- 1Swedish University of Agricultural Sciences, Department of Energy and Technology, Uppsala, Sweden
- 2Chemical Engineering Department, University of Cape Town, Cape Town, South Africa
- 3Future Water Institute, University of Cape Town, Cape Town, South Africa
- 4Civil Engineering Department, University of Cape Town, Cape Town, South Africa
Recycling resources excreted in human urine can help achieve a sustainable future and circular economy in the sanitation space. However, many studies researching different technologies for safely recycling urine do not use real human urine for experimentation, relying instead on recipes for making synthetic or artificial solutions that attempt to mimic the composition of real human urine. This methodological choice is the focus of this article, which points out that the real urine matrix is extremely complex, with a metabolome (>2,500 metabolites) that differs greatly from that of synthetic urine (<15 metabolites). Therefore, experimental results obtained using synthetic urine can also differ from those obtained using real urine. To exemplify this, we review published literature in terms of four aspects: i) solubility of chemicals and buffering capacity of urine, ii) dissolved organics and membrane fouling, iii) thermodynamic modelling of chemical speciation in urine, and iv) removal of pollutants from urine. We recognise that there is a place for synthetic urine in sanitation research and provide examples of studies where its use is appropriate. Lastly, based on literature from the medical sciences, we provide preliminary guidelines on protocols for preparing synthetic urine that could improve experimentation involving human urine and accelerate the water sector’s transition to circularity.
1 Introduction
In decentralised sanitation systems, human urine can be collected separately from other domestic wastewater and treated to make several useful products, such as water, fertiliser, biostimulants, chemicals and electricity (Larsen et al., 2013). Such recycling has the potential to reduce human transgression of several planetary boundaries, including those on biogeochemical flows of nitrogen and phosphorus (Perez-Mercado et al., 2022; Rockstrom et al., 2023). Separate treatment of urine can also benefit existing centralised wastewater treatment plants, e.g., by decreasing the nitrogen load and thus reducing the energy demand for nitrification (Wilsenach and Loosdrecht, 2006).
Recycling of human urine is a growing research topic within the water sector (Aliahmad et al., 2022). Several novel technologies for treating urine are currently being developed [for recent reviews on this topic, see Larsen et al. (Larsen et al., 2021)] and implemented in transdisciplinary initiatives like the Horizon Europe project “P2Green” (https://p2green.eu/) and the Australian Research Council research hub “NiCE” (https://www.nicehub.org/). A growing number of experts believe that decentralised treatment of wastewater could help the water sector accelerate its transition to circularity and that it is well poised to achieve such a paradigm shift (Guest et al., 2009; Larsen et al., 2013).
These developments are promising for our own research groups, which are also working in this area. However, a particular trend in current research on decentralised sanitation that gives us cause for concern, and which we address in this article, is the use of synthetic human urine. An analysis of urine research literature, specifically focusing on nutrient recovery, published over the past 5 years, shows an increasing trend in the use of synthetic urine (Supplementary Material, Supplementary Figure S1), with approximately 40% of these studies conducted exclusively with synthetic urine. Of particular concern is that among several publications that exclusively used synthetic urine, 21% referred to the type of urine used as “human urine” in the title of their publications (Supplementary Material, Supplementary Figure S1). For multiple reasons, many studies do not use real human urine in experimental work, and instead rely on recipes for making synthetic solutions that attempt to mimic the composition of real human urine. A simple search of the Scopus database shows that there are hundreds of published articles that have either used synthetic urine for experimentation or have developed assumptions, hypotheses and conclusions based on published studies using synthetic urine. Using synthetic solutions to simulate real fluids is a legitimate scientific method, and one that is not uncommon in wastewater research. In the decentralised sanitation sector, many recipes that can successfully mimic specific physical and chemical properties of human faeces, faecal sludge and greywater have been developed (Penn et al., 2018). In early experimental work, such as proof-of-concept research studies, use of simulants like synthetic urine can help advance science by shedding light on mechanistic aspects of a treatment technology. However, in some instances, using only synthetic urine for experimentation could be problematic from a methodological perspective, especially if protocols for preparing synthetic urine are not well-established and validated by comparison with real urine. To argue why this could be the case, in this article we highlight some of the differences in composition and properties of synthetic urine and real urine. We draw on selected published literature to show how differences between types of urine affect experimental results and their real-life implications. We recognise that there is a place for synthetic/artificial urine in sanitation research and provide examples of cases where its use is appropriate. Our intention is not to criticize studies or researchers that have used synthetic urine in the past. Both of our research groups have also conducted experimental work involving synthetic urine (Table 1). In fact, the observations we made during those studies regarding the differences between real urine and synthetic urine motivated us, in part, to write this perspective article. Overall, we think there is a risk that findings of experiments conducted solely with synthetic urine, especially when prepared using unvalidated protocols, may not be generalisable and transferrable to real-life sanitation systems involving real urine. For instance, studies conducted exclusively using synthetic urine might inadvertently cast a positive light on a urine treatment technology, even if that technology may not prove equally effective when applied to real urine. Therefore, in this perspective article, these authors aim to share with the research community working on decentralised sanitation certain concerns and important considerations when working with synthetic urine. We hope that the article will stimulate a discussion within the research community on the benefits of using real human urine for experimentation work.
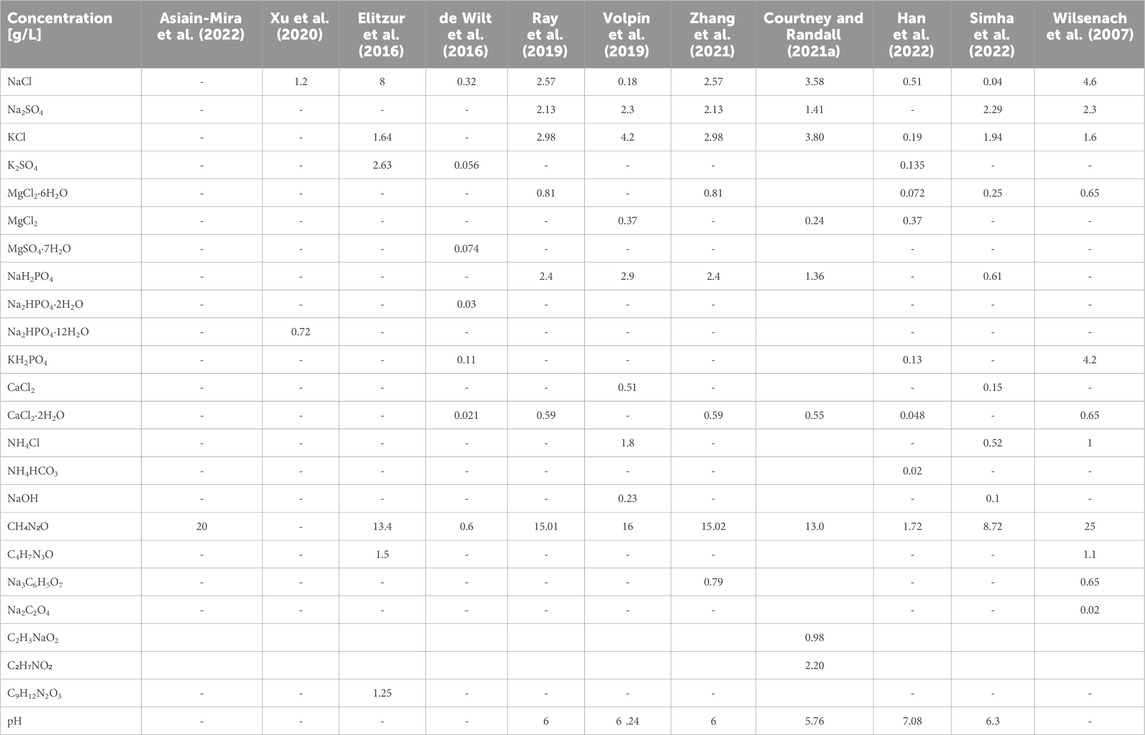
TABLE 1. Concentrations of different constituents in a selection of synthetic fresh human urine recipes described in the literature. Recipes are shown in increasing order of number of metabolites in urine.
2 Real human urine metabolome vs. synthetic human urine metabolome
Urination is the primary route by which the human body eliminates water-soluble wastes. Urine is generated when the kidneys remove water, water-soluble wastes and sugars from the bloodstream. The urine matrix is extremely complex. The major inorganic components of urine include ions such as sodium, potassium, chloride and ammonium, while the major organic metabolites include urea, creatinine, hippuric acid and citric acid (Putnam, 1971; Bouatra et al., 2013). Urine also normally contains several other ions, such as calcium, magnesium, phosphate and sulphate, as well as hundreds of metabolic breakdown products from the consumption of food and beverages, the body’s endogenous waste and exogenous compounds such as pharmaceutical drugs or drug metabolites. The human urine metabolome database (http://www.urinemetabolome.ca) lists more than 3,000 metabolites or metabolite species that have been detected in human urine using existing analytical methods and technologies, the majority of which are endogenous compounds. As illustrated in Figure 1, metabolites in real human urine span a wide range of concentrations (nearly 11 orders of magnitude), chemical structures and solubilities (0.0012 g/L for androsterone to 1000 g/L for ethanolamine, according to Bouatra et al. (Bouatra et al., 2013)). The concentration of an average metabolite in normal human urine varies by ± 50% (Bouatra et al., 2013), because a wide range of factors, including diet, health, age, gender and activity level, determine the composition of urine (Rose et al., 2015). However, irrespective of the gender or time of the day when it is collected, urine contains more than 90 metabolites with 100% occurrence (Bouatra et al., 2013), but the concentrations always vary. According to Putnam (Putnam, 1971), 68 metabolites contribute >99% of the solutes in human urine. In contrast, synthetic urine recipes in the sanitation field typically contain <15 metabolites (Table 1).
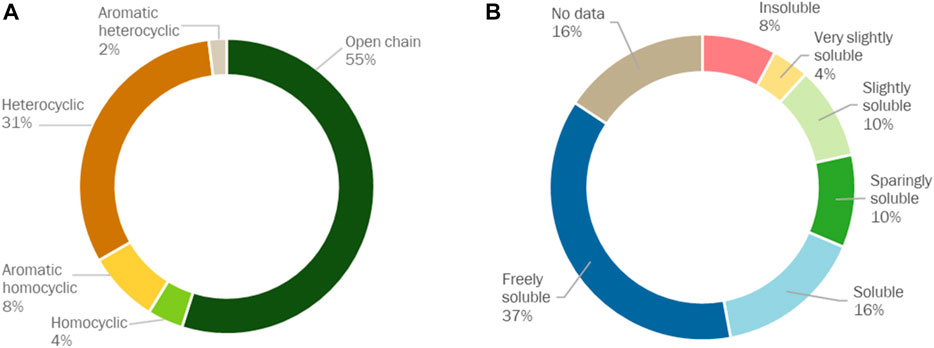
FIGURE 1. Distribution (%) of organic metabolites measured in real human urine by Putnam (Putnam, 1971) according to their (A) chemical structure and (B) solubility in water at 25°C. Standard solubility definitions were taken from WHO (WHO, 2022).
In published literature on wastewater treatment, it is common to encounter the use of synthetic urine or artificial urine, which is essentially a solution of the major inorganic and organic components in real urine, dissolved in water at room temperature. Table 1 lists a small selection of recipes that have been used in different studies to prepare synthetic urine or are referred to in studies as human urine. While this list is certainly not exhaustive, it clearly shows that there is considerable variation in the choice of inorganic and organic ingredients used for making urine, with some recipes using just one metabolite (Asiain-Mira et al., 2022) and some using up to 11 metabolites (Wilsenach et al., 2007). Urine also has a natural bacterial biome (Lewis et al., 2013) and virome (Li et al., 2023) which is not accounted for by these recipes. Therefore, no synthetic urine recipe, however complex, can ever be truly representative of real human urine.
3 Definitions for different types of urine
It is important to distinguish what type of urine a recipe is attempting to mimic. Fresh urine refers to urine collected immediately after it is excreted, although there is no consensus within the research community on how long after excretion urine can be considered fresh. In the literature, some studies have used freshly excreted urine immediately in experiments (Flanagan and Randall, 2018) or have stored it for a few hours (Vasiljev et al., 2022) or days (Simha et al., 2018; Ray et al., 2020) at different temperatures (3°C–30°C) before use. However, in general the term “fresh” is typically used to indicate that no urea hydrolysis has occurred in urine. If fresh urine is diluted with flushwater, it is referred to as diluted fresh urine (e.g., see synthetic recipe of Han et al. (Han et al., 2022) in Table 1). If fresh urine is treated to inhibit urease-catalysed hydrolysis of urea to ammonia, for instance by acidification (Simha et al., 2023), alkalisation (Randall et al., 2016) or oxidation (Lv et al., 2020), the literature defines it as stabilised fresh urine. If urea hydrolysis is not prevented and the majority of the nitrogen in urine is in the form of ammonia, then the urine is considered to be hydrolysed or ureolysed (Udert et al., 2003a). If all the urea excreted in fresh urine is not hydrolysed to ammonia, then the urine is considered to be partially hydrolysed (Tuantet et al., 2013). If hydrolysed urine is treated biologically to stabilise ammonia nitrogen, for instance by nitrification (Udert and Wachter, 2012), then literature defines it as biologically stabilised urine. However, studies using synthetic urine sometimes fail to report what type of urine their recipe represents. Each type of urine has a distinct composition and physical and chemical properties, and distinguishing between the types is important as it has implications for further urine treatment (see, e.g., Simbeye et al. (Simbeye et al., 2023) for an insight into the effect of type of human urine on recovery of phosphate as vivianite). This is one area where the research community would benefit by working together to develop consistent terminology and best practices for reporting experiments involving human urine.
4 Implications of the differences between synthetic urine and real urine
To highlight some key differences between synthetic urine and real urine and the implications of these differences, we conducted a non-systemic literature review, the results of which are summarised below.
4.1 Solubility of chemicals and buffering capacity of urine
Type of urine matrix affects the solubility of different chemicals and the capacity of urine to buffer changes in pH. For example, fresh urine can be dosed with sparingly soluble alkaline Earth chemicals such as Mg(OH)2 and Ca(OH)2 to inhibit urease activity and urea hydrolysis. However, Mg(OH)2 is 40% less soluble in synthetic urine and, due to lower buffering capacity, also has higher pH (>10.8) after treatment than real urine after treatment (pH < 10.6) (Simha et al., 2022). Studies by Ray et al. (Ray et al., 2018) and Simha et al. (2023) have shown that the acid dose needed to shift the pH of fresh urine to below 3.0 is at least two-fold higher for real urine. These are important observations since the stability of most urine treatment processes relies on accurately dosing chemicals. The operating costs of decentralised sanitation systems can also be significantly affected by the chemical demand for treating urine.
The prevailing pH affects both the solubility and the degradation of different organic metabolites in urine. For instance, the rate of degradation of creatine increases as the pH decreases (Jager et al., 2011). In studies on urine acidification and concentration by reverse osmosis, Courtney and Randall (Courtney and Randall, 2021b) have shown that crystals of uric acid dihydrate form in acidified real urine, but not in synthetic urine, resulting in scaling of the membrane surface. These observations are consistent with findings by Wang and Königsberger (Wang and Königsberger, 1998) that the solubility of uric acid decreases as the pH declines and ionic strength of the solution increases. These results suggest that reverse osmosis is not well suited for concentrating acidified urine, while other treatments such as evaporation are not significantly affected by biofouling and scaling. However, apart from three studies on reverse osmosis in the literature (viz. Ek et al. (Ek et al., 2006), Ray et al. (Ray et al., 2020), Courtney and Randall (Courtney and Randall, 2021b)), to our knowledge other studies have only used synthetic urine for experimentation.
Poorly soluble organic compounds tend to co-precipitate with inorganic compounds in real urine. In a study focusing on precipitation of phosphate from fresh urine as vivianite, Simbeye et al. (Simbeye et al., 2023) showed that relatively pure (95%) vivianite could be produced from synthetic urine, but that the purity of vivianite decreases to 75% when made from real urine. This is because organic metabolites in real urine form complexes with divalent ions such as Fe2+ and these complexes can grow around vivianite crystals (Wei et al., 2019) and limit its yield and purity. These differences ultimately affect process economics, as further treatment will be required to improve product purity (e.g., washing vivianite with solvents to selectively remove organic compounds).
4.2 Dissolved organic compounds and membrane fouling
Many synthetic urine recipes (Table 1) are made up of salts to replicate the major inorganic metabolites in urine. However, real urine also has a high organic component (about 10 g COD L-1 according to (Udert et al., 2006) or approximately 25% of the total dissolved solids estimated by Putnam (Putnam, 1971)). A research area where the missing organics in synthetic urine leads to significantly differing experimental results is in membrane research (reverse osmosis, nanofiltration, membrane distillation, etc.), specifically with regards to membrane fouling. For example, during membrane distillation, a crystalline deposit forms on the membrane when synthetic urine is used, while with real urine the deposit that forms is more complex and composed of a rich organic fraction (Kamranvand et al., 2018). Bacteria (Crane et al., 2022) and a combination of urinary sugars and protein (Guizani et al., 2016) have been shown to cause membrane fouling when urine is concentrated by forward osmosis. Zhang et al. (Zhang et al., 2023) found that fouling of a hollow fibre membrane contactor for treating real hydrolysed urine increases as the pH of urine decreases.
The solubility (Franks et al., 2024) and the charge (Wen-Qiong et al., 2019) of organic compounds are both affected by pH. If the charge on the membrane is the same as the charge on an organic compound, electrostatic repulsion between the organic compound and the membrane pores will increase, thus reducing the potential for fouling (Van Reis et al., 1997). Higher solubility means that a higher urine concentration factor can be achieved during treatment before organic compounds precipitate. The properties of the feed solution (pH, ionic strength) and the membrane (charge, hydrophobicity, roughness) affect thermodynamic interactions between dissolved organic compounds and the membrane surface, and influence the mechanisms that cause fouling (Tang et al., 2011).
Experimental results obtained when treating real urine are not always inferior to those obtained when treating synthetic urine. Studies by Courtney and Randall (Courtney and Randall, 2021b) and Pronk et al. (Pronk et al., 2006) observed increased rejection of urea during membrane treatment of real urine. Courtney and Randall (Courtney and Randall, 2021b) found that improved rejection of urea increased overall urea recovery from 79.2% to 85.5% when comparing synthetic urine and real urine. Pronk et al. (Pronk et al., 2006) attributed the increase in rejection of uncharged molecules such as urea to complexation of compounds with the organic substances in real urine. There is also evidence that urea promotes protein unfolding by directly interacting with polar moieties of proteins via hydrogen bonding (Bennion and Daggett, 2003). Unfortunately, synthetic urine cannot be improved by merely adding organics to the recipe used, as in many of these cases the exact organic compounds causing the fouling have not yet been identified (only that they are organic is known) (Courtney and Randall, 2021b; Crane et al., 2022). In these cases, using both real and synthetic urine for experiments becomes valuable for comparing fouling (or lack thereof).
4.3 Thermodynamic modelling of chemical speciation in urine
All models used to simulate a process in urine essentially model synthetic urine. As with making synthetic urine, it is challenging to include every metabolite excreted in real urine in a thermodynamic model. Many modelling databases do not include a comprehensive list of urinary metabolites. For example, the chemistry database of the thermodynamic modelling software OLI (OLI Systems and Inc, 2020) does not include major organic metabolites excreted in urine, such as creatine, creatinine and uric acid. The importance of including or excluding a metabolite will depend on the parameter being measured/simulated. Udert et al. (Udert et al., 2003b) developed a model using Aquasim to estimate the potential for mineral precipitation in sanitation systems that separately collect human urine and found good agreement between simulated and experimental results when the degree of dilution of urine by flushwater was accurately accounted for. Courtney et al. (Courtney et al., 2021) used the same thermodynamic model (in Aquasim) to simulate removal of calcium from human urine by air and CO2 bubbling and found that initially the model did not accurately simulate the pH of urine as a function of bubbling duration. They attributed this to the salinity of urine influencing the pKa of HCO3−/CO3−2 (Millero et al., 2006) and to inclusion of creatinine in the model affecting the buffering capacity of urine at pH 9.2. When adjusted for these two factors, the model accurately captured the change in pH of urine during bubbling (Courtney et al., 2021). Inaccurate simulation of chemical speciation in urine and using synthetic human urine with only a few metabolites therefore have implications for the design of experiments and treatment processes (e.g., operating conditions such as pH and temperature), and for analysis of experimental results (e.g., when evaluating the form and fate of plant-essential nutrients excreted in urine after treatment).
4.4 Removal of pollutants from urine
Several previous studies have used synthetic urine to evaluate removal of pollutants that can potentially be excreted in urine, such as residues and metabolites of pharmaceutical drugs, pesticides, hormones, personal care products, chemicals used for cleaning toilets and heavy metals (Landry et al., 2015; Sun et al., 2018; Almuntashiri et al., 2022; Goulart et al., 2022; Rodriguez et al., 2022; Yao et al., 2022). Many of these are presented as proof-of-concept studies, and there are often no follow-up studies to evaluate whether the treatments can be replicated with a real urine matrix. In some follow-up studies, clear and significant deviations have been observed between studies, primarily because of differences between the urine matrices. There is evidence that analytical detection of pollutants is significantly affected by the type of matrix studied. For instance, ionisation of the target analytes in LC-MS/MS can be affected by endogenous compounds present in urine (Rossmann et al., 2015). The matrix can also affect the removal efficiency of pollutants because the physical and chemical properties of real urine are different from those of synthetic urine, and no synthetic recipe has been developed to account for the hundreds of endogenous organic metabolites excreted in urine (Figure 1). For example, Solanki and Boyer (Solanki and Boyer, 2017) found that >90% removal of pharmaceuticals from synthetic urine could be achieved by adsorption onto biochar. However, in follow-up studies involving real urine, they found that removal of pharmaceuticals declined to 40% because of competition for adsorption sites by dissolved organic compounds naturally excreted in urine, such as urobilin (Solanki and Boyer, 2019). In a study evaluating degradation of 75 organic micropollutants (OMPs) by a UV-based advanced oxidation process, Demissie et al. (Demissie et al., 2023a) observed average ΣOMP degradation of 99% (±4%) in Milli-Q water, but only 55% (±36%) in real fresh urine. This is because endogenous organic compounds in urine can competitively absorb UV light (creatinine and amino acids have high UV absorbability according to Yokoyama et al. (Yokoyama et al., 2005)) and can scavenge free radicals. In another study, Demissie et al. (Demissie et al., 2023b) found that the UV dose needed to irreversibly denature jack bean (Canavalia ensiformis) urease (EC 3.5.1.5) in real fresh human urine was 25-fold higher than the dose needed to denature urease in synthetic fresh human urine. Unfortunately, there seems to be more literature available on treatment of synthetic human urine than on treatment of real human urine for removal of contaminants such as micropollutants.
5 Why and when to use synthetic urine for experimentation?
While synthetic urine is not exactly representative of real urine, it does have many uses and benefits. Below we list a few valid reasons why synthetic urine may be used in research:
1. The composition of real urine varies considerably, as it is influenced by diet, health, age, gender, activity level of people and other factors (Rose et al., 2015). The composition of synthetic urine can be fixed, which can be desirable when conducting experiments to evaluate the influence of several operating conditions on treatment objectives (Tarpeh et al., 2018). The experimental results obtained when synthetic urine is used are generally more consistent than results obtained using real urine with varying composition (Kabdaşlı et al., 2022).
2. The use of synthetic urine is appropriate in proof-of-concept research that proposes novel technologies and methods to treat urine, e.g., Arve and Popat (Arve and Popat, 2021). If experimentation using synthetic urine yields undesirable results, then further testing can be avoided, and time and resources can be saved. For instance, Ray et al. (Ray et al., 2018) showed that zinc and silver ions are not effective inhibitors of urease in synthetic fresh urine as they precipitate with phosphate and chloride naturally present in urine, although the inhibition of urease in soil by these heavy metal ions is well established.
3. Using a synthetic recipe makes it easier to reverse-engineer processes and identify metabolites or properties of urine that significantly affect the outcome of a treatment (Solanki and Boyer, 2019). In addition, different technologies can be more fairly compared against each other if the same synthetic urine recipe is used to evaluate their differences (Chen et al., 2023).
4. Synthetic urine can be helpful for developing new analytical methods for targeted analysis of metabolites (Scherr and Sarmah, 2011) and for educational purposes where the aim is to train students in analytical chemistry or wastewater engineering.
5. Computer-based thermodynamic models of chemical speciation must be validated in real experiments (Courtney and Randall, 2023). Synthetic urine is particularly helpful in such cases since it is not always possible to conduct a full metabolomic analysis of real urine. Many metabolites also do not exist in the chemistry databases of software tools.
6 Guidelines on protocols for preparing synthetic fresh human urine
As shown in Table 1, several recipes for preparing synthetic human urine are found in the water and sanitation literature. However, to the best of our knowledge, none of these recipes use well-established protocols for preparing synthetic urine, (Lienert and Larsen, 2009; Jewitt, 2011; Furlong et al., 2019; Simha et al., 2021), nor have they been validated by comparison with real urine specimens. On the other hand, there is extensive literature in the medical sciences focusing on the development of protocols for synthetic urine preparation in various research domains, including urology (Shafat et al., 2013), dermatology (Mayrovitz and Sims, 2001) and nephrology (Brooks and Keevil, 1997). These protocols have typically been designed to allow investigation of specific aspects, such as the formation of kidney stones (e.g., calcium oxalate dihydrate) in urine, study of renal physiology using in vitro cell culture (Chutipongtanate and Thongboonkerd, 2010), urinary tract infections and growth of urinary pathogens (Brooks and Keevil, 1997). More recent efforts have developed protocols for synthetic urine preparation that are not specific to an application, (Chutipongtanate and Thongboonkerd, 2010; Sarigul et al., 2019), and can be more universally used across research disciplines (Table 2). In fact, Sarigul et al. (2019) have shown that, by using attenuated total reflection-Fourier transform infrared spectroscopy (ATR-FTIR), their synthetic urine comes closest to mimicking real urine. This recipe differs from many of those presented in Table 1 in two ways. First, it does not just include major inorganic metabolites and urea, but also major organic metabolites like creatinine, uric acid, citrate, and oxalate, which are normally excreted in real urine. Secondly, the recipe adds both NaH2PO4·2H2O and Na2HPO4·2H2O which more accurately reflects the speciation of phosphate in real fresh urine, which eliminates the need for adding HCl or NaOH to adjust the pH of urine. Their protocol is also practical as it does not contain an unreasonable number of metabolites (<15). Therefore, to urine researchers working in the sanitation space, we recommend using the protocol developed by Sarigul, et al. (Sarigul et al., 2019) for preparing synthetic urine. However, considering that the concentration of different metabolites in urine varies (e.g., between 9.3 and 23.3 g L-1 for urea according to Putnam (Putnam, 1971)), we also suggest researchers to adjust the protocol (as shown by the “adjusted Putnam” recipe in Table 2) to prepare synthetic urine that accurately represents real urine produced in various geographical contexts. The key components of any synthetic urine recipe should include all major inorganic ions (Na+, K+, Mg2+, Ca2+, NH4+, Cl−, SO42-, PO4-P), and all major organic metabolites (urea, creatinine, uric acid, citrate, and oxalic acid).
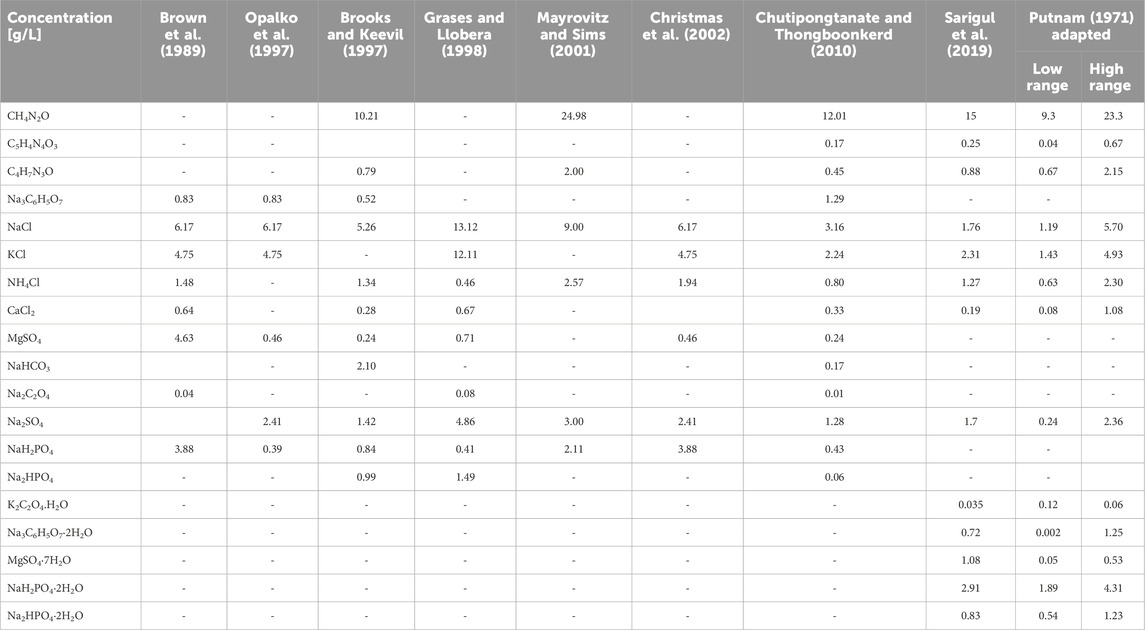
TABLE 2. Concentrations of different constituents in a selection of synthetic human urine recipes described in the medical sciences literature. To urine researchers working in the sanitation space, we recommend using the protocol developed by (Sarigul et al., 2019) and adapt it to account for variation in concentration of different metabolites excreted in urine. “Putnam adapted” shows the minimum and maximum acceptable limits for concentration of major metabolites in urine.
There are several sub-topics in sanitation-focused urine research, including the recovery of plant-essential nutrients and energy, removal of micropollutants, and inactivation of pathogens. These would need to be considered when adapting and using such a protocol. It is always possible to add more metabolites to synthetic urine depending on the question at hand (e.g., Brooks and Keevil (Brooks and Keevil, 1997) added bacteriological peptone to account for amino acids and short chain peptides and yeast extract to account for nucleic acids excreted in urine, respectively). This can be particularly relevant when experimentation involves developing methods to reduce malodour from urine-separating toilets or light irradiation to degrade pollutants in urine, as all protocols for making synthetic urine produce odourless and colourless urine.
7 Conclusion and the way forward
Research on innovative technologies to recycle the resources in human urine is highly relevant for achieving a sustainable future and circular economy in the sanitation space. As such research has real-life implications, it is critical to constantly evaluate methods and methodological choices used in experimentation on this topic. One such choice that researchers must make is whether to use synthetic urine or real human urine. In this article, we argue that it can be difficult to accurately replicate the complex metabolome and properties of real urine using a synthetic recipe. We provide select examples from literature to suggest that results obtained when synthetic urine is used can differ from those obtained when real urine is used. In some cases, results of experiments conducted with real urine are not as promising as those achieved with synthetic urine, but publication of negative results is still to the benefit of the entire research community. While there are several benefits and valid reasons for using synthetic urine in research, some of which we present in this article, we have also noted a lack of consistency in the protocols for preparing synthetic urine in sanitation research. To address this, we provide suggestions and preliminary guidelines on protocols for preparing and using artificial urine, inspired by literature from the medical sciences. We hope that this article initiates a discussion on methodological choices in urine research among the community engaged with decentralised sanitation systems. Overall, we have the following recommendations:
1. Whenever it is feasible, work with real urine. Urine can be collected in a depersonalised manner from several donors and pooled together. Increasing the number of donors or the collection period for urine can mitigate issues related to variability in urine composition.
2. When preparing synthetic urine, use protocols that have been evaluated and validated with real urine, such as the protocol outlined by (Sarigul et al., 2019) (See Table 2). Modify the protocol as shown in “Putnam adapted” in Table 2 to make synthetic urine that is representative of real urine produced in various geographical contexts. Include or exclude specific metabolites from the protocol depending on the research question, as this can be important when assessing different topics in sanitation-focused urine research, such as the degradation of micropollutants.
3. Follow up an experiment involving synthetic urine that yielded positive results with an experiment using real human urine, ideally within the same study. In studies where results of only synthetic urine are reported, authors should try to hypothesize how their results could differ in case real urine is used, raise any potential aspects that could be of concern, and recommend follow-up studies to use real urine to validate these hypotheses and/or confirm that similar results with real urine can be achieved.
4. Always clearly state in the article title, abstract and conclusions whether real or synthetic/artificial urine was used in experiments. In addition, specify within the article the type of urine used/replicated in the study (see Section 3 for definitions).
5. Work together as a community to develop terminology, definitions, methodologies and best practices for experimental work involving human urine.
Data availability statement
The original contributions presented in the study are included in the article/Supplementary Material, further inquiries can be directed to the corresponding author.
Author contributions
PS: Conceptualization, Data curation, Formal Analysis, Funding acquisition, Investigation, Methodology, Project administration, Resources, Validation, Visualization, Writing–original draft, Writing–review and editing. CC: Conceptualization, Data curation, Formal Analysis, Investigation, Methodology, Software, Validation, Visualization, Writing–original draft, Writing–review and editing. DR: Conceptualization, Investigation, Methodology, Software, Writing–original draft, Writing–review and editing.
Funding
The author(s) declare financial support was received for the research, authorship, and/or publication of this article. PS was supported by grants from Stiftelsen Lantbruksforskning for the project “Micropollutants-free sustainable beer production” (Grant number O-22-23-744) and the European Union’s Horizon Europe Research and Innovation Programme for the project “P2Green: Closing the gap between fork and farm for circular nutrient flows” (Grant number 101081883). Dyllon Randall and CC were supported by internal funding from the University of Cape Town and the August T Larsson Guest Researcher Programme at the Swedish University of Agricultural Sciences.
Conflict of interest
The authors declare that the research was conducted in the absence of any commercial or financial relationships that could be construed as a potential conflict of interest.
The author(s) declared that they were an editorial board member of Frontiers, at the time of submission. This had no impact on the peer review process and the final decision.
Publisher’s note
All claims expressed in this article are solely those of the authors and do not necessarily represent those of their affiliated organizations, or those of the publisher, the editors and the reviewers. Any product that may be evaluated in this article, or claim that may be made by its manufacturer, is not guaranteed or endorsed by the publisher.
Supplementary material
The Supplementary Material for this article can be found online at: https://www.frontiersin.org/articles/10.3389/fenvs.2024.1367982/full#supplementary-material
References
Aliahmad, A., Harder, R., Simha, P., Vinnerås, B., and McConville, J. (2022). Knowledge evolution within human urine recycling technological innovation system (TIS): focus on technologies for recovering plant-essential nutrients. J. Clean. Prod. 379, 134786. doi:10.1016/j.jclepro.2022.134786
Almuntashiri, A., Hosseinzadeh, A., Badeti, U., Shon, H., Freguia, S., Dorji, U., et al. (2022). Removal of pharmaceutical compounds from synthetic hydrolysed urine using granular activated carbon: column study and predictive modelling. J. Water Process Eng. 45, 102480. doi:10.1016/j.jwpe.2021.102480
Arve, P. H., and Popat, S. C. (2021). Stabilization of urea for recovery from source-separated urine using electrochemically synthesized hydrogen peroxide. ACS ES&T Eng. 1, 1642–1648. doi:10.1021/acsestengg.1c00194
Asiain-Mira, R., Smith, C., Zamora, P., Monsalvo, V. M., and Torrente-Murciano, L. (2022). Hydrogen production from urea in human urine using segregated systems. Water Res. 222, 118931. doi:10.1016/j.watres.2022.118931
Bennion, B. J., and Daggett, V. (2003). The molecular basis for the chemical denaturation of proteins by urea. Proc. Natl. Acad. Sci. 100, 5142–5147. doi:10.1073/pnas.0930122100
Bouatra, S., Aziat, F., Mandal, R., Guo, A. C., Wilson, M. R., Knox, C., et al. (2013). The human urine metabolome. PLoS One 8, e73076. doi:10.1371/journal.pone.0073076
Brooks, T., and Keevil, C. (1997). A simple artificial urine for the growth of urinary pathogens. Lett. Appl. Microbiol. 24, 203–206. doi:10.1046/j.1472-765x.1997.00378.x
Brown, P., Ackermann, D., and Finlayson, B. (1989). Calcium oxalate dihydrate (weddellite) precipitation. J. Cryst. growth 98, 285–292. doi:10.1016/0022-0248(89)90143-7
Chen, H., Shashvatt, U., Amurrio, F., Stewart, K., and Blaney, L. (2023). Sustainable nutrient recovery from synthetic urine by Donnan dialysis with tubular ion-exchange membranes. Chem. Eng. J. 460, 141625. doi:10.1016/j.cej.2023.141625
Christmas, K. G., Gower, L. B., Khan, S. R., and El-Shall, H. (2002). Aggregation and dispersion characteristics of calcium oxalate monohydrate: effect of urinary species. J. Colloid Interface Sci. 256, 168–174. doi:10.1006/jcis.2002.8283
Chutipongtanate, S., and Thongboonkerd, V. (2010). Systematic comparisons of artificial urine formulas for in vitro cellular study. Anal. Biochem. 402, 110–112. doi:10.1016/j.ab.2010.03.031
Courtney, C., Brison, A., and Randall, D. G. (2021). Calcium removal from stabilized human urine by air and CO(2) bubbling. Water Res. 202, 117467. doi:10.1016/j.watres.2021.117467
Courtney, C., and Randall, D. G. (2021a). Precipitation to remove calcium ions from stabilized human urine as a pre-treatment for reverse osmosis. Water Sci. Technol. 84, 3755–3768. doi:10.2166/wst.2021.479
Courtney, C., and Randall, D. G. (2021b). Concentrating stabilized urine with reverse osmosis: how does stabilization method and pre-treatment affect nutrient recovery, flux, and scaling? Water Res. 209, 117970. doi:10.1016/j.watres.2021.117970
Courtney, C., and Randall, D. G. (2023). Concentrating stabilized human urine using eutectic freeze crystallization for liquid fertilizer production. Water Res. 233, 119760. doi:10.1016/j.watres.2023.119760
Crane, L., Ray, H., Perreault, F., and Boyer, T. H. (2022). Recovery of urea from human urine using nanofiltration and reverse osmosis. ACS ES&T Water 3, 1835–1846. doi:10.1021/acsestwater.2c00336
Demissie, N. G., Simha, P., Lai, F. Y., Ahrens, L., Mussabek, D., Desta, A., et al. (2023a). Degradation of 75 organic micropollutants in fresh human urine and water by UV advanced oxidation process. Water Res. 242, 120221. doi:10.1016/j.watres.2023.120221
Demissie, N. G., Simha, P., Vasiljev, A., Vinnerås, B., Mussabek, D., Desta, A., et al. (2023b). Degradation of 75 organic micropollutants in fresh human urine and water by UV advanced oxidation process. Water Res. 24, 120221. doi:10.1016/j.watres.2023.120221
de Wilt, A., Butkovskyi, A., Tuantet, K., Leal, L. H., Fernandes, T. V., Langenhoff, A., et al. (2016). Micropollutant removal in an algal treatment system fed with source separated wastewater streams. J. Hazard Mater 304, 84–92. doi:10.1016/j.jhazmat.2015.10.033
Ek, M., Bergstrom, R., Bjurhem, J. E., Bjorlenius, B., and Hellstrom, D. (2006). Concentration of nutrients from urine and reject water from anaerobically digested sludge. Water Sci. Technol. 54, 437–444. doi:10.2166/wst.2006.924
Elitzur, S., Rosenband, V., and Gany, A. (2016). Urine and aluminum as a source for hydrogen and clean energy. Int. J. Hydrogen Energy 41, 11909–11913. doi:10.1016/j.ijhydene.2016.05.259
Flanagan, C. P., and Randall, D. G. (2018). Development of a novel nutrient recovery urinal for on-site fertilizer production. J. Environ. Chem. Eng. 6, 6344–6350. doi:10.1016/j.jece.2018.09.060
Franks, R., Bartels, C., and Nagghappan, L. N. S. P. (2024). “Membrane technology,” in Proceedings of the American water works association membrane technology conference (West Palm Beach, Florida: Palm Beach County Convention Center).
Furlong, C., Jegatheesan, J., Currell, M., Iyer-Raniga, U., Khan, T., and Ball, A. S. (2019). Is the global public willing to drink recycled water? A review for researchers and practitioners. Util. Policy 56, 53–61. doi:10.1016/j.jup.2018.11.003
Goulart, L. A., Moratalla, A., Cañizares, P., Lanza, M. R., Sáez, C., and Rodrigo, M. A. (2022). High levofloxacin removal in the treatment of synthetic human urine using Ti/MMO/ZnO photo-electrocatalyst. J. Environ. Chem. Eng. 10, 107317. doi:10.1016/j.jece.2022.107317
Grases, F., and Llobera, A. (1998). Experimental model to study sedimentary kidney stones. Micron 29, 105–111. doi:10.1016/s0968-4328(98)00006-7
Guest, J. S., Skerlos, S. J., Barnard, J. L., Beck, M. B., Daigger, G. T., Hilger, H., et al. (2009). A new planning and design paradigm to achieve sustainable resource recovery from wastewater. Environ. Sci. Technol. 43, 6126–6130. doi:10.1021/es9010515
Guizani, M., Fujii, T., Hijikata, N., and Funamizu, N. (2016). Salt removal from soil during rainy season of semi-arid climate following an assumed salt accumulation from previous cultivations fertilized with urine. Euro-Mediterranean J. Environ. Integration 1, 10. doi:10.1007/s41207-016-0010-9
Han, C., Yuan, X., Ma, S., Li, Y., Feng, Y., and Liu, J. (2022). Simultaneous recovery of nutrients and power generation from source-separated urine based on bioelectrical coupling with the hydrophobic gas permeable tube system. Sci. Total Environ. 824, 153788. doi:10.1016/j.scitotenv.2022.153788
Jager, R., Purpura, M., Shao, A., Inoue, T., and Kreider, R. B. (2011). Analysis of the efficacy, safety, and regulatory status of novel forms of creatine. Amino Acids 40, 1369–1383. doi:10.1007/s00726-011-0874-6
Jewitt, S. (2011). Geographies of shit: spatial and temporal variations in attitudes towards human waste. Prog. Hum. Geogr. 35, 608–626. doi:10.1177/0309132510394704
Kabdaşlı, I., Kuşçuoğlu, S., Tünay, O., and Siciliano, A. (2022). Assessment of K-struvite precipitation as a means of nutrient recovery from source separated human urine. Sustainability 14, 1082. doi:10.3390/su14031082
Kamranvand, F., Davey, C., Sakar, H., Autin, O., Mercer, E., Collins, M., et al. (2018). Impact of fouling, cleaning and faecal contamination on the separation of water from urine using thermally driven membrane separation. Sep. Sci. Technol. 53, 1372–1382. doi:10.1080/01496395.2018.1433688
Landry, K. A., Sun, P., Huang, C. H., and Boyer, T. H. (2015). Ion-exchange selectivity of diclofenac, ibuprofen, ketoprofen, and naproxen in ureolyzed human urine. Water Res. 68, 510–521. doi:10.1016/j.watres.2014.09.056
Larsen, T. A., Riechmann, M. E., and Udert, K. M. (2021). State of the art of urine treatment technologies: a critical review. Water Res. X 13, 100114. doi:10.1016/j.wroa.2021.100114
Larsen, T. A., Udert, K. M., and Lienert, J. (2013). Source separation and decentralization for wastewater management. London. IWA Publishing.
Lewis, D. A., Brown, R., Williams, J., White, P., Jacobson, S. K., Marchesi, J. R., et al. (2013). The human urinary microbiome; bacterial DNA in voided urine of asymptomatic adults. Front. Cell Infect. Microbiol. 3, 41. doi:10.3389/fcimb.2013.00041
Li, L., Cai, J., Eisenberg, J. N. S., Goetsch, H. E., Love, N. G., and Wigginton, K. R. (2023). Virus emissions from toilet flushing: comparing urine-diverting to mix flush toilets. ACS ES T Water 3, 457–464. doi:10.1021/acsestwater.2c00521
Lienert, J., and Larsen, T. A. (2009). High acceptance of urine source separation in seven European countries: a review. Environ. Sci. Technol. 44, 556–566. doi:10.1021/es9028765
Lv, Y., Li, Z., Zhou, X., Cheng, S., and Zheng, L. (2020). Stabilization of source-separated urine by heat-activated peroxydisulfate. Sci. Total Environ. 749, 142213. doi:10.1016/j.scitotenv.2020.142213
Mayrovitz, H. N., and Sims, N. (2001). Biophysical effects of water and synthetic urine on skin. Adv. Skin. Wound Care 14, 302–308. doi:10.1097/00129334-200111000-00013
Millero, F. J., Graham, T. B., Huang, F., Bustos-Serrano, H., and Pierrot, D. (2006). Dissociation constants of carbonic acid in seawater as a function of salinity and temperature. Mar. Chem. 100, 80–94. doi:10.1016/j.marchem.2005.12.001
Opalko, F. J., Adair, J. H., and Khan, S. R. (1997). Heterogeneous nucleation of calcium oxalate trihydrate in artificial urine by constant composition. J. Cryst. growth 181, 410–417. doi:10.1016/s0022-0248(97)00222-4
Penn, R., Ward, B. J., Strande, L., and Maurer, M. (2018). Review of synthetic human faeces and faecal sludge for sanitation and wastewater research. Water Res. 132, 222–240. doi:10.1016/j.watres.2017.12.063
Perez-Mercado, L. F., Perez-Mercado, C. A., Vinnerås, B., and Simha, P. (2022). Nutrient stocks, flows and balances for the Bolivian agri-food system: can recycling human excreta close the nutrient circularity gap? Front. Environ. Sci. 10, 956325. doi:10.3389/fenvs.2022.956325
Pronk, W., Biebow, M., and Boller, M. (2006). Electrodialysis for recovering salts from a urine solution containing micropollutants. Environ. Sci. Technol. 40, 2414–2420. doi:10.1021/es051921i
Putnam, D. F. (1971). Composition and concentrative properties of human urine. Washington, D.C.: National Aeronautics and Space Administration.
Randall, D. G., Krahenbuhl, M., Kopping, I., Larsen, T. A., and Udert, K. M. (2016). A novel approach for stabilizing fresh urine by calcium hydroxide addition. Water Res. 95, 361–369. doi:10.1016/j.watres.2016.03.007
Ray, H., Perreault, F., and Boyer, T. H. (2019). Urea recovery from fresh human urine by forward osmosis and membrane distillation (FO–MD). Environ. Sci. Water Res. Technol. 5, 1993–2003. doi:10.1039/c9ew00720b
Ray, H., Perreault, F., and Boyer, T. H. (2020). Rejection of nitrogen species in real fresh and hydrolyzed human urine by reverse osmosis and nanofiltration. J. Environ. Chem. Eng. 8, 103993. doi:10.1016/j.jece.2020.103993
Ray, H., Saetta, D., and Boyer, T. H. (2018). Characterization of urea hydrolysis in fresh human urine and inhibition by chemical addition. Environ. Sci. Water Res. Technol. 4, 87–98. doi:10.1039/c7ew00271h
Rockstrom, J., Gupta, J., Qin, D., Lade, S. J., Abrams, J. F., Andersen, L. S., et al. (2023). Safe and just Earth system boundaries. Nature 619, 102–111. doi:10.1038/s41586-023-06083-8
Rodriguez, E. E., Tarpeh, W. A., Wigginton, K. R., and Love, N. G. (2022). Application of plasma for the removal of pharmaceuticals in synthetic urine. Environ. Sci. Water Res. Technol. 8, 523–533. doi:10.1039/d1ew00863c
Rose, C., Parker, A., Jefferson, B., and Cartmell, E. (2015). The characterization of feces and urine: a review of the literature to inform advanced treatment technology. Crit. Rev. Environ. Sci. Technol. 45, 1827–1879. doi:10.1080/10643389.2014.1000761
Rossmann, J., Gurke, R., Renner, L. D., Oertel, R., and Kirch, W. (2015). Evaluation of the matrix effect of different sample matrices for 33 pharmaceuticals by post-column infusion. J. Chromatogr. B Anal. Technol. Biomed. Life Sci. 1000, 84–94. doi:10.1016/j.jchromb.2015.06.019
Sarigul, N., Korkmaz, F., and Kurultak, I. (2019). A new artificial urine protocol to better imitate human urine. Sci. Rep. 9, 20159. doi:10.1038/s41598-019-56693-4
Scherr, F. F., and Sarmah, A. K. (2011). Simultaneous analysis of free and sulfo-conjugated steroid estrogens in artificial urine solution and agricultural soils by high-performance liquid chromatography. J. Environ. Sci. Health B 46, 763–772. doi:10.1080/03601234.2012.597702
Shafat, M., Rajakumar, K., Syme, H., Buchholz, N., and Knight, M. M. (2013). Stent encrustation in feline and human artificial urine: does the low molecular weight composition account for the difference? Urolithiasis 41, 481–486. doi:10.1007/s00240-013-0608-1
Simbeye, C., Courtney, C., Simha, P., Fischer, N., and Randall, D. G. (2023). Human urine: a novel source of phosphorus for vivianite production. Sci. Total Environ. 892, 164517. doi:10.1016/j.scitotenv.2023.164517
Simha, P., Barton, M. A., Perez-Mercado, L. F., McConville, J. R., Lalander, C., Magri, M. E., et al. (2021). Willingness among food consumers to recycle human urine as crop fertiliser: evidence from a multinational survey. Sci. Total Environ. 765, 144438. doi:10.1016/j.scitotenv.2020.144438
Simha, P., Deb, C. K., Randall, D. G., and Vinnerås, B. (2022). Thermodynamics and kinetics of pH-dependent dissolution of sparingly soluble alkaline earth hydroxides in source-separated human urine collected in decentralised sanitation systems. Front. Environ. Sci. 10, 889119. doi:10.3389/fenvs.2022.889119
Simha, P., Senecal, J., Nordin, A., Lalander, C., and Vinnerås, B. (2018). Alkaline dehydration of anion–exchanged human urine: volume reduction, nutrient recovery and process optimisation. Water Res. 142, 325–336. doi:10.1016/j.watres.2018.06.001
Simha, P., Vasiljev, A., Randall, D. G., and Vinneras, B. (2023). Factors influencing the recovery of organic nitrogen from fresh human urine dosed with organic/inorganic acids and concentrated by evaporation in ambient conditions. Sci. Total Environ. 879, 163053. doi:10.1016/j.scitotenv.2023.163053
Solanki, A., and Boyer, T. H. (2017). Pharmaceutical removal in synthetic human urine using biochar. Environ. Sci. Water Res. Technol. 3, 553–565. doi:10.1039/c6ew00224b
Solanki, A., and Boyer, T. H. (2019). Physical-chemical interactions between pharmaceuticals and biochar in synthetic and real urine. Chemosphere 218, 818–826. doi:10.1016/j.chemosphere.2018.11.179
Sun, P., Li, Y., Meng, T., Zhang, R., Song, M., and Ren, J. (2018). Removal of sulfonamide antibiotics and human metabolite by biochar and biochar/H(2)O(2) in synthetic urine. Water Res. 147, 91–100. doi:10.1016/j.watres.2018.09.051
Tang, C. Y., Chong, T. H., and Fane, A. G. (2011). Colloidal interactions and fouling of NF and RO membranes: a review. Adv. colloid interface Sci. 164, 126–143. doi:10.1016/j.cis.2010.10.007
Tarpeh, W. A., Wald, I., Wiprächtiger, M., and Nelson, K. L. (2018). Effects of operating and design parameters on ion exchange columns for nutrient recovery from urine. Environ. Sci. Water Res. Technol. 4, 828–838. doi:10.1039/C7EW00478H
Tuantet, K., Janssen, M., Temmink, H., Zeeman, G., Wijffels, R. H., and Buisman, C. J. N. (2013). Microalgae growth on concentrated human urine. J. Appl. Phycol. 26, 287–297. doi:10.1007/s10811-013-0108-2
Udert, K. M., Larsen, T. A., Biebow, M., and Gujer, W. (2003a). Urea hydrolysis and precipitation dynamics in a urine-collecting system. Water Res. 37, 2571–2582. doi:10.1016/s0043-1354(03)00065-4
Udert, K. M., Larsen, T. A., and Gujer, W. (2003b). Estimating the precipitation potential in urine-collecting systems. Water Res. 37, 2667–2677. doi:10.1016/s0043-1354(03)00071-x
Udert, K. M., Larsen, T. A., and Gujer, W. (2006). Fate of major compounds in source-separated urine. Water Sci. Technol. 54, 413–420. doi:10.2166/wst.2006.921
Udert, K. M., and Wachter, M. (2012). Complete nutrient recovery from source-separated urine by nitrification and distillation. Water Res. 46, 453–464. doi:10.1016/j.watres.2011.11.020
Van Reis, R., Gadam, S., Frautschy, L. N., Orlando, S., Goodrich, E. M., Saksena, S., et al. (1997). High performance tangential flow filtration. Biotechnol. Bioeng. 56, 71–82. doi:10.1002/(sici)1097-0290(19971005)56:1<71::aid-bit8>3.0.co;2-s
Vasiljev, A., Simha, P., Demisse, N., Karlsson, C., Randall, D. G., and Vinnerås, B. (2022). Drying fresh human urine in magnesium-doped alkaline substrates: capture of free ammonia, inhibition of enzymatic urea hydrolysis and minimisation of chemical urea hydrolysis. Chem. Eng. J. 428, 131026. doi:10.1016/j.cej.2021.131026
Volpin, F., Chekli, L., Phuntsho, S., Ghaffour, N., Vrouwenvelder, J., and Shon, H. K. (2019). Optimisation of a forward osmosis and membrane distillation hybrid system for the treatment of source-separated urine. Sep. Purif. Technol. 212, 368–375. doi:10.1016/j.seppur.2018.11.003
Wang, Z., and Königsberger, E. (1998). Solubility equilibria in the uric acid–sodium urate–water system1Dedicated to Professor Heinz Gamsjäger on the occasion of his 65th birthday12Presented at the Twelfth Ulm-Freiberg Conference, Freiberg, Germany, 19–21 March 19972. Thermochim. Acta 310, 237–242. doi:10.1016/s0040-6031(97)00230-x
Wei, L., Hong, T., Li, X., Li, M., Zhang, Q., and Chen, T. (2019). New insights into the adsorption behavior and mechanism of alginic acid onto struvite crystals. Chem. Eng. J. 358, 1074–1082. doi:10.1016/j.cej.2018.10.110
Wen-Qiong, W., Yun-Chao, W., Xiao-Feng, Z., Rui-Xia, G., and Mao-Lin, L. (2019). Whey protein membrane processing methods and membrane fouling mechanism analysis. Food Chem. 289, 468–481. doi:10.1016/j.foodchem.2019.03.086
Wilsenach, J. A., and Loosdrecht, M. C. V. (2006). Integration of processes to treat wastewater and source-separated urine. J. Environ. Eng. 132, 331–341. doi:10.1061/(asce)0733-9372(2006)132:3(331)
Wilsenach, J. A., Schuurbiers, C. A., and van Loosdrecht, M. C. (2007). Phosphate and potassium recovery from source separated urine through struvite precipitation. Water Res. 41, 458–466. doi:10.1016/j.watres.2006.10.014
Xu, L., Yu, C., Tian, S., Mao, Y., Zong, Y., Zhang, X., et al. (2020). Selective recovery of phosphorus from synthetic urine using flow-electrode capacitive deionization (FCDI)-Based technology. ACS ES&T Water 1, 175–184. doi:10.1021/acsestwater.0c00065
Yao, H., Zhang, X., Liu, F., and Sun, S. (2022). Removal of carbamazepine (CBZ) from synthetic urine by FeOCl-coated ceramic membrane: the study of kinetic modeling. Chemosphere 298, 134222. doi:10.1016/j.chemosphere.2022.134222
Yokoyama, Y., Tsuji, S., and Sato, H. (2005). Simultaneous determination of creatinine, creatine, and UV-absorbing amino acids using dual-mode gradient low-capacity cation-exchange chromatography. J. Chromatogr. A 1085, 110–116. doi:10.1016/j.chroma.2005.01.030
Zhang, J., Li, K., Xie, M., Han, Q., Feng, L., Qu, D., et al. (2023). A new insight into the low membrane fouling tendency of liquid-liquid hollow fiber membrane contactor capturing ammonia from human urine. Water Res. 233, 119795. doi:10.1016/j.watres.2023.119795
Keywords: simulation, synthetic urine, membrane, micropollutants, nutrient recycling, wastewater treatment
Citation: Simha P, Courtney C and Randall DG (2024) An urgent call for using real human urine in decentralized sanitation research and advancing protocols for preparing synthetic urine. Front. Environ. Sci. 12:1367982. doi: 10.3389/fenvs.2024.1367982
Received: 09 January 2024; Accepted: 04 March 2024;
Published: 14 March 2024.
Edited by:
Efthalia Chatzisymeon, University of Edinburgh, United KingdomReviewed by:
Shamshad Ahmad, District Water Testing Laboratory Jal Nigam, IndiaSukalyan Sengupta, University of Massachusetts Dartmouth, United States
Copyright © 2024 Simha, Courtney and Randall. This is an open-access article distributed under the terms of the Creative Commons Attribution License (CC BY). The use, distribution or reproduction in other forums is permitted, provided the original author(s) and the copyright owner(s) are credited and that the original publication in this journal is cited, in accordance with accepted academic practice. No use, distribution or reproduction is permitted which does not comply with these terms.
*Correspondence: Prithvi Simha, UHJpdGh2aS5TaW1oYUBzbHUuc2U=