- 1Key Laboratory of Mountain Surface Processes and Ecological Regulation, Institute of Mountain Hazards and Environment, Chinese Academy of Sciences, Chengdu, Sichuan, China
- 2University of Chinese Academy of Sciences, Beijing, China
Introduction: Permafrost and seasonally frozen soil are widely distributed on the Qinghai–Tibetan Plateau, and the freezing–thawing cycle can lead to frequent phase changes in soil water, which can have important impacts on ecosystems.
Methods: To understand the process of soil freezing-thawing and to lay the foundation for grassland ecosystems to cope with complex climate change, this study analyzed and investigated the hydrothermal data of Xainza Station on the Northern Tibet from November 2019 to October 2021.
Results and Discussion: The results showed that the fluctuation of soil temperature showed a cyclical variation similar to a sine (cosine) curve; the deep soil temperature change was not as drastic as that of the shallow soil, and the shallow soil had the largest monthly mean temperature in September and the smallest monthly mean temperature in January. The soil water content curve was U-shaped; with increased soil depth, the maximum and minimum values of soil water content had a certain lag compared to that of the shallow soil. The daily freezing-thawing of the soil lasted 179 and 198 days and the freezing-thawing process can be roughly divided into the initial freezing period (November), the stable freezing period (December–early February), the early ablation period (mid-February to March), and the later ablation period (March–end of April), except for the latter period when the average temperature of the soil increased with the increase in depth. The trend of water content change with depth at all stages of freezing-thawing was consistent, and negative soil temperature was one of the key factors affecting soil moisture. This study is important for further understanding of hydrothermal coupling and the mechanism of the soil freezing-thawing process.
1 Introduction
Frozen soil is widely distributed in the northern hemisphere which is generally divided into short-time frozen soil, seasonal frozen soil, and permafrost (Zhang et al., 2019). In China, frozen soil is widely distributed in the Northeast, Northwest, and Qinghai–Tibetan Plateau, among which the seasonal frozen soil area accounts for approximately 46.3% of the national land area (Du, 2020). The diurnal and seasonal changes of heat lead to repeated freezing and thawing of soil liquid water at a certain depth (Chen et al., 2020), a process called soil freeze-thaw, which leads to frequent phase transitions of soil water (Liu et al., 2009), thus changing the thermodynamics, structure, and properties of soil. Soil freeze-thaw cycle has a far-reaching influence on the study of biogeochemical cycles (Zhou et al., 2008; Wu and Shen, 2010; Cao et al., 2021), hydrological processes (Jiang et al., 2018; Wang et al., 2021), and ecosystems (Zhang et al., 2012; Sanders-DeMott et al., 2018).
Foreign freeze-thaw studies mainly focus on Arctic and sub-Arctic regions, such as the tundra belt of northern Alaska (Ling and Zhang, 2006; Jiang et al., 2012; Yi et al., 2019; Douglas et al., 2020), near Abisko, northern Sweden (Larsen et al., 2002; Blume-Werry et al., 2019) and the Canadian Arctic (Kumar et al., 2013). These studies have discussed the effects of freeze-thaw cycles on soil bacterial communities (Larsen et al., 2002; Kumar et al., 2013; Lim et al., 2020), and the effects of plant root dynamics (Blume-Werry et al., 2019) on climate (heat and precipitation) (Jiang et al., 2012; Douglas et al., 2020) and snow cover (Ling and Zhang, 2006; Yi et al., 2019) influence the thickness of the active layer. Research on seasonal frozen soil in China is concentrated in the Northeast (Zhao et al., 2020; Liu et al., 2021), Northwest China (Chen et al., 2019), and the Tibetan Plateau regions. Known as the third pole of the earth, the Qinghai–Tibetan Plateau is a high-altitude region with strong solar radiation, widespread distribution of permafrost and seasonal frozen soil, and a fragile and sensitive ecosystem. In recent decades, the alpine grassland on the Qinghai–Tibetan Plateau has been extensively degraded due to climate change, overgrazing, and anthropogenic activities, resulting in changes in soil hydrothermal properties (Han et al., 2018), which further affected the soil freeze-thaw process. At present, the soil freeze-thaw process in permafrost regions and seasonally frozen ground regions has become one of the regional hot spots. In the past 20 years, some scholars have conducted regional or individual site analysis and research on the Qinghai–Tibetan Plateau based on site observation data. Data were obtained from Haibei Station (Dai et al., 2020), Tanggula Station (Zhao et al., 2021), Xainza Station (Fan et al., 2014; Li et al., 2014; Ran et al., 2019), the Qilian Mountains (Huang et al., 2023), and the Qinghai-Tibet Railway (Yang et al., 2000) and a comparison was conducted between multiple stations (Yang and Ma, 2012). Regarding the changes of soil freezing and thawing processes in the entire region of the Qinghai–Tibetan Plateau, many scholars have also compared the relationship between soil freezing and thawing processes and climate, hydrological processes, and vegetation in the Tibetan Plateau or localized areas through remote sensing observations (Chen et al., 2014; Zhao et al., 2019; Fu et al., 2022). In addition, progress has been made regarding research on the coupling of water and heat in the permafrost of the Qinghai–Tibetan Plateau. Hu et al. (2014) found a certain correlation between unfrozen water content and negative temperature by analyzing the active layer in the permafrost region of the Qinghai–Tibetan Plateau, and that the correlation becomes better as the depth increases. Wan (2012) analyzed the freeze-thaw process of different stations in the middle of the Qinghai–Tibetan Plateau and observed regional differences in the freeze-thaw process and hydrothermal status of soil at different stations. Dai et al. (2020) analyzed the characteristics of hydrothermal transport during the freeze-thaw process of seasonal frozen soil at Haibei Station and noticed that the freeze-thaw law of seasonal frozen soil was different from that of permafrost. Seasonal frozen soil showed characteristics of unidirectional freezing and bidirectional thawing and the correlation between unfrozen water content and soil temperature at each layer became weaker as the depth increased. However, the fitting effect was only a preliminary understanding of the law of hydrothermal transport at a single station for 1 year. Many scholars have conducted detailed analysis and discussion on the variation and coupling of water and heat in the freeze-thaw process. However, inter-annual climate fluctuations may lead to different freezing depths and degrees and the hydrothermal migration rules in the seasonal frozen soil region of the Qinghai–Tibetan Plateau were quite different. Therefore, long-term site observation data need to be clarified at this stage to provide data support for soil hydrothermal coupling simulation.
Taking the Xainza Alpine Grassland and Wetland Ecosystem Observation and Experiment Station of the Chinese Academy of Sciences as an example, this study analyzed the hydrothermal data from November 2019 to October 2021 to explore the hydrothermal characteristics of seasonal frozen soil, providing a theoretical basis for the freeze-thaw cycle changes under global changes and the optimal management and utilization of water resources on the Qinghai–Tibetan Plateau.
2 Materials and methods
2.1 Study area
The Xainza Alpine Grassland and Wetland Ecosystem Observation and Experiment Station (30.95°N, 88.7°E) of the Chinese Academy of Sciences (hereinafter referred to as Xainza Station) is the Ecological Observation Station located at a highest altitude in the world (altitude of 4,730 m), in the middle of Tibet, between the Gangdise Mountains and the largest lake (Selincuo) in Tibet, and is mainly seasonally frozen soil. It belongs to the arid monsoon climate in the subcold region of the plateau, with an average annual temperature of 0.4°C and annual precipitation of 298.6 mm from 1971–2000. It is arid and rainless with uneven seasonal distribution. The precipitation is concentrated in May to September, the air is thin, and the winter is windy. The vegetation type is alpine grassland, mainly composed of Stipa purpurea and Carex moorcrofii.
2.2 Data collection and processing
The soil temperature and unfrozen water content data of Xainza Station were obtained from the frozen soil monitoring and disaster warning service platform. The composition of the platform observation system was shown in Table 1.
As Xainza Station is located in the hinterland of the Qinghai–Tibetan Plateau, with bad weather and damaged instruments, the platform only recorded soil temperature and unfrozen water content from 1 November 2019 to 18 October 2021, with a measurement depth of 0–80 cm at an interval of 2.5 cm and a measurement interval of 1 h. The temperature data was collected from the Tibet Ecological Environment and Development Research Office of the Institute of Mountain Hazards and Environment, CAS and the data from 27 April 2021 to 7 June 2021 were missing. The unfrozen water data used in the study refers to the total soil water content in the thawing period and excludes the ice content in the freezing period. The poor environment cannot avoid data loss, therefore, daily data with excessive data loss must be deleted to avoid data deviation (Wang et al., 2021). When the data loss of the day exceeds one-quarter, that is, less than 18, all the data of the day will be deleted. A total of 11 days of data were deleted and the remaining data was processed. The observed hourly temperature and humidity data were averaged to obtain daily temperature and humidity data, monthly data, and annual data.
Soil temperature is used to reflect the soil heat condition, and the area surrounded by 0°C contours on the contour diagram is used to reflect the freezing time and depth of the soil. When the daily minimum temperature of soil Tmin < 0°C and the daily maximum temperature of soil Tmax > 0°C, the daily freeze-thaw cycle of soil occurs (that is, the soil thaws during the day and freezes at night). If Tmax < 0°C of a soil layer, the soil is completely frozen. When the mean daily temperature of soil Tmean > 0°C and Tmin < 0°C, the soil enters the ablation stage. If the Tmin> 0°C of a soil layer, that soil layer thaws completely (Yang et al., 2006). According to this, the freeze-thaw cycle stages were divided and the changes of water and heat in each stage were analyzed. Since the data changes of temperature and moisture were consistent, to simplify the analysis, the interval between the data of soil temperature and unfrozen water content was set to 10 cm, and the daily changes of the freeze-thaw process were determined by studying the changes in temperature and moisture in shallow soil. Thus, the data at 2.5 and 5 cm depth were added for analysis. Excel 2016 was used for data analysis and Origin 2,021 was used for plotting.
3 Results
3.1 Soil temperature change
The mean daily temperature during the monitoring period was 1.03°C, the maximum temperature was 14.62°C (25 July 2021), and the minimum temperature was −15.9°C (25 January 2020). Overall, the temperature from 2020–2021 was slightly higher than that in 2019–2020. The maximum daily surface soil temperature during the monitoring period was 20.82°C (7 August 2020) and the minimum was −10.8°C (24 January 2020). Figure 1 showed that the temperature and the daily average temperature of shallow soil fluctuated sharply, and the change in the daily average temperature of soil gradually flattened as the depth increased, indicating that the influence of air temperature on deep soil was less than that on shallow soil. Overall, the average daily temperature changes of air temperature and soil temperature at different depths were consistent, showing a trend of synchronous change. With time, the temperature fluctuation presented a periodic change that approximately formed a sine (cosine) curve. The maximum monthly mean temperature during the monitoring period was 16.38°C, which appeared in September 2020. The minimum value was −7.78°C, which occurred in January 2020. Consistent with the temperature, the average temperature of each depth in 2020–2021 was higher than that in 2019–2020. Figure 2 reveals that the temperature of each soil layer had obvious seasonal changes within the study time range and the soil profile at any depth had blue–green–yellow–orange (the redder the color, the hotter is the temperature; the bluer the color, the lower is the temperature) changes during the monitoring period, reflecting a cooling–warming–cooling process.
3.2 Change in soil water content
A close relationship occurred between soil water content and the freeze-thaw cycle, during which soil water changed phase constantly. The maximum daily water content during the monitoring period was 24.43%, which occurred on 2 November 2019. The soil below 20 cm has been frozen. The maximum monthly average moisture content was 21.01%, which occurred in November 2019. The moisture content of soil below 15 cm in 2020–2021 was higher than that in 2019–2020, because the temperature in 2020–2021 was higher, and the soil below 15 cm did not exhibit daily stable freezing and no water migration. The change of soil unfrozen water content in 2 years was shown in Figure 3. With an increase in soil depth, the soil moisture content increased first and then decreased, and the soil moisture content at 80 cm was the highest, which may be due to the fact that Xainza is located in the hinterland of the Qinghai–Tibetan Plateau, and is characterized by strong solar radiation and large evaporation of shallow soil moisture content, resulting in lower water content of shallow soil compared to deep soil. Overall, the soil moisture content experienced a gradual decrease–nadir–gradual increase, and the curve was U-shaped. With increased soil depth, the highest and lowest values of soil moisture content lagged compared with that of shallow soil.
3.3 Daily freeze-thaw process of soil
3.3.1 Division of soil freeze-thaw stage
According to the evaluation criteria (Yang et al., 2006), Table 2 showed the stages and duration of surface soil freeze-thaw during the study period (surface soil had the longest freeze-thaw cycle period). Xainza Station is in the seasonal frozen soil area, and exhibits a period of stable freezing in normal years. The precipitation decreased significantly (the least since 1981) and it was a regional strong warm winter, hence, the soil in Xainza Station of 2020–2021 did not have a stable freezing period in that year and was always in an unstable freeze-thaw period (Meteorological Bureau of the Tibet Autonomous Region, 2021; Meteorological Bureau of the Tibet Autonomous Region, 2022).
3.3.2 Variation characteristics of hydrothermal change during daily freeze-thaw cycle
To analyze the changes of water and heat in the freeze-thaw process, daily changes of soil water and heat in different stages were selected for analysis (Figure 4). After entering the initial freezing period, the soil temperature at 10 cm fluctuated to approximately 0°C, and the moisture content of shallow soil increased in the afternoon and decreased in the early morning, resulting in a daily freeze-thaw cycle (Figure 5). In the initial freezing period, the degree of soil freezing was weak. When the daily maximum temperature was lower than 0°C, the soil was completely frozen. At this time, as the temperature rose after sunrise, the temperature of shallow soil rose slightly; however, no thawing phenomenon leading to an increase in water content, occurred. After February, the temperature gradually rose and entered the early ablation stage. The temperature of the soil below 10 cm in the shallow layer fluctuated to approximately 0°C. The moisture content of the soil above 10 cm increased slightly and the soil below 10 cm exhibited a weak daily freeze-thaw cycle. When the temperature continued to rise and the average daily temperature was higher than 0°C, the ablation stage began. At this time, except for the temperature of the soil below 10 cm in the shallow layer, the temperature of other soils was higher than 0°C, and the moisture content of the soil above 10 cm further increased, and only the surface soil had a daily freeze-thaw cycle day and night.
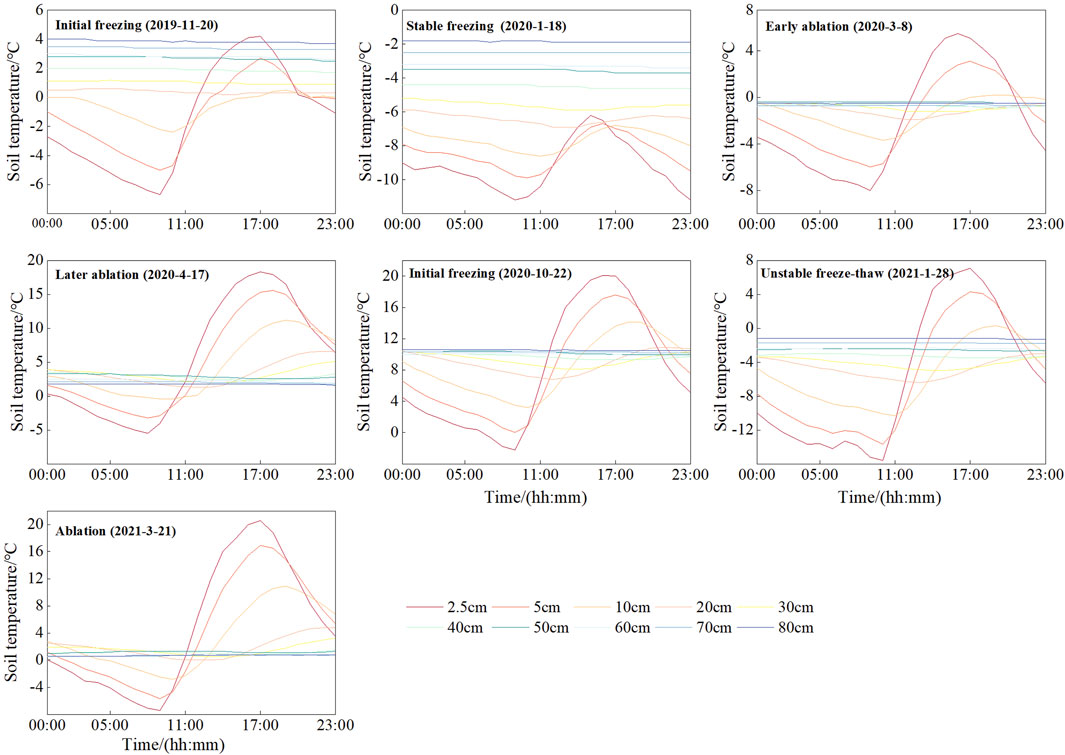
Figure 4. Daily variation of soil temperature in different soil layers during freezing-thawing stages from 2019 to 2021.
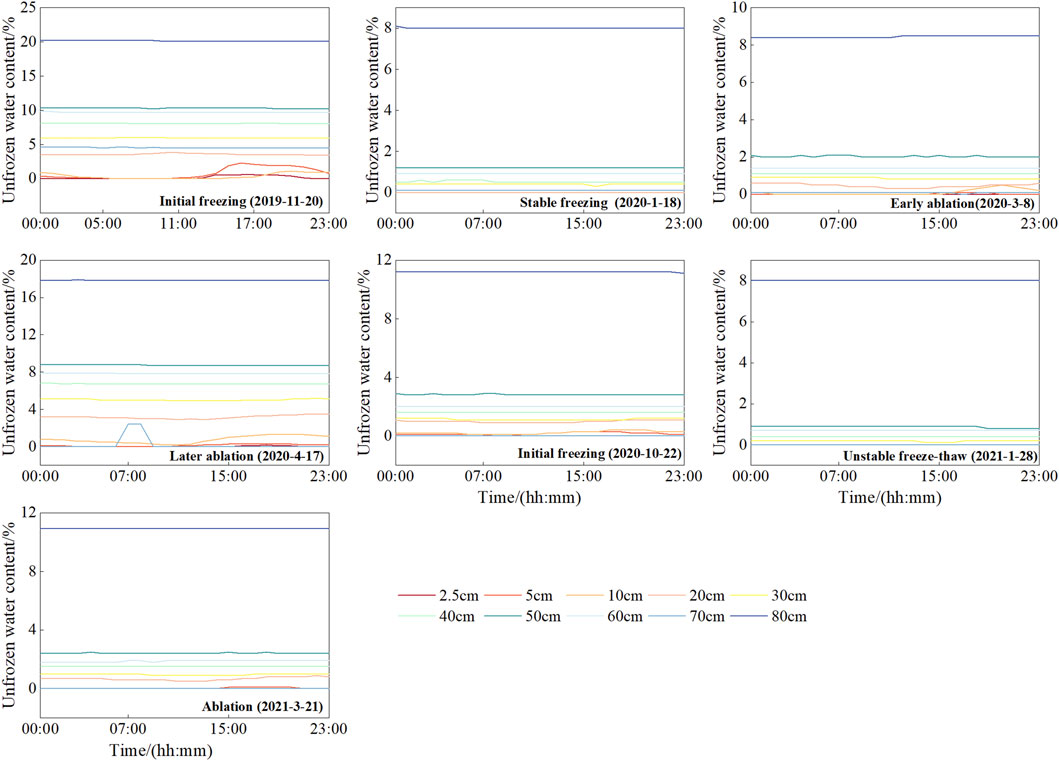
Figure 5. Daily variation of soil water content in different soil layers during freezing-thawing stages from 2019 to 2021.
3.3.3 Characteristics of hydrothermal change in the soil profile
To study the characteristics of the variations in hydrothermal change in soil profiles at different stages during the freeze-thaw process, the temperature and water content of soil profiles at different stages were analyzed. In 2019–2020, the surface layer of soil began to freeze from November 1 to February 10 of the next year and thawed completely at the end of April, lasting 179 days. In 2020–2021, freezing occurred from mid-October until the beginning of May of the next year, lasting 198 days.
As revealed by Figure 6A, the thickness of the frozen soil layer was approximately 15 cm in the initial freezing period (November). As the temperature decreased, the thickness of the frozen soil layer gradually increased. At 0–80 cm, the frozen soil layer was in the stable freezing and the early ablation periods. As the temperature increased, the surface soil began to thaw and the temperature of each layer increased relative to the period of stable freezing (unstable freeze-thaw period). The soil temperature increased with the increase of depth in the early freezing period, the stable freezing and the early ablation periods. In the later ablation period, the soil temperature decreased gradually with the increase of depth. The phase change of soil water during the freeze-thaw process redistributed the water content in the soil. It can be seen from Figure 6B that the variations in the water content at each stage of the freeze-thaw cycle in relation to depth was consistent, increasing first, then decreasing, and then increasing again with an increase in depth. Compared with the initial freezing period, the unfrozen water content of all soil layers decreased in the stable freezing period, however, increased in the thawing period. Figure 6B showed that the content of unfrozen water at a soil depth of 50 cm was higher than that in the surrounding soil, forming a water accumulation area. This may be because the water under the freezing front migrated to the freezing front under the action of a temperature gradient as the temperature dropped and the freezing depth increased (Taylor, et al., 1978). The unfrozen water content in the deep soil (70 cm) migrated to the frozen layer and the deep soil water content decreased.
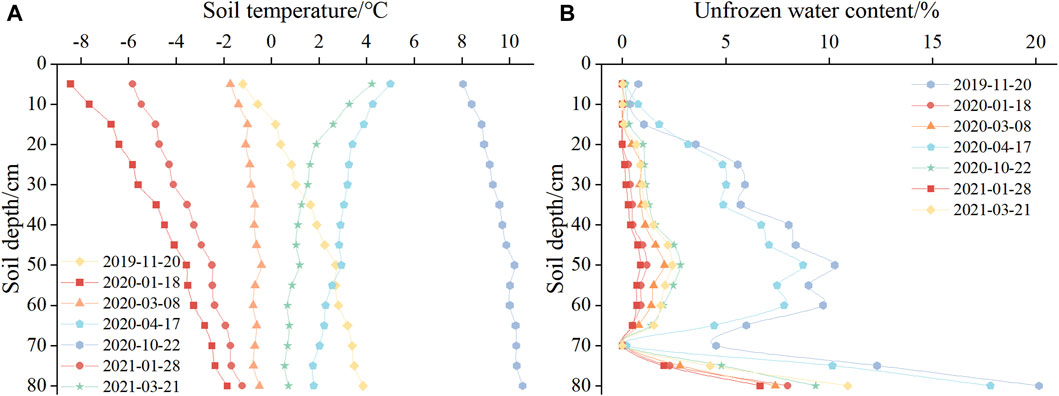
Figure 6. Profile of soil temperature (A) and water content (B) during the freezing-thawing period from 2019 to 2021.
3.3.4 Characteristics of soil hydrothermal coupling change
A correlation existed between the soil temperature at different depths and the content of unfrozen water during the freeze-thaw process (Table 3; Figures 7, 8). Although theoretically entire water freezes when the soil temperature is below 0°C, a fraction of the water remains liquid due to the influence of soil texture, salt, and other factors. Through the fitting of unfrozen water content and soil negative temperature, a certain dynamic equilibrium was observed between them, showing a power function relationship. With a decrease in soil temperature, part of the water also became frozen, and the final water content became close to 0, which indicated that the negative soil temperature is one of the key factors affecting the content of unfrozen water. The shallow soil was affected by the external meteorological environment and the deep unfrozen soil, hence, the fitting degree was poor and the fitting R2 value increases with increased depth. The fitting effect was poor at 50 and 80 cm, which may be related to the accumulation of water at 50 and 80 cm.
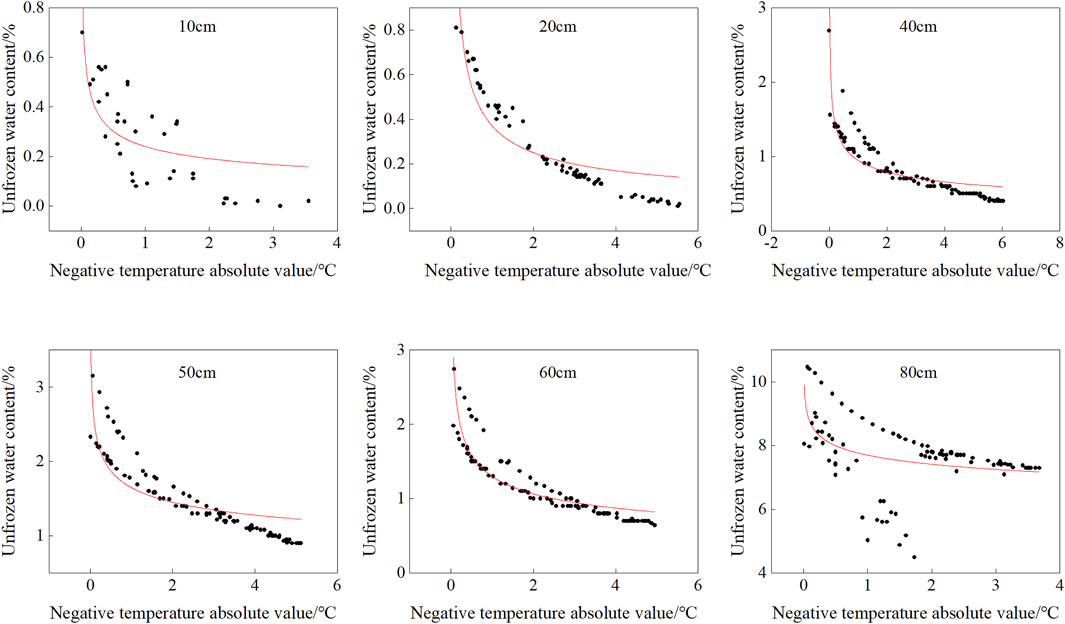
Figure 7. Relationship between absolute negative soil temperature and unfrozen water content in 2019–2020.
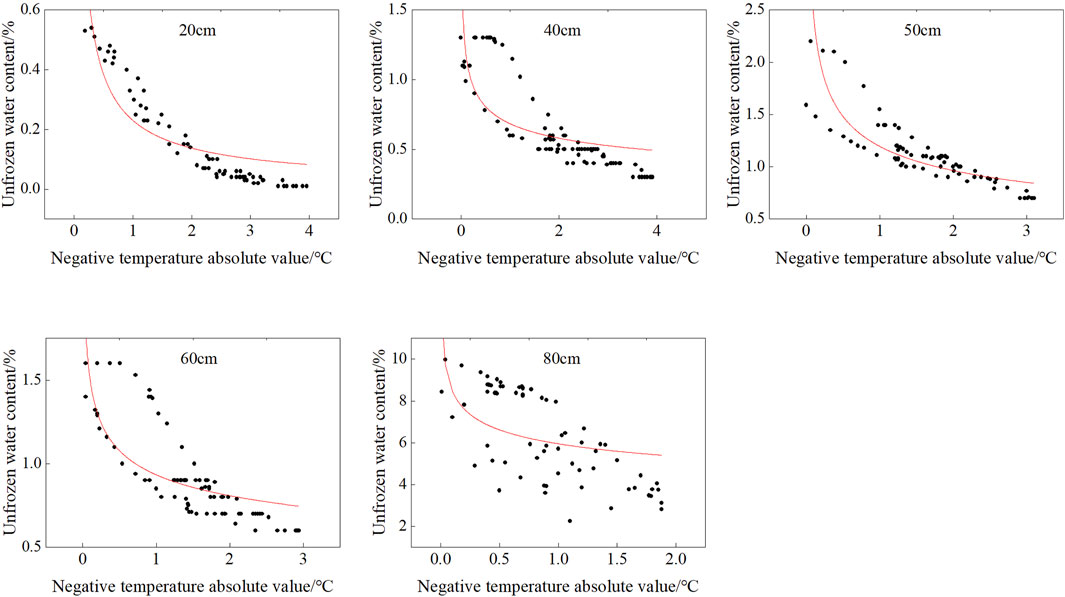
Figure 8. Relationship between absolute negative soil temperature and unfrozen water content in 2020–2021.
4 Discussion
Soil temperature and moisture are important variables in the study of land surface processes and will have a direct or indirect impact on climate change (Bai, 2020). On the Qinghai–Tibetan Plateau, soil freeze-thaw is conducive to maintaining the stability of grassland ecosystems (Wei et al., 2018). During the freeze-thaw process, the variation of soil water in all layers presented a similar trend, and the unfrozen water content of soil showed a “concave” graph. The soil water content changed steadily during the freezing period and was relatively large during the thawing period, which was consistent with the results of many researchers (Dai et al., 2020; Huang et al., 2023). Influenced by precipitation, evapotranspiration, surface runoff, and other factors, the variation range of soil water content gradually decreased with increased depth (Jiao et al., 2014).
The variation range of daily soil temperature during the freeze-thaw cycle gradually decreased with increased depth, because the shallow soil was more susceptible to external influences and had more energy exchange. With the increase in soil depth, the response of soil to atmospheric temperature gradually weakened, while the change trend of soil temperature in deep soil was gradually stable, and the daily soil temperature increased with the increase of soil depth. This was consistent with the findings of Wei et al. (2018), Li et al. (2014), and Huang et al. (2023). In the initial freezing period, most of the soil water existed in liquid form, while in the stable freezing period, the soil water content changed steadily. In the freezing process of soil, due to the influence of temperature potential on water transport, the frozen layer had a water-insulating effect, which caused the water to gather on the frozen floor; therefore, a high water value zone was formed in the deep layer. The water content of deep soil was higher than that of shallow soil (Dai et al., 2020). In the thawing stage, with the rise of air temperature, the closer the surface was, the greater the change in daily soil temperature. As the soil temperature rose, the soil began to thaw from top to bottom, the solid water in the soil transformed into liquid water, and the surface water content increased rapidly.
This study was consistent with that of Ran et al. (2019)and Fan et al. (2014). The maximum surface temperature of soil in the study year of Fan (2010–2011) was 10.48°C, the maximum temperature of soil in the study year of Ran (2015–2016) was 12.6°C, and the maximum daily temperature of soil in the study year of this paper was 20.82°C. The maximum temperature of soil in the study year of this paper increased significantly compared with that of previous studies; possibly because global warming was causing soil surface temperatures to rise. The duration of freeze-thaw in 2010–2011 was approximately 207 days, and the duration of freeze-thaw in 2015–2016 was 172 days. Compared with 179 and 198 days in this study, the duration of freeze-thaw in 2010–2011 was longer, and the duration of freeze-thaw in 2015–2016 was slightly shorter. A comparison of different stages of the freeze-thaw cycle showed that the initial freezing period was gradually longer, the time of stable freezing and early ablation was longer, and the time of late ablation was the shortest in 2015–2016 and the longest in 2010–2011. In 2016, the World Meteorological Organization (WMO) issued a bulletin showing that the 2015–16 EL Niño was one of the strongest on record and it is likely that El Niño caused high temperatures and short freeze-thaw periods in the study area (World Meteorological Organization, 2016). Excluding years with extreme weather events, from 2010 to 11 to 2020–21, the duration of freeze-thaw decreased, consistent with an increase in soil temperature, possibly caused by global warming.
Different geographical locations and other local factors also had an impact on the freeze-thaw process. Luo et al. (2014) studied the freeze-thaw process of the North field of Eling Lake (4,365 m), showing a pattern of unidirectional freezing and bidirectional thawing. The freeze-thaw duration of the north field of Eling Lake was 192 days, with freezing beginning in mid-to-late October and thawing beginning in early April. Although the altitude of Xainza Station was similar and the average annual temperature was higher than that of the north site of Eling Lake, the north site of Eling Lake had high silt content. The surface soil temperature rose obviously in the early thawing stage, and the thawing depth reached the deep soil faster. Therefore, although the thawing start time was later than that of this study, the overall freeze-thaw duration was similar. It was proved that different soil textures also affected the freeze-thaw process. Some studies have also shown that different slope orientation in the same region also have an impact on the freeze-thaw process. Studies on the freeze-thaw process of different slopes in the Anduo region have found that the freezing degree of the north slope was higher than that of the south slope at the initial stage of thawing and freezing, and the water content of the south slope was also higher than that of the north slope (Zhang et al., 2015). Freeze-thaw is also affected by plant types and cover conditions. Studies in permafrost areas have found that high vegetation cover will advance the time of soil freezing, delay the start time of soil thawing, and reduce the thickness of active layer. This was because swamps and wetlands with developed vegetation were rich in organic matter, which weakened the impact of solar radiation on the ground. It also increased heat dissipation after soil freezing (Wang et al., 2010; He et al., 2018; Dong et al., 2021). The active layer soil was found to freeze bidirectionally at the Tanggula Station, with the number of days of thawing more than those of freezing (Zhao et al., 2021). This was different from seasonally frozen soil that thawed bidirectionally, with the number of days of freezing more than those of thawing. It was due to the influence of the surface temperature and heat flow below the freeze depth in seasonally frozen soils.
Soil moisture and heat interact with each other, and several studies have shown that a certain dynamic coupling process occurs between soil temperature and moisture during the freeze-thaw process. Wang et al. (2012) took the D105 point on the Northern Tibet Plateau as an example and observed a decrease in temperature between unfrozen water content and temperature; the soil was frozen and the unfrozen water content was reduced. However, the conclusions drawn by Dai et al. (2020) and Huang et al. (2023) were contrary to the fitting results of Hu et al., suggesting that the fitting effect of shallow soil was higher than that of deep soil, possibly because too much water content approaching 0 affected the fitting effect. The power function fitting results conducted by Ran et al. (2019) on the water and heat of Xainza Station from 2015–2016 showed that the fitting effect became better as the depth increases, and the fitting effect was slightly worse for the 10 and 100 cm soil layers due to the influence of external meteorological conditions and the unfrozen soil below. This was significantly different from the fitting results in this paper and may be related to the fact that the strong El Niño event in 2015–2016 caused more than 20% more precipitation in Tibet in winter (Zhai et al., 2016). This study is only a preliminary understanding of hydrothermal coupling based on 2 years of hydrothermal data from Xainza Station. To reflect the whole hydrothermal coupling law, the hydrothermal coupling mechanism in the soil freeze-thaw process must be determined by combining long-term studies in the future.
The soil freeze-thaw cycle affects ecohydrological and surface processes and plays an important role in agriculture and the ecological environment (Qin et al., 2021). Soil freeze-thaw affected soil water storage, water transport, and infiltration capacity, resulting in water redistribution in the soil profile (Peng et al., 2016). Studies of the Kolyma River in northeastern Siberia have found that an increase in the active layer caused by permafrost warming inhibited summer river flow and reduced soil water stress in plants (Suzuki et al., 2021). As the water tower of Asia, the Tibetan Plateau is the main source of several rivers in Asia, and the freeze-thaw cycle of the soil on the Qinghai–Tibetan Plateau affects the water supply security of many rivers (Jiang et al., 2018). A study in the headwaters of the Yellow River on the Tibetan Plateau found that during soil thawing, liquid water storage increased with the water phase transition, and surface runoff did not change significantly with temperature increase until thawing was complete (Wu et al., 2018). The moisture anomaly in the previous fall on the Qinghai-Tibet Plateau was released when the soil thawed in spring, resulting in abnormal surface non-adiabatic heating, which further led to a decrease in summer precipitation in South China and the Yellow River Basin, and an increase in summer precipitation in the Yangtze River Basin and Northeast China (Yang and Wang, 2019). The interaction between soil freeze and thaw dynamics and soil water also affected plant growth. Soil evaporation in spring increased shallow water loss, which led to a decrease in plant rhizosphere water, further hindering plant germination and growth (He et al., 2024). In this study, only the changes in soil temperature and moisture were considered. In the future, soil freeze-thaw cycles can be further analyzed by combining changes in surface runoff and plant growth.
5 Conclusion
Through the analysis of the hydrothermal dynamics of Xainza Station from 2019 to 2021, the following conclusions are drawn.
(1) In general, the temperature of each soil depth showed a trend of synchronous change, and the temperature fluctuation presented a periodic change similar to a sine (cosine) curve. The temperature change of deep soil was not as drastic as that of shallow soil. The soil moisture content curve was U-shaped. When the soil depth was below 50 cm, the soil moisture content increased with an increase in soil depth, and a water accumulation area was formed at 80 cm.
(2) The process of soil freeze-thawing can be roughly divided into an initial freezing period, stable freezing period (unstable freeze-thaw period), and ablation period. The freeze-thaw period from 2019 to 2021 lasted 179 and 198 days, respectively. Daily freeze-thaw cycles occurred in the surface soil during the initial freezing period and the ablation period. The soil temperature increased with increasing depth in the early freezing period, stable freezing period (unstable freeze-thaw period), and early ablation period. The variation trend of water content in each stage of freeze-thawing with depth was consistent, increasing first, then decreasing and increasing with depth.
(3) Soil negative temperature was one of the key factors affecting the content of unfrozen water, and a power function correlation existed between soil water and heat. The correlation between 50 and 80 cm depth was poor in the freeze-thaw process, which may be related to the accumulation of water at 50 and 80 cm.
Data availability statement
The original contributions presented in the study are included in the article/Supplementary Material, further inquiries can be directed to the corresponding author.
Author contributions
JC: Writing–original draft, Writing–review and editing. YY: Writing–review and editing. YL: Writing–review and editing.
Funding
The author(s) declare that financial support was received for the research, authorship, and/or publication of this article. This research was supported by Sichuan Science and Technology Program (2023NSFSC0195).
Conflict of interest
The authors declare that the research was conducted in the absence of any commercial or financial relationships that could be construed as a potential conflict of interest.
Publisher’s note
All claims expressed in this article are solely those of the authors and do not necessarily represent those of their affiliated organizations, or those of the publisher, the editors and the reviewers. Any product that may be evaluated in this article, or claim that may be made by its manufacturer, is not guaranteed or endorsed by the publisher.
Supplementary material
The Supplementary Material for this article can be found online at: https://www.frontiersin.org/articles/10.3389/fenvs.2024.1411704/full#supplementary-material
References
Bai, Y. (2020). Study on variations of the hydro-thermal characteristics of frozen under different vegetation types in Qilian Mountain. Lanzhou, China: Gansu Agricultural University. doi:10.27025/d.cnki.ggsnu.2020.000086
Blume-Werry, G., Milbau, A., Teuber, L. M., Johansson, M., and Dorrepaal, E. (2019). Dwelling in the deep-strongly increased root growth and rooting depth enhance plant interactions with thawing permafrost soil. New Phytol. 223, 1328–1339. doi:10.1111/nph.15903
Cao, W., Sheng, Y., Wu, J. C., Chou, Y. L., Peng, E. X., and Leonid, G. (2021). Soil hydrological process and migration mode influenced by the freeze-thaw process in the activity layer of permafrost regions in Qinghai-Tibet Plateau. Cold Reg. Sci. Technol. 184, 103236. doi:10.1016/j.coldregions.2021.103236
Chen, J. J., Yi, S. H., Qin, Y., and Wang, X. Y. (2014). Responses of alpine grassland landscape in the source region of Shule River Basin to topgraphical factors and frozen ground types. Chin. J. Appl. Ecol. 25, 1599–1606. doi:10.13287/j.1001-9332.20140415.011
Chen, R., Yang, M. X., Wan, G. N., and Jia, W. X. (2020). Soil freezing-thawing processes on the Tibetan Plateau: a review based on hydrothermal dynamics. Prog. Geogr. 39, 1944–1958. doi:10.18306/dlkxjz.2020.11.014
Chen, X. M., Wen, F., Gu, Z. B., Zi, Y., and Liao, N. (2019). Study on the hydrothermal mechanism of seasonal frozen soil: a case study about the southern margin of the Gurbantunggut Desert. J. Irrig. Drain. 38, 51–56. doi:10.13522/j.cnki.ggps.20190279
Dai, L. C., Ke, X., Zhang, F. W., Du, Y. G., Li, Y. K., Guo, X. W., et al. (2020). Characteristics of hydro-thermal coupling during soil freezing-thawing process in seasonally frozen soil regions on the Tibetan Plateau. J. Glaciol. Geocryol. 42, 390–398.
Dong, X., Liu, C., Li, M., Ma, D., Chen, Q., and Zang, S. (2021). Variations in active layer soil hydrothermal dynamics of typical wetlands in permafrost region in the Great Hing’an Mountains, northeast China. Ecol. Indic. 129, 107880. doi:10.1016/j.ecolind.2021.107880
Douglas, T. A., Turetsky, M. R., and Koven, C. D. (2020). Increased rainfall stimulates permafrost thaw across a variety of Interior Alaskan boreal ecosystems. npj Clim. Atmos. Sci. 3, 28. doi:10.1038/s41612-020-0130-4
Du, Z. Y. (2020). Effects of freeze-thaw action on soil physicochemical and biological properties in the alpine grasslands. Ecol. Environ. Sci. 29, 1054–1061. doi:10.16258/j.cnki.1674-5906.2020.05.023
Fan, J. H., Lu, X. Y., and Wang, X. D. (2014). The freezing-thawing processes and soil moisture-energy distribution in permafrost active layer, Northern Tibet. J. Mt. Sci. 32, 385–392. doi:10.16089/j.cnki.1008-2786.2014.04.012
Fu, C. W., Hu, Z. Y., Yang, Y. X., Deng, M. S., Yu, H. P., Lu, S., et al. (2022). Responses of soil freeze-thaw processes to climate on the Tibetan Plateau from 1980 to 2016. Remote Sens. 14, 5907. doi:10.3390/rs14235907
Han, B. H., Zhou, B. R., Wu, R., Yan, Y. Q., Niu, D. C., and Fu, H. (2018). Characteristics of hydrothermal factors in soil freezing and thawing alternation over southern alpine grasslands in Qinghai Province. Meteor. Sci. Technol. 46, 361–368. doi:10.19517/j.1671-6345.20170231
He, R. X., Jin, H. J., Chang, X. L., Wang, Y. P., and Wang, L. Z. (2018). Freeze-thaw processes of active-layer soils in the nanweng’he river national natural reserve in the Da Xing’anling Mountains, northern northeast China. Sci. Cold Arid Reg. 10, 104–113.
He, S., Zhang, C. F., Meng, F. R., Bourque, C. P. A., Huang, Z. Y., Li, X., et al. (2024). Scrub-vegetation cover impact on soil water migration and temperature change during freeze-thaw events in sandy desert soils of northwest China. Catena 237, 107828. doi:10.1016/j.catena.2024.107828
Hu, G. J., Zhao, L., Li, R., Wu, T. H., Pang, Q. Q., Wu, X. D., et al. (2014). Analysis on the characteristics of water and heat transport during soil freezing and thawing in permafrost regions of the Qinghai Tibet Plateau. Soil 46, 355–360. doi:10.13758/j.cnki.tr.2014.02.026
Huang, J. Y., Zhao, C. Y., Wei, Y., Zang, F., Chang, Y. P., Hao, H., et al. (2023). Characteristics of soil water-heat at a subalpine meadow during the freezing and thawing periods in Tianlaochi catchment of the Qilian Mountain. J. Glaciol. Geocryol. 45, 1591–1601.
Jiang, H. R., Zhang, W. J., Yi, Y. H., Yan, K., Li, G. C., and Wang, G. X. (2018). The impacts of soil freeze/thaw dynamics on soil water transfer and spring phenology in the Tibetan Plateau. Arct. Antarct. Alp. Res. 50. doi:10.1080/15230430.2018.1439155
Jiang, Y. Y., Zhuang, Q. L., and O’donnell, J. A. (2012). Modeling thermal dynamics of active layer soils and near-surface permafrost using a fully coupled water and heat transport model. J. Geophys. Res.-Atmos. 117. doi:10.1029/2012JD017512
Jiao, Y. L., Li, R., Zhao, L., Wu, T. H., Xiao, Y., Hu, G. J., et al. (2014). Processes of soil thawing-freezing and features of soil moisture migration in the permafrost active layer. J. Glaciol. Geocryol. 36, 237–247.
Kumar, N., Grogan, P., Chu, H., Christiansen, C. T., and Walker, V. K. (2013). The effect of freeze-thaw conditions on arctic soil bacterial communities. Biology 2, 356–377. doi:10.3390/biology2010356
Larsen, K. S., Jonasson, S., and Michelsen, A. (2002). Repeated freeze-thaw cycles and their effects on biological processes in two arctic ecosystem types. Appl. Soil Ecol. 21, 187–195. doi:10.1016/S0929-1393(02)00093-8
Li, W. P., Fan, J. H., Sha, Y. K., Chen, Y. C., Sun, J., and Cheng, G. W. (2014). Soil temperature variation and thaw-freezing cycle in the alpine cold steppe Northern Tibetan Plateau. J. Mt. Sci. 32, 407–416. doi:10.16089/j.cnki.1008-2786.2014.04.007
Lim, P. P., Pearce, D. A., Convey, P., Lee, L. S., Chan, K. G., and Tan, G. Y. A. (2020). Effects of freeze-thaw cycles on High Arctic soil bacterial communities. Polar Sci 23, 100487. doi:10.1016/j.polar.2019.100487
Ling, F., and Zhang, T. J. (2006). Sensitivity of ground thermal regime and surface energy fluxes to tundra snow density in northern Alaska. Cold Reg. Sci. Technol. 44, 121–130. doi:10.1016/j.coldregions.2005.09.002
Liu, H. X., Fan, H. M., and Xu, X. Q. (2021). Simulation of water vertical migration in black soil during freezing-thawing process. J. Soil Water Conserv. 35, 169–173. doi:10.13870/j.cnki.stbcxb.2021.01.025
Liu, S., Yu, G. R., Jun, G., Michaki, S., Zhang, L. M., Zhao, F. H., et al. (2009). The thawing-freezing processes and soil moisture distribution of the steppe in Central Mongolian Plateau. Acta Pedol. Sin. 46, 46–51.
Luo, D. L., Jin, H. J., Lü, L. Z., and Wu, Q. B. (2014). Spatiotemporal characteristics of freezing and thawing of the active layer in the source areas of the Yellow River(SAYR). Chin. Sci. Bull. 59, 3034–3045. doi:10.1007/s11434-014-0189-6
Meteorological Bureau of the Tibet Autonomous Region (2021) Tibet autonomous region climate bulletin. Avaliable at: http://xz.cma.gov.cn/zfxxgk_85277/zwgk/qxbg/202106/t20210611_3406938.html (Accessed on May 24, 2023).
Meteorological Bureau of the Tibet Autonomous Region (2022) Tibet autonomous region climate bulletin. Avaliable at: http://xz.cma.gov.cn/zfxxgk_85277/zwgk/qxbg/202305/t20230501_5479689.html (Accessed on May 24, 2023).
Peng, X. Q., Frauenfeld, O. W., Cao, B., Wang, K., Wang, H. J., Su, H., et al. (2016). Response of changes in seasonal soil freeze/thaw state to climate change from 1950 to 2010 across China. J. Geophys. Res.-Earth Surf. 121, 1984–2000. doi:10.1002/2016JF003876
Qin, Y., Bai, Y., Chen, G., Liang, Y., Li, X., Wen, B., et al. (2021). The effects of soil freeze–thaw processes on water and salt migrations in the western Songnen Plain, China. Sci. Rep. 11, 3888. doi:10.1038/s41598-021-83294-x
Ran, H. W., Fan, J. H., and Huang, J. (2019). Characteristics of water and heat change during the freezing-thawing process of soil profiles in the alpine cold steppe, Northern Tibetan Plateau. Pratac. Sci. 36, 980–990+923.
Sanders-Demott, R., Sorensen, P. O., Reinmann, A. B., and Templer, P. H. (2018). Growing season warming and winter freeze-thaw cycles reduce root nitrogen uptake capacity and increase soil solution nitrogen in a northern forest ecosystem. Biogeochemistry 137, 337–349. doi:10.1007/s10533-018-0422-5
Suzuki, K., Park, H., Makarieva, O., Kanamori, H., Hori, M., Matsuo, K., et al. (2021). Effect of permafrost thawing on discharge of the Kolyma River, northeastern Siberia. Remote Sens 13, 4389. doi:10.3390/rs13214389
Taylor, G. S., and Luthin, J. N. (1978). Model for coupled heat and moisture transfer during soil freezing. Canadian Geotechnical Journal 15, 548–555. doi:10.1139/t78-058
Wan, G. N. (2012) Study on soil freezing-thawing processes and spatio-temporal variations in soil hydrological and thermal regimes on the Central Tibetan Plateau. Beijing, China: University of Chinese Academy of Sciences
Wang, G. X., Liu, L. A., Liu, G. S., Hu, H. C., and Li, T. B. (2010). Impacts of grassland vegetation cover on the active-layer thermal regime, northeast Qinghai-Tibet Plateau, China. Permafr. Periglac. Process. 21, 335–344. doi:10.1002/ppp.699
Wang, X. J., Yang, M. X., and Wan, G. N. (2012). Processes of soil thawing-freezing and features of ground temperature and moisture at D105 on the Northern Tibetan Platrau. J. Glaciol. Geocryol. 34, 56–63.
Wang, X. Q., Chen, R. S., Han, C. T., Yang, Y., Liu, J. F., Liu, Z. W., et al. (2021). Response of shallow soil temperature to climate change on the Qinghai-Tibetan Plateau. Int. J. Climatol. 41, 1–16. doi:10.1002/joc.6605
Wei, W. D., Liu, Y. H., Ma, H., and Li, J. L. (2018). Analysis of freeze-thaw action characteristics in shallow layer soil of degraded alpine steppe. Acta Agric. boreali.-occident. Sin. 27, 1358–1366.
World Meteorological Organization. (2016). El Niño/La Niña Update (February 2016). Avaliable at: https://wmo.int/files/el-ninola-nina-update-february-2016 (Accessed on May 24, 2023).
Wu, X., and Shen, Z. Y. (2010). Effects of freezing-thawing cycle on greenhouse gases production and emission from soil:A review. Chin. J. Ecol. 29, 1432–1439. doi:10.13292/j.1000-4890.2010.0213
Wu, X. L., Zhang, X., Xiang, X. H., Zhang, K., Jin, H. J., Chen, X., et al. (2018). Changing runoff generation in the source area of the Yellow River: mechanisms, seasonal patterns and trends. Cold Reg. Sci. Technol. 155, 58–68. doi:10.1016/j.coldregions.2018.06.014
Yang, J., and Ma, Y. M. (2012). Soil temperature moisture features of typical underlying surface in the Tibetan Plateau. J. Glaciol. Geocryol. 34, 813–820.
Yang, K., and Wang, C. H. (2019). Seasonal persistence of soil moisture anomalies related to freeze-thaw over the Tibetan Plateau and prediction signal of summer precipitation in eastern China. Clim. Dyn. 53, 2411–2424. doi:10.1007/s00382-019-04867-1
Yang, M. X., Yao, T. D., and Gou, X. H. (2000). Freeze-thaw process and water heat distribution characteristics of soil along the Qinghai Tibet Highway. Prog. Nat. Sci., 61–68.
Yang, M. X., Yao, T. D., Hirose, N., and Hideyuki, F. (2006). Daily freeze-thaw cycles of surface soil in the Qinghai Tibet Plateau. Chin. Sci. Bull. 1974-1976.
Yi, Y. H., Kimball, J. S., Chen, R. H., Moghaddam, M., and Miller, C. E. (2019). Sensitivity of active-layer freezing process to snow cover in Arctic Alaska. Cryosphere 13, 197–218. doi:10.5194/tc-13-197-2019
Zhai, P. M., Yu, R., Guo, Y. J., Li, Q. X., Ren, X. J., Wang, Y. Q., et al. (2016). The strong El Nino in 2015/2016 and its dominant impacts on global and China’s climate. Acta Meteor. Sin. 74, 309–321.
Zhang, B. G., Zhang, W., Liu, G. X., Chen, T., Wang, L., Zhang, G. S., et al. (2012). Effect of freeze-thaw cycles on the soil bacterial communities in different ecosystem soils in the Tibetan Plateau. J. Glaciol. Geocryol. 34, 1499–1507.
Zhang, F., Guo, L., Hao, J., and Yang, T. (2019). Analyses on the characteristics of seasonally frozen ground under snow cover and forest/grassland in Kunes Valley, western Tianshan, Xiniang. J. Glaciol. Geocryol 41, 316–323.
Zhang, Y. S., Ma, Y. Z., Zhang, Y. L., Gao, H. F., and Zhai, J. Q. (2015). Hillslope patterns in thaw-freeze cycle and hydrothermal regimes on Tibetan Plateau. Chin. Sci. Bull. 60, 664–673. doi:10.1360/n972014-00313
Zhao, L., Hu, G., Zou, D., Wu, X., Ma, L., Sun, Z., et al. (2019). Permafrost changes and its effects on hydrological processes on Qinghai-Tibet Plateau. Bull. Chin. Acad. Sci. 34, 1233–1246.
Zhao, L., Hu, G. J., Wu, X. D., Wu, T. H., Li, R., Pang, Q. Q., et al. (2021). Dynamics and characteristics of soil temperature and moisture of active layer in the central Tibetan Plateau. Geoderma 400, 115083. doi:10.1016/j.geoderma.2021.115083
Zhao, Q., Wu, C. L., Luo, P. A., Wang, K., Li, H. Z., and Huang, J. S. (2020). Variation and influencing factors of soil temperature and moisture during freezing and thawing period in a seasonal freezing agricultural area in Northeast China. J. Glaciol. Geocryol. 42, 986–995.
Keywords: soil freezing-thawing cycle, soil profile, soil temperature, soil moisture content, hydrothermal coupling
Citation: Chen J, Yan Y and Liu Y (2024) Characteristics of water and heat change during the freezing-thawing process at an alpine steppe in seasonally frozen ground of the Northern Tibetan plateau. Front. Environ. Sci. 12:1411704. doi: 10.3389/fenvs.2024.1411704
Received: 03 April 2024; Accepted: 06 June 2024;
Published: 20 June 2024.
Edited by:
Chamindra L. Vithana, Southern Cross University, AustraliaReviewed by:
Qingfeng Wang, Chinese Academy of Sciences (CAS), ChinaQingbai Wu, Chinese Academy of Sciences (CAS), China
Copyright © 2024 Chen, Yan and Liu. This is an open-access article distributed under the terms of the Creative Commons Attribution License (CC BY). The use, distribution or reproduction in other forums is permitted, provided the original author(s) and the copyright owner(s) are credited and that the original publication in this journal is cited, in accordance with accepted academic practice. No use, distribution or reproduction is permitted which does not comply with these terms.
*Correspondence: Yan Yan, eXlAaW1kZS5hYy5jbg==