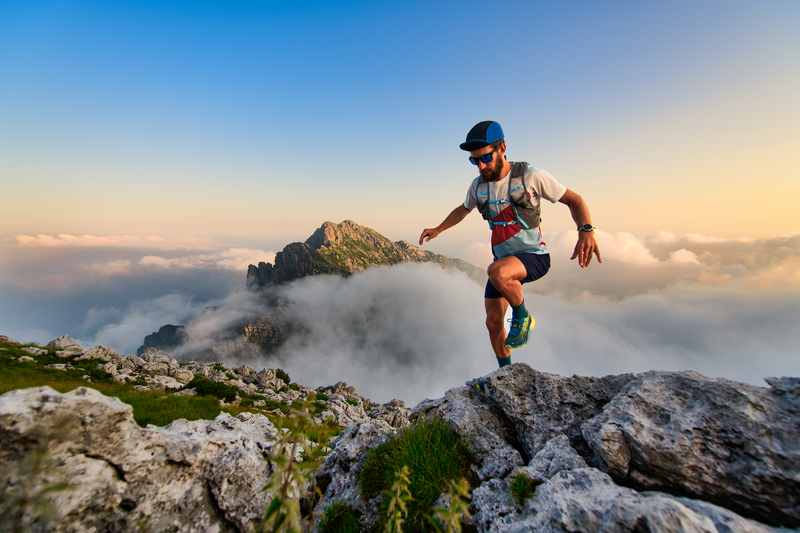
95% of researchers rate our articles as excellent or good
Learn more about the work of our research integrity team to safeguard the quality of each article we publish.
Find out more
ORIGINAL RESEARCH article
Front. Environ. Sci. , 26 August 2024
Sec. Toxicology, Pollution and the Environment
Volume 12 - 2024 | https://doi.org/10.3389/fenvs.2024.1439573
We examined the ecological and toxicological implications of the microplastic, Cyanox®53, found in sediments and varnish clams across seven beaches in Burrard Inlet, British Columbia (BC). Using the simulation models embedded within Estimation Programs Interface (EPI) Suite™, the potential persistence, bioaccumulation, and toxicity of Cyanox®53 was assessed to evaluate the risk to varnish clams foraging on sediment containing this contaminant. Moreover, we used a bioenergetic model, based on the blue-listed surf scoter species, to estimate the risk of daily ingestion of Cyanox®53 per body weight in overwintering seabirds. Our findings indicate that varnish clams collected from Burrard Inlet accumulate on average 0.46 particles of Cyanox®53/clam, and based on bioenergetic modeling, results in surf scoters potentially consuming 78 (for males) to 83 (for females) pieces of Cyanox®53 daily from foraged varnish clams. EPI Suite™ predicted Cyanox®53 to be persistent, however, unlikely to bioaccumulate as a “traditional” chemical. Furthermore, the estimation of potential acute and chronic toxicity of Cyanox®53 to aquatic organism surrogates, such as fish, Daphnia magna, and green algae, was inconclusive due to model variability and limitations within EPI Suite™. To fully understand the potential risks of Cyanox®53 further investigation is warranted.
Microplastics (MPs) are generally characterized as any plastic particle ranging from 1 μm to 5 mm in diameter, and can present in all manners of shapes, sizes, and colors in the aquatic environment (Thompson, 2015; Hermabessiere et al., 2017; Crawford and Quinn, 2019; Bendell et al., 2020; Bermúdez and Swarzenski, 2021). The origin in which MPs can arise from also varies, with some emerging from weathered macroplastics (>1 cm) that have undergone fragmentation (e.g., fibres from fishing nets, synthetic clothing, or tire remnants), and others coming from manufactured products (e.g., preproduction plastic resin pellets, industrial abrasives, or microbeads in personal care products) that have entered the waterways from plastic litter, treated wastewater release, or sewer overflow (Baztan et al., 2017; da Costa et al., 2017; Hermabessiere et al., 2017; Crawford and Quinn, 2019; Department of Fisheries and Oceans Canada, 2021). Regardless of the source, albeit noteworthy, MPs are now acknowledged as one of the most pervasive and formidable threats ever to endanger the marine environment (Crawford and Quinn, 2019). Whether it is due to not possessing a feeding mechanism capable of discriminating between prey and anthropogenic material, mistaking the MP as planktonic prey, or from ingesting prey containing MPs (i.e., trophic transfer), many aquatic organisms inadvertently ingest MPs as food (Moore, 2008; Lusher, 2015; Gallo et al., 2018; Luan et al., 2019). In some species, like shore crabs (Carcinus maenas) and blue mussels (Mytilus edulis L.), MPs can also be drawn into the body via gill cavity inhalation (Browne et al., 2008; van Moos et al., 2012; Watts et al., 2014; Lusher, 2015; Miller et al., 2017; Gallo et al., 2018). MP uptake has been observed in over 300 marine species, including zooplankton, benthic invertebrates, fish, sea reptiles, seabirds, and marine mammals (Gallo et al., 2018; Luan et al., 2019; Savoca et al., 2021). Without enzymatic pathways specific to synthetic polymer break down, the assimilation of MPs can inflict a variety of physical effects (depending on the size and shape) in most aquatic organisms, including gut obstruction and ulcerative lesions; weight loss from decreased food and nutrient intake; reduce respiration rates from MP entrapment in the gills; interfere with other tissues or organs (i.e., lysosomal, circulatory, hemolymph) via translocation; impact development, metabolic parameters, or cellular function; impair mobility, brain function and reproductive output; or reduce life expectancy (Browne et al., 2008; Teuten et al., 2009; Andrady, 2011; Besseling et al., 2013; Lambert et al., 2013; Wright et al., 2013b; da Costa et al., 2017; Law, 2017; Nelms et al., 2019; Hale et al., 2020).
MPs also pose the additional risk of exposing aquatic organisms to toxic chemicals associated with the plastic (Koelmans et al., 2014; Espinosa et al., 2016; Adam et al., 2019). Indeed, while plastic polymers are typically considered biochemically inert due to their large molecular size, they often contain hazardous non-polymeric substances (i.e., unreacted residual monomers, oligomers, catalyst remnants, polymerization solvents, and various chemical additives) deliberately added during processing to enhance the properties of the plastic and prevent ‘aging’ (Lithner et al., 2011a; Olabisi and Adewale, 2016; Gallo et al., 2018). In most instances, these substances are of low molecular weight and are either weakly or not completely bound to the polymer matrix (Organisation for Economic Co-operation and Development OECD Website, 2004; Lithner et al., 2011b). This increases their likelihood of leaching out of the plastic and into the surrounding medium until the appropriate octanol/water partition coefficient (Kow) is reached (Andrady, 2011). Moreover, it is rare and quite unlikely for organisms to be exposed to only one chemical in isolation, raising the prospect of co-contaminants interacting with one another and potentially inducing additive or synergistic effects (Rochman, 2015; Baztan et al., 2017). Persistent organic pollutants (POPs), such as alkylbenzenes, chlorinated hydrocarbons, polycyclic aromatic hydrocarbons (PAHs) and polychlorinated biphenyls, as well as inorganic contaminants like silver, titanium dioxide nanoparticles, barium, sulphur, and zinc from the environment have been known to absorb or concentrate in MPs (Teuten et al., 2009; Andrady, 2011; Fries et al., 2013; Khan et al., 2015). Evidence of non-persistent pollutants, such as commercially important plasticizers (e.g., dibutyl phthalate), or trace monomers like bisphenol A (BPA) and alkylphenols, have also been found at greater concentrations in MPs than in seawater or sediments, and are known to readily leach out and disrupt endocrine functionality of exposed organisms (Carlisle et al., 2009; ECHA, 2014; ECCC, 2018; Gallo et al., 2018). Even some synthetic phenolic antioxidants and related transformation products, that are normally used to impede oxidative reactions and extend plastic shelf-life, can exert hepatic toxicity, endocrine disrupting effects, and carcinogenicity if they were to release from the polymer matrix and become bioavailable within an organism (Lambert et al., 2013; da Costa et al., 2017; Liu and Mabury, 2020). Because of this, ‘plastic microbeads’ and ‘plastic manufactured items’ have been added to the list of toxic substances under Schedule 1 of the Canadian Environmental Protection Act (CEPA), and the composition and properties of most plastic additives have been revealed to public consumers (Espinosa et al., 2016; ECCC, 2020; ECCC, 2021; Plastic Soup Foundation, 2021). However, this is not the case for all as gaps in knowledge still remain for some compounds. For case in a point, and the focus of this study, limited information exists on the additive, Cyanox®53.
Cyanox®53 is chemically characterized as a white powdered alkylated bisphenol derivative, more specifically a primary (phenolic) antioxidant, with a specific gravity density of 1.74 (van Alphen and van Turnhout, 1973; McEntee, 1990; AccuStandard, 2018; Wang et al., 2019; Wiley, 2021). The additive was originally developed by Cytec Industries Inc. for the intended use of enhancing the stability of polymers (i.e., PE, PP, PS, and ABS) by providing protection against thermo-oxidative degradation that would otherwise weaken structural integrity or cause discoloration during thermal processing (Bolgar et al., 2016; Bendell et al., 2020; Solvay, 2020). Although the thermal stabilizer has since been discontinued and is no longer available for purchase in the public domain, the integration of Cyanox®53 has been documented in different products including rubbers, PE wiring and cable resins, and even in the packaging of feminine hygiene products (e.g., wrapper in organic cotton tampons) (Flick, 2001; Bolgar et al., 2016; Greenpeace Research Laboratories, 2019). Beyond these facts, however, little is known about Cyanox®53 as experimental data and model-based predictions are lacking. In fact, while information on other Cyanox® variants (e.g., Cyanox®2246, Cyanox®425, and Cyanox®1790) has been disclosed in an assortment of documents, literature regarding the ecological and/or toxicological significance of Cyanox®53 remains scarce. This is likely because particles like Cyanox®53 tend to get associated with the plastic polymers during MP surveys, rather than be classified as a true plastic product. As a result, their occurrence in the environment more often would go unreported. In the few times Cyanox®53 has been recorded in the environment, the antioxidant has been found to be disassociated from its inert carrier and abnormally presented as a white fibrous particle. For example, following a MP composition survey at inner Kongsfjord, Svalbard, the Norwegian Geotechnical Institute in collaboration with the Geographical Survey of Norway recovered white fragments of Cyanox®53, at a frequency 27%–64% of total MPs, in beach surface sediments at two sampling sites (Knutsen et al., 2019). Bendell et al. (2020) found fibrous particles of Cyanox®53, in addition to a high volume of MP fragments and spheres, in the digestive tracts of 26 varnish clams (Nuttallia obscurata) during an MP biomonitoring survey in Burrard Inlet, British Columbia (BC). Similarly, an investigation conducted by Achiluzzi (2022) recovered Cyanox®53 particle concentrations in varnish clams from False Creek, Vancouver, BC. Based on their findings, Bendell et al. (2020) and Achiluzzi (2022) proposed that Burrard Inlet, BC could be geographically an important source of Cyanox®53 from sediment to higher trophic levels. Still, further investigation into the ecological and toxicological implications of Cyanox®53 is needed.
At present, assessment of the toxicity of MPs poses quite a challenge to researchers as they not only act as a physical obstruction but also as a dynamic vector and reservoir of inherent additives and anthropogenic contaminants capable of inflicting physiological and toxicological damage to organisms. Traditionally, in vivo experiments were used by scientists as the fundamental risk assessment approach in identifying the toxicological mechanism and structure–activity relationship for specific chemicals (Hayes and Kruger, 2014). However, within recent decades the issue of whole-animal toxicity testing has become more pressing and contentious as concerns over the welfare and misuse of animals have been raised (Goldberg and Frazier, 1989; Hayes and Kruger, 2014). Even global regulations, including European Chemical Agency, REACH initiative, U.S. Toxic Substances Control Act, and the Canadian Environmental Protection Act, that once mandated in vivo toxicity testing, now encourage an increased reliance on in silico approaches to predict the intrinsic activity and potential absorption, distribution, metabolism and excretion characteristics of chemicals (Madden et al., 2020; Zhou et al., 2021). As such, less costly and non-invasive approaches, such as Quantitative Structure-Activity Relationship (QSAR) modeling, have been utilized more frequently over traditional in vivo experiments in the identification of physiochemical properties, and toxicological mechanism and structure–activity relationship for specific chemicals (Lapenna et al., 2010; Hayes and Kruger, 2014; OECD, 2022a; OECD, 2022b). The application of bioenergetic models have also provided a valuable tool for identifying factors influencing the feeding and growth of aquatic organisms, as well as for quantifying their consumption under varying environmental stressors and ecological conditions (Enders and Scruton, 2006). Here, we use a similar approach to gain some insight into the potential toxicity of Cyanox®53 and possible risk if it were to leach from its solid form.
In this present study, we examined the ecological and toxicological implications of Cyanox®53 concentrations found in sediments and varnish clams (N. obscurata) from Burrard Inlet, BC. Our objectives were as follows: i) detect the presence of Cyanox®53 within intertidal surface sediment and adult varnish clams across seven beaches in Burrard Inlet, B.C.; ii) employ attenuated total reflectance-Fourier transform infrared (ATR-FTIR), electrospray ionization-liquid chromatography/mass spectrometry (ESI-LC/MS), and proton nuclear magnetic resonance (1H-NMR) to propose a chemical structure for Cyanox®53; and from this structure, iii) estimate its environmental fate and potential toxicity via Estimation Programs Interface (EPI) Suite™, and the risk of daily ingestion of Cyanox®53 per body weight in overwintering seabirds using a bioenergetic model, with the surf scoter (M. perspicillata) as a representative species. The varnish clam was selected as it has shown to be an opportunistic feeder and effective biomonitor of MP pollution within sediments (Bendell et al., 2020). It is also well-established in the southern coastal regions of BC and represents a key diet item of the blue-listed surf scoter species, therefore making it an ecologically relevant choice for risk analysis (Gillespie et al., 1999; Gillespie et al., 2001; Palm et al., 2012; Brietzke and Starzomski, 2013; Chan and Bendell, 2013; Bendell et al., 2020). We predicted that Cyanox®53 would be the most abundant near the First Narrows strait (mouth of outer and inner harbor) where the majority of the Port of Vancouver is centralized. Secondly, we hypothesized that akin to other bisphenol-related compounds, Cyanox®53 may possess some degree of toxicity towards aquatic organisms and/or seabirds. As attention is often solely directed towards more commonly known MP polymers (e.g., polyethylene, polypropylene, and polyvinyl chloride), we aimed to gain a better understanding of the potential risk of Cyanox®53 to varnish clams and surf scoters given their important roles within the marine ecosystem.
Seven coastal sites in Burrard Inlet, BC (49.3174° N, 123.1913° W) were selected for sampling. These included Spanish Banks Beach (SB), Jericho Beach (J), English Bay Beach (EB), Third Beach (TB), Ambleside Park Beach (AP), Cates Park Beach (CP), and Barnet Marine Park Beach (BMP) (Figure 1) (Google Earth Pro, 2024). Descriptions, impact levels, substrate types, and GPS coordinates of each site are provided in the Supplementary Material.
Figure 1. Locations of the seven designated sampling sites in Burrard Inlet, BC. Yellow markers indicate sampling at AP, BMP, CP, EB, J, SB, and TB.
Sampling was conducted in late May to early June during a period of maximum low tides. Approximately 3.5 kg of surficial sediment was collected via ‘grab sampling’ technique at a depth of 10 cm from each site and placed into a labelled plastic bucket and sealed for prevention of contamination. Following collection, sediment samples were transported back to the laboratory and sieved separately for particles <5 mm into a pre-weighed aluminum tray using CE Tyler Canadian Standard 4.75 mm sieve. Newly sieved sediment was then subdivided into three 1 kg samples per study site. Each subsample was homogenized before being placed into a Precision® Gravity Convection Incubator at 60°C for 48 h or until completely dried. Once dried, samples were set aside for tagging and separation.
As described in Maes et al. (2017), a stock solution of zinc chloride (ZnCl2) (granular ACS reagent with a purity of >97%) at a density of 1.38 g/mL was prepared gravimetrically at room temperature by combining 420 g ZnCl2 with 700 mL analytical-grade water. Nile red dye (≥98.0% purity) stock solution in acetone (≥99.5% purity) was prepared to a final concentration of 10 μg/mL in a tinted vial and stored in a dark refrigerator at 4°C to prevent chemical degradation and microbial growth until tagging was conducted. Approximately 20 g of dry sediment was suspended in 30 mL ZnCl2 solution and 2 mL Nile red solution within a 50 mL centrifuge tube. Samples were shaken for 30 min on a Precision® Scientific Dubnoff Metabolic Shaking Incubator at 100 rpm. These steps of solution prep and staining were repeated for all subsamples.
Particles from sediment samples were separated by centrifuge based on the procedures laid out in Maes et al. (2017), with some minor modifications. Each centrifuge tube filled with the sediment mixture was centrifuged in a Beckman Allegra 64R centrifuge at 3900 g for 8 min with a braking speed of 9. The supernatant containing the stained particles was collected using a 5 mL glass Pasteur pipette and decanted through a Whatman™ 1001–090 Grade 1, Pore Size, 9 µm qualitative cellulose nitrate filter paper under vacuum filtration. This procedure of density suspension was repeated for each subsample. Dried filter papers were placed into Petri dishes and sealed with Parafilm® M all-purpose tape until inspection.
Twenty-five adult varnish clams varying in size were collected at each site in late May to early June (Permit # XMCFR 16 2020) from a depth of approximately 10 cm in the mid to high intertidal zones (N = 175). All collected bivalves were secured with a thick rubber band around their shell to prevent the loss of any particle contents and then placed into their respective site labelled Ziploc® bag for ease of transportation. At the laboratory, biometric data of internal tissue weight, shell dimensions (length, height, width) and weight of each bivalve was measured, and a corresponding condition indices value was determined using Equation 1 (Benali et al., 2015). Until needed, the bivalves were frozen at −4°C to preserve their internal tissues.
Several procedures have been recommended for tissue digestion in literature; however, after review, the ones suggested by Thiele et al. (2019) were adopted. Whole-organism tissues were removed from each bivalve shell using sterilized stainless-steel tools, and immediately placed into separate 100 mL glass Erlenmeyer flasks. No pooling of the samples was conducted to allow for the determination of particles per individual. Tissue digestion was carried out by incubating the removed tissue samples in freshly prepared 10% potassium hydroxide (KOH) (4 times the tissue volume) for 48 h at 60°C within a Precision® Gravity Convection Incubator. After 24 h, 2.5 mL of Rit Dye More® was added into the digestion mixture to stain any synthetic polymers purple for identification. A face wash containing polyethylene (PE) (Neutrogena® Oil-Free Acne Wash) was used as a positive control. Following incubation, each sample was vacuum filtered through a Whatman™ 1001–090 Grade 1, Pore Size, 9 µm qualitative cellulose nitrate filter paper and rinsed with 30% hydrogen peroxide (H2O2) to remove any biofilm or fat membrane remnants. Once dried, filter papers were placed into Petri dishes and sealed with Parafilm® M all-purpose tape until inspection.
Altogether, 21 replicates of sediment and 175 bivalves were evaluated. Filtrates extracted from bivalve samples were visually observed through a digital dissection microscope at ×60 magnification. As suggested in Maes et al. (2017), fluorescent tagged filtrates found in the sediment samples were visually inspected using an orange filter digital dissection microscope (×60 magnification) under blue excitation (420–495 nm). Any white fragmented/frayed particles suspected of being Cyanox®53 were submitted to ATR-FTIR spectroscopy for verification (Figure 2). Infrared spectra between 550 cm-1 and 4000 cm-1 were measured on a PerkinElmer FTIR Microscope Spotlight 200i, with the focus set to a 10 μm area of the particle using a diamond crystal. Before examination, each filter paper was sprayed with methanol (≥99.5% purity) to prevent loss of particles. Each ATR-FTIR spectra was assigned a polymer identify based on “best match” according to molecular bond vibrations and a “confidence” score ranging from 0% to 100%; a value of ≥70% was deemed as an acceptable level of confidence to account for weathering. Obtained ATR-FTIR spectra were compared with accessible online spectral databases (e.g., SpectraBase™). If a positively matched Cyanox®53 ATR-FTIR spectra was recovered (≥70% confidence score), the sample was reserved for follow-up analyses.
Total numbers of recovered Cyanox®53 and MP per bivalve and sediment sample were converted to number of particles per g of wet tissue weight (particles/g ww) and number of particles per kg of sediment dry weight (particles/kg dw), respectively. Biota-sediment accumulation factor (BSAF) ratios were calculated using an adapted formula from Alava (2021) to assess the bioaccumulation potentials of mean Cyanox®53 and/or MP concentrations in the bivalve tissue against that deposited in the sediments on a kg weight basis for each site (Equation 2). If the BSAF ratio was >1, bioaccumulation was deemed probable, but if the BSAF ratio was ≤1, then a lack of bioaccumulation was concluded.
Numerous studies have examined the leaching of additives from plastic pollution, with the most commonly used method being single-batch equilibrium leaching (Bridson et al., 2021). Adopting a similar approach, single-batch equilibrium leaching was carried out by placing 1 mg of the recovered Cyanox®53 and Cyanox®53-polyvinylidene fluoride (PVDF) particles into two separate 1 mL Pyrex® glass test tubes and immersing them in 200 μL of 99.9% deuterated dimethyl sulfoxide (DMSO-d6) (supplied by the Chemistry Department at Simon Fraser University) for 10 days without exchange of the solution. Samples were incubated in a Sybron Thermolyne™ Dri-bath heat block at 70°C while being shaken at 200 rpm on a Fisher Scientific™ Multi-Platform shaker to promote maximum partitioning.
A calibration standard of tetramethylsilane (0.01%) was used to evaluate the signal-to-noise ratio (between the 3–7 ppm range) and to ensure a linear calibration curve was produced. 1H-NMR measurements of the leached Cyanox®53-PVDF and Cyanox®53 samples were recorded on a Bruker Avance II 600 MHz spectrometer, equipped with a cryoprobe. Both 1H-NMR spectra were acquired with 1024 scans at 298 K, between −1 ppm and 14 ppm, and referenced to the residual DMSO resonance at 2.50 ppm. As references, 10 mg of dry extract 2.2′-methylenebis (4-ethyl-6-tert-butylphenol) (≥98.0% purity), 2.2′-methylenebis (6-tert-butyl-4-methylphenol) (≥99.0% purity), 4.4′-isopropylidenediphenol (≥99.0% purity), respectively, were dissolved into 2 mL of 99.9% DMSO-d6 separately. Their 1H-NMR spectra were also acquired on a Bruker Avance II 600 MHz spectrometer equipped with a cryoprobe at 298 K, between −1 ppm and 14 ppm, with a relaxation delay of 5 for 128 scans. The chromatographic separation was achieved on an Agilent 6210 TOF LC/MS using ESI-MS. Each sample concurrently ran on a C18 LC gradient from water-acetonitrile (0%–100%) for 8 min. Scans were ran in positive mode from 100 to 1500 m/z; a flow rate of 100 μL/min and an injection volume of 10 μL was used. All obtained m/z values were background corrected to account for the water-acetonitrile mobile phase (∼18 Da). Procedural blanks were processed and analyzed alongside NMR and MS analyses to account for potential ambient or processing contamination of samples. All collected NMR and MS data were analyzed in MestReNova, Version 14.2.1 (MestReNova, 2021).
To assess the potential risk of Cyanox®53 if it were to leach from its solid form, the amount of Cyanox®53 ingested daily by an overwintering sea duck species, the surf scoter (M. perspicillata), was estimated on a particles/kg body weight/day basis using the model from Bendell (2011) (Equation 3). Full details of the modeling approach can be found in Bendell (2011). In brief, the number of MPs ingested by a surf scoter each day can be estimated if one knows i) the daily kJ energy required by a surf scoter, ii) the kJ content of a varnish clam, iii) the number of clams ingested per day by a surf scoter, iv) the average number of MPs per clam, and v) the average size of a surf scoter. An average size of a surf scoter is ca. 1 kg, therefore the equation used to estimate MP ingestion would be:
Using this approach we calculated the average, minimum and maximum number of particles of Cyanox®53 that would be ingested on a daily basis, then as the total amount ingested over a 4-month overwintering period. It should be noted that these are estimates that assume a constant daily rate of ingestion. Energetic requirements of the scoter can vary daily based on prey availability with energy expenditure inversely related to amounts of prey available, and external factors such as weather (i.e., temperature variation and storm events).
Data collected from ATR-FTIR, 1H-NMR, and ESI-LC/MS analyses, and the documented structures of the purchased reference chemicals (see section 2.5.2), were used to propose a chemical structure for Cyanox®53. Conversion of the proposed structure into Simplified Molecular Input Line Entry System (SMILES) notation was conducted in JChem for Office, Version 21.11.10 (Cheminformatics Software, 2021). Estimated physical/chemical and environmental fate properties of Cyanox®53 were predicted using the Windows®-based screening level tool Estimation Program Interface (EPI) Suite™ Version 4.11 (US EPA, 2021). Potential acute and chronic toxicity values of Cyanox®53 to aquatic organism surrogates, such as fish, Daphnia magna, and green algae, were predicted with ECOSAR™ embedded in EPI SUITE, 2021. If the log Kow of Cyanox®53 was greater than the endpoint specific cut-off established, then no effects at saturation (NES) were expected for that endpoint. Additionally, if the predicted effect level exceeded the water solubility of Cyanox®53 by ≥ 10-fold, then NES was reported. Default settings were used to run the QSAR software, and no changes were made to the developers’ programming.
Cyanox®53 concentrations ranging from 1 to 5 particles/kg dw per site were detected in surface sediments from AP, BMP, EB, and SB. The highest mean concentration of Cyanox®53 was observed at both AP (1.67 ± 0.88 particles/kg dw) and EB (1.67 ± 1.67 particles/kg dw), while the lowest mean concentration was found at SB (0.33 ± 0.33 particles/kg dw) (Figure 3). MP concentrations ranging from 4 to 18 particles/kg dw per site were also detected in surface sediments from all seven sites. The highest mean concentration of MPs was reported at EB (15.7 ± 0.33 particles/kg dw) while the lowest mean concentration was found at J (6.67 ± 1.33 particles/kg dw) (Figure 3).
Figure 3. Mean particle concentrations (particles/kg dw) of microplastics (purple) and Cyanox®53 (teal) recovered from surface sediments at each sampling site in Burrard Inlet.
Bivalve biometric data did not require transformation for any of the seven bivalve populations as shell width (cm), height (cm), length (cm), volume (cm3), and tissue weight (g) were all found to be normally distributed following frequency distribution, normal quartile-quantile plotting, and Shapiro-Wilk normality tests (p > 0.05). A summary of mean biometric data and condition indices (g/cm3) for each site is provided in the Supplementary Material. Cyanox®53 concentrations were recovered in bivalves from four out of the seven surveyed sites (AP, BMP, EB, and SB). Between 4% and 44% of the individuals possessed Cyanox®53 within their digestive tract. The highest mean concentration of Cyanox®53 was observed at AP (0.25 ± 0.21 particles/g ww), while the lowest was detected at BMP (0.0048 ± 0.0048 particles/g ww) (Figure 4). MPs accumulated within the digestive tracts of almost all sampled bivalves, resulting in frequency levels ranging between 80% and 100%. The highest mean concentration of MPs was observed at AP (0.80 ± 0.21 particles/g ww), while the lowest was recorded at EB (0.19 ± 0.040 particles/g ww) (Figure 4).
Figure 4. Mean particle concentrations (particles/g ww) of microplastics (purple) and Cyanox®53 (teal) recovered from varnish clam at each sampling site in Burrard Inlet.
Collectively, particle concentrations accumulated in bivalve tissue exceeded concentrations deposited in surface sediment samples. Ratios ranging from 0 to 147 were calculated for Cyanox®53, while MPs demonstrated ratios between 12.2 and 88.7. A summary of these ratios is shown in Table 1.
Table 1. Biota-sediment accumulation factor ratios based on mean Cyanox®53 and microplastic concentrations recovered from sampled bivalves and sediments.
Estimates (mean with standard errors) of the daily amounts of Cyanox®53 particles ingested by the surf scoter are presented in Table 2. Ingestion rates are site dependent with birds foraging at AP ingesting the greatest amounts as opposed to birds that forage at BMP.
Table 2. Mean particle concentrations with standard error, frequency of Cyanox®53 recovered in bivalves and estimated number of particles ingested on a daily basis for male and female surf scoters foraging on varnish clams (from Bendell, 2011).
Ninety-two fragments (<5 mm) positively associated with Cyanox®53 at a ≥70% confidence were recovered from bivalve and sediment samples. Most of the fragments were identified as a chemical mixture of Cyanox®53 with either PVDF (49) or PE (8), while the remaining 35 fragments were assigned the chemical identity of Cyanox®53. ATR-FTIR spectrums of field-collected fragments are provided in the Supplementary Material. The following functional groups based on obtained ATR-FTIR sorption peaks were identified for Cyanox®53: hydroxyl alcohols (3600–3300 cm-1 and 950–800 cm-1), in-plane, out-of-plane and radical alkyls (3450–3300 cm-1, 2850–2750 cm-1, 1250–1000 cm-1, and 790–600 cm-1), and two aromatic rings (3100–2750 cm-1, 2600–2450 cm-1, 1800–1700 cm-1, and 1600–1250 cm-1).
Reference chemicals bisphenol A (BPA), Cyanox®2246, and Cyanox®425 returned m/z values that aligned with literature measurements. In the Cyanox®53 sample five distinct intensity peaks were observed at retention times 5.31, 6.06, 6.75, 7.14, and 7.46 min. Similarly, four individual intensity peaks were observed at retention times 5.26, 6.75, 7.16, and 7.46 min in the Cyanox®53-PVDF sample. No cross-contamination by PVDF was observed in the Cyanox®53-PVDF spectra. Through the process of elimination, the m/z value of 358.4 Da was deemed the authentic molecular mass for Cyanox®53. All obtained MS spectra are provided in the Supplementary Material.
Reference chemicals BPA, Cyanox®2246, and Cyanox®425 produced 1H-NMR spectra that aligned with literature measurements. Similar chemical shifts and integral values were obtained in the Cyanox®53 and Cyanox®53-PVDF samples. Peaks appeared at 8.47, 5.91, 5.86, 1.24, and 0.86 ppm, and corresponded to the integral values of 1.00 (hydroxyl), 1.08 (hydrogen), 1.08 (hydrogen), 9.52 (tert-butyl) and 3.36 (methyl), respectively. No cross-contamination by PVDF was observed in the Cyanox®53-PVDF spectra. Any peaks corresponding to water (3.50 ppm) and DMSO-d6 (2.50 ppm) were considered as negligible background. All obtained 1H-NMR spectra, and 1H-NMR measurements and structural assignments for each chemical, are provided in the Supplementary Material.
Collected ATR-FTIR, 1H-NMR, and ESI-LC/MS data, and the reference of documented structures for BPA, Cyanox®2246, and Cyanox®425, allowed for the elucidation of a feasible chemical formulation for Cyanox®53 as 2.2′-thiobis (3-methyl-5-tert-butylphenol) or C22H30O2S. Application of JChem Office directly translated 2.2′-thiobis (3-methyl-5-tert-butylphenol) to the SMILES notation of CC1 = C(SC2 = C(C)C=C(C=C2O) C(C)(C)C)C(O) = CC(=C1)C(C)(C)C. A chemical search by said SMILES notation in PubChem, ChemSpider, and CompTox Dashboard databases, however, returned no known CAS registry number. The proposed structure of Cyanox®53 along with the chemical structures of BPA, Cyanox®2246, and Cyanox®425 are shown in Figure 5.
Figure 5. Proposed molecular structure of Cyanox®53 compared to reference chemicals BPA, Cyanox®2246, and Cyanox®425.
Predictions of physical/chemical environmental fate and aquatic toxicity properties were made for Cyanox®53 (Table 3). Based on the atom/fragment contribution, a log Kow value of 8.24 was estimated by KOWWIN™. Melting and boiling points for Cyanox®53 were estimated at 196°C and 466°C, respectively by MPBPVP™. Water solubility estimates (at 25 °C) of 0.00191 mg/L (based on log Kow) and 0.0568 mg/L (based on chemical fragments) were calculated by WSKOWWIN™ and WATERNT™, respectively. BCF and BAF estimations in fish were acquired with BCFBAF™. The following estimates were obtained: BCF = 3,270 L/kg wet-wt (regression method based on Kow), BCF = 28.22 L/kg wet-wt (Arnot-Gobas upper trophic method), and BAF = 308 L/kg wet-wt (Arnot-Gobas upper trophic method). A biotransformation half-life in fish equivalent to 2.5 days (normalized to a 10 g fish) was also predicted. An ultimate survey and primary survey timeframe of 1.946 (months) and 2.966 (weeks), respectively, and a MITI linear model value of 0.0274 were estimated in the evaluation of rapid biodegradability. Accordingly, the BIOWIN™ model criteria for rapid biodegradation was not satisfied. Moreover, log Koc estimates of 6.30 (based on MCI method) and 5.82 (based on Kow) were obtained by KOCWIN™. Partitioning half-life times of Cyanox®53 in air, water, soil, and sediment under steady state conditions were estimated to be 1.27 h, 60 d, 120 d, and 540 d, respectively. An estimated maximum half-life persistence of 189 d was computed.
ECOSAR™ was used to estimate acute toxicity endpoints at 50% lethal concentration (LC50) for fish and D. magna, and 50% effective concentration (EC50) for green algae. For fish, a simulated 96 h exposure predicted a LC50 value of 0.0040 mg/L with a log Kow maximum of 7.0. A LC50 value of 0.0020 mg/L with a log Kow maximum of 5.5 was predicted for D. magna in a simulated 48 h exposure. A 96 h exposure manifested a EC50 value of 0.058 mg/L with a maximum log Kow of 6.4 for green algae. Chronic toxicity effect endpoints were also predicted for fish, D. magna and green algae based on a geometric mean between the lowest observed effects concentration (LOEC) and no observed effect concentration (NOEC) of the study. A maximum log Kow cut-off was set to 8.0 (indicative of a poorly soluble chemical) as a baseline for comparison in fish, D. magna and green algae. Chronic effect concentrations of 0.00078 mg/L, 0.00062 mg/L, and 0.020 mg/L were predicted for fish, D. magna, and green algae, respectively.
Our study recovered Cyanox®53 concentrations in the surface sediments and varnish clam of several sites in Burrard Inlet, BC. As predicted, Cyanox®53 concentrations were found to be the most abundant near the First Narrows strait (mouth of outer and inner harbor) where the majority of the Port of Vancouver is centralized. The highest levels were presented at AP and EB, with no significant difference being noted between these sites in either sampling medium. These results coincide with recent reports of Cyanox®53 concentrations bioaccumulating in marine coastal sediments and bivalves (Knutsen et al., 2019; Bendell et al., 2020; Achiluzzi, 2022). Like the previous surveys, a key characteristic of the Cyanox®53 particles in this study was their disassociation from its inert carrier and abnormal presentation as a white, fragmented entity. It is unknown how or why this alleged powdered additive continues to emerge as a fibrous solid in the environment. If recent environmental surveys are considered, however, a trend of Cyanox®53 particles appearing in areas with intense shoreline alterations and maritime activities manifests, in which case, one can speculate that a link exists between Cyanox®53 particles and anthropogenic pressures. Additional backing to this surmise comes from the fact that Cyanox®53 can be integrated as a thermal stabilizer into rubbers, cable resins, coating liners of PE wiring and thermally insulated piping, and that some Cyanox® variants can provide processing and thermal stability in rubbers products, acrylics, and polyolefins marketed to the construction industry (Flick, 2001; Cruce et al., 2014; Bolgar et al., 2016; Solvay, 2020). Alternatively, Cyanox®53 could be potentially associated with pyrotechnics.
Though it depends on scale of the event, fireworks are a significant source of MP debris that contribute to atmospheric, terrestrial, freshwater, and marine pollution (Devereux et al., 2022). On average a pyrotechnic mixture will contain roughly 10% of a polymeric binder, either made from a natural (e.g., starch), synthetic (e.g., novolac), or synthetic polymer (e.g., polyvinyl chloride) material (Naik and Patil, 2015; Toader et al., 2017; Devereux et al., 2022). In certain circumstances, fireworks may also include plastic attachments, such as a mortar and tube at the bottom to boost their momentum at launch, or a cone at the top to aid their flight pattern, leading to the discharge of additional plastic debris upon denotation (Naik and Patil, 2015; Devereux et al., 2022). Other toxic substances, metals, airborne particulates, and chemical pollutants have also been shown to accumulate near the fireworks display areas following detonation (Devereux et al., 2022). In Vancouver, BC, several fireworks events are held annually off-shore in EB, including New Year’s, Canada Day, Halloween, and most notoriously the three-night international fireworks competition “Honda Celebration of Light”. Incidentally, EB and AP were also two locations in this present study with the highest recovered Cyanox®53 particle concentrations. Considering this, along with the fact that a prominent number of the Cyanox®53 fragments possessed a shrapnel-like appearance and chemical association with PE or PVDF (two thermoplastics commonly used in packaging and industrial applications), it is plausible that Cyanox®53 could be incorporated as an additive either directly into the pyrotechnic composition, or indirectly in the outer plastic attachments if equipped, to offer stability. To explore these speculations further investigation is warranted.
A high abundance of MPs (i.e., high-density polyethylene, low-density polyethylene, polypropylene, polystyrene, and polyvinyl chloride) was also recovered in sediments and clam tissue, with concentrations being more abundantly prevalent than Cyanox®53 at all assessed sites in both sampling mediums. Expressed BSAF ratios demonstrated that bivalves accumulated higher mean particle concentrations, more frequently MPs (80%–100%), in their guts and gills than the intertidal sediment samples. This finding is unsurprising given that sediments act as a sink for negatively buoyant MPs, meaning bivalves, to varying degrees, will inevitably accumulate suspended MPs as they pull particles (i.e., diatoms, bacteria, phytoplankton, etc.) into their incurrent siphons from the water column, or collect organic detritus from the sediment with their foot (Gillespie et al., 1999; Gillespie et al., 2001; Hidalgo-Ruz et al., 2012; Cho et al., 2021; Ding et al., 2021). It is because of this that bivalve species (e.g., Venerupis philippinarum, Siliqua patula, M. edulis, and Crassostrea gigas) have a history of use as biomarkers of MPs contamination levels, as well as pollutants, hypoxia, and algal toxins, occupying the water column and surrounding sediments (Baechler et al., 2020; Hermabessiere et al., 2019; Bendell et al., 2020; Cho et al., 2021; Huffman Ringwood, 2021). In the case of this present study, the largely sessile, sediment dwelling varnish clams functioned as suspension and deposit feeders, thus increasing their likelihood of accumulating any marine debris and xenobiotic particles within the water interface and sediment (Gillespie et al., 2001). Ultimately, these results support the notion that opportunistic feeding bivalve species are suitable bioindicators of MP pollution. Furthermore, it demonstrates that opportunistic bivalves could be used as indicators of MP bioaccumulation, perhaps based on the function of retention time and/or elimination rate of the MPs in the bivalve, to support the categorization and screening of potentially bioaccumulative MPs and nanoplastics in bioaccumulation assessments (Alava, 2021).
Plastic ingestion has been documented in over 100 species of seabird (Laist, 1997). Examples include Trevail et al. (2015), who studied plastic ingestion in high Arctic Fulmarus glacialis from Svalbard, finding that 87.5% of the 40 sampled fulmars had ingested plastics, averaging 0.08 g or 15.3 ± 5.51 pieces per individual. This challenges the trend of decreasing plastic ingestion with distance from human activity. Regional comparisons showed average plastic ingestion ranging from 0.55 g in the English Channel to approximately 0.2 g in Canada, with over 80% of the populations, except Canada, having plastics in their stomachs. Another example is Provencher et al. (2014) who reviewed marine debris in North Atlantic seabirds, finding that of the 13 species studied, 5 contained ingested marine debris. Plastic ingestion frequency was low in common eiders (1%), but moderate in Leach’s storm-petrels, thick-billed murres, and Atlantic puffins (50%). Great shearwaters had the highest prevalence (71%), with a median of 2 plastic pieces per bird and up to 36 pieces in some individuals. Detailed plastics data was available for detailed examination from 11 great shearwaters (range 0–36) and the number of pieces in their GITs was relatively high (two individuals had 36 plastic pieces in their GITs). It was also shown that Northern fulmars had significant ingestion, with 51% of individuals containing plastics. In their review, Provencher et al. (2014) noted that a bird’s foraging mode is assumed to play a large role in how much marine debris it ingests. These authors suggested that diving birds, such as auks and sea ducks, are expected to have relatively low levels of plastics because they rarely feed at the water surface where most plastics tend to float. By contrast, surface-feeding birds, represented by great shearwaters, northern fulmars, Arctic terns, and Leach’s storm-petrels, are thought to be more susceptible to ingesting plastics with the findings of their review supporting this prediction, i.e., great shearwaters and northern fulmars exhibiting the highest levels of plastic ingestion (71% and 51% occurrence, respectively). Not considered, however, and as shown in our study, is the trophic transfer of MPs from sediments to sediment-ingesting bivalves such as the varnish clam and then to predators such as diving ducks.
Despite their potential hazards, the ingestion and effects of MPs are not fully understood. Yahya et al. (2023) reviewed the occurrence and pathways of MPs in aquatic environments, highlighting a significant gap in understanding their ingestion and effects. The available studies indicate health hazards such as gut leakage, pathogenic bacterial transfer, and increased toxicity, though most research has focused on fish, with limited information on seabirds. Sun et al. (2024) investigated the combined effects of PVC MPs and cadmium on Muscovy ducks, finding that co-exposure induced oxidative stress and fibrosis, disrupted mitochondrial structure and function, and promoted pancreas inflammation and fibrosis.
Recent work by Charlton-Howard et al. (2023) on the Flesh-footed Shearwater from Lord Howe Island provides one of the first studies to demonstrate the effects of solid plastics particles on seabird health. In their study, widespread scar tissue formation and changes to the prevalence of collagen within the stomach of the birds was associated with exposure to plastics (Charlton-Howard et al., 2023). The outcome of these findings was identified as a new plastic induced fibrotic disease, “Plasticosis”, a possible disease that the varnish clam eating bird populations could be susceptible to. MPs have also been shown to serve as carriers of viral diseases through adsorption, thus increasing the risk of environmental dissemination of water-borne viruses (Liu et al., 2022).
Burrard Inlet, which is part of the Salish Sea is recognized as one of the world’s most important bird areas supporting globally vital bird populations (Bowman et al., 2022). It is especially important to sea ducks of the Pacific Flyway during wintering, staging, spring migration, and molting. Indeed, this site is a major wintering location for 11 species of sea ducks, including Surf Scoter (Melanitta perspicillita), White-winged Scoter (Melanitta deglandi), Black Scoter (Melanitta americana), Bufflehead (Bucephala albeola), Common Goldeneye (Bucephala clangula), Barrow’s Goldeneye (Bucephala islandica), all three mergansers (Mergus spp.), Long-tailed Duck (Clangula hyemalis), and Harlequin Duck (Histrionicus) (Bowman et al., 2022). Lewis et al. (2008) and Palm et al. (2012) have noted that varnish clams make up the main diet for the wintering white-winged scoter, although Anderson et al. (2008) note that soft-bodied prey items are also of importance. Recently, Hollenberg and Demers (2017) reported that in addition to sea ducks, shorebirds such as the Black Oystercatcher (Haematopus bachmani) forage on varnish clams with little size selectivity.
To our knowledge, there have been no bioenergetic models applied to determine how much MP on a Gram basis is ingested by seabirds. The scoter duck in this case provides an opportunity to do so as: 1) they ingest solely one diet item, the varnish clam, 2) their energetic requirements on a daily basis are known and 3) their behavior with respect to overwintering in a particular area is known. Therefore, as done by Bendell (2011), a bioenergetics model was applied here to assess the amount of Cyanox®53 particles ingested by an overwintering sea duck species, using the surf scoter as a model. In Burrard Inlet, key overwintering months include November, December, January, and February (Bendell and Wan, 2010). Generally, scoters will have an energy intake of 1035 kJ/day and 1100 kJ/day for male and female scoters, respectively (Table 2; Bendell, 2011). Based on values provided by Simmons et al. (2014), a 36 mm varnish clam provides 4.64 kJ of energy; in the current study, the average size of the collected varnish clams was 47 mm, which provides 6.05 kJ of energy. The average number of Cyanox®53 particles recovered from varnish clams across the seven sites was 0.46 particles/clam. Considering these details, a male and female scoter will have ingested 78 and 83 particles of Cyanox®53, respectively, in one day. Over four wintering months, 120 days, male and female scoters will have ingested 9421.8 and 10,086 particles of Cyanox®53, respectively. According to Senathirajah et al. (2021), who estimated the weight of MPs based on size, the mean mass of an individual MP particle ranges from 2.8 to 3.99 g/particle x 10−3 for a 0–1 mm particle size; the same size range of Cyanox®53 particles collected in the present study. Using the average of this range, 3.5 g/particle x 10−3, a male and female scoter will have consumed 33 and 35 g, respectively. To put this estimate into perspective, the amount of Cyanox®53 consumed throughout the overwintering period would be the equivalent to a male and female scoter ingesting 5.5 and 6 plastic cutlery forks that weigh 6 g each. We use this analogy to demonstrate the degree to which bivalve-consuming sea ducks are at risk to MPs, such as Cyanox®53, within their primary diet items. It is not the recovery of the number of plastics within the animal at one point of time, but rather the cumulative effects of chronic exposure over a longer period of time. We know of no studies that have tried to assess the toxicological significance of this exposure.
As mentioned earlier, assessing the toxicity of MPs poses quite a challenge to researchers as they not only constitute a physical obstacle to aquatic life, but also a dynamic vector and potential reservoir of inherent additives and adsorbed hydrophobic anthropogenic contaminants. Within recent decades, QSAR modeling has become favored over conventional in vivo experimentation in the identification of physiochemical properties, and toxicological mechanism and structure–activity relationship for dissolved “neat” chemicals (Lapenna et al., 2010; Hayes and Kruger, 2014; OECD, 2022a; OECD, 2022b). Likewise, we applied a similar approach to estimate the potential persistence, bioaccumulation, and toxicity of Cyanox®53 based on a proposed chemical structure, assuming that there is the potential for this compound to leach from the solid phase. The proposed chemical structure for Cyanox®53, which was generated from collected ATR-FTIR, 1H-NMR, and ESI-LC/MS data and the use of BPA, Cyanox®2246, and Cyanox®425 as reference structures, was 2.2′-thiobis (3-methyl-5-tert-butylphenol). From this structure, a log Kow of 8.24 with a low water solubility of 0.00191 mg/L (0.0568 mg/L based on chemical fragments) at 25°C was predicted for Cyanox®53. As a rule, chemicals with high log Kow values (≥5) are deemed to be poorly water soluble and tend to partition to organic matter in soils and sediments within their surroundings (CEPA, 2013; Chi et al., 2018). Provided this, the same outcome would be assumed for Cyanox®53 in its chemical form. Further support to this inference comes from predictions of low mobility in sediment (high log Koc (>5)), low biodegradability (equivalent to 2 months before complete mineralization), partitioning half-lives of 120 and 540 days in soil and sediment, respectively, and a max half-life of 189 days in the environment. Together, these estimates suggest that Cyanox®53 is likely to persist in its chemical form via adsorption to organic matter in the surrounding soils and sediments, and thus have a prolonged fate in the environment.
Bioaccumulation potential is another important parameter to consider in the risk assessment of environmental pollutants. Highly bioaccumulative chemicals are of particular concern to biota given their potential to cause direct adverse health effects (i.e., physiological damage) or indirect toxicity in higher trophic level organisms or/and apex predators at the top of the food web (i.e., biomagnification) regardless of the ambient concentration (CEPA, 2013b). In the simplest model, log Kow can be used to screen the bioaccumulation potential of a chemical based on the assumption that the absorption of an organic substance is driven by its hydrophobicity (European Centre for Ecotoxicology and Toxicology of Chemicals, 2000). However, bioaccumulation potential is not solely dependent on hydrophobicity, rather other underlying mechanisms and physiological properties of an organism can affect the bioconcentration and bioaccumulation potentials of organic substances. For instance, many organic substances can undergo metabolism (biotransformation) and depuration, resulting in a decrease in the bioaccumulation potential of the parent compound (ECETC, 1998; CEPA, 2013a). Moreover, in scenarios where organisms are exposed to very hydrophobic substances (i.e., log Kow > 7.5), a reduction in bioavailability (and therefore bioaccumulation/bioconcentration potentials) can be expected because the chemical will be largely absorbed to any dissolved or particulate organic carbon suspended in the diet (Gobas and Morrison, 2000; Gobas, 2001; Arnot and Gobas, 2003; CEPA, 2013b). By this logic, while the predicted log Kow of Cyanox®53 appears to exceed the threshold set by Environment Canada (log Kow > 5), its measurement cannot provide a definite answer for bioaccumulation potential in its chemical form. Alternatively, BAF and BCF would provide a more realistic interpretation of bioaccumulation potential of a substance (CEPA, 2013a; CEPA, 2013b). In Canada, a criterion of 5000 for BAFs or BCFs are recommended as the threshold to address lipophilic substances with the potential to bioaccumulate and biomagnify (CEPA, 2013a). Concerning Cyanox®53, the predicted BAF (308 L/kg wet-wt Arnot-Gobas method) and BCF (3,270 L/kg wet-wt regression method; 28.22 L/kg wet-wt Arnot-Gobas method) values fell below theses benchmarks, indicating that Cyanox®53 would be unlikely to bioaccumulate or biomagnify up the food-chain in its chemical form. Such implication, however, does not apply to Cyanox®53 in its solid form as it is evident from recent surveys (i.e., Knutsen et al., 2019; Bendell et al., 2020; Achiluzzi, 2022), as well as this current study, that the additive is fully capable of bioaccumulating in sediments and bivalves as a physical particulate. Hence, further investigation into the bioaccumulation potential of Cyanox®53 is recommended. As a future study, one could analyze the retention time and depuration of Cyanox®53 particles accumulated in field-collected varnish clams at different time points (e.g., 1, 2, 4 and 8 d) post collection.
According to the ECOSAR™ model, the proposed chemical structure of this study was found to be too insufficiently water soluble to elicit toxicological effects at saturation in the acute and chronic exposure scenarios for fish, D. magna, or green algae when the predicted log Kow of 8.24 was used as the determinant. However, when the predicted water solubility values were applied as the determinants of toxicity, different results were seen. Specifically, if the predicted water solubility of 0.00191 mg/L (based on the predicted log Kow) was used for comparison, potential toxicological effects were simulated in the acute and chronic exposure scenarios for D. magna and fish surrogates, with NES being noted in the green algae. But, if the predicted water solubility of 0.0568 mg/L (based on chemical fragments) was used, potential toxicological effects were observed in acute and chronic exposure scenarios for D. magna, fish, and green algae. Clearly, there is a discrepancy between the predicted log Kow and water solubility values. It is because of this disconnect that a definitive answer cannot be given on the toxicological effects of Cyanox®53 based on the EPI Suite™ results.
The likelihood that an excessive amount of Cyanox®53 particles would leach out a high enough concentration to induce significant toxicity acutely before being discharged is considerably low. Moreover, given that frank toxicity was not observed in the varnish clams containing Cyanox®53 particles in this study, the potential of Cyanox®53 causing toxicological effects appears to decrease. All the same, it cannot be said for certain that a toxicological effect would not have eventually taken place on some level in the clams from chronic exposure. Indeed, rather than exert acute toxicity, perhaps the hazardous nature of Cyanox®53 particles stem from their ability to bioaccumulate as a solid chronically and potential to cause physical harm at the organ level, either directly in an organism or indirectly when predatory species unknowingly ingest contaminated prey (i.e., trophic transfer) (Gallo et al., 2018; Nelms et al., 2019). The only way to verify this theory would be to perform chronic in vitro or in vivo toxicity tests, such as Organisation for Economic Co-operation and Development (OECD) test guidelines No. 201, 204, 210, 211, or 230, with Cyanox®53 particles (OECD, 1984; OECD, 2009; OECD, 2011; OECD, 2012; OECD, 2013).
Although QSAR analyses have been deemed an accepted method in environmental risk screening, different levels of uncertainty and variability exist within each model and measured parameter. For instance, it is known that log Kow predictions in EPI Suite™ are derived from experimental Kow values of the nearest chemical analogs (Do et al., 2022). Because of this, it increases the probability of inaccurately predicting certain descriptors for a chemical. The simple solution to this issue would be to compare the predicted results to experimental data collected in model-based or laboratory-based research for verification. However, in the case of Cyanox®53, there are no model-based or experimental data for comparison in the literature, therefore making it very challenging to decisively measure its toxicological significance. Coupling this with the fact that the predictions seen in this present study, albeit valuable, are merely based on a feasible structure, the likelihood that uncertainty may exist in the predictions from EPI Suite™ arises. Even so, it should not be implied that the results observed in this study are completely invaluable or inaccurate. Instead, these results represent a baseline for comparison in future studies and should be validated with OECD test guidelines like No. 105, 107, 117, 123, and 301 (OECD, 1992; OECD, 1995a; OECD, 1995b; OECD, 2022a; OECD, 2022b; OECD, 2024).
Another plausible explanation as to why the toxicological predictions of this study were inconclusive could be related to the assumptions of EPI Suite™, namely, the assumption that all compounds exist as neatly dissolved chemicals. At present, most QSAR models are developed specifically for low molecular weight organic compounds, making it quite challenging to evaluate and mitigate the potential environmental impacts of MPs and associated additives (Brunning et al., 2022). In EPI Suite™, its chemical domain of applicability does not cover the full range of substances, nor does it currently produce accurate estimates for inorganic substances, organometallic substances, some ionizable organic compound, large molecular weight (>500 Da) substances, nanomaterials, perfluorinated and other halogenated compounds, compounds without functional groups, and compounds containing significant organic functional groups (Card et al., 2017). When it comes to Cyanox®53, it evidently does not adhere to the above chemical profiles, thus any predictions procured for Cyanox®53 falsely assumed that it existed as a dissolved chemical, rather than a solid particle. Such a misinterpretation can reduce the overall accuracy and predictive powers of a QSAR model. Not to mention, in assuming that Cyanox®53 only exists as a dissolved chemical in the environment, it neglects the possibility that Cyanox®53 particles could bioaccumulate within the tissue of an organism as a solid particulate (as seen in this study) and elicit eventual toxicity through chronic exposure. Concepts such as BCF, which assume chemicals move via passive diffusion, are not applicable to nanoparticles and MPs since their uptake is dependent on several parameters (including size, shape, volume, density, roughness, and polymer composition) and processes like the retention time and/or elimination rate in an organism, rather than the equilibrium partitioning (Alava, 2021; Brunning et al., 2022). To correct this oversight, development of QSARs that consider the influence of different parameters of MP fate, transport, physiochemical properties, and toxicity in the environment is required. Furthermore, robust data collection and research into relevant properties, such as the leachability and retention time of accumulated MPs and alike particles in biota, is warranted.
Given the lack of admissions on Cyanox®53, the evidence uncovered in this work shows promise for forthcoming analyses. It was only recently that the presence of Cyanox®53 in the environment had been documented, making this study one of the first to recover particle concentrations in sediments and bivalves from Burrard Inlet, BC. This is also the first study to provide ecological relevance and QSAR predictions of persistence, bioaccumulation, and toxicity for Cyanox®53. Our findings indicate that varnish clams collected from Burrard Inlet accumulate on average 0.46 particles of Cyanox®53/clam, and based on bioenergetic modeling, results in surf scoters potentially consuming 78 (for males) to 83 (for females) pieces of Cyanox®53 daily from foraged varnish clams. Like other bisphenol-related compounds, we hypothesized Cyanox®53 potentially possessed some degree of toxicity towards aquatic organisms and/or seabirds. EPI Suite™ predicted Cyanox®53 to be persistent, however, unlikely to bioaccumulate as a “traditional” chemical. Furthermore, the estimation of potential acute and chronic toxicity of Cyanox®53 to aquatic organism surrogates, such as fish, D. magna, and green algae, was inconclusive. Currently, QSAR models, such as those in EPI Suite™, function on the assumption that organic compounds exist as neatly dissolved chemicals in the environment, and thus can only provide accurate predictions for “traditional” chemicals. This assumption ultimately became a limitation in this study and further demonstrates that we have yet to develop the tools necessary to place an adequate risk or hazard on MPs and associated compounds within aquatic (and terrestrial) ecosystems. Still, the predictions estimated in this present study hold value as they represent a baseline for comparison in future works. If a definitive answer is ever to be obtained concerning the risk of Cyanox®53, however, consideration needs to be given to the development of polymer QSARs. In addition, further investigation into its potential toxicity, leachability, and retention time as a physical particle in organisms is required, most likely through in vitro and in vivo testing. In bridging these research gaps, it would offer unprecedented opportunities for further upscaling MP data collection, aid in the establishment of key parameters for inter-study or regulatory, and determine the degree to which MPs, such as Cyanox®53, could impact sea ducks and higher trophic predators within marine ecosystems.
The original contributions presented in the study are included in the article/Supplementary Material, further inquiries can be directed to the corresponding author.
The manuscript presents research on animals that do not require ethical approval for their study.
SR: Conceptualization, Data curation, Formal Analysis, Investigation, Methodology, Software, Validation, Visualization, Writing–original draft, Writing–review and editing. LB: Conceptualization, Funding acquisition, Methodology, Project administration, Resources, Supervision, Validation, Writing–original draft, Writing–review and editing.
The authors declare that financial support was received for the research, authorship, and/or publication of this article. Financial support was received from the Natural Sciences and Engineering Research Council of Canada (grant number: 31-611307).
The authors gratefully acknowledge the Natural Sciences and Engineering Research Council of Canada for the funding opportunity. We also thank Wen Zhou, Eric Ye, Shane Harrypersad, Curtis Eickhoff, and Isabelle Côté for their contributions.
The authors declare that the research was conducted in the absence of any commercial or financial relationships that could be construed as a potential conflict of interest.
All claims expressed in this article are solely those of the authors and do not necessarily represent those of their affiliated organizations, or those of the publisher, the editors and the reviewers. Any product that may be evaluated in this article, or claim that may be made by its manufacturer, is not guaranteed or endorsed by the publisher.
The Supplementary Material for this article can be found online at: https://www.frontiersin.org/articles/10.3389/fenvs.2024.1439573/full#supplementary-material
AccuStandard (2018). Plastic additive standards guide. Available at: https://www.accustandard.com/plastic-additive-catalog-2nd-edition.
Achiluzzi, E. (2022). Risk assessment: role of MPs through benthic invertebrate communities in False Creek, vancouver. MS thesis. Burnaby, BC: Simon Fraser University.
Adam, V., Yang, T., and Nowack, B. (2019). Toward an ecotoxicological risk assessment of microplastics: comparison of available hazard and exposure data in freshwaters. Environ. Toxicol. Chem. 38 (2), 436–447. doi:10.1002/etc.4323
Alava, J. J. (2021). “Proposing a bioaccumulation metric criteria framework for plastic particles in marine biota and foodwebs,” in Abstracts book: society of environmental Toxicology and Chemistry North America 42nd annual meeting - (SETAC SciCon4 2021) (Portland, OR), 25. Available at: https://scicon4.setac.org/wp-content/uploads/2021/11/SciCon4-abstract-book.pdf.
Anderson, E. M., Lovvorn, J. R., and Wilson, M. T. (2008). Reevaluating marine diets of surf and white-winged scoters: interspecific differences and the importance of soft-bodied prey. Condor 110 (2), 285–295. doi:10.1525/cond.2008.8458
Andrady, A. L. (2011). Microplastics in the marine environment. Mar. Pollut. Bull. 62 (8), 1596–1605. doi:10.1016/j.marpolbul.2011.05.030
Arnot, J. A., and Gobas, F. A. (2003). A generic QSAR for assessing the bioaccumulation potential of organic chemicals in aquatic food webs. QSAR Comb. Sci. 22 (3), 337–345. doi:10.1002/qsar.200390023
Baechler, B. R., Stienbarger, C. D., Horn, D. A., Joseph, J., Taylor, A. R., Granek, E. F., et al. (2020). Microplastic occurrence and effects in commercially harvested North American finfish and shellfish: current knowledge and future directions. Limnol. Oceanogr. Lett. 5 (1), 113–136. doi:10.1002/lol2.10122
Baztan, J., Bergmann, M., Booth, A., Broglio, E., Carrasco, A., Chouinard, O., et al. (2017). Breaking down the plastic age. Fate Impact Microplastics Mar. Ecosyst., 177–181. doi:10.1016/b978-0-12-812271-6.00170-8
Benali, I., Boutiba, Z., Merabet, A., and Chevre, N. (2015). Integrated use of biomarkers and condition indices in mussels (Mytilus galloprovincialis) for monitoring pollution and development of biomarker index to assess the potential toxic of coastal sites. Mar. Pollut. Bull. 95 (1), 385–394. doi:10.1016/j.marpolbul.2015.03.041
Bendell, L. I. (2011). Sea ducks and aquaculture: the cadmium connection. Ecotoxicology 20, 474–478. doi:10.1007/s10646-010-0584-y
Bendell, L. I., Lecadre, E., and Zhou, W. (2020). Use of sediment dwelling bivalves to biomonitor plastic particle pollution in intertidal regions; A review and study. Plos One 15 (5), e0232879. doi:10.1371/journal.pone.0232879
Bendell, L. I., and Wan, P. C. (2010). Application of aerial photography in combination with GIS for coastal management at small spatial scales: a case study of shellfish aquaculture. J. Coast. Conservation 15, 417–431. doi:10.1007/s11852-010-0101-8
Bermúdez, J. R., and Swarzenski, P. W. (2021). A microplastic size classification scheme aligned with universal plankton survey methods. MethodsX 8, 101516. doi:10.1016/j.mex.2021.101516
Besseling, E., Wegner, A., Foekema, E. M., van den Heuvel-Greve, M. J., and Koelmans, A. A. (2013). Effects of microplastic on fitness and PCB bioaccumulation by the Lugworm Arenicola marina (L.). Environ. Sci. and Technol. 47, 593–600. doi:10.1021/es302763x
Bolgar, M., Hubball, J., Groeger, J., and Meronek, S. (2016). Handbook for the chemical analysis of plastic and polymer additives. Boca Raton, Florida, United States: CRC Press.
Bowman, T. D., Churchill, J. L., Lepage, C., Badzinski, S. S., Gilliland, S. G., McLellan, N., et al. (2022). “Atlas of sea duck key habitat sites in North America,” in Sea duck joint venture. Available at: https://seaduckjv.org/science-resources/sea-duck-key-habitat-sites-atlas/.
Bridson, J. H., Gaugler, E. C., Smith, D. A., Northcott, G. L., and Gaw, S. (2021). Leaching and extraction of additives from plastic pollution to inform environmental risk: a multidisciplinary review of analytical approaches. J. Hazard. Mater. 414, 125571. doi:10.1016/j.jhazmat.2021.125571
Brietzke, C., and Starzomski, B. (2013). Biodiversity of the central coast: surf scoter, skunk-headed coot, Melanitta perspicillata. The Starzomski lab research and teaching at the university of victoria. Available at: https://www.centralcoastbiodiversity.org/surf-scoter-bull-melanitta-perspicillata.html.
Browne, M. A., Dissanayake, A., Galloway, T. S., Lowe, D. M., and Thompson, R. C. (2008). Ingested microscopic plastic translocates to the circulatory System of the mussel, Mytilus edulis (L.). Environ. Sci. and Technol. 42 (13), 5026–5031. doi:10.1021/es800249a
Brunning, H., Sallach, J. B., Zanchi, V., Price, O., and Boxall, A. (2022). Toward a framework for environmental fate and exposure assessment of polymers. Environ. Toxicol. Chem. 41 (3), 515–540. doi:10.1002/etc.5272
Canadian Environmental Protection Act (2013a). Ecological state of the science report on decabromodiphenyl ether (decaBDE). Available at: https://www.ec.gc.ca/lcpe-cepa/default.asp?lang=En&n=B901A9EB&offset=3 (Accessed March 18, 2022).
Canadian Environmental Protection Act (2013b). Guidance manual for the risk evaluation framework for sections 199 and 200 of CEPA 1999: decisions on environmental emergency plans. Available at: https://www.canada.ca/en/environment-climate-change/services/canadian-environmental-protection-act-registry/historical/guidance-manual-risk-evaluation-framework-cepa-decisions-environmental-emergency-plans/process.html;%20https://www.ec.gc.ca/lcpe-cepa/default.asp?lang=En&n=B901A9EB&offset=3 (Accessed March 18, 2022).
Card, M. L., Gomez-Alvarez, V., Lee, W. H., Lynch, D. G., Orentas, N. S., Lee, M. T., et al. (2017). History of EPI Suite™ and future perspectives on chemical property estimation in US Toxic Substances Control Act new chemical risk assessments. Environ. Sci. Process. Impacts 19 (3), 203–212. doi:10.1039/c7em00064b
Carlisle, J., Chan, D., Golub, M., Henkel, S., Painter, P., and Wu, K. L. (2009). Toxicological Profile for bisphenol A (tech.). Available at: http://www.opc.ca.gov/webmaster/ftp/project_pages/MarineDebris_OEHHA_ToxProfiles/BisphenolAFinal.pdf.
Chan, K., and Bendell, L. I. (2013). Potential effects of an invasive bivalve, Nuttallia obscurata, on select sediment attributes within the intertidal region of coastal British Columbia. J. Exp. Mar. Biol. Ecol. 444, 66–72. doi:10.1016/j.jembe.2013.03.013
Charlton-Howard, H. S., Bond, A. L., Rivers-Auty, J., and Lavers, J. L. (2023). Plasticosis’: characterising macro- and microplastic-associated fibrosis in seabird tissues. J. Hazard. Mater. 450, 131090. doi:10.1016/j.jhazmat.2023.131090
Cheminformatics Software (2021). Cheminformatics software for the next generation of scientists. ChemAxon Bp. Hung. Available at: http://www.chemaxon.com (Accessed September 02, 2021).
Chi, Y., Zhang, H., Huang, Q., Lin, Y., Ye, G., Zhu, H., et al. (2018). Environmental risk assessment of selected organic chemicals based on TOC test and QSAR estimation models. J. Environ. Sci. 64, 23–31. doi:10.1016/j.jes.2016.11.018
Cho, Y., Shim, W. J., Jang, M., Han, G. M., and Hong, S. H. (2021). Nationwide monitoring of microplastics in bivalves from the coastal environment of Korea. Environ. Pollut. 270, 116175. doi:10.1016/j.envpol.2020.116175
Cruce, C. J., Giardello, M. A., Conley, B. L., and Stephen, A. R. (2014). Thermal insulation. CA 2 915 486 A1.
da Costa, J. P., Duarte, A. C., and Rocha-Santos, T. A. (2017). Microplastics – occurrence, fate and behaviour in the environment. Charact. Analysis Microplastics Compr. Anal. Chem., 1–24. doi:10.1016/bs.coac.2016.10.004
Department of Fisheries and Oceans Canada (2021). Microplastics. Available at: https://www.dfo-mpo.gc.ca/science/environmental-environnement/microplastics-microplastiques/index-eng.html.
Devereux, R., Westhead, E. K., Jayaratne, R., and Newport, D. (2022). Microplastic abundance in the thames river during the new year period. Mar. Pollut. Bull. 177, 113534. doi:10.1016/j.marpolbul.2022.113534
Ding, J., Sun, C., He, C., Li, J., Ju, P., and Li, F. (2021). Microplastics in four bivalve species and basis for using bivalves as bioindicators of microplastic pollution. Sci. Total Environ. 782, 146830. doi:10.1016/j.scitotenv.2021.146830
Do, A. T. N., Kim, Y., Ha, Y., and Kwon, J. H. (2022). Estimating the bioaccumulation potential of hydrophobic ultraviolet stabilizers using experimental partitioning properties. Int. J. Environ. Res. Pub. 19 (7), 3989. doi:10.3390/ijerph19073989
Enders, E. C., and Scruton, D. A. (2006). Potential application of bioenergetics models to habitat modeling and importance of appropriate metabolic rate estimates with special consideration for Atlantic salmon. Can. Tech. Rep. Fish. Aquat. Sci. 2641, 40.
Environment and Climate Change Canada (2018). “Canadian environmental protection act, 1999,” in Federal environmental quality guidelines (tech.). Available at: https://www.canada.ca/content/dam/eccc/documents/pdf/pded/bpa/20180626-BPA-EN.pdf.
Environment and Climate Change Canada (2020). Canada gazette, Part I, volume 154, number 41: order adding a toxic substance to schedule 1 to the Canadian environmental protection act, 1999 (tech.). Available at: https://gazette.gc.ca/rp-pr/p1/2020/2020-10-10/html/reg1-eng.html.
Environment and Climate Change Canada (2021). Toxic substances list: schedule 1 (Tech.). Available at: https://www.canada.ca/en/environment-climate-change/services/canadian-environmental-protection-act-registry/substances-list/toxic/schedule-1.html.
EPI SUITE (2021). EPI SUITE™ for Microsoft® Windows, version 4.11. US EPA. Washington, DC: United States Environmental Protection Agency. Available at: https://www.epa.gov/tsca-screening-tools/epi-suitetm-estimation-program-interface (Accessed September 02, 2021).
Espinosa, C., Esteban, M. Á., and Cuesta, A. (2016). Microplastics in aquatic environments and their toxicological implications for fish. Toxicol. - New Aspects This Sci. Conundrum, 113–145. doi:10.5772/64815
European Centre for Ecotoxicology and Toxicology of Chemicals (1998). QSARs in the assessment of environmental fate and effects of chemicals; tech. Report No. 74; European Centre for Ecotoxicology and Toxicology of chemicals: Brussels, Belgium. Available at: https://www.ecetoc.org/wp-content/uploads/2014/08/ECETOC-TR-074.pdf (Accessed January 21, 2022).
European Centre for Ecotoxicology and Toxicology of Chemicals (2000). Persistent organic pollutants (POPs) response to UNEP/INC/CEG-I annex 1; tech. Report No. 41; European Centre for Ecotoxicology and Toxicology of chemicals: Brussels, Belgium. Available at: https://www.ecetoc.org/wp-content/uploads/2014/08/DOC-0412.pdf.
European Chemical Agency (2022). European chemical agency. Available at: http://echa.europa.eu/ (Accessed March 20, 2022).
European Chemicals Agency (2014). Dibutyl phthalate (DBP) (support document). Available at: https://echa.europa.eu/documents/10162/e4edaefa-84a4-4972-89f0-470cd64bc949.
Flick, E. W. (2001). Plastics additives: an industrial guide. 3rd ed., vol. 1. Norwich, New York, United States: William Andrew Publishing.
Fries, E., Dekiff, J. H., Willmeyer, J., Nuelle, M., Ebert, M., and Remy, D. (2013). Identification of polymer types and additives in marine microplastic particles using pyrolysis-GC/MS and scanning electron microscopy. Environ. Sci. Process. and Impacts 15 (10), 1949. doi:10.1039/c3em00214d
Gallo, F., Fossi, C., Weber, R., Santillo, D., Sousa, J., Ingram, I., et al. (2018). Marine litter plastics and microplastics and their toxic chemicals components: the need for urgent preventive measures. Environ. Sci. Eur. 30 (1), 13. doi:10.1186/s12302-018-0139-z
Gillespie, G., Rusch, B., Gormican, S. J., Marshall, R., and Munroe, D. (2001). Further investigations of the Fisheries potential of the exotic varnish clam (Nuttallia obscurata) in British Columbia. CSAS research document. Fish. Oceans Can. Ott. (ONT).
Gillespie, G. E., Parker, M., and Merilees, W. (1999). Distribution, abundance, biology and Fisheries potential of the exotic varnish clam (Nuttallia obscurata) in British Columbia. Canadian science advisory secretariat [CSAS] research document. Fish. Oceans Can. Ott. (ONT).
Gobas, F. A. (2001). “Assessing bioaccumulation factors of persistent organic pollutants in aquatic food-chains,” in Persistent organic pollutants (Springer), 145–165. doi:10.1007/978-1-4615-1571-5_6
Gobas, F. A. P. C., and Morrison, H. A. (2000). Bioconcentration and biomagnification in the aquatic environment. Handb. Prop. Estim. methods Chem., 189–231.
Goldberg, A. M., and Frazier, J. M. (1989). Alternatives to animals in toxicity testing. Sci. Am. 261 (2), 24–30. doi:10.1038/scientificamerican0889-24
Google Earth Pro 7.3.6.9796 (2024). Burrard inlet British Columbia 49°18' 9.77" N, 123°3' 59.5" W, elevation 0m. Available at: http://www.google.com/earth/index.html (Accessed May 15, 2024).
Greenpeace Research Laboratories (2019) “Identification of polymer type used for a selection of organic-certified tampon applicators and their packaging on sale in the UK,”. England: Greenpeace Research Laboratories School of Biosciences, 1–9. Rep. No. GRL-AR-2019-04).
Hale, R. C., Seeley, M. E., La Guardia, M. J., Mai, L., and Zeng, E. Y. (2020). A global perspective on microplastics. J. Geophys. Res. Oceans 125, e2018JC014719. doi:10.1029/2018JC014719
A. W. Hayes, and C. L. Kruger (2014). Hayes' principles and methods of Toxicology. 6th ed. (Boca Raton, Florida, United States: CRC Press).
Hermabessiere, L., Dehaut, A., Paul-Pont, I., Lacroix, C., Jezequel, R., Soudant, P., et al. (2017). Occurrence and effects of plastic additives on marine environments and organisms: a review. Chemosphere 182, 781–793. doi:10.1016/j.chemosphere.2017.05.096
Hermabessiere, L., Paul-Pont, I., Cassone, A. L., Himber, C., Receveur, J., Jezequel, R., et al. (2019). Microplastic contamination and pollutant levels in mussels and cockles collected along the channel coasts. Environ. Pollut. 250, 807–819. PMID: 31039474. doi:10.1016/j.envpol.2019.04.051
Hidalgo-Ruz, V., Gutow, L., Thompson, R. C., and Thiel, M. (2012). Microplastics in the marine environment: a review of the methods used for identification and quantification. Environ. Sci. Technol. 46 (6), 3060–3075. PMID: 22321064. doi:10.1021/es2031505
Hollenberg, E. J., and Demers, E. (2017). Black oystercatcher (Haematopus bachmani) foraging on varnish clams (Nuttallia obscurata) in Nanaimo. Br. Columbia. B. C. Birds 27 (7).
Huffman Ringwood, A. (2021). Bivalves as biological sieves: bioreactivity pathways of microplastics and nanoplastics. Biol. Bull. 241 (2), 185–195. doi:10.1086/716259
Khan, F. R., Syberg, K., Shashoua, Y., and Bury, N. R. (2015). Influence of polyethylene microplastic beads on the uptake and localization of silver in zebrafish (Danio rerio). Environ. Pollut. 206, 73–79. doi:10.1016/j.envpol.2015.06.009
Knutsen, H., Singdahl-Larsen, C., and Bonnevie Cyvin, J. (2019). MPs in svalbard fjords and bjørnøy transect sediments; Tech. report No. 20190263-01-R; Oslo, Norway: Norwegian Geotechnical Institute. Available at: https://www.ngu.no/upload/Publikasjoner/Rapporter/2019/2019_027.pdf (Accessed June 23, 2021)
Koelmans, A. A., Besseling, E., and Foekema, E. M. (2014). Leaching of plastic additives to marine organisms. Environ. Pollut. 187, 49–54. doi:10.1016/j.envpol.2013.12.013
Laist, D. W. (1997). “Impacts of marine debris: entanglement of marine life in marine debris including a comprehensive list of species with entanglement and ingestion records,” in Marine debris. Editors J. M. Coe, and D. B. Rogers (New York: Springer), 99–139.
Lambert, S., Sinclair, C., and Boxall, A. (2013). Occurrence, degradation, and effect of polymer-based materials in the environment. Rev. Environ. Contam. Toxicol. 227, 1–53. doi:10.1007/978-3-319-01327-5_1
Lapenna, S., Fuart-Gatnik, M., and Worth, A. (2010). Review of QSAR models and software tools for predicting acute and chronic systemic toxicity. Luxembourg: Publications Office of the European Union. Tech. report EUR 24639 EN.
Law, K. L. (2017). Plastics in the marine environment. Annu. Rev. Mar. Sci. 9 (1), 205–229. doi:10.1146/annurev-marine-010816-060409
Lewis, T. L., Esler, D., and Boyd, W. S. (2008). Foraging behavior of surf scoters (Melanitta perspicillata) and white-winged scoters (M. Fusca) in relation to clam density: inferring food availability and habitat quality. Auk 125 (1), 149–157. doi:10.1525/auk.2008.125.1.149
Lithner, D., Larsson, Å., and Dave, G. (2011a). Environmental and health hazard ranking and assessment of plastic polymers based on chemical composition. Sci. Total Environ. 409 (18), 3309–3324. doi:10.1016/j.scitotenv.2011.04.038
Lithner, D., Nordensvan, I., and Dave, G. (2011b). Comparative acute toxicity of leachates from plastic products made of polypropylene, polyethylene, PVC, acrylonitrile–butadiene–styrene, and epoxy to Daphnia magna. Environ. Sci. Pollut. Res. 19 (5), 1763–1772. doi:10.1007/s11356-011-0663-5
Liu, R., and Mabury, S. A. (2020). Synthetic phenolic antioxidants: a review of environmental occurrence, fate, human exposure, and toxicity. Environ. Sci. and Technol. 54 (19), 11706–11719. doi:10.1021/acs.est.0c05077
Liu, Z., Wang, H., and Zhang, S. (2022). An enhanced risk assessment framework for MPs occurring in the Westerscheldt estuary. Sci. Total Environ., 153006. doi:10.1016/j.scitotenv.2022.153006
Luan, L., Wang, X., Zheng, H., Liu, L., Luo, X., and Li, F. (2019). Differential toxicity of functionalized polystyrene microplastics to clams (Meretrix meretrix) at three key development stages of life history. Mar. Pollut. Bull. 139, 346–354. doi:10.1016/j.marpolbul.2019.01.003
Lusher, A. (2015). “Microplastics in the marine environment: distribution, interactions and effects,” in Marine anthropogenic litter. Editors M. Bergmann, L. Gutow, and M. Klages (Cham: Springer). doi:10.1007/978-3-319-16510-3_10
Madden, J. C., Enoch, S. J., Paini, A., and Cronin, M. T. (2020). A review of in silico tools as alternatives to animal testing: principles, resources and applications. Altern. Lab. Anim. 48 (4), 146–172. doi:10.1177/0261192920965977
Maes, T., Jessop, R., Wellner, N., Haupt, K., and Mayes, A. G. (2017). A rapid-screening approach to detect and quantify microplastics based on fluorescent tagging with Nile Red. Sci. Rep. 7 (1), 44501. doi:10.1038/srep44501
McEntee, T. C. (1990) Compositions containing antimicrobial agents in combination with stabilizers, 4 891 391. Chicago, Illinois, United States: US.
MestReNova (2021). Mestrelab Research S.L.: Santiago de Compostela. Version 14.2.1. Spain. Available at: https://mestrelab.com/ (Accessed August 29, 2021).
Miller, M. E., Kroon, F. J., and Motti, C. A. (2017). Recovering microplastics from marine samples: a review of current practices. Mar. Pollut. Bull. 123 (1-2), 6–18. doi:10.1016/j.marpolbul.2017.08.058
Moore, C. J. (2008). Synthetic polymers in the marine environment: a rapidly increasing, long-term threat. Environ. Res. 108 (2), 131–139. doi:10.1016/j.envres.2008.07.025
Naik, V., and Patil, K. C. (2015). High energy materials. Resonance 20 (5), 431–444. doi:10.1007/s12045-015-0200-9
Nelms, S. E., Barnett, J., Brownlow, A., Davison, N. J., Deaville, R., Galloway, T. S., et al. (2019). MPs in marine mammals stranded around the British coast: ubiquitous but transitory? Sci. Rep. 9 (1), 1075. doi:10.1038/s41598-018-37428-3
OECD (2022). OECD quantitative structure-activity relationships Project [(Q)SARs]. Available at: https://www.oecd.org/chemicalsafety/risk-assessment/oecdquantitativestructure-activityrelationshipsprojectqsars.html (Accessed March 16, 2022).
O. Olabisi, and K. Adewale (2016). Handbook of thermoplastics (Boca Raton, Florida, United States: CRC Press), 41.
Organisation for Economic Co-operation and Development (OECD) (2004). “Emission scenario document on plastic additives. Series on emission scenario documents, No. 3,” in OECD environmental health and safety publications (Paris: Environment Directorate).
Organisation for Economic Co-operation and Development (OECD) (2024). OECD quantitative structure-activity relationships Project [(Q)SARs]. Available at: https://www.oecd.org/chemicalsafety/risk-assessmentoecdquantitativestructureactivityrelationshipsprojectqsars.html.
Organisation for Economic Co-operation and Development (OECD) Website (1984). OECD guidelines for the testing of chemicals, section 2 test No. 204: fish, prolonged toxicity test: 14-day study. Paris, France: OECD Publishing. doi:10.1787/9789264069985-en
Organisation for Economic Co-operation and Development (OECD) Website (1992). OECD guidelines for the testing of chemicals, section 3 test No. 301: ready biodegradability. Paris, France: OECD Publishing. doi:10.1787/9789264070349-en
Organisation for Economic Co-operation and Development (OECD) Website (1995a). OECD guidelines for the testing of chemicals, section 1 test No. 105: water solubility. Paris, France: OECD Publishing. doi:10.1787/9789264069589-en
Organisation for Economic Co-operation and Development (OECD) Website (1995b). OECD guidelines for the testing of chemicals, section 1 test No. 107: partition coefficient (n-octanol/water): shake flask method. Paris, France: OECD Publishing. doi:10.1787/9789264069626-en
Organisation for Economic Co-operation and Development (OECD) Website (2009). OECD guidelines for the testing of chemicals, section 2 test No. 230: 21-day fish assay. A short-term screening for oestrogenic and androgenic activity, and aromatase inhibition. Paris, France: OECD Publishing. doi:10.1787/9789264076228-en
Organisation for Economic Co-operation and Development (OECD) Website (2011). OECD guidelines for the testing of chemicals, section 2 test No. 201: freshwater alga and cyanobacteria, growth inhibition test. Paris, France: OECD Publishing. doi:10.1787/9789264069923-en
Organisation for Economic Co-operation and Development (OECD) Website (2012). OECD guidelines for the testing of chemicals, section 2 test No. 211: Daphnia magna reproduction test. Paris, France: OECD Publishing. doi:10.1787/9789264185203-en
Organisation for Economic Co-operation and Development (OECD) Website (2013). OECD guidelines for the testing of chemicals, section 2 test No. 210: fish, early-life stage toxicity test. Paris, France: OECD Publishing. doi:10.1787/9789264203785-en
Organisation for Economic Co-operation and Development (OECD) Website (2022a). OECD guidelines for the testing of chemicals, section 1 test No. 117: partition coefficient (n-octanol/water), HPLC method. Paris, France: OECD Publishing. doi:10.1787/9789264069824-en
Organisation for Economic Co-operation and Development (OECD) Website (2022b). OECD guidelines for the testing of chemicals, section 1 test No. 123: partition coefficient (1-octanol/water): slow-stirring method. Paris, France: OECD Publishing. doi:10.1787/9789264015845-en
Palm, E. C., Esler, D., Anderson, E. M., and Wilson, M. T. (2012). Geographic and temporal variation in diet of wintering white-winged scoters. Waterbirds 35, 577–589. doi:10.1675/063.035.0407
Plastic Soup Foundation (2021). Canada declares plastic toxic and plans to ban SUP items. Available at: https://www.plasticsoupfoundation.org/en/2021/06/canada-declares-plastic-toxic-and-plans-to-ban-sup-items/.
Provencher, J. F., Bond, A. L., Hedd, A., Montevecchi, W. A., Muzaffar, S. B., Courchesne, S. J., et al. (2014). Prevalence of marine debris in marine birds from the North Atlantic. Mar. Pollut. Bull. 84, 411–417. doi:10.1016/j.marpolbul.2014.04.044
Rochman, C. M. (2015). “The complex mixture, fate and toxicity of chemicals associated with plastic debris in the marine environment,” in Marine anthropogenic litter. Editors M. Bergmann, L. Gutow, and M. Klages (Berlin: Springer), 117–140.
Savoca, M. S., Mcinturf, A. G., and Hazen, E. L. (2021). Plastic ingestion by marine fish is widespread and increasing. Glob. Change Biol. 27 (10), 2188–2199. doi:10.1111/gcb.15533
Senathirajah, K., Attwood, S., Bhagwat, G., Carbery, M., Wilson, S., and Palanisami, T. (2021). Estimation of the mass of microplastics ingested–A pivotal first step towards human health risk assessment. J. Hazard. Mater. 404, 124004. doi:10.1016/j.jhazmat.2020.124004
Simmons, B. L., Sterling, J., and Watson, J. C. (2014). Species and size-selective predation by raccoons (Procyon lotor) preying on introduced intertidal clams. Can. J. zoology 92 (12), 1059–1065. doi:10.1139/cjz-2014-0108
Solvay. 2020. CYANOX®. Available at: https://www.solvay.com/en/brands/cyanox (Accessed May/01/2024).
Sun, J., Su, F., Chen, Y., Wang, T., Ali, W., Jin, H., et al. (2024). Co-exposure to PVC microplastics and cadmium induces oxidative stress and fibrosis in duck pancreas. Sci. Total Environ. 927, 172395. doi:10.1016/j.scitotenv.2024.172395
Teuten, E. L., Saquing, J. M., Knappe, D. R., Barlaz, M. A., Jonsson, S., Björn, A., et al. (2009). Transport and release of chemicals from plastics to the environment and to wildlife. Philosophical Trans. R. Soc. B Biol. Sci. 364 (1526), 2027–2045. doi:10.1098/rstb.2008.0284
Thiele, C. J., Hudson, M. D., and Russell, A. E. (2019). Evaluation of existing methods to extract microplastics from bivalve tissue: adapted KOH digestion protocol improves filtration at single-digit pore size. Mar. Pollut. Bull. 142, 384–393. doi:10.1016/j.marpolbul.2019.03.003
Thompson, R. C. (2015). “Microplastics in the marine environment: sources, consequences and solutions,” in Marine anthropogenic litter. Editors M. Bergmann, L. Gutow, and M. Klages (Cham: Springer). doi:10.1007/978-3-319-16510-3_7
Toader, G., Rotariu, T., Rusen, E., Tartiere, J., Esanu, S., Zecheru, T., et al. (2017). New solvent-free polyurea binder for plastic pyrotechnic compositions. Mater. Plast. 54 (1), 22–28. doi:10.37358/MP.17.1.4777
Trevail, A. M., Gabrielsen, G. W., Kühn, S., and Van Franeker, J. A. (2015). Elevated levels of ingested plastic in a high Arctic seabird, the northern fulmar (Fulmarus glacialis). Polar Biol. 38, 975–981. doi:10.1007/s00300-015-1657-4
United States Environmental Protection Agency (2021). KABAM version 1.0 user's guide and technical documentation - appendix F -description of equations used to calculate the BCF, BAF, BMF, and BSAF values. Available at: https://www.epa.gov/pesticide-science-and-assessing-pesticide-risks/kabam-version-10-users-guide-and-technical-3#F1 (Accessed March 20, 2022).
van Alphen, J., and van Turnhout, C. M. (1973). Rubber chemicals. Dordrecht Reidel Publishing Company.
van Moos, N., Burkhardt-Holm, P., and Köhler, A. (2012). Uptake and effects of microplastics on cells and tissue of the blue mussel, Mytilus edulis L. After an experimental exposure. Environ. Sci. and Technol. 46 (20), 11327–11335. doi:10.1021/es302332w
Wang, L., Stephen, A. R., Boothe, P. W., Schulze, T., Giardello, M. A., Trimmer, M. S., et al. (2019). Adhesion promoters and gel-modifiers for olefin metathesis compositions. US 10 457 597.
Watts, A. J., Lewis, C., Goodhead, R. M., Beckett, S. J., Moger, J., Tyler, C. R., et al. (2014). Uptake and retention of microplastics by the shore crab Carcinus maenas. Environ. Sci. and Technol. 48 (15), 8823–8830. doi:10.1021/es501090e
Wiley, J. (2021). CYANOX 53. Available at: https://spectrabase.com/spectrum/D4wMn8dQ8OL.
Wright, S. L., Rowe, D., Thompson, R. C., and Galloway, T. S. (2013b). Microplastic ingestion decreases energy reserves in marine worms. Curr. Biol. 23 (23), R1031–R1033. doi:10.1016/j.cub.2013.10.068
Yahya, H., Karim, S. N., Yahaya, N., Syed Abd Halim, S. A. S., Zanuari, F. I., and Yahya, H. N. Occurrence and pathways of microplastics, quantification protocol and adverseeffects of microplastics towards freshwater and seawater biota. Food Res. 2023, 7, 164–180. doi:10.26656/fr.2017.7(5).1335).133
Keywords: plastic additive, microplastics, Cyanox®53, environmental fate, toxicity, EPI suite TM, scoter duck bioenergetic model, varnish clams
Citation: Renkers SL and Bendell LI (2024) Investigating the ecological and toxicological significance of Cyanox®53 recovered from intertidal sediments and varnish clam. Front. Environ. Sci. 12:1439573. doi: 10.3389/fenvs.2024.1439573
Received: 28 May 2024; Accepted: 07 August 2024;
Published: 26 August 2024.
Edited by:
Nsikak U. Benson, Topfaith University, NigeriaReviewed by:
Youji Wang, Shanghai Ocean University, ChinaCopyright © 2024 Renkers and Bendell. This is an open-access article distributed under the terms of the Creative Commons Attribution License (CC BY). The use, distribution or reproduction in other forums is permitted, provided the original author(s) and the copyright owner(s) are credited and that the original publication in this journal is cited, in accordance with accepted academic practice. No use, distribution or reproduction is permitted which does not comply with these terms.
*Correspondence: Stephanie L. Renkers, c3JlbmtlcnNAc2Z1LmNh
Disclaimer: All claims expressed in this article are solely those of the authors and do not necessarily represent those of their affiliated organizations, or those of the publisher, the editors and the reviewers. Any product that may be evaluated in this article or claim that may be made by its manufacturer is not guaranteed or endorsed by the publisher.
Research integrity at Frontiers
Learn more about the work of our research integrity team to safeguard the quality of each article we publish.