- National Key Laboratory of Intense Pulsed Radiation Simulation and Effect, Northwest Institute of Nuclear Technology, Xi’an, China
A study for atmospheric transport is essential for the consequence assessment of severe nuclear accidents since radionuclides could be released from the nuclear facility into the atmosphere and cause radioactive pollution in the environment. Atmospheric transport behaviors are strongly related with meteorological conditions, which can obviously influence the transport and diffusion characteristics of radioactive materials in the atmosphere; thus, it is meaningful to investigate the coupling effects between meteorological processes and transport behaviors of radioactive materials. To evaluate the influence of meteorological conditions on atmospheric transport, meteorological parameters for different seasons were first acquired by the weather research forecast model. Furthermore, atmospheric transport behaviors of radioactive materials were simulated by the meso-scale numerical model under different meteorological conditions, and numerical analyses were conducted toward transport and deposition behaviors of radioactive materials. In addition, the influence of FDDA (four-dimensional data assimilation) on meteorological parameters and atmospheric transport behaviors was researched. The present study is important for strengthening consequence assessment for severe nuclear accident and made it possible to apply the data assimilation technology in further research works.
1 Introduction
Peaceful use of nuclear power made the world develop rapidly in the past several decades. However, people should realize that potential risks may be hidden behind the application of the nuclear power technology (Kessler et al., 2014). For instance, the Chernobyl accident in 1986 indicated that a massive amount of radioactive materials in the reactor can be released from the nuclear power plant to the atmosphere and transport to downwind regions far away from the nuclear facility, even causing long-term radioactive contamination to these areas (Ma et al., 2023). Thus, nuclear safety is an important issue in nuclear power research, and the effects of radioactive contamination in the environment should be taken into consideration theoretically.
According to the investigation of the Chernobyl accident (Brandt and Christensen, 2002), parts of radioactive materials transported and fell down to some Nordic countries, which are thousands of kilometers far away from the Chernobyl nuclear power plant. Such an investigation indicates that transport and diffusion behaviors of radionuclides in the atmosphere are typical meso-scale issues (Mazzaro et al., 2019), which means atmospheric movements should be considered in this spatial scale.
Figure 1 shows the schematic diagram for atmospheric transport under the condition of severe accident.
Transport and diffusion of radioactive materials are a result of joint forces in the atmosphere, such as pressure gradient force, viscous force, gravity, and inertia force. The pressure gradient force is strongly coupled with meteorological conditions, so transport characteristics in the atmosphere are complicated spatially and temporally compared with local-scale research. In addition, terrain characteristics could also lead to complex 3D (three-dimensional) atmospheric flow fields near the surface and furthermore change the movement of radioactive materials; thus, variation for underlying surfaces and terrain elevation should also be parameterized in meso-scale analysis. Generally speaking, variation of meteorological conditions has a significant influence on the transport and diffusion of radioactive materials. Therefore, coupling effects between radionuclide movement and atmospheric flow fields during severe nuclear accident are of importance and should be analyzed in detail.
After the Chernobyl and Fukushima accidents occurred, an increased number of researchers began to focus on research for aftermath evaluation in the environment, and atmospheric dispersion analysis has become a vital process in consequence assessment. In the near-range research field, de Sampaio (2008) introduced the computational fluid dynamics (CFD) method for analysis of atmospheric dispersion in the vicinity of nuclear power plants, which shows the importance of utilizing the CFD method in studying the dispersion problem in the local-scale problem. Barbero et al. (2015) used CFD codes to investigate the local-scale atmospheric dispersion and deposition of the particles based on an event of release in November 2007 in Spain, and the region of influence for particles in different diameters was acquired by CFD simulation. Wang et al. (2020) studied radionuclide atmospheric dispersion by 3D CFD methods, which can combine GIS and multiple technical methods to simulate dispersion accurately. In addition to application in nuclear engineering, the CFD method was also applied widely in chemical industrial research, Xu et al. (2020) and Tran et al. (2020) separately researched chemical gas dispersion in the atmosphere, and data on the chemical concentrations in different locations were acquired by their simulation.
Although CFD technology can simulate complicated dispersion behaviors in local-scale accurately, it is not suitable for larger-scale study because of the lack of meteorological parameterization. Thus, other methods were developed by researchers to assess the consequences of severe accident on a larger scale. For example, Korsakissok et al. (2013) presented atmospheric dispersion simulations of the Fukushima Nuclear Power Plant accident, using the Gaussian puff model pX, and Klein et al. (2021) studied the atmospheric transport of radionuclides from a hypothetical accident at the Sellafield reprocessing plant by the SNAP model. The Lagrangian particle tracking model was adopted by Ouyang et al. (2019) to simulate the atmospheric diffusion of radionuclides above the sea, and they analyzed the influence of sea absorption on dispersion behaviors. Baklanov et al. (2008) proposed a methodology for long-term evaluation of the atmospheric transport and deposition of harmful release under severe nuclear accidents, and deposition fields of 137Cs were obtained by dispersion modeling. As for the global-scale study, atmospheric and marine modeling has been carried out by Povinec et al. (2013) to predict the dispersion of radionuclides worldwide in order to assess the impact of the accident on the environment. All of these studies imply that consequence assessment for severe accident is apparently a large-scale issue; thus, meteorological processes can dramatically change transport and behaviors of radioactive materials released from the nuclear site, and 3D meteorological conditions should be considered in detail in model parameterization before dispersion modeling.
In addition to the consequence assessment study under severe nuclear accident, the transport study for neutron is also essential in application for nuclear technology; for instance, Wang et al. (2023) and Fu et al. (2022) researched the influence of complex terrain and rainfall on atmospheric transport behaviors for prompt neutron flux; thus, atmospheric transport for radioactive materials is of significance to study.
With the development of computer science and numerical simulation, the data assimilation technology has become increasingly essential within the weather forecast research field, which can incorporate dynamic observation data into the theoretical forecasting model to reduce error and realize optimal estimation. For instance, Zang et al. (2022) developed a 3DVAR data assimilation (DA) system and evaluated the impact of assimilating aerosol optical depth (AOD) measurements on aerosol analyses. In the research field for atmospheric pollution, underestimation for pollutant estimation was found via applying observational meteorological nudging (Jia et al., 2021). Johansson et al. (2022) presented an operational urban air quality modeling system ENFUSER considering data assimilation, and Carrassi et al. (2018) provided an up-to-date review of the data assimilation technology in the research field of geosciences. Obviously, the application for data assimilation has proven that the combination of model prediction and observation data provides a more insightful view on research in the future, than any of them independently.
In this paper, the influence of different meteorological conditions on numerical simulation for transport and deposition behaviors of radioactive materials under hypothetical severe accident was evaluated by the meso-scale model. Transport trajectories in different seasons were first simulated and clustered to investigate the general tendency for transport behaviors under different meteorological conditions. Furthermore, transport and simulation behaviors of radioactive materials for a hypothetical severe accident under different seasonal conditions were simulated by the 3D coupling model in the Eulerian scheme, and important parameters such as concentration, distribution, and deposition on the ground were acquired and analyzed in detail. Based on the work conducted, the FDDA (four dimensional data assimilation) method was adopted in meteorological simulation, and a typical case was selected to test the influence of FDDA on meteorological parameters and atmospheric transport behaviors. Finally, important meteorological parameters, concentration, and deposition fields were contrasted and evaluated with and without FDDA.
2 Analysis for transport trajectories
In order to analyze the influence of meteorological conditions on transport behaviors, hypothetical severe accident scenarios in the Daya Bay Nuclear Power Plant would be researched in detail in the following parts. The Daya Bay Nuclear Power Plant is located in the Dapeng Peninsula of Shenzhen City, which is close to Hong Kong. Because the Daya Bay Nuclear Power Plant is adjacent to the Guangdong–Hong Kong–Macao Greater Bay Area, nuclear safety issues in this power plant is of significance to research in detail (Cao et al., 2000).
Transport trajectories of radioactive materials in different months were simulated with the aid of clustering analysis by HYSPLIT (Draxler and Hess, 1997) to make an overall investigation on the transport behaviors under different meteorological conditions. Detailed information for HYSPLIT can be found on the website (Draxler and Rolph, 2006).
The possibility for each transport trajectory is already marked in Figure 2. It can be concluded from the figure that meteorological conditions are variable in spring; thus, there is no distinct rule and transport trajectories are erratic and unpredictable. Through the long summer of this area, the prevailing southwest monsoon in East Asia makes southerly wind blow easily, and transport trajectories own high possibilities to move northward. In autumn and winter, under the control of atmosphere circulation over East Asia, the northerly wind is predominant, and there is a good chance for atmospheric pollutants to move southward. In addition, there is also less probability for pollutants to move east.
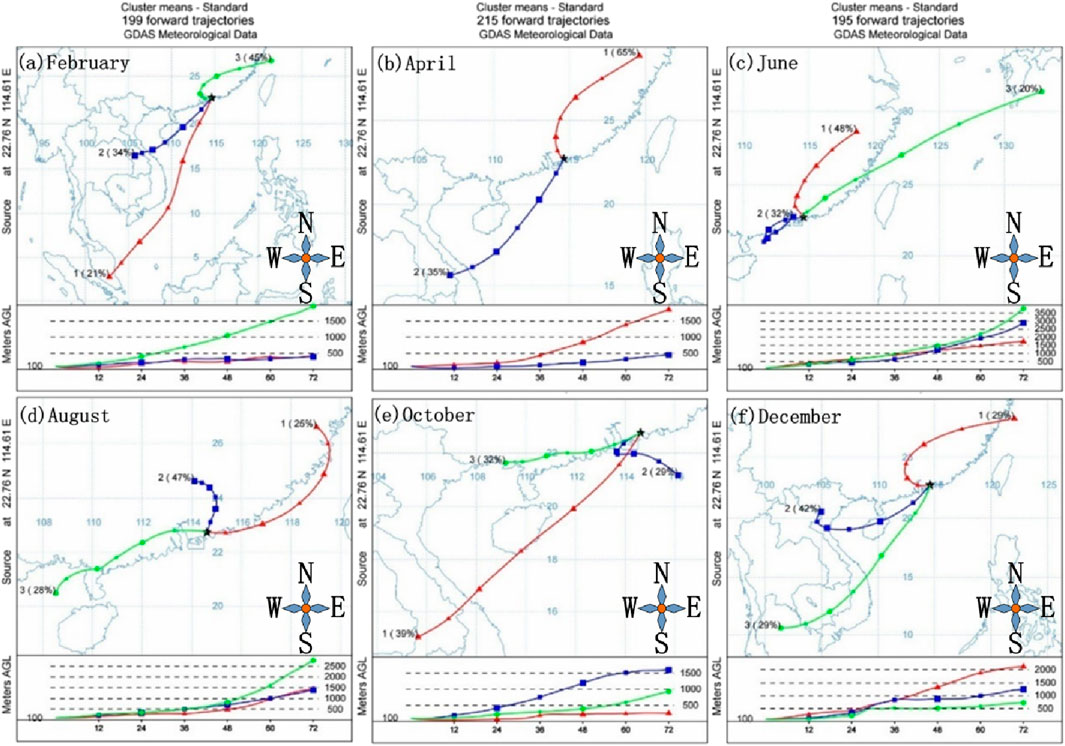
Figure 2. Transport trajectories in different months by clustering analysis. (A) Transport trajectories in February. (B) Transport trajectories in April. (C) Transport trajectories in June. (D) Transport trajectories in August. (E) Transport trajectories in October. (F) Transport trajectories in December.
In all, the transport behaviors of atmospheric pollutants apparently have obvious seasonality, and transport trajectories are strongly coupled with seasonal variation in this area, which can change the prevailing wind direction and further change the movement routine of pollutants. Therefore, it is essential to investigate the transport and deposition characteristics of radioactive materials under different meteorological conditions in detail, which would be discussed in the next section.
3 Meso-scale simulation for transport and deposition behaviors
In this section, meso-scale numerical analysis would be conducted deeply to study the coupling effects between meteorological variation and transport behaviors. The simulation method, meteorological analysis, and further material transport and deposition results would be discussed as follows.
3.1 Brief introduction for the simulation method
In the present research, the WRF-CMAQ coupling model is utilized to simulate meteorological processes and material transport behaviors numerically. The Weather Research and Forecasting Model (WRF) (Srivastava et al., 2015) is a typical meso-scale numerical model for simulating meteorological processes and provides the necessary meteorological parameters for driving transport and deposition simulation for atmospheric pollutants. The Community Multi-scale Air Quality (CMAQ) model (Ulas et al., 2010) is good at dealing with pollutants’ transport issues in the atmosphere. With the aid of the Meteorology–Chemistry Interface Processor (MCIP), meteorological parameters simulated by WRF can be easily received by CMAQ, which make it possible to apply the WRF-CMAQ coupling model in numerical analysis for atmospheric transport under the scenario of severe nuclear accident.
The conservative equation for the meso-scale model can be expressed in the Eulerian scheme (Hass et al., 1991) as
where
Based on this conservative equation, accidental release, meteorological parameters, and activity concentration for radioactive materials can be treated numerically, and Equation 1 can be solved by the finite element method (FEM).
Dry deposition flux
where
In addition to dry deposition, wet deposition is also a great contribution for the overall deposition effects. In the present meso-scale model, the calculation for wet deposition accounts for both in-cloud and below-cloud scavenging coefficients, which are influenced by factors such as precipitation rate and cloud water content. The resulting wet deposition flux is calculated as the product of the scavenging coefficient and the pollutant concentration (Binkowski and Roselle, 2003), integrated over the depth of the precipitation event. The expression for wet deposition flux
where
In order to apply such a method in consequence assessment, a sub-module for source release was developed (Ma et al., 2022) recently, which can parameterize source term data for accidental release and then generate an input file in network common data form (NetCDF) format, which can be identified by CMAQ for further numerical calculation. Thus, the 3D numerical simulation method in the Eulerian scheme was performed for severe accident.
As aforementioned, seasonal variation for meteorological conditions would influence the atmospheric movement and then change transport trajectories of radioactive materials. Thus, to investigate such effects in detail, four typical time durations in each season were selected for detailed meteorological simulation and further transport analysis. Reanalysis datasets (ds083.2) distributed by the National Center For Atmospheric Research in U.S. (NCEP) would be adopted as meteorological input files in WRF simulation.
As shown in Figure 3, three nested-grid domains would be adopted to enhance the resolution in the innermost region, which would be a simulation domain for detailed analysis.
In the WRF simulation, terrain effects were already parameterized in the model; thus, variation for elevation and underlying surface can be considered in simulation. Domain simulation parameters are shown in Table 1.
In addition to WRF simulation, source term characteristics under the hypothetical severe accident are also necessary for transport and deposition simulation. Here, 137Cs is mainly considered in source term analysis because it is released as an aerosol form and can cause long-term effects in the environment.
Accumulated radioactivity for 137Cs in the reactor core can be estimated by Equation 4 (USNRC, 1975):
where
Based on the estimation for accumulated radioactivity and release fraction under accidental scenario, the amount for accidental release can be calculated, which can be utilized as source term input parameters for transport and deposition simulation in CMAQ.
Parameters for accidental release are given in Table 2.
3.2 Meteorological analysis
With the aid of WRF simulation, meteorological parameterization can be performed by the model, and further meteorological processes can be simulated. In this section, simulation results for meteorological parameters under different seasonal conditions would be discussed.
Figure 4 shows the time-varying wind vectors in March in the simulation domain, which illustrates that southerly wind was predominant on the sea, and wind above the sea was smooth and steady. However, wind fields on the land own different characteristics. As shown in the figure, wind vectors varied apparently in different time points, both direction and magnitude were changing with time, which means terrain effects in the land area are strongly coupled with atmospheric movement; thus, wind fields on the land are changeable compared with wind above the sea. Because the nuclear power plant is located along the coast, wind vector distribution would be complicated and significantly influence transport and deposition behaviors of radioactive materials.
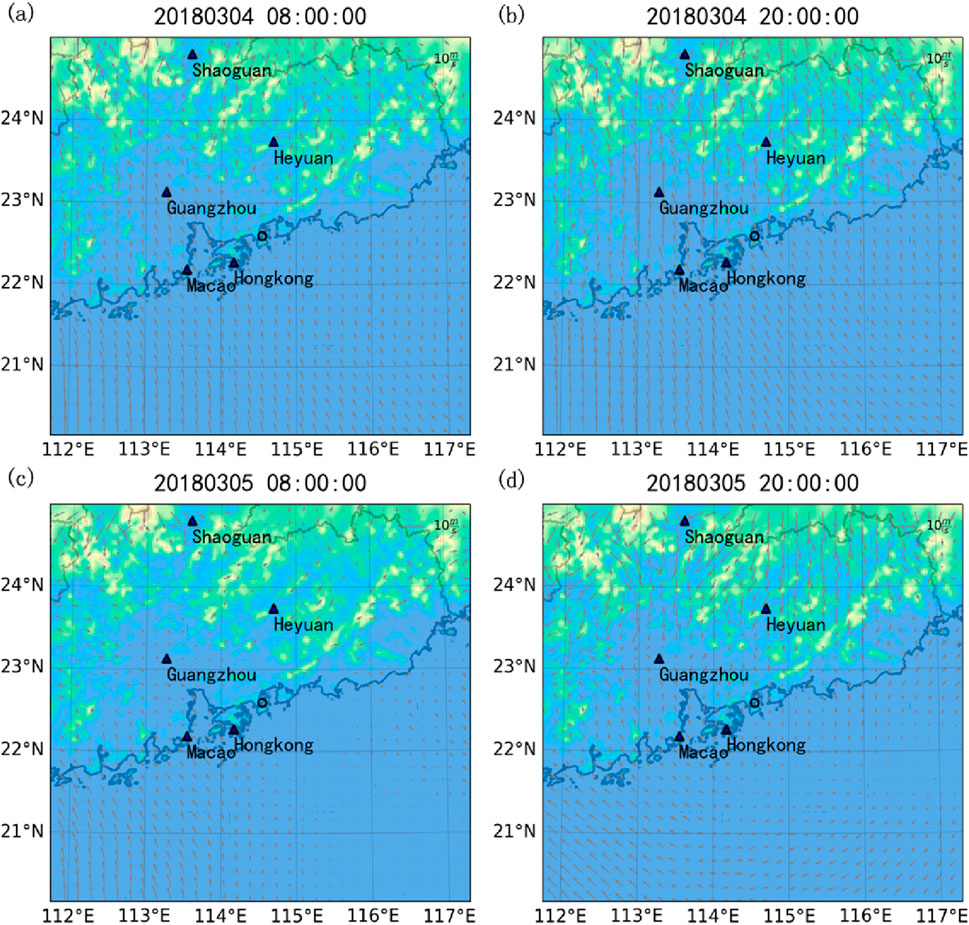
Figure 4. Wind vector distribution in different time points. (A) Wind vector distribution in 8:00, March 4th, 2018. (B) Wind vector distribution in 20:00, March 4th, 2018. (C) Wind vector distribution in 8:00, March 5th, 2018. (D) Wind vector distribution in 20:00, March, 5th, 2018.
Thus, to evaluate the influence of seasonal variation on meteorological processes, wind vector distributions in different months were simulated and compared with each other (Figure 5).
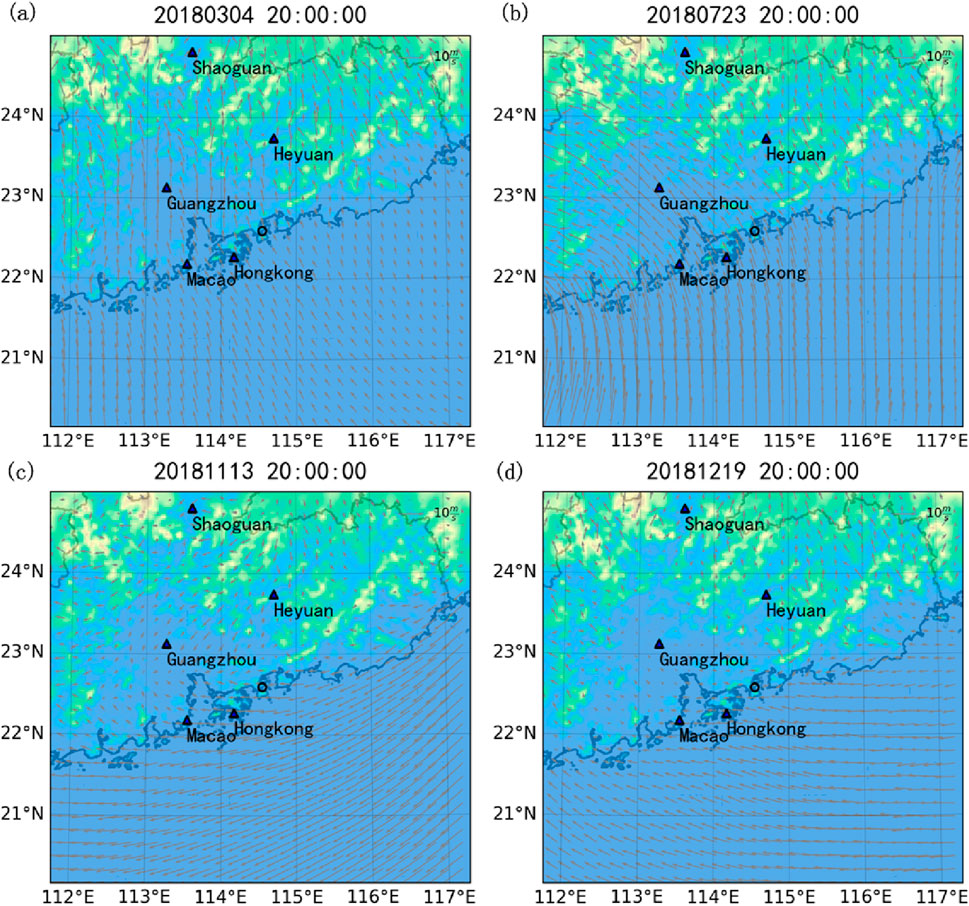
Figure 5. Wind vector distribution in different months. (A) Wind vector distribution in March. (B) Wind vector distribution in July. (C) Wind vector distribution in November. (D) Wind vector distribution in December.
Evidently, wind vector distribution shows significant differences in different seasons. In spring and summer, wind from southeast sea was prevailing in the simulated area. Distinctive sea breeze occurred, especially in summer; thus, wind speed can exceed 10 m/s in some parts of the region, which was obviously higher than that in other seasons. In autumn and winter, the prevailing wind direction was changed, and easterly wind was predominant in proximity to the nuclear power plant; thus, it can be predicted that radioactive pollutants own a high possibility to transport to the Greater Bay Area in these two periods.
In the location of the nuclear power plant, comparison of wind speed in the planet boundary layer at different heights is shown in Figure 6. Wind speeds in autumn and winter are obviously lower than that in the other two seasons, which implies stronger atmospheric transport abilities for radioactive materials in spring and summer.
It can be summarized from meteorological simulation that wind vector distributions in four seasons are different compared with each other and that, prevailing wind direction and wind speed are changeable in different seasons. In addition, simulation results imply significant differences for wind fields between land and sea; thus, atmospheric movement characteristics in the simulation domain are sensitive to seasonal variation, which would obviously change transport behaviors of radioactive materials.
Based on the meteorological simulation above, observation data from two static meteorological stations in Guangdong Province were selected, and for specific humidity and air temperature, simulation results and observation data were contrasted with each other, as shown in Figure 7.
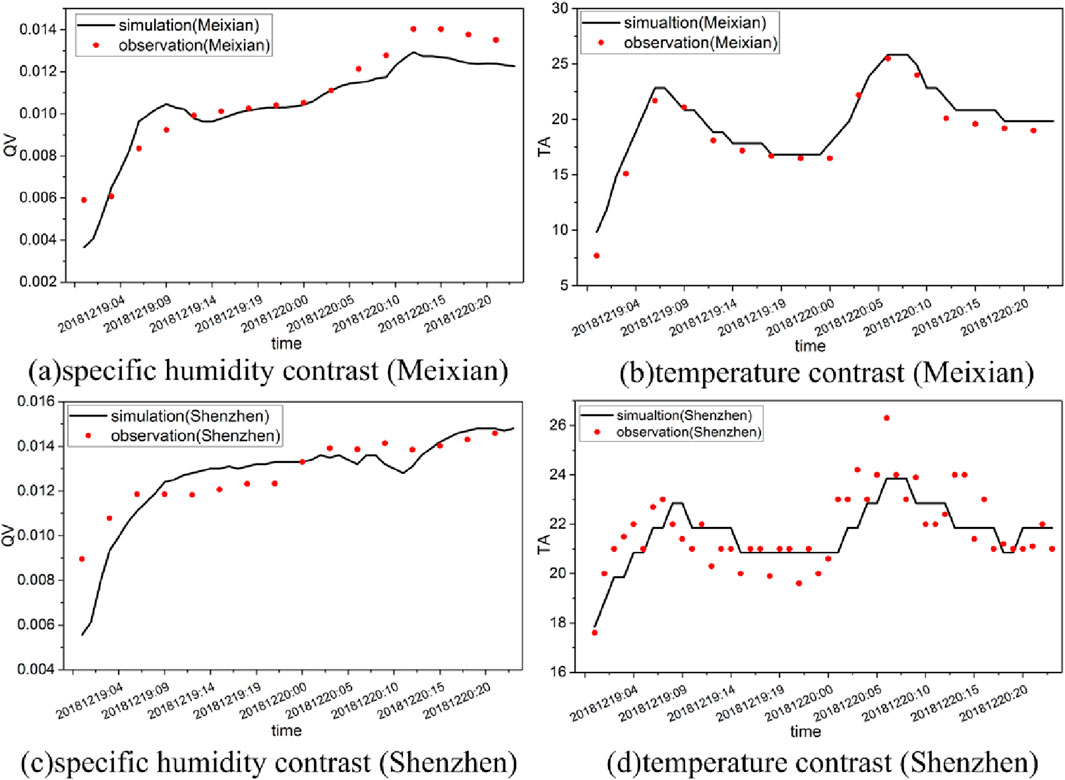
Figure 7. Comparison between simulation results and observation data. (A) Specific humidity contrast for Meixian. (B) Temperature contrast for Meixian. (C) Specific humidity contrast for Shenzhen. (D) Temperature contrast for Shenzhen.
As shown in the figure, meteorological simulation results for specific humidity and air temperature are in good accordance with observation data acquired from two static meteorological stations, which can verify the correctness and reliability for meteorological simulation results in the present study.
3.3 Analysis for transport and deposition behaviors
Atmospheric movement is strongly coupled with transport and deposition behaviors of radioactive pollutants. As wind vector distributions are sensitive to seasonal variation, transport and deposition behaviors would also show different characteristics, which should be analyzed in detail.
On the basis of WRF simulation results, meteorological parameters can be input into the CMAQ model to calculate transport and deposition behaviors for radioactive materials; thus, transport and deposition parameters can be simulated and corresponding characteristics can be analyzed. Transport and deposition characteristics in March are analyzed in detail in this section.
Figure 8 illustrates the concentration distribution of 137Cs after hypothetical accidental release in March. It is evident that simulation results for the concentration distribution are corresponding with wind vector distribution. Because southerly wind was predominated in this period, most of the radioactive pollutants would transport and diffuse northeastward of the nuclear power plant, and Heyuan City in Guangdong Province would be contaminated severely, in which the activity concentration can approach 100 Bq/m3 8 h after accidental release. With time, radioactive materials in the atmosphere would be diffused, and the activity concentration would decrease gradually.
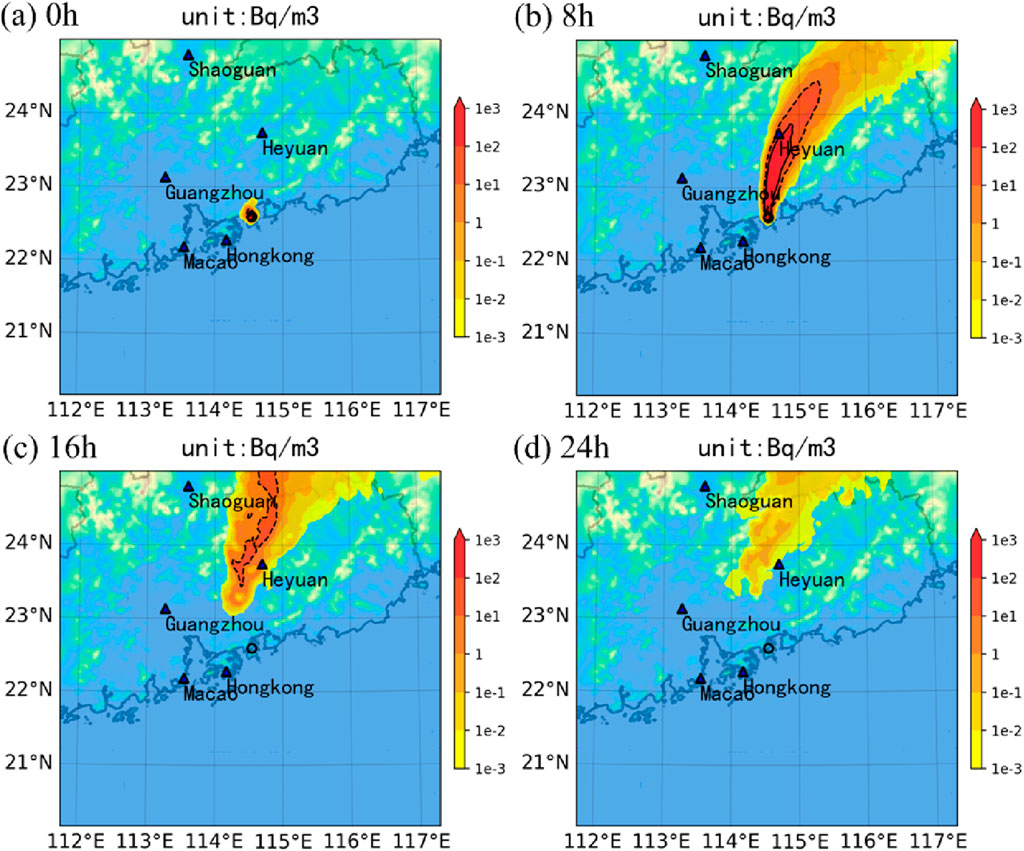
Figure 8. Activity concentration for 137Cs in different time points after accidental release. (A) 0 h after accidental release. (B) 8 h after accidental release. (C) 16 h after accidental release. (D) 24 h after accidental release.
Different from the activity concentration, deposition behaviors on the ground shows apparent accumulative effects, which is essential for the study of health effects; thus, deposition parameters should be discussed as follows.
As shown in Figure 9, most of the radioactive aerosols would deposit on the northeast area of the nuclear power plant, and the accumulative deposit on flux in Heyuan City can exceed 103 Bq/m2, which is in accordance with the concentration distribution for 137Cs. It can also be found from the figure that terrain characteristics would have an influence on the simulation results of deposition. As the border areas of Guangdong and Fujian are mountainous, contaminated regions in the northeast of the simulation domain show strong discrete characteristics; thus, radioactive materials would be easier to aggregate in valleys, as shown in the figure.
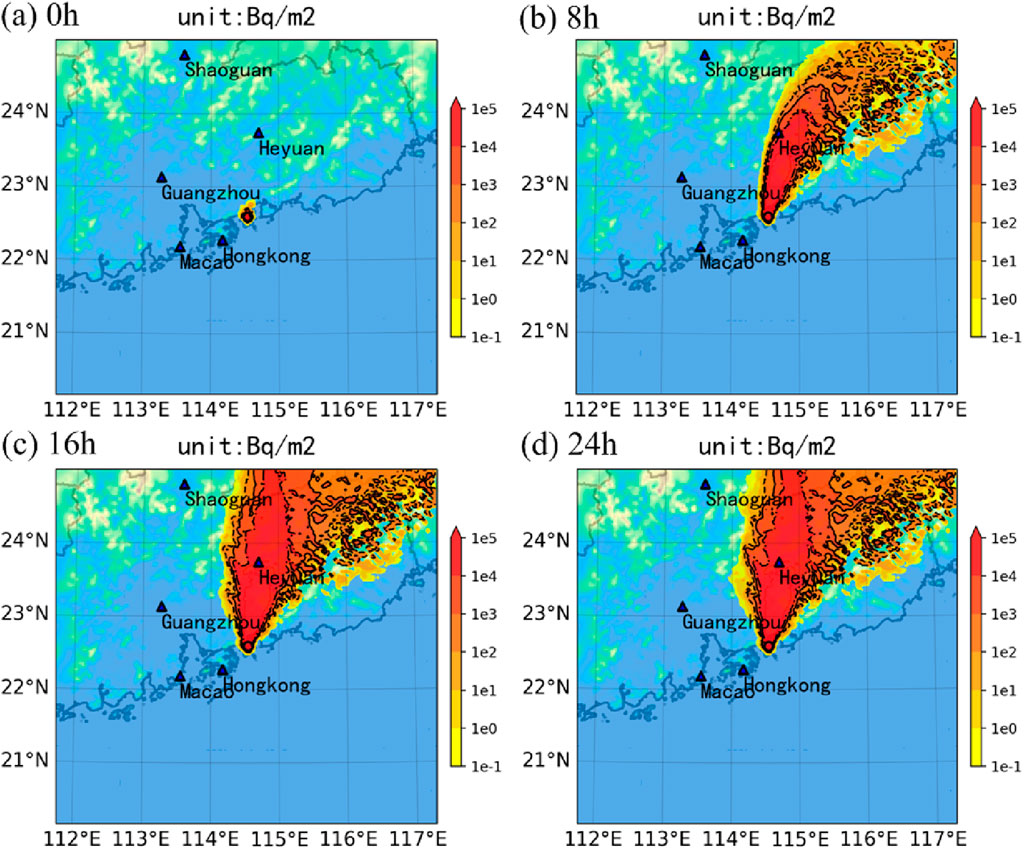
Figure 9. Accumulative deposition flux for 137Cs on the ground. (A) 0 h after accidental release. (B) 8 h after accidental release. (C) 16 h after accidental release. (D) 24 h after accidental release.
To analyze the local characteristics in Heyuan City quantitatively, time-varying plots for concentration and accumulative deposition flux are given in Figure 10. Based on calculation results, the maximum concentration for 137Cs can reach 180 Bq/m3, and accumulative deposition flux can exceed 1.6 × 105 Bq/m2 when becoming stable, which means the city would be contaminated heavily under such condition.
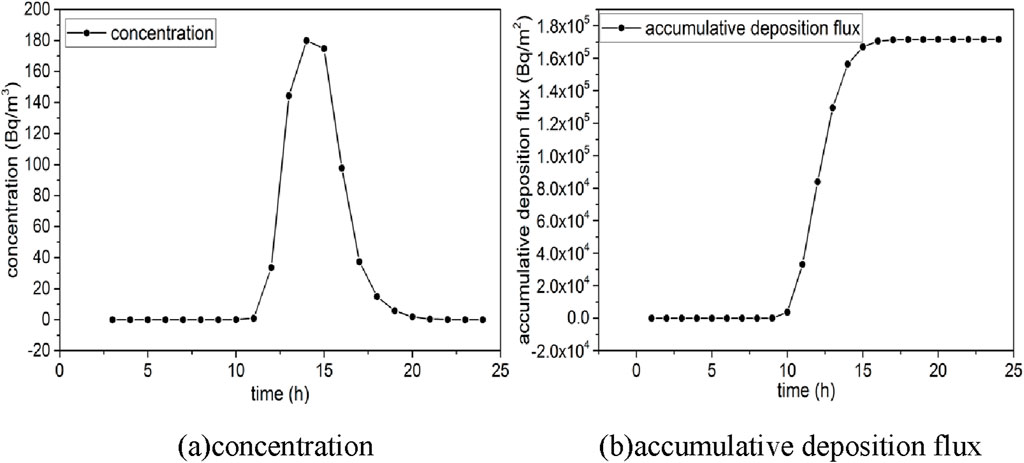
Figure 10. Time-varying plots for concentration and accumulative deposition flux. (A) Time-varying plot for concentration. (B) Time-varying plot for accumulative deposition flux.
3.4 Numerical study for transport and deposition behaviors under different meteorological conditions
To assess the influence of meteorological variation on transport and deposition characteristics, accumulative deposition flux contours in different seasons were first contrasted with each other (Figure 11).
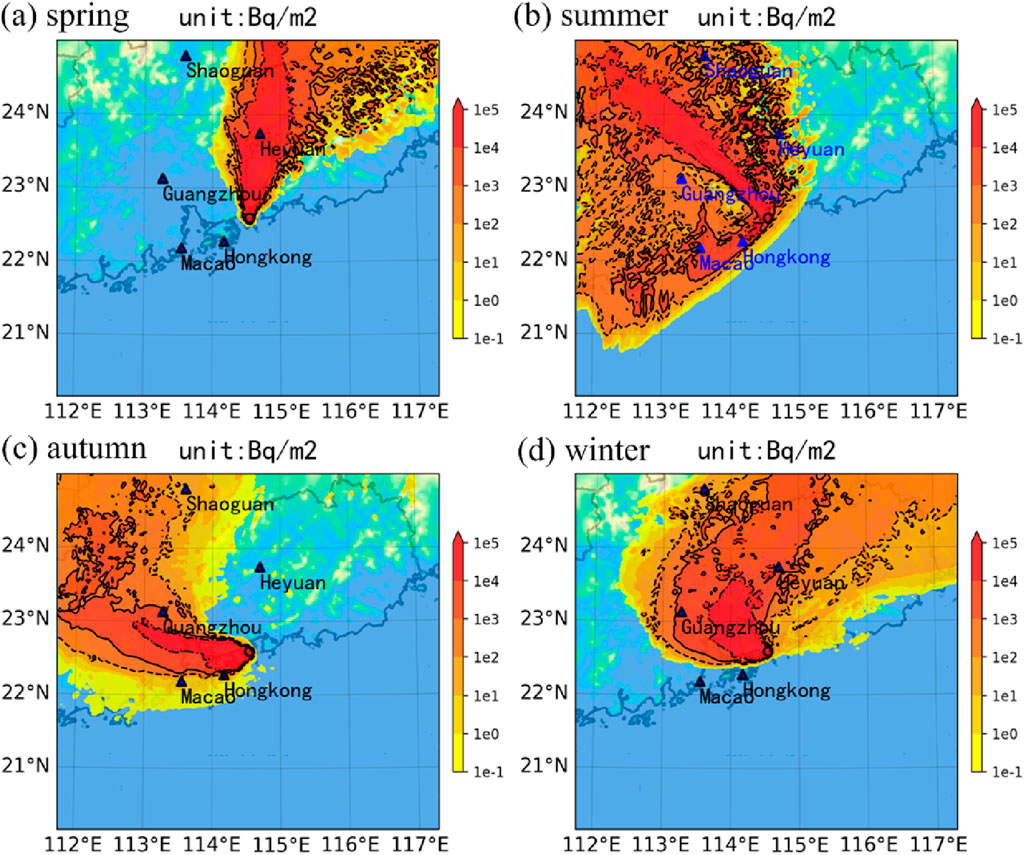
Figure 11. Accumulative deposition flux for 137Cs on the ground in different seasons (24 h after accidental release). (A) Accumulative deposition flux in spring. (B) Accumulative deposition flux in summer. (C) Accumulative deposition flux in autumn. (D) Accumulative deposition flux in winter.
Apparently, distribution for accumulative deposition flux in different seasons demonstrates that transport characteristics for radioactive aerosols can change dramatically under different meteorological conditions. Due to the reason that prevailing wind direction is varying in different months, the contaminated regions in the simulation domain would also be different. For simulation periods in spring and winter, contaminated regions are mainly located in the northeastern part of the domain, and Heyuan City would be contaminated apparently, as mentioned before. For simulation periods in summer and autumn, contaminated regions are located in the western part of the domain, and Guangzhou City would be contaminated, judging from the figure.
On the basis of accumulative deposition flux contours simulated by the model, accumulative deposition flux for a specific city (contaminated, as judged from the figure) in different seasons were compared with each other, as shown in Figure 12.
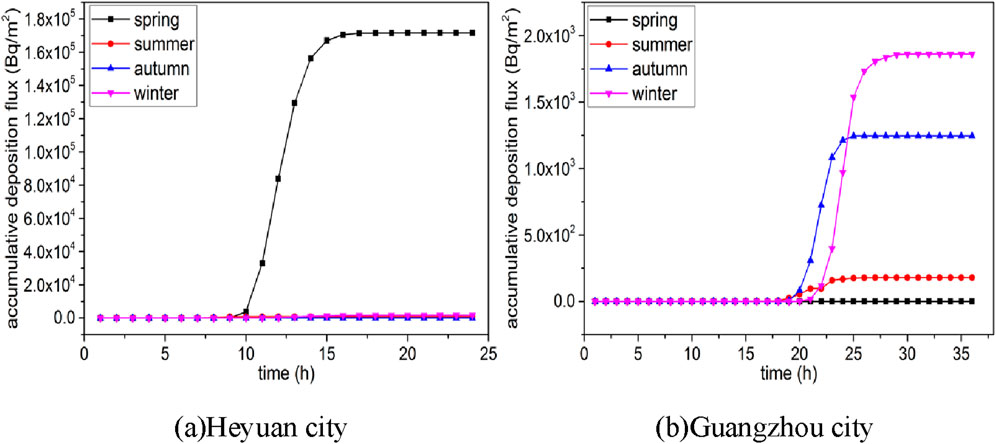
Figure 12. Time-varying plots for accumulative deposition flux in different seasons for two representative cities. (A) Time-varying plot for accumulative deposition flux in different seasons (Heyuan). (B) Time-varying plot for accumulative deposition flux in different seasons (Guangzhou).
Obviously, in terms of radioactive contamination, meteorological conditions in spring are the worst compared with other seasons for Heyuan City, in that accumulated deposition flux can reach the level of 105 Bq/m2 in spring, which is dramatically higher than the level of 103 Bq/m2 in the other two seasons. For Guangzhou City, although the difference of deposition flux is not as great as that of Heyuan City, the influence of meteorological variation on deposition flux can also be found in the figure. Different from Heyaun City, meteorological conditions in winter are the worst, and the corresponding accumulative deposition flux can exceed 2 × 103 Bq/m2, but in summer, accumulative deposition flux can be reduced more than an order of magnitude compared with the worst condition. All of these analyses reveal that different meteorological conditions can change transport and deposition characteristics of radioactive materials on a large scale and furthermore change radioactive contamination characteristics of some important regions; thus, further study in this research field is meaningful, and some preventive measures should be considered.
As shown in Figure 11, Guangzhou, Heyuan, Hong Kong, Macao, and Shaoguan are all contaminated under meteorological conditions in summer; thus, time-varying accumulative deposition flux for these five cities was compared (Figure 13). Obviously, Hong Kong and Macao are two severely contaminated cities; especially for Hong Kong, the accumulative deposition flux exceeded 6 × 103 Bq/m2. As for other three cities, the accumulative deposition flux would not exceed 103 Bq/m2.
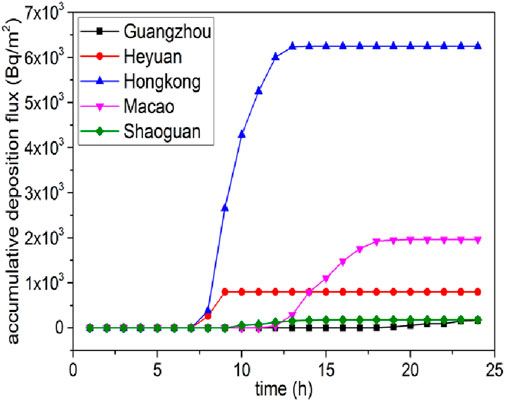
Figure 13. Time-varying plots for accumulative deposition flux under summer condition for five representative cities.
4 Sensitivity analysis for transport behaviors with and without FDDA
With the development of numerical analysis in atmospheric research, the data assimilation technology is becoming more and more essential during numerical simulation, which is able to integrate observing data into the numerical model. Nowadays, the FDDA technology is widely used in atmospheric research based upon meso-scale models, which can assimilate different kinds of meteorological variables into the simulating domain and modify neighboring space and time.
Data assimilation for model variable X (Pan et al., 2015) can be expressed by Equation 5.
where
Although FDDA is getting popular in meso-scale meteorological analysis, researchers hardly introduce this technology into consequence assessment under severe nuclear accident to modify the simulation results.
In this section, FDDA was utilized in radioactive contamination assessment, and WRF simulations for the same period (20,181,219 ∼ 20,181,220) were performed with and without FDDA to investigate the influence of such technology on simulation for meteorological variables and transport behaviors. Ds351.0 datasets and ds461.0 datasets distributed by NCEP were adopted and integrated into the model for data assimilation.
In order to analyze the influence of FDDA on simulation results, time-varying activity concentration and accumulative deposition flux with and without FDDA for two different cities were contrasted with each other, as shown in Figure 14.
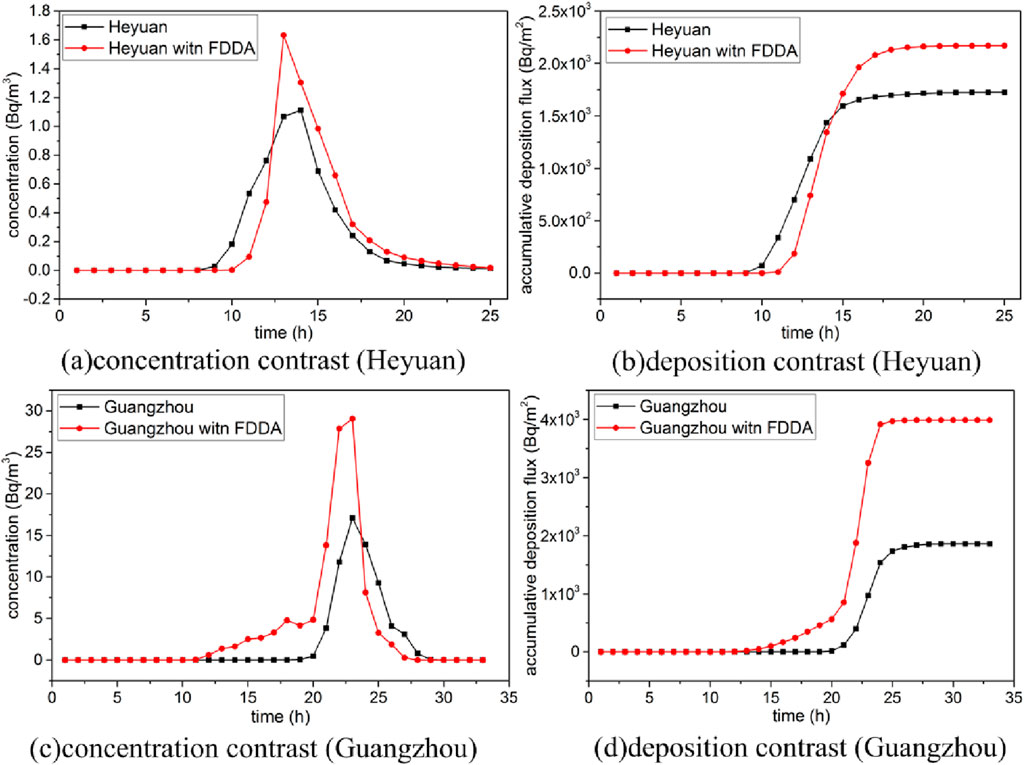
Figure 14. Comparison of simulation results with and without FDDA. (A) Concentration contrast for Heyuan. (B) Deposition contrast for Heyuan. (C) Concentration contrast for Guangzhou. (D) Deposition contrast for Guangzhou.
Evidently, the figure illustrates that the FDDA technology can obviously affect simulation results, not only maximum concentration and deposition flux but also approaching time for radioactive materials can be modified. For instance, when considering FDDA, the approaching time in Heyuan City was 9 h after accidental release. However, without FDDA, the approaching time in Heyuan City was delayed for an hour. As for Guangzhou, the approaching time was 12 h after accidental release when considering FDDA, but it can be delayed for 8 h without FDDA. In addition, the maximum activity concentration in Guangzhou was dramatically increased with FDDA. In addition to the activity concentration, accumulative deposition flux can also tell this kind of difference. As shown in the figure, accumulative deposition flux with FDDA is significantly higher than that without FDDA, which means under the calculation conditions discussed in the paper, lack of FDDA may underestimate the degree of radioactive contamination; thus, FDDA is necessary and should be considered in consequence assessment for severe nuclear accident.
5 Conclusion
In this paper, in order to investigate the influence of meteorological conditions on transport behaviors of radioactive materials quantitatively, numerical analysis for transport behaviors under different meteorological conditions was conducted. Including three-dimensional meteorological parameters (such as wind vectors) and activity concentration, different kinds of deposition flux were acquired and analyzed in detail. In addition, the FDDA technology was used evaluate its abilities in simulation result modification.
According to this research, some conclusions can be drawn as follows:
(1) Based on transport trajectories’ analysis, transport behaviors of atmospheric pollutants have obvious seasonality under severe nuclear accident, which means prevailing wind direction and movement routine of pollutants in different seasons can be changed apparently; thus, meteorological forecast should be taken into consideration in prediction of the atmospheric movement of radioactive pollutants.
(2) Meso-scale simulation results illustrate that radioactive contaminations are affected by terrain characteristics. It can be concluded from simulation results that distribution for radioactive materials would be obviously discrete in mountainous regions, especially in northeast of Guangdong Province, where radioactive contamination would aggregate in valleys.
(3) FDDA technology was applied in this work, which implies that such technology can significantly affect simulation results, even for the same simulation period. Thus, FDDA would be an essential method and should be analyzed in detail in consequence assessment for severe nuclear accident.
Moreover, the present study strongly implies that transport behavior of radioactive materials under severe nuclear accident is interdisciplinary among nuclear engineering/technology, atmospheric physics, and data assimilation technology; thus, it can be predicted that integration of these various discipline and new technologies would be more and more necessary in further research and application.
Data availability statement
The original contributions presented in the study are included in the article/supplementary material; further inquiries can be directed to the corresponding authors.
Author contributions
ZM: conceptualization, methodology, software, and writing–original draft. ZL: conceptualization, supervision, and writing–review and editing. XT: software, supervision, and writing–review and editing. LL: conceptualization and writing–review and editing. LB: methodology and writing–review and editing. CS: conceptualization, supervision, and writing–review and editing. DL: software, supervision, and writing–review and editing. BW: data curation, visualization, and writing–review and editing. YZ: visualization and writing–review and editing. PH: visualization and writing–review and editing. TM: data curation and writing–review and editing. LC: conceptualization, supervision, and writing–review and editing.
Funding
The author(s) declare that financial support was received for the research, authorship, and/or publication of this article. The paper is supported by National Natural Science Foundation of China (No. 12305191).
Conflict of interest
The authors declare that the research was conducted in the absence of any commercial or financial relationships that could be construed as a potential conflict of interest.
Publisher’s note
All claims expressed in this article are solely those of the authors and do not necessarily represent those of their affiliated organizations, or those of the publisher, the editors, and the reviewers. Any product that may be evaluated in this article, or claim that may be made by its manufacturer, is not guaranteed or endorsed by the publisher.
References
Baklanov, A., Sørensen, J. H., and Alexander, M. (2008). Methodology for probabilistic atmospheric studies using long-term dispersion modelling. Environ. Model Assess. 13, 541–552. doi:10.1007/s10666-007-9124-4
Barbero, R., Cuadra, D., Domingo, J., Iranzo, A., and Gallego, E. (2015). Investigation of the near-range dispersion of particles unexpectedly released from a nuclear power plant using CFD. Environ. Fluid Mech. 15, 67–83. doi:10.1007/s10652-014-9359-y
Binkowski, F. S., and Roselle, S. J. (2003). Models-3 community multiscale air quality (CMAQ) model aerosol component. 1. Model description. J. Geophys. Res. 108, 4183. doi:10.1029/2001jd001409
Brandt, J. H., and Christensen, L. M. F. (2002). Modelling transport and deposition of caesium and iodine from the Chernobyl accident using the DREAM model. Atmos. Chem. Phys. 2, 397–417. doi:10.5194/acp-2-397-2002
Bruno, S. (2007). A review of parameterizations for modelling dry deposition and scavenging of radionuclides. Atmos. Environ. 41, 2683–2698. doi:10.1016/j.atmosenv.2006.11.057
Cao, J. Z., Yeung, M. R., Wong, S. K., Ehrhardt, J., and Yu, K. (2000). Adaptation of COSYMA and assessment of accident consequences for Daya Bay nuclear power plant in China. J. Environ. Radioact. 48, 265–277. doi:10.1016/s0265-931x(99)00077-6
Carrassi, A., Bocquet, M., Bertino, L., and Evensen, G. (2018). Data assimilation in the geosciences: an overview of methods, issues, and perspectives. WIREs Clim. Change 9, e535. doi:10.1002/wcc.535
de Sampaio, P. A. B., Junior, M. A. G., and Lapa, C. M. F. (2008). A CFD approach to the atmospheric dispersion of radionuclides in the vicinity of NPPs. Nucl. Eng. Des. 238, 250–273. doi:10.1016/j.nucengdes.2007.05.009
Draxler, R. R., and Hess, G. D., (1997), Description of the HYSPLIT4 modeling system, NOAA technical memorandum ERL ARL-224.
Draxler, R. R., and Rolph, G. D. (2006). Hybrid single-particle Lagrangian integrated trajectory (HYSPLIT) model. Maryland: NOAA Air Resources Laboratory.
Fu, Y., Shang, P., Zuo, Y., Zhu, J., and Niu, S. (2022). Monte Carlo simulation of the effect of rainfall on neutron atmospheric transport. Mod. Appl. Phys. 13, 2.
Hass, H., Jakobs, H. J., Memmesheimer, M., Ebel, A., and Chang, J. S. (1991). “Simulation of a wet deposition case in Europe using the European Acid deposition model (EURAD),”. Air pollution modeling and its applications. Editors H. van Dop, and D. G. Steyn (New York: Plenum), 8, 205–213. doi:10.1007/978-1-4615-3720-5_17
Jia, M., Huang, X., Ding, K, Liu, Q., Zhou, D., and Ding, A. (2021). Impact of data assimilation and aerosol radiation interaction on Lagrangian particle dispersion modelling. Atmos. Environ. 247, 118179. doi:10.1016/j.atmosenv.2020.118179
Johansson, L., Karppinen, A., Kurppa, M., Kousa, A., Niemi, J. V., and Kukkonen, J. (2022). An operational urban air quality model ENFUSER, based on dispersion modelling and data assimilation. Environ. Model. and Softw. 156, 105460. doi:10.1016/j.envsoft.2022.105460
Kessler, G., Veser, A., Schlüter, F. H., Raskob, W., Landman, C., and Päsler-Sauer, J. (2014). The risks of nuclear energy technology. Sci. Policy Rep.
Klein, H., Bartnicki, J., Brown, J. E., Hosseini, A., Lind, O. C., Ytre-Eide, M. A., et al. (2021). Consequences for Norway from a hypothetical accident at the Sellafield reprocessing plant: atmospheric transport of radionuclides. J. Environ. Radioact. 237, 106703. doi:10.1016/j.jenvrad.2021.106703
Korsakissok, I., Mathieu, A., and Didier, D. (2013). Atmospheric dispersion and ground deposition induced by the Fukushima Nuclear Power Plant accident: a local-scale simulation and sensitivity study. Atmos. Environ. 70, 267–279. doi:10.1016/j.atmosenv.2013.01.002
Ma, Z., Ma, T., Wang, B., Li, D., Su, C., Liu, L., et al. (2022). Meso-scale numerical analysis for transport and deposition behaviors of radioactive aerosols under severe nuclear accident. Prog. Nucl. Energy 150, 104314. doi:10.1016/j.pnucene.2022.104314
Ma, Z., Tang, X., Ma, T., Liu, L., Wang, B., Li, D., et al. (2023). “Numerical study on transport and deposition characteristics of radioactive materials under severe accident based on meso-scale Eulerian coupling model,” in 30th international conference on nuclear engineering, may 21-26, 2023. Kyoto, Japan.
Mazzaro, L. J., Koo, E., Munoz-Esparza, D., Lundquist, J. K., and Linn, R. R. (2019). Random force perturbations: a new extension of the cell perturbation method for turbulence generation in multiscale atmospheric boundary layer simulations. J. Adv. Model. Earth Syst. 11, 2311–2329. doi:10.1029/2019MS001608
Ouyang, K., Chen, W., and He, Z. (2019). Analysis of the radioactive atmospheric dispersion induced by ship nuclear power plant severe accident. Ann. Nucl. Energy 127, 395–399. doi:10.1016/j.anucene.2018.12.020
Pan, L., Liu, Y., Liu, Y., Li, L., Jiang, Y., Cheng, W., et al. (2015). Impact of four-dimensional data assimilation (FDDA) on urban climate analysis. J. Adv. Model. Earth Syst. 7, 1997–2011. doi:10.1002/2015ms000487
Povinec, M. G., Holý, K., Holý, K., Hirose, K., Lujaniené, G., Nakano, M., et al. (2013). Dispersion of Fukushima radionuclides in the global atmosphere and the ocean. Appl. Radiat. Isotopes 81, 383–392. doi:10.1016/j.apradiso.2013.03.058
Srivastava, P. K., Islam, T., Gupta, M., George, P., and Dai, Q. (2015). WRF dynamical downscaling and bias correction schemes for NCEP estimated hydro-meteorological variables. Water Resour. Manag. 29, 2267–2284. doi:10.1007/s11269-015-0940-z
Tran, Vu, Ng, E. Y. K., and Skote, M. (2020). CFD simulation of dense gas dispersion in neutral atmospheric boundary layer with OpenFOAM. Meteorology Atmos. Phys. 132, 273–285. doi:10.1007/s00703-019-00689-2
Ulas, Im, Markakis, K., Unal, A., Kindap, T., Poupkou, A., Incecik, S., et al. (2010). Study of a winter PM episode in Istanbul using the high resolution WRF/CMAQ modeling system. Atmos. Environ. 44, 3085–3094. doi:10.1016/j.atmosenv.2010.05.036
USNRC (1975). Reactor safety study: an assessment of accident risks in US commercial nuclear power plants. NRC.
Wang, X., Wang, Y., Liao, Y., Wang, D., Du, F., Ma, Y., et al. (2020). 3D numerical simulation of radionuclides atmospheric dispersion in Chinese nuclear safety regulation. Asia-Pacific J. Chem. Eng. 15. doi:10.1002/apj.2492
Wang, X., Zhu, J., Zuo, Y., Niu, S., Shang, P., Yang, P., et al. (2023). Influence of complex terrain on prompt neutron radiation field of nuclear detonation. Mod. Appl. Phys. 14, 3.
Xu, G., Lim, A., Gopalan, H., Lou, J., and Poh, H. J. (2020). CFD simulation of chemical gas dispersion under atmospheric boundary conditions. Int. J. Comput. Methods 17 (5), 1940011. doi:10.1142/s0219876219400115
Keywords: nuclear accident, atmospheric transport, meteorology, radioactive materials, data assimilation
Citation: Ma Z, Li Z, Tang X, Liu L, Bao L, Su C, Li D, Wang B, Zhangsun Y, Hu P, Ma T and Chen L (2024) Numerical study for atmospheric transport of radioactive materials for hypothetical severe nuclear accident under different meteorological conditions. Front. Environ. Sci. 12:1455273. doi: 10.3389/fenvs.2024.1455273
Received: 26 June 2024; Accepted: 28 August 2024;
Published: 19 September 2024.
Edited by:
Chen Siyu, Lanzhou University, ChinaReviewed by:
Ioannis Konstantinos Christodoulakis, American College of Greece, GreeceMirjana B. Radenkovic, University of Belgrade, Serbia
Copyright © 2024 Ma, Li, Tang, Liu, Bao, Su, Li, Wang, Zhangsun, Hu, Ma and Chen. This is an open-access article distributed under the terms of the Creative Commons Attribution License (CC BY). The use, distribution or reproduction in other forums is permitted, provided the original author(s) and the copyright owner(s) are credited and that the original publication in this journal is cited, in accordance with accepted academic practice. No use, distribution or reproduction is permitted which does not comply with these terms.
*Correspondence: Zhenhui Ma, bWF6aGVuaHVpQG5pbnQuYWMuY24=; Lixin Chen, Y2hlbmxpeGluQG5pbnQuYWMuY24=