- 1Changzhi Medical College, Changzhi, China
- 2Department of Applied Chemistry, College of Science, China Agricultural University, Beijing, China
Background: With the growing use of nitrogen-containing pesticides in agriculture, their residues in the environment have raised significant public health concerns.
Objective: This study aimed to develop a novel PPy-DBSA/IT-SPME coating to enhance the detection efficiency of polar nitrogen-containing pesticides in water matrices.
Methods: The preparation conditions were optimized, including pyrrole concentration at 7.0 mol/L, DBSA concentration at 0.014 mol/L, oxidant concentration at 0.35 mol/L, and a coating cycle repeated 10 times. High-performance liquid chromatography-tandem mass spectrometry (HPLC-MS/MS) was employed for detection.
Results: The novel coating effectively adsorbed 19 different categories of polar nitrogen-containing pesticides, including sulfonylureas, triazolopyrimidines, diphenyl ether herbicides, benzoylurea insecticides, and phenylurea herbicides, with unadsorbed rates below 10%. The analytical method achieved an average recovery rate of 61.92% to 115.21%, with an RSD below 5.0%. Detection and quantification limits ranged from 0.012 to 0.524 μg/L and 0.127 to 5.243 μg/L, respectively.
Conclusion: The developed method is green, efficient, simple, and cost-effective. It offers an environmentally friendly and user-friendly approach for the detection of polar nitrogen-containing pesticide residues, demonstrating good recovery and precision. This method holds potential for wide application in environmental monitoring and food safety.
Introduction
Polar nitrogen-containing pesticides are widely used in modern agricultural production due to their high efficiency, low toxicity, and broad application range. These pesticides, including various chemical categories such as triazine, neonicotinoid, and sulfonylurea, play a crucial role in pest and weed control. However, their extensive use also poses potential environmental risks as they can enter water bodies through surface runoff, infiltration, and other pathways, threatening aquatic ecosystems and human health. Therefore, monitoring and assessing the residual levels of these pesticides in the environment are of paramount importance (Chow et al., 2020; Berni et al., 2021; Gormez et al., 2022).
The extraction, preconcentration, and detection of pesticides from various samples pose significant challenges due to the complexity of the analyzed samples and the low concentrations of the target analytes (Guo et al., 2020; Bagheri et al., 2022). The primary objectives of sample preparation are to remove interferences and preconcentrate the target analytes. Liquid-liquid extraction (LLE) is a popular sample pretreatment method for pesticide preconcentration, known for its simplicity and direct application (Yuan et al., 2020; Hammad et al., 2022; Lemos et al., 2022). However, traditional sample pretreatment methods like LLE often involve the use of large amounts of organic solvents, are labor-intensive, and lack efficiency (Al Shirity et al., 2023). These limitations have led researchers to seek more efficient and environmentally friendly alternative techniques (Khalil, 2020; Moreno et al., 2020; Saini et al., 2021).
Solid-phase microextraction (SPME) technology, characterized by its miniaturization, solvent-free nature, automation, and high sensitivity, has emerged as a powerful tool for sample pretreatment (Manousi et al., 2020; Kim and Karthikraj, 2021; Dugheri et al., 2022). In particular, in-tube SPME (IT-SPME) has been widely applied in the analysis of pollutants in environmental, food, and biological samples due to its simplicity and excellent extraction performance (Kataoka, 2021; Badawy et al., 2022). The key to the success of IT-SPME lies in the proper selection of the coating material, as the concentration equilibrium between the target analytes and the adsorbent occurs on the coating (Lasarte-Aragonés et al., 2020; Peng and Cao, 2021; Agatonovic-Kustrin et al., 2023). Common coating materials for IT-SPME are porous polydivinylbenzene (Supel-Q), TRB-5 (95% dimethyl/5% diphenyl polysiloxane), carboxylic molecular sieves, polydimethylsiloxane, and polyethylene glycol (Sun et al., 2022). However, commercial IT-SPME coatings are not only expensive but also impractical for many applications (Asiabi et al., 2018; Wang et al., 2019). Moreover, these commercial coatings exhibit poor selectivity toward target analytes. Therefore, the development of new selective materials to enhance extraction selectivity is necessary (Chu et al., 2021).
Polypyrrole (PPy), as a conductive polymer, has shown great potential in the field of SPME due to its unique electronic structure and adsorption properties (Du et al., 2021; Ma et al., 2021). The adsorption capacity of PPy can be significantly enhanced by introducing dopants (Khan et al., 2021; Pal et al., 2023; Yu et al., 2024). In particular, dodecylbenzene sulfonic acid (DBSA), as a surfactant, not only improves the thermal and mechanical stability of PPy upon doping but also provides additional adsorption sites through its sulfonic acid group, benzene ring, and long alkyl chain, thereby enhancing its adsorption capacity for polar nitrogen-containing pesticides. In the fields of sensors and electrocatalysis, PPy, with its conjugated double bond structure, can generate π-π interactions, dipole-dipole interactions, electrostatic interactions, and hydrophobic effects, making it an ideal adsorbent for polar and ionic compounds (Hussain et al., 2021; Du et al., 2023). However, pure PPy exhibits low thermal stability (thermal decomposition temperature of approximately 230°C) and a small surface area, limiting its application in extraction performance (Ju et al., 2022; Wu et al., 2022). To overcome these limitations, doping with other materials has become an effective strategy to improve PPy’s performance (Yin et al., 2022; Zhang et al., 2022; Özuğur Uysal et al., 2022). For example, surfactant-doped polypyrroles not only improve their thermal and mechanical stability but also greatly enhance the performance of the adsorbent through the functional groups such as sulfonic acid, benzene ring, and long alkyl chain in their molecular structure (Begum et al., 2021; Jain et al., 2023). Studies have shown that compared to PPy coatings doped with sodium p-toluenesulfonate, sodium tetrafluoroborate, and sodium perchlorate, PPy coatings doped with tetrakis (4-sulfonate phenyl)porphyrin nickel (II) salt (NiPcTs) exhibit up to a fivefold higher extraction efficiency for benzene derivatives, owing to the presence of the benzene moiety in NiPcTs, which increases the π-π interaction between benzene derivatives and the coating (Mahato et al., 2020; Ke et al., 2022; Geng et al., 2023). These findings confirm the tremendous potential of doped polypyrroles as adsorbents in improving extraction efficiency.
In summary, we prepared dodecylbenzene sulfonic acid-doped polypyrrole in-tube solid-phase microextraction coating (PPy-DBSA/IT-SPME) using chemical oxidation on a polytetrafluoroethylene tube (PFA) supported material and used it for the adsorption of 19 nitrogen-containing pesticides in environmental water. The extraction length of this method employing PPy-DBSA is 15 cm with an inner diameter of 0.75 mm, making it a compact and portable extraction device. In comparison to extraction methods such as vortexing, centrifugation, nitrogen blow, and heating, this approach facilitates on-site trace extraction, eliminating the need for sample preservation, transport, and other processes, thus being more environmentally friendly, cost-effective, and green. In this study, we prepared DBSA-doped PPy coating on a polytetrafluoroethylene tube (PFA) through chemical oxidation and extensively characterized it. By adjusting the pyrrole concentration, dopant concentration, oxidant concentration, and the number of coating cycles, we optimized the adsorption performance of the coating to achieve efficient extraction of 19 different nitrogen-containing pesticides in environmental water samples. The results of this study not only provide new insights into the design and application of PPy-based SPME coatings but also offer a new and efficient sample pretreatment strategy for environmental monitoring and food safety analysis.
Materials and methods
Reagents
Pyrrole (99% purity), dodecylbenzene sulfonic acid (90% purity), and ammonium persulfate (98% superior purity) were purchased from Shanghai Aladdin (China). Isopropanol (chromatographic grade), methanol (chromatographic grade), and acetone (chromatographic grade) were purchased from Fisher Scientific (United States). Formic acid (88% analytical purity) and chloric acid (superior purity) were purchased from China National Pharmaceutical Group Chemical Reagent Co., Ltd. (Shanghai, China). Ultrapure water was purchased from Zhejiang Hangzhou Wahaha Co., Ltd. (China).
Nineteen types of nitrogen-containing pesticides, including Imidacloprid (96.0%), Acetamiprid (99.0%), Atrazine (97.0%), Simazine (100.0%), Daimuron (99.0%), Monolinuron (99.0%), Metsulfuron-methyl (98.0%), Pyrazosulfuron-ethyl (99.0%), Alachlor (97.0%), Butachlor (95.0%), Pirimicarb (99.0%), Propamocarb (99.0%), Diflubenzuron (97.0%), Hexaflumuron (99.0%), Triadimefon (99.8%), Fipronil (98.0%), and Fomesafen (99.5%), were purchased from Shanghai Aladdin (China) and Bioline Science & Technology Co., Ltd. Florasulam (99.8%) was purchased from Alta Scientific Co., Ltd., and Penoxsulam (99.0%) was purchased from Shanghai Aladdin Biochemical Technology Co., Ltd.
Standard solutions of 19 nitrogen-containing pesticides (each at a concentration of 100 μg/L) were prepared using HPLC-grade methanol and stored at 4°C. A soluble polytetrafluoroethylene (PFA) column with an inner diameter of 0.75 mm was purchased from Valco Instruments Company Inc. (Houston, United States). Details about its basic properties are provided in Supplementary Table S1.
Instrumentation and conditions
The Shimadzu 30AD/8030 LC-MS/MS system (R < 0.7 FWHM) provided by Shimadzu Corporation (Tokyo, Japan) was equipped with an Agilent POROSHELL 120 EC-C18 liquid chromatography column. Chromatographic conditions were as follows: the column used was an Agilent POROSHELL 120 EC-C18 with dimensions of 2.1 × 50 mm and particle size of 2.7 μm. The injection volume was 2.0 μL, and the flow rate was set at 0.2 mL/min. The mobile phase consisted of 0.1% formic acid in water as phase A and methanol as phase B. A gradient elution program was employed: 0.00–0.50 min, 10% methanol; 0.50–4.50 min, 50% methanol; 4.50–8.50 min, 70% methanol; 8.50–10.05 min, 90% methanol; 10.05–12.00 min, 10% methanol. Mass spectrometry conditions were set as follows: the scan mode was selected as multiple reaction monitoring (MRM), the desolvation line temperature was set at 250°C, the heating temperature was 400°C, the interface voltage was 4.5 kV, the collision-induced dissociation (CID) gas pressure was 230 kPa, the drying gas flow rate was 15.0 L/min, and the nebulizer gas flow rate was 3.0 L/min. Retention times, qualitative ions, collision energy, and other mass spectrometry parameters for the 19 nitrogen-containing pesticides are detailed in Supplementary Table S2. SEM scanning electron microscope was obtained from Carl Zeiss AG (Jena, Germany), model Sigma 500. FTIR Fourier-transform infrared spectroscopy equipment was obtained from Thermo Fisher Scientific (Shanghai, China).
Preparation of PPy-DBSA/IT-SPME column
In this study, we prepared a poly pyrrole (PPy) film on the inner surface of a 0.75 mm inner diameter, 15 cm long PFA capillary using the chemical oxidation polymerization method. The preparation process involved several steps. Firstly, the capillary was cleaned using acetone to remove surface impurities. Next, concentrated perchloric acid was used to etch the inner surface, enhancing the adhesion for subsequent coatings, followed by drying with nitrogen gas. Then, a 0.5 mL solution of pyrrole monomer and the dopant DBSA in isopropanol was injected into the capillary, forming a pre-coat layer. Subsequently, a 0.5 mL solution of ammonium persulfate (APS) oxidizer was injected to initiate the polymerization reaction, resulting in the formation of the PPy film. The polymerization reaction caused a color change on the inner surface of the capillary from yellow to black, indicating the successful formation of PPy. Finally, the polymerized capillary was washed with 2.0 mL of methanol to remove unreacted monomers and by-products and dried with nitrogen gas.
To optimize the preparation conditions of the PPy-DBSA/IT-SPME column, we varied the concentrations of pyrrole and DBSA, the concentration of APS, and the number of coating cycles. The specific experimental conditions were as follows: pyrrole concentrations were set at 1, 3, 5, 7, and 9 mol/L, with a fixed DBSA concentration of 0.05 mol/L, an APS concentration of 0.35 mol/L, and 10 coating cycles. DBSA concentrations were adjusted to 0.007, 0.014, 0.07, 0.14, and 0.35 mol/L while maintaining a pyrrole concentration of 7 mol/L, an APS concentration of 0.35 mol/L, and 10 coating cycles. APS concentrations were 0.10, 0.15, 0.25, 0.35, 0.45, and 0.55 mol/L, with pyrrole and DBSA concentrations at 7 mol/L and 0.014 mol/L, respectively, and 10 coating cycles. The number of coating cycles varied between 4, 6, 8, 10, and 12, while the pyrrole and DBSA concentrations were 7.0 mol/L and 0.014 mol/L, and the APS concentration was 0.35 mol/L.
Solid-phase microextraction process within the column
In this study, a PPy-DBSA/IT-SPME capillary column was employed for the direct extraction of a nitrogen-containing pesticide standard mixture solution with 19 concentration of 100 μg/L. Methanol was used as the desorption solvent, followed by analysis using HPLC-MS. Prior to the extraction process, the PPy-DBSA/IT-SPME column was activated sequentially using 1 mL of methanol and 1 mL of deionized water. Subsequently, a nitrogen-containing pesticide mixed with an aqueous solution of 100 μL was extracted at a flow rate of 1 drop per second through the column and transferred into a new sample vial. This extraction and transfer process was repeated five times, which constituted one cycle. After completing five cycles, the liquid inside the column was collected in the sample vial for further analysis. Then, a wash step was performed by eluting five times with 100 μL of methanol, with the eluate also collected in the same sample vial. The obtained samples were directly analyzed using the Shimadzu 30AD/8030 LC-MS/MS system. After the analysis, the PPy-DBSA/IT-SPME column was washed with 2.0 mL of methanol and dried with nitrogen gas for subsequent use.
Results and discussion
Characterization of PPy-DBSA/IT-SPME coating
The structure of the polythiophene (PPy) coating doped with dodecylbenzene sulfonic acid (DBSA) was extensively characterized using Fourier-transform infrared spectroscopy (FT-IR) and scanning electron microscopy (SEM). The study also investigated the effect of different preparation conditions on the adsorption properties of the coating.
FT-IR spectroscopy analysis (Figure 1) revealed absorption peaks near 1540 cm−1 and 1450 cm−1, corresponding to the asymmetric and symmetric stretching vibrations of the PPy rings, respectively. This suggests that the structure of the PPy rings remains unchanged during the DBSA doping process. Peaks at 1260 cm−1 (C-N absorption), 2,922 cm−1 (DBSA aliphatic C-H symmetric stretching vibration), and 1038 cm−1 (-SO3− group absorption) indicate the successful introduction of the sulfonic acid group into the polymer framework. The presence of S-O and C-S stretching vibration peaks in the 700–500 cm−1 range further confirms the introduction of the sulfonic acid group. Moreover, the absence of the N-H vibration peak of the pyrrole ring at 3,400 cm−1 in the spectrum indicates that changes in experimental conditions do not affect the composition of the polymer chains. The absence of the N-H vibration peak at 3,400 cm−1 suggests that when DBSA is bound to the main chain of PPy, the doping position is at the N atom of the main chain. The relatively stable position of functional groups in the polymer chain is further supported by the insignificant effect of changes in experimental conditions on the peak positions observed in the FT-IR spectrum.
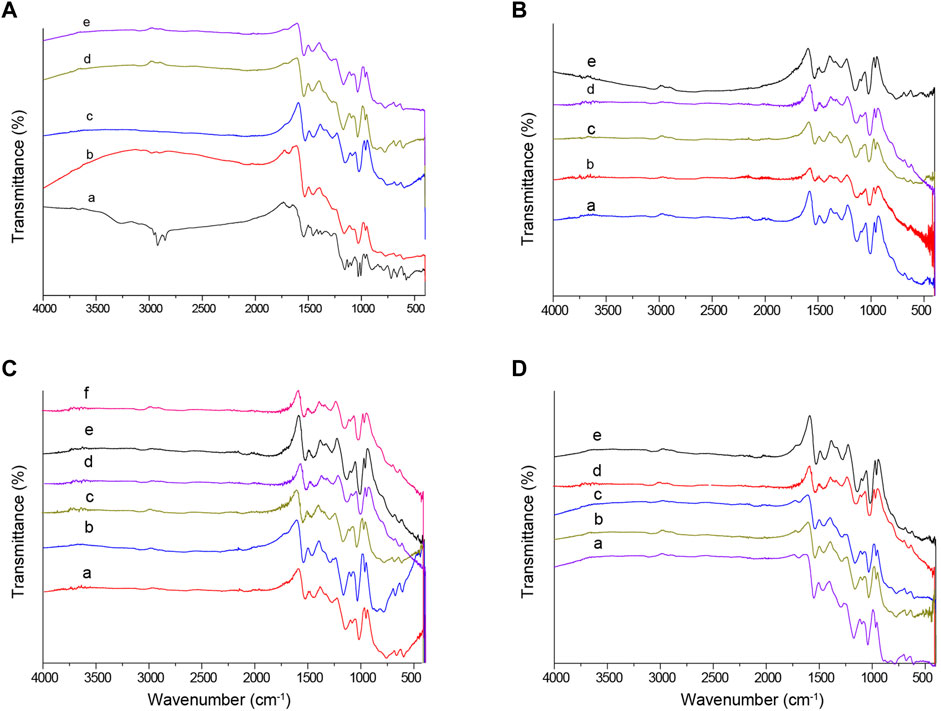
Figure 1. Infrared spectra of PPy-DBSA under different experimental conditions. Note: (A) Pyrrole concentration (a. 1.0 M, b. 3.0 M, c. 5.0 M, d. 7.0 M, e. 9.0 M); (B) DBSA concentration (a. 0.007, b. 0.014, c. 0.07, d. 0.14, e. 0.35); (C) APS concentration (a. 0.10 M, b. 0.15 M, c. 0.25 M, d. 0.35 M, e. 0.45 M, f. 0.55 M); (D) Coating cycles (a. 4, b. 6, c. 8, d. 10, e. 12).
Next, we characterized the morphology of PPy prepared by chemical oxidative polymerization. DBSA, as a doping ion, follows the protonic acid doping mechanism during the polymerization process. The nitrogen atoms in the polythiophene chain acquire positive charges in an acidic environment, and to maintain charge neutrality, DBSA− ions dissolved in the solution, with larger size or electron clouds, have the opportunity to adsorb onto the polymer surface and embed into its matrix by electrostatic interaction. Firstly, the effect of pyrrole concentration on the thickness and surface morphology of the PPy coating was investigated. With increasing pyrrole concentration, the coating thickness decreased from 300 nm to 170 nm, and the surface transformed from smooth to porous, which significantly improved the adsorption capability of the coating. Particularly at a pyrrole concentration of 7.0 mol/L, the coating exhibited the optimal adsorption performance for 19 different pesticides (Figure 2A). Subsequently, the effect of DBSA concentration on the particle size and surface morphology of PPy was elucidated. At DBSA concentration of less than 0.014 mol/L, PPy particles showed smaller average diameters (100–130 nm) and regular shapes, which contributed to the formation of porous coating structures, thereby enhancing adsorption capability (Figure 2B). However, an increase in DBSA concentration led to the disorderly deposition of particles due to hydrophobic interactions, reducing the effective surface area and resulting in decreased adsorption capability (Figure 2B). Furthermore, the influence of oxidant concentration on the thickness and adsorption performance of the PPy coating was explored. Low oxidant concentration (0.1 mol/L) resulted in thin coatings that made it difficult to form stable films. As the oxidant concentration increased, the coating thickness and particle abundance increased, leading to improved adsorption capability. However, at excessively high oxidant concentrations (0.35 mol/L), further increase in coating thickness did not enhance adsorption capability and may hinder the interaction between the analyte and the coating (Figure 2C). Finally, the impact of coating cycles on the thickness and adsorption performance of the coating was considered. The thickness of the coating increased with the number of coating cycles, but at 10 cycles, the thickness decreased to approximately 170 nm, which resulted in the optimal adsorption capacity for nitrogen-containing pesticides (Figure 2D). This indicates that an appropriate thickness and porous structure of the coating contribute to enhanced adsorption performance.
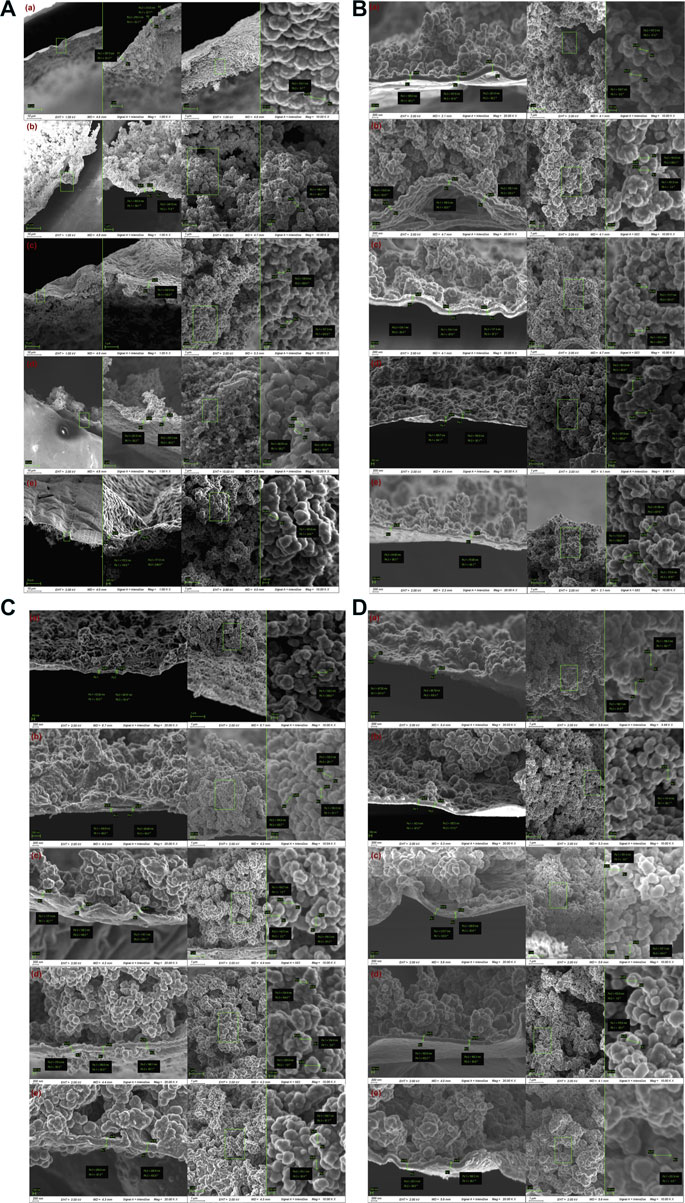
Figure 2. SEM images of PPy-DBSA coating under different pyrrole concentrations. Note: (A) Effect of pyrrole concentration on the surface morphology of PPy coating. Pyrrole concentrations: a. 1.0 M, b. 3.0 M, c. 5.0 M, d. 7.0 M, e. 9.0 M. (B) Effect of DBSA concentration on the particle size and surface morphology of PPy coating. DBSA concentrations: a. 0.007 M, b. 0.014 M, c. 0.07 M, d. 0.14 M, e. 0.35 M. (C) Effect of oxidant concentration on the coating thickness and adsorption performance of PPy. Oxdidant concentrations: a. 0.15 M, b. 0.25 M, c. 0.35 M, d. 0.45 M, e. 0.55 M. (D) Effect of coating cycles on the coating thickness and adsorption performance. Coating cycles: a. 4 times, b. 6 times, c. 8 times, d. 10 times, e. 12 times.
In summary, by fine-tuning the structure of the PPy-DBSA coating, we successfully prepared a solid-phase microextraction coating with excellent adsorption capabilities. The coating, with a thickness range of 150–200 nm and an average particle size of approximately 100–130 nm, exhibits a regular particle morphology and provides abundant effective adsorption sites for polar nitrogen-containing pesticides. This is attributed to the role of the coating thickness as the substrate carrying the surface structure that truly functions. The rich three-dimensional structure formed by the well-ordered deposition of PPy particles on the substrate surface is a key factor influencing the adsorption capability of the coating. The porous surface and non-adhesive nature of the PPy particles increase the effective surface area and provide more adsorption sites, resulting in improved adsorption capability.
Effect of polypyrrole concentration on pesticide adsorption
In order to investigate the impact of preparation conditions on the extraction efficiency of the coating, a total of 19 nitrogen-containing pesticides were selected for the experiment. These included nicotine derivatives, triazines, sulfonylureas, triazoles, diphenyl ethers, substituted ureas, benzoic ureas, aminomethyl esters, amides, triazoles, and phenylpyrazoles. The performance of the PPy-dodecylbenzene sulfonic acid (PPy-DBSA) coating with integrated IT-SPME was evaluated based on the unadsorbed rate and desorption rate of the 19 polar nitrogen-containing pesticides. A lower unadsorbed rate indicated stronger adsorption capacity of the coating, and a combined desorption rate and unadsorbed rate closer to 100.0% indicated better coating performance. Using these criteria, the influence of PPy concentration on the adsorption capacity of the coating was investigated.
The concentration of PPy is a key factor affecting the extraction efficiency. On the one hand, increasing the PPy concentration could lead to an increase in the degree of polymerization and thickness of the coating, providing more adsorption sites and enhanced loading capacity, which is consistent with our electron microscopy characterization results. On the other hand, an increase in PPy concentration allows for a higher density of π electrons and N-H bonds, resulting in more π-π and hydrogen bonding interactions, thereby achieving greater adsorption activity. However, when the PPy concentration is below 1.0 mol/L, the coating becomes too thin to form an effective layer. When the concentration exceeds 9.0 mol/L, it can lead to column blockage. Therefore, the coating can only function within a certain range of PPy concentrations. The results of the study demonstrated that as the PPy concentration increased from 1.0 mol/L to 7.0 mol/L, the unadsorbed rate of the PPy-DBSA/IT-SPME coating for 16 nitrogen-containing pesticides, excluding Alachlor, Pirimicarb, and Propamocarb, significantly decreased. When the PPy concentration reached 7.0 mol/L, the PPy-DBSA/IT-SPME coating exhibited the highest adsorption capacity for most of the pesticides (Figure 3). Therefore, a concentration of 7.0 mol/L was selected as the optimal concentration for all subsequent experiments.
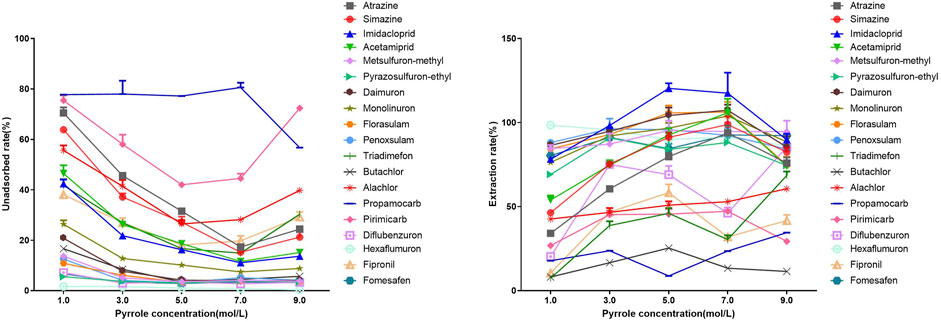
Figure 3. Influence of pyrrole concentration on the adsorption of polar nitrogen-containing pesticides by PPy-DBSA/IT-SPME coating. Note: DBSA concentration: 0.050 mol/L, APS concentration: 0.35 mol/L, coating cycles: 10.
DBSA concentration and adsorption capacity
The following investigation examines the influence of DBSA concentration on the adsorption capacity of the coating. It has been reported in the literature that the dopant DBSA plays a crucial role in the adsorption process. On the one hand, this could be attributed to the long alkyl chains of DBSA acting as flexible spacers between PPy chains, preventing the polymeric chains from tightly stacking together. This promotes the formation of a looser, more porous structure in the polypyrrole, leading to an increased effective specific surface area. This finding is in line with the SEM results obtained. On the other hand, the benzene rings and long alkyl chains in DBSA molecules act as active binding sites, allowing for multiple types of interactions with target analytes. The results indicated that the coating exhibited strong interactions with Metsulfuron-methyl, Pyrazosulfuron-ethyl, Florasulam, Penoxsulam, Fomesafen, Daimuron, Diflubenzuron, and Hexaflumuron, while being minimally affected by increasing concentrations of DBSA. The coating demonstrated an increased non-adsorption rate for Atrazine, Simazine, Imidacloprid, Acetamiprid, and Monolinuron with increasing DBSA concentration. Conversely, the non-adsorption rate of Propamocarb, Pirimicarb, Alachlor, Butachlor, Triadimefon, and Fipronil decreased with increasing DBSA concentration, suggesting a strong interaction between these pesticides and DBSA. Furthermore, the varying molecular structures of different pesticides also influenced their interaction with DBSA, highlighting the potential of PPy-DBSA coatings for extracting pesticides with diverse molecular structures (Figure 4). Therefore, a DBSA concentration of 0.14 mol/L was determined as the optimal condition for extracting Propamocarb, Pirimicarb, Alachlor, Butachlor, Triadimefon, and Fipronil. In order to ensure the extraction efficiency of the coating for most polar nitrogen-containing pesticides, a DBSA concentration of 0.014 mol/L was selected as the optimal condition for all subsequent experiments.
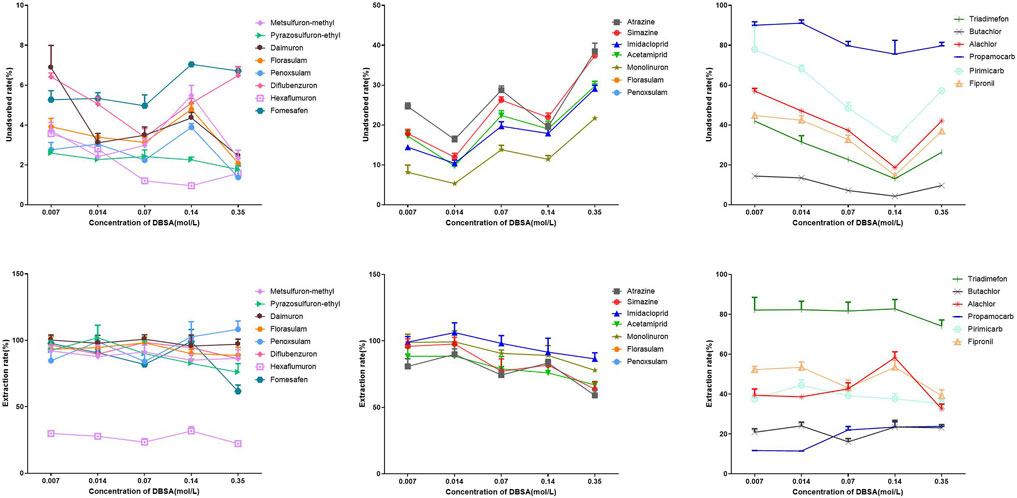
Figure 4. Influence of DBSA concentration on the adsorption of polar nitrogen-containing pesticides by PPy-DBSA/IT-SPME coating. Note: Pyrrole concentration: 7.0 mol/L, APS concentration: 0.35 mol/L, coating cycles: 10.
Oxidant concentration and adsorption performance
It is well-known that the concentration of oxidants affects the rate and extent of pyrrole polymerization, with higher oxidant concentrations resulting in higher polymer yields. Therefore, we explored the influence of oxidant concentration on the adsorption capacity of the coatings. Figure 5 illustrates the impact of oxidant concentration on the adsorption of 19 pesticides. As the APS concentration increased from 1.0 mol/L to 0.35 mol/L, the unadsorbed rate of the coatings towards the 19 pesticides gradually decreased. Electron microscopy characterization revealed that with the increase in oxidant concentration, the reaction became more intense and thorough, leading to a significant accumulation of PPy particles on the surface of the coatings and thus increasing the effective specific surface area, consequently enhancing the adsorption capacity. However, further increasing the oxidant concentration did not show a substantial improvement in the adsorption capacity of the PPy-DBSA/IT-SPME coatings; in fact, it resulted in the waste of reagents due to excessive oxidation. Moreover, the influence of varying the oxidant concentration on the adsorption capacity of the 19 pesticides exhibited similar patterns. Therefore, the correlation between oxidant concentration and the adsorption selectivity of the coatings towards the 19 pesticides was relatively weak. Apart from the fungicide Propamocarb, the remaining 18 nitrogen-containing pesticides achieved desirable adsorption capabilities at an oxidant concentration of 0.35 mol/L. Hence, for all subsequent experiments, we selected 0.35 mol/L as the optimal oxidant concentration.
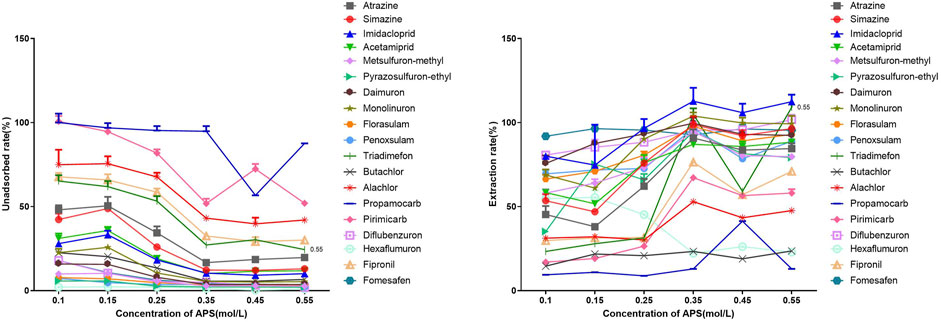
Figure 5. Influence of APS concentration on the adsorption of polar nitrogen-containing pesticides by PPy-DBSA/IT-SPME coating. Note: Pyrrole concentration: 7.0 mol/L, DBSA concentration: 0.014 mol/L, coating cycles: 10.
Effect of coating cycles on adsorption
The characterization results obtained from electron microscopy revealed that increasing the number of coating cycles leads to an increase in the thickness of the PPy coating, which significantly affects its extraction performance. This study analyzed the impact of the number of coating cycles on the coating’s adsorption capacity. Therefore, the efficiency of the coating in extracting 19 different pesticides was investigated by varying the number of coating cycles from 4 to 12 (Figure 6). The results demonstrated that as the number of coating cycles increased from 4 to 10, the unadsorbed fraction of 18 nitrogen-containing pesticides, except for Propamocarb, gradually decreased, indicating an increase in extraction efficiency. This may be attributed to the enhanced loading capacity of the thicker coating, enabling it to adsorb more target compounds, especially those present at low concentrations. Inadequate coating thickness can restrict extraction efficiency. However, when the number of coating cycles was 8 and 12, the unadsorbed fraction of the 19 pesticides was unexpectedly high. Infrared characterization revealed that coatings with 8 and 12 coating cycles had a thickness of approximately 200 nm. These thicker coatings not only increased the likelihood of column clogging but also possibly covered the internal adsorption sites, resulting in a decrease in adsorption capacity. Therefore, only within a certain range of coating cycles can an increase in the number of cycles allow for the adsorption of more target compounds. Additionally, the impact of changing the number of coating cycles on the adsorption capacity of the 19 pesticides behaved similarly, indicating a weak correlation between the number of coating cycles and the coating’s selectivity for the 19 pesticides. To ensure a robust and durable coating while achieving optimal adsorption efficiency, a coating cycle of 10 was selected as the optimal preparation condition for all subsequent experiments.
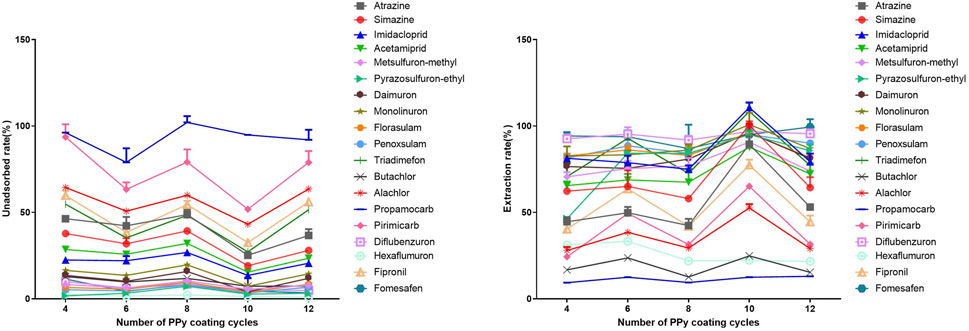
Figure 6. Influence of PPy coating cycles on the adsorption of polar nitrogen-containing pesticides by PPy-DBSA/IT-SPME coating. Note: Pyrrole concentration: 7.0 mol/L, DBSA concentration: 0.014 mol/L, APS concentration: 0.35 mol/L.
Validation results of PPY-SDBA/IT-SPME-HPLC-MS/MS method
Subsequently, under optimized working conditions (pyrrole concentration of 7.0 mol/L, DBSA concentration of 0.014 mol/L, oxidant concentration of 0.35 mol/L, and a coating cycle of 10), we validated the effectiveness of the method through a series of experiments including linearity, limit of detection (LOD), limit of quantification (LOQ), precision, and method repeatability. Figure 7 displays chromatograms of 19 compounds under the optimal conditions, showing symmetric peak shapes. Additionally, all compound detections were completed within 12 min. The linearity of the developed method was tested in the range of 0.01–1.00 mg/L, with three replicates at each concentration. A standard curve was obtained by plotting the average peak area against sample concentration, as shown in Supplementary Table S3. The linear dynamic range for compounds such as Atrazine, Simazine, Imidacloprid, Acetamiprid, Metsulfuron-methyl, Pyrazosulfuron-ethyl, Daimuron, Monolinuron, Florasulam, Penoxsulam, Triadimefon, Butachlor, Alachlor, Propamocarb, Pirimicarb, Diflubenzuron, Hexaflumuron, Fipronil, Fomesafen ranged from 0.01 to 1.00 mg/L. All linear dynamic ranges exhibited excellent linearity (R2 > 0.999). The limits of detection (LODs, S/N = 3) and limits of quantification (LOQs, S/N = 10) were calculated based on the signal-to-noise ratio of a standard solution with a concentration of 0.01 mg/L. The LODs ranged from 0.012 to 0.524 μg/L, while the LOQs ranged from 0.127 to 5.243 μg/L. Furthermore, in blank water samples, 10 μg/L, 100.0 μg/L, and 500.0 μg/L concentrations of nitrogen-containing pesticide compounds were added. These samples were subjected to analysis under the optimized instrument and preparation conditions to determine the recovery rates of the 19 nitrogen-containing pesticide compounds in water. Five parallel experiments were conducted at each added concentration level. The results, listed in Supplementary Table S4, indicate that the method yielded recovery rates between 61.92% and 115.21% for the 19 nitrogen-containing pesticide compounds, with an RSD of less than 5.0%. This demonstrates the method’s strong applicability, sensitivity, and good recovery rates. Supplementary Figures S1, S2 represent chromatograms of the blank solution and water samples with 100.0 μg/L concentrations of the 19 nitrogen-containing pesticides, respectively.
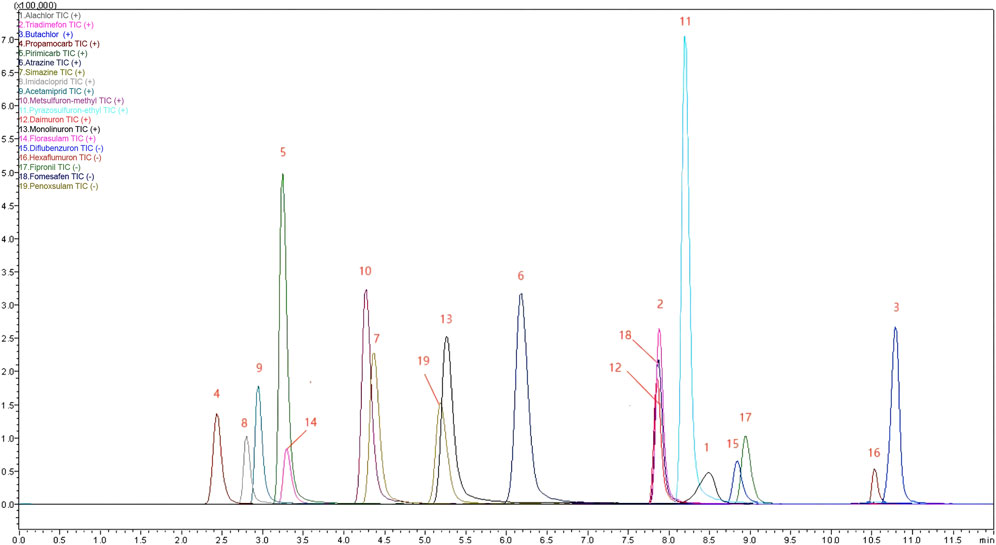
Figure 7. Chromatograms of 19 Pesticides. Note: (1) Alachlor, concentration 0.10 mg/L; (2) Triadimefon, concentration 0.05 mg/L; (3) Butachlor, concentration 0.10 mg/L; (4) Propamocarb, concentration 0.02 mg/L; (5) Pirimicarb, concentration 0.05 mg/L; (6) Atrazine, concentration 0.05 mg/L; (7) Simazine, concentration 0.10 mg/L; (8) Diflubenzuron, concentration 0.20 mg/L; (9) Acetamiprid, concentration 0.20 mg/L; (10) Metsulfuron-methyl, concentration 0.50 mg/L; (11) Pyrazosulfuron-ethyl, concentration 0.50 mg/L; (12) Daimuron, concentration 0.02 mg/L; (13) Monolinuron, concentration 0.50 mg/L; (14) Florasulam, concentration 0.10 mg/L; (15) Diflubenzuron, concentration 0.50 mg/L; (16) Hexaflumuron, concentration 0.10 mg/L; (17) Fipronil, concentration 0.50 mg/L; (18) Fomesafen, concentration 2.0 mg/L; (19) Penoxsulam, concentration 5.0 mg/L. Conditions: flow rate of 0.2 mL/min, injection volume of 2.0 μL, column temperature at 40°C, mobile phase composed of 0.1% formic acid in water (solvent A) and methanol (solvent B).
Comparison of analytical methods for detecting multiple pesticide residues in environmental water samples
To highlight the advantages of the PPy-SDBA/IT-SPME combined with HPLC-MS/MS method, we compared our proposed approach with other methods used for analyzing multiple pesticide residues in environmental water samples, as outlined in Supplementary Table S5. The comparative analysis reveals that solid-phase extraction (WANG Liang, 2009; Xin-Yan et al., 2015; Ya-Na et al., 2020) generally requires a substantial volume, large volumes of solvents, extended processing times, and specialized equipment; in contrast, the ionic liquid solvent flotation method (WANG Liang, 2009), though offering smaller sample volumes, involves significant solvent usage and extended processing times. The molecular imprinting method (Guo-Long et al., 2020), while requiring minimal solvent usage, involves larger sample volumes and prolonged material preparation times. Magnetic solid-phase extraction (Ming et al., 2016) entails large sample solution volume yet utilizes comparatively less solvent and shorter processing times. On the other hand, dispersive liquid-liquid extraction (Sereshti et al., 2020) offers quick material preparation times, minimal sample and solvent volumes, with our method requiring merely 0.1 mL sample volume and reduced material preparation and extraction process time. Additionally, our method employs a PPy-DBSA extraction length of 15 cm with an inner diameter of 0.75 mm, making it a compact and portable extraction device. Compared to extraction methods involving techniques like vortexing, centrifugation, nitrogen blow, and heating, our method facilitates on-site trace extraction, eliminating the need for sample preservation, transportation, thus presenting a more environmentally friendly and cost-effective solution.
In summary, the PPy-DBSA/IT-SPME coating prepared under optimal conditions demonstrates excellent adsorption efficacy for 19 polar nitrogen-containing pesticides. The coating is effective at adsorbing sulfonylureas, triazolopyrimidines, diphenyl ether herbicides, benzoylurea insecticides, and phenylurea herbicides, with unadsorbed rates below 10.0% (Li Y. et al., 2020; Silva et al., 2020). For neonicotinoid and triazine pesticides, the minimum unadsorbed rates range from 11.9% to 16.4%, with Alachlor at 18.6%, Butachlor at 4.3%, Triadimefon at 13.0%, and Fipronil at 14.7%. The experimental approach combining PPy-SDBA/IT-SPME with HPLC-MS/MS has introduced a green, efficient, simple, and cost-effective analytical method suitable for detecting polar nitrogen-containing pesticide residues in water matrices. This method achieves average recovery rates for the 19 polar nitrogen-containing pesticides ranging from 61.92% to 115.21%, with RSDs below 5.0%. The detection limits (LODs, S/N = 3) and quantification limits (LOQs, S/N = 10) range from 0.012 to 0.524 μg/L and 0.127–5.243 μg/L, respectively, resulting in satisfactory recovery rates. Furthermore, this method requires minimal sample and organic solvent usage, is portable, simple to operate, and time-efficient, offering a convenient and highly effective means of extracting polar nitrogen-containing pesticide residues from water.
This study successfully developed a novel solid-phase microextraction (SPME) coating based on polypyrrole (PPy) and dodecylbenzene sulfonic acid (DBSA) to enhance the extraction efficiency of polar nitrogen-containing pesticides in water. In comparison to previous studies, the major innovation of this study lies in the combination of PPy’s excellent electrical conductivity and chemical stability with DBSA’s surfactant properties, resulting in a significant improvement in the coating’s adsorption capacity for polar pesticides. Furthermore, compared to traditional SPME coatings like polydimethylsiloxane (PDMS), the PPy-DBSA coating demonstrates superior performance in terms of selectivity and adsorption capacity. This development of a new coating not only offers new research perspectives in the field of material science but also brings about breakthroughs in environmental monitoring technology (Puckert et al., 2017).
In terms of coating preparation techniques, this study employed a chemical oxidation method to prepare the PPy-DBSA coating. This method demonstrates better coating uniformity and adhesion compared to traditional physical adsorption or chemical bonding techniques (Wu et al., 2020; Xue et al., 2023). The chemical oxidation method effectively controls the growth of polymer chains, resulting in a more uniform and compact coating (Lee et al., 2021; Bui et al., 2022). Additionally, this method helps enhance the chemical stability and durability of the coating, which is particularly crucial for long-term environmental monitoring. Compared to other preparation methods, the chemical oxidation method exhibits significant advantages in terms of simplicity, cost-effectiveness, and environmental friendliness (Arif et al., 2022; Maggini et al., 2022).
In terms of coating performance, the PPy-DBSA/IT-SPME coating demonstrates excellent performance in extracting polar nitrogen-containing pesticides from water samples. This performance enhancement can be mainly attributed to the doping of DBSA, which not only improves the hydrophilicity of the PPy coating but also enhances its adsorption capacity for polar pesticides. In terms of the coating’s microstructure, the PPy-DBSA coating exhibits finer and more uniform particle distribution, which helps increase the contact area between the coating and analytes, thereby improving adsorption efficiency. Compared to traditional SPME coatings, the PPy-DBSA coating shows significant improvements in adsorption rate and selectivity, especially for highly polar pesticides.
In terms of coating optimization conditions, this study systematically investigates the effects of pyrrole concentration, DBSA concentration, oxidant concentration, and coating deposition cycles on coating performance. It is found that pyrrole concentration significantly affects coating formation and performance, with excessively high or low concentrations diminishing the adsorption performance of the coating. The optimization of DBSA concentration aims to balance the hydrophilicity and oil affinity of the coating to ensure efficient adsorption of polar pesticides. Furthermore, the concentration of oxidant and the number of coating deposition cycles are also crucial factors influencing coating performance (Arif et al., 2022; Maggini et al., 2022). The identification of these optimization conditions not only improves the extraction efficiency of the coating but also provides important references for future coating design.
The PPy-DBSA/IT-SPME coating holds broad application prospects in the fields of environmental monitoring and food safety. In terms of environmental monitoring, this novel coating can be used to detect residues of polar nitrogen-containing pesticides in water, aiding in the assessment of water quality safety and pollution levels. In the field of food safety, this coating can be applied to detect pesticide residues in agricultural products, ensuring food quality and consumer health. Due to its efficient extraction performance and simple operational process, the PPy-DBSA/IT-SPME coating significantly enhances the efficiency and accuracy of sample handling, providing important practical value for environmental and food safety monitoring.
Compared to existing SPME technologies and high-performance liquid chromatography-tandem mass spectrometry (HPLC-MS/MS) techniques, the PPy-DBSA/IT-SPME coating demonstrates advantages in various aspects (Fanti et al., 2020; Hu et al., 2022; Liu et al., 2023). Firstly, this coating has lower preparation costs and is easy to operate, making it suitable for large-scale applications (Manousi et al., 2020; Gionfriddo and Gómez-Ríos, 2021; Mollahosseini et al., 2022). Secondly, the PPy-DBSA coating exhibits more efficient and accurate detection of polar nitrogen-containing pesticides than traditional techniques due to its outstanding adsorption performance and specific recognition ability. However, the PPy-DBSA coating may have limitations in dealing with large molecules or non-polar compounds, which require further optimization in future research.
In summary, this study presents the first use of the chemical oxidation method to prepare a DBSA-doped polypyrrole solid-phase microextraction (PPy-DBSA/IT-SPME) coating on the inner wall of a PFA tube (Figure 8). The research found that the concentrations of pyrrole and DBSA, the concentration of the oxidant, and the number of coating cycles all affect the thickness, morphology, and adsorption performance of the coating (Hemmati et al., 2017). Due to its porous structure, high surface area, and multifunctional adsorption characteristics, this adsorbent exhibits excellent extraction and adsorption capabilities for polar nitrogen-containing pesticides in water (Li D. et al., 2020). Specifically, the concentrations of pyrrole and DBSA predominantly influence the adsorption selectivity of the coating for different nitrogen-containing pesticide structures, while the concentrations of the oxidant and the number of coating cycles have relatively minor effects (Harjo et al., 2020). Under the optimum preparation conditions (pyrrole concentration: 7.0 mol/L, DBSA concentration: 0.014 mol/L, oxidant concentration: 0.35 mol/L, coating cycles: 10), the coating shows negligible unadsorbed rates for various pesticide classes (Hemmati et al., 2017; Zhang et al., 2018). Specifically, the unadsorbed rates for sulfonylureas, triazolinones, diphenyl ethers herbicides, benzoylurea insecticides, and phenylurea herbicides are all below 10.0% (Li Y. et al., 2020; Silva et al., 2020). The lowest unadsorbed rates for neonicotinoids and triazines pesticides are 11.9%–16.4%, while Alachlor is 18.6%, Butachlor is 4.3%, Triadimefon and Fipronil are 13.0% and 14.7%, respectively (Ishtiaq et al., 2020; Pachú et al., 2021; Wang et al., 2022). These recovery results are satisfactory.
Therefore, the PPy-DBSA/IT-SPME coating developed in this study demonstrates excellent application prospects for the detection of polar nitrogen-containing pesticides in environmental water samples. The high adsorption capacity and exceptional selectivity of the coating provide an effective new tool for the multi-residue analysis of nitrogen-containing pesticides. The successful development of the PPy-DBSA/IT-SPME column not only expands the application range of polypyrrole in environmental monitoring but also establishes a foundation for the advancement of pesticide residue analysis techniques in the future. Its scientific value lies in providing a novel SPME coating material with efficient extraction capabilities and excellent selectivity, offering a new solution for environmental monitoring and food safety detection. Its clinical value is demonstrated by its ability to effectively monitor polar nitrogen-containing pesticides in environmental water samples, contributing to the evaluation and control of the potential risks of these pesticides to public health.
Despite the excellent performance of the PPy-DBSA/IT-SPME coating, this study still has some limitations. For example, the long-term stability and durability of the coating have not been thoroughly investigated, and the repeatability and reliability of the coating in practical applications need further validation. Additionally, the preparation process of the coating may require meticulous operation and optimization, which could limit its application in large-scale production.
Future research should focus on further optimizing the PPy-DBSA/IT-SPME coating, including improving its stability and repeatability, as well as simplifying the preparation process. Furthermore, the application of this technology to a wider range of sample types and more target analytes should be explored to broaden its scope in environmental monitoring and food safety. In addition, combining this technique with modern analytical technologies such as mass spectrometry can further enhance the sensitivity and accuracy of detection, providing a more powerful tool for environmental and food safety monitoring.
Data availability statement
The raw data supporting the conclusion of this article will be made available by the authors, without undue reservation.
Author contributions
RM: Conceptualization, Supervision, Writing–review and editing. SY: Investigation, Writing–original draft, Writing–review and editing. YfL: Data curation, Visualization, Writing–original draft, Writing–review and editing. YnL: Resources, Validation, Writing–original draft, Writing–review and editing. XM: Conceptualization, Supervision, Writing–review and editing.
Funding
The author(s) declare that financial support was received for the research, authorship, and/or publication of this article. This study was supported by Science and Technology Innovation Program for Higher Education Institutions in Shanxi Province in 2022 (Project No. 2022L381).
Conflict of interest
The authors declare that the research was conducted in the absence of any commercial or financial relationships that could be construed as a potential conflict of interest.
Publisher’s note
All claims expressed in this article are solely those of the authors and do not necessarily represent those of their affiliated organizations, or those of the publisher, the editors and the reviewers. Any product that may be evaluated in this article, or claim that may be made by its manufacturer, is not guaranteed or endorsed by the publisher.
Supplementary material
The Supplementary Material for this article can be found online at: https://www.frontiersin.org/articles/10.3389/fenvs.2024.1350170/full#supplementary-material
SUPPLEMENTARY FIGURE S1 | Chromatogram of the blank solution.
SUPPLEMENTARY FIGURE S2 | Chromatogram representing water samples with added concentrations of 100.0 μg/L of 19 nitrogen-containing pesticides. Note: (A) Chromatogram of the spiked water sample recovery test, with all 19 nitrogen-containing pesticides added at a concentration of 100.0 μg/L. (B) Zoomed-in chromatogram of Penoxsulam.
References
Agatonovic-Kustrin, S., Gegechkori, V., Kobakhidze, T., and Morton, D. (2023). Solid-phase microextraction techniques and application in food and horticultural crops. Mol. 28 (19), 6880. doi:10.3390/molecules28196880
Al Shirity, Z. N., Westra, N., Hateren, K. V., Munnink, T. H. O., Kosterink, J. G. W., Mian, P., et al. (2023). Validation of an LC-MS/MS assay for rapid and simultaneous quantification of 21 kinase inhibitors in human plasma and serum for therapeutic drug monitoring. J. Chromatogr. B, Anal. Technol. Biomed. life Sci. 1229, 123872. doi:10.1016/j.jchromb.2023.123872
Arif, D., Hussain, Z., Abbasi, A. D., and Sohail, M. (2022). Ag functionalized In2O3 derived from MIL-68(in) as an efficient electrochemical glucose sensor. Front. Chem. 10, 906031. doi:10.3389/fchem.2022.906031
Asiabi, H., Yamini, Y., Shamsayei, M., and Mehraban, J. A. (2018). A nanocomposite prepared from a polypyrrole deep eutectic solvent and coated onto the inner surface of a steel capillary for electrochemically controlled microextraction of acidic drugs such as losartan. Mikrochim. acta 185 (3), 169. doi:10.1007/s00604-018-2684-y
Badawy, M. E. I., El-Nouby, M. A. M., Kimani, P. K., Lim, L. W., and Rabea, E. I. (2022). A review of the modern principles and applications of solid-phase extraction techniques in chromatographic analysis. Anal. Sci. Int. J. Jpn. Soc. Anal. Chem. 38 (12), 1457–1487. doi:10.1007/s44211-022-00190-8
Bagheri, A. R., Aramesh, N., and Haddad, P. R. (2022). Applications of covalent organic frameworks and their composites in the extraction of pesticides from different samples. J. Chromatogr. A 1661, 462612. doi:10.1016/j.chroma.2021.462612
Begum, B., Bilal, S., Shah, A. U. H. A., and Röse, P. (2021). Physical, chemical, and electrochemical properties of redox-responsive polybenzopyrrole as electrode material for faradaic energy storage. Polymers 13 (17), 2883. doi:10.3390/polym13172883
Berni, I., Menouni, A., El Ghazi, I., Godderis, L., Duca, R. C., and Jaafari, S. E. (2021). Health and ecological risk assessment based on pesticide monitoring in Saïss plain (Morocco) groundwater. Environ. Pollut. (Barking, Essex 1987) 276, 116638. doi:10.1016/j.envpol.2021.116638
Bui, H. L., Huang, S. D., Lee, B. P., Lan, M. Y., and Huang, C. J. (2022). Catechol-functionalized sulfobetaine polymer for uniform zwitterionization via pH transition approach. Colloids surfaces. B, Biointerfaces 220, 112879. doi:10.1016/j.colsurfb.2022.112879
Chow, R., Scheidegger, R., Doppler, T., Dietzel, A., Fenicia, F., and Stamm, C. (2020). A review of long-term pesticide monitoring studies to assess surface water quality trends. Water Res. X 9, 100064. doi:10.1016/j.wroa.2020.100064
Chu, T., Abbassi, B. E., and Zytner, R. G. (2021). Life-cycle assessment of full-scale membrane bioreactor and tertiary treatment technologies in the fruit processing industry. Water Environ. Res. a Res. Publ. Water Environ. Fed. 94 (1), e1661. Advance online publication. doi:10.1002/wer.1661
Du, H., Zhang, Z., Jiang, X., Wang, J., Yi, W., Li, X., et al. (2023). Enhancement of NO2 gas sensing properties of polypyrrole by polarization doping with DBS: experimental and DFT studies. ACS Appl. Mater. interfaces. Advance online publication. doi:10.1021/acsami.3c12154
Du, J., Zhao, F., and Zeng, B. (2021). Preparation of functionalized graphene and ionic liquid co-doped polypyrrole solid phase microextraction coating for the detection of benzoates preservatives. Talanta 228, 122231. doi:10.1016/j.talanta.2021.122231
Dugheri, S., Mucci, N., Cappelli, G., Trevisani, L., Bonari, A., Bucaletti, E., et al. (2022). Advanced solid-phase microextraction techniques and related automation: a review of commercially available technologies. J. Anal. methods Chem. 2022, 1–15. doi:10.1155/2022/8690569
Fanti, F., Vincenti, F., Montesano, C., Serafini, M., Compagnone, D., and Sergi, M. (2020). dLLME-μSPE extraction coupled to HPLC-ESI-MS/MS for the determination of F2α-IsoPs in human urine. J. Pharm. Biomed. analysis 186, 113302. doi:10.1016/j.jpba.2020.113302
Geng, H., Lupton, E. J., Ma, Y., Sun, R., Grigsby, C. L., Brachi, G., et al. (2023). Hybrid polypyrrole and polydopamine nanosheets for precise Raman/photoacoustic imaging and photothermal therapy. Adv. Healthc. Mater. 12 (27), e2301148. doi:10.1002/adhm.202301148
Gionfriddo, E., and Gómez-Ríos, G. A. (2021). Analysis of food samples made easy by microextraction technologies directly coupled to mass spectrometry. J. mass Spectrom. JMS 56 (1), e4665. doi:10.1002/jms.4665
Gormez, E., Golge, O., González-Curbelo, M. Á., and Kabak, B. (2022). Monitoring and exposure assessment of fosetyl aluminium and other highly polar pesticide residues in sweet cherry. Mol. 28 (1), 252. doi:10.3390/molecules28010252
Guo, Z., Huang, S., Wang, J., and Feng, Y. L. (2020). Recent advances in non-targeted screening analysis using liquid chromatography - high resolution mass spectrometry to explore new biomarkers for human exposure. Talanta 219, 121339. doi:10.1016/j.talanta.2020.121339
Guo-Long, Z., Xiao-Guo, M., and Yin-Ming, F. (2020). Determination of tetracyclines in environmental water samples by high performance liquid chromatography with multi-template molecularly imprinted polymer magnetic solid phase extraction. Instrum. ANA 39 (06), 749–755. doi:10.3969/j.issn.1004-4957.2020.06.008
Hammad, S. F., Abdallah, I. A., Bedair, A., and Mansour, F. R. (2022). Homogeneous liquid-liquid extraction as an alternative sample preparation technique for biomedical analysis. J. Sep. Sci. 45 (1), 185–209. doi:10.1002/jssc.202100452
Harjo, M., Järvekülg, M., Tamm, T., Otero, T. F., and Kiefer, R. (2020). Concept of an artificial muscle design on polypyrrole nanofiber scaffolds. PloS one 15 (5), e0232851. doi:10.1371/journal.pone.0232851
Hemmati, M., Rajabi, M., and Asghari, A. (2017). Ultrasound-promoted dispersive micro solid-phase extraction of trace anti-hypertensive drugs from biological matrices using a sonochemically synthesized conductive polymer nanocomposite. Ultrason. sonochemistry 39, 12–24. doi:10.1016/j.ultsonch.2017.03.024
Hu, C., Zhang, Y., Zhou, Y., Xiang, Y. J., Liu, Z. F., Wang, Z. H., et al. (2022). Tetrodotoxin and its analogues in food: recent updates on sample preparation and analytical methods since 2012. J. Agric. food Chem. 70 (39), 12249–12269. doi:10.1021/acs.jafc.2c04106
Hussain, M., Hasnain, S., Khan, N. A., Bano, S., Zuhra, F., Ali, M., et al. (2021). Design and fabrication of a fast response resistive-type humidity sensor using polypyrrole (ppy) polymer thin film structures. Polymers 13 (18), 3019. doi:10.3390/polym13183019
Ishtiaq, F., Bhatti, H. N., Khan, A., Iqbal, M., and Kausar, A. (2020). Polypyrole, polyaniline and sodium alginate biocomposites and adsorption-desorption efficiency for imidacloprid insecticide. Int. J. Biol. Macromol. 147, 217–232. doi:10.1016/j.ijbiomac.2020.01.022
Jain, A., Nabeel, A. N., Bhagwat, S., Kumar, R., Sharma, S., Kozak, D., et al. (2023). Fabrication of polypyrrole gas sensor for detection of NH3 using an oxidizing agent and pyrrole combinations: studies and characterizations. Heliyon 9 (7), e17611. doi:10.1016/j.heliyon.2023.e17611
Ju, Y., Hu, Y., Yang, P., Xie, X., and Fang, B. (2022). Extracellular vesicle-loaded hydrogels for tissue repair and regeneration. Mater. today Bio 18, 100522. doi:10.1016/j.mtbio.2022.100522
Kataoka, H. (2021). In-tube solid-phase microextraction: current trends and future perspectives. J. Chromatogr. A 1636, 461787. doi:10.1016/j.chroma.2020.461787
Ke, Q., Liu, Y., Xiang, R., Zhang, Y., Du, M., Li, Z., et al. (2022). Nitrogen-doped porous core-sheath graphene fiber-shaped supercapacitors. Polymers 14 (20), 4300. doi:10.3390/polym14204300
Khalil, A. M. (2020). The genome editing revolution: review. J. Genet. Eng. Biotechnol. 18 (1), 68. doi:10.1186/s43141-020-00078-y
Khan, M. I., Almesfer, M. K., Elkhaleefa, A., Shigidi, I., Shamim, M. Z., Ali, I. H., et al. (2021). Conductive polymers and their nanocomposites as adsorbents in environmental applications. Polymers 13 (21), 3810. doi:10.3390/polym13213810
Kim, U. J., and Karthikraj, R. (2021). Solid-phase microextraction for the human biomonitoring of environmental chemicals: current applications and future perspectives. J. Sep. Sci. 44 (1), 247–273. doi:10.1002/jssc.202000830
Lasarte-Aragonés, G., Lucena, R., and Cárdenas, S. (2020). Effervescence-assisted microextraction-one decade of developments. Mol. 25 (24), 6053. doi:10.3390/molecules25246053
Lee, J. T., Wyatt, B. C., Davis, G. A., Masterson, A. N., Pagan, A. L., Shah, A., et al. (2021). Covalent surface modification of Ti3C2Tx MXene with chemically active polymeric ligands producing highly conductive and ordered microstructure films. ACS Nano 15 (12), 19600–19612. doi:10.1021/acsnano.1c06670
Lemos, V. A., Barreto, J. A., Santos, L. B., de Assis, R. D. S., Novaes, C. G., and Cassella, R. J. (2022). In-syringe dispersive liquid-liquid microextraction. Talanta 238 (Pt 1), 123002. doi:10.1016/j.talanta.2021.123002
Li, D., Fang, Y., and Zhang, X. (2020b). Bacterial detection and elimination using a dual-functional porphyrin-based porous organic polymer with peroxidase-like and high near-infrared-light-enhanced antibacterial activity. ACS Appl. Mater. interfaces 12 (8), 8989–8999. doi:10.1021/acsami.9b20102
Li, Y., Zhang, Q., Yu, Y., Li, X., and Tan, H. (2020a). Integrated proteomics, metabolomics and physiological analyses for dissecting the toxic effects of halosulfuron-methyl on soybean seedlings (Glycine max merr.). Plant physiology Biochem. PPB 157, 303–315. doi:10.1016/j.plaphy.2020.10.033
Liu, J., Li, G., Liu, J., Wang, P., Wu, D., Zhang, X., et al. (2023). Recent progress on toxicity and detection methods of polychlorinated biphenyls in environment and foodstuffs. Crit. Rev. Anal. Chem. 53 (4), 928–953. doi:10.1080/10408347.2021.1997570
Ma, M., Wei, Y., Wei, H., Liu, X., and Liu, H. (2021). High-efficiency solid-phase microextraction performance of polypyrrole enhanced titania nanoparticles for sensitive determination of polar chlorophenols and triclosan in environmental water samples. RSC Adv. 11 (46), 28632–28642. doi:10.1039/d1ra04405b
Maggini, V., Calvi, L., Pelagatti, T., Gallo, E. R., Civati, C., Privitera, C., et al. (2022). An optimized terpene profile for a new medical cannabis oil. Pharmaceutics 14 (2), 298. doi:10.3390/pharmaceutics14020298
Mahato, N., Jang, H., Dhyani, A., and Cho, S. (2020). Recent progress in conducting polymers for hydrogen storage and fuel cell applications. Polymers 12 (11), 2480. doi:10.3390/polym12112480
Manousi, N., Tzanavaras, P. D., and Zacharis, C. K. (2020). Bioanalytical hplc applications of in-tube solid phase microextraction: a two-decade overview. Mol. 25 (9), 2096. doi:10.3390/molecules25092096
Ming, G., Feng, Y., Ke-Ling, J., Jiao, L., and Hai, S. (2016). Determination of tetracycline antibiotics in environmental water using magnetic solid phase extraction combined with high performance liquid chromatography-tandem mass spectrometry. Chin. J. Chromatogr. 34 (04), 407–413. doi:10.3724/SP.J.1123.2015.11038
Mollahosseini, A., Rastegari, M., and Panahi-Dehghan, M. (2022). Electrospun polyacrylonitrile/clinoptilolite coating for SPME of PAHs from water samples. J. Chromatogr. Sci. 60 (4), 401–407. doi:10.1093/chromsci/bmab082
Moreno, C., Wykes, T., Galderisi, S., Nordentoft, M., Crossley, N., Jones, N., et al. (2020). How mental health care should change as a consequence of the COVID-19 pandemic. lancet. Psychiatry 7 (9), 813–824. doi:10.1016/S2215-0366(20)30307-2
Özuğur Uysal, B., Nayır, Ş., Açba, M., Çıtır, B., Durmaz, S., Koçoğlu, Ş., et al. (2022). 2D Materials (WS2, MoS2, MoSe2) Enhanced Polyacrylamide Gels for Multifunctional Applications. Gels 8 (8), 465. doi:10.3390/gels8080465
Pachú, J. K. S., Macedo, F. C. O., da Silva, F. B., Malaquias, J. B., Ramalho, F. S., Oliveira, R. F., et al. (2021). Imidacloprid-mediated stress on non-Bt and Bt cotton, aphid and ladybug interaction: approaches based on insect behaviour, fluorescence, dark respiration and plant electrophysiology. Chemosphere 263, 127561. doi:10.1016/j.chemosphere.2020.127561
Pal, C. A., Choi, J. S., Angaru, G. K. R., Lingamdinne, L. P., Choi, Y. L., Koduru, J. R., et al. (2023). Efficiency of Ppy-PA-pani and Ppy-PA composite adsorbents in Chromium(VI) removal from aqueous solution. Chemosphere 337, 139323. doi:10.1016/j.chemosphere.2023.139323
Peng, L. Q., and Cao, J. (2021). Modern microextraction techniques for natural products. Electrophoresis 42 (3), 219–232. doi:10.1002/elps.202000248
Puckert, C., Tomaskovic-Crook, E., Gambhir, S., Wallace, G. G., Crook, J. M., and Higgins, M. J. (2017). Electro-mechano responsive properties of gelatin methacrylate (GelMA) hydrogel on conducting polymer electrodes quantified using atomic force microscopy. Soft matter 13 (27), 4761–4772. doi:10.1039/c7sm00335h
Saini, R. K., Prasad, P., Shang, X., and Keum, Y. S. (2021). Advances in lipid extraction methods-A review. Int. J. Mol. Sci. 22 (24), 13643. doi:10.3390/ijms222413643
Sereshti, H., Jazani, S. S., Nouri, N., and Shams, G. (2020). Dispersive liquid-liquid microextraction based on hydrophobic deep eutectic solvents: application for tetracyclines monitoring in milk. Microchem. J., 158. doi:10.1016/j.microc.2020.105269
Silva, F. B., Costa, A. C., Müller, C., Nascimento, K. T., Batista, P. F., Vital, R. G., et al. (2020). Dipteryx alata, a tree native to the Brazilian Cerrado, is sensitive to the herbicide nicosulfuron. Ecotoxicol. Lond. Engl. 29 (2), 217–225. doi:10.1007/s10646-019-02154-7
Sun, M., Li, C., Sun, M., Feng, Y., Feng, J., Sun, H., et al. (2022). Preparation and application of graphene oxide functionalized melamine-formaldehyde aerogel coated solid-phase microextraction tube. J. Chromatogr. 40 (10), 889–899. doi:10.3724/SP.J.1123.2021.12032
Wang, J., Zhang, D., Xu, K., Hui, N., and Wang, D. (2022). Electrochemical assay of acetamiprid in vegetables based on nitrogen-doped graphene/polypyrrole nanocomposites. Mikrochim. acta 189 (10), 395. doi:10.1007/s00604-022-05490-4
Wang, X., Huang, P., Ma, X., Du, X., and Lu, X. (2019). Enhanced in-out-tube solid-phase microextraction by molecularly imprinted polymers-coated capillary followed by HPLC for Endocrine Disrupting Chemicals analysis. Talanta 194, 7–13. doi:10.1016/j.talanta.2018.10.027
Wang Liang, Y. y.-S., Wen-Shuai, Z. H. U., and Hua-Ming, L. I. (2009). Chin. J. Anal. Chem. 37 (01), 72–76.
Wu, K., Ye, Q., Wu, R., Chen, S., and Dai, H. (2020). Carbon dioxide adsorption behaviors of aluminum-pillared montmorillonite-supported alkaline earth metals. J. Environ. Sci. (China) 98, 109–117. doi:10.1016/j.jes.2020.05.025
Wu, S., Dong, T., Li, Y., Sun, M., Qi, Y., Liu, J., et al. (2022). State-of-the-art review of advanced electrospun nanofiber yarn-based textiles for biomedical applications. Appl. Mater. today 27, 101473. doi:10.1016/j.apmt.2022.101473
Xin-Yan, G., Na, W., Li-Jun, H., Yong, Z., Jing, X., Jiu-Chang, W., et al. (2015). Simultaneous detection of 25 kinds of veterinary antibiotics in soil, manure and water samples using liquid chromatography-tandem mass spectrometry. Chin. J. Anal. Chem. 43 (01), 13–20. doi:10.11895/j.issn.0253-3820.140546
Xue, Q., Wu, Y., Hao, J., Ma, L., Dang, Y., Zhu, J. J., et al. (2023). NiO-NiMoO4 nanocomposites on multi-walled carbon nanotubes as efficient bifunctional electrocatalysts for total water splitting. ACS Appl. Mater. interfaces 15 (26), 31470–31477. doi:10.1021/acsami.3c04714
Ya-Na, W., Jie, P., Shuang, X., Zhu, R., Nan, Z., Xin-Mei, H., et al. (2020). Determination of 40 antibiotics in surface water by solid phase extraction-high performance liquid chromatography-tandem mass spectrometry. Environ. Chem. 39 (01), 188–196. doi:10.7524/j.issn.0254-6108.2019021304
Yin, Z., Lu, B., Chen, Y., and Guo, C. (2022). Advances of commercial and biological materials for electron transport layers in biological applications. Front. Bioeng. Biotechnol. 10, 900269. doi:10.3389/fbioe.2022.900269
Yu, G., Wang, C. Y., Dong, W., Tian, Y. W., Wang, Z., Lu, J., et al. (2024). Anion-doped polypyrrole three-dimensional framework enables adsorption and conversion in lithium-sulfur batteries. J. colloid interface Sci. 654 (Pt A), 201–211. doi:10.1016/j.jcis.2023.10.033
Yuan, T. F., Le, J., Wang, S. T., and Li, Y. (2020). An LC/MS/MS method for analyzing the steroid metabolome with high accuracy and from small serum samples. J. lipid Res. 61 (4), 580–586. doi:10.1194/jlr.D119000591
Zhang, H., Gu, Q., Wallace, G. G., and Higgins, M. J. (2018). Effect of electrochemical oxidation and reduction on cell de-adhesion at the conducting polymer-live cell interface as revealed by single cell force spectroscopy. Biointerphases 13 (4), 041004. doi:10.1116/1.5022713
Keywords: in-tube solid-phase microextraction, polypyrrole, dodecylbenzene sulfonic acid, nitrogen-containing pesticides, environmental monitoring, coating optimization
Citation: Ma R, Yu S, Li Y, Lin Y and Ma X (2024) Enhancing the efficiency of polypyrrole-dodecylbenzene sulfonic acid in-tube solid-phase microextraction coating for analysis of nitrogen-containing pesticides in water environments. Front. Environ. Sci. 12:1350170. doi: 10.3389/fenvs.2024.1350170
Received: 05 December 2023; Accepted: 22 April 2024;
Published: 20 May 2024.
Edited by:
R. Busquets, Kingston University, United KingdomReviewed by:
Mohammad Rizwan Khan, King Saud University, Saudi ArabiaAntonella Aresta, University of Bari Aldo Moro, Italy
Constantinos K. Zacharis, Aristotle University of Thessaloniki, Greece
Copyright © 2024 Ma, Yu, Li, Lin and Ma. This is an open-access article distributed under the terms of the Creative Commons Attribution License (CC BY). The use, distribution or reproduction in other forums is permitted, provided the original author(s) and the copyright owner(s) are credited and that the original publication in this journal is cited, in accordance with accepted academic practice. No use, distribution or reproduction is permitted which does not comply with these terms.
*Correspondence: Rong Ma, bXI5NzQzODc5NzdAY3ptYy5lZHUuY24=; Xiaodong Ma, ZG9uZ3htQGNhdS5lZHUuY24=
†These authors share first authorship