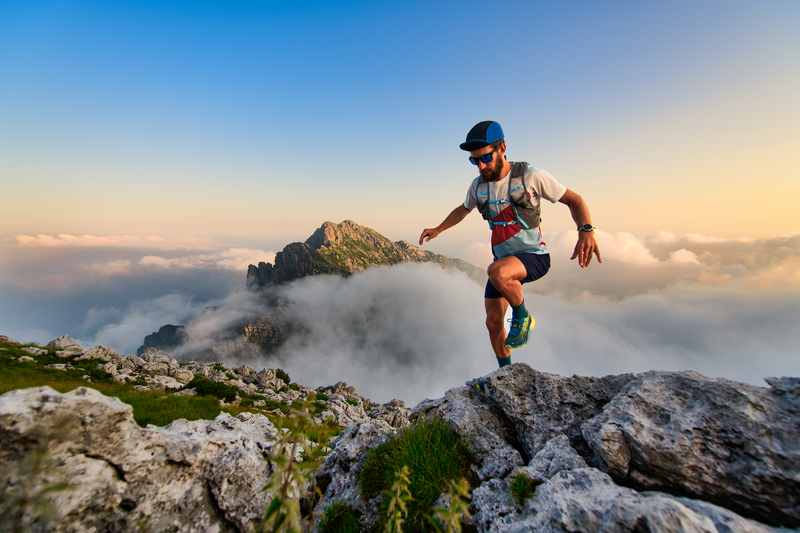
95% of researchers rate our articles as excellent or good
Learn more about the work of our research integrity team to safeguard the quality of each article we publish.
Find out more
ORIGINAL RESEARCH article
Front. Environ. Sci. , 24 June 2024
Sec. Biogeochemical Dynamics
Volume 12 - 2024 | https://doi.org/10.3389/fenvs.2024.1407291
This article is part of the Research Topic Plant Litter Decomposition: Pattern, Process, and Element Cycling View all 5 articles
The differences in mass loss of leaf litter are primarily thought to be driven by microbial activity, especially by fungi. However, the existence of such differences across large spatial scales has not been well explored in field studies and the underlying mechanisms of difference are still unclear, especially for the role of different fungal guilds in driving different mass losses. We conducted a 1-year decomposition study within each of four coastal wetlands in China to test the difference in mass loss across a large spatial scale (ranging from 26° N to 41° N in latitude). In each wetland, six sites including three composed of P. australis and three composed of another dominant plant species typically in coastal ecosystems were selected. We used P. australis leaf litter as the standard decomposition material, placing it into litter bags with mesh sizes 1 mm and 4 mm, respectively. Final litter mass loss was examined approximately after 3, 9 and 12 months. The different mass loss was quantified using additional mass loss at P. australis sites compared to that at another species sites. We found that the mass loss of leaf litter of P. australis showed a clear difference across multiple coastal wetlands only at later stages of decomposition, which was independent of mesofauna (mesh size) contribution to decomposition. Furthermore, the observed difference in mass loss was primarily attributed to the dissimilarities in initial soil fungal community, particularly the symbiotrophic fungi, rather than the soil bacterial community. Our results provide empirical evidence of a large-scale difference in mass loss in litter decomposition and have linked the observed difference to different soil fungal guilds. These results indicate that symbiotrophic fungi might play a direct or indirect role in driving difference in mass loss, which contributes to a better understanding and invites in-depth further investigation on the underlying microbe-driven mechanisms of the difference.
The home-field advantage (HFA) is a well-known phenomenon in decomposition processes, in which plant litter has the tendency to decompose faster in its ‘home’ environment than in its ‘away’ environment even when in a similar abiotic environment (Hunt et al., 1988; Gholz et al., 2000; Ayres et al., 2009; Zheng et al., 2023). The phenomenon shows that the decomposition rate of litter is significantly different under different habitat conditions. Various studies have directly targeted the existence (or absence) of difference in mass loss between different habitat conditions in different types of ecosystems, such as forests, grasslands or wetlands (Gholz et al., 2000; Palozzi and Lindo, 2018; Yeung et al., 2019; Yuan et al., 2019), using either field or greenhouse experiments with different litter types (Purahong et al., 2019; Veen et al., 2019; Wu et al., 2019). However, the difference in decomposition rate is still inconsistently supported by empirical data (Chapman and Koch, 2007; Gießelmann et al., 2011; Freschet et al., 2012; Bachega et al., 2016; Ma et al., 2023), and very few studies have tested difference in mass loss between different habitat conditions across large spatial scales in the field (but see Veen et al., 2019 in a greenhouse experiment). It is still unknown whether difference in mass loss between different habitat conditions in the decomposition process of certain plant species still exists in the field across a large spatial scale.
The existence of difference in mass loss might depend on the dissimilarities in multiple factors between different habitat conditions, such as climatic conditions, aboveground plant species and belowground soil microclimatic conditions or decomposer organisms (Zhu et al., 2024) (Figure 1). One important hypothesis predicts that the difference may result from the local adaptation of the soil organisms to decompose litter from the plant species above them (Bardgett and Walker, 2004; Wardle, 2013). This adaptation might alter physiological activities, shifts in community composition, evolution, or a combination of these processes (Milcu and Manning, 2011). Evidence showed that all size classes of soil fauna might contribute to difference in mass loss (Li X et al., 2021), and large dissimilarities in soil microbial communities between different habitat conditions might result in stronger difference. However, for certain plant species at a large spatial scale, whether different groups of soil fauna or microbes, as well as other environmental factors, might affect the difference in mass loss in decomposition has remained unknown. Especially for soil microbes, it is virtually unknown which groups of soil microbes really matter in driving the difference across large spatial scales.
Figure 1. (A) Study sites of the experiment. Five species are PA: Phragmites australis; SS: Suaeda salsa; IC: Imperata cylindrica; SA: Spartina anglica; CM: Cyperus malaccensis. Solid filled circles and squares were the sites where litter bags were lost completely when harvesting. (B) Conceptual framework of our study, including potential factors driving difference in mass loss, i.e., additional decomposition at PA sites, of P. australis litter decomposition.
Previous studies have shown that difference in mass loss was mostly driven by soil microbial communities, especially by soil fungi (Austin et al., 2014; Lin et al., 2019; Veen et al., 2019; Li et al., 2020; Liu L. et al., 2023). More specifically, fungi might contribute to the difference in mass loss, even though possibly only some specific dominant fungi matter to decomposition rather than the whole fungal community (Lin et al., 2019; Veen et al., 2019; Ma Y et al., 2023). Fungal communities are mainly considered as primary decomposers, because many of them can produce a wide range of extracellular enzymes and degrade recalcitrant organic compounds in plant litter (Boer et al., 2005; van der Wal et al., 2012; Voriskova and Baldrian, 2013; Liu et al., 2022). However, we still lack a deeper understanding about the functional role of fungi in driving the difference in decomposition rate. It remains unknown which functional groups or guilds of soil fungi really matter in driving the difference in mass loss. Two prominent publications could help us to further categorize the soil fungal community into different functional groups or guilds, i.e., Pathotrophs, Saprotrophs, Symbiotrophs or their combinations (Nguyen et al., 2016; Põlme S et al., 2020), and hence help to answer the above question.
In terms of testing the difference in mass loss across large spatial scales, P. australis might be a good candidate species, because P. australis as a cosmopolitan grass is dominant in many wetland ecosystems and widely spread both globally and locally (Brix et al., 2014; Hu et al., 2017; Yin et al., 2022; Liu S. et al., 2023). Moreover, P. australis can expand or invade into new communities, with various consequences to ecosystem functioning (Meyerson et al., 2010; Cui et al., 2019). Such an expansion or invasion of P. australis into other plant communities can lead to negative impacts on the local ecology, most notably through decreased biodiversity (Wails et al., 2021) or it can alter the decomposition of other plant species (Cui et al., 2019), which may indirectly also affect biodiversity. This might have important implications for the testing of difference in mass loss in P. australis litter decomposition across large spatial scale: whether an expansion or invasion by P. australis might have ecological consequences via the difference in mass loss across large spatial scales.
Here we conducted a large-scale decomposition experiment (ranging from 26° N to 41° N in latitude) to examine the leaf litter decomposition rates of P. australis, and tested whether it showed difference in mass loss at sites under P. australis communities, compared to the decomposition rates at sites under another respective dominant plant species across Chinese coastal regions. This experimental design ruled out the litter quality effect on testing the difference (Milcu and Manning, 2011), and simplified our study system to better explore the role of the dissimilarity in belowground biota in driving the difference. Therefore, we hypothesized that (1) the difference in mass loss should mainly depend on the dissimilarities in microbial communities and soil properties between different habitat conditions; (2) other factors such as different soil fauna groups and different stages of decomposition might also matter for the strength of the difference in decomposition across large spatial scales. In addition, we were able to test which taxonomic group(s) or functional guild(s) really matter when testing the difference in a large spatial scale along the Chinese coast. This might improve our understanding about the underlying mechanisms that drive the difference in mass loss and provide important implications for linking soil microbial community to ecosystem functioning.
We selected four national Nature Reserves along Chinese coasts as our study sites (Figure 1): (1) Liao River Estuary National Reserve (40°45′- 41°08′N, 121°28′- 122°00′E) in Panjin (PJ), Liaoning Province; (2) Dafeng Elk National Nature Reserve (32°56′- 33°36′N, 120°42′- 120°51′E) in Yancheng (YC), Jiangsu Province; (3) Hangzhou Bay Wetland Nature Reserve (30°17′- 30°23′N, 121°03′- 121°09′E) in Ningbo (NB), Zhejiang Province; (4) Minjiang River Estuary Wetland National Nature Reserve (26°01′- 26°03′N, 119°36′- 119°41′E) in Fuzhou (FZ), Fujian Province. In each Natural Reserve, we selected six sites with two different types of plant communities as two different habitat conditions in which to conduct our litter decomposition experiment, i.e., three with P. australis (PA) and another three with the respective most dominant plant species whose distribution overlapped to some extent with PA distribution. The other dominant species were Suaeda salsa (SS) in PJ, Imperata cylindrica (IC) in YC, Spartina anglica (SA) in NB and Cyperus malaccensis (CM) in FZ (Figure 1). Note that, for other dominant species sites, we only chose those without PA coexistence to minimize its potential effects.
For our large-scale decomposition experiment, we selected leaf litter of P. australis as the standard decomposition materials. Newly senesced leaf litter of P. australis was sampled from the shoot directly in April 2018 and air-dried at room temperature in the lab for later use. All the litter from different sites was mixed thoroughly to obtain one common litter pool to be used for all litterbags. We used nylon litterbags (15 cm × 20 cm) with two different mesh sizes: 1 mm (small mesh size, S-bag) and 4 mm (large mesh size, L-bag); the latter was assumed to provide access for most soil mesofauna. We weighed around 2.5 g air-dried litter for each litter bag and in total prepared 720 litterbags (4 natural reserves × 2 types of plant community × 3 sites × 2 mesh sizes × 3 harvests × 5 replicates). Every eight litters bags (4 S-bags and 4 L-bags) with a spacing distance of 50 cm were alternately attached to a 5 m nylon rope as a block and vertically inserted into the top layer soil (0–10 cm) with a shovel. The soil was then pushed back into place to close the opening, trying to minimize disturbance. Every five lines (blocks) of litter bags were incubated in parallel in each site, with distances of at least 1 m between blocks. Both ends of each rope were secured with big stones or plastic tubes in order to document the position of each site. Due to the large spatial distance among different sites in different nature reserves, the starting and ending dates for each site were not the same, and there was slightly difference in the duration of decomposition for each site (Supplementary Table S1).
Approximately, we harvested litter bags after 3, 9 and 12 months. For each harvest at each site, we collected one S-bag and one L-bag from each block in order to avoid any systematic error due to litterbag layout and sampling, so we collected 10 litter bags from each site for each harvest. The collection of the bags was completed by following the wire tether to the substrate surface, cutting the wire, and carefully removing the bag from the substrate. Because of high disturbance by human activity, we lost some of our litter bags before harvest, for example, from one site where the transition of land use destroyed our incubation site. We could not find back litter bags in three sites due to the strong hydrological dynamics of intertidal marshes. After each harvest, the litterbags were transported back to the lab, where they were gently washed in distilled water to remove soil and extraneous plant and animal material while minimizing losses of soluble organic compounds. The remaining litter samples were put into envelopes and were oven-dried to constant weight at 80°C. The litter mass loss fraction was calculated as (m0 - mi)/m0, where m0 is the (estimated based on initial water content) oven-dried weight of the initial litter and mi refers to the oven-dried weight of the remaining litter at each harvest i. In order to test difference in mass loss, we calculated the additional decomposition at P. australis sites, as the mass losses in P. australis sites in a given nature reserve minus the mass loss in the site dominated by another dominant plant species in the same nature reserve.
In each site, we randomly collected three top soil samples (0–10 cm depth) at the start of the experiment. Soil samples were brought into the laboratory for the measurement of soil bacterial and fungal communities as well as soil properties. The initial soil properties included total organic carbon (TOC), total nitrogen (TN), soil total phosphorus (TP), and pH (Supplementary Table S1). TOC was assessed using the TOC analyzer (SSM 5000A; Schimadzu, Japan). TN was assessed using the VarioMAX CN element analyzer (Macro Elemental Analyzer System GmbH, Hanau, Germany). TP was analyzed by inductively coupled plasma emission spectroscopy (Perkin Elmer Optima 3000 ICP Spectrometer, Waltham, MA, USA. pH was measured using a Mettler Toledo pH meter (Instrument Teknik, Umeå, Sweden).
The initial soil microbial community, i.e., bacteria and fungi, was measured using the second-generation next throughput sequencing technology (MiSeq high throughput sequencing, 16Sr DNA sequences). Microbial DNA was extracted from soil samples using the soil DNA Kit (Omega Bio-tek, Norcross, GA, U.S.). The bacterial 16S and fungal 18S ribosomal RNA gene were amplified by PCR using primers 515F 5′-GTGCCAGCMGCCGCGG-3′, 907R 5′-CCGTCAATTCMTTTRAGTTT-3′and SSU0817F 5′-TTAGCATGGAATAATRRAATAGGA-3′ and 1196R 5′-TCTGGACCTGGTGAGTTTCC-3′ respectively. PCR reactions were performed in triplicate 20 μL mixture containing 4 μL of 5 × FastPfu Buffer, 2 μL of 2.5 mM dNTPs, 0.8 μL of each primer (5 μM), 0.4 μL of FastPfu Polymerase, and 10 ng of template DNA. Amplicons were extracted from 2% agarose gels and purified using the AxyPrep DNA Gel Extraction Kit (Axygen Biosciences, Union City, CA, U.S.) and quantified using QuantiFluor ™ -ST (Promega, U.S.). The total OTU number, the microbial diversity indices, such as Chao1, Shannon diversity index, Simpson diversity index, were quantified for further analysis. To test the effect of different functional groups of initial soil fungi and link their dissimilarity (home - away) to HFA, we firstly used a recently developed open annotation tool (FunGuild; Nguyen et al., 2016) to automatically assign some OTUs into different fungal guilds via Phython 3.7.3 and then manually assign some other OTUs based on the published database (Chen et al., 2019). In total, we assigned 70 high-abundance OTUs into six fungal guilds: Saprotroph, Symbiotroph, Pathotroph, Saprotroph + Symbiotroph, Pathotroph + Saprotroph, Pathotroph + Saprotroph + Symbiotroph. Then we summed the relative abundances of the OTUs that had been categorized into the same functional guild. The dissimilarity in each fungal guild was calculated as the absolute difference in the ‘summed’ abundances between different habitat conditions. Raw sequencing data are deposited at The Sequence Read Archive under accession number SRP325005 and SRP325012.
All data were checked for the assumptions of homogeneity of variance with a Levene’s test and normality using a Q-Q plot before analysis. We firstly conducted two-way ANOVAs to test the effects of different habitat conditions, mesh sizes and their interaction on litter mass losses of different harvests; in parallel, we conducted similar two-way ANOVAs to test for effects of aboveground dominant plant species, mesh sizes and their interaction on litter mass loss of different harvests. To test the effects of soil microbial (bacterial and fungal) community on the difference in mass losses, we calculated the dissimilarity in soil microbial diversity index (OTUs, Sob, Chao1, Shannon and inversed Simpson index) as the absolute difference between different habitat conditions, i.e., values in P. australis sites–values in other dominant species sites. We also calculated the dissimilarity in soil properties and soil microbial community as the Bray-Curtis distances between soil microbial community between different habitat conditions; for this, we only included the OTUs whose total number was more than 20 across all sites. The dissimilarity of soil properties, fungal and bacterial communities between different habitat conditions were calculated using ‘vegdist’ function in vegan package. Dissimilarity of soil bacterial and fungal communities were displayed using nonmetric multidimensional scaling (NMDS) based on a Bray-Curtis distance matrix (Supplementary Figure S1). Then we conducted general linear models to test the effects of dissimilarity in soil properties, soil bacterial and fungal communities on the different in mass loss of different mesh sizes as well as of different harvests. We also conducted general linear models to test the effect of climate on different in mass loss, using climatic data collected from ‘WorldClim’ (www.worldclim.org). In addition, we tested how dissimilarity in different functional guilds of fungi and in the relative abundance of top five dominant fungal taxa (at class level) between different habitat conditions affected different in mass loss of different harvests using simple regression analysis. All analyses were performed in R version 3.6 (RR Core Team, 2013).
Our 24 sites ranged across 15 degrees of latitude. Soil TOC ranged from 0.081% to 3.144%; soil TN ranged from 0.026% to 0.270%; soil TP ranged from 0.027% to 0.096; soil pH ranged from 6.16 to 8.89 (Supplementary Table S1). Soil fungal diversity ranged from 53 to 374 in terms of Sobs diversity index, 0.230 to 4.109 in terms of Shannon diversity index, 53.1 to 389.0 in terms of Chao diversity index, 1.0 to 24.4 in terms of Inv-simpson diversity index and 27823 to 65572 in terms of number of OTUs; soil bacteria diversity ranged from 1,352 to 3,022 in terms of Sobs diversity index, 3.744 to 7.086 in terms of Shannon diversity index, 1709.6 to 4,178.1 in terms of Chao diversity index, 6.4 to 565.3 in terms of Inv-simpson diversity index and 20199 to 49274 in terms of number of OTUs (Supplementary Table S2).
There were significant differences in mass loss after 9 and 12 months between between different habitat conditions (Figures 2B, C, p = 0.02 & p = 0.03), but only a trend in mass loss difference after 3 months (Figure 2A, p = 0.09). When separating other species sites into different aboveground plant species, there were significant differences in the mass losses after 9 and 12 months between PA and SS, and between PA and CM (Figures 2E, F, p < 0.01), while there was no site effect after 3 months (Figure 2D). Mesh size had significant effects on litter mass loss of all three harvests (Figure 2, p < 0.01), but no significant effects on differences in mass losses between different habitat conditions across all three harvests.
Figure 2. Effects of sites in terms of different habitat conditions (A–C), or in terms of aboveground species (D–F), and mesh size on litter mass loss at different decomposition stages and on additional decomposition at PA sites (E). PA site indicates that litter was decomposed under Phragmites australis (PA) community, while other site indicates the litter was decomposed in a site dominated by other plant species, such as Suaeda salsa (SS), Imperata cylindrica (IC), Spartina anglica (SA), Cyperus malaccensis (CM). PA_SS, PA_IC, PA_SA and PA_CM represent the PA sites in pairs with the corresponding plant species in other site. Values are means ± S.E. * represent the significance (p < 0.05) of the difference between PA and the other dominant plant species.
There was an overall positive correlation between differences in mass loss and the Bray-Curtis distances in initial soil fungal communities between different habitat conditions (Figure 3 left panel, p < 0.01), and this correlation remained for differences in mass loss after 9 months (Figure 3 left panel, p < 0.01) and differences in mass loss after 12 months (Figure 3 left panel, p < 0.01). However, there were no significant correlation between differences in mass loss and the Bray-Curtis distances in bacterial communities between different habitat conditions (Figure 3 right panel, p > 0.05). In addition, there was no significant correlation between differences in mass loss and the dissimilarities in climatic factors or soil properties (Supplementary Table S3).
Figure 3. Relationships between the differences in mass losses and initial (A) soil fungal dissimilarities, and (B) soil bacterial dissimilarities, both in Bray-Curtis distances between different habitat conditions. Mesh size and different stages were indicated by different shapes and colors respectively. Stages 1, 2, 3 correspond to the mass losses after 3, 9 and 12 months. Only significant regressions are shown, and p values and R2 of the regression lines are shown.
As to different functional groups of fungi, there were significant positive correlations between differences in mass loss and the dissimilarities in Symbiotroph composition (Figure 4, R = 0.64, p < 0.01). There were no significant correlations between differences in mass loss and the other two functional guilds, i.e., Pathotrophs and Saprotrophs (Figure 4, p > 0.05). Moreover, there were significant correlations between differences in mass loss and the dissimilarities in the following taxonomic groups: Dothideomycetes (Supplementary Table S4, p < 0.01), Agaricomycetes (Supplementary Table S4, p < 0.01) and Eurotiomycetes (Supplementary Table S4, p = 0.03).
Figure 4. Relationships between the difference in mass loss and the dissimilarities (PA- Other) in composition of different functional groups of soil fungi. Different mesh sizes are shown in different shapes, and different decomposition stages are shown in different colors. Only significant regressions are shown, but p and R values are shown in each subplot. Stages 1, 2, 3 correspond to the mass loss after 3, 9 and 12 months.
Using a litter transplantation experiment, we tested the existence of difference in mass loss of P. australis litter decomposition across a large spatial scale within coastal wetlands. Our results showed that there were clear and consistent differences in mass loss and that this difference mostly depended on the dissimilarities in initial soil fungal communities between different habitat conditions, but not on those in soil bacterial communities. Moreover, we found that difference tended to be more evident at later stages of decomposition, and could be better explained by the dissimilarities in soil symbiotrophic fungi between different habitat conditions, rather than by those in soil pathotrophic or saprotrophic fungi. Below we discuss our findings and highlight the implications of the observed large-scale difference in coastal wetlands as well as the possible underlying microbial-driven mechanisms.
The widespread distribution of P. australis in coastal wetlands has allowed us to test the generality of differences in the mass loss across a spatial scale. Overall, we did find a difference in the decomposition of P. australis across Chinese coastal areas and the magnitude of difference increased with the dissimilarities in initial soil fungal communities between different habitat conditions. This may be due to the fact that there is a home-field advantage (HFA) in the process of litter decomposition. These results were consistent with multiple recent studies, indicating that soil fungi play a vital role in driving the HFA (Miura et al., 2015; Purahong et al., 2019; Ma et al., 2023). Moreover, our results also showed that increasing dissimilarity in the dominant fungal taxa, i.e., Dothideomycetes, Agaricomycetes, and Eurotiomycetes between different habitat conditions soils corresponded with stronger differences in mass loss. Previous studies have also shown that the existence or absence of HFA in decomposition might be related to some specific taxa of fungi but not all fungi (Lin et al., 2019; Veen et al., 2019), or depended on different body sizes of soil microbes (Li et al., 2020). However, no previous studies have tried to link different functional groups of soil fungi, i.e., fungal guilds, to the differences in mass loss. Our results have shown that the dissimilarity in composition within the Symbiotroph group between different habitats was positively correlated with the observed difference in mass loss, while the differences in mass loss was not significantly correlated with compositional dissimilarities within either the Pathotroph or Saprotroph group (Figure 4; Supplementary Figure S2). Symbiotrophic and saprotrophic fungi might affect plant litter decomposition differently, and symbiotrophic fungi might indirectly regulate litter decomposition rates by restraining activities of more efficient litter saprotrophs (Bödeker et al., 2016). Moreover, some of the symbiotrophic fungi associated with plant roots, are capable of oxidizing organic matter (Lindahl and Tunlid, 2015; Nguyen et al., 2016; Choreño-Parra and Treseder, 2024). Therefore, we hypothesize that symbiotrophic fungi associated with the P. australis roots versus those of other dominants might be a predominant driver for the observed difference in mass loss. To take this further, in-depth study should specifically test the extent to which differences is linked to dissimilarities in the extent and genetic/functional composition of symbiotrophic networks in sites dominated by P. australis versus other dominants. Glomeromycota (Glomeromycetes), the taxon representing symbiotrophic fungi, were not among the dominant fungi in our experiment, but our in-depth exploration revealed that they might still have an important role to play in differences in mass loss in our study: the dissimilarities in composition within the Glomeromycetes between different habitat conditions were positively correlated with the observed difference in mass loss (Supplementary Figure S3). This could give symbiotrophic fungi either a direct role in differences in mass loss or an indirect role via influencing the abundance of the saprotrophic taxa that were involved in our study (e.g., Agaricomycetes).
However, our results only partially supported our first hypothesis, because we found that the magnitude of difference did not change with the dissimilarity of soil bacterial communities between different habitat conditions. These results might be due to bacteria having much faster turnover in composition as related to their short lifespans. Thus soil bacteria at t0 may not represent the community composition well through decomposition time, while for fungi t0 sampling may have been more representative of the entire decomposition period. Note that our study only focused on whether the microbial communities in the initial soil, rather than those on the remaining litter, can explain the observed difference. Both sources of microbial community measures are important indicators for understanding the microbe-driven decomposition process: the former focused on examining the role of soil origin in driving HFA (Elias et al., 2020), while the latter focused on quantifying the contribution of microbe colonization on the remaining litter to HFA (Lin et al., 2019) and hence might be more sensitive to litter qualities. Examining the dissimilarity in soil fungal communities might provide more direct evidence to test the soil microbial adaptation hypotheses in HFA studies (Bardgett and Walker, 2004; Ayres et al., 2009; Wardle, 2013). Our experimental design ruled out the effects of litter quality on the existence of difference in mass loss; instead, it zoomed in on the belowground soil biota or incubation environmental drivers on difference.
The existence or absence of HFA in decomposition might depend on different stages of decomposition (Ayres et al., 2009; Güsewell and Gessner, 2009; Purahong et al., 2019) and on the identity of the species growing at other habitat conditions, but not on the dissimilarity of soil properties or different soil fauna groups in coastal habitats. As for the latter, the presence of mesofauna appeared not to interfere with the relationship between HFA and fungal community composition, as evidenced indirectly by a lack of interaction with the mesh size treatment. Our results for decomposition stage showed that there were significantly higher differences in mass loss after 9 and 12 months of decomposition (Figure 1), and the strength (regression coefficient) of the relationship between difference in mass loss between different habitat conditions and dissimilarity of initial soil fungal communities only increased at later stages of decomposition (Figure 3). These results might be explained by most fungi being considered to have low nutritional needs and especially tending to decompose recalcitrant compounds, and therefore might be better adapted to later decomposition stages with poor-quality litter substances. Different decomposing fungi in the soil might have strong inertia and only attack the most recalcitrant compounds such as lignin at later stages of decomposition (Perez et al., 2013). On the other hand, there might be a large amount of variation in HFA among plant species, as was shown previously through reciprocal transplants between different plant species (Ayres et al., 2009). If we investigate different plant species at other dominant species sites in our study, there was only a significant HFA effect when comparing PA sites with SS and CM sites. This might be due to the microbial community between PA and SS or CM being more distinct than those between PA and other dominants (Supplementary Figure S1). More distinct decomposer communities in ‘home’ sites may generate stronger HFA effects than when different habitat conditions soils have a similar composition (Veen et al., 2015a). In addition, our results showed that dissimilarities in soil properties could not explain the difference in mass loss either, and different groups of soil fauna (as deduced from differences in differences in mass loss between mesh sizes) did not affect the relationship between initial soil fungi dissimilarity and the difference in the mass loss (Figure 3). These results re-emphasize that, among soil biota, it is mainly soil fungi that play a predominant role in driving the HFA in decomposition.
Our results have provided empirical evidence on the existence of a large-scale difference in the mass loss of P. australis litter across coastal wetlands, and has linked the difference in the mass loss with different initial soil fungal guilds, notably symbiotrophic fungi and, to a lesser extent, saprotrophic fungi. These results might improve our understanding about nutrient cycling within coastal wetlands, and provide a potential mechanism via different functional groups of soil fungi to explain the inconsistent results about the difference in mass loss across different types of ecosystems. To further reveal the microbe-driven difference in mass loss, we suggest that similar large-scale decomposition should be conducted in other types of ecosystems. Such future studies should further investigate the microbial community in more detail and try to quantify the relative contribution of different soil fungi to difference in mass loss, with particular emphasis on the separate and interactive contributions of symbiotrophic and saprotrophic fungi.
The datasets presented in this study can be found in online repositories. The names of the repository/repositories and accession number(s) can be found in the article/Supplementary Material.
YP: Writing–original draft, Data curation, Investigation. XP: Methodology, Writing–original draft, Investigation. LC: Writing–review and editing, Methodology. WL: Writing–review and editing, Investigation. YH: Writing–review and editing. JC: Writing–review and editing.
The author(s) declare that financial support was received for the research, authorship, and/or publication of this article. This study was supported by Yunnan Science and Technology Talent and Platform Program (202305AM340008) and National Key R&D Program of China (2017YFC0506200).
We thank Zhiguo Dou, Zhiwei Liu and others for helping with the experiment.
The authors declare that the research was conducted in the absence of any commercial or financial relationships that could be construed as a potential conflict of interest.
All claims expressed in this article are solely those of the authors and do not necessarily represent those of their affiliated organizations, or those of the publisher, the editors and the reviewers. Any product that may be evaluated in this article, or claim that may be made by its manufacturer, is not guaranteed or endorsed by the publisher.
The Supplementary Material for this article can be found online at: https://www.frontiersin.org/articles/10.3389/fenvs.2024.1407291/full#supplementary-material
Austin, A. T., Vivanco, L., González-Arzac, A., and Pérez, L. I. (2014). There's no place like home? An exploration of the mechanisms behind plant litter–decomposer affinity in terrestrial ecosystems. New Phytol. 204, 307–314. doi:10.1111/nph.12959
Ayres, E., Steltzer, H., Simmons, B. L., Simpson, R. T., Steinweg, J. M., Wallenstein, M. D., et al. (2009). Home-field advantage accelerates leaf litter decomposition in forests. Soil Biol. Biochem. 41, 606–610. doi:10.1016/j.soilbio.2008.12.022
Bachega, L. R., Bouillet, J., Piccolo, M. D. C., Saint-andré, L., Bouvet, J., Nouvellon, Y., et al. (2016). Decomposition of Eucalyptus grandis and Acacia mangium leaves and fine roots in tropical conditions did not meet the Home Field Advantage hypothesis. For. Ecol. Manag. 359, 33–43. doi:10.1016/j.foreco.2015.09.026
Bardgett, R. D., and Walker, L. R. (2004). Impact of coloniser plant species on the development of decomposer microbial communities following deglaciation. Soil Biol. Biochem. 36, 555–559. doi:10.1016/j.soilbio.2003.11.002
Bödeker, I. T., Lindahl, B. D., Olson, Å., and Clemmensen, K. E. (2016). Mycorrhizal and saprotrophic fungal guilds compete for the same organic substrates but affect decomposition differently. Funct. Ecol. 30, 1967–1978. doi:10.1111/1365-2435.12677
Boer, W. d., Folman, L. B., Summerbell, R. C., and Boddy, L. (2005). Living in a fungal world: impact of fungi on soil bacterial niche development. Fems Microbiol. Rev. 29, 795–811. doi:10.1016/j.femsre.2004.11.005
Brix, H., Ye, S., Laws, E. A., Sun, D., Li, G., Ding, X., et al. (2014). Large-scale management of common reed, Phragmites australis, for paper production: a case study from the Liaohe Delta, China. Ecol. Eng. 73, 760–769. doi:10.1016/j.ecoleng.2014.09.099
Chapman, S. K., and Koch, G. W. (2007). What type of diversity yields synergy during mixed litter decomposition in a natural forest ecosystem? Plant soil 299, 153–162. doi:10.1007/s11104-007-9372-8
Chen, L., Swenson, N. G., Ji, N., Mi, X., Ren, H., Guo, L., et al. (2019). Differential soil fungus accumulation and density dependence of trees in a subtropical forest. Science 366, 124–128. doi:10.1126/science.aau1361
Choreño-Parra, E. M., and Treseder, K. K. (2024). Mycorrhizal fungi modify decomposition: a meta-analysis. New Phytol., 1–12. doi:10.1111/nph.19748
Cui, L., Pan, X., Li, W., Zhang, X., Liu, G., Song, Y. B., et al. (2019). Phragmites australis meets Suaeda salsa on the “red beach”: effects of an ecosystem engineer on salt-marsh litter decomposition. Sci. Total Environ. 693, 133477. doi:10.1016/j.scitotenv.2019.07.283
Elias, B., Makisumi, S., Thiel, U., and Williamson, G. (2020). “The hodge theory of soergel bimodules, introduction to soergel bimodules,” in Introduction to soergel bimodules. Editors B. Elias, S. Makisumi, U. Thiel, and G. Williamson (Springer Nature), 347–367. doi:10.1007/978-3-030-48826-0_18
Freschet, G. T., Aerts, R., and Cornelissen, J. H. C. (2012). Multiple mechanisms for trait effects on litter decomposition: moving beyond home-field advantage with a new hypothesis. J. Ecol. 100, 619–630. doi:10.1111/j.1365-2745.2011.01943.x
Gholz, H. L., Wedin, D. A., Smitherman, S. M., Harmon, M. E., and Parton, W. J. (2000). Long-term dynamics of pine and hardwood litter in contrasting environments: toward a global model of decomposition. Glob. Change Biol. 6, 751–765. doi:10.1046/j.1365-2486.2000.00349.x
Gießelmann, U. C., Martins, K. G., Brändle, M., Schädler, M., Marques, R., and Brandl, R. (2011). Lack of home-field advantage in the decomposition of leaf litter in the Atlantic Rainforest of Brazil. Appl. Soil Ecol. 49, 5–10. doi:10.1016/j.apsoil.2011.07.010
Güsewell, S., and Gessner, M. O. (2009). N: P ratios influence litter decomposition and colonization by fungi and bacteria in microcosms. Funct. Ecol. 23, 211–219. doi:10.1111/j.1365-2435.2008.01478.x
Hu, Y. K., Zhang, Y. L., Liu, G. F., Pan, X., Yang, X., Li, W. B., et al. (2017). Intraspecific N and P stoichiometry of Phragmites australis: geographic patterns and variation among climatic regions. Sci. Rep. 7, 43018–8. doi:10.1038/srep43018
Hunt, H., Ingham, E., Coleman, D., Elliott, E., and Reid, C. (1988). Nitrogen limitation of production and decomposition in prairie, mountain meadow, and pine forest. Ecology 69, 1009–1016. doi:10.2307/1941256
Li, X., Dong, W., Song, Y., Zhan, W. L., and Zheng, Y. S. (2021). Soil mesofauna participating in driving home-field advantage differ between litter mass loss and nutrient release. Appl. Soil Ecol. 163, 103909. doi:10.1016/j.apsoil.2021.103909
Li, Y., Veen, G. F., Hol, W. H. G., Vandenbrande, S., Hannula, S. E., ten Hooven, F. C., et al. (2020). ‘Home’ and ‘away’ litter decomposition depends on the size fractions of the soil biotic community. Soil Biol. Biochem. 144, 107783. doi:10.1016/j.soilbio.2020.107783
Lin, D., Pang, M., Fanin, N., Wang, H., Qian, S., Zhao, L., et al. (2019). Fungi participate in driving home-field advantage of litter decomposition in a subtropical forest. Plant Soil 434, 467–480. doi:10.1007/s11104-018-3865-5
Lindahl, B. D., and Tunlid, A. (2015). Ectomycorrhizal fungi - potential organic matter decomposers, yet not saprotrophs. New Phytol. 205, 1443–1447. doi:10.1111/nph.13201
Liu, L., Guo, Y., Wu, Y., Wu, Y., Yin, M., Guo, X., et al. (2023a). Revealing biogeographic patterns in genetic diversity of native and invasive plants and their association with soil community diversity in the Chinese coast. Oikos 2024, e10116. doi:10.1111/oik.10116
Liu, S., García-Palacios, P., Tedersoo, L., Guirado, E., van der Heijden, M., Wagg, C., et al. (2022). Phylotype diversity within soil fungal functional groups drives ecosystem stability. Nat. Ecol. Evol. 6 (7), 900–909. doi:10.1038/s41559-022-01756-5
Liu, S., Plaza, C., Ochoa-Hueso, R., Trivedi, C., Wang, J., Trivedi, P., et al. (2023b). Litter and soil biodiversity jointly drive ecosystem functions. Glob. Change Biol. 29 (22), 6276–6285. doi:10.1111/gcb.16913
Ma, Y., Cai, R., Zhong, H., Wu, L., and Ge, G. (2023). The home-field advantage of litter decomposition in lake wetlands and the community characteristics of bacterial and eukaryotic decomposers. Plant Soil 483 (1), 109–130. doi:10.1007/s11104-022-05727-4
Meyerson, L. A., Viola, D. V., and Brown, R. N. (2010). Hybridization of invasive Phragmites australis with a native subspecies in North America. Biol. Invasions 12, 103–111. doi:10.1007/s10530-009-9434-3
Milcu, A., and Manning, P. (2011). All size classes of soil fauna and litter quality control the acceleration of litter decay in its home environment. Oikos 120, 1366–1370. doi:10.1111/j.1600-0706.2010.19418.x
Miura, T., Niswati, A., Swibawa, I. G., Haryani, S., Gunito, H., Shimano, S., et al. (2015). Diversity of fungi on decomposing leaf litter in a sugarcane plantation and their response to tillage practice and bagasse mulching: implications for management effects on litter decomposition. Microb. Ecol. 70, 646–658. doi:10.1007/s00248-015-0620-9
Nguyen, N. H., Song, Z., Bates, S. T., Branco, S., Tedersoo, L., Menke, J., et al. (2016). FUNGuild: an open annotation tool for parsing fungal community datasets by ecological guild. Fungal Ecol. 20, 241–248. doi:10.1016/j.funeco.2015.06.006
Palozzi, J. E., and Lindo, Z. (2018). Are leaf litter and microbes team players? Interpreting home-field advantage decomposition dynamics. Soil Biol. Biochem. 124, 189–198. doi:10.1016/j.soilbio.2018.06.018
Perez, G., Aubert, M., Decaëns, T., Trap, J., and Chauvat, M. (2013). Home-Field Advantage: a matter of interaction between litter biochemistry and decomposer biota. Soil Biol. Biochem. 67, 245–254. doi:10.1016/j.soilbio.2013.09.004
Põlme, S., Abarenkov, K., Henrik, N. R., Lindahl, B., Clemmensen, K., Kauserud, H., et al. (2020). FungalTraits: a user-friendly traits database of fungi and fungus-like stramenopiles. Fungal Divers. 105, 1–16. doi:10.1007/s13225-020-00466-2
Purahong, W., Kahl, T., Kruger, D., Buscot, F., and Hoppe, B. (2019). Home-field advantage in wood decomposition is mainly mediated by fungal community shifts at “home” versus “away”. Microb. Ecol. 78, 725–736. doi:10.1007/s00248-019-01334-6
van der Wal, A., Geydan, T. D., Kuyper, T. W., and de Boer, W. (2012). A thready affair: linking fungal diversity and community dynamics to terrestrial decomposition processes. Fems Microbiol. Rev. 37, 477–494. doi:10.1111/1574-6976.12001
Veen, G., Freschet, G. T., Ordonez, A., and Wardle, D. A. (2015a). Litter quality and environmental controls of home-field advantage effects on litter decomposition. Oikos 124, 187–195. doi:10.1111/oik.01374
Veen, G., Snoek, B. L., Bakx-Schotman, T., Wardle, D. A., and van der Putten, W. H. (2019). Relationships between fungal community composition in decomposing leaf litter and home-field advantage effects. Funct. Ecol. 33, 1524–1535. doi:10.1111/1365-2435.13351
Voriskova, J., and Baldrian, P. (2013). Fungal community on decomposing leaf litter undergoes rapid successional changes. ISME J. 7, 477–486. doi:10.1038/ismej.2012.116
Wails, C. N., Baker, K., Blackburn, R., Valle´, A. D., Heise, J., Herakovich, H., et al. (2021). Assessing changes to ecosystem structure and function following invasion by Spartina alterniflora and Phragmites australis: a meta-analysis. Biol. Invasions 23 (9), 2695–2709. doi:10.1007/s10530-021-02540-5
Wardle, D. A. (2013) Communities and ecosystems: linking the aboveground and belowground components (MPB-34). Princeton University Press.
Wu, C., Zhang, Z., Wang, H., Huang, G., Shu, C., Kong, F., et al. (2019). Home-field advantage of CWD decomposition in subtropical forests varied by field sites. For. Ecol. Manag. 444, 127–137. doi:10.1016/j.foreco.2019.04.051
Yeung, A. C., Kreutzweiser, D. P., and Richardson, J. S. (2019). Stronger effects of litter origin on the processing of conifer than broadleaf leaves: a test of home-field advantage of stream litter breakdown. Freshw. Biol. 64, 1755–1768. doi:10.1111/fwb.13367
Yin, M., Liu, L., Wu, Y., Sheng, W., Ma, X., Du, N., et al. (2022). Effects of litter species and genetic diversity on plant litter decomposition in coastal wetland. Ecol. Indic. 144, 109439. doi:10.1016/j.ecolind.2022.109439
Yuan, X., Niu, D., Wang, Y., Boydston, A., Guo, D., Li, X., et al. (2019). Litter decomposition in fenced and grazed grasslands: a test of the home-field advantage hypothesis. Geoderma 354, 113876. doi:10.1016/j.geoderma.2019.07.034
Zheng, J., Li, S., Wang, H., Dai, X., Meng, S., Jiang, L., et al. (2023). Home-field advantage meets priming effect in root decomposition: implications for belowground carbon dynamics. Funct. Ecol. 37 (3), 676–689. doi:10.1111/1365-2435.14251
Keywords: different habitats, large spatial scale, litter decomposition, soil fungi guilds, coastal wetlands
Citation: Ping Y, Pan X, Cui L, Li W, Hu Y and Cornelissen JHC (2024) Functional composition of initial soil fungi explains the difference in mass loss of Phragmites australis litter in different habitat conditions across multiple coastal wetlands. Front. Environ. Sci. 12:1407291. doi: 10.3389/fenvs.2024.1407291
Received: 26 March 2024; Accepted: 16 May 2024;
Published: 24 June 2024.
Edited by:
Mukesh K. Gautam, The City University of New York, United StatesReviewed by:
Shengen Liu, Fujian Agriculture and Forestry University, ChinaCopyright © 2024 Ping, Pan, Cui, Li, Hu and Cornelissen. This is an open-access article distributed under the terms of the Creative Commons Attribution License (CC BY). The use, distribution or reproduction in other forums is permitted, provided the original author(s) and the copyright owner(s) are credited and that the original publication in this journal is cited, in accordance with accepted academic practice. No use, distribution or reproduction is permitted which does not comply with these terms.
*Correspondence: Lijuan Cui, d2V0bGFuZHMxMDhAMTI2LmNvbQ==
†These authors have contributed equally to this work
Disclaimer: All claims expressed in this article are solely those of the authors and do not necessarily represent those of their affiliated organizations, or those of the publisher, the editors and the reviewers. Any product that may be evaluated in this article or claim that may be made by its manufacturer is not guaranteed or endorsed by the publisher.
Research integrity at Frontiers
Learn more about the work of our research integrity team to safeguard the quality of each article we publish.