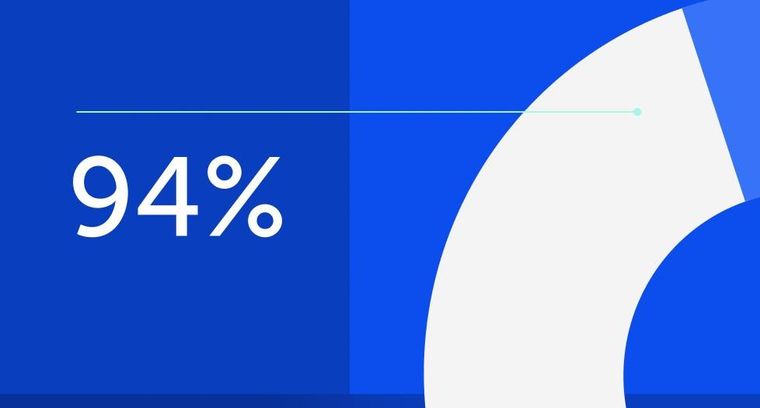
94% of researchers rate our articles as excellent or good
Learn more about the work of our research integrity team to safeguard the quality of each article we publish.
Find out more
ORIGINAL RESEARCH article
Front. Environ. Sci., 08 May 2024
Sec. Toxicology, Pollution and the Environment
Volume 12 - 2024 | https://doi.org/10.3389/fenvs.2024.1370820
This article is part of the Research TopicRemediation and Health Risks of Heavy Metal Contaminated SoilsView all 14 articles
Landfills and waste disposal sites in the Basque Country are summarized in the inventory of soils that either currently support or have supported potentially polluting activities or facilities (Law 4/2015). Notably, “Landfill 17,” located in Gernika-Lumo, has been receiving, for decades, sewage sludges from the local wastewater treatment plant (WWTP) as agricultural amendment. In order to decontaminate and recover soil functionality, a combination of bioremediation (which involved bioagumentation and phyto- and vermitechnologies) and complementary bioremediation strategy (i.e., promotion and maintenance of the native vegetation) was implemented in situ. Physicochemical and ecotoxicological characterization were achieved. Furthermore, an ecotoxicological assessment of the soils upon flora and fauna was carried out through the application of different bioassays and biomarkers. Additionally, an integrative biomarker response (IBR/n) index was calculated to provide a holistic view of the soil general status. Critical pollutants [Cd, Cr, Ni, Pb, benzo(a)pyrene, and dieldrin] were observed in most of the treated sites. Microbial parameters did not present remarkable differences among sites. However, plant indicators pointed the non-treated site (MN8) as the unhealthiest. This was also observed in earthworms’ immune system, where cytotoxicity appears when exposed to non-treated soils. In conclusion, this field study showed that the combination of bioaugmentation, phytoremediation with native species, and vermiremediation is highly useful in eliminating mixed contamination, improving soil health, and ultimately restoring ecosystem functionality and biodiversity.
The intensification of human activities and rapid industrialization stands as a primary cause of environmental pollution and degradation (Antoci et al., 2018) mainly due to faulty spillages, landfills, and industrial waste disposals, threatening human and ecosystem health. Soil pollution has emerged as a major environmental concern due to its relevance supporting essential services for the society (Míguez et al., 2020). Thus, the degradation and loss of ecosystems pose a great threat to human safety and health, leading to scarcity of productive lands. In order to mitigate the ecological and human health risks linked with soil pollution, the recognition of the resulting ecological and human health hazards requires management and remediation techniques, with the last one relying on physicochemical and, where feasible, biological remediation technologies (Jan et al., 2015). Nowadays, physicochemical techniques are widely used, although they can lead to problems such as soil erosion or fertility and functionality loss, thus breaking ecosystem balance and inducing negative and irreversible effects upon biodiversity (Jan et al., 2015; Morillo and Villaverde, 2017). As a sustainable alternative, several biological remediation (bioremediation) methods, such as those based on microbes (e.g., bioaugmentation), plants (e.g., phytoremediation), and earthworms (vermiremediation), have been demonstrated to be cost-effective tools, despite the drawback of being more time-consuming processes to mitigate. Bioaugmentation, phytoremediation, and vermiremediation have recently been applied in soils concomitantly polluted with organic and inorganic compounds (hereafter co-polluted) due to their high removal efficiencies (Lacalle et al., 2018; Lacalle et al., 2020; Aparicio et al., 2021), which are even higher when combined (Lacalle et al., 2020; Aparicio et al., 2021; Urionabarrenetxea et al., 2021).
In 2012, 16.5% of the total surface of the Basque Country (Spain) was considered potentially contaminated, while 2.7%–6.5% was considered already contaminated (Basque Government, 2023). Thus, the Basque Country, 2023 Government developed an inventory of soils that support or have supported potentially polluting activities or facilities (Law 4/2015) that has been updated in 2022 (Basque Government, 2023). This inventory includes “Landfill 17,” located in Gernika-Lumo (Basque Country, Spain), where sewage sludges—from the local wastewater treatment plant (WWTP)—were diffusely scattered for decades as amendment with agricultural purposes. Here, several quantitative risk analyses were carried out by the University of the Basque Country (UPV/EHU) highlighting the worst case scenarios (MN1, MN2, MN3, MN4, MN5, MN7, and MN8) with threatening pollutants [cadmium, lead, nickel, chromium benzo(a)pyrene, and dieldrin] (Urionabarrenetxea et al., 2021). Based on the gathered data, the local administration prohibited the use of these soils with agricultural purposes, suggesting the application of remediation techniques. Thus, Urionabarrenetxea et al. (2021) conducted a field trial in “Landfill 17” to ascertain the best bioremediation technologies (bioaugmentation and phyto- and vermiremediation) or their combination for contaminant reduction. As a result of the mentioned study, the combination of the three technologies was selected as the most efficient method and applied at large scale in the whole “Landfill 17” by using Burkholderia xenovorans LB400 and Penibacillus sp. bacterial strains for the bioaugmentation; alfalfa (Medicago sativa) for phytoremediation; and Eisenia fetida earthworms for vermiremediation.
Subsequently, quantitative risk analysis showed the efficiency, in terms of risk reduction, of the large-scale application of the triple bioremediation technique. However, and after considering the nature of the contamination, the local administration kept banning the use of lands with agricultural purposes and recommended keeping remediating the area in the following years. Therefore, in April 2021, a complementary bioremediation strategy based on the promotion and maintenance of the native vegetation was carried out at “Landfill 17.” The main objective of the study was twofold: to continue stabilizing and removing soil contaminants and, simultaneously, restore and recover ecosystem health and biodiversity, all through the establishment of native herbaceous and woody species, such as Salix sp. or Alnus sp.
To assess the effectiveness of a bioremediation process, it is necessary to evaluate the ecosystem’s health status through biological indicators spanning different trophic levels (Pankhurst et al., 1997; Garbisu et al., 2007), in order to assess aboveground and belowground biota status. Microbial parameters measured in soil microbial communities (e.g., soil respiration, microbial carbon biomass, and functional diversity) along with plant production and diversity are essential for comprehensively assessing soil health and recovery, due to their direct linkage with ecosystem processes and their high sensitivity (Pankhurst et al., 1997; Gómez- Sagasti et al., 2012, 2021).
Furthermore, toxicity assays are useful tools to evaluate and monitor the presence of soil contamination and its effects (Aparicio et al., 2019; Urionabarrenetxea et al., 2021, 2022). These assays rely on the use of sentinel/model organisms to evaluate toxicity. To assess soil phytotoxicity, one of the most used assays is the root elongation test. The US-EPA (1982) guideline recommends the use of Cucumis sativus L. as the sentinel species, among others, due to its sensitivity and quick response (Visioli et al., 2014; Franco et al., 2017; Lacalle et al., 2018). Similarly, Eisenia fetida earthworm has been widely used as an indicator of soil health and toxicity (Irizar et al., 2015b) in standard tests (OECD, 1984) and field works (Aparicio et al., 2021; Urionabarrenetxea et al., 2021) to evaluate the toxic effects of single or mixtures of pollutants. Earthworms can take up chemicals via the dermis—from leachates or porewater and via the digestive tract—by soil ingestion (Rodriguez-Campos et al., 2014).
Biomarkers are measurements at the tissue, cellular, biochemical, and molecular levels that have been raised as a tool to detect changes at lower organization levels, providing early signs of alterations at the population, community, or ecosystem levels (Cajaraville et al., 1993). Recently, the use of multi-biomarker approaches has gained significance in evaluating the health status of co-polluted soils from a holistic point of view (Htwe et al., 2022). For instance, oxidative stress can not only be assessed by measuring glutathione-S-transferase (GST), carboxylesterase (CbE), and catalase (CAT) activities but also by measuring carbonyl protein and malondialdehyde (MDA) content. GST is a phase II biotransformation enzyme that helps detoxify pollutants by conjugating xenobiotics with GSH and making them get more easily excreted (Hellou et al., 2012). CbE is an esterase that belongs to a superfamily of enzymes that hydrolyze carboxylic ester bonds and is another enzyme that aids in the excretion of xenobiotics (Wang et al., 2018). CAT, on the other hand, consists of an antioxidant enzyme, responsible for the degradation of H2O2, preventing the formation and accumulation of reactive oxygen species (ROS) that can damage cells (Asensio et al., 2013). Carbonyl protein is a marker of protein oxidative damage in earthworms under stress (Vasylkiv et al., 2010), and MDA is a product of lipid membrane peroxidation that is formed upon oxidative stress through the production of reactive oxygen species; it can reflect the level of biological damage, being an indicator of the cellular membrane status (Pirinccioglu et al., 2010).
At the cellular level, immune cells of earthworms named coelomocytes have been widely studied in the last decades as the target system to assess contaminated sites, as they have demonstrated the capacity to predict alterations caused by contamination (Plytycz and Morgan, 2011a; Kwak et al., 2014; Irizar et al., 2015a; García-Velasco et al., 2019; Urionabarrenetxea et al., 2022). E. fetida coelomocytes are divided into two main subpopulations: amoebocytes and eleocytes. Amoebocytes accomplish phagocytosis of foreign particles, while eleocytes contribute to homeostasis in earthworms (Plytycz et al., 2009; Plytycz et al., 2010). Similarly, when organisms are exposed to environmental pollution, reactive oxygen species (ROS) are produced, leading to oxidative damage in proteins, nucleic acids, lipids, and other macromolecules (Hellou et al., 2012).
To achieve a holistic perspective of the health status of an ecosystem (Beliaeff and Burgeot, 2002; Broeg and Lehtonen, 2006), the integrative biomarker response (IBR/n) index is a great tool that integrates biomarkers and biological indicators at different biological levels and provides a reliable picture of the health status of a population or community.
As noted, field-scale bioremediation studies are required with a comprehensive risk assessment that includes physical, chemical, and biological, as well as ecotoxicological indicators. The present field study addresses this gap in knowledge and aims to 1) characterize soil’s potential toxicity and soil health status of the co-polluted “Landfill 17” and 2) assess the recovery of soil health and biodiversity in one and a half years after the implementation of the complementary remediation strategy, not only through physical–chemical indicators but also through biological and ecotoxicological indicators representative of the soil biota (microbial, plants, and worms).
In October 2022, a year-and-a-half after the establishment of the complementary bioremediation strategy, the soil and vegetation samples were collected from the six sampling sites previously selected by the administration for pollution and remediation monitoring at “Landfill 17,” located in Gernika-Lumo (Basque Country, Spain). These sites were denoted as MN1, MN2, MN3, MN4, MN5, and MN7. Each of them had a size of 2 m × 2 m. As we have indicated above, MN8 was considered to be the control (non-treated) site.
Three biological replicates were uniformly distributed in each site, using quadrats of 50 cm × 50 cm size each. First, the vegetation cover of each quadrat was manually collected to estimate plant biomass and plant biodiversity and to determine phytoextraction. Subsequently, sets of soil samples were manually collected in each quadrat to a depth of 0.2 m for the analysis of physical–chemical and biological properties and ecotoxicological assays.
For the ecotoxicological assays, cucumber (Cucumis sativus L.) seeds and Eisenia fetida earthworms were used. Cucumber C. sativus seeds were obtained from a commercial provider (Semillas Batlle) and maintained at constant temperature (4°C) and dark conditions, under humidity not exceeding 10%. Among the stocked seeds, only those without abnormalities and similar size were chosen. Meanwhile, E. fetida earthworms were purchased from the commercial supplier Lombrinatur (Almeria, Spain), and specimens were maintained under constant and controlled conditions (19°C and 60% relative humidity until the experiment). Stocked earthworms were weekly fed with antibiotic-free horse manure. For the assay, clitellated organisms between 300 and 600 mg were employed (OECD, 1984).
Once in the terrarium, the soil samples were sieved (2 mm) and kept in the cold chamber to be used for organic matter content determination and chemical and ecotoxicological characterization. Additionally, the water holding capacity (WHC) of each biological replicate was estimated based on the dry and humidity weights of the soils in order to achieve OECD (1984) ideal conditions for the standardized test.
In soil samples, the organic matter was quantified with weight loss on ignition at 550°C; a complete chemical characterization was also carried out encompassing the analysis of up to 84 compounds. For this chemical characterization, 200 g of each soil sample was used. Heavy metals (As, Cd, Cr, Cu, Hg, Mo, Ni, Pb, and Zn) were analyzed through ICP-MS (Inductively coupled plasma mass spectrometry). Meanwhile, the majority of phenols, hydrocarbons, and aromatic and halogenated compounds were analyzed by using GC-MS (Gas chromatography/Mass spectrometry). A part of the hydrocarbons were analyzed using GS-FID (Gas Chromatography With Flame Ionization Detection).
This study focused on Cd, Cr, Ni, Pb, benzo(a)pyrene, and dieldrin, as they are classified as critical pollutants by the local government. The pollution index (PI) was calculated according to Hakanson (1980), so the obtained data were normalized for each pollutant according to the reference values in the legislation (VIE-B values classified as “other uses”) established by the Basque Country norm (Law 4/2015). The following equation was used: PI = C/Cn, where C corresponds to soil concentration and Cn corresponds to threshold value from the Basque norm. Then, the sum of all individual pollution indexes was obtained for each site.
At the same time, soil leachates were prepared as indicated by the Spanish legislation BOE, 2002 A-1697 (BOE núm. 25), following the German standard DIN 38414-S4 (Deutsches Institut fur Normung, 1984 480/id) (Urionabarrenetxea et al., 2022). Thus, 10 grams of dry soil were mixed with 100 mL of distilled water and stirred for 24 h to afterwards be filtered through a 0.45-μm pore PVDF filter. Ni, Cr, Cd, and Pb were quantified through GC-FID by external analysis in SGIker general services of UPV/EHU, Leioa, and the leachates were stored at 4°C until use.
In order to assess microbial communities, parameters related with their activities and functional richness were measured in three soil biological replicates per sample. Basal respiration (mg C kg−1 h−1) was determined following ISO 16072 (2002), measuring CO2 evolution. According to Epelde et al. (2008), the average well color development (AWCD), number of utilized substrates (NUS), and Shannon’s diversity index (H′ = −Σpi log2 pi) were determined from Biolog EcoPlates™.
Plants from each quadrat were harvested and taxonomically identified at the genus level and, if possible, at the species level in order to determine the diversity of each site. Plant diversity was calculated using the Shannon Index [H′ = −Σ(pi log2 pi), where pi is the proportional dry weight of the i species]. Once in the laboratory, shoots of woody and herbaceous plants were separated by species, dried at 80°C for 48 h, and weighted to determine the production of plant biomass.
Metal extraction in plants was determined in dried shoots, although for Salix atrocinerea, only the leaves were analyzed. Following the US-EPA Method 3051A (2007) protocol with modifications, the plant samples were oven dried at 80°C for 48 h to be milled afterward. A representative and homogeneous analytical sample of each biological replicate weighing approximately 0.25 g was subjected to acid digestion. Once the digestion process was completed, the samples were cooled and diluted with ultrapure water until a maximum concentration of HNO3 <1%. All samples were externally analyzed by AGRUPAlab (reference UNE-EN ISO/IEC 17025:2017), where Cd, Cr, and Ni were quantified through ICP-MS using all inner standards, following the QA/QC criteria established by the ENAC (Spanish National Accreditation Body).
To assess soil phytotoxicity, the root elongation bioassay with cucumber (C. sativus) was carried out following the EPA 850.4230 modified protocol (Lacalle et al., 2018).Briefly, the seeds were pre-germinated under controlled conditions (photoperiod 14/10 h day/night, temperature 25/18°C day/night, and photosynthetic photon flux density of 100 μmol photon m−2 s−1). Concurrently, 10 g of soil from each biological replicate of each site was sieved (2 mm) and dried in an incubator for 7 days at room temperature (25°C) to prevent microbial community damage. Dried soils were placed in Petri dishes with 10 mL of deionized water. Finally, a black filter paper (Filter-Lab® 1457) was placed on top of each Petri dish with moistened soil.
After 72 h, seven pre-germinated seeds were positioned in the dishes with the soils and sealed with Parafilm® to be incubated in a Sanyo germination chamber for another 72 h under the same conditions above. Images of the seedlings were taken at the beginning and after the incubation to be processed utilizing Fiji-ImageJ software (Schindelin et al., 2012). Root elongation was calculated using the formula
In order to evaluate potential toxicity through dermal exposure, the OECD “filter paper test” guideline (OECD, 1984) was followed, with mortality and weight loss being the main endpoints. A total of 10 technical replicates per treatment were prepared in vials containing 1 mL of elutriate and one specimen. The experiment was carried out under constant temperature (19°C) and dark conditions. Earthworms were weighted at the beginning and after 72 h; mortality was recorded after this period.
Soil test with E. fetida (OECD, 1984) was performed to consider a more realistic scenario where the digestive path could also be relevant for the toxicity assessment. In this case, three biological replicates per group were used, and OECD soils were used as the negative control (labeled as “Control”). All soil samples were moistened with deionized water up to 40% of their WHC, as previously estimated (Section 2.2.2). In each glass container, 750 g of soil and 10 specimens were placed. After 14 days (under 19°C and constant light conditions), mortality and weight loss were measured.
The composition of OECD artificial soil was (dry weight): 70% of industrial sand, 20% kaolin clay, and 10% sphagnum peat. A pH of 6.0 ± 0.5 was achieved by adding 0.1% of powdered calcium carbonate (CaCO3).
After soil testing, five earthworms per biological replicate were separated for chemical analysis. After depurating their gut contents in a filter paper for 24 h, the earthworms were preserved at −80°C and subjected to lyophilization. An external chemical analysis through ICP-MS was carried out by SGiker General Services at EHU/UPV (Leioa) after a previous microwave digestion. The metal species Cd, Cr, Ni, and Pb were detected using all inner standards following the QA/QC criteria established by the ENAC (Spanish Agency for Accreditation).
Four earthworms per treatment were reacquired after 14 days of exposure and gently massaged to remove soil particles retained in their digestive tracts. Coelomocyte extrusion was done by immersing them into extrusion fluid (1 mL, PBS with 0.02% EDTA/earthworm) and subjecting them to electrical stimulation (9 V) (Irizar et al., 2014a). This allows the release of coelomic fluid and coelomocytes from the dorsal pores (Irizar et al., 2014a).
The obtained solution was transferred into falcon tubes and centrifuged. The supernatant was then removed, and the pellet was resuspended with PBS solution. Afterward, the cells were counted in a hemocytometer (Neubauer chamber), and the final concentration was adjusted to 1.106 cells mL−1 in all treatments. Regarding the viability assay, solutions were placed in 96-well microplates and incubated to let coelomocytes stabilize and then centrifuged to complete cell adhesion to the bottom.
Coelomocyte viability was assessed by the calcein–acetoxymethyl (calcein-AM) assay according to García-Velasco et al. (2019). This is a fluorometric method that provides a simple, rapid, and accurate response of cytoxicity by dyeing with calcein-AM. Dye enters viable cells where it is converted by intracellular esterases to calcein that provides an intense signal when (with an intact plasma membrane) is retained by cells. Firstly, the supernatant was discarded from the plates and the cells were incubated with 2.5 µM calcein-AM (100 µL per well, six replicates).
In parallel, three wells per experimental group were filled with 100 µL of PBS instead of calcein-AM to measure basal fluorescence of the cells. A cleaning step was carried out by removing the supernatant and replacing it with PBS twice. Excitation was performed at 490 ± 20 nm, and emission was measured at 520 ± 20 nm in a Flex fluorometer for the plates.
Two earthworms per biological replicate were taken from the soil test containers after 14 days of exposure, frozen directly in liquid nitrogen, and kept at −80°C for further analyses. A total of 200 mg of the post-clitellar section of the earthworm was dissected according to Irizar et al. (2014b) and homogenized using homogenization buffer (50 mM Tris-sucrose, pH 7.4, 1:2 w/v) in a Precellys 24-Dual at 4°C. The suspension was centrifuged, and the supernatant was collected and divided in aliquots, which were preserved at −80°C and used to analyze the activity of the enzymes: glutathione-S-transferase (GST), carboxylesterase (CbE), catalase (CAT), and acetylcholinesterase (AChE) and the levels of malondialdehyde (MDA), carbonyl proteins, and total protein quantification. All analyses were performed in 96-well flat-bottom microplates, measuring each biological replicate in duplicate using a BioTek Eon microplate spectrophotometer at 20°C.
Oxidative stress in E. fetida was assessed measuring the levels of 1) detoxification enzymes, reflected by glutathione S-transferase (GST) and carboxylesterase (CbE); 2) effects upon the antioxidant defense system, by analyzing catalase activity (CAT). Additionally, to evaluate general stress and effects of pollution in the nervous system, acetylcholinesterase (AChE) was measured (Tiwari et al., 2016).
Thus, GST activity was determined according to Keen et al. (1976), measuring the formation of the CDNB-GSH conjugate at 340 nm in 28 µL of the sample for 2 min. CAT activity was analyzed according to Aebi (1984), where H2O2 consumption by CAT in 3 µL of the sample for 1 min was measured at 240 nm. CbE and AChE enzyme activities were analyzed separately according to Ellman et al. (1961), using a 5,5-dithiobis-2-nitrobenzoic acid (DTNB) solution. For CbE, the reaction started when 10 µL phenylthioacetate was added to a mixture of 5 µL of the sample with DTNB. Similarly, for AChE, acetylthioacetate iodine was used as the substrate in a 10-µL sample. The absorbance at 412 nm was followed for 2 min.
For protein quantification, 10 µL of the sample was analyzed through the Bradford method (Bradford, 1976), utilizing 200 µL of the Bradford reagent. Quantification was performed measuring absorbance at 595 nm, and concentrations were obtained through a calibration curve developed with bovine serum albumin as the standard, whose concentrations ranged from 0 to 3.5 mg mL−1. Enzyme activities were expressed as U mg−1 protein or mU mg−1 protein, U corresponds to the enzyme units. It is important to note that enzyme activity has been represented as percentage with respect to the control.
Lipid peroxidation was assessed by quantifying carbonyl protein and MDA. Carbonylated proteins were quantified by adapting the method from Parvez and Raisuddin (2005), measuring 2,4-dinitrophenylhydrazine protein derivate at 370 nm. Protein carbonyl levels were expressed as percentage with respect to the control and calculated using the quantified protein content and the corresponding extinction coefficient (Ɛ370 = 0.022 mM−1 cm−1). MDA was determined following Almeida et al. (2005) by measuring spectrophotometrically the thiobarbituric acid derivate of malonaldehyde at 535 nm. The MDA levels were expressed as percentage with respect to the control and calculated based on a tetramethoxypropane standard curve with concentration values within 0 and 1.5 μmol mL−1.
Data analyses were performed using the RStudio software (version 4.2.2) and graphs using Excel. Test for outliers was carried out using the Grubbs test and removed if detected according to the reported criteria (Meshalkin et al., 2023). Data normality and homoscedasticity were checked using the Shapiro–Wilk and Levene’s tests, respectively. Parametric data were studied with one-way ANOVA coupled with Tukey for pairwise comparisons and Dunnett’s test, to study differences between sites (MN1–MN7) with the non-treated site (MN8) and negative controls. Non-parametric data were studied using the Kruskal–Wallis test, followed by Dunn’s test. The confidence at the 95% level was considered.
To integrate the different biomarker responses from “Landfill 17” soils at different biological and ecological levels, biomarkers from the biochemical to the community levels—acetylcholinesterase (AChE), glutathione S-transferase (GST), calcein content (CAL), plant biomass (PB), and basal respiration (BR)—were selected to provide a holistic and simplified view of the general state of the soil (Beliaeff and Burgeoot, 2002; Marigómez et al., 2013).
The IBR was calculated using a multivariate method, according to the following procedure: 1) calculation of the mean and standard deviation for each sample; 2) standardization of the data for each sample xi′ = (xi − x)/s, where x is the standardized value of the biomarker, xi represents the biomarker value of each sample, x is the mean value, and s is the standard deviation of the biomarkers calculated from all compared samples; 3) addition of the standardized value calculated for each sample to the absolute standardized value of the minimum value in the data set: yi = xi′ + |xmin′|; 4) making of the start plot and calculation of the triangular areas as Ai = (yi × yi + 1 × sinα)/2, where yi and “yi + 1” are the standardized values of each biomarker and the next biomarker in the start plot, respectively, and “α” is the angle (in radians) formed by the two consecutive axes; and 5) calculation of the IBR index, IBR = ΣAi·IBR value is directly dependent on the number of biomarkers used, so the obtained values of IBR must be divided by the number of biomarkers used (IBR/n), according to Broeg and Lehtonen (2006).
At the end of the remediation strategy, in October 2022, the concentrations of all the critical pollutants [Cd, Cr, Ni, Pb, and B(a)P] were reduced compared with levels in 2021 (Table 1) in all the soils, except in MN3, where the metal concentrations were higher (Table 2). Cd and B(a)P still were above the reference values established by the Basque Country norm (Law 4/2015) in all sites. Despite this, MN8 soils (non-treated) remain the most polluted site, with values of Cd, Ni, and B(a)P above the reference values.
Table 1. Basque Country norm thresholds of the critical compounds [cadmium, chromium, nickel, lead, benzo(a) pyrene, and dieldrin] and their measured concentrations in all the selected soils from “Landfill 17” in 2021 and its respective pollution index (PI).
Table 2. Basque Country norm thresholds of the critical compounds [cadmium, chromium, nickel, lead, benzo(a) pyrene, and dieldrin], their measured concentrations, the respective pollution index of each soil, and the organic matter (OM) content in “Landfill 17” soils collected in October 2022.
In 2022, soils from “Landfill 17” showed a higher presence of heavy metals than dieldrin and B(a)P, which were considerably below the overall (Table 2). In fact, dieldrin was under the limit of detection in all the sampling points (Table 2). Regarding heavy metals, Cr displayed the highest average concentrations with values ranging from 77.93 mg·kg−1 (MN4) to 371.67 mg·kg−1 (MN8, non-treated). MN8 also presented high levels of Ni, achieving a value of 154.00 mg·kg−1. Meanwhile, MN3 soils (historically the most polluted) showed the highest concentrations for Cd and Pb, 12.07 and 85.13 mg·kg−1, respectively. As overall, the highest pollution indexes were obtained for MN3 and MN8 points. According to the reference values, Cd concentrations trespassed the thresholds in all the soils except for MN7. Furthermore, MN8 showed values above the norm for all the critical compounds apart from Pb (Table 2). Regarding the organic matter (OM) content (Table 2), all the soils showed relatively high values, with the highest values being measured in MN3 (11.97) and MN4.
Leachates obtained from “Landfill 17” soils reached remarkably low concentrations, as it is represented in Supplementary Table S2. Despite this, MN1 soils presented the highest values for Cd, Ni, and Pb of 7.4∙10−3, 5.69∙10–2, and 4.2∙10–3 mg·kg−1, respectively. For Cr, the maximum value was recorded in MN8 soils (1.19∙10−1 mg·kg−1). On the opposite end, MN7 soils (farthest location from the WWTP) showed the lowest concentrations of Cd, Cr, Ni, and Pb of 8∙10−4, 5.4∙10−3, 6.8∙10−3, and 9∙10−4 mg·kg−1, respectively.
Of the microbial parameters (Supplementary Figures S4–S7), basal respiration, Shannon diversity index, and NUS did not show significant differences between treatments. However, significantly higher values in MN3 (0.58) were observed for AWCD than were for MN1 (0.51), MN2 (0.50), and MN8 (0.50). Likewise, basal microbial respiration reached the maximum in MN4 soils (3.23 mg C·kg−1·h−1) and the minimum in MN2 (2.44 mg C·kg−1·h−1). For the Shannon index, similar values were recorded in all the sampling points with estimations approximately 3.93 (MN3) and 3.60 (MN7). Following a similar trend, NUS also achieved the highest value in MN3 soils (19.33) and the lowest in MN7 (15.30).
Plant biomass showed significant differences in MN1 and MN7 compared to MN8 soils, which having the highest plant diversities displayed the lowest biomass values (Figure 1). Statistical differences were also seen between MN3 and MN1 with a higher biomass value recorded in MN1. MN1 showed the highest biomass and low diversity due to the dominating presence of Salix atrocinerea (Figure 1). Although no significant differences were obtained for plant diversity (Shannon index), Poaceae family individuals emerged as the most abundant in terms of diversity, apart from MN7 and MN8 sampling points. Epilobium hirsutum was the second most abundant, growing at MN2, MN3, and MN4. Overall, the highest diversity values were recorded at MN8, MN7, and MN3 sites, while MN2 and MN1 exhibited the lowest plant diversity levels.
Figure 1. Plant diversity expressed as percentage considering total biomass as 100%. Biomass of each soil is represented with the size of the graph which is normalized with these values.
Metal extraction, represented in Supplementary Table S8, showed remarkably higher rates of Cd in MN1 (sites subjected to the dominating presence of Salix atrocinerea), exhibiting 10-fold higher extraction values than the rest of the sites, whose values ranged from 2.59 g·ha−1 (MN8) to 12.28 g·ha−1 (MN7). Ni extracted concentrations by the vegetation reached the maximum in the MN1 site (17.91 g·ha−1), while the lowest contents were measured in MN3 and MN8 sites. Contrariwise, the Cr MN1 site presented the lowest concentrations and the highest in MN3 and MN8, where the intragroup variability for this pollutant exceeded 100% of the mean value, masking differences with the other sites.
C. sativus pre-germinated seeds did not show statistical differences in root elongation when exposed to “Landfill 17” soils for 72 h, as can be seen in Supplementary Figure S9. Despite a similar growth being described in all the samples with values ranging from 62% to 83%, exposure to MN3 soils determined a slightly higher (not significant) growth rate for C. sativus roots. On the contrary, a lower growth tendency was exhibited by pre-germinated seeds on being exposed to MN1 soils for 72 h.
After 72 h of exposure to the leachate-moistened filter paper, the recorded mortalities were all below 10%, and no significant weight losses were recorded. Nevertheless, earthworm weight loss in this test showed lower values in MN3 (12.68%) than in MN1, MN4, and MN8, thus these soils displayed the highest weight losses for dermal exposure, reaching 20.40%, 20.65%, and 20.57%, respectively.
After 14 days of exposure to co-polluted soils from “Landfill 17,” earthworm mortality was lower than 10%. The weight loss recorded variability within MN1, MN2, MN3, MN4, and MN8 soils trespassed above a 100% of the mean value, thus no significant differences were recorded. Moreover, as is represented in Figure 2, earthworms gained weight with respect to their initial weight with some of the treatments. This happened notably when exposed to MN8 soils, where the lowest weight loss value was achieved (−11.22%). On the contrary, earthworms exposed to the control (OECD soils) displayed the highest weight loss value, losing 17.93% of their initial value.
Figure 2. Weight loss (% respect initial weight) of Eisenia fetida earthworms exposed for 14 days to “Landfill 17” soils moistened up to 40% of the WHC of each soil. Error bar represents standard deviation. Different letters represent significant differences studied through one-way ANOVA (p-value < 0.05).
In earthworms exposed to landfill soils for 14 days, Cd was the most accumulated metal in tissues for all the treatments compared with the control, exhibiting the highest values for MN1, MN2, and MN8 at 17.27, 16.73, and 11.54 mg·kg−1, respectively (Table 3). The highest Cr, Ni, and Pb accumulations were measured in MN8. Contrariwise, Pb was the pollutant with the lowest accumulation values, with quantifications from 0.56 (control) to 5.39 mg·kg−1 (MN8).
Table 3. Concentrations (mg·kg−1) of cadmium, chromium, nickel, and lead in Eisenia fetida tissue after 14 days of exposure to “Landfill 17” soils measured through ICP-MS.
After 14 days of exposure, calcein signal was measured on coelomocytes extruded from earthworms, as represented in Supplementary Figure S10, which presented significantly lower values in MN8 (79.26% respect to the control) as against MN3, MN4, and MN7 where the signal displayed the highest levels of 127.3%, 126.66%, and 122.00%, respectively.
The GST activity (Figure 3A) did not significantly differ from the control at all the points. However, earthworms exposed to MN1 soils showed significantly higher enzyme activity values (reaching an average value of 131.18% with respect to the control) than MN2 and MN8 soils that showed enzyme activities of approximately 52.80% and 49.92%, respectively.
Figure 3. Glutathione S-transferase (GST) (A), carboxylesterase (CbE) (B), catalase (CAT) (C), acetylcholinesterase (AChE) (D), carbonyl protein (E), and malondialdehyde (MDA) (F) activities measured in Eisenia fetida after 14 days of exposure to “Landfill 17” soils. Error bar represents standard deviation. Different letters indicate significant differences analyzed through Tukey and Dunn’s tests (p-value < 0.05). A lack of letters indicates the absence of statistical differences among groups.
The CbE activity (Figure 3B) in earthworms belonging to the control, MN1, and MN2 soils showed a trend of increase in relation to the other groups; however, there were no significant differences (p > 0.05). The mean for MN1 and MN2 was 88.06% and 106.47%, respectively, while the values for MN3, MN4, and MN7 varied from 54.26% to 56.00%. However, earthworms exposed to MN8 soils showed light CbE activity inhibition with an average value of 72.06%.
For CAT activity (Figure 3.C), the control showed significantly higher activity than MN2 and MN4 soils, presenting the highest inhibition levels with an average value of 63.82% and 63.26%, respectively. The highest production was also recorded in MN1 soils, with an average value of 113.20% with respect to the control and an important variability within the replicates. However, no significant differences were noted.
The AChE activity (Figure 3D) was significantly decreased in the MN4 group (55.65%) when compared to the control and MN1, with 100% and 126.75%, respectively. For the other groups, there was also a decrease in the activity compared to the control, although differences were not significant.
Protein carbonyl (Figure 3E) increased in all exposure groups compared to the control; however, no significant differences were recorded. Furthermore, for MDA (Figure 3F), the levels at points MN2 (142.53%), MN3 (114.40%), and MN4 (109.28%) were higher than those of the control (100%), MN1 (80.30%), and MN8 (78.38%), but there were no significant differences between the groups. It has to be taken into account that the remarkable variability between replicates exhibited in MDA for most of the treatments, which included the control group, exceeded 50% of the mean value.
The responses of five biomarkers (AChE, GST, CAL, PB, and BR) are represented in six axes start site in Supplementary Figure S11. The general distribution of the integrative response index displays the most severe affectation in MN8 impated (2.91) explained by GST and CAL, even though AChE was the less responsive biomarker in these soils. Contrariwise, AChE showed the highest signal at MN4, where basal respiration was shown to be the most sensitive parameter, but GST and CAL could not explain the affectation of these soils. Although in MN1, AChE and basal respiration were the two most sensitive parameters, while the CAL signal did not explain the impact on this point.
In addition, MN3 and MN7 were more influenced by basal respiration, and MN3 is also explained by GST, CAL, and AChE signals. As MN7 showed the lowest affectation according to the IBR/n index (1.26), the remaining biomarkers did not seem to be related with the affectation in this soil. Despite MN2 also exhibiting a low affectation value (1.37), basal respiration turned out as the most sensitive parameter, supported by weight loss and GST signal; AChE is slightly related with the degree of impact in this soil.
As it is represented in Figure 4, the general overview of this index denotes the worst ecosystem health status in the MN8 soils, followed by MN1. On the contrary, following the index, the healthiest soils are the ones from MN2.
After 1.5 years of complementary remediation strategy, the concentrations of Cd, Cr, Ni, Pb, benzo(a)pyrene, and dieldrin were found reduced in all the points, except in MN3. In this point, metal concentrations increased maybe due to the diffuse character of the contamination and the high agronomical alterations suffered during the intensive research assay carried out by Urionabarrenetxea et al. (2021). Soils from MN8 (non-treated) exhibited values for Cr, Cd, Ni, and B(a)P above the legal thresholds in the Basque Country.
In fact, metals were removed between 20% and 32%, while B(a)P exhibited elimination yields approximately 50% (Supplementary Material). For dieldrin, the final concentration could not be obtained because they were under the LOD, although they were below the reference value. The abovementioned reductions were probably influenced by plant growth that improves rhizosphere interactions by releasing root exudates. These interactions help to create a suitable environment for microbial development, increasing rhizodegradation and microbial degradation processes (Reilley et al., 1996; Epelde et al., 2009; Stefanowicz et al., 2012; Nui et al., 2021; Gómez-Sagasti et al., 2021). Different authors (Park et al., 2011; Niu et al., 2021) have already reported that plants can influence metal uptake and/or immobilization through different interactions in the rhizosphere. However, plant growth was not the unique factor to be taken into consideration regarding pollutants toxicity. It is essential to consider bioavailability affected by soil physicochemical properties, such as OM content, cation exchange capacity, granulometry, or pH, due to their strong influence on pollutants adsorption and availability to biological organisms (Berthelot et al., 2009; Lacalle et al., 2018). In agreement with other studies (Gondek and Kopec, 2006; Gondek et al., 2014; Lacalle et al., 2018; Urionabarrenetxea et al., 2021, 2022), metals are strongly absorbed by soil OM content through the formation of strong metal-OM ligands. The aged character of contamination from “Landfill 17,” where active sludges were poured 30 years ago, leads to highly persistent OM-metal bounds, making the pollution remarkably recalcitrant (Lacalle et al., 2018; Urionabarrenetxea et al., 2021). This is directly linked to low metal concentrations (mostly ppb order) present in the leachates, suggesting that soil physicochemical properties, along with the influence of rhizosphere interactions, hindered the migration of contaminants to the aqueous matrix. This hypothesis is reinforced by the fact that if the pollution is mobile, it would have already been washed and transported through the fluvial system.
Following the same pattern, a low migration of the compounds was observed (Supplementary Table S3), with Pb being less mobilized with a migration between 0.014% and 0.055%. This could be related to the predominance of non-soluble species that could be strongly attached to the soil, thereby affecting the removal efficiency. Otherwise, Cr also had low migration to the lixiviates. Gondek and Kopec (2006), Lacalle et al. (2020), and Urionabarrenetxea et al. (2021) reported that this metal is strongly correlated with OM levels, suggesting the potential formation of metal-OM ligands. However, MN1 showed the highest Cd mobilization (1.25%), probably due to the notorious presence of Salix sp., whose organic acid and peptides in root exudates release metals from soil colloids due to changes in pH (Greger, 2005).
Once the soil quality improvement was assessed, the soil health status was diagnosed by using measurements at different levels of biological complexity and applying the following three different methodological approaches: evaluating soil microbiological communities, regarding the effects upon soil flora, and studying the impacts upon soil fauna (i.e., earthworms).
Microorganisms (especially bacteria and fungi) form most of the soil biomass and diversity, performing a key role in different soil processes and participating in the majority of the soil ecosystem services. This makes microbial parameters good descriptors of the ecosystem health status (Garbisu et al., 2007; Gómez-Sagasti et al., 2012). In fact, AWCD, NUS, and the Shannon index are frequently used to assess microbial communities' functional richness. Thus, AWCD differences in MN3 may be related to the alterations linked with the intensive experimental procedure carried out by Urionabarrenetxea et al. (2021). However, NUS and the Shannon index did not present significant differences. Furthermore, basal respiration did not present significant differences among groups, so it can be stated that conditions for microbiota are similar (or similarly affected) among the sites, mainly because the microbial parameters are carried out in the native bacterial communities of the sites and all the treated sites had the same bioaugmentation treatment. These native microbial communities must be highly adapted to soil conditions of the site, unlike non-native plants and worms used in bioassays.
Regarding ecological amelioration and restoration of contaminated soils, plant growth and diversity are frequently used as indicators of soil health status (Gómez-Sagasti et al., 2021). In fact, the scarce but diverse native flora found in MN8 soils (non-treated) could suggest a competition between species for resources. Moreover, the existing pollution together with high flood probability due to the proximity to the estuary could also be related with the scarce biomass generated. On the other hand, the highest production and a less diverse native vegetation were present in MN1, dominated by Salix atrocinerea, a species tolerant to a variety of conditions such as flooding and disturbed soils. The high biomass of this species can explain the high phytoextraction at the site MN1 and could greatly contribute to remove metals and organic pollutants in this site. It must be mentioned that a high percentage of Epilobium hirsutum was observed in MN4 soils. It was reported by Biurrun et al. (2008) that this species together with Mentha longifolia shaped the hygronitrophilous communities in the Basque Country. These communities are commonly linked with degraded environments settled over alluvial deposits as is the case of MN4, which is at the closest point to the WWTP and the most altered by inert material or human activity.
As could be expected, soils subjected to complementary treatment exerted a positive effect on plant production. Although alfalfa was planted at the site, in the long term, it was progressively displaced by the colonization of native vegetation, better adapted to the periodic flooding conditions of the site. In fact, we find greater plant biodiversity and observe that most of the final biomass was due to native vegetation contributing to the improvement of soil health. Regarding metal accumulation in plant tissues, low values for metals were recorded in all the sites, maybe due to the already mentioned low metal bioavailability. However, the non-treated MN8 soils showed the lowest accumulation values, especially for Cd. On the contrary, MN1 showed the highest accumulation values, which is positively correlated with the presence of S. atrocinerea. This fast-growing species has a very high biomass, and it has been reported to be a high metal accumulator (Landberg and Greger, 1996; Unterbrunner et al., 2007), although different variations can be found between species (Greger, 2005). In accordance with the obtained results, Moreno-Jimenez et al. (2009) had reported high extraction of Cd by Salix atrocinerea. This, together with its tolerance to several edaphoclimatic factors make this species an excellent candidate for remediation soils such as those in this study with mixed contamination and periodic flooding.
Phytotoxicity of treated “Landfill 17” soils was carried out with the root elongation test. Despite root elongation being considered the most sensitive endpoint, as its inhibition indicates the first evident effect of metal toxicity (Ali et al., 2004; Visioli et al., 2014), no significant differences in root elongation were observed among the groups. Thus, it can be suggested that “Landfill 17” soils did not exert any phytotoxic effects upon C. sativus seedlings. These may be explained by the low concentrations observed in the leachates and phytoextracted fraction, which could suggest that pollutants' migration and/or the available fraction was very low.
Efroymson et al. (1997) developed, for terrestrial plants, a toxicological benchmark for contaminants with a potential concern effect, where the established thresholds were 4 mg Cd·kg−1, 1 mg Cr·kg−1, 50 mg Pb·kg−1, and 30 mg Ni·kg−1. The concentrations estimated in this work are found to be far from the abovementioned values. Furthermore, Aparicio et al. (2019) obtained for Cr, in Lactuca sativa—also a dicotyledonous species, an EC50 value of 90 mg·kg−1, while An et al. (2004) reported in C. sativus EC50 values for Cd and Pb of approximately 102 and 403 mg·kg−1, respectively, which are also very far from those measured in this study.
In order to evaluate the impacts upon soil fauna, different standard assays were carried out with Eisenia fetida earthworms. The chosen approach was to assess the different uptake pathways in order to elucidate the origin of potential toxicities. Pollutant uptake in earthworms occurs through two different pathways: via the dermis incorporating them from the aqueous matrix and via the digestive tract, by soil ingestion (Rodriguez-Campos et al., 2014). To assess the potential toxicity of the soil water fraction, an OECD filter paper test (OECD-207, 1984) was carried out. Nevertheless, the lack of significant differences between the groups and the observed low weight losses suggested the absence of toxicity via the dermis, just as Urionabarrenetxea et al., observed in 2021. This is something expected, taking into consideration the low metal values observed in the leachates and the LC50 values observed in the bibliographical review. For instance, Boyd et al. (2001) reported in Caenorhadbitis elegans, LC50 in Tifton soils of 18 mg Cd·kg−1, 34 mg Ni·kg−1, and 43 mg Pb·kg−1, while Aparicio et al. (2019) reported a value of 30 mg·kg−1 for Cr.
The assessment of the exposure through dermal contact and the digestive tract was carried out following OECD artificial soil testing guidelines (1984), where neither significant mortality nor weight loss was recorded. Indeed, in some groups, the earthworms gained biomass, while the control group showed the highest weight loss. This is directly related with the high OM contents of the “Landfill 17” soils, which was the destination of sewage sludge discharges for decades (Urionabarrenetxea et al., 2022). In this sense, different authors have reported a positive correlation between soil ingestion and OM content in E. fetida, improving their nutritional status and reducing potential biomass loss when facing pollutant exposure (Irizar et al., 2014a, 2015b; Lacalle et al., 2020; Urionabarrenetxea et al., 2021). However, and as previously discussed, soil organic matter affects metal bioavailability and toxicity by forming different organometallic ligands, and consequently, metal accumulation in earthworm tissues increases due to the incorporation of polluted soil colloids (Irizar et al., 2015b). The metal accumulation tendency found in this study presents a positive correlation with the biomass recorded. Thereby, MN8 is the group showing the lowest biomass loss (in fact, weight was gained) but the highest metal accumulation rates.
The assessment of earthworm coelomocytes has an important role in the study of immunological responses, providing valuable information about the early response to pollutants (Plytycz et al., 2010; Kwak et al., 2014; García Velasco et al., 2019). In this study, coelomocyte viability and cytotoxicity were assessed by using the calcein-AM assay. After 14 days of exposure to soils from “Landfill 17,” MN8 soils (non-treated) showed a significant decrease in calcein signal, suggesting strong cell damage and cytotoxicity. Similarly, Irizar et al. (2015a) and Plytycz and Morgan (2011a) have reported changes in coelomocyte viability (cytotoxicity) when exposed to Cr. At the same time, MN1 and MN2 exhibited a slight decrease in calcein retention, suggesting that Cd presence in these sites could induce affectation upon immune system and cell integrity (Irizar et al., 2015a; Aparicio et al., 2019). However, the lower retention present in the control (OECD soil) may be related to different soil conditions when compared with “Landfill 17” soils. Furthermore, slight alterations in retention ability could also be influenced by soil pH conditions and coelomocyte subpopulation variations: amoebocytes can phagocyte death eleocytes (Irizar, 2015a), which are more sensitive to metal pollution (Plytycz et al., 2009; Plytycz et al., 2010; Plytycz et al., 2011b; Irizar et al., 2015a).
Alterations in the activity of the enzymes could occur due to the different contaminants present in “Landfill 17” soils. The significant increase in GST activity observed in earthworms exposed to MN1 soils indicates an activation of the detoxification mechanism, allowing the organism to overcome, totally or partially, the stress caused by exposure to xenobiotics (Liu et al., 2015). Similar results were observed in Eisenia fetida when exposed to Cd (Liang et al., 2022). Moreover, the decrease in CAT activity in MN2 and MN4 may suggest a greater accumulation of H2O2 and potential formation of ROS (Lisbôa et al., 2021). However, under the conditions studied, there was no occurrence of oxidative stress due to the absence of alterations on protein carbonyl and MDA levels in all groups. This may be related to the increase in and/or unchanged activity of the GST and CbE enzymes, which can exert a protective effect and prevent the occurrence of oxidative damage, also an alleviation of lipid peroxidation (Wheelock et al., 2008; Hellou et al., 2012).
To evaluate neurotoxicity, the activity of the AChE enzyme was determined. This enzyme acts in the transmission of nerve impulses and has the function of hydrolyzing the neurotransmitter acetylcholine (Tiwari et al., 2016). The decrease in AChE in earthworms may result in less locomotion capacity, which also compromises feeding and reproduction, leading to death (Gambi et al., 2007; Wang et al., 2015). Thus, the decrease in AChE activity observed in MN4 may have occurred due to exposure to a mixture of metals such as dieldrin and B(a)P present at this location. The activity of this enzyme may decrease depending on the type of contaminant and the species of earthworm. In fact, a decrease in AChE was observed in Eisenia andrei when exposed to Pb, in E. fetida when exposed to soils contaminated with Cr, Ni, and Pb (Zheng et al., 2013), in Eisenia fetida when exposed to Ni, Zn, Cd, Cu and Hg (Frasco et al., 2005; Zheng et al., 2013), in E. fetida when exposed to a mixture of Cd and B(a)P (Zhang et al., 2019), and in E. fetida when exposed to insecticide imidacloprid (Wang et al., 2015).
For the IBR/n index, the recommendations of Asensio et al. (2013) about using multiple organisms to achieve an accurate approximation of the whole ecosystem status were followed. Thus, the integrative biomarker response index was developed with five of the most representative measured biological parameters and biomarkers from each organism to integrate responses at different levels of biological complexity (molecular > enzyme activity > cellular > community) and represent, from a holistic point of view, the ecosystem status based on soil–microorganism–plant–earthworm interactions. Hence, according to the IBR/n index developed by Aparicio et al. (2021) for microbiota, basal respiration (BR) was considered to represent microbial communities’ activity. The most representative parameter considered to represent the vegetation was plant biomass, which exhibited significant differences between treatments. For earthworms, different representative biomarkers (biochemical and cellular) exhibiting significant differences were considered: at the cellular level—CAL and at biochemical level—AChE and GST.
The signals at the lower biological levels (GST and CAL) were more responsive against stress related with pollution, just as basal respiration gives a global response more based on stress provoked by environmental conditions. High affectation of the ecosystem status was readily evidenced in non-treated MN8, while in MN4, a remarkable stress over microbial communities and high neurotoxic effects upon fauna could be observed. Meanwhile, the best ecosystem health was recorded in MN7, the furthest point from the WWTP.
After the application of the complementary remediation strategy for 1.5 years in a soil with mixed contamination, the concentrations of the critical compounds [Cd, Cr, Ni, Pb, benzo(a)pyrene, and dieldrin] were reduced in most of the “Landfill 17” sampled points, where a high sequestration of the pollutants was present due to several factors such as OM content, aged contamination, the presence of plants, or even the enhancement of rhizosphere interactions.
Microbiological parameters did not indicate differences between non-treated (MN8) and treated sites due to the adaptation of native microbial communities to the soil conditions of the sites. However, the treated sites presented a notable colonization of native vegetation and increased plant biomass. In our case, the spontaneous native vegetation helped in the recovery of the soil and ecosystem health. However, it should be taken into account that due to their limitations soils can make difficult the growth of other cultivars in sites. The bioassays did not present phytotoxicity upon C. sativus or toxicity upon E. fetida earthworms. Nevertheless, in the sites subjected to the complementary remediation strategy, earthworms could alleviate toxicity through detoxifying and immune systems. Additionally, the IBR indexes helped integrate multiple organisms’ information in a helpful and comprehensive way for scientists, regulators, and other stakeholders. Therefore, the combination of bioremediation technologies (micro-, phyto-, and vermiremediation) followed by a complementary strategy has been proven to be an accurate approach to improve soil functionality, quality, and health under natural conditions.
The original contributions presented in the study are included in the article/Supplementary Material; further inquiries can be directed to the corresponding author.
The manuscript presents research on animals that do not require ethical approval for their study. As the animals are not included in Annex I of the Royal Decree 53/2013, assessment and follow-up by the Ethics and Teaching Commission (CEID) was not required.
AP-V: Data curation, formal analysis, investigation, methodology, manuscript writing—original draft, review, and editing. EU: Data curation, formal analysis, investigation, methodology, project administration, supervision, validation, manuscript writing—original draft, review, and editing. UA: Data curation, investigation, methodology, project administration, supervision, manuscript writing—original draft, review, and editing. CR: Data curation, investigation, manuscript writing—original draft, review, and editing. MG-S: Investigation, methodology, supervision, manuscript writing—original draft, review, and editing. NG-V: Investigation, methodology, manuscript writing—original draft, review, and editing. BZ: Investigation, methodology, supervision, manuscript writing—original draft, review, and editing. MA: Investigation, methodology, supervision, manuscript writing—original draft, review, and editing. LE: Methodology, project administration, resources, supervision, manuscript writing—original draft, review, and editing. CG: Funding acquisition, methodology, project administration, resources, supervision, manuscript writing—original draft, review, and editing. JB: Funding acquisition, methodology, project administration, resources, supervision, manuscript writing—original draft, review, and editing. MS: Funding acquisition, methodology, project administration, resources, supervision, manuscript writing—original draft, review, and editing.
The authors declare financial support was received for the research, authorship, and/or publication of this article. This study was supported by the Phy2SUDOE (SOE4/P5/E1021) project funded by the Interreg Sudoe Program through the European Regional Development Fund (ERDF), PRADA project (PID2019110055RB-C21 and PID 2019-110055RB-C22) from MINECO, and the Consolidated Research Grant of the Basque Government (GV IT-1648-22).
The authors declare that the research was conducted in the absence of any commercial or financial relationships that could be construed as a potential conflict of interest.
All claims expressed in this article are solely those of the authors and do not necessarily represent those of their affiliated organizations, or those of the publisher, editors, and reviewers. Any product that may be evaluated in this article, or claim that may be made by its manufacturer, is not guaranteed or endorsed by the publisher.
The Supplementary Material for this article can be found online at: https://www.frontiersin.org/articles/10.3389/fenvs.2024.1370820/full#supplementary-material
WWTP, wastewater treatment plant; CTA, catalase; AChE, acetylcholinesterase; GST, glutathione S-transferase; GSH, glutathione; CbE, carboxylesterase; MDA, malondialdehyde; BR, basal respiration; IBR/n, integrated biological response index; PI, pollution index; calcein-AM, calcein–acetoxymethyl.
Ali, N. A., Ater, M., Sunahara, G. I., and Robidoux, P. Y. (2004). Phytotoxicity and bioaccumulation of copper and chromium using barley (Hordeum vulgare L.) in spiked artificial and natural forest soils. Ecotoxicol. Environ. Saf. 57 (3), 363–374. doi:10.1016/S0147-6513(03)00074-5
Almeida, E. A., Bainy, A. C. D., Dafre, A. L., Gomes, O. F., Medeiros, M. H. G., and Di Mascio, P. (2005). Oxidative stress in digestive gland and gill of the brown mussel (Perna perna) exposed to air and re-submersed. J. Exp. Mar. Biol. Ecol. 318, 21–30. doi:10.1016/j.jembe.2004.12.007
An, Y.-J., Kim, Y.-M., Kwon, T.-I., and Jeong, S.-W. (2004). Combined effect of copper, cadmium, and lead upon Cucumis sativus growth and bioaccumulation. Sci. Total Environ. 326, 85–93. doi:10.1016/j.scitotenv.2004.01.002
Antoci, A., Galeotti, M., and Sordi, S. (2018). Environmental pollution as engine of industrialization. Commun. Nonlinear Sci. Numer. Simul. 58, 262–273. doi:10.1016/j.cnsns.2017.06.016
Aparicio, J. D., Garcia-Velasco, N., Urionabarrenetxea, E., Soto, M., Álvarez, A., and Polti, M. A. (2019). Evaluation of the effectiveness of a bioremediation process in experimental soils polluted with chromium and lindane. Ecotoxicol. Environ. Saf. 181, 255–263. doi:10.1016/j.ecoenv.2019.06.019
Aparicio, J. D., Lacalle, R. G., Artetxe, U., Urionabarrenetxea, E., Becerril, J. M., Polti, M. A., et al. (2021). Successful remediation of soils with mixed contamination of chromium and lindane: integration of biological and physico-chemical strategies. Environ. Res. 194, 110666. doi:10.1016/j.envres.2020.110666
Asensio, V., Rodríguez-Ruiz, A., Garmendia, L., Andre, J., Kille, P., Morgan, A. J., et al. (2013). Towards an integrative soil health assessment strategy: a three tier (integrative biomarker response) approach with Eisenia fetida applied to soils subjected to chronic metal pollution. Sci. Total Environ. 442, 344–365. doi:10.1016/j.scitotenv.2012.09.048
Basque Country (2023). Inventory of soils with potentially contaminating activities or facilities. Available at: https://www.geo.euskadi.eus/geobisorea.
Basque Government (2012). Plan de Suelos Contaminados del País Vasco (2007-2012). Available at: http://www.ingurumena.ejgv.euskadi.eus/r49orokorra/es/contenidos/plan/suelos_contaminados/es_plan/adjuntos/plan_suelos_contaminados.pdf.
Beliaeff, B., and Burgeot, T. (2002). Integrated biomarker response: a useful tool for ecological risk assessment. Environ. Toxicol. Chem. 21 (6), 1316–1322. doi:10.1002/etc.5620210629
Berthelot, Y., Trottier, B., and Robidoux, P. (2009). Assessment of soil quality using bioaccessibility-based models and a biomarker index. Environ. Int. 35 (1), 83–90. doi:10.1016/j.envint.2008.07.008
Biurrun, I., García-Mijangos, I., Crespo, M. B., and Fernández-González, F. (2008). Los herbazales higronitrófilos de Epilobium hirsutum y Mentha longifolia en los cursos fluviales de la Península Ibérica. Lazaroa 29, 69–86.
BOE núm. 25. Sect. I. Disposiciones generales. 3507-3521. Boletin Oficial del Estado, Ministerio de Medio Ambiente (2002). Available at: https://www.boe.es/eli/es/rd/2001/12/27/1481
Boyd, W. A., Stringer, V. A., and Williams, P. L. (2001). Metal LC50s of a soil nematode compared to published earthworm data. Environ. Toxicol. Risk Assess. Sci. Policy, Stand. Implic. Environ. Decis. 10, 223–235. doi:10.1520/STP10257S
Bradford, M. M. (1976). A rapid and sensitive method for the quantitation of microgram quantities of protein utilizing the principle of protein-dye binding. Elsevier BV 72, 248–254. doi:10.1006/abio.1976.9999
Broeg, K., and Lehtonen, K. K. (2006). Indices for the assessment of environmental pollution of the Baltic Sea coasts: integrated assessment of a multi-biomarker approach. Mar. Pollut. Bull. 53 (8–9), 508–522. doi:10.1016/j.marpolbul.2006.02.004
Cajaraville, M. P., Marigómez, I., and Angulo, E. (1993). Correlation between cellular and organismic responses to oil-induced environmental stress in mussels. Sci. Tot. Environ. 134, 1353–1371. doi:10.1016/S0048-9697(05)80142-1
Efroymson, R. A., Will, M. E., Ii, G. W. S., and Wooten, A. C., 1997. Toxicological benchmarks for screening contaminants of potential concern for effects on terrestrial plants. doi:10.2172/10107985
Ellman, G. L., Courtney, K. D., Andres, V., and Featherstone, R. M. (1961). A new and rapid colorimetric determination of acetylcholinesterase activity. Biochem. Pharmacol. 7 (2), 88–95. doi:10.1016/0006-2952(61)90145-9
Epelde, L., Becerril, J. M., Hernández-Allica, J., Barrutia, O., and Garbisu, C. (2008). Functional diversity as indicator of the recovery of soil health derived from Thlaspi caerulescens growth and metal phytoextraction. Appl. Soil Ecol. 39 (3), 299–310. doi:10.1016/j.apsoil.2008.01.005
Epelde, L., Mijangos, I., Becerril, J. M., and Garbisu, C. (2009). Soil microbial community as bioindicator of the recovery of soil functioning derived from metal phytoextraction with sorghum. Soil Biol. Biochem. 41 (9), 1788–1794. doi:10.1016/j.soilbio.2008.04.001
Franco, H. A., Silva, M. E. R. V. D., Silveira, M. F. D., Marques, M. R. D. C., and Thode Filho, S. (2017). Impact of landfill leachate on the germination of Cucumber (Cucumis sativus). Rev. Eletrônica Em Gestão, Educ. Tecnol. Ambient. 21, 32. doi:10.5902/2236117029719
Frasco, M. F., Fournier, D., Carvalho, F., and Guilhermino, L. (2005). Do metals inhibit acetylcholinesterase (AChE)? Implementation of assay conditions for the use of AChE activity as a biomarker of metal toxicity. Biomarkers 10 (5), 360–375. doi:10.1080/13547500500264660
Gambi, N., Pasteris, A., and Fabbri, E. (2007). Acetylcholinesterase activity in the earthworm Eisenia andrei at different conditions of carbaryl exposure. Comp. Biochem. Physiology Part C Toxicol. Pharmacol. 145 (4), 678–685. doi:10.1016/j.cbpc.2007.03.002
Garbisu, C., Becerril, J. M., Epelde, L., and Alkorta, I. (2007). Bioindicadores de la calidad del suelo: herramienta metodológica para la evaluación de la eficacia de un proceso fitorremediador.
Garcia-Velasco, N., Irizar, A., Urionabarrenetxea, E., Scott-Fordsmand, J. J., and Soto, M. (2019). Selection of an optimal culture medium and the most responsive viability assay to assess AgNPs toxicity with primary cultures of Eisenia fetida coelomocytes. Ecotoxicol. Environ. Saf. 183, 109545. doi:10.1016/j.ecoenv.2019.109545
Gómez-Sagasti, M. T., Alkorta, I., Becerril, J. M., Epelde, L., Anza, M., and Garbisu, C. (2012). Microbial monitoring of the recovery of soil quality during heavy metal phytoremediation. Water, Air, & Soil Pollut. 223 (6), 3249–3262. doi:10.1007/s11270-012-1106-8
Gómez-Sagasti, M. T., Garbisu, C., Urra, J., Míguez, F., Artetxe, U., Hernández, A., et al. (2021). Mycorrhizal-assisted phytoremediation and intercropping strategies improved the health of contaminated soil in a peri-urban area. Front. Plant Sci. 12, 693044. doi:10.3389/fpls.2021.693044
Gondek, K., Baran, A., and Kopec, M. (2014). The effect of low-temperature transformation of mixtures of sewage sludge and plant materials on content, leachability and toxicity of heavy metals. Chemos 117, 33–39. doi:10.1016/j.chemosphere.2014.05.032
Gondek, K., and Kopec, M. (2006). Heavy metal binding by organic substance in sewage sludge of various origin. EJPAU Environ. Dev. 9 (3). Available at: http://www.ejpau.media.pl/volume9/issue3/art-01.html.
Hakanson, L. (1980). An ecological risk index for aquatic pollution control. A sedimentological approach. Water Res. 14 (8), 975–1001. doi:10.1016/0043-1354(80)90143-8
Hellou, J., Ross, N. W., and Moon, T. W. (2012). Glutathione, glutathione S-transferase, and glutathione conjugates, complementary markers of oxidative stress in aquatic biota. Environ. Sci. Pollut. Res. 19 (6), 2007–2023. doi:10.1007/s11356-012-0909-x
Htwe, T., Chotikarn, P., Duangpan, S., Onthong, J., Buapet, J., and Sinutok, S. (2022). Integrated biomarker responses of rice associated with grain yield in copper-contaminated soil. Environ. Sci. Pollut. Res. 29, 8947–8956. doi:10.1007/s11356-021-16314-y
Irizar, A., Duarte, D., Guilhermino, L., Marigómez, I., and Soto, M. (2014a). Optimization of NRU assay in primary cultures of Eisenia fetida for metal toxicity assessment. Ecotoxicology 23 (7), 1326–1335. doi:10.1007/s10646-014-1275-x
Irizar, A., Izagirre, U., Diaz De Cerio, O., Marigómez, I., and Soto, M. (2014b). Zonation in the digestive tract of Eisenia fetida: implications in biomarker measurements for toxicity assessment. Comp. Biochem. Physiology Part C Toxicol. Pharmacol. 160, 42–53. doi:10.1016/j.cbpc.2013.11.006
Irizar, A., Rivas, C., García-Velasco, N., De Cerio, F. G., Etxebarria, J., Marigómez, I., et al. (2015a). Establishment of toxicity thresholds in subpopulations of coelomocytes (amoebocytes vs. eleocytes) of Eisenia fetida exposed in vitro to a variety of metals: implications for biomarker measurements. Ecotoxicology 24 (5), 1004–1013. doi:10.1007/s10646-015-1441-9
Irizar, A., Rodríguez, M. P., Izquierdo, A., Cancio, I., Marigómez, I., and Soto, M. (2015b). Effects of soil organic matter content on cadmium toxicity in Eisenia fetida: implications for the use of biomarkers and standard toxicity tests. Archives Environ. Contam. Toxicol. 68 (1), 181–192. doi:10.1007/s00244-014-0060-4
ISO 16072 (2002). Soil quality - laboratory methods for determination of microbial soil respiration. Geneva: International Organization for Standardization ISO.
Jan, A. T., Ali, A., and Rizwanul Haq, Q. M. (2015). “Phytoremediation,” in Soil remediation and plants (Germany: Elsevier), 63–84. doi:10.1016/B978-0-12-799937-1.00003-6
Keen, J. H., Habig, W. H., and Jakoby, W. B. (1976). Mechanism for the several activities of the glutathione S-transferases. J. Biol. Chem. 251 (20), 6183–6188. doi:10.1016/S0021-9258(20)81842-0
Kwak, J. I., Kim, S. W., and An, Y.-J. (2014). A new and sensitive method for measuring in vivo and in vitro cytotoxicity in earthworm coelomocytes by flow cytometry. Environ. Res. 134, 118–126. doi:10.1016/j.envres.2014.07.014
Lacalle, R. G., Aparicio, J. D., Artetxe, U., Urionabarrenetxea, E., Polti, M. A., Soto, M., et al. (2020). Gentle remediation options for soil with mixed chromium (VI) and lindane pollution: biostimulation, bioaugmentation, phytoremediation and vermiremediation. Heliyon 6 (8), e04550. doi:10.1016/j.heliyon.2020.e04550
Lacalle, R. G., Gómez-Sagasti, M. T., Artetxe, U., Garbisu, C., and Becerril, J. M. (2018). Brassica napus has a key role in the recovery of the health of soils contaminated with metals and diesel by rhizoremediation. Sci. Total Environ. 618, 347–356. doi:10.1016/j.scitotenv.2017.10.334
Landberg, T., and Greger, M. (1996). Differences in uptake and tolerance to heavy metals in Salix from unpolluted and polluted areas. Appl. Geochem. 11, 175–180. doi:10.1016/0883-2927(95)00082-8
Liang, X., Zhou, D., Wang, J., Li, Y., Liu, Y., and Ning, Y. (2022). Evaluation of the toxicity effects of microplastics and cadmium on earthworms. Sci. Total Environ. 836, 155747. doi:10.1016/j.scitotenv.2022.155747
Lisbôa, R. D. M., Storck, T. R., Silveira, A. D. O., Wolff, D., Tiecher, T. L., Brunetto, G., et al. (2021). Ecotoxicological responses of Eisenia andrei exposed in field-contaminated soils by sanitary sewage. Ecotoxicol. Environ. Saf. 214, 112049. doi:10.1016/j.ecoenv.2021.112049
Liu, K., Chen, L., Zhang, W., Lin, K., and Zhao, L. (2015). EPR detection of hydroxyl radical generation and oxidative perturbations in lead-exposed earthworms (Eisenia fetida) in the presence of decabromodiphenyl ether. Ecotoxicology 24 (2), 301–308. doi:10.1007/s10646-014-1378-4
Marigómez, I., Garmendia, L., Soto, M., Orbea, A., Izagirre, U., and Cajaraville, M. P. (2013). Marine ecosystem health status assessment through integrative biomarker indices: a comparative study after the Prestige oil spill “Mussel Watch.”. Ecotoxicology 22 (3), 486–505. doi:10.1007/s10646-013-1042-4
Meshalkin, V. P., Kozlovskiy, R. A., Kozlovskiy, M. R., Ibatov, Y. A., Voronov, M. S., Kozlovskiy, I. A., et al. (2023). Experimental and mathematical analysis of the kinetics of the low-waste process of butyl lactate synthesis. Energies 16 (4), 1746. doi:10.3390/en16041746
Míguez, F., Gómez-Sagasti, M. T., Hernández, A., Artetxe, U., Blanco, F., Castañeda, J. H., et al. (2020). In situ phytomanagement with Brassica napus and bio-stabilised municipal solid wastes is a suitable strategy for redevelopment of vacant urban land. Urban For. Urban Green. 47, 126550. doi:10.1016/j.ufug.2019.126550
Moreno-Jiménez, E., Peñalosa, J. M., Manzano, R., Carpena-Ruiz, R. O., Gamarra, R., and Esteban, E. (2009). Heavy metals distribution in soils surrounding an abandoned mine in NW Madrid (Spain) and their transference to wild flora. J. Hazard. Mater. 162 (2–3), 854–859. doi:10.1016/j.jhazmat.2008.05.109
Morillo, E., and Villaverde, J. (2017). Advanced technologies for the remediation of pesticide-contaminated soils. Sci. Total Environ. 586, 576–597. doi:10.1016/j.scitotenv.2017.02.020
Niu, H., Wu, H., Chen, K., Sun, J., Cao, M., and Luo, J. (2021). Effects of decapitated and root-pruned Sedum alfredii on the characterization of dissolved organic matter and enzymatic activity in rhizosphere soil during Cd phytoremediation. J. Hazard. Mater. 417, 125977. doi:10.1016/j.jhazmat.2021.125977
OECD (1984). Terrestrial plant: growth test OECD. Guideline for chemicals, No., 208. Organization for economic cooperation and development, Paris on the crossroads of remediation. Soil Rem. Plant, 63–84.
Pankhurst, C. E., Doube, B. M., and Gupta, V. V. S. R. (1997). in Biological indicators of soil health: synthesis. En biological indicators of soil health. Editors C. E. Pankhurst, B. M. Doube, and V. V. S. R. Gupta (New York, USA: CAB International), 419–435.
Park, J. H., Lamb, D., Paneerselvam, P., Choppala, G., Bolan, N., and Chung, J. W. (2011). Role of organic amendments on enhanced bioremediation of heavy metal(loid) contaminated soils. J. Hazard. Mater. 185, 549–574. doi:10.1016/j.jhazmat.2010.09.082
Parvez, S., and Raisuddin, S. (2005). Protein carbonyls: novel biomarkers of exposure to oxidative stress-inducing pesticides in freshwater fish Channa punctata (Bloch). Environ. Toxicol. Pharmacol. 20 (1), 112–117. doi:10.1016/j.etap.2004.11.002
Pirinccioglu, A. G., Gökalp, D., Pirinccioglu, M., Kizil, G., and Kizil, M. (2010). Malondialdehyde (MDA) and protein carbonyl (PCO) levels as biomarkers of oxidative stress in subjects with familial hypercholesterolemia. Clin. Biochem. 43 (15), 1220–1224. doi:10.1016/j.clinbiochem.2010.07.022
Plytycz, B., Kielbasa, E., Grebosz, A., Duchnowski, M., and Morgan, A. J. (2010). Riboflavin mobilization from eleocyte stores in the earthworm Dendrodrilus rubidus inhabiting aerially contaminated Ni smelter soil. Chemosphere 81 (2), 199–205. doi:10.1016/j.chemosphere.2010.06.056
Plytycz, B., Klimek, M., Homa, J., Mazur, A. I., Kruk, J., and Morgan, A. J. (2011b). Species-specific sensitivity of earthworm coelomocytes to dermal metal (Cd, Cu, Ni, Pb, Zn) exposures: methodological approach. Pedobiologia 54, S203–S210. doi:10.1016/j.pedobi.2011.06.002
Plytycz, B., Lis-Molenda, U., Cygal, M., Kielbasa, E., Grebosz, A., Duchnowski, M., et al. (2009). Riboflavin content of coelomocytes in earthworm (Dendrodrilus rubidus) field populations as a molecular biomarker of soil metal pollution. Environ. Pollut. 157 (11), 3042–3050. doi:10.1016/j.envpol.2009.05.046
Plytycz, B., and Morgan, A. J. (2011a). Riboflavin storage in earthworm chloragocytes/eleocytes in an eco-immunology perspective. Int. Sci. J. 8, 199–209.
Reilley, K. A., Schwab, A. P., and Banks, M. K. (1996). Dissipation of polycyclic aromatic hydrocarbons in the rhizosphere. Dissipation Polycycl. Aromatic Hydrocarbons Rhizosphere 25, 212–219. doi:10.2134/jeq1996.00472425002500020002x
Rodriguez-Campos, J., Dendooven, L., Alvarez-Bernal, D., and Contreras-Ramos, S. M. (2014). Potential of earthworms to accelerate removal of organic contaminants from soil: a review. Appl. Soil Ecol. 79, 10–25. doi:10.1016/j.apsoil.2014.02.010
Schindelin, J., Arganda-Carreras, I., Frise, E., Kaynig, V., Longair, M., Pietzsch, T., et al. (2012). Fiji: an open-source platform for biological-image analysis. Nat. Methods 9 (7), 676–682. doi:10.1038/nmeth.2019
Stefanowicz, A. M., Kapusta, P., Szarek-Łukaszewska, G., Grodzińska, K., Niklińska, M., and Vogt, R. D. (2012). Soil fertility and plant diversity enhance microbial performance in metal-polluted soils. Sci. Total Environ. 439, 211–219. doi:10.1016/j.scitotenv.2012.09.030
Tiwari, R. K., Singh, S., Pandey, R. S., and Sharma, B. (2016). Enzymes of earthworm as indicators of pesticide pollution in soil. Adv. Enzyme Res. 04, 113–124. doi:10.4236/aer.2016.44011
Unterbrunner, R., Puschenreiter, M., Sommer, P., Wieshammer, G., Tlustoˇs, P., Zupan, M., et al. (2007). Heavy metal accumulation in trees growing on contaminated sites in Central Europe. Environ. Pollut. 148, 107–114. doi:10.1016/j.envpol.2006.10.035
Urionabarrenetxea, E., Garcia-Velasco, N., Anza, M., Artetxe, U., Lacalle, R., Garbisu, C., et al. (2021). Application of in situ bioremediation strategies in soils amended with sewage sludges. Sci. Total Environ. 766, 144099. doi:10.1016/j.scitotenv.2020.144099
Urionabarrenetxea, E., Garcia-Velasco, N., Zaldibar, B., and Soto, M. (2022). Impacts of sewage sludges deposition on agricultural soils: effects upon model soil organisms. Comp. Biochem. Physiology Part C Toxicol. Pharmacol. 255, 109276. doi:10.1016/j.cbpc.2022.109276
US- EPA (1982). Seed germination/root elongation toxicity tests EC12. Office of Toxic Substances, Washington, DC: US Environmental Protection Agency.
US-EPA Method 3051A (2007). Microwave assisted acid digestion of sediments, sludges, soils and oils. third ed. Washington, DC: US Environmental Protection Agency.
Vasylkiv, O.Yu., Kubrak, O. I., Storey, K. B., and Lushchak, V. I. (2010). Cytotoxicity of chromium ions may be connected with induction of oxidative stress. Chemosphere 80 (9), 1044–1049. doi:10.1016/j.chemosphere.2010.05.023
Visioli, G., Conti, F. D., Gardi, C., and Menta, C. (2014). Germination and root elongation bioassays in six different plant species for testing Ni contamination in soil. Bull. Environ. Contam. Toxicol. 92 (4), 490–496. doi:10.1007/s00128-013-1166-5
Wang, K., Huang, Y., Li, X., and Chen, M. (2018). Functional analysis of a carboxylesterase gene associated with isoprocarb and cyhalothrin resistance in Rhopalosiphum padi(L.). Front. Physiology 9, 992. doi:10.3389/fphys.2018.00992
Wang, K., Qi, S., Mu, X., Chai, T., Yang, Y., Wang, D., et al. (2015). Evaluation of the toxicity, AChE activity and DNA damage caused by imidacloprid on earthworms, Eisenia fetida. Bull. Environ. Contam. Toxicol. 95 (4), 475–480. doi:10.1007/s00128-015-1629-y
Wheelock, C. E., Phillips, B. M., Anderson, B. S., Miller, J. L., Miller, M. J., and Hammock, B. D. (2008). Applications of carboxylesterase activity in environmental monitoring and toxicity identification evaluations (TIEs). Rev. Environ. Contam. Toxicol. 195, 117–178. doi:10.1007/978-0-387-77030-7_5
Zhang, L., Zhou, L., Han, L., Zhao, C., Norton, J. M., Li, H., et al. (2019). Benzo(a)pyrene inhibits the accumulation and toxicity of cadmium in subcellular fractions of Eisenia fetida. Chemosphere 219, 740–747. doi:10.1016/j.chemosphere.2018.12.083
Keywords: biomarkers, integrative biomarker response, landfill, mixed contamination, phytoremediation
Citation: Pérez-Vázquez A, Urionabarrenetxea E, Artetxe U, Rutkoski CF, Gomez-Sagasti MT, Garcia-Velasco N, Zaldibar B, Anza M, Epelde L, Garbisu C, Becerril JM and Soto M (2024) Integrative assessment of in situ combined bioremediation strategies applied to remediate soils spilled with sewage sludges. Front. Environ. Sci. 12:1370820. doi: 10.3389/fenvs.2024.1370820
Received: 15 January 2024; Accepted: 18 March 2024;
Published: 08 May 2024.
Edited by:
Qi Liao, Central South University, ChinaReviewed by:
Agnieszka Klimkowicz-Pawlas, Institute of Soil Science and Plant Cultivation, PolandCopyright © 2024 Pérez-Vázquez, Urionabarrenetxea, Artetxe, Rutkoski, Gomez-Sagasti, Garcia-Velasco, Zaldibar, Anza, Epelde, Garbisu, Becerril and Soto. This is an open-access article distributed under the terms of the Creative Commons Attribution License (CC BY). The use, distribution or reproduction in other forums is permitted, provided the original author(s) and the copyright owner(s) are credited and that the original publication in this journal is cited, in accordance with accepted academic practice. No use, distribution or reproduction is permitted which does not comply with these terms.
*Correspondence: M. Soto, bWFudS5zb3RvQGVodS5ldXM=
Disclaimer: All claims expressed in this article are solely those of the authors and do not necessarily represent those of their affiliated organizations, or those of the publisher, the editors and the reviewers. Any product that may be evaluated in this article or claim that may be made by its manufacturer is not guaranteed or endorsed by the publisher.
Research integrity at Frontiers
Learn more about the work of our research integrity team to safeguard the quality of each article we publish.