- 1Institute of Crop Sciences and Plant Breeding, Grass and Forage Sciences/Organic Agriculture, Chris-tian-Albrechts-University Kiel, Kiel, Germany
- 2Grass Based Dairy Systems, Animal Production Systems Group, Wageningen University (WUR), Wageningen, Netherlands
The intensification of dairy and biogas production in Northwestern Europe has led to the conversion of permanent grasslands to arable land, mainly for silage maize production, resulting in significant soil organic carbon (SOC) losses, highlighting the need for implementing alternative management practices during land use change (LUC) for effective climate change mitigation. This study evaluated the impact of incorporating annual grass-clover leys in 3-year arable rotations and slurry application to mitigate SOC losses during LUC. We compared this approach to a continuous silage maize and a permanent grassland on sandy loam soil in Northern Germany. The experiments were simultaneously established at two adjacent 17-year-old sites of permanent grassland and arable cropping, with different levels of initial SOC when the experiment was established. The SOC dynamics in the upper soil layer (0–30 cm) were evaluated by annual 12-year sampling (2011–2022). The cropping systems were unfertilized (N0) or fertilized (N1) using cattle slurry at a rate of 240 kg N ha-1 year-1. The study reveals substantial SOC losses following the conversion of the permanent grassland to grass-clover (ley) based rotation or continuous silage maize, with reductions of 22% and 31%, respectively, compared to baseline levels of the permanent grassland. However, over the 12-year period, the grass-clover ley-based crop rotation demonstrated a 30% reduction in SOC losses compared to continuous silage maize, without compromising dry matter yield. Conversely, the conversion of arable land to grasslands led to SOC increases ranging from 10% to 30%. This recovery was only half the SOC losses observed in the grassland conversion for the same period, indicating a slow-in, fast-out effect during LUC. However, the transition from ley-containing forage rotation to continuous silage maize incurred significant SOC losses of 11%. Overall, these findings underscore the imperative of integrating ley phases to mitigate SOC losses, particularly in high-biomass-yield cropping systems. As a 1-year ley phase was insufficient to sustain carbon sequestration in arable crop rotations, extended ley residence times should be considered.
1 Introduction
Soil organic carbon (SOC) storage is an important global atmospheric sink of carbon dioxide (CO2). It is also essential to maintaining ecosystem balance and productivity, as it improves multiple physicochemical and biological soil properties. In recent years, the rise in atmospheric CO2 concentration has drawn increasing attention towards agricultural-related greenhouse gas (GHG) emissions, especially from land use change (LUC), which is considered the greatest contributor of GHG emissions derived from agriculture, contributing four out of the nine billion Mg CO2 eq produced in 2018 (Smith et al., 2014; FAO, 2021). In Northwest Europe, permanent grassland areas are often tilled for grassland reseeding or replaced by intensive arable systems for dairy and biogas production (Taube et al., 2014). While this happens, mixed farming systems combining both livestock and grain crop production with significant shares of temporary grasslands for grazing are undergoing an accelerated decrease in acreage in the last decades. This has favoured specialized arable systems, dominated by annual grain and whole-plant silage systems, and has excluded livestock and grasslands from arable farming systems (Wilkins, 2008; Ryschawy et al., 2013).
Silage maize production has gained importance to cover the demand for biogas production, intensified by the political efforts to transition to renewable energy production (Levin et al., 2021). As a result, silage maize acreage has increased by 132%, while grassland area shrunk by 28% in about 13 years (1998–2010) (Taube et al., 2014). Silage maize production has increased biomass and biogas yields per production area compared to grassland at the expense of a significant reduction in soil C sequestration (SCS), which is considered an essential component of climate mitigation strategies (Reinsch et al., 2021). Accordingly, significant amounts of CO2 and other more potent GHGs like nitrous oxide (N2O) are released due to increased organic matter mineralization and reduced C inputs after establishing arable systems (Taube et al., 2014; Reinsch et al., 2018b; Loges et al., 2018). Meanwhile, the annual contribution of maize to SOC was reported to be much lower than its proportion of the crop rotation (Poyda et al., 2022).
Meanwhile, the sequestration of additional carbon in soil organic matter (SOM) presents a viable mitigation strategy to address the rising atmospheric CO2 levels, as recognized by the 4‰ Initiative (Soussana et al., 2019). Launched by France during the UNFCCC COP21 in 2015, this initiative aims to capture CO2 from the atmosphere and enhance global SOC stocks by 0.4% annually, with a specific emphasis on agricultural lands. Regulating the negative impact of LUC and land intensification on SOC is essential to mitigating agricultural-related GHG emissions and maintaining the rise in global temperatures under 1.5°C (Smith, 2008; Yang et al., 2018; Rumpel et al., 2020). This can be achieved by changing cropping patterns to include more herbaceous plants on agricultural soils (Paustian et al., 2016). However, amounts of SOC change slowly in most cropping systems in temperate climates, and the amount depends on the initial SOC content, the amount of input and the decomposition rate of added organic matter and soil type (Smith, 2014). A recent study has shown that arable crop fields have a considerable potential to store SOC at a higher storage efficiency and over a longer time (Georgiou et al., 2022). These authors reported that soils furthest from their mineralogical capacity are more effective at accruing carbon, sequestering 3-times higher in soils at one-tenth of their capacity than at one-half.
Previous research has determined that increasing the time of photosynthetic activity or plant cover throughout the year to increase net primary productivity (NPP), leaving crop residues on the field, as well as reducing the use of tillage can all have a positive impact on SOC, either by promoting its accumulation or by reducing its losses (Weil, 2000; De Los Rios et al., 2022a; 2022b). A comprehensive review (King and Blesh, 2018) reported the benefits of using perennial crops and cover crops to increase the SCS of arable lands over cereal and grain-only rotations. Grasslands have a considerable potential to accumulate SOC due to their greater belowground productivity, their positive effects on soil aggregate stability due to the absence of tillage, and the protection provided by plant cover against erosion compared to arable lands (Soussana et al., 2004). The introduction of grass clover leys within a cropping system, dedicated to vegetation for livestock feeding, contributes to various input services like soil conservation and nutrient cycling, as well as output services such as water purification, climate regulation, and habitat provision for biodiversity conservation, in addition to forage production (Martin et al., 2020; Taube et al., 2023). As pointed out by earlier authors (Soussana et al., 2004), ley-arable systems present an intermediate SCS between grasslands and arable cropping systems without leys. However, the efficacy of crop rotations in sequestering SOC hinges significantly on their perenniality, which is deemed more crucial for augmenting SOC stocks and stabilization efficiency than crop diversity, emphasizing the inclusion of ley phases featuring grasses and/or legumes to enhance carbon sequestration (Lemaire et al., 2015; King et al., 2020; Levin et al., 2021). Thus, adopting temporary grasslands or leys into arable systems is expected to be very beneficial in mitigating SOC losses during LUC.
However, a root-shoot relation shift occurs when grasslands are displaced by annual crops like silage maize or cash crops (Poyda et al., 2021). Most of the net primary production of the annual crops is allocated aboveground and removed from the field, leading to a lower substrate for C cycling (Bolinder et al., 2007). A previous study (Struck et al., 2019) showed that the fraction of belowground net primary productivity (fBNPP) for maize is less than 10%, while it is more than 30% for grassland (Chen et al., 2016) under temperate climate conditions. Accordingly, Poyda et al. (2022) concluded that SOC stocks within silage maize cropping systems could be increased or sustained at higher levels solely by incorporating legume-grass leys in the rotations. Indeed, in crop rotations with differing proportions of clover-grass leys in Germany, Levin et al. (2021) observed positive SOC changes in 96% of the plots investigated, independent of the rotation type and the fertilization level. Moreover, tillage use is intensified yearly to establish new crops. Instead, as occurs in mixed crop–livestock systems, adopting grass leys into crop rotations could bring higher belowground residues and reduce tillage, facilitating high retention of the added C, compared to crop rotations containing only annual crops (De Los Rios et al., 2022a). Additionally, the manure produced by livestock can be incorporated back into the system, providing benefits as fertilizers to enhance primary production and as a source of C inputs to raise SOC levels. Both effects could compensate for the losses in C inputs due to high biomass removal and relatively low primary production, as in semi-intensive organic farming systems (Soussana et al., 2004; Levin et al., 2021).
Existing research indicates that converting grassland to arable land can result in a decrease in SOC stocks ranging from 10% to 50% compared to pre-conversion levels over a span of two to 3 decades (Guo and Gifford, 2002; Soussana et al., 2004; Don et al., 2011). Conversely, the reverse conversion process has shown the potential to increase SOC by up to 20% compared to pre-conversion levels over a similar timeframe. Furthermore, studies have demonstrated that the conversion of croplands to grassland leads to enhancements in N content, microbial biomass, and enzyme activities (Li et al., 2024). However, this transition is also associated with reduced soil pH and available phosphorus content, which may have implications for crop productivity, particularly as restored grasslands tend to absorb more phosphorus for growth. While these effects are well known, studies report overall rates for SOC losses occurring after conversion to arable land without distinguishing the losses arising for different types of arable LUC under similar soil and climatic conditions. This is particularly true in adopting leys directly after grassland conversion to arable land or replacing mixed-crop–livestock with continuous silage maize systems, where information is still scarce. An increased interest has recently been shown in using forage and silage from grass-clover swards and maize crops, particularly in low-input systems such as organic farming (Vellinga et al., 2004; Levin et al., 2021). These comparisons could contribute to identifying management approaches that could be implemented to minimize the negative impact of LUC on SOC losses and soil fertility when higher biomass yields are sought for milk production or energy production from renewable resources. Given that loam soils achieved saturation of soil organic carbon (SOC) approximately 10 years following reseeding, as reported by (Elias et al., 2023), the present study aims to evaluate the impacts of different agricultural land use change (LUC) on SOC dynamics 12 years after converting either permanent grassland or arable land in a paired-site investigation. The hypotheses proposed are:
i. Converting permanent grassland to arable cropping systems results in soil organic carbon (SOC) losses, while the reverse conversion leads to SOC accumulation, regardless of post-conversion management strategies.
ii. Arable systems incorporating temporary grasslands or grass clover leys exhibit lower SOC losses compared to those without such components.
2 Materials and methods
2.1 Site description
The study site, located at the experimental farm “Lindhof” (54° 27 55 N; 9° 57 55 E; 15 m a.s.l.), northern Germany, has a typical maritime climate with a mean annual long-term temperature of 9.4 °C and a mean long-term yearly precipitation of 759 mm (1991–2020) distributed evenly throughout the year. The annual rainfall and mean temperatures during the experimental years are shown in Table 1. The soil type is classified as a Eutric Luvisol or Cambisol (Reinsch et al., 2018b; Loges et al., 2018) with a texture is identified as a sandy loam (13% clay, 26% silt, and 61% sand) and a pH of 6.0. Until 1994, the site was managed under intensive conventional arable cropping, following a 5-year crop rotation cycle: silage maize, winter wheat, winter barley, winter oilseed rape, and fallow (see Figure 1). The field received 240 kg N/ha/year, split between mineral N and cattle slurry. From 1994, the site was split into an arable crop rotation and grassland and was managed according to the German Organic Grower’s Association “Bioland” guidelines, prohibiting synthetic fertilizers and pesticides. A 5-year mixed crop rotation with a 1-year undersown grass-clover (winter wheat/undersown grass-clover—grass-clover—oats—potatoes-faba beans) was located on arable land. For the grassland, a grass-clover mixture was undersown into the first organically managed cereal crop (winter wheat), which was later maintained as permanent grassland. The 1994 seed mixture of 30 kg ha−1 of a grass-clover commercial seed mix comprised of perennial ryegrass (Lolium perenne;70%), smooth meadow-fescue (Poa pratensis; (12%), timothy grass (Phleum pratense;12%) and white clover (Trifolium repens;6%). Apart from the leguminous crops, the sites received organic N fertilization of 60 kg N ha-1 year-1 either as cattle slurry to the cereals or as solid manure to the potatoes until 2010, when the current study was initiated. After those years of different management history, the SOC content (0–30 cm soil layer) in the arable land and grassland by 2010 was 1.0% and 1.6%, respectively.
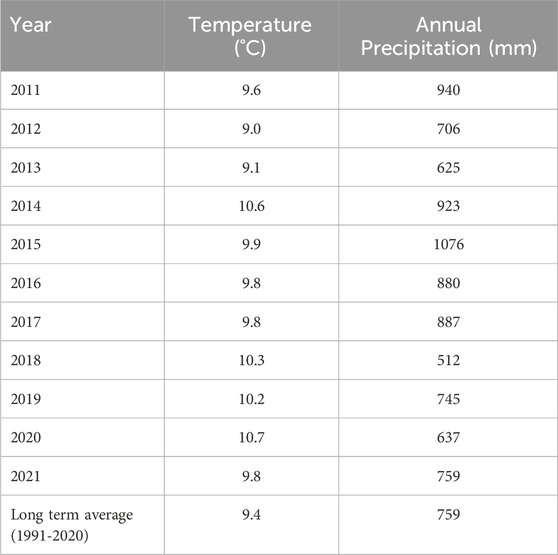
Table 1. Table showing the Average annual temperature and precipitation from year 1980–2021. Long term average of Temperature (1990–2019) is 9.30C while the long-term average of precipitation (1990–2019) is 775.17 mm/m2.
2.2 Experimental setup and management
Land use and management changes occurred in parallel in 2010 at both the arable and grassland sites (Figure 1). In the arable land site, continuous silage maize (CM), a 3-year forage crop rotation (FR) with a 1-year grass clover ley, consisting of perennial ryegrass, red clover (Trifolium pratense) and white clover (silage maize (whole crop silage)-winter wheat/grass-clover–grass-clover), and a new permanent grassland (PGr) —composed of 70% perennial ryegrass, 12% timothy grass, 12% smooth meadow-fescue and 6% white clover—were established (Supplementary Table S1). The initial 5-year mixed crop rotation was reduced to a 3-year mixed rotation (MR) with a 1-year ley (winter wheat/undersown grass-clover—grass-clover—oats) and was used as control (unchanged system). In the grassland site, some parts were converted to CM, some to FR, and some were reseeded (PGr), similar to the arable site, whereas some grassland parts were left undisturbed to serve as control treatment.
For both sites, similar management was followed. The grass-clover was sown in spring under the winter wheat, and it was harvested once in autumn in the year of establishment, followed by four cuts in the main year, and then ploughed in spring. Thus, a slight change in the management of permanent grassland was introduced in 2010, where a mixed system (1–2 silage cuts followed by 3–4 grazing cycles by cattle) was changed to a four-cuts per year system. Similar harvesting frequencies were observed in the PGr, with four cuts per year, whereas in the annual crops, the whole aboveground biomass was removed for silage, grain, and straw for bedding. In all conversions and reseeding events, soil cultivation was performed using conventional moldboard ploughing followed by harrowing using a spring-tine harrow, except for the undersown grass-clover.
In the experimental setup, cropping systems were established as the main plot factor and N rates as the sub-plot factor at both sites. Each main plot measured in minimum 12 m × 6 m. Each cropping system and N rate combination was initialized with three replicates per each crop per N rate. The treatments are presented in Table 2. The cropping systems at both sites were either unfertilized (N0) or fertilized with nitrogen (N1) using cattle slurry (C/N ratio = 10.9), applied with trailing hoses. The N1 treatments received 240 kg ha-1 annually in the grassland control, in PGr and CM systems, whereas the crop rotations FR and the control only received 240 kg ha-1 in two out of 3 years due to no N application in the grass-clover ley, making an annualized N input of 160 kg ha-1 in the crop rotation (Supplementary Table S2). It must be noted that before the start of the experiment, none of the leguminous crops received organic N fertilization (of on average 60 kg N/ha and year either as cattle slurry to the cereals or as solid manure to the potatoes). Accordingly, the absence of these organic fertilizers in the N0 treatment is also a change; this was more than double in the N1 treatment. The N0 and N1 treatments adopted in 2010 suggest a lower and higher N input, respectively, than the previous management system.
2.3 Dry matter yield determination
Using a plot-scale forage harvester, we estimated dry matter yields from permanent grassland, grass-clover, and whole crop silage (Haldrup, Loegstor, Denmark). The fresh matter was harvested over 12 m, with a cutting width of 1.5 m and a cutting height of 5 cm. Harvesting occurred at dough maturity for maize plots, with a whole plant dry matter content of 32% and a cutting height of 25 cm, using a two-row plot-scale forage harvester (Haldrup, Loegstor, Denmark). Dry matter content was determined by oven-drying all biomass samples at 58 °C for 48 h.
2.4 Soil sampling and carbon analysis
Soil sampling was performed at both sites from the autumn of 2010 before ploughing up the grassland until 2022 each year. Each plot sample was collected annually using a soil auger (inner Ø 2 cm) at 0–30 cm soil depth, equivalent to ploughing depth. The samples were oven-dried at 30 °C and sieved to pass a 2 mm mesh size for C analysis. The C content (%) was determined using a CN analyser (Vario Max CN, Elementar) (ISO). The presence/absence of carbonates was tested using a solution of cold, 1-normal hydrochloric acid (1N HCl) by combining one part concentrated HCl (37%) with 11 parts distilled water (Soil Survey Division Staff, 2018). No carbonates were detected within the samples after testing, thus, the estimated total C (%) were assumed to be SOC concentration. The SOC stocks were also calculated according to Eq. 1 using a mean soil bulk density of 1.55 Mg m-3 as differences between the systems did not affect (p > 0.05) soil bulk density (Supplementary Figure S1). Soil bulk density was assessed using the intact core method (Blake and Hartge, 1986), whereby undisturbed soil core samples were obtained from each plot using a stainless steel coring ring (50 mm internal diameter and 50 mm length). Samples were collected from the middle section of the 0–30 cm depth (i.e. 10-20 cm), oven-dried at 105°C for 24 h. Apparent C Recovery (ACR) of added C from slurry, which sought to assess the Carbon-Storage Efficiency (CSE) of the slurry C by the cropping systems, was estimated using Eq. 2.
where OC is the organic carbon concentration (%), LT is the layer thickness (cm), and BD is the topsoil bulk density (g m−3).
where YN1 and YN0 are the fertilized treatment and corresponding non-fertilized control SOC stocks, respectively, and Ci is the slurry C input.
2.5 Data analysis and statistics
The impact of the studied factors (site, cropping system, N rate and time) on SOC and DM variables was assessed using an analysis of covariance within a linear mixed-effects model (Bates and Watts, 1988), employing statistical software R (version 4.3.3; R Core Team, 2024). These statistical techniques were chosen for their suitability in analyzing longitudinal data with multiple factors while providing insights into the interaction effects of different factors. Before analyses, normal distribution and homogeneity of variance assumptions for residuals were assumed after graphical residual analysis. Following significant results, pairwise comparisons were carried out to identify specific differences between factor levels while controlling other factors. Various regression analyses were conducted to predict annual SOC changes due to LUC that allowed assessment of the studied factors’ effect on the rate change in SOC over time. The analyses followed a specific order: linear, exponential and composite models; however, exponential regression outperformed linear models (Supplementary Figures S3A, B). Nonetheless, neither could accurately capture drastic SOC decline observed particularly during first year grassland conversion leading to significant underestimation of SOC losses. Therefore a composite regression approach was adopted denoting amalgamation two distinct regression lines on single plot depicting combined trends or relationship yielding superior fits for data especially converted grassland systems (R2 from 0.02 to 0.92 to 0.59–0.96; see Figure 5; Table 3). This composite approach better captured dynamic changes in SOC following land use alteration offering improved predictive accuracy.
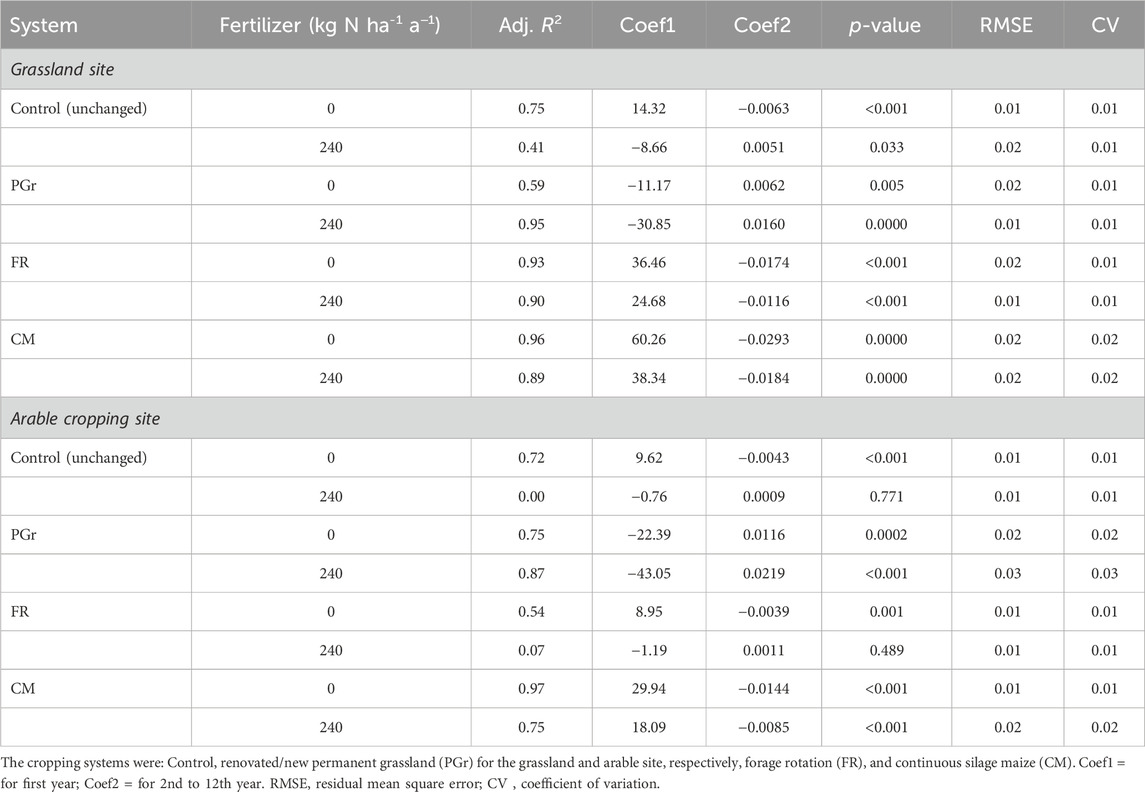
Table 3. Results of composite regression analysis of changes in SOC for the factors cropping system, N rate and site.
3 Results
3.1 Effects of LUC on changes in SOC concentration
The effects of grassland conversion on Soil Organic Carbon (SOC) concentration were influenced by various factors, including cropping system, nitrogen (N) application, and site-year-specific interactions (Supplementary Table S3; Figure 2). Ploughing to establish perennial grassland (PGr) resulted in a significant initial decline in SOC concentration, with a 12% decrease observed in the early years compared to the unconverted permanent grassland control (1.6% ± 0.01% to 1.4% ± 0.04%). Although subsequent years showed gradual increases in SOC content under both N0 and N1 conditions, the initial losses were not fully compensated for under N0 conditions, resulting in a net loss of approximately 3.0 Mg ha-1 of SOC over 12 years following land conversion (Figure 3). In contrast, the N1 treatment exhibited a gain of about 6.5 Mg ha-1, reaching levels similar to the unchanged system (Figure 4). Slurry application mitigated the initial decline in SOC concentration, highlighting its role in SOC management during grassland conversion (Figures 3, 4).
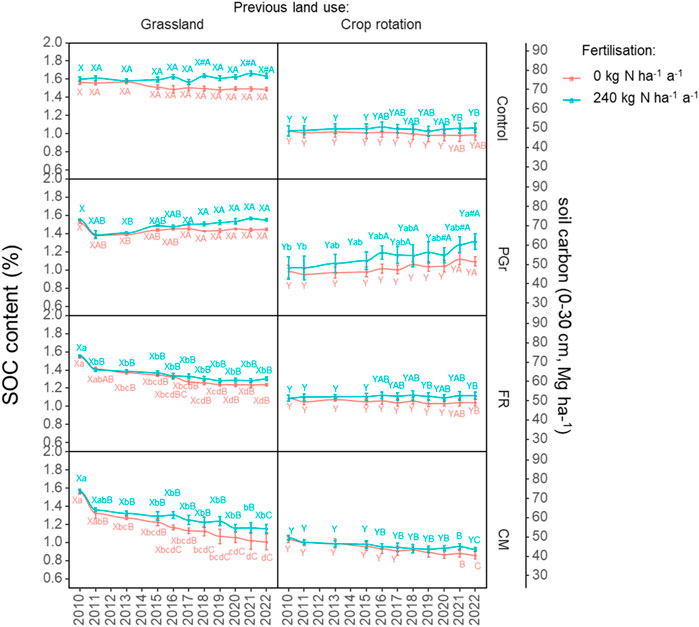
Figure 2. Soil organic carbon (SOC) content (mean ± SE) of the different treatments between 2010 and 2022 The cropping systems were: Control (undisturbed grassland/unchanged system), re-established permanent grassland (PGr), forage rotation (FR), and continuous silage maize (CM); N rates were 0 (N0) and 240 kg N ha-1 (N1). abcDifferent lowercase letters indicate significant differences between the years within a cropping system. ABCDifferent capital letters indicate significant differences between cropping systems, within a site, N rate and year. The absence of letters indicates no statistical differences were detected (p > 0.05).
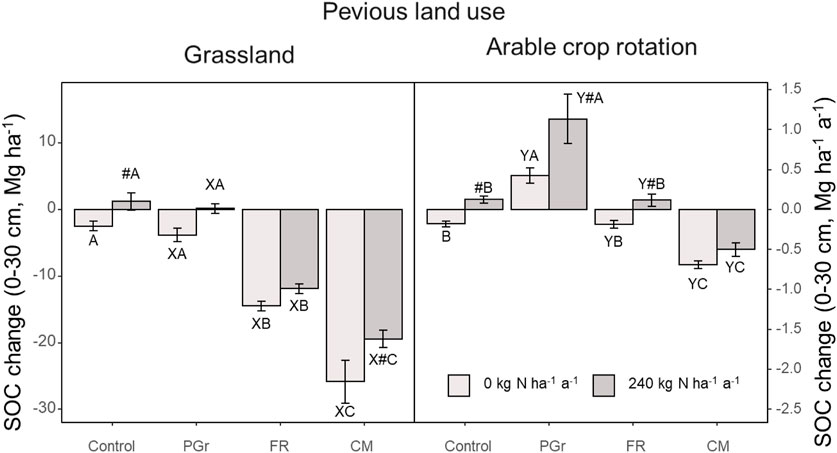
Figure 3. Differences in the amount of soil carbon (Mg ha-1) as difference between the beginning and end of the experimental period “as well as average per year” (Mg ha-1a−1) N rates were 0 (N0) and 240 kg N ha-1 (N1) applied as slurry. ABC indicate system differences within same site; XY is the site differences for same system; # indicate the mean significantly higher for N1.
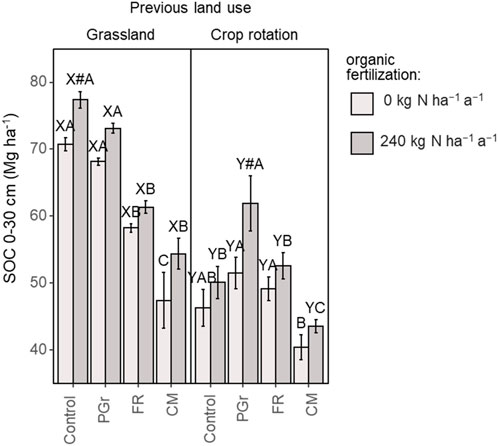
Figure 4. Soil organic carbon (Mg ha-1) at the end of the experimental period in 2022 depending on previous land use and organic fertilization. N rates were 0 (N0 = light grey) and 240 kg N ha-1 (N1 = dark-grey). The cropping systems were: Control (undisturbed grassland/unchanged system), re-established permanent grassland (PGr), forage rotation (FR), and continuous silage maize (CM); N rates were 0 (N0) and 240 kg N ha-1 (N1). #Indicates significant N application effect. XYDifferent letters indicate significant differences between different sites with same cropping system and N rate. ABCDifferent capital letters indicate significant differences between cropping systems within a site with same N rate. The absence of letters indicates no statistical differences were detected (p > 0.05).
Conversely, arable systems (FR and CM) at the grassland site experienced drastic declines in SOC content, with annual losses occurring particularly under the N0 regime from the sixth to the eighth years of the study. Average annual SOC losses for FR and CM systems were 25% and 38%, respectively, in the treatment without slurry (N0) compared to the initial SOC levels (Figure 3). These losses corresponded to decreased SOC stocks of 18 and 27 Mg ha-1, respectively. Lower SOC losses were observed in ley-containing arable rotations compared to continuous maize (CM), with ley-containing systems experiencing reduced SOC losses of 19% and 25% under slurry treatments in FR and CM, respectively (Figure 3). Additionally, adopting ley-containing arable rotations reduced SOC losses by 30% compared to continuous maize (Figure 4), emphasizing the potential of grass clover ley-containing systems in mitigating SOC decline during land use change.
Arable land conversion exhibited distinct effects on Soil Organic Carbon (SOC) dynamics compared to permanent grassland conversion. Unlike grassland conversion, arable land conversion did not immediately lead to drastic reductions in SOC content in established systems. The permanent grassland (PGr) system, when converted from arable land, consistently demonstrated increasing SOC levels, regardless of the nitrogen (N) application level. Under both N0 and N1 conditions, SOC content increased by 10% and 30%, respectively, over the measurement period, surpassing 10 Mg SOC ha-1. At the end of the study, PGr SOC content was higher for both N0 and N1 treatments compared to the unchanged arable crop rotation control, indicating a consistent trend of increasing SOC levels over time, further enhanced by nitrogen application (Figures 3, 4).
In contrast, the effects of arable land conversion on SOC dynamics varied depending on the specific crop rotation system. The FR system displayed slight declining and increasing trends under N0 and N1 conditions, respectively, with insignificant differences in final SOC stocks compared to the unchanged system. Conversely, converting to continuous silage maize (CM) resulted in SOC losses regardless of the nitrogen rate, with higher losses observed under N0 conditions. These losses were approximately three times smaller than those observed during grassland-to-CM conversion. The ley-containing rotation (FR) demonstrated lower losses or gains compared to CM, resulting in higher final SOC stocks at both grassland and arable sites (Figures 3, 4).
The predictability of SOC changes varied across sites, systems, and N application yet remained relatively high (R2 = 0.75–0.97) for the continuous maize system, irrespective of the pre-conversion land use or N status of soils, but relatively poor for the slurry treatments under crop rotation. Moreover, the analyses show that the first-year post-conversion SOC changes were negatively affected by slurry application in all systems. Comparing the change rates of N1 to N0 suggests that the slurry application reduced the sequestration rate or increased the losses in the first year after LUC (Table 3).
3.2 Slurry application effect on SOC changes
Annual changes in Soil Organic Carbon (SOC) were influenced by slurry fertilization across both study sites (Figures 2, 3). Slurry application demonstrated the capacity to sequester SOC or mitigate loss magnitudes. In the control system receiving slurry at the grassland site, a consistent sequestration of SOC was noted at a rate of 0.2 Mg ha-1 a−1 (p < 0.05). Conversely, considerable SOC losses were observed at the N0 rate, quantified at −0.3 Mg ha-1 a−1 (p < 0.001). Similarly, at both sites, N application led to increased SOC content over time, with the final SOC stocks being 8%–9% (3.8–6.6 Mg SOC ha-1) higher for the N1 treatment compared to N0 (Figure 4). Within the permanent grassland system at both sites, both N rates exhibited increases in SOC after initial losses in the first year, with the N1 treatment showing a pronounced increase in SOC at a rate of 0.7 Mg ha-1 a−1 (p < 0.01), contrasting with the 0.3 Mg ha-1 a−1 observed for N0 (p < 0.001). Slurry application effectively reduced SOC loss rates in ley-containing arable rotations by 38% compared to N0 at the grassland site and maintained SOC content in ley-containing arable rotations at the arable site, with significant SOC decreases observed under N0 conditions. Slurry application to continuous silage maize systems effectively reduced SOC loss rates at both sites, with a greater effect observed at the grassland site compared to the arable site (Supplementary Figure S2).
The yearly slurry applications, contributing 21–32 Mg C ha-1 across treatments (calculated on a C/N ratio of 10.99 and N rate of 160–240 kg ha-1), played a pivotal role in curbing C losses and fostering increased C storage, as previously noted. However, this response of SOC storage to slurry application appeared to vary depending on the initial site conditions and cropping system. At the arable site, the highest slurry C recovery (0.867 Mg C ha-1 a−1) was observed in the PGr system, while the lowest (0.270 Mg C ha-1 a−1) was noted in the CM system (Table 4). Furthermore, the impact of slurry on SOC storage in the CM system was approximately twice as high at the grassland site compared with the arable site, although it remained similar for the FR system across both site conditions (Table 4). Overall, positive ACR of slurry C was observed for all systems. Specifically, apparent slurry C recoveries varied from 16% to 22% at the grassland site, with the CM and control systems showing relatively higher recoveries (approximately 21%) than FR or PGr (about 16%). At the arable site, PGr displayed relatively higher slurry recovery (approximately 33%) than FR, CM, or control (less than 20%).
3.3 Landuse change and dry matter productivity
A distinct cyclical yearly pattern emerged in DM yield with fluctuations across forage systems, irrespective of prior land use or soil N status (Figure 5A). Years such as 2015 and 2018 marked the lowest recorded yields (approximately 6.0 Mg ha-1), contrasting with more favorable yields, such as in 2017 (approximately 14 Mg ha-1 in both FR and CM and 10 Mg ha-1 in PGr) across cropping systems. Substantial site differences in DM yield across systems were observed in the initial years, with the initial grassland site maintaining relatively higher yields than the arable site temporally. Although the yield gaps between the two sites narrowed by the second to fourth year of the study, depending on the cropping system, average DM yields of 9.6 ± 2.0 Mg ha-1 observed across systems at the initial grassland site were relatively higher than yields of 8.3 ± 1.6 Mg ha-1 at the initial arable cropping site. DM yields were 0.9–1.2 Mg ha-1 higher at the grassland site than at the arable cropping site, with significant differences (p < 0.05) observed for FR and CM under both N0 and N1 conditions (Figure 5B), translating to 14%–24% higher yields for the grassland site than the arable site.
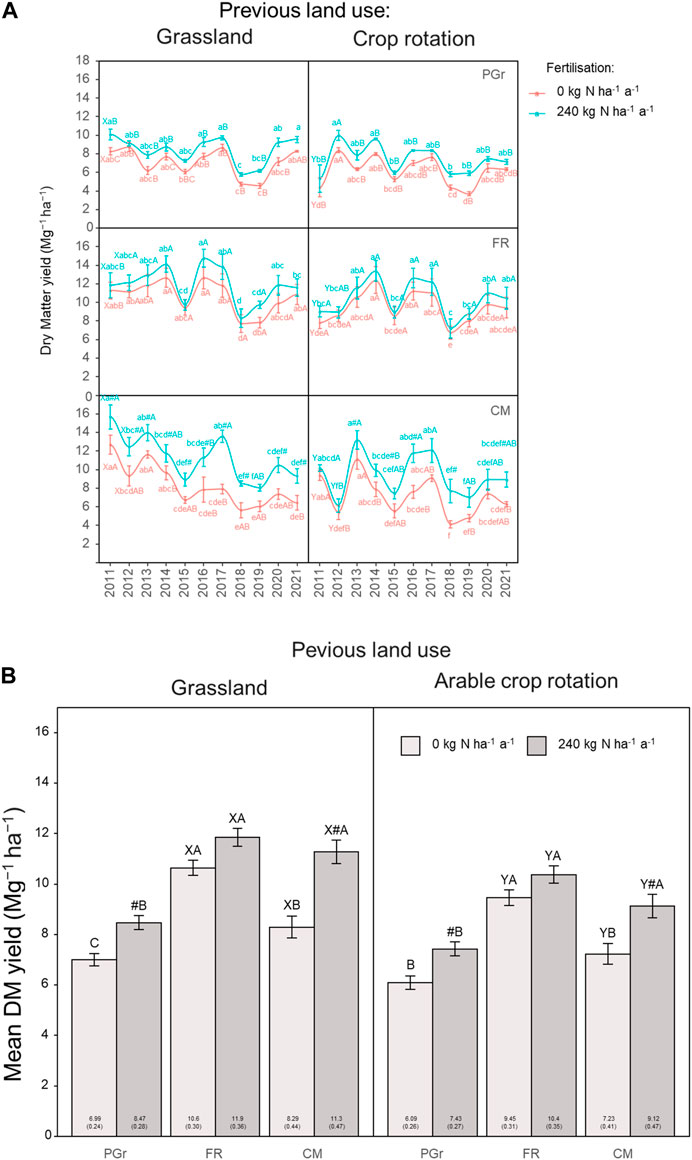
Figure 5. (A) Dry Matter yields presented as temporal variations over the experimental period comparing forage production from the systems, depending on previous land use and organic fertilization application. abcDifferent lowercase letters indicate significant differences between the years within a cropping system. ABCDifferent capital letters indicate significant differences between cropping systems, within a site, N rate and year. XY indicate differences between sites for each system and year. #indicates that the mean for N1 is significantly higher within a system and year. The absence of letters indicates no statistical differences were detected (p > 0.05). (B) Dry Matter yields presented as average of the harvest years 2011–2021, comparing forage production from the systems, depending on previous land use and organic fertilization application. ABCDifferent capital letters indicate significant differences between cropping systems, within a site and N rate. XY indicate differences between sites for each system. #indicates that the mean for N1 is significantly higher within a system and year. The absence of letters indicates no statistical differences were detected (p > 0.05).
Applying nitrogen increased the DM yield of systems by 1.3–3.0 Mg ha-1 (13%–36%) and 1.0–1.9 Mg ha-1 (10%–26%) at the grassland and arable cropping sites, respectively. While the increased DM yield due to N input was significant for PGr and CM at both sites, systematic relative differences in the order FR > CM > PGr were observed. Under the two previous land use and soil N conditions, the FR systems consistently yielded higher DM than the PGr, and FR systems at both sites yielded higher DM than CM under N0 conditions, with yields being similar under N1 conditions (Figure 5B).
4 Discussion
4.1 Effect of long-term grassland and arable land management on SOC stocks
The long-term maintenance of SOC equilibrium in agroecosystems hinges upon the intricate interplay of factors related to C inputs, outputs, and the duration of C residing within the soil matrix. The effectiveness of long-term C storage within ecosystems depends on the equilibrium between retained C within protected or chemically resistant compartments and the extent of C removal via processes like leaching and lateral transfer. Thus, the CSE of soils encompasses both biological and non-biological mechanisms. Due to increased internal C recycling and subsequent losses, C accumulation increasingly relies on protective mechanisms limiting accessibility to decomposers and abiotic removal processes. Factors such as climate, vegetation type, soil type and quality, the composition of soil biological communities, and management practices all influence C sequestration potential (Garnett et al., 2017). Accordingly, under similar climatic conditions, differences in C cycling might be regulated by vegetation type and quality of organic inputs, which might influence biological communities. Invariably, the final SOC stocks observed for the grasslands (51–77 Mg C ha-1) and arable crop fields (40–61 Mg C ha-1) in the current study (Figure 6) were in the range of 40–115 Mg C ha-1 and 29–83 Mg C ha-1, respectively, reported for three United Kingdom grassland chrono-sequence studies, 20 years after reseeding (Elias et al., 2023). Similar to our observation, these authors reported no difference in soil bulk density due to land use.
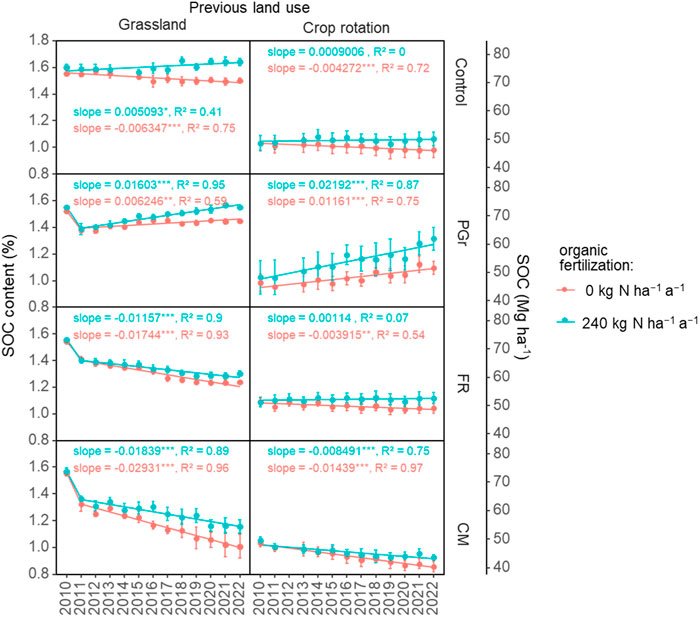
Figure 6. Annual soil organic carbon (SOC) change rates of the different treatments for the period between 2010 and 2022. The cropping systems were: Control, renovated/new permanent grassland (PGr) for the grassland and arable site, respectively, forage rotation (FR), and continuous silage maize (CM); N rates were 0 (N0) and 240 kg N ha-1 (N1) applied as slurry.
Generally, grasses help to stabilize SOC in inter and intra-aggregates due to their high rooting density and release of root exudates with binding properties. On the other hand, rotations, might report a higher SOC mineralization coefficient than grasslands, as rotations have a higher degree of soil disturbance than grasslands. However, the rate of SOC decline was marginally higher for the grassland than the arable site (slope = −0.006 vs. −0.004) in the current study. An incubation study (Ghimire et al., 2019) noted a similar trend, reporting a more pronounced temperature-induced effect on SOC mineralization in grassland sites relative to arable ones. Accordingly, these authors reported a lower SOC mineralization coefficient for arable crop rotations than grasslands. They suggested an enhanced potential for stabilizing labile C reserves in systems integrated with legumes instead of grass-only systems. The capacity of microorganisms to adapt to climate change and variability varies between grasslands and agricultural soils, influencing their substrate utilization and, consequently, SOC dynamics (Hopkins et al., 2014). In light of the arable site’s practice of crop rotation involving the incorporation of grass-clover could increase the influx of high-quality fresh organic matter to influence microbial substrate utilization (Wang et al., 2015), thus leading to positive shifts in SOC storage.
4.2 Effect of LUC on SCS
LUCs between grassland and cropland usually have a consistent direction of SOC change (loss or gain). Our findings reveal a consistent pattern of SOC loss following grassland conversion to arable cropping, with a pronounced decline in the first year of conversion, regardless of cropping system or N application (Figure 3), corroborating previous studies (Solomon et al., 2007; Wang et al., 2009; Holder et al., 2019; Tang et al., 2019). The drastic decline in SOC concentration following grassland conversion can be partly due to enhanced soil aeration from tillage, which promotes mineralisation and SOC loss (Chen et al., 2010; Ding et al., 2013). Grassland conversion significantly alters soil physical-chemical characteristics, with tillage transforming large soil aggregates into finer ones, increasing vulnerability to erosion and dissolved organic C (DOC) leaching (Six et al., 2000). This, combined with the effects of wind and water erosion due to reduced plant canopy and mulch cover, further exacerbates C loss (Guo and Gifford, 2002; Solomon et al., 2007; Wang et al., 2009; Chen et al., 2010; Nautiyal et al., 2010; Li et al., 2014). Further, the C/N ratio of soil organic matter, which regulates its degree of decomposition and quality (Batjes, 1996), decreases markedly after grassland conversion (Tang et al., 2019). This presents a challenge where N fertilizer application is unable to compensate for SOC losses, particularly as soil N levels tend to remain high after conversion (Wang et al., 2009; Chen et al., 2010; Reinsch et al., 2018b). Also, soil aggregate fragmentation through tillage and changes in C and N may cause a shift in soil microbial communities to favour high mineralization potentially (Chen et al., 2010). However, the precise impact on C losses remains unclear (Tang et al., 2019).
In contrast to the rapid decline observed in SOC content following permanent grassland conversion, the transition from arable land did not yield an immediate substantial reduction in SOC within established systems. It appears the low initial level, due to the long history of tillage which prevented the accumulation of SOC in the arable soils, left little SOC to be lost at that site. Moreover, land-use change from arable crop rotation to grassland led to C sequestration, with even higher sequestration rates when slurry was applied. Previous studies have reported similar trends (Poeplau et al., 2011). As observed in this study, the initial SOC level of a site before conversion has been shown to influence CSE. For instance, a previous study (Doblas-Rodrigo et al., 2022) reported higher SOC sequestration at sites with lower initial SOC content relative to sites with higher values. The lower response of SOC storage at the grassland site relative to the arable crop site can attributed to differences in C/N ratio as it is influential in regulating N cycling in soils. Thus, C storage tends to decrease in soils with a high C/N ratio, such as grasslands and tends to increase in soils of low C/N ratio, such as in croplands, in response to N addition (Lu et al., 2011).
However, overall, final SOC stocks under the reseeded grassland (PGr) were relatively high compared with the arable systems (FR of CM), similar to earlier reports (Guillaume et al., 2022; Elias et al., 2023). The relatively greater plant diversity within the PGr system could partially account for the observed higher SCS. Previous research (Lange et al., 2023) demonstrated that increased plant diversity led to elevated C levels in the topsoil. These increases were linked to fresh inputs from various plants, resulting in soil organic matter that was less processed and decomposed. In addition to the C inputs, the lack of soil disturbance could have favoured lower turnover rates for both the native SOC and the freshly added organic matter, promoting the formation of stable aggregates and prolonging the mean residence time of the different organic matter fractions (Reinsch et al., 2018a; De Los Rios et al., 2022a).
The reduced SOC stocks in arable soils, in contrast to grasslands, may stem from multiple causes: lower biomass C input (Chen et al., 2016; De Los Rios et al., 2022a), increased potential for inorganic and dissolved organic N and DOC leaching from the soil profile, and the repetitive cycle of crop growth and harvesting, leading to soil organic matter depletion (Hussain et al., 2020; Antony et al., 2022). The consistent decline in SOC of the arable cropping systems (FR and CM) after grassland conversion can be attributed to the lower belowground biomass allocation and crop harvest, which leads to reduced litter input and organic matter accumulation (Li et al., 2018; Tang et al., 2019). However, it is essential to acknowledge that the study duration may not have been sufficient for the soils to reach a steady state, as SOC decomposition in grassland-converted-to-cropland can persist over extended periods (Barré et al., 2010; Li et al., 2018), attaining a new equilibrium after 17 to over 120 years, especially when deep SOC are considered (Guo and Gifford, 2002; Poeplau et al., 2011). Although the current study considered only the topsoil, this decline has been shown to extend below the 30 cm depth (Guo and Gifford, 2002; Don et al., 2011; Ding et al., 2013). Deep soil layers beyond the top 30 cm may also play a substantial role in SOC dynamics, as they receive inputs from plant roots, root exudates, and translocation processes, often overlooked in monitoring efforts (Rumpel and Kögel-Knabner, 2011). Thus, a comprehensive assessment of SOC change may necessitate a broader consideration of soil depths and long-term observations.
4.3 The role of slurry application on SOC sequestration after LUC
The current study reveals that N application substantially altered the C storage trajectory of the unchanged permanent grassland and the arable rotation system, steering it from a negative trajectory to a positive one. This also improved the SCS rates of the grasslands (PGr) at both sites compared to the unfertilized treatment. The fertilization benefits observed in our study agree with earlier authors (Conant et al., 2017), who reported 12% higher SCS rates with N applications for grassland ecosystems compared with unfertilized trials across multiple regions. Another study (Hirte et al., 2021) reported 52% higher SCS rates in organic-fertilized trials compared with inorganic-fertilized trials, indicating the additional benefits of adding inputs from manure to cropping systems. An insufficient supply of nutrients can trigger a phenomenon known as nutrient mining, wherein microbial decomposition of soil organic matter leads to a reduction in native SOC reserves (Kirkby et al., 2014). Conversely, judicious nutrient management via fertilization strategies can augment SOC concentrations within grasslands (Conant et al., 2001), with alterations in soil N content exhibiting a robust correlation with changes in SOC (Schipper et al., 2014; Nyameasem et al., 2020). Applying organic manure exerts a direct and indirect influence on C stock, adding carbon compounds and nutrients to the soil. The infusion of substantial nutrients aids in sustaining biomass productivity, thereby bolstering SOC reservoirs through augmented organic matter inputs (Lal, 2004; Richardson et al., 2014; van Groenigen et al., 2017; Crème et al., 2020). Thus, for the grasslands to respond positively to N inputs regarding SOC storage, increased belowground net primary production must result (Loges et al., 2018; De Los Rios et al., 2022a).
Accordingly, N application increases belowground and aboveground C inputs into soils, but the effect on C sequestration in soils has been minimal (Lu et al., 2011) because N application stimulates biomass allocation to the aboveground relative to belowground and also stimulates soil respiratory C loss (Lu et al., 2011). Previous studies suggested that a large part of the effects observed in the belowground production in the old grassland was due to slurry application, but the decomposition rate of carbon input from roots was high within one vegetation period (Chen et al., 2016). Evidence suggests that increased carbon flow into the soil pool due to enhanced crop productivity could trigger feedback mechanisms within microbial communities, thereby accelerating the mineralization of soil organic matter (Sanderman et al., 2017). For the grasslands in this study, one can argue that N application might not be solely responsible for the higher SOC retention by the grassland systems, but the shift towards grass-dominated swards, as the nitrogen cycling in the system, is similar between N0 and N1 (see Chen et al., 2016).
While the N source lowered biological N fixation, reduced the white clover (WC) content, and thus reduced C inputs from WC roots, the slurry N + C accelerates the grass component and thus ensures higher C inputs originating from grassroots (Chen et al., 2016). Moreover, the application of slurry has been shown to increase bacterial and fungal abundances, leading to higher respiration rates, which suggest greater decomposition of organic matter (Pintarič et al., 2022). With its higher moisture content, cattle slurry may demonstrate higher microbial C use efficiency than plant debris-derived biomass (Butcher et al., 2020). Presumably, the increased moisture and N content in cattle slurry enhanced microbial access to nutrients and potentially fostered better growth and C assimilation efficiency.
Manure application played a crucial role in mitigating SOC losses from the arable systems at both sites, with unfertilized treatments showing lower SOC storage rates compared to fertilized treatments, particularly evident in young-seeded grasslands (Loges et al., 2018). The benefits of slurry application were more pronounced in continuous maize systems due to their relatively low carbon inputs compared to ley-containing forage rotations (Culley et al., 1981). However, achieving positive SOC storage rates in continuous silage maize systems would necessitate substantial slurry applications, raising concerns about excessive nitrogen deposition and associated environmental problems (Lu et al., 2011). Slurry applications contributed to maintaining SOC equilibrium in ley-containing rotations at the arable site, despite adverse effects on white clover shares (Loges et al., 2018). While the use of manure to promote SOC storage is debated due to off-site carbon transfer and its no-net SOC effect, its application can either promote SOC or maintain soil fertility levels, depending on the cropping system, particularly benefiting arable systems with ley integration (Paustian et al., 2019; Poeplau, 2021). These findings are pertinent not only for organic farming systems but also for conventional systems, where soil fertility relies heavily on organic matter recycling (Loges et al., 2018). The study revealed that slurry carbon had minimal impact on soil SOC sequestration or loss mitigation, particularly in arable systems, with fertilizer-induced carbon retention ranging from 10% to 22% (Table 4). This finding is consistent with previous research showing low retention of digestate carbon and its negligible influence on isotopic composition in crop rotations (Poyda et al., 2022). Likewise, studies have reported low carbon retention from organic fertilizers like liquid dairy manure in monocropped cereals and silage maize (Maillard et al., 2016; De Los Rios et al., 2022b). Legume-grass mixtures and silage maize showed low sensitivity to fertilization, likely due to high biological nitrogen fixation in unfertilized treatments and limited impact of fertilization on root carbon inputs in crop rotations (Poyda et al., 2022). Additionally, organic fertilizers may promote SOC decomposition through a positive priming effect, offsetting most additional carbon inputs (Sun et al., 2019). The low carbon recovery efficiency observed indicates that slurry application or plant biomass inputs alone cannot ensure carbon sequestration in these cropping systems (Jones et al., 2017). These findings underscore the sensitivity of SOC to environmental and management factors, contributing to declining carbon stocks in global grasslands under climate change (Doblas-Rodrigo et al., 2022; Dondini et al., 2023).
4.4 Dry matter production
A cyclical yearly pattern in DM yield emerged across various forage systems, regardless of prior land use or soil N status. These fluctuations reflect the influence of varying weather conditions on crop growth, with years marked by drought during critical growth phases resulting in lower yields, while years with adequate soil moisture yield more favorably (Koca and Erekul, 2016; Börner, 2021). However, these fluctuations were not mirrored in changes in SOC, possibly because drought conditions could lead to a higher root mass fraction in grasslands, altering belowground biomass allocation (Möhl et al., 2023). Despite narrowing yield gaps over time, the initial grassland site consistently displayed higher average DM yields than the arable cropping site across various cropping systems, indicating the enduring productivity advantage of grassland environments. Grassland conversion led to increased residual plant material in the soil, accelerating the decay of native SOC and potentially enhancing soil physical properties (Reinsch et al., 2018a). The superior biomass yield observed in systems at the converted grassland site, even without additional nitrogen input, may be attributed to rapid mineralization of carbon and nitrogen, enhancing nutrient availability and sustaining production. However, this accelerated mineralization also reduced SOC stocks, as the influx of biomass carbon inputs could not fully compensate for the losses incurred.
Systematic differences in DM yield response to nitrogen application, with certain crops exhibiting higher increases than others, emphasize the importance of considering crop-specific responses when optimizing nitrogen management strategies. The effectiveness of the ley phase on subsequent crop yield depends on the proportion of legumes in the ley phase, with higher fertilizer rates in grassland ley not necessarily enhancing the yield of the follow-on crop (Grange et al., 2022). Consistently higher DM yields of certain systems compared to others highlight their potential for enhancing overall productivity, with differences attributed to factors such as crop types and plant diversity in rotations (see Supplementary Table S4; Loges et al., 2018; Grange et al., 2022).
4.5 Implications of the study
The present study uncovered that the potential of arable systems post grassland conversion falls significantly short of the aspirational goal advocated by the “4 per mille” initiative, a conclusion similarly acknowledged by Guillaume et al. (2022). Thus, slurry and biomass C input alone may not ensure C sequestration at an accelerated rate after land conversion, and it is easier and faster for soils to lose than to gain C (Jones et al., 2017). Accordingly, grasslands can act as C sinks but cannot act as perpetual C sinks (Smith, 2014), calling for a combination of practices to ensure that C added to the soil is effectively stored. These might include adopting no-tillage practices during grassland conversion (Struck et al., 2019; De Los Rios et al., 2022b), including multiple species and tannin-rich swards in grasslands (Nyameasem et al., 2020) or leys for biomass production, and extending the ley phase and inclusion of cover crops to build enough organic matter. For instance, in a 70-year experiment (Johnston et al., 2017), the introduction of 3-year grass or grass + clover leys in a 5-year ley–arable rotation significantly augmented SOC in the topsoil over 30–40 years, reaching a seemingly stable equilibrium of around 1.3% SOC. Extending the ley length to 8 years in a 10-year rotation unveiled that % of the SOC had not attained equilibrium after more than 30 years, emphasizing the dynamic nature of SOC responses. Furthermore, with a substantial positive impact on SOC levels from the periodic application of farmyard manure every fifth year, Lu et al. (2011) concluded that N surplus is necessary for enhancing SOC stocks in soils but not for maintaining these stocks and thus, the main driver is not N but C input.
4.6 Limitations of the study and potential directions for future research
Optimizing C storage via the above practices will require a comprehensive understanding of regulating factors. For instance, fertilization can increase plant biomass and further promote soil C sequestration in non-tilled, permanent grasslands (Cenini et al., 2015). However, N and phosphorous inputs to grasslands may change soil microbial communities’ taxonomic and functional composition (Leff et al., 2015) to influence the entire belowground ecosystem. The current study considered only the topsoil, but earlier studies (Guo and Gifford, 2002; Don et al., 2011; Ding et al., 2013) have shown that the effect of LUC may extend below the 30 cm depth. Deep soil layers beyond the top 30 cm may also play a substantial role in SOC dynamics, as they receive inputs from plant roots, root exudates, and translocation processes, often overlooked in monitoring efforts, especially as the carbon turnover in the layer beneath the plough layer can constitute 40%–50% of that in the topsoil (Rumpel and Kögel-Knabner, 2011; Poyda et al., 2022). Moreover, the mineral-associated organic C fraction might be higher in the subsoil than the topsoil regardless of land use and greater in arable soils than in grasslands (Antony et al., 2022), either transported from the topsoil as dissolved organic matter via percolating water or released into deeper layers by extensive plant roots (Leinemann et al., 2018). Thus, a comprehensive assessment of SOC change may necessitate a broader consideration of soil depths and long-term observations.
5 Conclusion
The conversion of permanent grassland to arable land is restricted in the EU, but the projected growth in the human population is expected to drive LUC in the coming decades. This poses risks of significant SOC losses and elevated GHG emissions. To mitigate these effects, alternative agricultural management practices during LUC are crucial. One strategy involves incorporating grass leys into arable land, although our study shows that this only partially prevents SOC losses. However, it does mitigate negative impacts on SOC levels, especially compared to arable systems reliant solely on annual crops, even under nitrogen-deficient conditions. Additionally, incorporating manure applications into grasslands can elevate soil carbon sequestration rates, while arable rotations including ley crops help maintain soil fertility levels. The importance of management decisions during LUC is paramount, as they significantly influence SOC dynamics within a short timeframe. Therefore, exploring and implementing alternative approaches to conventional grassland-to-arable land conversion is crucial for minimizing adverse environmental impacts.
Data availability statement
The raw data supporting the conclusion of this article will be made available by the authors, without undue reservation.
Author contributions
JN: Formal Analysis, Software, Visualization, Writing–original draft, Writing–review and editing. JD: Writing–original draft, Writing–review and editing. CK: Data curation, Formal Analysis, Software, Validation, Visualization, Writing–review and editing. TR: Conceptualization, Methodology, Supervision, Writing–review and editing. AP: Conceptualization, Methodology, Supervision, Writing–review and editing. FT: Conceptualization, Funding acquisition, Project administration, Resources, Supervision, Writing–review and editing. RL: Conceptualization, Data curation, Funding acquisition, Investigation, Methodology, Writing–review and editing.
Funding
The author(s) declare that financial support was received for the research, authorship, and/or publication of this article. The European Commission, through the seventh Framework Programme (Project ID: 289328, Funded under FP7-KBBE, CANTOGETHER (Crops and Animals TOGETHER) project), provided financial support in the first 3 years of the study.
Acknowledgments
The authors sincerely thank R. Kopp and T. Ehmsen for their invaluable technical assistance during fieldwork. Additionally, the Evangelisches Studienwerk Villigst Foundation is gratefully acknowledged for supporting JDLR through a doctoral scholarship within the research program entitled “Third Ways of Feeding the World.”
Conflict of interest
The authors declare that the research was conducted in the absence of any commercial or financial relationships that could be construed as a potential conflict of interest.
Publisher’s note
All claims expressed in this article are solely those of the authors and do not necessarily represent those of their affiliated organizations, or those of the publisher, the editors and the reviewers. Any product that may be evaluated in this article, or claim that may be made by its manufacturer, is not guaranteed or endorsed by the publisher.
Supplementary material
The Supplementary Material for this article can be found online at: https://www.frontiersin.org/articles/10.3389/fenvs.2024.1399197/full#supplementary-material
References
Antony, D., Collins, C. D., Clark, J. M., and Sizmur, T. (2022). Soil organic matter storage in temperate lowland arable, grassland and woodland topsoil and subsoil. Soil Use Manag. 38, 1532–1546. doi:10.1111/sum.12801
Barré, P., Eglin, T., Christensen, B. T., Ciais, P., Houot, S., Kätterer, T., et al. (2010). Quantifying and isolating stable soil organic carbon using long-term bare fallow experiments. Biogeosciences 7, 3839–3850. doi:10.5194/bg-7-3839-2010
Bates, D. M., and Watts, D. G. (1988) Nonlinear regression analysis and its applications. New York: Wiley.
Batjes, N. h. (1996). Total carbon and nitrogen in the soils of the world. Eur. J. Soil Sci. 47, 151–163. doi:10.1111/j.1365-2389.1996.tb01386.x
Blake, G. R., and Hartge, K. H. (1986). “Bulk density,” in Methods of soil analysis, Part 1—physical and mineralogical methods. Editor A. Klute (Madison, Wisconsin: American Society of Agronomy), 363–382.
Bolinder, M. A., Janzen, H. H., Gregorich, E. G., Angers, D. A., and VandenBygaart, A. J. (2007). An approach for estimating net primary productivity and annual carbon inputs to soil for common agricultural crops in Canada. Agric. Ecosyst. Environ. 118, 29–42. doi:10.1016/j.agee.2006.05.013
Börner, B. (2021). Development of Germany’s climate - 2019 monitoring report. Available at: https://www.umweltbundesamt.de/en/topics/climate-energy/climate-impacts-adaptation/impacts-of-climate-change/monitoring-report-2019/development-of-germanys-climate-2019-monitoring (Accessed December 12, 2023).
Buckeridge, K. M., Mason, K. E., McNamara, N. P., Ostle, N., Puissant, J., Goodall, T., et al. (2020). Environmental and microbial controls on microbial necromass recycling, an important precursor for soil carbon stabilization. Commun. Earth Environ. 1, 36–39. doi:10.1038/s43247-020-00031-4
Butcher, K. R., Nasto, M. K., Norton, J. M., and Stark, J. M. (2020). Physical mechanisms for soil moisture effects on microbial carbon-use efficiency in a sandy loam soil in the western United States. Soil Biol. Biochem. 150, 107969. doi:10.1016/j.soilbio.2020.107969
Cenini, V. L., Fornara, D. A., McMullan, G., Ternan, N., Lajtha, K., and Crawley, M. J. (2015). Chronic nitrogen fertilization and carbon sequestration in grassland soils: evidence of a microbial enzyme link. Biogeochemistry 126, 301–313. doi:10.1007/s10533-015-0157-5
Chen, D. D., Zhang, S. H., Dong, S. K., Wang, X. T., and Du, G. Z. (2010). Effect of land-use on soil nutrients and microbial biomass of an alpine region on the northeastern Tibetan plateau, China. Land Degrad. Dev. 21, 446–452. doi:10.1002/ldr.990
Chen, S.-M., Lin, S., Loges, R., Reinsch, T., Hasler, M., and Taube, F. (2016). Independence of seasonal patterns of root functional traits and rooting strategy of a grass-clover sward from sward age and slurry application. Grass Forage Sci. 71, 607–621. doi:10.1111/gfs.12222
Conant, R. T., Cerri, C. E. P., Osborne, B. B., and Paustian, K. (2017). Grassland management impacts on soil carbon stocks: a new synthesis. Ecol. Appl. 27, 662–668. doi:10.1002/eap.1473
Conant, R. T., Paustian, K., and Elliott, E. T. (2001). Grassland management and conversion into grassland: effects on soil carbon. Ecol. Appl. 11, 343–355. doi:10.1890/1051-0761(2001)011[0343:GMACIG]2.0.CO;2
Crème, A., Rumpel, C., Malone, S. L., Saby, N. P. A., Vaudour, E., Decau, M.-L., et al. (2020). Monitoring grassland management effects on soil organic carbon—a matter of scale. Agronomy 10, 2016. doi:10.3390/agronomy10122016
Culley, J. L. B., Phillips, P. A., Hore, F. R., and Patni, N. K. (1981). Soil chemical properties and removal of nutrients by corn resulting from different rates and timing of liquid dairy manure applications. Can. J. Soil. Sci. 61, 39–46. doi:10.4141/cjss81-005
De Los Rios, J., Poyda, A., Reinsch, T., Kluß, C., Taube, F., and Loges, R. (2022a). Integrating crop-livestock system practices in forage and grain-based rotations in northern Germany: potentials for soil carbon sequestration. Agronomy 12, 338. doi:10.3390/agronomy12020338
De Los Rios, J., Poyda, A., Taube, F., Kluß, C., Loges, R., and Reinsch, T. (2022b). No-till mitigates SOC losses after grassland renovation and conversion to silage maize. Agriculture 12, 1204. doi:10.3390/agriculture12081204
Ding, F., Hu, Y., Li, L.-J., Li, A., Lian, P.-Y., Zeng, D.-H., et al. (2013). Changes in soil organic carbon and total nitrogen stocks after conversion of meadow to cropland in Northeast China. Plant Soil 373, 659–672. doi:10.1007/s11104-013-1827-5
Doblas-Rodrigo, Á., Gallejones, P., Artetxe, A., Rosa, E., del Hierro, Ó., and Merino, P. (2022). Grassland contribution to soil organic carbon stock under climate change scenarios in Basque Country (Spain). Reg. Environ. Change 22, 34. doi:10.1007/s10113-022-01877-4
Don, A., Schumacher, J., and Freibauer, A. (2011). Impact of tropical land-use change on soil organic carbon stocks – a meta-analysis. Glob. Change Biol. 17, 1658–1670. doi:10.1111/j.1365-2486.2010.02336.x
Dondini, M., Martin, M., De Camillis, C., Uwizeye, A., Soussana, J.-F., Robinson, T., et al. (2023). Global assessment of soil carbon in grasslands-From current stock estimates to sequestration potential. FAO. doi:10.4060/cc3981en
Elias, D. M. O., Mason, K. E., Howell, K., Mitschunas, N., Hulmes, L., Hulmes, S., et al. (2023). The potential to increase grassland soil C stocks by extending reseeding intervals is dependent on soil texture and depth. J. Environ. Manag. 334, 117465. doi:10.1016/j.jenvman.2023.117465
FAO (2021). Emissions due to agriculture. Global, regional and country trends 2000–2018. Available at: http://www.fao.org/3/cb3808en/cb3808en.pdf.
Garnett, T., Godde, C., Muller, A., Röös, E., Smith, P., and de Boer, I. (2017). Ruminating on cattle, grazing systems, methane, nitrous oxide, the soil carbon sequestration question – and what it all means for greenhouse gas emissions. Available at: https://edepot.wur.nl/427016.
Georgiou, K., Jackson, R. B., Vindušková, O., Abramoff, R. Z., Ahlström, A., Feng, W., et al. (2022). Global stocks and capacity of mineral-associated soil organic carbon. Nat. Commun. 13, 3797. doi:10.1038/s41467-022-31540-9
Ghimire, R., Bista, P., and Machado, S. (2019). Long-term management effects and temperature sensitivity of soil organic carbon in grassland and agricultural soils. Sci. Rep. 9, 12151. doi:10.1038/s41598-019-48237-7
Grange, G., Brophy, C., and Finn, J. A. (2022). Grassland legacy effects on yield of a follow-on crop in rotation strongly influenced by legume proportion and moderately by drought. Eur. J. Agron. 138, 126531. doi:10.1016/j.eja.2022.126531
Guillaume, T., Makowski, D., Libohova, Z., Elfouki, S., Fontana, M., Leifeld, J., et al. (2022). Carbon storage in agricultural topsoils and subsoils is promoted by including temporary grasslands into the crop rotation. Geoderma 422, 115937. doi:10.1016/j.geoderma.2022.115937
Guo, L. B., and Gifford, R. M. (2002). Soil carbon stocks and land use change: a meta analysis. Glob. Change Biol. 8, 345–360. doi:10.1046/j.1354-1013.2002.00486.x
Hirte, J., Walder, F., Hess, J., Büchi, L., Colombi, T., van der Heijden, M. G., et al. (2021). Enhanced root carbon allocation through organic farming is restricted to topsoils. Sci. Total Environ. 755, 143551. doi:10.1016/j.scitotenv.2020.143551
Holder, A. J., Clifton-Brown, J., Rowe, R., Robson, P., Elias, D., Dondini, M., et al. (2019). Measured and modelled effect of land-use change from temperate grassland to Miscanthus on soil carbon stocks after 12 years. Glob. Change Biol. Bioenergy 11, 1173–1186. doi:10.1111/gcbb.12624
Hopkins, F. M., Filley, T. R., Gleixner, G., Lange, M., Top, S. M., and Trumbore, S. E. (2014). Increased belowground carbon inputs and warming promote loss of soil organic carbon through complementary microbial responses. Soil Biol. Biochem. 76, 57–69. doi:10.1016/j.soilbio.2014.04.028
Hussain, M. Z., Robertson, G. P., Basso, B., and Hamilton, S. K. (2020). Leaching losses of dissolved organic carbon and nitrogen from agricultural soils in the upper US Midwest. Sci. Total Environ. 734, 139379. doi:10.1016/j.scitotenv.2020.139379
Johnston, A. E., Poulton, P. R., Coleman, K., Macdonald, A. J., and White, R. P. (2017). Changes in soil organic matter over 70 years in continuous arable and ley–arable rotations on a sandy loam soil in England. Eur. J. Soil Sci. 68, 305–316. doi:10.1111/ejss.12415
Jones, S. K., Helfter, C., Anderson, M., Coyle, M., Campbell, C., Famulari, D., et al. (2017). The nitrogen, carbon and greenhouse gas budget of a grazed, cut and fertilised temperate grassland. Biogeosciences 14, 2069–2088. doi:10.5194/bg-14-2069-2017
King, A. E., and Blesh, J. (2018). Crop rotations for increased soil carbon: perenniality as a guiding principle. Ecol. Appl. 28, 249–261. doi:10.1002/eap.1648
King, A. E., Congreves, K. A., Deen, B., Dunfield, K. E., Simpson, M. J., Voroney, R. P., et al. (2020). Crop rotations differ in soil carbon stabilization efficiency, but the response to quality of structural plant inputs is ambiguous. Plant Soil 457, 207–224. doi:10.1007/s11104-020-04728-5
Kirkby, C. A., Richardson, A. E., Wade, L. J., Passioura, J. B., Batten, G. D., Blanchard, C., et al. (2014). Nutrient availability limits carbon sequestration in arable soils. Soil Biol. Biochem. 68, 402–409. doi:10.1016/j.soilbio.2013.09.032
Koca, Y. O., and Erekul, O. (2016). Changes of dry matter, biomass and relative growth rate with different phenological stages of corn. Agric. Agric. Sci. Procedia 10, 67–75. doi:10.1016/j.aaspro.2016.09.015
Lal, R. (2004). Soil carbon sequestration impacts on global climate change and food security. Science 304, 1623–1627. doi:10.1126/science.1097396
Lange, M., Eisenhauer, N., Chen, H., and Gleixner, G. (2023). Increased soil carbon storage through plant diversity strengthens with time and extends into the subsoil. Glob. Change Biol. 29, 2627–2639. doi:10.1111/gcb.16641
Leff, J. W., Jones, S. E., Prober, S. M., Barberán, A., Borer, E. T., Firn, J. L., et al. (2015). Consistent responses of soil microbial communities to elevated nutrient inputs in grasslands across the globe. Proc. Natl. Acad. Sci. 112, 10967–10972. doi:10.1073/pnas.1508382112
Leinemann, T., Preusser, S., Mikutta, R., Kalbitz, K., Cerli, C., Höschen, C., et al. (2018). Multiple exchange processes on mineral surfaces control the transport of dissolved organic matter through soil profiles. Soil Biol. Biochem. 118, 79–90. doi:10.1016/j.soilbio.2017.12.006
Lemaire, G., Gastal, F., Franzluebbers, A., and Chabbi, A. (2015). Grassland–cropping rotations: an avenue for agricultural diversification to reconcile high production with environmental quality. Environ. Manag. 56, 1065–1077. doi:10.1007/s00267-015-0561-6
Levin, K. S., Auerswald, K., Reents, H. J., and Hülsbergen, K.-J. (2021). Effects of organic energy crop rotations and fertilisation with the liquid digestate phase on organic carbon in the topsoil. Agronomy 11, 1393. doi:10.3390/agronomy11071393
Li, W., Ciais, P., Guenet, B., Peng, S., Chang, J., Chaplot, V., et al. (2018). Temporal response of soil organic carbon after grassland-related land-use change. Glob. Chang. Biol. 24, 4731–4746. doi:10.1111/gcb.14328
Li, Y., Li, Y., Zhang, Q., Xu, G., Liang, G., Kim, D.-G., et al. (2024). Enhancing soil carbon and nitrogen through grassland conversion from degraded croplands in China: assessing magnitudes and identifying key drivers of phosphorus reduction. Soil Tillage Res. 236, 105943. doi:10.1016/j.still.2023.105943
Li, Y., Yu, H., Chappell, A., Zhou, N., and Funk, R. (2014). How much soil organic carbon sequestration is due to conservation agriculture reducing soil erosion? Soil Res. 52, 717–726. doi:10.1071/SR14078
Liu, L., Sayer, E. J., Deng, M., Li, P., Liu, W., Wang, X., et al. (2023). The grassland carbon cycle: mechanisms, responses to global changes, and potential contribution to carbon neutrality. Fundam. Res. 3, 209–218. doi:10.1016/j.fmre.2022.09.028
Loges, R., Bunne, I., Reinsch, T., Malisch, C., Kluß, C., Herrmann, A., et al. (2018). Forage production in rotational systems generates similar yields compared to maize monocultures but improves soil carbon stocks. Eur. J. Agron. 97, 11–19. doi:10.1016/j.eja.2018.04.010
Lu, M., Zhou, X., Luo, Y., Yang, Y., Fang, C., Chen, J., et al. (2011). Minor stimulation of soil carbon storage by nitrogen addition: a meta-analysis. Agric. Ecosyst. Environ. 140, 234–244. doi:10.1016/j.agee.2010.12.010
Maillard, É., Angers, D. A., Chantigny, M., Lafond, J., Pageau, D., Rochette, P., et al. (2016). Greater accumulation of soil organic carbon after liquid dairy manure application under cereal-forage rotation than cereal monoculture. Agric. Ecosyst. Environ. 233, 171–178. doi:10.1016/j.agee.2016.09.011
Martin, G., Durand, J.-L., Duru, M., Gastal, F., Julier, B., Litrico, I., et al. (2020). Role of ley pastures in tomorrow’s cropping systems. A review. A Rev. Agron. sustain. Dev. 40, 17. doi:10.1007/s13593-020-00620-9
McNally, S. R., Laughlin, D. C., Rutledge, S., Dodd, M. B., Six, J., and Schipper, L. A. (2015). Root carbon inputs under moderately diverse sward and conventional ryegrass-clover pasture: implications for soil carbon sequestration. Plant Soil 392, 289–299. doi:10.1007/s11104-015-2463-z
Miltner, A., Bombach, P., Schmidt-Brücken, B., and Kästner, M. (2012). SOM genesis: microbial biomass as a significant source. Biogeochemistry 111, 41–55. doi:10.1007/s10533-011-9658-z
Möhl, P., Vorkauf, M., Kahmen, A., and Hiltbrunner, E. (2023). Recurrent summer drought affects biomass production and community composition independently of snowmelt manipulation in alpine grassland. J. Ecol. 111, 2357–2375. doi:10.1111/1365-2745.14180
Nautiyal, C. S., Chauhan, P. S., and Bhatia, C. R. (2010). Changes in soil physico-chemical properties and microbial functional diversity due to 14 years of conversion of grassland to organic agriculture in semi-arid agroecosystem. Soil Tillage Res. 109, 55–60. doi:10.1016/j.still.2010.04.008
Nyameasem, J. K., Reinsch, T., Taube, F., Domozoro, C. Y. F., Marfo-Ahenkora, E., Emadodin, I., et al. (2020). Nitrogen availability determines the long-term impact of land use change on soil carbon stocks in grasslands of southern Ghana. SOIL 6, 523–539. doi:10.5194/soil-6-523-2020
Paustian, K., Larson, E., Kent, J., Marx, E., and Swan, A. (2019). Soil C sequestration as a biological negative emission strategy. Front. Clim. 1, 8. doi:10.3389/fclim.2019.00008
Paustian, K., Lehmann, J., Ogle, S., Reay, D., Robertson, G. P., and Smith, P. (2016). Climate-smart soils. Nature 532, 49–57. doi:10.1038/nature17174
Pintarič, S., Suhadolc, M., and Eler, K. (2022). Straw management and slurry application affect the soil microbial community composition and its activity. Agronomy 12, 2781. doi:10.3390/agronomy12112781
Poeplau, C. (2021). Grassland soil organic carbon stocks along management intensity and warming gradients. Grass Forage Sci. 76, 186–195. doi:10.1111/gfs.12537
Poeplau, C., Don, A., Vesterdal, L., Leifeld, J., Van Wesemael, B., Schumacher, J., et al. (2011). Temporal dynamics of soil organic carbon after land-use change in the temperate zone – carbon response functions as a model approach. Glob. Change Biol. 17, 2415–2427. doi:10.1111/j.1365-2486.2011.02408.x
Poyda, A., Levin, K. S., Hülsbergen, K.-J., and Auerswald, K. (2022). Perennial crops can compensate for low soil carbon inputs from maize in ley-arable systems. Plants 12, 29. doi:10.3390/plants12010029
Poyda, A., Reinsch, T., Struck, I. J., Skinner, R. H., Kluß, C., and Taube, F. (2021). Low assimilate partitioning to root biomass is associated with carbon losses at an intensively managed temperate grassland. Plant Soil 460, 31–50. doi:10.1007/s11104-020-04771-2
R Core Team (2024). _R: a language and environment for statistical computing_. Available at: https://www.R-project.org/.
Reinsch, T., Loges, R., Kluß, C., and Taube, F. (2018a). Effect of grassland ploughing and reseeding on CO2 emissions and soil carbon stocks. Agric. Ecosyst. Environ. 265, 374–383. doi:10.1016/j.agee.2018.06.020
Reinsch, T., Loges, R., Kluß, C., and Taube, F. (2018b). Renovation and conversion of permanent grass-clover swards to pasture or crops: effects on annual N2O emissions in the year after ploughing. Soil Tillage Res. 175, 119–129. doi:10.1016/j.still.2017.08.009
Reinsch, T., Struck, I. J. A., Loges, R., Kluß, C., and Taube, F. (2021). Soil carbon dynamics of no-till silage maize in ley systems. Soil Tillage Res. 209, 104957. doi:10.1016/j.still.2021.104957
Richardson, A. E., Kirkby, C. A., Banerjee, S., and Kirkegaard, J. A. (2014). The inorganic nutrient cost of building soil carbon. Carbon Manag. 5, 265–268. doi:10.1080/17583004.2014.923226
Rumpel, C., Amiraslani, F., Chenu, C., Garcia Cardenas, M., Kaonga, M., Koutika, L.-S., et al. (2020). The 4p1000 initiative: opportunities, limitations and challenges for implementing soil organic carbon sequestration as a sustainable development strategy. Ambio 49, 350–360. doi:10.1007/s13280-019-01165-2
Rumpel, C., and Kögel-Knabner, I. (2011). Deep soil organic matter-a key but poorly understood component of terrestrial C cycle. Plant Soil 338, 143–158. doi:10.1007/s11104-010-0391-5
Ryschawy, J., Choisis, N., Choisis, J. P., and Gibon, A. (2013). Paths to last in mixed crop–livestock farming: lessons from an assessment of farm trajectories of change. Animal 7, 673–681. doi:10.1017/S1751731112002091
Sanderman, J., Creamer, C., Baisden, W. T., Farrell, M., and Fallon, S. (2017). Greater soil carbon stocks and faster turnover rates with increasing agricultural productivity. SOIL 3, 1–16. doi:10.5194/soil-3-1-2017
Schipper, L. A., Parfitt, R. L., Fraser, S., Littler, R. A., Baisden, W. T., and Ross, C. (2014). Soil order and grazing management effects on changes in soil C and N in New Zealand pastures. Agric. Ecosyst. Environ. 184, 67–75. doi:10.1016/j.agee.2013.11.012
Six, J., Elliott, E. T., and Paustian, K. (2000). Soil macroaggregate turnover and microaggregate formation: a mechanism for C sequestration under no-tillage agriculture. Soil Biol. Biochem. 32, 2099–2103. doi:10.1016/S0038-0717(00)00179-6
Smith, P. (2008). Land use change and soil organic carbon dynamics. Nutr. Cycl. Agroecosyst 81, 169–178. doi:10.1007/s10705-007-9138-y
Smith, P. (2014). Do grasslands act as a perpetual sink for carbon? Glob. Change Biol. 20, 2708–2711. doi:10.1111/gcb.12561
Smith, P., Clark, H., Dong, H., Elsiddig, E. A., Haberl, H., Harper, R., et al. (2014) Chapter 11 - agriculture, forestry and other land use (AFOLU). Cambridge, United Kingdom: Cambridge University Press. Available at: http://www.ipcc.ch/pdf/assessment-report/ar5/wg3/ipcc_wg3_ar5_chapter11.pdf (Accessed December 12, 2023).
Soil Science Division Staff (2018) Soil Survey manual. Agriculture handbook No. 18. SW Washington DC, USA: United States Department of Agriculture.
Solomon, D., Lehmann, J., Kinyangi, J., Amelung, W., Lobe, I., Pell, A., et al. (2007). Long-term impacts of anthropogenic perturbations on dynamics and speciation of organic carbon in tropical forest and subtropical grassland ecosystems. Glob. Change Biol. 13, 511–530. doi:10.1111/j.1365-2486.2006.01304.x
Soussana, J.-F., Loiseau, P., Vuichard, N., Ceschia, E., Balesdent, J., Chevallier, T., et al. (2004). Carbon cycling and sequestration opportunities in temperate grasslands. Soil Use Manag. 20, 219–230. doi:10.1111/j.1475-2743.2004.tb00362.x
Soussana, J.-F., Lutfalla, S., Ehrhardt, F., Rosenstock, T., Lamanna, C., Havlík, P., et al. (2019). Matching policy and science: rationale for the “4 per 1000-soils for food security and climate” initiative. Soil Tillage Res. 188, 3–15. doi:10.1016/j.still.2017.12.002
Soussana, J. F., Tallec, T., and Blanfort, V. (2010). Mitigating the greenhouse gas balance of ruminant production systems through carbon sequestration in grasslands. animal 4, 334–350. doi:10.1017/S1751731109990784
Struck, I. J., Reinsch, T., Herrmann, A., Kluß, C., Loges, R., and Taube, F. (2019). Yield potential and nitrogen dynamics of no-till silage maize (Zea mays L.) under maritime climate conditions. Eur. J. Agron. 107, 30–42. doi:10.1016/j.eja.2019.04.009
Sun, Z., Liu, S., Zhang, T., Zhao, X., Chen, S., and Wang, Q. (2019). Priming of soil organic carbon decomposition induced by exogenous organic carbon input: a meta-analysis. Plant Soil 443, 463–471. doi:10.1007/s11104-019-04240-5
Tang, S., Guo, J., Li, S., Li, J., Xie, S., Zhai, X., et al. (2019). Synthesis of soil carbon losses in response to conversion of grassland to agriculture land. Soil Tillage Res. 185, 29–35. doi:10.1016/j.still.2018.08.011
Taube, F., Gierus, M., Hermann, A., Loges, R., and Schönbach, P. (2014). Grassland and globalization – challenges for north-west European grass and forage research. Grass Forage Sci. 69, 2–16. doi:10.1111/gfs.12043
Taube, F., Nyameasem, J. K., Fenger, F., Alderkamp, L., Kluß, C., and Loges, R. (2023). Eco-efficiency of leys—the trigger for sustainable integrated crop-dairy farming systems. Grass Forage Sci. n/a, 1–12. doi:10.1111/gfs.12639
van Groenigen, J. W., van Kessel, C., Hungate, B. A., Oenema, O., Powlson, D. S., and van Groenigen, K. J. (2017). Sequestering soil organic carbon: a nitrogen dilemma. Environ. Sci. Technol. 51, 4738–4739. doi:10.1021/acs.est.7b01427
Vellinga, Th. V., van den Pol-van Dasselaar, A., and Kuikman, P. J. (2004). The impact of grassland ploughing on CO2 and N2O emissions in The Netherlands. Nutrient Cycl. Agroecosyst. 70, 33–45. doi:10.1023/B:FRES.0000045981.56547.db
Wang, H., Boutton, T. W., Xu, W., Hu, G., Jiang, P., and Bai, E. (2015). Quality of fresh organic matter affects priming of soil organic matter and substrate utilization patterns of microbes. Sci. Rep. 5, 10102. doi:10.1038/srep10102
Wang, Q., Zhang, L., Li, L., Bai, Y., Cao, J., and Han, X. (2009). Changes in carbon and nitrogen of Chernozem soil along a cultivation chronosequence in a semi-arid grassland. Eur. J Soil Sci. 60, 916–923. doi:10.1111/j.1365-2389.2009.01174.x
Weil, R. R. (2000) Soil organic matter in temperate agroecosystems: long-term experiments in north America. Boca Raton, FL: CRC Press. doi:10.1017/S0889189300008456
Wilkins, R. J. (2008). Eco-efficient approaches to land management: a case for increased integration of crop and animal production systems. Philos. Trans. R. Soc. Lond B Biol. Sci. 363, 517–525. doi:10.1098/rstb.2007.2167
Keywords: soil carbon sequestration, climate change mitigation, ley-arable systems, grasslands, cattle slurry
Citation: Nyameasem JK, De Los Rios J, Kluß C, Reinsch T, Poyda A, Taube F and Loges R (2024) Incorporating leys in arable systems as a mitigation strategy to reduce soil organic carbon losses during land-use change. Front. Environ. Sci. 12:1399197. doi: 10.3389/fenvs.2024.1399197
Received: 12 March 2024; Accepted: 24 April 2024;
Published: 14 May 2024.
Edited by:
Ilan Stavi, Dead Sea and Arava Science Center, IsraelReviewed by:
Yuan Li, Lanzhou University, ChinaCurtis Dell, United States Department of Agriculture, United States
Copyright © 2024 Nyameasem, De Los Rios, Kluß, Reinsch, Poyda, Taube and Loges. This is an open-access article distributed under the terms of the Creative Commons Attribution License (CC BY). The use, distribution or reproduction in other forums is permitted, provided the original author(s) and the copyright owner(s) are credited and that the original publication in this journal is cited, in accordance with accepted academic practice. No use, distribution or reproduction is permitted which does not comply with these terms.
*Correspondence: John Kormla Nyameasem, am55YW1lYXNAdW5pLWJvbm4uZGU=
†Present address: John Kormla Nyameasem, Institute of Crop Science and Resource Conservation (INRES), University of Bonn, Bonn, Germany