- 1Leibniz Centre for Agricultural Landscape Research (ZALF), Müncheberg, Germany
- 2Warsaw University of Life Sciences (SGGW), Department of Soil Science, Warsaw, Poland
The growing interest in amorphous silica (ASi) within the fields of soil science and ecology underscores the necessity for a reliable protocol to estimate ASi contents in soil. Alkaline wet chemical extraction methods are commonly employed for silicon (Si) extraction from operationally defined (x-ray) amorphous Si phases or short-range ordered mineral phases in soils and marine sediments. In our study we conducted a comparative analysis of four alkaline extraction methods (1% sodium carbonate, 0.5 M sodium carbonate, 0.2 M sodium hydroxide, and 0.1 M Tiron), assessing their extraction selectivity as well as effectiveness using soils artificially enriched with varying, defined amounts of ASi. While extraction effectiveness was evaluated by determining the recovery rate of initially added ASi, extraction selectivity was determined by measuring aluminum (Al) and iron (Fe) concentrations as indicators of the dissolution of non-target mineral phases. Microwave plasma atom emission spectrometry was used to analyze Al, Fe, and Si concentrations in the extracts. Our results indicate that extraction with 0.2 M sodium hydroxide yields the best outcomes in terms of both extraction effectiveness and selectivity. This more recent extraction technique is conducted at the most alkaline pH (13.3) of all four methods tested, but at ambient temperature (21°C) decreasing the dissolution of non-target mineral phases. Though, no wet-chemical extraction used on heterogeneous samples like soil is precisely selective, and thus able to quantify the target analyte only. Hence, data obtained by such procedures still need to be interpreted with caution considering all their limitations.
Introduction
Growing evidence suggests that silicon (Si) plays a vital role in maintaining plant health and soil functions under pressure, which has spurred significant research into Si biogeochemistry (reviewed in Schaller et al., 2021; Katz et al., 2021). Silicon has a variety of functions in plant biology including the defense against biotic and abiotic stresses (Puppe et al., 2023a; de Tombeur et al., 2023). In addition, amorphous silica (ASi) amendment can increase soils’ water holding capacity and plant available water, hence may play a more important role in soil water relation than currently recognized (Schaller et al., 2020; Zarebanadkouki et al., 2022).
Despite Si is the second most abundant element of the earth crust, soil Si availability for organisms (e.g., plants, sponges, or protists) is limited as they can take up Si only in the form of monomeric silicic acid (H4SiO4; Katz et al., 2021; Schaller et al., 2021). Soil solid phases of Si comprise crystalline forms like quartz and its polymorphs, feldspar, mica, olivine, pyroxene, and secondary clay minerals like illite, smectite and kaolinite, as well as short-range ordered phases (e.g., allophane, imogolite, opal-CT) and (x-ray) amorphous phases. Amorphous Si can be either of biogenic (bASi, e.g., phytoliths, testate amoeba shells, sponge spicules) or minerogenic (silica included in pedogenic oxides or precipitated onto other soil constituents in acidic soil environments) origin (Veerhoff and Brümmer, 1993; Sauer et al., 2006; Puppe, 2020).
The ultimate source of silicic acid for plant uptake is the weathering of the parent material comprising the solid phases named above. Once taken up by plants silicic acid condensates forming bASi in the form of phytogenic silica. Plant availability of Si depends on its concentration in solution which is driven by site conditions (e.g., soil pH, mineral composition, solubility of primary and secondary mineral phases) and management practices (e.g., straw recycling; Klotzbücher et al., 2018; 2020; Puppe et al., 2021). The solubility of solid mineral phases increases with decreasing crystallinity (crystalline < short-range ordered < (x-ray) amorphous) of these compounds which impacts the ability to provide dissolved Si (Cornelis and Delvaux, 2016). Hence, short-ranged ordered Al/Si phases and (x-ray) amorphous Si phases represent an important source of plant available Si (Schaller et al., 2021; Cornu et al., 2022).
However, due to intensified land use these Si sources substantially declined in agricultural soils, which is mainly caused by the removal of Si-rich biomass by harvest, especially of Si accumulating cereal crops (Struyf et al., 2010; Puppe et al., 2021). In agricultural soil-plant systems a quantification of the ASi pool is crucial for the estimation of the potential Si availability for plants. A poor soil Si status may be mitigated by Si fertilization or a change in management practice, e.g., by straw recycling (Puppe et al., 2021; Barão, 2022). High silicic acid concentrations in soil solution may lead to a precipitation as ASi, and may reduce translocation of potentially toxic metal cations like copper and cadmium (Stein et al., 2020; Stein et al., 2021; Puppe et al., 2023a). Furthermore, ASi addition was found to increase phosphorus availability as shown for fen peats as well as arctic and paddy soils (Schaller et al., 2019; 2022; Hömberg et al., 2020).
Research of the last decades provided different alkaline wet-chemical extraction methods to extract Si from operationally defined (x-ray) amorphous and short-range ordered mineral phases (e.g., allophanic and imogolite-type compounds) in soils and (marine) sediments (Sauer et al., 2006; Schaller et al., 2021). In general, these extraction techniques use strong bases (e.g., sodium hydroxide (NaOH)), weak bases (e.g., sodium carbonate (Na2CO3)) or complexation agents like Tiron (Foster, 1953; Biermans and Baert, 1977; DeMaster, 1991; Kendrick and Graham, 2004) as the solubility of ASi strongly increases at alkaline pH (Iler, 1979). However, these various extraction methods were developed for different purposes. Most of them aimed for purification of clay minerals for further x-ray diffraction (XRD) analyses of these crystalline phases.
Foster (1953) introduced a hot 0.5 M NaOH extraction method to extract Si from biogenic and minerogenic ASi, and aluminum oxides/hydroxides occluded in montmorillonite. Hashimoto and Jackson (1960) modified this method to extract Si and Al from allophane and kaolinite-halloysite by shortening the extraction time to decrease the dissolution of non-target silicate minerals. Follett et al. (1965) developed a 2-step Na2CO3 method to investigate inorganic amorphous material in the clay fractions of two soils. Additionally, they accessed the effects of the extraction on the clay fraction by various analytical techniques (e.g., XRD). Biermans and Baert (1977) used a hot 0.1 M 4.5-dihydroxy-l.3-benzenedisulfonic acid (disodium salt) (Tiron, C6H4Na2O8S2) solution to extract aluminum (Al), iron (Fe) and Si oxides/hydroxides from soil samples. In the early 1980s DeMaster (1981) introduced a 1% Na2CO3 extraction method to extract Si from biogenic ASi in marine sediments implementing a linear (mineral) correction of Si extracted from non-target phases (e.g., clay minerals) for the first time. Another approach was proposed by Koning et al. (2002). They used an alkaline leaching technique (0.5 M NaOH) for the simultaneous analysis of bASi and Al in marine sediments to discriminate between silica from biogenic and non-biogenic fractions. Various studies have since employed Na2CO3 and NaOH extractions to extract ASi from soil samples, e.g., the phytolith pool (Meunier et al., 2014; Barão et al., 2015; Schaller et al., 2021 and references therein).
Several attempts have been made to compare the existing alkaline extraction methods considering their extraction effectiveness and selectivity towards ASi. Follett et al. (1965) observed that NaOH extracted more Si from SROAS, but similar amounts of Si from kaolinite, illite and montmorillonite compared with Na2CO3. In a comparative study Kodama and Ross (1991) investigated the differences between NaOH (Hashimoto and Jackson, 1960), Na2CO3 (Follett et al., 1965), and Tiron (Biermans and Baert, 1977). From their results they concluded that all extractants dissolve Si from non-target mineral phases e.g., kaolinite and microcline, especially NaOH. Additionally they showed that Tiron extracts as much Si from Fe phases as a sodium oxalate extraction. Barão et al. (2015) compared the methods of DeMaster (1981) and Koning et al. (2002) to access biogenic ASi in different samples including marine sediments and soil. They concluded that the reliability of ASi extraction data decreases with decreasing initial ASi content of the sample as Si originating from non-target silicates dominates the Si solution concentration in the extracts, even after linear mineral correction. As most of the commonly used extraction methods aiming for ASi estimation in natural samples were insufficient considering their extraction selectivity and capacity Georgiadis et al. (2015) refined and modified the NaOH protocol introduced by Kamatani and Oku (2000). Georgiadis et al. (2015) observed that especially reaction temperature drives the dissolution of non-target mineral phases (selectivity) while having a lesser influence on extraction capacity of ASi. However, solution pH was shown to have the largest impact on extraction capacity. Thus, they recommend a cold extraction method using 0.2 M NaOH adjusted to pH 13.3 (Georgiadis et al., 2015).
However, to the best of our knowledge no attempt has yet been made to compare the recent low temperature extraction method of Georgiadis et al. (2015) with well-established protocols (e.g., 1% Na2CO3, 0.5 M Na2CO3, 0.1 M Tiron), partly suggesting higher extraction temperatures. For the first time, a comparative analysis was conducted using a soil artificially enriched with differing amounts of ASi to address this knowledge gap. Extraction effectiveness was analyzed by determining the recovery rate of initially added ASi. Extraction selectivity was determined by measuring Al and Fe concentrations as indicators of the dissolution of non-target mineral phases such as SROAS and Fe oxides/hydroxides. We aimed to comparatively assess four alkaline Si extraction protocols (0.2 M NaOH, 1% Na2CO3, 0.5 M Na2CO3, and Tiron) and to evaluate their selectivity and quantification effectiveness in extracting ASi from soil.
Results
Extraction effectiveness
Extraction effectiveness of the extractants was analyzed by determining the corresponding recovery rates of ASi added to the soil samples in different, defined amounts. Therefore, Si concentrations in the extracts were used to calculate the corresponding Si contents in the soil samples after subtracting the values obtained from control samples (no ASi added). The Si contents obtained from extractions with Tiron, 1% Na2CO3, and 0.2 M NaOH are in accordance with the added amounts (Figure 1). However, 0.5 M Na2CO3 showed a relatively poor extraction effectiveness as only minor amounts of the added ASi were extracted. The mean deviation of the extracted contents compared to the added contents was the smallest for 0.2 M NaOH (0.06%), followed by Tiron with 0.12% and 1% Na2CO3 with 0.15%. The 0.5 M Na2CO3 showed the highest difference (1.13%). Figure 2 shows the ASi content extracted by the respective extractant including the extracted background ASi content of the control. ASi contents obtained from all extraction procedures were very highly correlated with the added ASi contents (Figures 1, 2). The strongest correlation was found for 0.2 M NaOH (r = 0.999, p < 0.001), followed by Tiron (r = 0.996, p < 0.001), 0.5 M Na2CO3 (r = 0.991, p < 0.001), and 1% Na2CO3 (r = 0.981, p = 0.001). 0.5 M Na2CO3 extractions resulted in ASi contents that were markedly below the target range (Figures 1, 2). Tiron extracted the most Si from the control compared with NaOH and Na2CO3 extractions (Figure 3A).
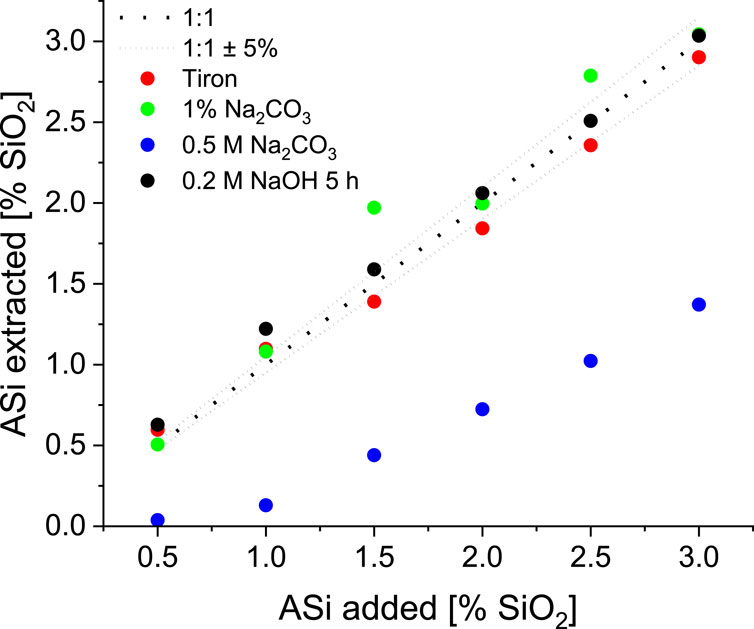
Figure 1. Relationship between extracted amorphous silica (ASi) contents and added ASi displayed in % SiO2. Silicon content extracted from the control (i.e., a Haplic Luvisol without ASi addition) is subtracted. The 1:1 line represents the optimum extraction effectiveness of the added ASi. The grey dotted lines represent the range of ±5% deviation from the optimum extraction of the target analyte.
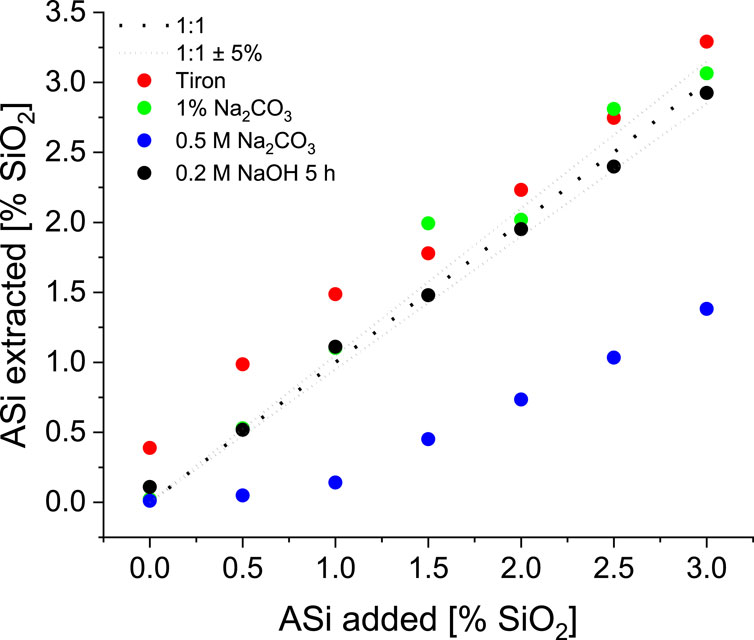
Figure 2. Relationship between extracted amorphous silica (ASi) contents and added ASi displayed in % SiO2. Background Si contents of the control (i.e., a Haplic Luvisol without ASi addition) are included. The 1:1 line represents the optimum extraction effectiveness of the added ASi. The grey dotted lines represent the range of ±5% deviation from the optimum extraction of the target analyte.
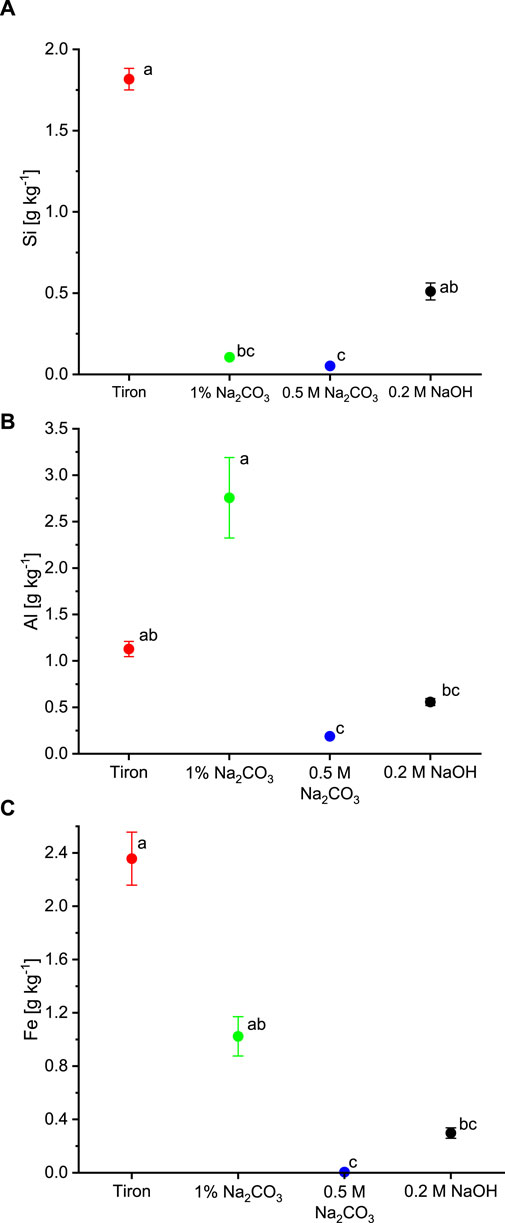
Figure 3. Si (A), Al (B) and Fe (C) contents (g kg−1) of the control (i.e., a Haplic Luvisol without amorphous silica addition) extracted by the respective alkaline extraction protocols. Different letters indicate statistically significant differences (p < 0.05, Kruskal–Wallis ANOVA).
Extraction selectivity
To distinguish between Si derived from minerogenic ASi sources or other mineral phases, Al and Fe concentrations were also measured in the extracts. A detection of these two elements after extraction accounts for the dissolution of non-target phases with respect to ASi (e.g., clay minerals or Fe oxides/hydroxides).
Aluminum and Fe were extracted with each extractant, however, in varying amounts (Figure 3 b and c) . Sodium carbonate (1%) extracted the highest amount of Al (mean 2.8 g kg−1), followed by Tiron (mean 1.1 g kg−1) and NaOH (5 h, mean 0.6 g kg−1). Sodium carbonate (0.5 M) extracted the least Al (mean 0.2 g kg−1) from the control (i.e., a Haplic Luvisol without ASi enrichment). Tiron extracted the highest Fe contents (mean 2.4 g kg−1), followed by 1% Na2CO3 (mean 1.0 g kg−1), and NaOH (mean 0.3 g kg−1). Again, 0.5 M Na2CO3 extracted the least Fe (mean 0.01 g kg−1). The highest Si contents were extracted by Tiron (mean 1.8 g kg−1), followed by NaOH (5 h, mean 0.6 g kg−1) and 1% Na2CO3 (mean 0.1 g kg−1). Again, 0.5 M Na2CO3 also extracted the least Si (mean 0.5 g kg−1) from the control (Figure 3A).
Due to the different solid-to-solution ratios (0.5 M Na2CO3 = 1:25; 0.2 M NaOH = 1:400; Tiron = 1:1,000; 1% Na2CO3 = 1:1,333) the concentrations in solution of the control samples varied strongly from the contents calculated for the solid phase. In solution, Si concentrations were the highest for 0.5 M Na2CO3 (mean 2.17 ± 0.04 mg L−1), followed by Tiron (mean 1.79 ± 0.03 mg L−1) and NaOH (mean 0.13 ± 0.01 mg L−1). Sodium carbonate (1%) showed the lowest Si concentration in solution of the control extracts (mean 0.08 ± 0.01 mg L−1) and the highest extracted Si contents due to the widest solid-to-solution ratio. The Al concentrations in the extracts of the control sample were the highest in the 0.5 M Na2CO3 (mean 7.56 ± 0.17 mg L−1), followed by Tiron (mean 0.94 ± 0.05 mg L−1) and 1% Na2CO3 (mean 0.21 ± 0.02 mg L−1). Sodium hydroxide (0.2 M) showed the lowest Al concentrations (mean 0.15 ± 0.01 mg L−1) in the extracts of the control. Iron concentrations in the extracts of the control samples were the highest for Tiron (mean 1.97 ± 0.14 mg L−1), followed by 0.5 M Na2CO3 (mean 0.20 ± 0.01 mg L−1) and 1% Na2CO3 and 0.2 M NaOH, which both showed a mean Fe concentration of 0.08 ± 0.01 mg L−1 in the extracts. Noteworthy, the Al concentration in the 0.5 M Na2CO3 extracts sharply decreased with increasing contents of initially added ASi. While the Al concentration in the control extracts was 7.56 mg L−1, it declined to 0.04 mg L−1 for the samples enriched with 3.0% ASi.
Discussion
Extraction effectiveness
Sodium hydroxide extraction at room temperature (developed by Kamatani and Oku, 2001; modified by Georgiadis et al., 2015) was the most effective extracting agent as it showed the smallest mean deviation between added and extracted ASi over the whole ASi content range. Differences in extraction effectiveness can be explained by the differing pH values of the extraction solutions with NaOH being the most alkaline solution (pH = 13.3) compared with the other extractants (0.5 M Na2CO3, pH = 11.8; 1% Na2CO3, pH = 11.2; Tiron, pH = 10.5). In general, the solubility of ASi strongly increases with pH (Iler, 1979; Georgiadis et al., 2015), so that more Si is released into solution with increasing pH of the extractant. However, Tiron having the lowest pH of all extractants showed comparable results with NaOH considering extraction effectiveness of ASi. Tiron is operating with different reaction mechanisms compared with NaOH and Na2CO3. Besides being an alkaline extractant, Tiron also acts as a complexing agent for Al and Fe (Biermans and Baert, 1977) and it dissolves allophane, imogolite, and poorly crystalline hydrous Fe oxides as effective as an oxalate extraction (acidic extraction targeting poorly crystalline Fe oxides in soil) (Kodama and Ross, 1991). Additionally, Tiron extraction is conducted at 80°C whereas the NaOH is examined at ambient conditions. The accuracy of ASi quantification further declined for the two Na2CO3 extractants. This is in line with other studies stating that Na2CO3 extraction is often too mild, i.e., the extraction capacity of Na2CO3 is lower compared to other alkaline extractants used for total dissolution of ASi phases in soil (Kodama and Ross, 1991) or plant samples (Puppe et al., 2023b). Hence, the pH of the Na2CO3 extractants may not be sufficiently alkaline to dissolve ASi with a single extraction (Kodama and Ross, 1991). However, comparing the two Na2CO3 extractions (Table 1), the DeMaster (1981) extraction conducted at 80°C showed a better extraction effectiveness targeting ASi than the method developed by Breuer and Herrmann (1999), which is conducted at ambient (room) temperature. These results may be explained by the higher extraction temperature of the DeMaster (1981) protocol (80°C vs 21°C). The 0.5 M Na2CO3 extraction procedure basically failed to extract the added ASi contents. The narrow solid-to-solution ratio of this protocol resulted in relatively high Si and Al concentrations in solution. Furthermore, the ionic strength (IS) of 0.5 M Na2CO3 is the highest of all used extractants. The precipitation of Si and/or Al:Si phases depends on their own concentration in solution, the solution pH and the IS of the solution (Dietzel, 2002; 2005). Al and Si solution concentrations where the highest in the 0.5 M Na2CO3 extracts as well as the IS of the extractant. Hence, Al and/or Si may underwent (co-)precipitation of ASi and/or short-range ordered Al:Si phases. Consequently, the ability of 0.5 M Na2CO3 to extract Si may be sufficiently high. However, Al and Si may be removed from solution due to their (co-)precipitation. This assumption is underpinned by the decrease of Al solution concentration with increasing Si concentration in the extracts. The lowest Al concentrations were observed in the samples with highest ASi (3%) addition, whereas the highest Al concentrations were measured in the control samples without any ASi addition. Thus, this method may just be suitable for samples in which low ASi contents are expected.
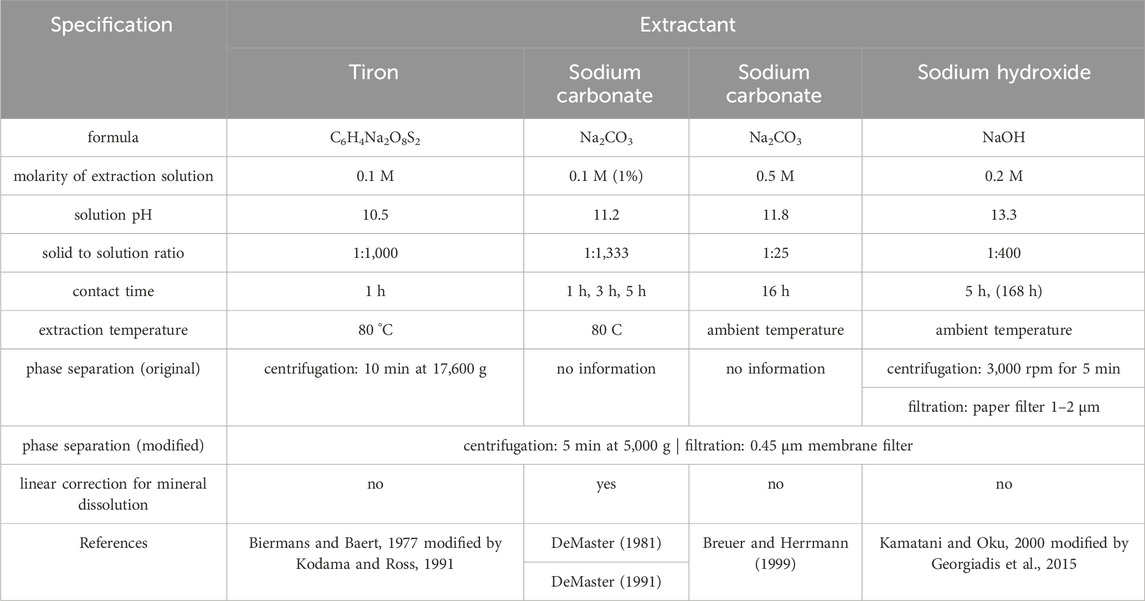
Table 1. Summary of alkaline extraction methods applied including information on original and modified extraction protocols.
Extraction selectivity
Extraction selectivity was observed by analyzing Al and Fe concentrations in the extracts. Increasing Al and Fe concentrations are an indicator for the dissolution of Al and Fe bearing mineral phases like clay minerals or iron oxides. As these non-target minerals also release Si into solution ASi concentrations in the extracts are overestimated to a certain extent (decreased extraction selectivity).
The low ASi concentrations in the 0.5 M Na2CO3 extracts were rather resulting from precipitation of Al and/or Si than from an insufficient dissolution of ASi itself. This is why this method is not further discussed regarding extraction selectivity below. Extraction of Si from ASi phases was most selective using the method developed by Georgiadis et al. (2015), as the lowest amounts of Al and Fe were extracted by 0.2 M NaOH at ambient (room) temperature. Hence, during this extraction the least Si release from clay minerals was observed. These results can be explained by the relatively low extraction temperature, as Si release from clay minerals and primary silicates decreases with temperature (Georgiadis et al., 2015). Georgiadis et al. (2015) showed that at ambient conditions minor amounts of Al and Si are released from smectite and no Al was released from kaolinite and feldspar samples. Dissolution of these mineral phases increased with temperature. In our study, the Na2CO3 (1%) extraction performed at 85°C extracted the most Al from the control samples, followed by Tiron. As described above these results can be explained by the relatively high temperature of these extractions. However, compared with the hot NaOH extraction, Tiron was found to attack crystalline minerals to a lesser extent (Kodama and Ross, 1991). The most Fe was extracted by Tiron. This is in line with the findings of Kodama and Ross (1991) showing that Tiron extracts as much Fe and Al from Fe- and Al-oxides as an oxalate extraction.
DeMaster’s (1981) extraction is the only method of the four methods tested in our study, which takes the dissolution of mineral phases into account by correcting the amount of Si derived from mineral dissolution. Such a correction works well on sediment samples from aquatic environments (DeMaster, 1981). These correction procedures require constant Al:Si ratios of ASi and other mineral phases. However, as soils are usually characterized by more diverse clay mineral assemblages with varying Al:Si ratios and ASi with varying amounts of Al, the preconditions for a meaningful correction are less well fulfilled in soil compared to sediment samples (Sauer et al., 2006). Barão et al. (2015) found substantial contributions from non-biogenic Si sources during alkaline extractions of Si following a modified DeMaster (1981) protocol even after linear mineral correction. They suggested that continuously monitored Al:Si ratios during extraction (Koning et al., 2002) may be used to differentiate between biogenic and non-biogenic sources of the extracted Si. Moreover, they concluded that the term ASi may be inappropriate regarding samples where silicate minerals contribute to the extracted Si to a large extent (e.g., samples from subsoil horizons, which are predominantly characterized by low ASi contents). Georgiadis et al. (2015) showed that Al and Fe concentrations decreased with decreasing extraction temperature indicating a decreasing dissolution of non-target Si bearing solid phases. This is generally corroborated by the results of our study. Out of the four alkaline extraction methods tested, NaOH (0.2 M) extraction at ambient (room) temperature showed the best performance regarding extraction selectivity and effectiveness. However, mineral phases may exist as a continuum from completely disordered phases to well crystallized material (Follett et al., 1965), making it hard to distinguish between crystalline, short-range ordered and amorphous phases. When interpreting results of wet-chemical extractions, one must bear in mind that no wet-chemical extraction method is completely selective and able to quantify the target analyte exclusively. Hence, general identification of individual species by such extractions has been shown to be impossible (Barão et al., 2015; Rennert, 2019). This restriction needs to be considered to avoid improper and careless interpretation of wet-chemical extraction data. Nevertheless, these methods provide a cost-effective and easily manageable analytical approach, especially compared with spectroscopic approaches, which require laborious data analyses and are characterized by relatively high costs (Rennert, 2019).
Conclusion
As ASi gets increasing attention in soil science and ecology, the demand for a reliable protocol to estimate ASi in soil samples becomes imperative. Our study aimed to comparatively assess four established alkaline Si extraction protocols (0.2 M NaOH, 1% Na2CO3, 0.5 M Na2CO3, and Tiron) evaluating their selectivity and quantification effectiveness in extracting ASi from soil. Extraction effectiveness, measured by tracking artificial ASi added to soil samples in different amounts, revealed that the NaOH (0.2 M) extraction performed at ambient (room) temperature was the most effective method. This extraction agent showed the smallest mean deviation between added and extracted ASi across the entire ASi content range of the samples. Concerning extraction selectivity, which is crucial for distinguishing between Si derived from ASi sources and non-target soil constituents, measurements of Al and Fe concentrations in the extracts indicated that NaOH also demonstrated the highest selectivity, releasing the least amounts of Al and Fe from non-target phases. Therefore, NaOH (0.2 M, room temperature) emerged as the most promising extractant striking a balance between extraction effectiveness and selectivity. It is essential to recognize, however, that no wet-chemical extraction method is entirely selective, and thus, an exclusive quantification of the target analyte alone is not possible. Consequently, data obtained through such procedures should be interpreted with caution, considering their inherent limitations. Despite these constraints, wet-chemical extraction methods remain a cost-effective and manageable analytical approach for ASi estimation, especially considering the absence of available spectroscopic methods for ASi quantification in soils. Future research on alkaline wet-chemical extraction should aim to refine protocols by concurrent spectroscopic analyses identifying and characterizing the removed compounds.
Materials and methods
Soil sampling and preparation
Soil samples were collected at ZALF’s landscape laboratory, the “AgroScapeLab Quillow” (Uckermark, Germany). An Ap horizon of a Haplic Luvisol developed from boulder clay was sampled using an auger at a sampling depth of 20 cm. After sampling, the soil was air dried at room temperature, homogenized, and sieved (<2 mm). The soil was characterized by a bulk density of 1.51 g cm−3, a sand content of 586 g kg−1, a silt content of 321 g kg−1, and a clay content of 93 g kg−1 (Rieckh et al., 2012).
To test the extraction effectiveness of the alkaline extractants an artificial ASi compound (Aerosil 300; Evonik Industries, Essen, Germany) in varying amounts (i.e., 0.5%, 1.0%, 1.5%, 2.0%, 2.5%, and 3.0%) was added to the soil. Aerosil, a pyrogenic ASi, was used as it showed physicochemical properties comparable to biogenic ASi (Schaller et al., 2019; 2020). The soil without ASi addition served as control.
Extraction procedures
All tested methods were modified regarding phase separation as phase separation by filtering and centrifugation significantly alters solution phase concentration (Rennert, 2019; Rennert and Lenhardt, 2022). In this study all samples were centrifuged at 5,000 g for 5 min (Table 1). The supernatants were subsequently filtered (0.45 µm, PET membrane syringe filters; Macherey-Nagel, Düren, Germany).
The extractions were performed in fivefold repetition for each ASi treatment and extraction method.
NaOH
It is known that hot NaOH extraction dissolves clay minerals to a significant extent (e.g., Follett et al., 1965; Kamatani and Oku, 2001). Hence, hot NaOH extraction is not sufficiently selective for Si estimation from amorphous pools, so that an optimized NaOH extraction protocol was used in this study following Kamatani and Oku (2001), modified by Georgiadis et al. (2015) extracting ASi at ambient temperature (21 °C). Therefore 8 g of NaOH (Carl Roth, Karlsruhe, Germany) were dissolved in 1,000 mL of deionized water (18.2 MΩ·cm, silica <2 ppb) resulting in a 0.2 M NaOH solution. Adjustment of pH to 13.3 was conducted by dropwise adding of concentrated NaOH. Subsequently, 100 mg of soil sample were weighted into 50 mL centrifuge tubes. Then, 40 mL of 0.2 M NaOH were added to the samples (solid/solution ratio = 1:400). Samples were gently shaken using a swivel roller shaker. After five and 168 h, the samples were centrifuged and subsequently filtered.
Na2CO3
Sodium carbonate extraction was applied according to two different protocols. One followed DeMaster (1981) (1% Na2CO3, pH = 11.2, 85 °C, solid/solution = 30 mg:40 mL) and the other Breuer and Herrmann (1999) (0.5 M Na2CO3, pH = 11.8, ambient temperature, solid/solution = 2 g:50 mL).
For extraction after DeMaster (1991) 10.086 g of Na2CO3 (Carl Roth, Karlsruhe, Germany) were dissolved in 1,000 mL of deionized water (18.2 MΩ·cm, silica <2 ppb) resulting in a 1% Na2CO3 solution. Subsequently, 30 mg of soil sample were weighed in 50 mL centrifuge tubes and exposed to 40 mL of 1% Na2CO3 solution at 85 °C for 5 h. Samples were gently shaken by hand before heating in a water bath and after every hour while heating. Subsamples were taken after 1, 3 and 5 h. After sampling, the extracts were centrifuged and filtered. The weight percentage of extracted ASi was plotted against time. The extrapolated intercept at time zero corresponds to the silica content of (biogenic) amorphous sources of the sample. This analytical method is based on the distinction between the rapid dissolution of silica from minerogenic and biogenic (x-ray) amorphous sources and the slower release of Si from coexisting clay minerals, avoiding overestimation of Si from the ASi pool.
For extraction after Breuer and Herrmann (1999) 52.995 g of Na2CO3 (Carl Roth, Karlsruhe, Germany) were dissolved in 1,000 mL of deionized water (18.2 MΩ·cm, silica <2 ppb) resulting in a 0.5 M Na2CO3 solution. Subsequently, 2 g of soil were weighed in 100 mL polyethylene bottles and exposed to 50 mL of 0.5 M Na2CO3 solution at ambient temperature for 16 h on a swivel roller shaker. Finally, the extracts were centrifuged and filtrated.
Tiron
Tiron extraction procedure followed the method developed by Biermans and Baert (1977) and modified by Kodama and Ross (1991). The extraction solution was prepared by dissolving 31.42 g of Tiron (4,5-dihydroxy−1,3-benzene-disulfonic acid [disodium salt], C6H4Na2O8S2; Roth, Karlsruhe, Germany) in 800 mL of deionized water (18.2 MΩ·cm, silica <2 ppb), followed by addition of 100 mL Na2CO3 solution (Carl Roth, Karlsruhe, Germany; 5.3 g Na2CO3 in 100 mL deionized water) under constant stirring. The pH was adjusted to 10.5 by incrementally adding 4 M NaOH solution (Carl Roth, Karlsruhe, Germany). For extraction, 30 mg of soil were weighed into 50 mL centrifuge tubes. Subsequently, 30 mL of Tiron solution were added and then heated at 80°C in a water bath for 1 h. Prior heating and after 30 min samples were gently shaken by hand. Phase separation was conducted by centrifuging (5,000 g for 5 min) and filtration (0.45 µm).
Spectrometric analyses
Silicon concentrations in the extracts were analyzed by microwave-plasma atomic emission spectrometry (MP-AES; 4210 MP-AES instrument, Agilent, Waldbronn, Germany) using its most sensitive atomic lines with negligible interferences at 251.611 nm (Puppe et al., 2024). To test the selectivity of the extractants Al and Fe concentrations were measured at 396.152 nm and 371.993 nm, respectively. Blank sample element concentrations were subtracted from soil sample element concentrations in the respective extracts before further calculations. Element (Al, Fe, and Si) contents in the soil samples were calculated considering the respective weighed soil portions, extractant volumes, and the degree of dilution (1:10).
Statistical analyses
Linear relationships in the data set were analyzed via Pearson’s (r) correlations (α level of 0.05). Differences between means were tested with the Kruskal–Wallis analysis of variance (ANOVA) followed by pairwise multiple comparisons (Dunn’s post hoc test). Statistical analyses were performed with the software package SPSS Statistics (version 22.0.0.0, IBM Corp.).
Data availability statement
The raw data supporting the conclusion of this article will be made available by the authors, without undue reservation.
Author contributions
MS: Conceptualization, Formal Analysis, Investigation, Methodology, Validation, Visualization, Writing–original draft, Writing–review and editing. DP: Writing–original draft, Writing–review and editing, Formal Analysis, Visualization. DK: Writing–review and editing. CB: Investigation, Writing–review and editing. JS: Conceptualization, Funding acquisition, Project administration, Resources, Writing–review and editing.
Funding
The author(s) declare that financial support was received for the research, authorship, and/or publication of this article. The research leading to these results has received funding from the Leibniz Cooperative Excellence (K378/2021).
Acknowledgments
The analytical support by Dinkayehu Asrade and Oliver Hunfeld is gratefully acknowledged.
Conflict of interest
The authors declare that the research was conducted in the absence of any commercial or financial relationships that could be construed as a potential conflict of interest.
Publisher’s note
All claims expressed in this article are solely those of the authors and do not necessarily represent those of their affiliated organizations, or those of the publisher, the editors and the reviewers. Any product that may be evaluated in this article, or claim that may be made by its manufacturer, is not guaranteed or endorsed by the publisher.
References
Barão, L. (2022). The use of Si-Based fertilization to improve agricultural performance. J. Soil Sci. Plant Nutr. 23 (1), 1096–1108. doi:10.1007/s42729-022-01106-1
Barão, L., Vandevenne, F., Clymans, W., Frings, P. J., Ragueneau, O., Meire, P., et al. (2015). Alkaline-extractable silicon from land to ocean: a challenge for biogenic silicon determination. Limnol. Oceanogr. Methods 13 (7), 329–344. doi:10.1002/lom3.10028
Biermans, V., and Baert, L. (1977). Selective extraction of the amorphous Al, Fe and Si oxides using an alkaline Tiron solution. Clay Min. 12, 127–135. doi:10.1180/claymin.1977.012.02.03
Breuer, J., and Herrmann, L. (1999). Eignung der Extraktion mit Natriumbikarbonat für die Charakterisierung von bodenbildenden Prozessen. Mittlg. Dtsch. Bodenk. Ges. 91, 1375–1378.
Cornelis, J.-T., and Delvaux, B. (2016). Soil processes drive the biological silicon feedback loop. Funct. Ecol. 30, 1298–1310. doi:10.1111/1365-2435.12704
Cornu, S., Meunier, J., Ratié, C., Ouédraogo, F., Lucas, Y., Merdy, P., et al. (2022). Allophanes, a significant soil pool of silicon for plants. Geoderma 412, 115722. doi:10.1016/j.geoderma.2022.115722
DeMaster, D. J. (1981). The supply and accumulation of silica in the marine environment. Geochim. Cosmochim. Acta 45 (10), 1715–1732. doi:10.1016/0016-7037(81)90006-5
DeMaster, D. J. (1991). “Measuring biogenic silica in marine sediments and suspended matter,” in Geophysical monograph (American Geophysical Union), 363–367.
De Tombeur, F., Raven, J. A., Toussaint, A., Johnson, S. N., Cooke, J. R., Hartley, S., et al. (2023). Why do plants silicify? Trends Ecol. Evol. 38 (3), 275–288. doi:10.1016/j.tree.2022.11.002
Dietzel, M. (2002). “Interaction of polysilicic and monosilicic acid with mineral surfaces,” in Water-rock interaction. Editors I. Stober,, and K. Bucher (Netherlands: Kluwer), 207–235.
Dietzel, M. (2005). Impact of cyclic freezing on precipitation of silica in Me-SiO2-H2O systems and geochemical implications for cryosoils and -sediments. Chem. Geol. 216, 79–88. doi:10.1016/j.chemgeo.2004.11.003
Follett, E. A. C., McHardy, W. J., Mitchell, B. D., and Smith, B. F. L. (1965). Chemical dissolution techniques in the study of soil clays: Part I. Clay Min. 6, 23–34. doi:10.1180/claymin.1965.006.1.04
Foster, M. (1953). Geochemical studies of clay minerals III. The determination of free silica and free alumina in montmorillonites. Geochim. Cosmochim. Acta 3 (2–3), 143–154. doi:10.1016/0016-7037(53)90003-9
Georgiadis, A., Sauer, D., Breuer, J., Herrmann, L., Rennert, T., and Stahr, K. (2015). Optimising the extraction of amorphous silica by NaOH from soils of temperate-humid climate. Soil Res. 53 (4), 392. doi:10.1071/sr14171
Hashimoto, J., and Jackson, M. L. (1960). “Rapid dissolution of allophane and kaolinite-halloysite after dehydration,” in Proceedings of the 7th nat. Conf. Clays clay miner. Editor E. Ingerson (Elsevier), 102–113.
Hömberg, A., Obst, M., Knorr, K.-H., Kalbitz, K., and Schaller, J. (2020). Increased silicon concentration in fen peat leads to a release of iron and phosphate and changes in the composition of dissolved organic matter. Geoderma 374, 114422. doi:10.1016/j.geoderma.2020.114422
Iler, R. K. (1979) The chemistry of silica: solubility, polymerization, colloid and surface properties and biochemistry. Wiley.
Katz, O., Puppe, D., Kaczorek, D., Prakash, N. B., and Schaller, J. (2021). Silicon in the soil-plant continuum: intricate feedback mechanisms within ecosystems. Plants (Basel) 10 (4), 652. doi:10.3390/plants10040652
Kendrick, K. J., and Graham, R. C. (2004). Pedogenic silica accumulation in chronosequence soils, Southern California. Soil Sci. Soc. Am. J. 68, 1295–1303. doi:10.2136/sssaj2004.1295
Klotzbücher, T., Klotzbücher, A., Kaiser, K., Merbach, I., and Mikutta, R. (2018). Impact of agricultural practices on plant-available silicon. Geoderma 331, 15–17. doi:10.1016/j.geoderma.2018.06.011
Klotzbücher, T., Treptow, C., Kaiser, K., Klotzbücher, A., and Mikutta, R. (2020). Sorption competition with natural organic matter as mechanism controlling silicon mobility in soil. Sci. Rep. 10 (1), 11225. doi:10.1038/s41598-020-68042-x
Kodama, H., and Ross, G. J. (1991). Tiron dissolution method used to remove and characterize inorganic components in soils. Soil Sci. Soc. Am. J. 55, 1180–1187. doi:10.2136/sssaj1991.03615995005500040047x
Koning, E., Epping, E., and van Raaphorst, W. (2002). Determining biogenic silica in marine samples by tracking silicate and aluminium concentrations in alkaline leaching solutions. Aquat. Geochem. 8, 37–67. doi:10.1023/A:1020318610178
Meunier, J. D., Keller, C., Guntzer, F., Riotte, J., Braun, J. J., and Anupama, K. (2014). Assessment of the 1% Na2CO3 technique to quantify the phytolith pool. Geoderma 216, 30–35. doi:10.1016/j.geoderma.2013.10.014
Puppe, D. (2020). Review on protozoic silica and its role in silicon cycling. Geoderma 365, 114224. doi:10.1016/j.geoderma.2020.114224
Puppe, D., Buhtz, C., Kaczorek, D., Schaller, J., and Stein, M. (2024). Microwave plasma atomic emission spectroscopy (MP-AES) — a useful tool for the determination of silicon contents in plant samples? Front. Environ. Sci. 12, 1378922. doi:10.3389/fenvs.2024.1378922
Puppe, D., Kaczorek, D., Buhtz, C., and Schaller, J. (2023b). The potential of sodium carbonate and Tiron extractions for the determination of silicon contents in plant samples – a method comparison using hydrofluoric acid digestion as reference. Front. Environ. Sci. 11, 1145604. doi:10.3389/fenvs.2023.1145604
Puppe, D., Kaczorek, D., Schaller, J., Barkusky, D., and Sommer, M. (2021). Crop straw recycling prevents anthropogenic desilication of agricultural soil–plant systems in the temperate zone – results from a long-term field experiment in NE Germany. Geoderma 403, 115187. doi:10.1016/j.geoderma.2021.115187
Puppe, D., Kaczorek, D., Stein, M., and Schaller, J. (2023a). Silicon in plants: alleviation of metal(loid) toxicity and consequential perspectives for phytoremediation. Plants 12, 2407. doi:10.3390/plants12132407
Rennert, T. (2019). Wet-chemical extractions to characterise pedogenic Al and Fe species – a critical review. Soil Res. 57 (1), 1. doi:10.1071/sr18299
Rennert, T., and Lenhardt, K. R. (2022). Does phase separation quantitatively affect Al, Fe, and Si extraction from soil using acid oxalate? Geoderma 412, 115728. doi:10.1016/j.geoderma.2022.115728
Rieckh, H., Gerke, H. H., and Sommer, M. (2012). Hydraulic properties of characteristic horizons depending on relief position and structure in a hummocky glacial soil landscape. Soil Tillage Res. 125, 123–131. doi:10.1016/j.still.2012.07.004
Sauer, D., Saccone, L., Conley, D. J., Herrmann, L., and Sommer, M. (2006). Review of methodologies for extracting plant-available and amorphous Si from soils and aquatic sediments. Biogeochemistry 80 (1), 89–108. doi:10.1007/s10533-005-5879-3
Schaller, J., Cramer, A., Carminati, A., and Zarebanadkouki, M. (2020). Biogenic amorphous silica as main driver for plant available water in soils. Sci. Rep. 10 (1), 2424. doi:10.1038/s41598-020-59437-x
Schaller, J., Faucherre, S., Joß, H., Obst, M., Goeckede, M., Planer-Friedrich, B., et al. (2019). Silicon increases the phosphorus availability of Arctic soils. Sci. Rep. 9 (1), 449. doi:10.1038/s41598-018-37104-6
Schaller, J., Puppe, D., Kaczorek, D., Ellerbrock, R., and Sommer, M. (2021). Silicon cycling in soils revisited. Plants 10 (2), 295. doi:10.3390/plants10020295
Schaller, J., Wu, B., Amelung, W., Hu, Z., Stein, M., Lehndorff, E., et al. (2022). Silicon as a potential limiting factor for phosphorus availability in paddy soils. Sci. Rep. 12, 16329. doi:10.1038/s41598-022-20805-4
Stein, M., Georgiadis, A., Gudat, D., and Rennert, T. (2020). Formation and properties of inorganic Si-contaminant compounds. Environ. Pollut. 265, 115032. doi:10.1016/j.envpol.2020.115032
Stein, M., Georgiadis, A., Ingwersen, J., and Rennert, T. (2021). Does silica addition affect translocation and leaching of cadmium and copper in soil? Environ. Pollut. 288, 117738. doi:10.1016/j.envpol.2021.117738
Struyf, E., Smis, A., Van Damme, S., Garnier, J., Govers, G., Van Wesemael, B., et al. (2010). Historical land use change has lowered terrestrial silica mobilization. Nat. Commun. 1, 129. doi:10.1038/ncomms1128
Veerhoff, M., and Brümmer, G. W. (1993). Bildung schlechtkristalliner bis amorpher Verwitterungsprodukte in Stark bis extrem versauerten Waldböden. Z. Pflanzenernähr. Bodenkd. 156, 11–17. doi:10.1002/jpln.19931560103
Keywords: amorphous silica, SROAS, wet-chemical extraction, soil silicon, alkaline extraction method comparison
Citation: Stein M, Puppe D, Kaczorek D, Buhtz C and Schaller J (2024) Silicon extraction from x-ray amorphous soil constituents: a method comparison of alkaline extracting agents. Front. Environ. Sci. 12:1389022. doi: 10.3389/fenvs.2024.1389022
Received: 20 February 2024; Accepted: 02 May 2024;
Published: 15 May 2024.
Edited by:
Sören Thiele-Bruhn, University of Trier, GermanyReviewed by:
Zsuzsanna Balogh-Brunstad, Hartwick College, United StatesBjörn Klaes, University of Trier, Germany
Copyright © 2024 Stein, Puppe, Kaczorek, Buhtz and Schaller. This is an open-access article distributed under the terms of the Creative Commons Attribution License (CC BY). The use, distribution or reproduction in other forums is permitted, provided the original author(s) and the copyright owner(s) are credited and that the original publication in this journal is cited, in accordance with accepted academic practice. No use, distribution or reproduction is permitted which does not comply with these terms.
*Correspondence: Mathias Stein, bWF0aGlhcy5zdGVpbkB6YWxmLmRl
†ORCID: Mathias Stein, orcid.org/0000-0002-7356-3045