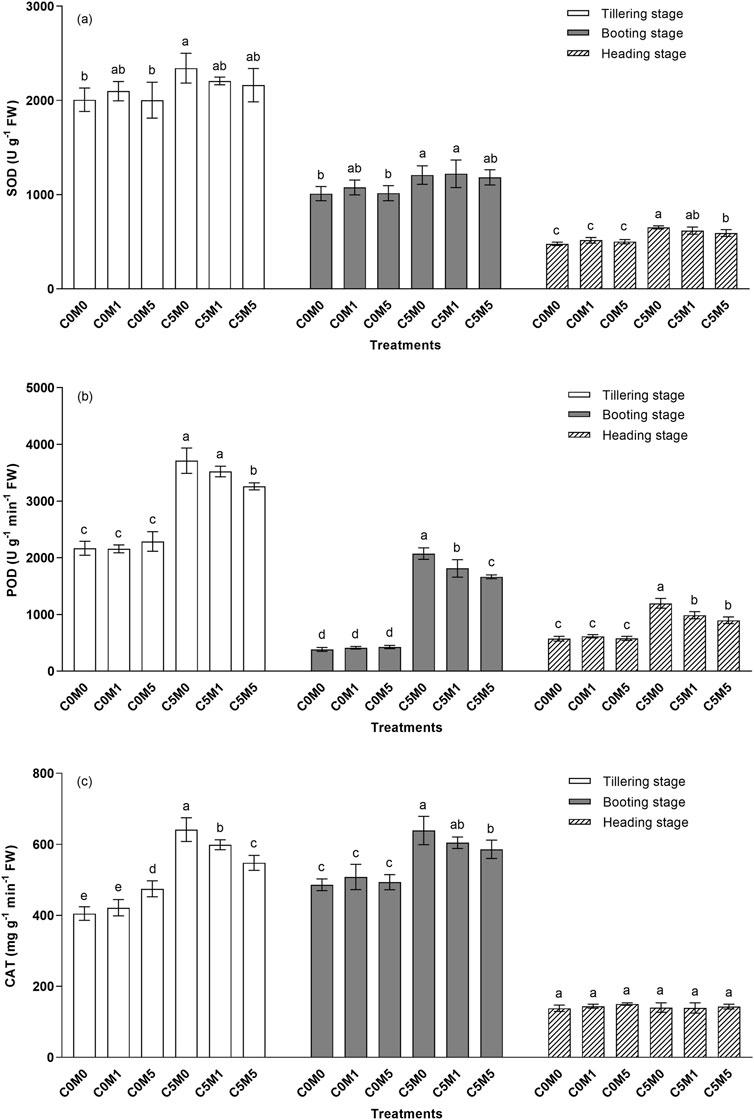
Figure 4. Variations in the activities of SOD (A), POD (B), and CAT (C) in Oryza sativa L leaves. Mean values ±S.D. are shown (n = 3). Significant differences (p < 0.05) between treatment groups in the same stage of growth are represented by different letters above the column.
At various growth stages, the POD activities in C5M0 were greater than in the C0M0 treatment, as indicated in Figure 4B. Under Cd stress conditions, 500 mg kg-1 MWCNTs addition markedly reduced POD activity. At the tillering stage, POD activity in C5M5 was reduced by 12.2% compared to the C5M0 treatment. POD activities in the C5M1 and C5M5 treatments were 12.6% and 19.7% lower at the booting stage, respectively, than in the C5M0 group. Relative to C5M0 treatment, both C5M1 and C5M5 lowered POD activities by 17.5% and 25.0%, respectively, during the heading stage. Cd and MWCNTs have a interaction on the POD activities at the tillering (F = 6.82, P < 0.05), booting (F = 12.44, P < 0.01), and heading (F = 12.79, P < 0.01) stages (Supplementary Table S2).
Figure 4C demonstrates that exposure to Cd stress resulted in elevated CAT activities during the tillering and booting stages. In the C5M0 treatments, CAT activities rose by 58.3% and 31.5% compared to the C0M0 treatment. At the tillering and booting stages, the activities of CAT in the C5M5 treatment decreased by 14.5% and 8.3% compared with those in the C5M0 treatment, respectively. Cd and MWCNTs have a interaction on the CAT activities at the tillering stage (F = 19.45, P < 0.01) (Supplementary Table S2).
4 Discussion
Due to the huge specific surface area, unique aperture structures, and high surface activity, etc., MWCNTs can adsorb coexisting contaminants and influence the bioavailability and biotoxicity of those contaminants (Torre-Roche et al., 2013). Here, it was found that the bioavailable Cd amount in soil was substantially reduced after adding 500 mg kg-1 of MWCNTs, which in turn led to a drop in the Cd concentration in rice plant tissues. Cd bioavailability may be decreased if MWCNTs aggregate in the soil or on the surface of the roots. Gong et al. (2019) discovered that adding MWCNTs led to a 21%–30% reduction in the Cd content in ramie roots. The effect of MWCNTs on coexisting contaminant bioavailability/uptake depended on the uptake of the MWCNTs/contaminant complex in plants (Gong et al., 2017; Rossi et al., 2017; Huang et al., 2018). The translocation of the MWCNTs/contaminant complex in the soil-plant system varies greatly with growth medium, plant species, MWCNTs diameter, length and concentration, etc. (Shen et al., 2018). The findings indicated that adding 500 mg kg-1 of MWCNTs decreased the Cd content in roots, shoots, and rice, suggesting poor mobility of the MWCNTs/contaminant complex in the soil-rice system.
Plant height, biomass, and tillering number are often used as morphological indicators to judge Cd stress on growth (Zhang et al., 2019). Here, plant height and root biomass were found to be considerably reduced under 5.0 mg kg-1 Cd levels, indicating that Cd stress impeded the growth of O. sativa L. This was more noticeable at the tillering stage.
Previous research has documented the inhibitory impact of MWCNTs on growth in plants (Hao et al., 2018; Moll et al., 2016). Here, plant height was shown to be reduced under 500 mg kg-1 MWCNTs addition at the tillering stage. Additionally, the biomass of the roots and shoots were also dropped under MWCNTs addition. MWCNTs may negatively impact the growth of O. sativa L. by attaching to or being absorbed by plant roots, leading to plant cell death and membrane damage (Hao et al., 2018). Similar outcomes were also noted by Cao et al. (2018), who observed that adding 50 and 500 mg kg-1 MWCNTs to the soil markedly reduced the diameter and shrinkage of cortical cells in roots as well as the lengths of shoots and roots.
Under Cd stress conditions, the addition of MWCNTs enhanced plant height and total biomass. This suggested that adding MWCNTs could lessen the harmful impacts of Cd. Chen et al. (2021) discovered in their investigation that using MWCNTs could lessen heavy metal toxicities (Cd and As) by increasing the fresh weights of shoots and roots. The use of MWCNTs had a beneficial effect on the morphology of Boehmeria nivea L. under Cd stress, according to Gong et al. (2019).
The amount of chlorophyll in plants can influence photosynthesis, which in turn can impact plant growth. During the tillering stage, in particular, the chlorophyll content of plant leaves diminished noticeably after exposure to Cd stress. The substitution of a metal element in chlorophyll by Cd resulted in reduced chlorophyll contents (Agrawal and Mishra, 2009; Khan et al., 2018). The addition of MWCNTs (500 mg kg-1) dramatically raised the leaf chlorophyll contents during the booting and tillering stages under 5 mg kg-1 Cd. This indicated that MWCNT addition alleviated Cd toxicity by promoting leaf photosynthesis. Our conclusion is in line with that of Gao et al. (2020), who observed that under high Cd levels, the chlorophyll content was enhanced by foliar graphene oxide treatment. Meanwhile, the increase in chlorophyll content is a possible reason for the increase in plant height and biomass in the MWCNTs addition treatments.
Soluble proteins are crucial for preserving the integrity of the cytomembrane when exposed to stress (Lewis et al., 2019). A higher content of soluble protein indicates greater stress on plants (Fazelian et al., 2020; Geng et al., 2020; Rico et al., 2013). Here, the soluble protein content increased upon exposure to both single MWCNTs and single Cd. MWCNTs and Cd can damage root cell structures and functions, activate defensive systems, and lead to an increase in protein synthesis (Dai et al., 2020; Guan et al., 2020). Under Cd stress condition, the addition of MWCNTs (500 mg kg-1) decreased the soluble protein content during the booting and heading stage. Based on the findings, the toxicity of MWCNTs combined with Cd was less than that of Cd alone.
MDA is produced by lipid peroxidation in plants; its concentration is frequently utilized as a marker of oxidative stress (Huang et al., 2016). Mishra et al. (2006) found that stress caused by heavy metals could increase MDA levels in plants. Cd stress significantly increased MDA concentration across all growth stages in this study. Cd exposure aggravated the peroxidation of membrane lipids, leading to a significant accumulation of MDA (Ahmad et al., 2021). Figure 3B shows that the MDA content at various growth stages was significantly reduced after the addition of MWCNTs under Cd stress conditions, suggesting that the MWCNTs decreased the oxidative stress generated by Cd. Chen et al. (2021) and Sharifi et al. (2021) both documented comparable findings. The reduction of oxidative stress may be attributed to the decreased bioaccumulation of Cd caused by the addition of MWCNTs.
Furthermore, Cd stress can induce metabolic abnormalities in plants, leading to increased production of reactive oxygen species (ROS) (Cuypers et al., 2011; Huang et al., 2012; Abdel Latef, 2013). Antioxidant enzymes can reduce ROS damage to plant cells by the elimination of excess ROS (Fidalgo et al., 2011; Kumar et al., 2012). Figure 4 shows that the activities of the antioxidant enzymes POD, SOD, and CAT in leaves were higher in the C5M0 treatment than in the C0M0 treatment, indicating that Cd stress improved these activities. Chaudhary and Sharma (2009) reported similar findings, indicating that Cd stress activated the antioxidant defense system. Under 5.0 mg kg-1 Cd stress condition, 500 mg kg-1 MWCNTs addition reduced POD and CAT, especially at the tillering and booting stages. Additionally, alterations in antioxidant enzyme activity suggested that the addition of MWCNTs reduced Cd-induced toxicity in O. sativa L. Similarly, Rong et al. (2018) discovered that MWCNTs increased oxidative damage resulting from Pb and Cd in seedlings of Vicia faba L.
Soil pH has significant positive correlation (p < 0.01) with MWCNTs concentration and cation exchange capacity, however, has significant negative correlation (p < 0.01) with bioavailable Cd content in soil, root, shoot, and grain (Supplementary Figure S2). Based on the aforementioned findings, it can be inferred that the incorporation of MWCNTs increase the soil pH and CEC, and then cause decrease of the Cd bioavailability in soil and the bioaccumulation of Cd in rice plant tissues. This, in turn, influences the activities of antioxidant enzymes and oxidative stress. In addition to impacting the toxicity of coexisted Cd, MWCNTs also affect the migration of Cd in soil, which may cause pollution diffusion. Furthermore, the potential risks of MWCNTs to plants, animals, and microorganisms in soil ecosystems are still poorly understood. In future, appropriate risk assessment method for nanoparticles needs to be established, especially at the soil ecosystem, to prevent their potential negative environmental impacts.
5 Conclusion
Here, the separate and combined impacts of Cd and MWCNTs on the growth of rice plants were investigated. The findings indicated that exposure to Cd stress resulted in a marked reduction in root biomass, plant heights, and chlorophyll contents in the leaves, together with elevated levels of POD, SOD, and CAT activities. The growth of rice was slightly impacted by the introduction of a single MWCNT, whereas the addition of MWCNTs (500 mg kg-1) substantially decreased plant height while simultaneously increasing soluble protein contents and CAT activity in the leaves during the tillering stage. The inclusion of MWCNTs (500 mg kg-1) under high Cd levels led to a marked decrease in the amount of bioavailable Cd in the soil as well as in the roots, shoots, and grains. Cd in combination with MWCNTs (500 mg kg-1) resulted in decreased levels of soluble protein, MDA, and antioxidant enzyme activity relative to Cd alone. The results indicated that MWCNTs alleviated Cd-induced toxicity in rice growth. However, the comprehensive impacts of Cd and MWCNTs on plant growth in different soil conditions (soil texture, pH, organic matter content and ionic strength, etc.) still need further research.
Data availability statement
The raw data supporting the conclusions of this article will be made available by the authors, without undue reservation.
Author contributions
JL: Formal Analysis, Methodology, Writing–original draft. XW: Formal Analysis, Methodology, Writing–original draft. WZ: Conceptualization, Writing–review and editing.
Funding
The author(s) declare that financial support was received for the research, authorship, and/or publication of this article. This research was funded by the Science and Technology Project of Hebei Education Department, grant number BJ2021023, and the Scientific Research Foundation of Hebei University of Economics and Business, grant number 2021ZD01 and 2021YB01.
Conflict of interest
The authors declare that the research was conducted in the absence of any commercial or financial relationships that could be construed as a potential conflict of interest.
Publisher’s note
All claims expressed in this article are solely those of the authors and do not necessarily represent those of their affiliated organizations, or those of the publisher, the editors and the reviewers. Any product that may be evaluated in this article, or claim that may be made by its manufacturer, is not guaranteed or endorsed by the publisher.
Supplementary material
The Supplementary Material for this article can be found online at: https://www.frontiersin.org/articles/10.3389/fenvs.2024.1469172/full#supplementary-material
References
Abdel Latef, A. A. (2013). Growth and some physiological activities of pepper (Capsicum annuum L.) in response to cadmium stress and mycorrhizal symbiosis. J. Agric. Sci. Technol. 15, 1437–1448. doi:10.1016/j.biosystemseng.2013.07.010
Agrawal, S. B., and Mishra, S. (2009). Effects of supplemental ultraviolet-B and cadmium on growth, antioxidants and yield of Pisum sativum L. Ecotoxicol. Environ. Safe. 72 (2), 610–618. doi:10.1016/j.ecoenv.2007.10.007
Ahmad, A., Yasin, N. A., Khan, W. U., Akram, W., Wang, R., Shah, A. A., et al. (2021). Silicon assisted ameliorative effects of iron nanoparticles against cadmium stress: attaining new equilibrium among physiochemical parameters, antioxidative machinery, and osmoregulators of Phaseolus lunatus. Int. J. Phytoremediation 166, 874–886. doi:10.1016/j.plaphy.2021.06.016
Babbitt, C. W., and Moore, E. A. (2018). Sustainable nanomaterials by design. Nat. Nanotechnol. 13, 621–623. doi:10.1038/s41565-018-0235-7
Bradford, M. M. (1976). A rapid and sensitive method for the quantitation of microgram quantities of protein utilizing the principle of protein-dye binding. Anal. Biochem. 72, 248–254. doi:10.1016/0003-2697(76)90527-3
Cao, W., Gong, J., Zeng, G., Qin, M., Qin, L., Zhang, Y., et al. (2022). Impacts of typical engineering nanomaterials on the response of rhizobacteria communities and rice (Oryza sativa L.) growths in waterlogged antimony-contaminated soils. J. Hazard. Mater. 430, 128385. doi:10.1016/j.jhazmat.2022.128385
Cao, W., Gong, J., Zeng, G., Song, B., Zhang, P., Li, J., et al. (2020). Abiotic mediation of common ions on the co-exposure of CeO2 NPs with Sb(III) or Sb(V) to Glycine max (Linn.) Merrill. (Soybean): impacts on uptake, accumulation and physiochemical characters. Environ. Pollut. 267, 115594. doi:10.1016/j.envpol.2020.115594
Chaudhary, S., and Sharma, Y. K. (2009). Interactive studies of potassium and copper with cadmium on seed germination and early seedling growth in maize (Zea mays L.). J. Environ. Biol. 30, 427–432.
Chen, J. L., Zeng, X. Y., Yang, W. J., Xie, H. J., Ashraf, U., Mo, Z., et al. (2021). Seed priming with multiwall carbon nanotubes (MWCNTs) modulates seed germination and early growth of maize under cadmium (Cd) toxicity. J. Soil Sci. Plant Nut. 21 (3), 1793–1805. doi:10.1007/s42729-021-00480-6
Chen, X., Wang, J., Hayat, K., Zhang, D., and Zhou, P. (2021). Small structures with big impact: multi-walled carbon nanotubes enhanced remediation efficiency in hyperaccumulator Solanum nigrum L. under cadmium and arsenic stress. Chemosphere 276, 130130. doi:10.1016/j.chemosphere.2021.130130
Cuypers, A., Karen, S., Jos, R., Kelly, O., Els, K., Tony, R., et al. (2011). The cellular redox state as a modulator in cadmium and copper responses in Arabidopsis thaliana seedlings. J. Plant Physiol. 168, 309–316. doi:10.1016/j.jplph.2010.07.010
Dai, Y., Chen, F., Yue, L., Li, T., Jiang, Z., Xu, Z., et al. (2020). Uptake, transport, and transformation of CeO2 nanoparticles by strawberry and their impact on the rhizosphere bacterial community. ACS Sustain. Chem. Eng. 8, 4792–4800. doi:10.1021/acssuschemeng.9b07422
Fazelian, N., Yousefzadi, M., and Movafeghi, A. (2020). Algal response to metal oxide nanoparticles: analysis of growth, protein content, and fatty acid composition. BioEnergy Res. 13, 944–954. doi:10.1007/s12155-020-10099-7
Fidalgo, F., Freitas, R., Ferreira, R., Pessoa, A. M., and Teixeira, J. (2011). Solanum nigrum L. antioxidant defence system isozymes are regulated transcriptionally and posttranslationally in Cd-induced stress. Environ. Exp. Bot. 72, 312–319. doi:10.1016/j.envexpbot.2011.04.007
Gao, M. L., Chang, X. P., Yang, Y. J., and Song, Z. G. (2020). Foliar graphene oxide treatment increases photosynthetic capacity and reduces oxidative stress in cadmium-stressed lettuce. Plant Physiol. Bioch 154, 287–294. doi:10.1016/j.plaphy.2020.06.021
Geng, L., Yang, Z., and Xu, Z. (2020). Effects of antimony contamination in soil on the nutrient composition of three green leafy vegetables. J. Soils Sediment. 20, 2217–2224. doi:10.1007/s11368-020-02577-4
Gong, X., Huang, D., Liu, Y., Peng, Z., Zeng, G., Xu, P., et al. (2017). Remediation of contaminated soils by biotechnology with nanomaterials: bio-behavior, applications, and perspectives. Criti. Rev. Biotechnol. 38, 455–468. doi:10.1080/07388551.2017.1368446
Gong, X. M., Huang, D. L., Liu, Y. G., Zeng, G. M., Wang, R. Z., Xu, P., et al. (2019). Roles of multiwall carbon nanotubes in phytoremediation: cadmium uptake and oxidative burst in Boehmeria nivea (L.) Gaudich. Environ. Sci. Nano. 6, 851–862. doi:10.1039/c8en00723c
Guan, X., Gao, X., Astrid, A., Eleanor, S. S., Xu, J., Laughton, S., et al. (2020). CuO nanoparticles alter the rhizospheric bacterial community and local nitrogen cycling for wheat grown in a calcareous soil. Environ. Sci. Technol. 54, 8699–8709. doi:10.1021/acs.est.0c00036
Hao, Y., Ma, C. X., Zhang, Z., Song, Y., Cao, W., Guo, J., et al. (2018). Carbon nanomaterials alter plant physiology and soil bacterial community composition in a rice-soil-bacterial ecosystem. Environ. Pollut. 232, 123–136. doi:10.1016/j.envpol.2017.09.024
Hatami, M. (2017). Toxicity assessment of multi-walled carbon nanotubes on Cucurbita pepo L. under well-watered and water-stressed conditions. Ecotoxicol. Environ. Safe. 142, 274–283. doi:10.1016/j.ecoenv.2017.04.018
HJ1315 (2023). Soil and sediment-determination of 19 total metals elements-Inductively coupled plasma mass spectrometry. Ministry Ecol. Environ. People's Repub. China. Available at: https://www.mee.gov.cn/ywgz/fgbz/bz/bzwb/jcffbz/202312/t20231208_1058562.shtml (Accessed November 27, 2023).
Hochella, M. F., Mogk, D. W., Ranville, J., Allen, I. C., Luther, G. W., Marr, L. C., et al. (2019). Natural, incidental, and engineered nanomaterials and their impacts on the Earth system. Science. 363, eaau8299. doi:10.1126/science.aau8299
Hu, Y., Zhang, P., Zhang, X., Liu, Y., Feng, S., Guo, D., et al. (2021). Multi-wall carbon nanotubes promote the growth of maize (Zea mays) by regulating carbon and nitrogen metabolism in leaves. J. Agric. Food Chem. 69 (17), 4981–4991. doi:10.1021/acs.jafc.1c00733
Huang, D. L., Qin, X., Peng, Z. W., Liu, Y. G., Gong, X. M., Zeng, G., et al. (2018). Nanoscale zero-valent iron assisted phytoremediation of Pb in sediment: impacts on metal accumulation and antioxidative system of Lolium perenne. Ecotox. Environ. Safe. 153, 229–237. doi:10.1016/j.ecoenv.2018.01.060
Huang, D. L., Wang, Y., Zhang, C., Zeng, G. M., Lai, C., Wan, J., et al. (2016). Influence of morphological and chemical features of biochar on hydrogen peroxide activation: implications on sulfamethazine degradation. Rsc Adv. 6, 73186–73196. doi:10.1039/C6RA11850J
Huang, H. G., Gupta, D. K., Tian, S. K., Yang, X. E., and Li, T. X. (2012). Lead tolerance and physiological adaptation mechanism in roots of accumulating and non-accumulating ecotypes of Sedum alfredii. Environ. Sci. Pollut. Res. 19, 1640–1651. doi:10.1007/s11356-011-0675-1
Jordan, J. T., Oates, R. P., Subbiah, S., Payton, P. R., Singh, K. P., Shah, S. A., et al. (2020). Carbon nanotubes affect early growth, flowering time and phytohormones in tomato. Chemosphere 256, 127042. doi:10.1016/j.chemosphere.2020.127042
Khan, W. U., Yasin, N. A., Ahmad, S. R., Ali, A., Ahmad, A., Akram, W., et al. (2018). Role of Burkholderia cepacia CS8 in Cd-stress alleviation and phytoremediation by Catharanthus roseus. Int. J. Phytoremediation 20 (6), 581–592. doi:10.1080/15226514.2017.1405378
Kumar, A., Prasad, M. N. V., and Sytar, O. (2012). Lead toxicity, defense strategies and associated indicative biomarkers in Talinum triangulare grown hydroponically. Chemosphere 89, 1056–1065. doi:10.1016/j.chemosphere.2012.05.070
Lahiani, M. L., Dervishi, E., Chen, J. H., Nima, Z., Gaume, A., Biris, A. S., et al. (2013). Impact of carbon nanotube exposure to seeds of valuable crops. ACS Appl. Mater. Interfaces. 5, 7965–7973. doi:10.1021/am402052x
Lee, D. H., and Lee, C. B. (2000). Chilling stress-induced changes of antioxidant enzymes in the leaves of cucumber: in gel enzyme activity assays. Plant Sci. 159 (1), 75–85. doi:10.1016/S0168-9452(00)00326-5
Lee, S. M., Raja, P. M. V., Esquenazi, G. L., and Barron, A. R. (2018). Effect of raw and purified carbon nanotubes and iron oxide nanoparticles on the growth of wheatgrass prepared from the cotyledons of common wheat (triticum aestivum). Environ. Sci. Nano. 5 (1), 103–114. doi:10.1039/C7EN00680B
Lewis, R. W., Bertsch, P. M., and McNear, D. H. (2019). Nanotoxicity of engineered nanomaterials (ENMs) to environmentally relevant beneficial soil bacteria - a critical review. Nanotoxicology 13, 392–428. doi:10.1080/17435390.2018.1530391
Liu, K., Chen, Y., Li, S. Y., Wang, W. L., Zhang, W. Y., Zhang, H., et al. (2023). Differing responses of root morphology and physiology to nitrogen application rates and their relationships with grain yield in rice. Crop J. 11 (2), 618–627. doi:10.1016/j.cj.2022.07.019
Long, Z., Ma, C., Zhu, J., Wang, P., Zhu, Y., and Liu, Z. (2022). Effects of Carbonaceous materials with different structures on cadmium fractions and microecology in cadmium-contaminated soils. Int. J. Environ. Res. Public Health. 19, 12381. doi:10.3390/ijerph191912381
Mishra, S., Srivastava, S., Tripathi, R. D., Govindarajan, R., Kuriakose, S. V., and Prasad, M. (2006). Phytochelatin synthesis and response of antioxidant during cadmium stress in Baccopa monnieri L. Plant Physiol. 44, 25–37. doi:10.1016/j.plaphy.2006.01.007
Moll, J., Gogos, A., Bucheli, T. D., Widmer, F., and van der Heijden, M. G. (2016). Effect of nanoparticles on red clover and its symbiotic microorganisms. J. Nanobiotechnology 14, 36. doi:10.1186/s12951-016-0188-7
Pandey, K., Lahiani, M. H., Hicks, V. K., Hudson, M. K., Green, M. J., and Khodakovskaya, M. (2018). Effects of carbon-based nanomaterials on seed germination, biomass accumulation and salt stress response of bioenergy crops. PLoS One 13, e0202274. doi:10.1371/journal.pone.0202274
Peng, C., Xu, C., Liu, Q. L., Sun, L. J., Luo, Y. M., and Shi, J. (2017). Fate and transformation of CuO nanoparticles in the soil-rice system during the life cycle of rice plants. Environ. Sci. Technol. 51, 4907–4917. doi:10.1021/acs.est.6b05882
Priester, J. H., Ge, Y., Mielke, R. E., Horst, A. M., Moritz, S. C., Espinosa, K., et al. (2012). Soybean susceptibility to manufactured nanomaterials with evidence for food quality and soil fertility interruption. P. Natl. Acad. Sci. USA. 109, E2451–E2456. doi:10.1073/pnas.1205431109
Rico, C. M., Morales, M. I., Barrios, A. C., McCreary, R., Hong, J., Lee, W. Y., et al. (2013). Effect of cerium oxide nanoparticles on the quality of rice (Oryza sativa L.) grains. J. Agric. Food Chem. 61, 11278–11285. doi:10.1021/jf404046v
Rong, H., Wang, C. R., Yu, X. R., Fan, J. B., Jiang, P., Wang, Y., et al. (2018). Carboxylated multi-walled carbon nanotubes exacerbated oxidative damage in roots of Vicia faba L. seedlings under combined stress of lead and cadmium. Ecotoxicol. Environ. Safe. 161, 616–623. doi:10.1016/j.ecoenv.2018.06.034
Rossi, L., Sharifan, H., Zhang, W. L., Schwab, A. P., and Ma, X. M. (2017). Mutual effects and in-planta accumulation of co-existing cerium oxide nanoparticles and cadmium in hydroponically grown soybean (Glycine max (L.) Merr.). Environ. Sci. Nano 5, 150–157. doi:10.1039/c7en00931c
Ruotolo, R., Maestri, E., Pagano, L., Marmiroli, M., White, J. C., and Marmiroli, N. (2018). Plant response to metal-containing engineered nanomaterials: an omics-based perspective. Environ. Sci. Technol. 52, 2451–2467. doi:10.1021/acs.est.7b04121
Sainao, W. Q., Cao, J. X., Pang, H. L., Shi, Z. Z., and Feng, H. Q. (2020). Multi-walled carbon nanotubes: their effects on the physiological responses of Oryza sativa L. seedlings and the toxicity of trichlorobenzene. Chin. J. Appl. Environ. Biol. 26 (3), 534–542. doi:10.19675/j.cnki.1006-687x.2019.07037
Sharifi, P., Bidabadi, S. S., Zaid, A., and Arafat, A. H. A. L. (2021). Efficacy of multi-walled carbon nanotubes in regulating growth performance, total glutathione and redox state of Calendula officinalis L. cultivated on Pb and Cd polluted soil. Ecotoxicol. Environ. Safe. 213, 112051. doi:10.1016/j.ecoenv.2021.112051
Shen, X., Li, S., Zhang, H., Chen, W., Yang, Y., Li, J., et al. (2018). Effect of multiwalled carbon nanotubes on uptake of pyrene by cucumber (Cucumis sativus L.): mechanistic perspectives. NanoImpact 10, 168–176. doi:10.1016/j.impact.2018.05.001
Song, B., Zeng, G. M., Gong, J. L., Zhang, P., Deng, J. Q., Deng, C., et al. (2017). Effect of multi-walled carbon nanotubes on phytotoxicity of sediments contaminated by phenanthrene and cadmium. Chemosphere 172, 449–458. doi:10.1016/j.chemosphere.2017.01.032
Tiwari, D. K., Dasgupta-Schubert, N., Villaseñor Cendejas, L. M., Villegas, J., Carreto Montoya, L., and Borjas García, S. E. (2014). Interfacing carbon nanotubes (CNT) with plants: enhancement of growth, water and ionic nutrient uptake in maize (Zea mays) and implications for nanoagriculture. Appl. Nanosci. 4, 577–591. doi:10.1007/s13204-013-0236-7
Torre-Roche, R. D. L., Hawthorne, J., Deng, Y., Xing, B., Cai, W. J., Newman, L. A., et al. (2013). Multiwalled carbon nanotubes and C60 fullerenes differentially impact the accumulation of weathered pesticides in four agricultural plants. Environ. Sci. Technol. 47 (21), 12539–12547. doi:10.1021/es4034809
Wan, J., Wang, R., Bai, H., Wang, Y., and Xu, J. (2020). Comparative physiological and metabolomics analysis reveals that single-walled carbon nanohorns and ZnO nanoparticles affect salt tolerance in sophora alopecuroides. Environ. Sci. Nano. 7 (10), 2968–2981. doi:10.1039/D0EN00582G
Wang, C. R., Liu, H. T., Chen, J. Y., Tian, Y., Shi, J., Li, D. D., et al. (2014). Carboxylated multi-walled carbon nanotubes aggravated biochemical and subcellular damages in leaves of broad bean (Vicia faba L.) seedlings under combined stress of lead and cadmium. J. Hazard. Mater. 274, 404–412. doi:10.1016/j.jhazmat.2014.04.036
Xu, C., Peng, C., Sun, L., Zhang, S., Huang, H., Chen, Y., et al. (2015). Distinctive effects of TiO2 and CuO nanoparticles on soil microbes and their community structures in flooded paddy soil. Soil Biol. and Biochem. 86, 24–33. doi:10.1016/j.soilbio.2015.03.011
Yang, X. X., Zhang, X. M., Shu, X., Zhang, W., Kai, J. R., Tang, M., et al. (2022). Effects of multi-walled carbon nanotubes in soil on earthworm growth and reproduction, enzymatic activities, and metabolomics. Ecotoxicol. Environ. Safe. 245, 114158. doi:10.1016/j.ecoenv.2022.114158
Yao, T., Liu, L., Tan, S., Li, H., Liu, X., Zeng, A., et al. (2021). Can the multi-walled carbon nanotubes be used to alleviate the phytotoxicity of herbicides in soils? Chemosphere 283, 131304. doi:10.1016/j.chemosphere.2021.131304
Zhai, G. S., Gutowski, S. M., Walters, K. S., Yan, B., and Schnoor, J. L. (2015). Charge, size, and cellular selectivity for multiwall carbon nanotubes by maize and soybean. Environ. Sci. Technol. 49, 7380–7390. doi:10.1021/acs.est.5b01145
Zhang, C., Lai, C., Zeng, G. M., Huang, D. L., Yang, C. P., Wang, Y., et al. (2016). Efficacy of carbonaceous nanocomposites for sorbing ionizable antibiotic sulfamethazine from aqueous solution. Water Res. 95, 103–112. doi:10.1016/j.watres.2016.03.014
Zhang, W., Long, J. H., Li, J., Zhang, M., Xiao, G. L., Ye, X., et al. (2019). Impact of ZnO nanoparticles on Cd toxicity and bioaccumulation in rice (Oryza sativa L.). Environ. Sci. Pollut. Res. 26, 23119–23128. doi:10.1007/s11356-019-05551-x
Zhao, Q., Ma, C. X., White, J. C., Dhankher, O. P., Zhang, X. J., Zhang, S., et al. (2017). Quantitative evaluation of multi-wall carbon nanotube uptake by terrestrial plants. Carbon 114, 661–670. doi:10.1016/j.carbon.2016.12.036
Zhao, R., Ren, W., Wang, H., Li, Z., Teng, Y., and Luo, Y. (2022). Nontargeted metabolomic analysis to unravel alleviation mechanisms of carbon nanotubes on inhibition of alfalfa growth under pyrene stress. Sci. Total Environ. 852, 158405. doi:10.1016/j.scitotenv.2022.158405
Keywords: cadmium, multiwall carbon nanotubes, oxidative stress, plant growth, soil pollution
Citation: Long J, Wang X and Zhang W (2024) Combined toxicity of multiwall carbon nanotubes and cadmium on rice (Oryza sativa L.) growth in soil. Front. Environ. Sci. 12:1469172. doi: 10.3389/fenvs.2024.1469172
Received: 23 July 2024; Accepted: 30 August 2024;
Published: 09 September 2024.
Edited by:
Xiaoping Xin, University of Florida, United StatesReviewed by:
Jaya Nepal, Cornell University, United StatesWiqar Ahmad, University of Agriculture, Pakistan
Copyright © 2024 Long, Wang and Zhang. This is an open-access article distributed under the terms of the Creative Commons Attribution License (CC BY). The use, distribution or reproduction in other forums is permitted, provided the original author(s) and the copyright owner(s) are credited and that the original publication in this journal is cited, in accordance with accepted academic practice. No use, distribution or reproduction is permitted which does not comply with these terms.
*Correspondence: Wei Zhang, emhhbmdkYXcwMDdAMTYzLmNvbQ==