- 1Department of Botany, School of Natural Sciences, Trinity College Dublin, The University of Dublin, Dublin, Ireland
- 2Department of Civil, Structural and Environmental Engineering, School of Engineering, Trinity College Dublin, The University of Dublin, Dublin, Ireland
- 3Department of Microbiology, Moyne Institute of Preventive Medicine, School of Genetics and Microbiology, The University of Dublin, Dublin, Ireland
- 4Trinity Centre for Environmental Humanities, School of History and Humanities, Trinity College Dublin, The University of Dublin, Dublin, Ireland
- 5Department of Mechanical Engineering, School of Engineering, Trinity College Dublin, The University of Dublin, Dublin, Ireland
Non-point source pollution and water eutrophication from agricultural runoff present global challenges that impact ground and surface waters. The search for a feasible and sustainable mitigation strategy to combat this issue remains ongoing. This scoping review aims to explore one potential solution by examining relevant literature on agricultural practices of the past and recent edge-of-field measures, designed to ameliorate the impacts of agricultural runoff on soil and water quality. The study focuses on integrating findings from diverse research fields into a novel myco-phytoremediation approach, which involves the synergistic relationship of plants, arbuscular mycorrhizal fungi, and plant beneficial bacteria within vegetative buffer strips. The implementation of these augmented buffer strips enhances nutrient retention in the soil, reduces runoff volume, promotes biodiversity, and increases plant biomass. This biomass can be converted into biochar, an effective sorbent that can be used to filter dissolved and particulate nutrients from surface waterways. The resulting nutrient-rich biochar can be repurposed as a form of bio-fertiliser, optimizing fertiliser consumption and subsequently reducing the depletion rate of phosphorus, a limited resource. This paper investigates a circular model of abatement of agricultural runoff via maximal nutrient retention and subsequent recycling of nitrogen and phosphorus back into the agricultural system. The key impact lies in its contribution to addressing the issue of non-point source pollution and eutrophication by encouraging multidisciplinary research aimed at solving these complex environmental issues.
1 Introduction
Eutrophication is the phenomenon where water bodies experience an excessive influx of nutrients (Kumar et al., 2020). This is a natural process that occurs gradually over time, leading to the degradation of aquatic ecosystems (Yu et al., 2020). However, anthropogenic activities such as improper sewage disposal, use of agrochemicals, and industrial by-products can trigger cultural eutrophication by elevating the nutrient concentrations in the water body beyond the required level for a balanced ecosystem (Kumar et al., 2020). Notably, in agricultural systems, the major source of nutrient pollution comes from surface runoff, leading to high amounts of phosphorus (P) and nitrogen (N) species mobilising into freshwater and marine systems (Xia et al., 2020). Runoff rates have been reported to be 9.5% and 3.3% of P and N respectively for cropland dosed with 196 kg/ha of N and 87 kg/ha P per year (Harmel et al., 2009; as cited by Xia et al., 2020). Eutrophication is known to cause serious ecological problems: excessive nutrient levels has been linked to vertebrate, invertebrate and plant biodiversity losses as well as a decrease in cross-taxon congruency (Bunting et al., 2016; Wang et al., 2021). Additionally, eutrophication of water bodies utilised for drinking and irrigation purposes can have detrimental consequences for human health, such as an increased incidence of harmful algal blooms (van Beusekom, 2018). These pose a greater threat when cyanobacteria are the dominant species (Barçante et al., 2020). These highly prevalent and competitive phytoplankton (Paerl and Otten, 2013) are responsible for producing toxic chemical compounds that can greatly compromise water quality and raise sanitation alarms if they reach elevated growth levels (Padedda et al., 2017). The European Environmental Agency (EEA), the United Nations Environment Programme (UNEP) and several nations have recognised the severity of this issue, with the former indicating that 18% of European groundwater is of poor status due to nitrates (European waters, 2018). Since 1991, the EU’s Nitrate Directive has attempted to regulate and reduce N pollution from point and diffuse sources (European waters, 2018). Despite this, the United Nations does not currently include nitrate or other forms of agricultural pollution as part of the Sustainable Development Goal 14: Life Below Water (Environment, 2019).
The relative abundance of N and P provide limits to bacterial and algal growth, and therefore eutrophication, in salt and freshwater ecosystems, respectively (Schlesinger and Bernhardt, 2013; Guignard et al., 2017). The bioavailable forms of these nutrients are industrially manufactured and commercially available to farmers in the form of artificial fertilisers. However, empirical evidence has demonstrated that fertiliser application in agricultural settings often exceeds the optimal nutrient requirements of crops, resulting in a consistent surplus of nutrients available in the soil following each fertiliser application (Yli-Halla, 2016).
This review will provide an interdisciplinary overview of the current knowledge on agricultural pollution and existing edge of field technologies aimed at mitigating this issue. The central hypothesis underlying this research is that the synergistic interactions among plants, arbuscular mycorrhizal fungi (AMF) and plant beneficial bacteria (PBB) can be harnessed within an engineered vegetative buffer strip (VBS) as a novel form of myco-phytoremediation. Buffer functioning could be improved by enhancing these synergies, leading to higher quantities of N and P assimilated by vegetation, mitigating groundwater pollution arising from agricultural runoff. To prevent the release of accumulated nutrients into the environment, the VBS will be harvested and repurposed as biochar, which serves as an in-stream nutrient sorbent. Moreover, the loaded biochar can subsequently be applied to farmland as a form of bio-fertiliser. This innovative strategy (termed the NuReCycle approach) would not only minimise fertiliser costs, but also facilitate the recovery, reuse and recycling of excess nutrients originating from agricultural runoff (Figure 1). Grounding this methodology in the historical context of agricultural intensification, as demonstrated in Section 1, could ease adoption within existing agricultural systems.
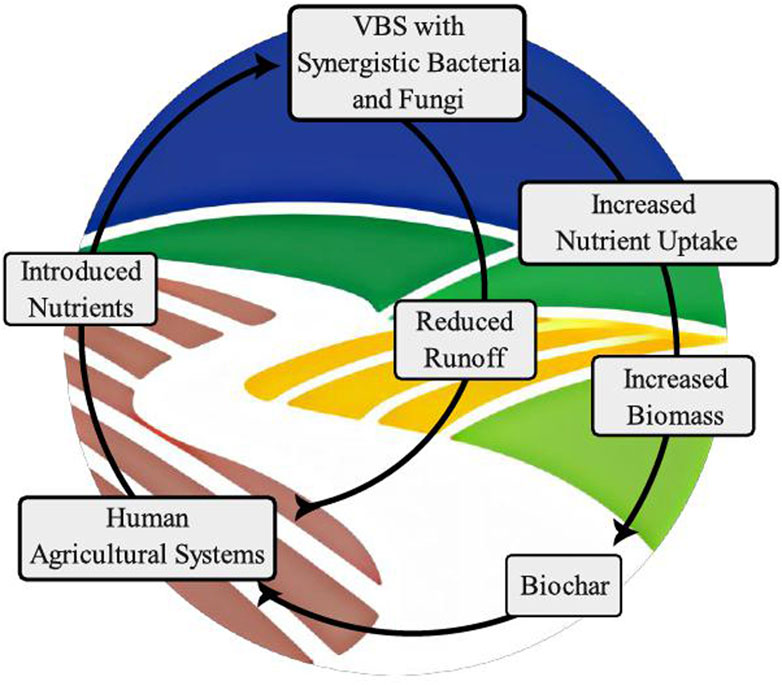
Figure 1. Graphical model of the NuReCycle’s proposed interdisciplinary intervention, showing the interactions of the myco-phyto engineered vegetative buffer strip, biochar-based nutrient capture, and human agricultural systems.
2 Human dynamics of agricultural systems
This literature review employs a multi-disciplinary perspective to understand the full scope of knowledge on agricultural pollution and its mitigation strategies, including the abiotic, biotic, and human components of agricultural systems. Environmental history is a subdiscipline of history which attempts to understand the ever-changing perception humans have of their environments and narratives of interaction and influence between human and nonhuman actors. This places environmental history in unique position to investigate agricultural pollution in the context of anthropogenic activities.
Our understanding of the narrative and impacts of agricultural developments has already benefited greatly from the research of environmental historians. Donald Worster’s call for an explicit agricultural focus to understand the ways in which capitalism has transformed nature from the 19th century to the present, highlights one of the key advantages of the discipline. Such historians have detailed how instrumental agricultural has been in simplifying “the complex forces and interaction, beings and processes” that we recognise as nature, to the abstracted commodity of “land” (Worster, 1990). Environmental historians of agriculture have also contributed to our understanding of the mechanisms of change in agricultural practice, modelling knowledge transfer between scientific bodies like soil testing labs and agricultural advisory services and farmers and farm communities (Uekoetter, 2006; Mícheál, 2021). The 20th century transition to an industrial model of agricultural production is also a well-established topic in environmental history. Several authors have drawn attention to the ways in which energy intensive systems of mechanisation and irrigation entangled contemporary agricultural systems with networks of fossil energy (McKittrick, 2012; Watson, 2020).
Despite the role of fertilisers as a primary driver of agricultural industrialisation and fossilisation, as well as being an important aspect of pollution and environmental awareness, their analysis as a historical subject has primarily been limited to the late 19th century. Two major contributions to the subject are “Guano and the opening of the Pacific world” and “The First Green Revolution” (Melillo, 2012; Cushman, 2013). A detailed account provided by the former entails the extraction and import of Peruvian guano and Chilean nitrate to North America and Europe, beginning in the mid-19th century. The latter contributed to our understanding of the political economy of this trade through an exploration of the system of slavery and coerced labour that underpinned this vast export trade. An eco-Marxist analysis of this history argues that Britain’s dependence on imported guano and phosphorus from the Pacific world during this time created a metabolic rift between what the upper limit of the surrounding agricultural system and the intensive output demanded by the British colonial market (Foster and Clark, 2018).
Analysis of the 19th century guano trade provides key insights into how this influx of nutrients into Western agriculture caused significant agricultural intensification and laid ground for the development of intensive agricultural systems we recognise today. However, limited research into the later and more profound intensification brought about by the 20th century regime change, facilitated by the Haber-Bosch Process, and its impact on global agricultural systems and the global N cycle has been conducted from a historical viewpoint. One exception has used the role of N in agriculture as an “organising device” to showcase capitalism as a driver of innovation, but offers little historical analysis of N’s role in the material changes of modern agriculture (Gorman, 2013).
Fertiliser in the 20th century has primarily been discussed within a larger debate about the Anthropocene. In this context, global fertiliser consumption has increased exponentially from 14.5 million tons in 1950 to 171.5 million tons in 2010, while an annual introduction of N to global coastal zones has similarly increased from 18.7 million tons/year in 1950 to 79.7 million tons/year in 2000, representing indicators that have been described as “The Great Acceleration” and “1950s Syndrome” (Pfister, 2010; Steffen et al., 2015).
This Anthropogenic turning point refers to a period of accelerated economic growth in the global North which, coupled with a steady decline in fossil fuel prices, resulted in exponential growth in energy, raw materials and accompanying environmental degradation. Countries that were subject to post-war international development programs such as The Marshall Plan and The Green Revolution experienced this to a higher degree (Pfister, 2010). High inputs from fertiliser, fuel and irrigation used in crop and livestock production drastically changed the net energy outputs in these industrialised agricultural systems. In the case of North American livestock operations, where animals utilise a significant proportion of their metabolic budget to survive, less energy is generated by the system than is introduced. It has been shown that Kansas cattle farmers in 1959 used 2.5 times more energy to distribute groundwater for feed corn the in the production of marketable beef (Watson, 2020). An understanding of contemporary fertiliser manufacturing’s reliance on natural gas as a primary feedstock helps to clarify this correlation between the low post-war fuel prices and increased fertiliser use, but much still remains to be explored in the development of fossil-fuelled intensive agricultural systems of the 20th century.
The pollution of Irish surface waters has been recognized since the 1980s, with the deterioration of water bodies such as Lough Sheelin in counties Meath, Westmeath, and Cavan serving as a primary motivator in the establishment of Ireland’s Environmental Protection Agency (EPA) in 1993 (Environmental Protection Agency, 1989; Houses of the Oireachtas, 1991). According to the Irish EPA, 46% of Ireland’s surface water is of moderate, poor, or bad water quality, and the number of water bodies of high ecological status is in steady decline. The EPA cites agricultural run-off as the primary cause of this deterioration, with elevated levels of nitrate and phosphorous being of especially high concern (Trodd and O’Boyle, 2022).
Historically, the intensification of Ireland’s agricultural system followed the rest of northern Europe by around half a century (Figure 2). The introduction of mineral fertilisers coincided with the introduction of national and EU-wide awareness and regulation of environmental problems. The importance of agriculture to the Irish economy has led economic historians of Ireland to highlight the topic in their own research (Bielenberg and Ryan, 2016; Ó Gráda and O’Rourke, 2021). Much of our understanding of Irish agricultural history has come from institutional histories, like those written about Ireland’s Department of Agriculture and the Agricultural Advisory Service, which explain the role of the state in developing and maintaining specific agricultural systems (Daly, 2002; Mícheál, 2021). Ireland’s fertiliser industry has also been explored in the context of the connection between heavy industry and agriculture over the course of the 20th century (Cooper and Davis, 2004). Additionally, an understanding of how farmers navigate the institutional framework by gauging their responses to pressure and promise of the European Union’s Common Agricultural Policy has also been investigated (Tovey, 1992; Crowley, 2003). Lastly, the wide-ranging volume on “history and heritage” of farming in Ireland provides a scientific grounding to the impacts of different agricultural regimes, from the medieval to the contemporary on Ireland’s landscape and biodiversity (Feehan, 2003). A notable dearth in these accounts and other agricultural histories of Ireland is an effort to move beyond the disciplinary boundaries of the humanities and to connect their histories with contemporary agricultural issues at a level deeper than rhetoric. Embedding a historic analysis of diffuse agricultural pollution within an interdisciplinary framework will uncover more effective models for mitigation efforts.
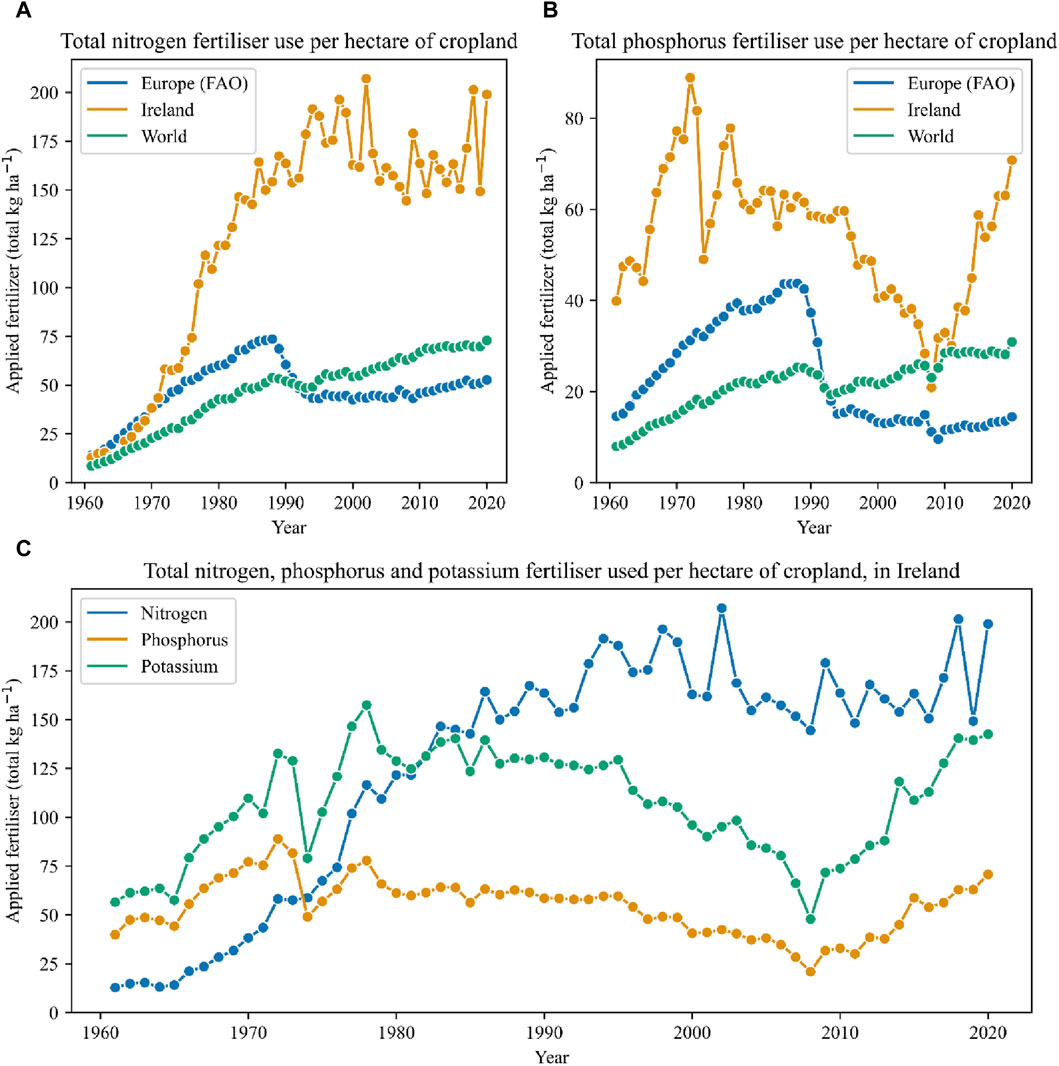
Figure 2. Mass of total nitrogen (A) and total phosphorus (B) spread as fertiliser per hectare of cropland in Ireland, Europe and globally. Mass of fertiliser used per hectare of cropland, by nutrient type, in Ireland (C). Data compiled from fertiliser use per hectare of cropland from the FAO’s dataset, covering 1961-2020, retrieved on 22-02-2023 from https://www.fao.org/faostat/enfitdata/RFN.
3 Vegetative buffer strips
The capacity of VBSs to reduce the concentration of N and P compounds in agricultural runoff has been extensively reviewed in the past (Barling and Moore, 1994; Dosskey, 2001; Hickey and Doran, 2004; Helmers et al., 2008). Several studies have consolidated their effectiveness by outlining the reliability and efficacy of buffer strips. In the presence of VBSs, total suspended solids (TSS) and total runoff volume (TRV) are reportedly reduced by 30%–94% and 33%–90% respectively (Supplementary Table S1). Similar results can be observed for total nitrogen (TN) and total phosphorus (TP), exhibiting respective reductions of 23%–78% and 37%–80% (Supplementary Table S1, in Supplementary Material). Though the results show reasonable variation, it can be stated that VBSs perform well under a range of different physical, environmental and anthropogenic conditions.
High sedimentation and infiltration rates in buffer strips substantially reduce runoff volume while increasing the contact time between nutrients and vegetation, resulting in increased plant assimilation, subsequently reducing nutrient concentrations in adjacent streams (Figure 3) (Borin et al., 2005). Moreover, the large quantities of suspended solids intercepted by VBSs can also be attributed to the combined effort of these processes (Duchemin and Hogue, 2009). Reducing the load of TSS is not only conducive to general water quality, but it also holds implications for reducing the eutrophic capability of runoff. There appears to be a strong correlation between sediment capture and reduction to TP loads in runoff. This phenomenon is likely due to the strong affinity of P to its sediment-bound form (Borin et al., 2005). Hence, the ability of VBSs to reduce P concentrations in runoff is directly attributable to their ability to efficiently intercept suspended sediment. The mobility of P in soil is relatively poor, making it quite amenable to phytoextraction (Hu et al., 2023). Nitrogenous soil compounds on the other hand exhibit more variable interactions with the buffer strip on account of their elevated mobility. Ammoniacal nitrogen in water exists in an equilibrium between dissociated ammonia and the ammonium ion, both of which are highly water-soluble and show little affinity for particulates (Hu et al., 2023). Dissolved nitrates are readily microbially transformed and subsequently assimilated by the vegetation (Lv and Wu, 2021).
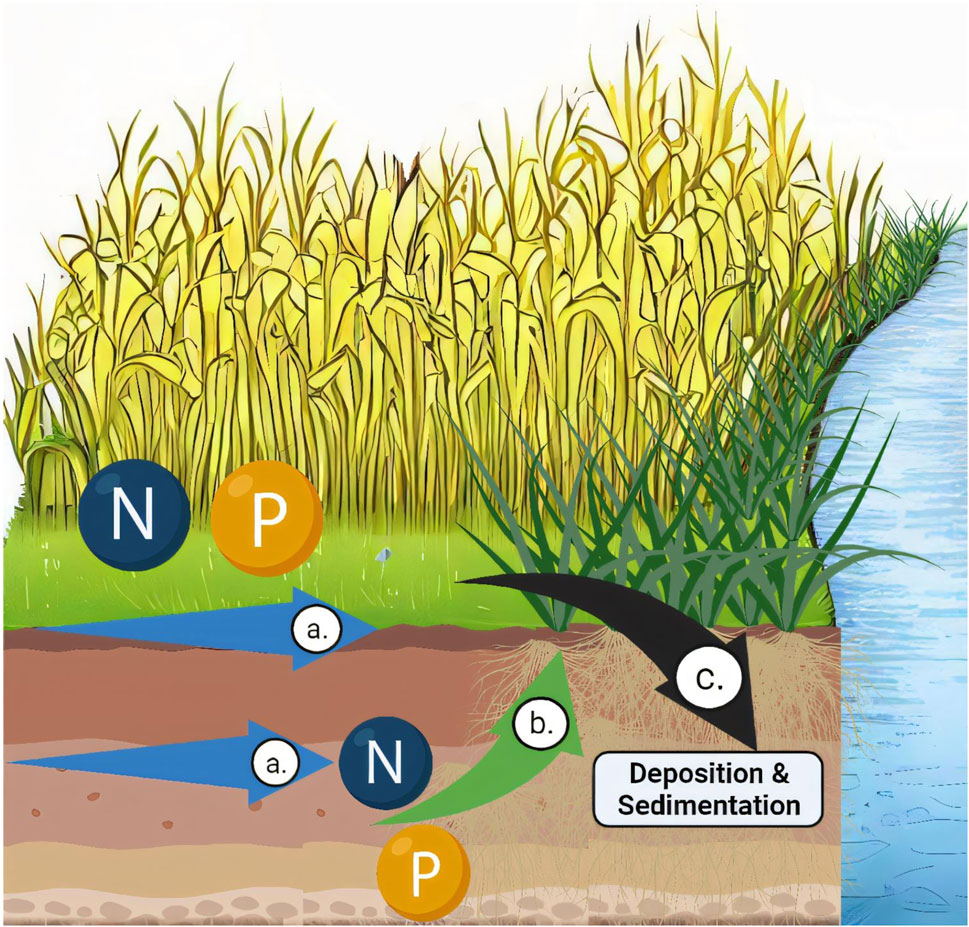
Figure 3. A vegetative buffer strip and the mechanisms that underpin its capacity for nutrient interception. Runoff, including subsurface and overland flows, is reduced by vegetative buffer strips (a.). Plant assimilation (b.) of nitrogen and phosphorus compounds occurs when subsurface flows reach the dense rhizosphere. Overland flow is slowed by the aerial region of the vegetation, facilitating infiltration (c.). The suspended solids are deposited and undergo a process known as sedimentation. These mechanisms increase the contact time between nutrients and plant roots, enhancing plant nutrient uptake and thus, reducing the concentration of nitrogen and phosphorus in runoff. This mitigation approach can greatly reduce the risk of eutrophication. N = Nitrogen. P = Phosphorus. Blue arrow (a.) = Runoff. Green arrow (b.) = Plant nutrient assimilation. Black arrow (c.) = Infiltration. Created with BioRender.com.
Buffer efficacy is limited by several factors. Preferential flow (PF) paths increase the risk of soluble pollutants bypassing the VBS (Allaire et al., 2015). These channels can form along large tree roots. Positive feedback loops arise when soil particles are detached by the concentrated flow, resulting in a larger path with an elevated flow rate and subsequent increased sediment erosion (Allaire et al., 2015). PF paths can be avoided through the use of vegetation with fine, deep rooting systems that provide homogenous cover and density as these facilitate uniform sheet flow (Borin et al., 2005; Allaire et al., 2015). Regular vegetation harvesting can also be implemented to encourage further plant nutrient uptake and to ensure nutrients do not return to the rhizosphere (Allaire et al., 2015). If left undisturbed, as much as 80% of the assimilated nitrogen can return to a wooded buffer strip through senescence and decay (Lv and Wu, 2021). High rainfall intensity augments the volume and velocity of runoff, greatly influencing a buffer’s ability to intercept nutrients. It presents a double antagonism; increasing the risk of soil erosion and occurrence of PF channels, while reducing the contact time between nutrients and the VBS (Hu et al., 2023). Climate can also be a limiting factor in buffer efficacy, as a result of the dearth of plant growth and therefore nutrient interception over the winter months. The occurrence of freeze-thaw cycles further exacerbates this problem, as these conditions render soils liable to releasing P into adjacent streams (Kieta et al., 2018).
The ability of VBSs to effectively intercept and remove nutrients from agricultural runoff is complex, site-specific and dynamic. Plant absorption appears to have the greatest impact on nutrient removal from runoff which in itself is governed by plant biomass, morphology, and growth characteristics (Hu et al., 2023). Vegetation with high hydraulic resistance, density and uniformity converts runoff into sheet flow prior to entering the buffer. In addition to reducing flow velocity, this avoids the creation of PF paths (Saleh et al., 2018). Grasses and shrubs achieve this while also improving pollutant interception by facilitating infiltration through increasing the surface roughness of the soil (Lv and Wu, 2021). Infiltration is further enhanced by increased root density, leading to greater interception of particulates and sorption of percolating P (Stutter et al., 2009). Woody plants are less prone to lodging, which hampers buffer efficiency by creating PF pathways, as a result of their more developed rooting systems and higher biomass (Dunn et al., 2022). Wooded buffers exhibit an increased ability to trap sediment, an important quality when considering the close functional relationship between fine grain sediment and P (Dunn et al., 2022). A combination of a grassed strip and wooded buffer exhibits increased hydraulic resistance, infiltration rates, and reduced runoff volume (Duchemin and Hogue, 2009). Vegetation with high initial growth rates show increased effectiveness early on, likely due to greater cover. However, plants capable of producing more biomass are substantially more effective long-term (Kavian et al., 2018). Low vegetation cover not only increases the occurrence of PF channels, but it also makes the soil more susceptible to water erosion (Duchemin and Hogue, 2009).
Substantial rates of nutrient interception has been achieved with 15 m wide buffers (Lv and Wu, 2021). This width provides the plant and microbial communities with sufficient contact time to transform and assimilate nutrient infiltrates that move latitudinally through the VBS. Non-significant differences in N uptake from runoff are observed by further increasing buffer width. (Lv and Wu, 2021). However, there is evidence that narrow buffers exhibit similar capabilities for abating nutrient runoff, limiting losses to economically viable land while protecting against eutrophication (Borin et al., 2010). Buffer age is another mitigating factor that requires consideration. The initial soil disturbance and low vegetation cover provided by recently established buffers can result in poor filtering efficiency, though fast growing plants such as grasses will reach maturity faster (Dunn et al., 2022). Mature buffers have been shown to eliminate dissolved nitrates and P from runoff almost entirely from runoff (Borin et al., 2010). This is primarily due to their relatively increased plant biomass and vegetation cover. However, instances of older buffers becoming sources, rather than sinks, of N and P have also been recorded (Borin et al., 2005). This can be explained by P adsorption sites within the buffer strip becoming saturated. As there are few exit pathways for P other than plant assimilation, extreme rainfall events pose considerable risks to water quality as they mobilise these P-enriched soils, leading to elevated nutrient concentrations in adjacent streams (Stutter et al., 2009). Particulate phosphorus (PP) has a strong affinity for sediment, rendering it inaccessible to plants. Microbial solubilization transforms PP into a more mobile and therefore bioavailable form (Stutter et al., 2009). These microbial communities are more dominant in the VBSs than the adjoining farmland, resulting in enhanced mineralisation of N and P compounds, which holds implications for increased plant productivity and biomass (Stutter et al., 2012). Microbial processing may be required to unlock the large quantity of immobilised P stored in the existing P pool from decades of fertiliser application (Stutter et al., 2009). The manipulation of microbial communities that augment biogeochemical cycles could be incorporated into VBSs to offset nutrient leaching and improve plant assimilation.
Unmanaged VBSs can pose considerable risks of nutrient leaching, P saturation and overall reduced buffer efficiency. Harvesting the vegetation prior to senescence alleviates P enrichment by removing P bound in biomass but also by facilitating successive nutrient uptake as additional plants are introduced. This method is far less destructive than topsoil removal which renders the VBSs vulnerable to soil erosion and thus increased TSS and PP in runoff (Hille et al., 2019). Moreover, vegetation harvesting substantially reduces P stocks in VBSs topsoils, thus reducing the total soil P pool in the long term. Therefore, harvesting is an effective management strategy that directly and immediately reduces the risk of P leaching while also ameliorating the total soil P pool (Hille et al., 2019). The difference in reductions to soil P levels achieved by one or multiple harvests per year is insignificant due to reduced vegetation cover and biomass accomplished by multiple establishments over a single growth period (Hille et al., 2019). A single harvesting event is also more commercially viable for landowners, estimated to cost $6-11 per hectare period (Hille et al., 2019). Moreover, the economic gains provided by this strategy makes it amenable to farmers; harvested vegetation provides a source of timber, pulp or biofuel, granting significant monetary value to previously unprofitable land (Borin et al., 2010). Hence, this strategy presents landowners with both environmental and economic incentives to establish and maintain VBSs, greatly improving buffer efficacy, and thus ameliorating water quality.
Although VBSs have long been posited as a low-intensity strategy for filtering agricultural runoff, they cannot be the sole focus of mitigating non-point source (NPS) pollution due to soil nutrient enrichment. A management regime must be put in place prior to the establishment of a buffer strip, such as the planned removal of vegetation. A novel use for these cuttings is their conversion into biochar, a substance that can be utilised in-stream to filter and adsorb both dissolved and particulate nutrients. An increased focus should also be placed on the manipulation of soil microbial communities, such as AMF and PBB, in order to increase the bioavailability of N and P for plants, facilitating enhanced plant nutrition and thus improved buffer functioning. If used in conjunction with each other, these strategies present an innovative, sustainable method of reducing and recycling nutrients from agricultural runoff. In conclusion, rather than being considered an end of pipe solution to NPS pollution, VBSs should be integrated as part of a greater system for reducing and recycling nutrients in agroecosystems. Additional edge of field technologies that aid in nutrient control will be discussed in further detail in this review.
4 Microbial interactions
A synergistic relationship can be observed between plants, AMF and PBB which aids plant N and P acquisition (Nanjundappa et al., 2019). This symbiosis provides vegetation with more N from organic matter than plants grown with AMF or PBB alone, with this relationship being responsible for as much as 50% of the total plant N absorption (Hestrin et al., 2019). This synergism of plants, AMF and PBB could be harnessed in a VBS as a form of myco-phytoremediation; a novel edge of field technology to recover and recycle nutrients from agricultural runoff.
Phytoremediation refers to the collection of methodologies that utilise plants to take up, reduce and degrade pollutants, of both environmental and anthropogenic origins, to restore a site to a healthy condition (Peer et al., 2005). A similar methodology, mycoremediation, employs the abilities of fungi for nutrient cycling and degradation of organic materials. Recent efforts have been made to introduce a hybrid of these forms, myco-phytoremediation, which intends to capitalise on the synergism between fungi, bacteria and plants to mitigate soil and water pollution (Rubin and Görres, 2021). Myco-phytoremediation has been used to increase crop yields (Hijri, 2016), enhance plant tolerance to abiotic stress such as salinity and drought (Pavithra and Yapa, 2018; Djighaly et al., 2020) while also improving plant resistance to biotic stresses, including pests and diseases (Vannette and Hunter, 2011; Song et al., 2015; Pozo Jiménez et al., 2016). These have substantial implications for improving the sustainability of the agricultural sector by reducing reliance on chemical fertilisers, pesticides and fungicides, all of which can have negative impacts on fluvial and terrestrial ecosystems. This strategy also holds potential for mitigating the eutrophic effect of agricultural runoff by integrating AMF and PBB into VBSs.
PBB include many bacteria that encourage plant growth, including phosphate solubilising bacteria (PSB), mycorrhizae helper bacteria (MHB) and N-fixing bacteria (Figure 4). PBB play a major role in the phytoremediation of metals from soil (Alves et al., 2022). While the phytoremediation of N and P is a novel concept, the benefits that PBB provide as a biofertilizer via improved nutrient adsorption (Chiaranunt and White, 2023) could be harnessed in a VBS for increased plant growth. Other advantages of PBB include phytohormone production, abiotic stress relief and pathogen control (Das et al., 2022; Chiaranunt and White, 2023).
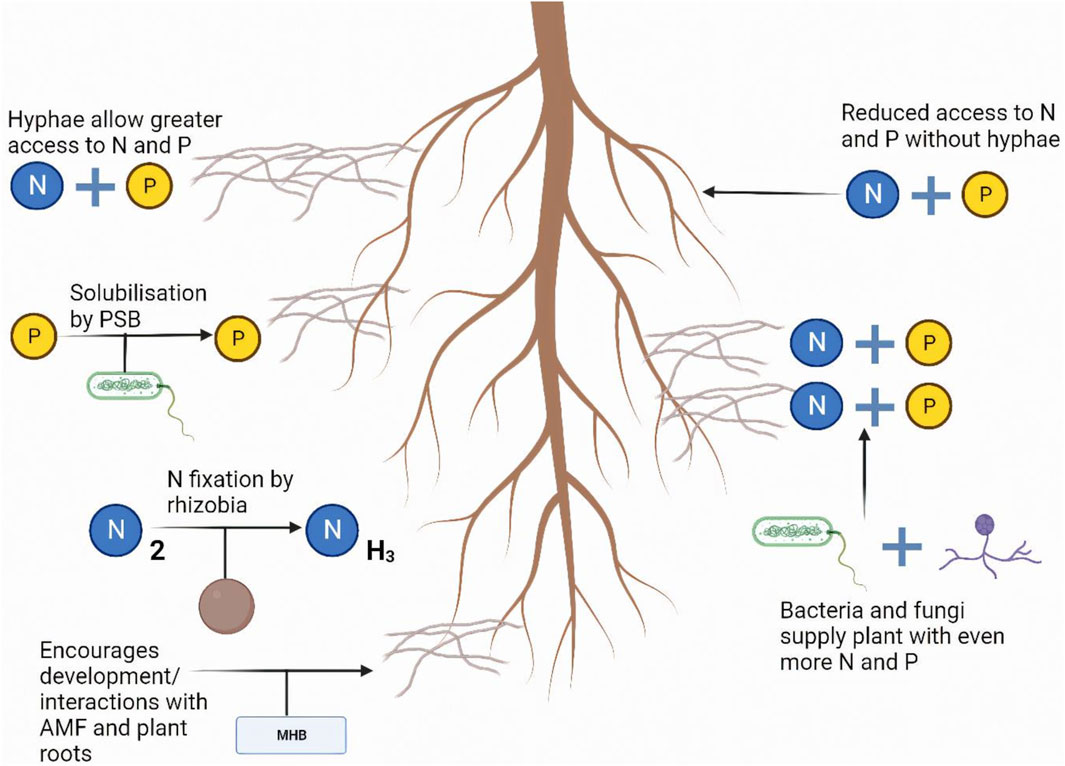
Figure 4. The interactions between plant beneficial bacteria and arbuscular mycorrhizal fungi and the benefits provided to plant roots. The mycorrhizal hyphae extend far beyond the root network, providing the plant with an increased N and P supply. Without hyphae, plants have reduced access to N and P. Phosphate solubilising bacteria convert phosphate into a soluble and therefore bioavailable form for plants, while mycorrhizal helper bacteria enhance the development of the synergism between arbuscular mycorrhizal fungi and plant roots. The synergistic relationship between PBB and AMF enables a plant to absorb even more N and P. Created with BioRender.com.
A synergistic relationship exists between MHB and AMF that improves plant productivity. MHB encourage the development and interactions between plant roots, AMF and associated bacteria by enhancing fungal spore germination, hyphal growth and root colonisation (Nasslahsen et al., 2022; Sangwan and Prasanna, 2022). There are two functionally different groups of MHB; those that affect pre-existing symbioses and those that encourage the development of new synergistic relationships (Frey-Klett et al., 2007). This ability to encourage symbiotic relationships has significant potential to improve buffer functioning.
Nitrogen is a vital element for plant growth and development, although the main source of N, atmospheric N (N2), cannot be absorbed by plant roots. It must first be converted to ammonia (NH3) through biological fixation, mediated by N-fixing bacteria (Vocciante et al., 2022). While the VBS functions to reduce, rather than increase, soil N concentrations, encouraging N-fixing bacteria, such as Rhizobia, through the planting of legumes in fields lowers requirements for artificial N fertiliser (Wei et al., 2022). Alternatively, denitrifying bacteria could be used to remove soil N, though nitrous oxide (N2O), a greenhouse gas that depletes ozone, is a harmful output of this process (Zhou et al., 2022).
A novel strategy for enhancing VBSs is the introduction of PSB. These microorganisms facilitate plant P absorption (Nacoon et al., 2021), which is fundamental to plant growth. They have been reported to improve crop growth in wheat (Wang Z et al., 2022), maize (Beltran-Medina et al., 2023) and rice (Fitriatin et al., 2022). Common genera of PSB include Pseudomonas, Bacillus, Arthrobacter, Rhodococcus, Serratia, Gordonia, Chryseobacterium, Azotobacter, Klebsiella and Enterobacter amongst others (Sharma et al., 2013).
It is difficult for plant roots to absorb both organic and inorganic P from soil on account of its low solubility (Liang et al., 2019; Vocciante et al., 2022). Plants are reliant on members of the soil microbiome, such as fungi and bacteria, and their interactions, to access N and P bound to organic molecules which are otherwise inaccessible to the plants (Bhanse et al., 2022). As AMF are unable to absorb insoluble P, they recruit the solubilisation activity of PSB, converting it to orthophosphate. AMF can readily absorb this compound and supply it to its host plant (Nacoon et al., 2021). PSB use multiple methods to enhance the bioavailability of P for plants. These include the production of organic acids, inorganic acids, siderophores, Indole–3–Acetic Acid (IAA), 1-aminocyclopropane 1-carboxylic acid (ACC) deaminase production and organic P mineralisation (Etesami et al., 2021). By producing organic and inorganic acids, PSB adjust the soil pH to the ideal range for maximal P availability (Etesami et al., 2021). Siderophore production solubilises unavailable P (Sharma et al., 2013; Etesami et al., 2021) while IAA and ACC-Deaminase encourage root hair development, increasing the root surface area and thus facilitates greater P adsorption (Hayat et al., 2010; Emami et al., 2019; Etesami et al., 2021). Moreover, PSB secrete phosphatases that mineralise organic P (Sharma et al., 2013).
The introduction of PBB and symbiotic fungi into a VBS may not be successfully established. Therefore, it may be more effective to encourage the growth of microbial communities already present in a VBS. It is vital that the many factors that alter the relationship between plants, AMF and PBB are further identified. Plant root exudates modify the rhizosphere microbiome, encouraging the growth of beneficial fungi and bacteria (Vives-Peris et al., 2020). The way in which these plants selectively choose their ideal microbiota must be investigated. The growth of the extra-radical mycelium of AMF can also be suppressed by the soil microbial community and by the soil type (Svenningsen et al., 2018). Furthermore, high P availability in soil can inhibit AMF establishment with plant roots (Balzergue et al., 2013). In N-rich soils the synergy between plants, AMF and bacteria is disrupted, negatively affecting the ability to absorb N from organic matter (Hestrin et al., 2019). These factors have implications for the effectiveness of a myco-phytoremediation approach in a VBS, specifically to for optimal functioning in N- and P-rich soil. Various agricultural methods like deep ploughing in tillage farming must be taken into account, as these can negatively impact AMF by damaging the spores, hyphae and colonised plant roots (Rubin and Görres, 2021). Agricultural fungicides represent another obstacle, with these chemicals having been shown to negatively repress AMF colonisation of tomato roots (Okiobe et al., 2022).
AMF are intimately involved in plant nutrition, being responsible for supplying considerable quantities of P and N to their associated plant partners (Smith and Read, 2010). Their hyphal biomass extends far beyond the reaches of plant roots, acquiring nutrients otherwise inaccessible to vegetation (Martínez-García et al., 2017). This synergistic relationship positions AMF as excellent candidates for a myco-phytoremediation approach to help mitigating agricultural runoff and nutrient leaching. Through the facilitation of increased plant nutrient uptake, it is evident that mycorrhizal plants intercept a higher quantity of pollutants from runoff. It has been reported that TP concentrations in runoff and leachate have been reduced by 10%–44% in the presence of AMF (Supplementary Table S2). The strategic placement of AMF into VBSs could target diffuse agricultural pollution at its source, providing major improvements to water quality.
While interest in mycorrhizal fungi is growing, publications on field-based experiments investigating their impact on agricultural runoff remain few (Zhang et al., 2015; Zhang et al., 2016); the majority of investigations occur in greenhouse microcosm settings. Though these are not entirely representative of field conditions, they are indicative of the capacity of AMF to intercept nutrients. Although AMF appear to play a role in N cycling, more variable results can be observed than those involving P (Supplementary Table S2). A limited number of studies have exhibited an increased concentration of N, likely due to the high solubility of nitrates (Singh and Craswell, 2021), which reduces the contact and subsequent reaction time in the soil matrix. Ammonium adheres more to soil particles as it is less mobile than nitrates (Jeng and Vagstad, 2009). These results outline a decreasing trend of groundwater pollution in the presence of AMF, positing these microorganisms as ideal candidates for the bioremediation of agricultural runoff (Supplementary Table S2). These trends are governed by both direct and indirect mechanisms of AMF.
AMF reduce nutrient pollution through direct uptake of N and P. An overall increase in P cycling is observed in the presence of AMF, as otherwise unavailable soil P sources are mobilised (Bender et al., 2015). This is achieved via enhanced mineralization of N and P compounds in addition to the release of chelating agents (Fall et al., 2022), increasing the abundance of bioavailable compounds that are allocated towards hyphal growth. AMF absorb significantly more nutrients than their plant partners due to their hyphae being orders of magnitude narrower than roots, increasing their surface area and providing access to nutrients in micropore spaces inaccessible to plants (Allen, 2011). AMF immobilise large quantities of nutrients in the hyphal biomass, acting as a sink for both N and P (Bender et al., 2015). Additionally, this dense mycelial network physically binds the soil together while the exudation of glomalin joins micro-aggregates together into macro-aggregates, improving soil structure, water holding capacity, infiltration rates while reducing soil erosion (Zhang et al., 2016; He et al., 2021; Fall et al., 2022). This is crucial to minimising the concentration of TSS, water volume and nutrient load from runoff. These direct mechanisms position AMF as ideal candidates for a myco-phytoremediation strategy for mitigating groundwater pollution. On the other hand, AMF can also decrease the risk of eutrophication indirectly by enhancing the efficacy of VBSs. The improvements to soil nutrient availability and plant nutrition, in concurrence with the translocation of N and P from fungi to plants, (Van Der Heijden, 2010; Zhang et al., 2016; He et al., 2021), have the knock-on effect of increasing plant biomass (Corkidi et al., 2011), which subsequently enhances buffer functioning. Moreover, the increased plant biomass would facilitate an elevated rate of transpiration, further reducing runoff volume. This would further improve infiltration rates and thus buffer efficiency, potentially mitigating the eutrophic effect of agricultural pollution. In effect, AMF can directly uptake and store nutrients in addition to improving VBS performance, enhancing their potential for abating groundwater pollution.
The variability that can be observed between treatments (Supplementary Table S2) can be traced to a number of influencing factors. Plant nutrient demand varies with differing growth forms. The quick, plastic growth of grasses demands a high, short-term nutrient supply, to which AMF can provide. In contrast, slow growing, woody perennials have less immediate nutrient requirements and so nutrient uptake can be limited (Corkidi et al., 2011). The integration of AMF into buffer zones would thus influence the vegetation type, a critical factor that determines buffer performance. Edaphic factors are another source of variation, as outlined by reduced root colonisation in heath soils, in comparison to pasture soils (Bender et al., 2015). Similarly, mycorrhizal colonisation can be limited by high levels of fertiliser (Wang et al., 2009). Under such challenging conditions, the ability of AMF to reduce runoff nutrient concentrations may be restricted. It is crucial to understand that the role of AMF in nutrient cycling and plant nutrition is context dependent and may be limited by a number of factors. These must be considered prior to integration into a myco-phytoremediation plan.
In the presence of AMF, leaching of both N and P is substantially reduced under heavy rainfall conditions (Martínez-García et al., 2017; He et al., 2021). It is evident that AMF minimise the risk of nutrient loss from extreme weather events, a major limitation of VBSs. A strategy combining AMF inoculation and reduced fertiliser treatment poses considerable benefits. Reductions to fertiliser application of just 20% in conjunction with AMF inoculation leads to N and P runoff being lowered by 27.2% and 44% respectively without compromising crop yields (Zhang et al., 2015; Zhang et al., 2016). This regime would be significantly more amenable to farmers by providing both economic and environmental incentives, potentially leading to a greater implementation of AMF into agro-ecosystems.
The mycorrhizal synergism with plants gives rise to multiple ecosystem services such as enhanced nutrient cycling, increased plant biomass and improved ecosystem resilience to extreme weather events. Consequently, these interactions can improve buffer functioning, directly contributing to the reduction of groundwater nutrient pollution. These findings suggest that AMF have potential for application in the abatement of N and P losses, particularly at field borders which are vulnerable to runoff and leaching events. Moreover, it is evident the maintenance of mycorrhizal systems improves the nutrient use efficiency of the land, an essential facet of sustainable P management. As P is the limiting factor of freshwater eutrophication, it is critical that this nutrient is preferentially mitigated. Secondly, as P sources are estimated to become increasingly scarce over the next century (Daneshgar et al., 2018), further research into sustainably reusing and reducing P is needed. However, a substantial amount of research is required before AMF can be integrated into a myco-phytoremediation plan. Baseline knowledge of mycorrhizal diversity is limited. Robust taxonomic identification, screening and field trials of native AMF species may reveal individuals with elevated nutrient cycling capabilities. Similarly, most of the literature focuses on the effect of a single AMF species on nutrient uptake, yet this is not entirely representative of plant-fungal interactions in the field. A broad-range species approach (Chen et al., 2017) may enhance the capacity of AMF for biochemical cycling and subsequent buffer functioning. Further investigations into the synergistic relationship between plants, AMF and PBB are also necessary. Bacteria in these systems are responsible for the provision of significant amounts of the nutrients that AMF transfer to plants, yet they are understudied. In short, the integration of AMF into VBSs ultimately has potential to reduce the risk of eutrophication by directly intercepting and storing nutrients, in addition to enhancing buffer performance through improving a VBS’s ability to capture and reuse N and P from agricultural runoff. It is vital that the vegetation is harvested at the end of the growing season to ensure that the nutrients bound in the plant biomass are not released back into the environment. A novel method for reusing these cuttings is their conversion into a sorbent, such as biochar, for nutrient capture.
5 Biochar
Over time, soils that support VBS tend to accumulate high levels of P and N (Fortier et al., 2020). If these areas are not managed properly, they can become sources of diffuse pollution through leaching and decomposition of plant material (Zak et al., 2018). Thus, a surplus of VBS biomass is, in effect, both a solution to nutrient pollution but also a challenge to manage. In place of topsoil remove, an effective measure for mitigating soil P accumulation is to harvest the plant biomass from a VBS (Hille et al., 2019).
The NuReCycle project offers a dual approach to addressing water and soil nutrient pollution by converting VBS biomass to biochar. This process simultaneously manages the retention of soil nutrients by using an enhanced VBS in conjunction with an in-stream biochar reactor, a device that filters dissolved and particulate nutrients that are present in surface waterways. This produces a nutrient-rich biochar that can be repurposed as a fertiliser once near-maximum adsorption capacity is reached (Wang H et al., 2020). This creates a closed, circular system for nutrient capture and recycling in an agricultural setting.
Different carbon categories, such as activated charcoal, biochar and charcoal, share comparable production techniques, raw materials and structures. However, they are differentiated by their respective end uses. Charcoal is primarily used as a fuel source, while biochar is utilised for soil remediation as well as a feed supplement. On the other hand, activated charcoal is employed in the elimination of water and air impurities, in addition to having several medical applications (Man et al., 2021).
Biochar is a char material that is specifically intended for soil amendment purposes. It is produced through the partial oxidation of biomass, typically by heating or combusting biomass while partially or completely blocking oxygen inflow. The end product is a black, porous solid of amorphous carbon that results from thermal decomposition (Guo et al., 2015). Various techniques, such as pyrolysis, hydrothermal carbonization, gasification, torrefaction, and microwave heating have been used to produce biochar from plant-derived organic matter (Mohan et al., 2014; González et al., 2017; Fang et al., 2018). The variety of biomass feedstock used to produce biochar is extensive and includes crop and forest residues, wood chips, algae, sewage sludge, manures, and organic municipal solid waste (Colantoni et al., 2016; Xiong et al., 2019).
Biochar is a versatile resource that is valuable for soil remediation because it enhances multiple factors associated with increased soil fertility. These include nutrient availability, microbial activity, organic matter content and water retention in soil, and crop yields. It is also associated with decreased fertiliser requirements, greenhouse gas emissions, nutrient leaching and soil erosion (Sohi et al., 2009; Woolf et al., 2010). However, despite the potential benefits of biochar, it is important to acknowledge its limitations. The process of producing biochar from biomass can result in the production of by-products such as oil and hydrocarbon gases, which are often discarded in favour of biochar production for soil amendment purposes (Verheijen et al., 2010). Furthermore, the effectiveness of biochar as a soil amending expedient is highly dependent on the compatibility between the soil’s biogeoproperties and the biochemical characteristics of the biochar - if the match is not suitable, the application of biochar can negatively impact soil fertility and the health of the surrounding microbiome (Gorovtsov et al., 2020).
Nevertheless, historical evidence exists on the use of biochar for soil improvement, with the practice dating back several millennia (Denevan, 1995; Brewer et al., 2009). Researchers in the central Amazon forest have found a particularly fertile and characteristically dark soil named Terra Preta do Índio (TPI, or indigenous’ black soil, in Brazilian Portuguese, also named Amazonian Dark Earths, or ADE) (Brewer et al., 2009; Schmidt et al., 2014). Although not originally produced with the intention of amending soil properties, TPI formed as a product of sedentary human settlement in the Amazon forest, during the pre-Columbian era, through piling human and animal waste, ash and charcoal, and ceramics (Woods and McCann, 1999).
The inadvertent formation of anthropogenic soils, such as TPI, extends throughout the Amazon basin and potentially had widespread occurrence in the past (Levis et al., 2018). The exceptional productivity of TPI soil can be attributed, in part, to its significant biochar content and the numerous benefits it provides (Glaser et al., 2001). Therefore, recognizing the potential of biochar, integrating the biomass of VBS as biochar feedstock presents a sustainable alternative for remediating soil and water pollution. By harnessing biochar’s properties to enhance fertiliser and nutrient adsorption, reduce nutrient leaching, and promote the breakdown of pesticides, the use of VBS biomass as biochar can foster soil fertility, support plant growth, and enhance metabolic microbial activity (Varjani et al., 2019).
Using engineered biochar as a sorbent of phosphate from water is considered a relatively novel approach to environmental management practices, presenting a eco-friendly end-of-life alternative to biomass residues (Yao et al., 2013; Zhang et al., 2023). When biochar reaches its maximum load capacity, spent biochar has become enriched with nutrients and can be applied as fertiliser to doubly function as a carbon sink, the fixation of carbon in a stable form that would otherwise be released as CO2 via decomposition, and a soil remediation agent (Lan et al., 2021).
Pristine biochar has a limited capacity to adsorb anionic species, such as orthophosphate (PO43-) and nitrate (NO3−) due to its alkaline properties: functional groups at its surface structure create a repelling net negative charge (Beesley et al., 2011; Zhang et al., 2013). Engineered biochars were produced to improve its physico-chemical properties compared to conventional pristine biochar (Xiang et al., 2019). However, more research is required to fill the gap in understanding the relationship between engineering biochar and its sorption of differently charged nutrient forms (Yao et al., 2013).
Biochar interacts with pollutant species differently, based on its surface composition and structure and on pollutants’ chemical properties (Figure 5) (Li et al., 2017; El-Naggar et al., 2019; Premarathna et al., 2019; Cheng et al., 2021; Marcińczyk et al., 2022). Studies have demonstrated that biochar’s ability to remove pollutants from water and soil is associated with its particle size (Chen et al., 2017). Parameters like particle size, shape, and internal structure are crucial factors that influence biochar’s porous structure properties and performance (Liu Z et al., 2017). Biochar consists of a complex structure of interconnected micropores, mesopores, and macropores of varying sizes (Lian and Xing, 2017). The production technique and engineered modifications determine the pore size and surface structure of biochar (Guo et al., 2022; Deng et al., 2023).
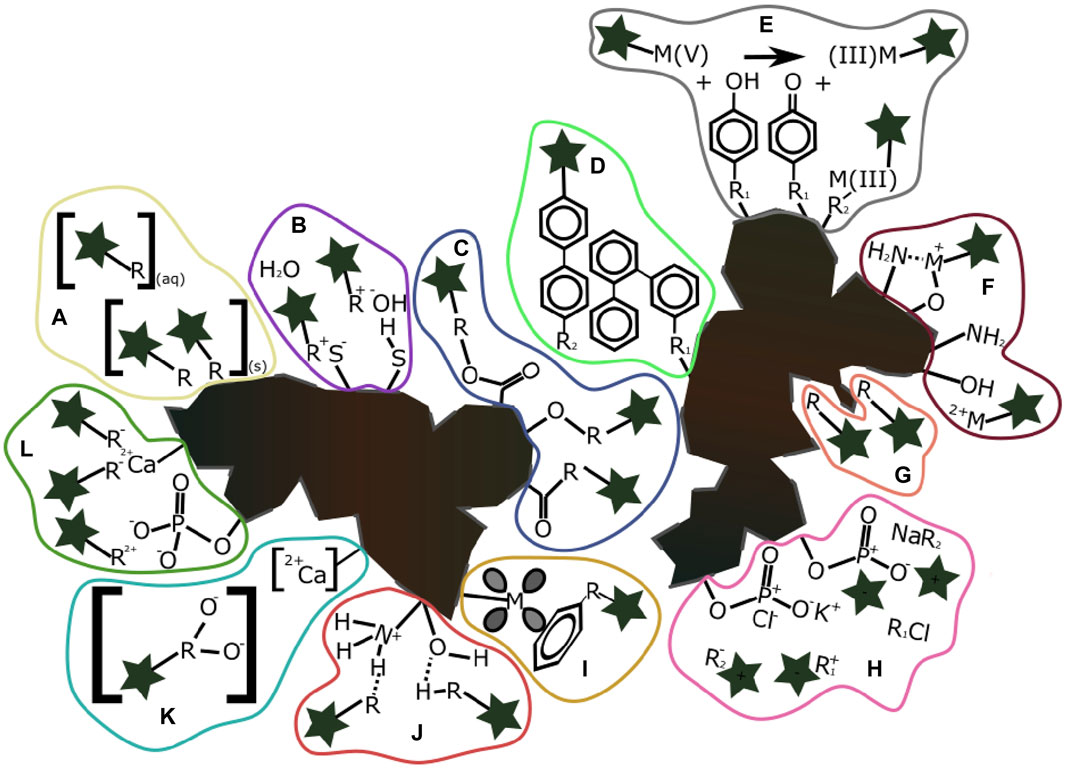
Figure 5. Examples of chemical interactions and reactions responsible for biochar’s ability to remediate soil and water (Li et al., 2017; El-Naggar et al., 2019; Premarathna et al., 2019; Cheng et al., 2021; Marciticzyk, Ok and Oleszczuk, 2022). (A) precipitation reaction; (B) acid-base reaction; (C) oxygen functional groups organic reactions; (D) hydrophobic interactions; (E) redox reactions; (F) complex reactions; (G) physisorption; (H) ion exchange reactions; 0 it-it reactions; (J) hydrogen bonds; (K) ionic interactions; (L) electrostatic interactions. “M” stands for any given metal and “R” stands for any given heteroatom. The stars represent the pollutant’s chemical structure while biochar particles are represented by the two irregular shapes in the centre.
Various methods are available to achieve the required temperature and pressure for thermal decomposition of biomass, leading to carbonisation (Supplementary Table S3). Provided with enough heat, biomass can undergo thermochemical decomposition under anoxic or hypoxic conditions. The products of a pyrolytic reaction are threefold: a solid (biochar), a liquid (bio-oil), and synthetic gases (or syngas: a mixture of gases that include, for example, hydrogen, methane, carbon monoxide and dioxide) (Ong et al., 2019). Pyrolysis results are heavily reliant on operating parameters like heating rate, residence time, and operating temperature (Xiang et al., 2020): biochar yield is optimised when the operating temperature remains under 450°C, whereas for a greater yield of bio-oil, a temperature range of 450°C–800°C is desired, and operating temperatures above 800°C typically result in an increased decomposition of biomass and subsequent formation of non-condensable hydrocarbon gases (Tomczyk et al., 2020). Both residence time and heating rate have a combined effect on biochar yield, as increasing the reaction time at a low heating rate (slow pyrolysis) can yield an average of 35% of the initial dry biomass weight in biochar, while a high heating rate and reduced residence time (fast pyrolysis) tends to make the production of bio-oil and syngas more efficient (Tomczyk et al., 2020).
Upon thermally converting biomass to char, the obtained solid is labelled pristine biochar. The term ‘engineered biochar’, in contrast to ‘pristine biochar’, is used to define biochar that has been modified or activated via physical, chemical or biological approaches to better suit environmental applications (Kazemi Shariat Panahi et al., 2020). Biochar’s capacity to act as a filter for water pollutants is a function of its physico-chemical properties, the production method and feedstock used, in addition to the various mechanisms for the different interactions between biochar surface and natural wastewater dissolved compounds or suspended particles (Qiu et al., 2022). Various strategies have been devised for the modification of biochar (Huang et al., 2021): one approach involves pre-treatment of the dry biomass, wherein the biomass is subjected to treatment before pyrolysis; a second approach is post-treatment of dry biochar, which entails subjecting the biomass to pyrolysis first and then subsequently modifying the resulting char; a third approach involves the simultaneous mixing of the biomass and treatment reagents, facilitating both pyrolysis and modification reactions to occur concurrently.
The use of biochar in environmental applications, particularly in agricultural wastewater treatment, is limited by its properties. Pristine biochars typically have a negatively charged surface and lack acidic functional groups on the surface (Marcińczyk et al., 2022). Thus, pristine biochar is not effective in adsorbing anionic nutrient leachate from soils, such as nitrate and phosphate, without further modification (Qiu et al., 2022). As a result of the limited adsorption performance of pristine biochar, several engineering approaches have been developed to improve its ability to remove pollutants and enhance its overall efficiency (Li et al., 2017; El-Naggar et al., 2019; Premarathna et al., 2019; Wang L et al., 2020; Cheng et al., 2021; Huang et al., 2021; Marcińczyk et al., 2022; Wang L et al., 2022). Over the last 5 years, steam activation was utilised in 6% of the 15,802 published papers gathered using Google Scholar and Scopus search engines, whereas ball milling activation was used in 12%. The most prevalent designed biochar alteration is acid treatment (24%), with alkaline treatments accounting for 10% of total treatments. The most unique alteration employed methanol for biochar activation (0.1% of total treatments). A sunburst diagram (Figure 6) was produced to provide a brief summary of the common modifications published.
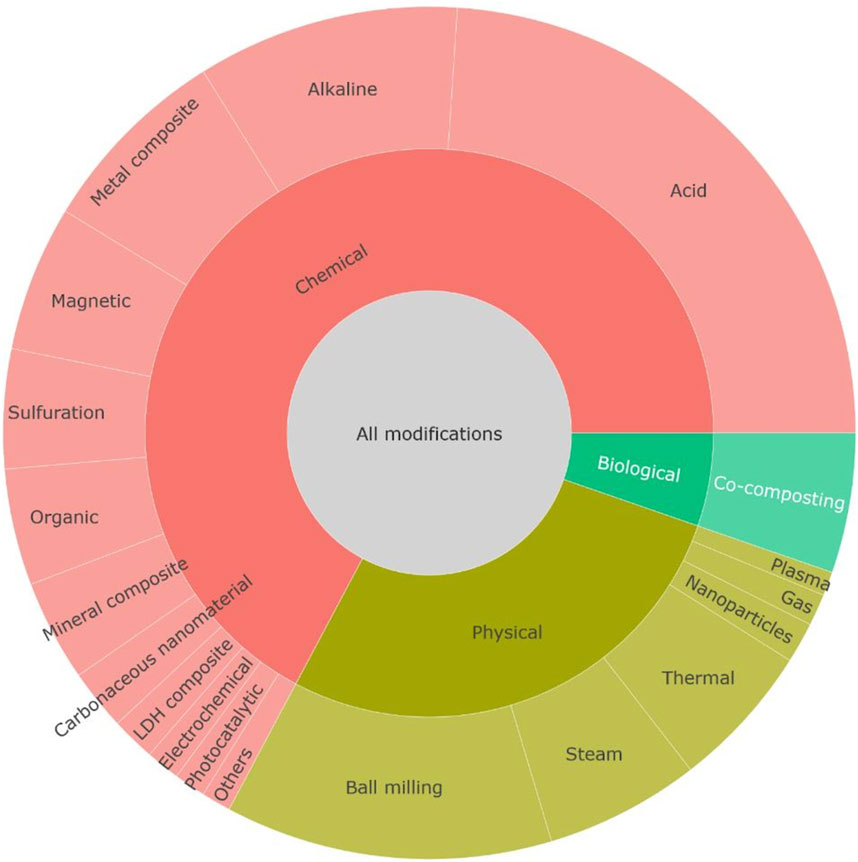
Figure 6. A sunburst diagram of engineered biochar treatments and modification types (Li et al., 2017; El-Naggar et al., 2019; Premarathna et al., 2019; L. Wang et al., 2020, 2022; Cheng et al., 2021; Huang, Lee and Huang, 2021; Marcińczyk, Ok and Oleszczuk, 2022). The category “Others”, under chemical modifications, includes nitrogenation, oxidant, micro- organism composites and methanol modifications; metal composite category includes both metal salt and metal oxides. LDH stands for layered double hydroxide. Under physical modifications, the nanoparticle category refers to the use of deposited nanoparticles as activators.
While engineered biochar usage as a soil amendment technique has been extensively studied (Wang C et al., 2022), the interactions between soil living organisms and biochar remain complex and often contentious, with gaps in research knowledge (Gorovtsov et al., 2020). A healthy soil ecosystem is fundamental to the growth and survival of flora and microbiota, thus, beneficial biotic-abiotic interactions are fundamental for the regulation of life in the ecosystem (Tan et al., 2022).
The application of biochar to soils can improve soil microbial diversity, abundance, and mobility, leading to enhanced resilience to management practices (Nepal et al., 2023). Biochar can boost the suppression of soil pathogens in crops by improving soil biological quality through modifying plant root exudate composition, changing soil microbiochemical properties, and inducing mechanisms for plants’ systemic defences (Alaylar et al., 2021). Additionally, biochar-treated soil may provide a more favourable medium for beneficial soil bacteria to thrive, as demonstrated by the increased abundance of soil organic matter and micronutrients in lentils inoculated with rhizobia (Ali et al., 2022).
Biochar is thought to provide a fitting environment for soil microbes due to its effects on soils including enhanced soil aeration, increased water content, decreased soil compaction, pH remediation (Laghari et al., 2016). Nonetheless, inherent properties like surface area, degree of porosity, electric charge and surface functional groups can trigger positive/negative responses in soil microbiome (Zhu et al., 2017). Furthermore, several fungal species can digest biochar by producing a range of extracellular enzymes (Liu et al., 2021). However, biochar has been found to have negative effects on the growth of AMF, resulting in a reduction in their populations in the soil (Birk et al., 2009; Mukherjee et al., 2014; Liu C et al., 2017). Other studies found that biochar, when combined with AMF inoculation, has resulted in increased plant growth (Akhter et al., 2015) and bacterial abundance (Huang et al., 2017). Therefore, it is important to provide the appropriate type of biochar to the relevant soil type in order to maximise soil quality.
Filling the present knowledge gaps regarding nutrient pollution in agroecosystems necessitates a holistic methodology. A more thorough examination of the synergies between plants, biochar, AMF, and PBB is required for optimal agroecological management. Future studies should focus on determining when biochar is most beneficial to soil and microbiome health, including understanding its long-term persistence, determining optimal application rates under varied biochar-soil-plant environmental conditions, and elucidating specific mechanisms influencing soil biotic properties. Further research on this topic will progress with the ever-increasing awareness of environmental issues globally, particularly the ones faced by natural water bodies today, which provide a major driver for the development of novel technologies.
6 Conclusion and future perspective
Water pollution from agricultural activities is a global issue, but its impacts and systems can be studied on a local scale. VBSs have been shown to effectively mitigate agricultural pollution, though they do experience a host of limitations. In light of this, the integration of a myco-phytoremediation strategy into VBSs emerges as a promising approach to improve the sustainability of agricultural systems. As of now, the research into the myco-phytoremediation of N and P in VBSs is limited. Existing studies have primarily focused on utilising AMF and PBB to enhance plant growth and facilitate N and P uptake when soil concentrations are low (Hestrin et al., 2019; Yu et al., 2022). There is a need to identify the factors that encourage or hinder the microbial interactions and synergy between plants, AMF and PBB to optimising their use in VBSs. Further investigations into the identification of AMF and PBB species is required prior to further characterise and enhance this synergistic approach. Although successful manipulation of the soil microbiome from VBSs could lead to commercial seed mixes inoculated with AMF and PBB, the transition from greenhouse to field trails has shown variable and inconsistent results (Chiaranunt and White, 2023). Another potential application for AMF and PBB is the reduction of fertiliser requirements for plants in agricultural fields via improved plant nutrient acquisition. This not only reduces our reliance on chemical fertiliser but also provides farmers with an economic incentive by lowering input costs.
Although VBSs and biochar have been extensively studied as primary forms of nutrient abatement, the focus has largely been on short-term impacts, leaving a crucial knowledge gap regarding their long-term effectiveness. To comprehensively understand the implications of biochar application, extensive field research is needed that encompasses nutrient and water use efficiency, net carbon sequestration, greenhouse gas emissions, and changes in soil quality over extended periods. Despite significant efforts, effective treatment methods for nutrient remediation on agricultural drainage networks remain an ongoing challenge. In this regard, combined multidisciplinary approaches can prove to be an effective way forward. The interdisciplinary approach as supported by the findings of this literature review, offers a promising strategy for mitigating agricultural pollution and serves as a potential model for environmental remediation initiatives worldwide. Addressing these pressing concerns demands continued collaborative efforts and sustained research in order to safeguard the environment and human wellbeing of the future.
Author contributions
LQ: Conceptualization, Data curation, Formal Analysis, Investigation, Methodology, Validation, Visualization, Writing–original draft, Writing–review and editing. DF: Conceptualization, Data curation, Formal Analysis, Investigation, Methodology, Validation, Visualization, Writing–original draft, Writing–review and editing. BJ: Conceptualization, Data curation, Formal Analysis, Investigation, Methodology, Visualization, Writing–original draft, Writing–review and editing. GC: Conceptualization, Data curation, Formal Analysis, Investigation, Methodology, Validation, Visualization, Writing–original draft, Writing–review and editing. MM: Conceptualization, Formal Analysis, Software, Validation, Visualization, Writing–original draft, Writing–review and editing, Funding acquisition, Resources. TH Resources, Writing–review and editing. DT: Resources, Writing–review and editing. LG: Resources, Writing–review and editing. DO’C: Resources, Writing–review and editing, Conceptualization, Formal Analysis, Funding acquisition, Investigation, Methodology, Project administration, Supervision, Validation, Visualization, Writing–original draft.
Funding
The author(s) declare that financial support was received for the research, authorship, and/or publication of this article. The funding was received from Kinsella Foundation through the Kinsella Challenge-Based E3 Multi-Disciplinary Project Awards at Trinity College Dublin on the project NuReCycle. The details are given and there were only 4 of these awards given out in 2021.
Acknowledgments
The authors would like to acknowledge the generous financial support of the Kinsella Foundation and the support of the TCD E3 team. We would also like to thank Katja Bruisch from the Trinity Centre for Environmental Humanities, School of History and Humanities at Trinity College Dublin for her valuable insights and advice. In addition, we extend our gratitude to the technical and administrative staff in the School of Natural Sciences, School of Genetics and Microbiology, and especially the School of Engineering at Trinity College Dublin.
Conflict of interest
The authors declare that the research was conducted in the absence of any commercial or financial relationships that could be construed as a potential conflict of interest.
Publisher’s note
All claims expressed in this article are solely those of the authors and do not necessarily represent those of their affiliated organizations, or those of the publisher, the editors and the reviewers. Any product that may be evaluated in this article, or claim that may be made by its manufacturer, is not guaranteed or endorsed by the publisher.
Supplementary material
The Supplementary Material for this article can be found online at: https://www.frontiersin.org/articles/10.3389/fenvs.2024.1340565/full#supplementary-material
Abbreviations
P, Phosphorus; N, Nitrogen; TP, Total Phosphorus; TN, Total Nitrogen; VBS, Vegetative Buffer Strip; AMF, Arbuscular Mycorrhizal Fungi; TSS, Total Suspended Solids; PBB, Plant Beneficial Bacteria; PSB, Phosphate Solubilizing Bacteria; MHB, Mycorrhizal Helper Bacteria; PF, Preferential Flow; NPS, Non-point source.
References
Alaylar, B., Güllüce, M., Egamberdieva, D., Wirth, S., and Bellingrath-Kimura, S. D. (2021). Biochar mediated control of soil-borne phytopathogens. Environ. Sustain. 4 (2), 329–334. doi:10.1007/s42398-021-00187-5
Ali, A., Ahmad, W., Munsif, F., Khan, A., Nepal, J., Wójcik-Gront, E., et al. (2022). Residual effect of finely-ground biochar inoculated with bio-fertilization impact on productivity in a lentil–maize cropping system. Agronomy 12 (9), 2036. doi:10.3390/agronomy12092036
Allaire, S. E., Sylvain, C., Lange, S. F., Thériault, G., and Lafrance, P. (2015). Potential efficiency of riparian vegetated buffer strips in intercepting soluble compounds in the presence of subsurface preferential flows. PloS one 10 (7), e0131840. doi:10.1371/journal.pone.0131840
Allen, M. F. (2011). Linking water and nutrients through the vadose zone: a fungal interface between the soil and plant systems. J. Arid Land 3 (3), 155–163. doi:10.3724/SP.J.1227.2011.00155
Alves, A. R. A., Yin, Q., Oliveira, R., Silva, E. F., and Novo, L. A. (2022). Plant growth-promoting bacteria in phytoremediation of metal-polluted soils: current knowledge and future directions. Sci. Total Environ. 838, 156435. doi:10.1016/j.scitotenv.2022.156435
Balzergue, C., Chabaud, M., Barker, D. G., Bécard, G., and Rochange, S. F. (2013). High phosphate reduces host ability to develop arbuscular mycorrhizal symbiosis without affecting root calcium spiking responses to the fungus. Front. Plant Sci. 4, 426. doi:10.3389/fpls.2013.00426
Barçante, B., Nascimento, N. O., Silva, T. F., Reis, L. A., and Giani, A. (2020). Cyanobacteria dynamics and phytoplankton species richness as a measure of waterbody recovery: response to phosphorus removal treatment in a tropical eutrophic reservoir. Ecol. Indic. 117, 106702. doi:10.1016/j.ecolind.2020.106702
Barling, R. D., and Moore, I. D. (1994). Role of buffer strips in management of waterway pollution: a review. Environ. Manag. 18, 543–558. doi:10.1007/bf02400858
Beesley, L., Moreno-Jiménez, E., Gomez-Eyles, J. L., Harris, E., Robinson, B., and Sizmur, T. (2011). A review of biochars’ potential role in the remediation, revegetation and restoration of contaminated soils. Environ. Pollut. 159 (12), 3269–3282. doi:10.1016/j.envpol.2011.07.023
Beltran-Medina, I., Romero-Perdomo, F., Molano-Chavez, L., Gutiérrez, A. Y., Silva, A. M. M., and Estrada-Bonilla, G. (2023). Inoculation of phosphate-solubilizing bacteria improves soil phosphorus mobilization and maize productivity. Nutrient Cycl. Agroecosyst. 126 (1), 21–34. doi:10.1007/s10705-023-10268-y
Bender, S. F., Conen, F., and Van der Heijden, M. G. (2015). Mycorrhizal effects on nutrient cycling, nutrient leaching and N2O production in experimental grassland. Soil Biol. Biochem. 80, 283–292. doi:10.1016/j.soilbio.2014.10.016
Bhanse, P., Kumar, M., Singh, L., Awasthi, M. K., and Qureshi, A. (2022). Role of plant growth-promoting rhizobacteria in boosting the phytoremediation of stressed soils: opportunities, challenges, and prospects. Chemosphere 303, 134954. doi:10.1016/j.chemosphere.2022.134954
Bielenberg, A., and Ryan, R. (2016). An economic history of Ireland since independence. London: Routledge.
Birk, J., Steiner, C., Teixiera, W., Zech, W., and Glaser, B. (2009). “Microbial response to charcoal amendments and fertilization of a highly weathered tropical soil,” in Amazonian dark Earths: wim sombroek’s vision. Editor W. I. Woods (Dordrecht: Springer Netherlands), 309–324. doi:10.1007/978-1-4020-9031-8_16
Borin, M., Passoni, M., Thiene, M., and Tempesta, T. (2010). Multiple functions of buffer strips in farming areas. Eur. J. Agron. 32 (1), 103–111. doi:10.1016/j.eja.2009.05.003
Borin, M., Vianello, M., Morari, F., and Zanin, G. (2005). Effectiveness of buffer strips in removing pollutants in runoff from a cultivated field in North-East Italy. Agric. Ecosyst. Environ. 105 (1–2), 101–114. doi:10.1016/j.agee.2004.05.011
Brewer, C. E., Schmidt-Rohr, K., Satrio, J. A., and Brown, R. C. (2009). Characterization of biochar from fast pyrolysis and gasification systems. Environ. Prog. Sustain. Energy 28 (3), 386–396. doi:10.1002/ep.10378
Bunting, L., Leavitt, P., Simpson, G., Wissel, B., Laird, K., Cumming, B., et al. (2016). Increased variability and sudden ecosystem state change in Lake Winnipeg, Canada, caused by 20th century agriculture. Limnol. Oceanogr. 61 (6), 2090–2107. doi:10.1002/lno.10355
Chen, J., Li, S., Liang, C., Xu, Q., Li, Y., Qin, H., et al. (2017). Response of microbial community structure and function to short-term biochar amendment in an intensively managed bamboo (Phyllostachys praecox) plantation soil: effect of particle size and addition rate. Sci. Total Environ. 574, 24–33. doi:10.1016/j.scitotenv.2016.08.190
Cheng, N., Wang, B., Wu, P., Lee, X., Xing, Y., Chen, M., et al. (2021). Adsorption of emerging contaminants from water and wastewater by modified biochar: a review. Environ. Pollut. 273, 116448. doi:10.1016/j.envpol.2021.116448
Chiaranunt, P., and White, J. F. (2023). Plant beneficial bacteria and their potential applications in vertical farming systems. Plants 12 (2), 400. doi:10.3390/plants12020400
Colantoni, A., Evic, N., Lord, R., Retschitzegger, S., Proto, A., Gallucci, F., et al. (2016). Characterization of biochars produced from pyrolysis of pelletized agricultural residues. Renew. Sustain. Energy Rev. 64, 187–194. doi:10.1016/j.rser.2016.06.003
Cooper, M., and Davis, J. (2004). The Irish fertiliser industry: a history. Dublin: Irish Academic Press.
Corkidi, L., Merhaut, D. J., Allen, E. B., Downer, J., Bohn, J., and Evans, M. (2011). Effects of mycorrhizal colonization on nitrogen and phosphorus leaching from nursery containers. HortScience 46 (11), 1472–1479. doi:10.21273/hortsci.46.11.1472
Crowley, E. (2003). The evolution of the Common Agricultural Policy and social differentiation in rural Ireland. Econ. Soc. Rev. 34 (1), 65–85.
Cushman, G. T. (2013). Guano and the opening of the Pacific world: a global ecological history. Cambridge, New York: Cambridge University Press.
Daly, M. E. (2002). The first department: a history of the Department of Agriculture. Dublin: Institute of Public Administration.
Daneshgar, S., Callegari, A., Capodaglio, A., and Vaccari, D. (2018). The potential phosphorus crisis: resource conservation and possible escape technologies: a review. Resources 7 (2), 37. doi:10.3390/resources7020037
Das, P. P., Singh, K. R., Nagpure, G., Mansoori, A., Singh, R. P., Ghazi, I. A., et al. (2022). Plant-soil-microbes: a tripartite interaction for nutrient acquisition and better plant growth for sustainable agricultural practices. Environ. Res. 214, 113821. doi:10.1016/j.envres.2022.113821
Denevan, W. M. (1995). “2 Prehistoric agricultural methods as models for sustainability,” in Advances in plant pathology. Editors J. H. Andrews, and I. C. Tommerup (Academic Press), 21–43.
Deng, L., Zhao, Y., Sun, S., Feng, D., and Zhang, W. (2023). Thermochemical method for controlling pore structure to enhance hydrogen storage capacity of biochar. Int. J. Hydrogen Energy 48, 21799–21813. doi:10.1016/j.ijhydene.2023.03.084
Djighaly, P. I., Ndiaye, S., Diarra, A. M., and Dramé, F. A. (2020). Inoculation with arbuscular mycorrhizal fungi improves salt tolerance in C. glauca (Sieb). J. Mat. Environ. Sci. 11, 1616–1625.
Dosskey, M. G. (2001). Toward quantifying water pollution abatement in response to installing buffers on crop land. Environ. Manag. 28 (5), 577–598. doi:10.1007/s002670010245
Duchemin, M., and Hogue, R. (2009). Reduction in agricultural non-point source pollution in the first year following establishment of an integrated grass/tree filter strip system in southern Quebec (Canada). Agric. Ecosyst. Environ. 131 (1–2), 85–97. doi:10.1016/j.agee.2008.10.005
Dunn, R. M., Hawkins, J. M. B., Blackwell, M. S. A., Zhang, Y., and Collins, A. L. (2022). Impacts of different vegetation in riparian buffer strips on runoff and sediment loss. Hydrol. Process. 36 (11), e14733. doi:10.1002/hyp.14733
El-Naggar, A., Lee, S. S., Rinklebe, J., Farooq, M., Song, H., Sarmah, A. K., et al. (2019). Biochar application to low fertility soils: a review of current status, and future prospects. Geoderma 337, 536–554. doi:10.1016/j.geoderma.2018.09.034
Emami, S., Alikhani, H. A., Pourbabaei, A. A., Etesami, H., Sarmadian, F., and Motessharezadeh, B. (2019). Effect of rhizospheric and endophytic bacteria with multiple plant growth promoting traits on wheat growth. Environ. Sci. Pollut. Res. 26 (19), 19804–19813. doi:10.1007/s11356-019-05284-x
Environment, U. N. (2019). Frontiers 2018/19: emerging issues of environmental concern. Available at: http://www.unep.org/resources/frontiers-201819-emerging-issues-environmental-concern (Accessed April 6, 2023).
Etesami, H., Jeong, B. R., and Glick, B. R. (2021). Contribution of arbuscular mycorrhizal fungi, phosphate–solubilizing bacteria, and silicon to P uptake by plant. Front. Plant Sci. 12, 699618. doi:10.3389/fpls.2021.699618
European waters (2018). European waters - assessment of status and pressures. Publication 7. Copenhagen, Denmark: European Environment Agency. Available at: https://www.eea.europa.eu/publications/state-of-water (Accessed April 6, 2023).
Fall, A. F., Nakabonge, G., Ssekandi, J., Founoune-Mboup, H., Apori, S. O., Ndiaye, A., et al. (2022). Roles of arbuscular mycorrhizal fungi on soil fertility: contribution in the improvement of physical, chemical, and biological properties of the soil. Front. Fungal Biol. 3, 723892. doi:10.3389/ffunb.2022.723892
Fang, J., Zhan, L., Ok, Y. S., and Gao, B. (2018). Minireview of potential applications of hydrochar derived from hydrothermal carbonization of biomass. J. Industrial Eng. Chem. 57, 15–21. doi:10.1016/j.jiec.2017.08.026
Feehan, J. (2003). Farming in Ireland: history, heritage and environment. Dublin: University College Dublin, Faculty of Agriculture.
Fitriatin, B. N., Mulyani, O., Herdiyantoro, D., Alahmadi, T. A., and Pellegrini, M. (2022). Metabolic characterization of phosphate solubilizing microorganisms and their role in improving soil phosphate solubility, yield of upland rice (Oryza sativa L.), and phosphorus fertilizers efficiency. Front. Sustain. Food Syst. 6. doi:10.3389/fsufs.2022.1032708
Fortier, J., Truax, B., Gagnon, D., and Lambert, F. (2020). Soil nutrient availability and microclimate are influenced more by genotype than by planting stock type in hybrid poplar bioenergy buffers on farmland. Ecol. Eng. 157, 105995. doi:10.1016/j.ecoleng.2020.105995
Frey-Klett, P., Garbaye, J., and Tarkka, M. (2007). The mycorrhiza helper bacteria revisited. New Phytol. 176 (1), 22–36. doi:10.1111/j.1469-8137.2007.02191.x
Glaser, B., Haumaier, L., Guggenberger, G., and Zech, W. (2001). The “Terra Preta” phenomenon: a model for sustainable agriculture in the humid tropics. Naturwissenschaften 88 (1), 37–41. doi:10.1007/s001140000193
González, M. E., Cea, M., Reyes, D., Romero-Hermoso, L., Hidalgo, P., Meier, S., et al. (2017). Functionalization of biochar derived from lignocellulosic biomass using microwave technology for catalytic application in biodiesel production. Energy Convers. Manag. 137, 165–173. doi:10.1016/j.enconman.2017.01.063
Gorman, H. S. (2013). The story of N: a social history of the nitrogen cycle and the challenge of sustainability. New Brunswick, N.J.: Rutgers University Press.
Gorovtsov, A. V., Minkina, T. M., Mandzhieva, S. S., Perelomov, L. V., Soja, G., Zamulina, I. V., et al. (2020). The mechanisms of biochar interactions with microorganisms in soil. Environ. Geochem. Health 42 (8), 2495–2518. doi:10.1007/s10653-019-00412-5
Guignard, M. S., Leitch, A. R., Acquisti, C., Eizaguirre, C., Elser, J. J., Hessen, D. O., et al. (2017). Impacts of nitrogen and phosphorus: from genomes to natural ecosystems and agriculture. Front. Ecol. Evol. 5, doi:10.3389/fevo.2017.00070
Guo, M., He, Z., and Uchimiya, S. M. (2015). in Introduction to biochar as an agricultural and environmental amendment. Editors M. Guo, Z. He, and S. M. Uchimiya (Madison, WI, USA: American Society of Agronomy and Soil Science Society of America), 1–14.
Guo, T., Tian, W., and Wang, Y. (2022). Effect of pore structure on CO2 adsorption performance for ZnCl2/FeCl3/H2O(g) Co-activated walnut shell-based biochar. Atmosphere 13 (7), 1110. doi:10.3390/atmos13071110
Harmel, R. D., Smith, D., Haney, R., and Dozier, M. (2009). Nitrogen and phosphorus runoff from cropland and pasture fields fertilized with poultry litter. J. Soil Water Conservation 64 (6), 400–412. doi:10.2489/jswc.64.6.400
Hayat, R., Ali, S., Amara, U., Khalid, R., and Ahmed, I. (2010). Soil beneficial bacteria and their role in plant growth promotion: a review. Ann. Microbiol. 60 (4), 579–598. doi:10.1007/s13213-010-0117-1
He, Y., Yang, R., Lei, G., Li, M., Li, T., Zhan, F., et al. (2021). Arbuscular mycorrhizal fungus–induced decrease in phosphorus loss due to leaching in red soils under simulated heavy rainfall. J. soils sediments 21 (2), 881–889. doi:10.1007/s11368-020-02849-z
Helmers, M. J., Isenhart, T. M., Dosskey, M., Dabney, S. M., and Strock, J. S. (2008). Buffers and vegetative filter strips.
Hestrin, R., Hammer, E. C., Mueller, C. W., and Lehmann, J. (2019). Synergies between mycorrhizal fungi and soil microbial communities increase plant nitrogen acquisition. Commun. Biol. 2 (1), 233. doi:10.1038/s42003-019-0481-8
Hickey, M. B. C., and Doran, B. (2004). A review of the efficiency of buffer strips for the maintenance and enhancement of riparian ecosystems. Water Qual. Res. J. 39 (3), 311–317. doi:10.2166/wqrj.2004.042
Hijri, M. (2016). Analysis of a large dataset of mycorrhiza inoculation field trials on potato shows highly significant increases in yield. Mycorrhiza 26 (3), 209–214. doi:10.1007/s00572-015-0661-4
Hille, S., Graeber, D., Kronvang, B., Rubæk, G. H., Onnen, N., Molina-Navarro, E., et al. (2019). Management options to reduce phosphorus leaching from vegetated buffer strips. J. Environ. Qual. 48 (2), 322–329. doi:10.2134/jeq2018.01.0042
Houses of the Oireachtas (1991). Environmental protection bill, 1990 [seanad]: second stage (resumed).
Hu, Y., Gao, L., Ma, C., Wang, H., and Zhou, C. (2023). The comprehensive reduction capacity of five riparian vegetation buffer strips for primary pollutants in surface runoff. Appl. Sci. 13 (6), 3898. doi:10.3390/app13063898
Huang, D., Liu, L., Zeng, G., Xu, P., Huang, C., Deng, L., et al. (2017). The effects of rice straw biochar on indigenous microbial community and enzymes activity in heavy metal-contaminated sediment. Chemosphere 174, 545–553. doi:10.1016/j.chemosphere.2017.01.130
Huang, W.-H., Lee, D.-J., and Huang, C. (2021). Modification on biochars for applications: a research update. Bioresour. Technol. 319, 124100. doi:10.1016/j.biortech.2020.124100
Jeng, A. S., and Vagstad, N. (2009). Potential nitrogen and phosphorus leaching from soils fertilized with meat and bone meal. Acta Agric. Scand. Sect. B - Plant Soil Sci. 59 (3), 238–245. doi:10.1080/09064710802024164
Kavian, A., Saleh, I., Habibnejad, M., Brevik, E. C., Jafarian, Z., and Rodrigo-Comino, J. (2018). Effectiveness of vegetative buffer strips at reducing runoff, soil erosion, and nitrate transport during degraded hillslope restoration in northern Iran. Land Degrad. Dev. 29 (9), 3194–3203. doi:10.1002/ldr.3051
Kazemi Shariat Panahi, H., Dehhaghi, M., Ok, Y. S., Nizami, A. S., Khoshnevisan, B., Mussatto, S. I., et al. (2020). A comprehensive review of engineered biochar: production, characteristics, and environmental applications. J. Clean. Prod. 270, 122462. doi:10.1016/j.jclepro.2020.122462
Kieta, K. A., Owens, P. N., Lobb, D. A., Vanrobaeys, J. A., and Flaten, D. N. (2018). Phosphorus dynamics in vegetated buffer strips in cold climates: a review. Environ. Rev. 26 (3), 255–272. doi:10.1139/er-2017-0077
M. Kumar, D. D. Snow, and R. Honda (2020). Emerging issues in the water environment during anthropocene: a south east asian perspective (Singapore: Springer Singapore). doi:10.1007/978-981-32-9771-5
Laghari, M., Naidu, R., Xiao, B., Hu, Z., Mirjat, M. S., Hu, M., et al. (2016). Recent developments in biochar as an effective tool for agricultural soil management: a review. J. Sci. Food Agric. 96 (15), 4840–4849. doi:10.1002/jsfa.7753
Lan, Y., Du, Q., Tang, C., Cheng, K., and Yang, F. (2021). Application of typical artificial carbon materials from biomass in environmental remediation and improvement: a review. J. Environ. Manag. 296, 113340. doi:10.1016/j.jenvman.2021.113340
Levis, C., Flores, B. M., Moreira, P. A., Luize, B. G., Alves, R. P., Franco-Moraes, J., et al. (2018). How people domesticated amazonian forests. Front. Ecol. Evol. 5. doi:10.3389/fevo.2017.00171
Li, H., Dong, X., da Silva, E. B., de Oliveira, L. M., Chen, Y., and Ma, L. Q. (2017). Mechanisms of metal sorption by biochars: biochar characteristics and modifications. Chemosphere 178, 466–478. doi:10.1016/j.chemosphere.2017.03.072
Lian, F., and Xing, B. (2017). Black carbon (biochar) in water/soil environments: molecular structure, sorption, stability, and potential risk. Environ. Sci. Technol. 51 (23), 13517–13532. doi:10.1021/acs.est.7b02528
Liang, J., Klingl, A., Lin, Y. Y., Boul, E., Thomas-Oates, J., and Marín, M. (2019). A subcompatible rhizobium strain reveals infection duality in Lotus. J. Exp. Bot. 70 (6), 1903–1913. doi:10.1093/jxb/erz057
Liu, C., Liu, F., Ravnskov, S., Rubæk, G. H., Sun, Z., and Andersen, M. N. (2017). Impact of wood biochar and its interactions with mycorrhizal fungi, phosphorus fertilization and irrigation strategies on potato growth. J. Agron. Crop Sci. 203 (2), 131–145. doi:10.1111/jac.12185
Liu, L., Li, J., Wu, G., Shen, H., Fu, G., and Wang, Y. (2021). Combined effects of biochar and chicken manure on maize (Zea mays L.) growth, lead uptake and soil enzyme activities under lead stress. PeerJ 9, e11754. doi:10.7717/peerj.11754
Liu, Z., Dugan, B., Masiello, C. A., and Gonnermann, H. M. (2017). Biochar particle size, shape, and porosity act together to influence soil water properties. PLOS ONE 12 (6), e0179079. doi:10.1371/journal.pone.0179079
Lv, J., and Wu, Y. (2021). Nitrogen removal by different riparian vegetation buffer strips with different stand densities and widths. Water Supply 21 (7), 3541–3556. doi:10.2166/ws.2021.119
Man, K. Y., Chow, K. L., Man, Y. B., Mo, W. Y., and Wong, M. H. (2021). Use of biochar as feed supplements for animal farming. Crit. Rev. Environ. Sci. Technol. 51 (2), 187–217. doi:10.1080/10643389.2020.1721980
Marcińczyk, M., Ok, Y. S., and Oleszczuk, P. (2022). From waste to fertilizer: nutrient recovery from wastewater by pristine and engineered biochars. Chemosphere 306, 135310. doi:10.1016/j.chemosphere.2022.135310
Martínez-García, L. B., De Deyn, G. B., Pugnaire, F. I., Kothamasi, D., and van der Heijden, M. G. A. (2017). Symbiotic soil fungi enhance ecosystem resilience to climate change. Glob. Change Biol. 23 (12), 5228–5236. doi:10.1111/gcb.13785
McKittrick, M. (2012). “Industrial agriculture,” in A companion to global environmental history. Editors J. R. McNeill, and E. S. Mauldin (Oxford: Wiley-Blackwell).
Melillo, E. D. (2012). The first green revolution: debt peonage and the making of the nitrogen fertilizer trade, 1840–1930. Am. Hist. Rev. 117 (4), 1028–1060. doi:10.1093/ahr/117.4.1028
Mícheál, Ó. F. (2021). Developing rural Ireland: a history of the Irish agricultural advisory service. Dublin: Wordwell.
Mohan, D., Sarswat, A., Ok, Y. S., and Pittman, C. U. (2014). Organic and inorganic contaminants removal from water with biochar, a renewable, low cost and sustainable adsorbent – a critical review. Bioresour. Technol. 160, 191–202. doi:10.1016/j.biortech.2014.01.120
Mukherjee, A., and Lal, R. (2014). The biochar dilemma. Soil Res. 52 (3), 217–230. doi:10.1071/SR13359
Nacoon, S., Jogloy, S., Riddech, N., Mongkolthanaruk, W., Ekprasert, J., Cooper, J., et al. (2021). Combination of arbuscular mycorrhizal fungi and phosphate solubilizing bacteria on growth and production of Helianthus tuberosus under field condition. Sci. Rep. 11 (1), 6501. doi:10.1038/s41598-021-86042-3
Nanjundappa, A., Bagyaraj, D. J., Saxena, A. K., Kumar, M., and Chakdar, H. (2019). Interaction between arbuscular mycorrhizal fungi and Bacillus spp. in soil enhancing growth of crop plants. Fungal Biol. Biotechnol. 6 (1), 23. doi:10.1186/s40694-019-0086-5
Nasslahsen, B., Prin, Y., Ferhout, H., Smouni, A., and Duponnois, R. (2022). Mycorrhizae helper bacteria for managing the mycorrhizal soil infectivity. Front. Soil Sci. 2. doi:10.3389/fsoil.2022.979246
Nepal, J., Ahmad, W., Munsif, F., Khan, A., and Zou, Z. (2023). Advances and prospects of biochar in improving soil fertility, biochemical quality, and environmental applications. Front. Environ. Sci. 11, 1114752. doi:10.3389/fenvs.2023.1114752
Ó Gráda, C., and O’Rourke, K. H. (2021). The Irish economy during the century after partition. Econ. Hist. Rev. 75, 336–370. doi:10.1111/ehr.13106
Okiobe, S. T., Meidl, P., Koths, T., Olschewsky, D., Rillig, M. C., and Lammel, D. R. (2022). Root colonization by arbuscular mycorrhizal fungi is reduced in tomato plants sprayed with fungicides. Front. Agron. 4. doi:10.3389/fagro.2022.1028195
Ong, H. C., Chen, W. H., Farooq, A., Gan, Y. Y., Lee, K. T., and Ashokkumar, V. (2019). Catalytic thermochemical conversion of biomass for biofuel production: a comprehensive review. Renew. Sustain. Energy Rev. 113, 109266. doi:10.1016/j.rser.2019.109266
Padedda, B. M., Sechi, N., Lai, G. G., Mariani, M. A., Pulina, S., Sarria, M., et al. (2017). Consequences of eutrophication in the management of water resources in Mediterranean reservoirs: a case study of Lake Cedrino (Sardinia, Italy). Glob. Ecol. Conservation 12, 21–35. doi:10.1016/j.gecco.2017.08.004
Paerl, H. W., and Otten, T. G. (2013). Harmful cyanobacterial blooms: causes, consequences, and controls. Microb. Ecol. 65 (4), 995–1010. doi:10.1007/s00248-012-0159-y
Pavithra, D., and Yapa, N. (2018). Arbuscular mycorrhizal fungi inoculation enhances drought stress tolerance of plants. Groundw. Sustain. Dev. 7, 490–494. doi:10.1016/j.gsd.2018.03.005
Peer, W. A., Baxter, I. R., Richards, E. L., Freeman, J. L., and Murphy, A. S. (2005). “Phytoremediation and hyperaccumulator plants,” in Molecular biology of metal homeostasis and detoxification (Berlin, Heidelberg: Springer), 299–340.
Pfister, C. (2010). “The “1950s Syndrome” and the transition from a slow-going to a rapid loss of global sustainability,” in The turning points of enviornmental history. Editor F. Uekoetter, 90–118.
Pozo Jiménez, M. J., Troncho, P., Gamir, J., Pozo, M. J., Camañes, G., Cerezo, M., et al. (2016). The nitrogen availability interferes with mycorrhiza-induced resistance against botrytis cinerea in tomato. Front. Microbiol. 7, 1598. doi:10.3389/fmicb.2016.01598
Premarathna, K. S. D., Rajapaksha, A. U., Sarkar, B., Kwon, E. E., Bhatnagar, A., Ok, Y. S., et al. (2019). Biochar-based engineered composites for sorptive decontamination of water: a review. Chem. Eng. J. 372, 536–550. doi:10.1016/j.cej.2019.04.097
Qiu, B., Shao, Q., Shi, J., Yang, C., and Chu, H. (2022). Application of biochar for the adsorption of organic pollutants from wastewater: modification strategies, mechanisms and challenges. Sep. Purif. Technol. 300, 121925. doi:10.1016/j.seppur.2022.121925
Rubin, J. A., and Görres, J. H. (2021). Potential for mycorrhizae-assisted phytoremediation of phosphorus for improved water quality. Int. J. Environ. Res. Public Health 18 (1), 7. doi:10.3390/ijerph18010007
Saleh, I., Kavian, A., Habibnezhad Roushan, M., and Jafarian, Z. (2018). The efficiency of vegetative buffer strips in runoff quality and quantity control. Int. J. Environ. Sci. Technol. 15 (4), 811–820. doi:10.1007/s13762-017-1411-2
Sangwan, S., and Prasanna, R. (2022). Mycorrhizae helper bacteria: unlocking their potential as bioenhancers of plant–arbuscular mycorrhizal fungal associations. Microb. Ecol. 84 (1), 1–10. doi:10.1007/s00248-021-01831-7
Schlesinger, W. H., and Bernhardt, E. S. (2013). Biogeochemistry: an analysis of global change. Academic Press.
Schmidt, M. J., Rapp Py-Daniel, A., de Paula Moraes, C., Valle, R. B., Caromano, C. F., Texeira, W. G., et al. (2014). Dark earths and the human built landscape in Amazonia: a widespread pattern of anthrosol formation. J. Archaeol. Sci. 42, 152–165. doi:10.1016/j.jas.2013.11.002
Sharma, S. B., Sayyed, R. Z., Trivedi, M. H., and Gobi, T. A. (2013). Phosphate solubilizing microbes: sustainable approach for managing phosphorus deficiency in agricultural soils. SpringerPlus 2 (1), 587. doi:10.1186/2193-1801-2-587
Singh, B., and Craswell, E. (2021). Fertilizers and nitrate pollution of surface and ground water: an increasingly pervasive global problem. SN Appl. Sci. 3 (4), 518. doi:10.1007/s42452-021-04521-8
Sohi, S., Lopez-Capel, E., Krull, E., and Bol, R. (2009). Biochar, climate change and soil: a review to guide future research. Collingwood, Victoria: CSIRO, 65.
Song, Y., Chen, D., Lu, K., Sun, Z., and Zeng, R. (2015). Enhanced tomato disease resistance primed by arbuscular mycorrhizal fungus. Front. Plant Sci. 6, 786. doi:10.3389/fpls.2015.00786
Steffen, W., Broadgate, W., Deutsch, L., Gaffney, O., and Ludwig, C. (2015). The trajectory of the anthropocene: the Great acceleration. Anthropocene Rev. 2 (1), 81–98. doi:10.1177/2053019614564785
Stutter, M. I., Chardon, W. J., and Kronvang, B. (2012). Riparian buffer strips as a multifunctional management tool in agricultural landscapes: introduction. J. Environ. Qual. 41 (2), 297–303. doi:10.2134/jeq2011.0439
Stutter, M. I., Langan, S. J., and Lumsdon, D. G. (2009). Vegetated buffer strips can lead to increased release of phosphorus to waters: a biogeochemical assessment of the mechanisms. Environ. Sci. Technol. 43 (6), 1858–1863. doi:10.1021/es8030193
Svenningsen, N. B., Watts-Williams, S. J., Joner, E. J., Battini, F., Efthymiou, A., Cruz-Paredes, C., et al. (2018). Suppression of the activity of arbuscular mycorrhizal fungi by the soil microbiota. ISME J. 12 (5), 1296–1307. doi:10.1038/s41396-018-0059-3
Tan, S., Narayanan, M., Thu Huong, D. T., Ito, N., Unpaprom, Y., Pugazhendhi, A., et al. (2022). A perspective on the interaction between biochar and soil microbes: a way to regain soil eminence. Environ. Res. 214, 113832. doi:10.1016/j.envres.2022.113832
Tomczyk, A., Sokołowska, Z., and Boguta, P. (2020). Biochar physicochemical properties: pyrolysis temperature and feedstock kind effects. Rev. Environ. Sci. Bio/Technology 19 (1), 191–215. doi:10.1007/s11157-020-09523-3
Tovey, H. (1992). Developing agribusiness: some implications for Irish farming. Cork: Department of Sociology, U.C.C.
Trodd, W., and O’Boyle, S. (2022). Water quality in Ireland 2016-2021. Wexford: Environmental Protection Agency.
Uekoetter, F. (2006). “Know your soil: transitions in farmers’ and scientists knowledge in Germany,” in Soils and societies: perspectives from environmental history. Editors J. R. McNeill, and V. Winiwarter (Isle of Harris: White Horse Press).
van Beusekom, J. E. E. (2018). “Eutrophication,” in Handbook on marine environment protection: science, impacts and sustainable management. Editors M. Salomon, and T. Markus (Cham: Springer International Publishing), 429–445. doi:10.1007/978-3-319-60156-4_22
Van Der Heijden, M. G. (2010). Mycorrhizal fungi reduce nutrient loss from model grassland ecosystems. Ecology 91 (4), 1163–1171. doi:10.1890/09-0336.1
Vannette, R. L., and Hunter, M. D. (2011). Plant defence theory re-examined: nonlinear expectations based on the costs and benefits of resource mutualisms. J. Ecol. 99 (1), 66–76. doi:10.1111/j.1365-2745.2010.01755.x
Varjani, S., Kumar, G., and Rene, E. R. (2019). Developments in biochar application for pesticide remediation: current knowledge and future research directions. J. Environ. Manag. 232, 505–513. doi:10.1016/j.jenvman.2018.11.043
Verheijen, F., Jeffery, S., Bastos, A., Van Der Velde, M., and Diafas, I. (2010). Biochar application to soils: a critical scientific review of effects on soil properties, processes and functions. LU: publications Office. Available at: https://data.europa.eu/doi/10.2788/472.
Vives-Peris, V., de Ollas, C., Gómez-Cadenas, A., and Pérez-Clemente, R. M. (2020). Root exudates: from plant to rhizosphere and beyond. Plant Cell Rep. 39 (1), 3–17. doi:10.1007/s00299-019-02447-5
Vocciante, M., Grifoni, M., Fusini, D., Petruzzelli, G., and Franchi, E. (2022). The role of plant growth-promoting rhizobacteria (PGPR) in mitigating plant’s environmental stresses. Appl. Sci. 12 (3), 1231. doi:10.3390/app12031231
Wang, C., Luo, D., Zhang, X., Huang, R., Cao, Y., Liu, G., et al. (2022). Biochar-based slow-release of fertilizers for sustainable agriculture: a mini review. Environ. Sci. Ecotechnology 10, 100167. doi:10.1016/j.ese.2022.100167
Wang, H., García Molinos, J., Heino, J., Zhang, H., Zhang, P., and Xu, J. (2021). Eutrophication causes invertebrate biodiversity loss and decreases cross-taxon congruence across anthropogenically-disturbed lakes. Environ. Int. 153, 106494. doi:10.1016/j.envint.2021.106494
Wang, H., Xiao, K., Yang, J., Yu, Z., Yu, W., Xu, Q., et al. (2020). Phosphorus recovery from the liquid phase of anaerobic digestate using biochar derived from iron−rich sludge: a potential phosphorus fertilizer. Water Res. 174, 115629. doi:10.1016/j.watres.2020.115629
Wang, L., Ok, Y. S., Tsang, D. C. W., Alessi, D. S., Rinklebe, J., Mašek, O., et al. (2022). Biochar composites: emerging trends, field successes and sustainability implications. Soil Use Manag. 38 (1), 14–38. doi:10.1111/sum.12731
Wang, L., Ok, Y. S., Tsang, D. C. W., Alessi, D. S., Rinklebe, J., Wang, H., et al. (2020). New trends in biochar pyrolysis and modification strategies: feedstock, pyrolysis conditions, sustainability concerns and implications for soil amendment. Soil Use Manag. 36 (3), 358–386. doi:10.1111/sum.12592
Wang, M.-Y., Hu, L. B., Wang, W. H., Liu, S. T., Li, M., and Liu, R. J. (2009). Influence of long-term fixed fertilization on diversity of arbuscular mycorrhizal fungi. Pedosphere 19 (5), 663–672. doi:10.1016/S1002-0160(09)60161-2
Wang, Z., Zhang, H., Liu, L., Li, S., Xie, J., Xue, X., et al. (2022). Screening of phosphate-solubilizing bacteria and their abilities of phosphorus solubilization and wheat growth promotion. BMC Microbiol. 22 (1), 296. doi:10.1186/s12866-022-02715-7
Watson, A. (2020). “The single most important factor”: fossil fuel energy, groundwater, and irrigation on the high plains, 1955-1985. Agric. Hist. 94 (4), 629–663. doi:10.3098/ah.2020.094.4.629
Wei, Z., Maxwell, T., Robinson, B., and Dickinson, N. (2022). Grasses procure key soil nutrients for clovers. Nat. Plants 8 (8), 923–929. doi:10.1038/s41477-022-01210-1
Woods, W. I., and McCann, J. M. (1999). The anthropogenic origin and persistence of amazonian dark earths. Yearb. Conf. Lat. Am. Geogr. 25, 7–14.
Woolf, D., Amonette, J. E., Street-Perrott, F. A., Lehmann, J., and Joseph, S. (2010). Sustainable biochar to mitigate global climate change. Nat. Commun. 1 (1), 56. doi:10.1038/ncomms1053
Worster, D. (1990). Transformations of the earth: toward an agroecological perspective in history. J. Am. Hist. 76 (4), 1087–1106. doi:10.2307/2936586
Xia, Y., Zhang, M., Tsang, D. C. W., Geng, N., Lu, D., Zhu, L., et al. (2020). Recent advances in control technologies for non-point source pollution with nitrogen and phosphorous from agricultural runoff: current practices and future prospects. Appl. Biol. Chem. 63 (1), 8–13. doi:10.1186/s13765-020-0493-6
Xiang, W., Zhang, X., Chen, J., Zou, W., He, F., Hu, X., et al. (2020). Biochar technology in wastewater treatment: a critical review. Chemosphere 252, 126539. doi:10.1016/j.chemosphere.2020.126539
Xiang, Y., Xu, Z., Wei, Y., Zhou, Y., Yang, X., Yang, Y., et al. (2019). Carbon-based materials as adsorbent for antibiotics removal: mechanisms and influencing factors. J. Environ. Manag. 237, 128–138. doi:10.1016/j.jenvman.2019.02.068
Xiong, X., Yu, I. K., Tsang, D. C., Bolan, N. S., Sik Ok, Y., Igalavithana, A. D., et al. (2019). Value-added chemicals from food supply chain wastes: state-of-the-art review and future prospects. Chem. Eng. J. 375, 121983. doi:10.1016/j.cej.2019.121983
Yao, Y., Gao, B., Chen, J., Zhang, M., Inyang, M., Li, Y., et al. (2013). Engineered carbon (biochar) prepared by direct pyrolysis of Mg-accumulated tomato tissues: characterization and phosphate removal potential. Bioresour. Technol. 138, 8–13. doi:10.1016/j.biortech.2013.03.057
Yli-Halla, M. (2016). “Fate of fertilizer P in soils: inorganic pathway,” in Phosphorus in agriculture: 100 % zero. Editors E. Schnug, and L. J. De Kok (Dordrecht: Springer Netherlands), 27–40. doi:10.1007/978-94-017-7612-7_3
Yu, C., Li, Z., Xu, Z., and Yang, Z. (2020). Lake recovery from eutrophication: quantitative response of trophic states to anthropogenic influences. Ecol. Eng. 143, 105697. doi:10.1016/j.ecoleng.2019.105697
Yu, L., Zhang, H., Zhang, W., Liu, K., Liu, M., and Shao, X. (2022). Cooperation between arbuscular mycorrhizal fungi and plant growth-promoting bacteria and their effects on plant growth and soil quality. PeerJ 10, e13080. [Preprint]. doi:10.7717/peerj.13080
Zak, D., Kronvang, B., Carstensen, M. V., Hoffmann, C. C., Kjeldgaard, A., Larsen, S. E., et al. (2018). Nitrogen and phosphorus removal from agricultural runoff in integrated buffer zones. Environ. Sci. Technol. 52 (11), 6508–6517. doi:10.1021/acs.est.8b01036
Zhang, J., Guo, Z., Liang, S., Kang, Y., Hu, Z., Xie, H., et al. (2023). Sustainable application of biochar for water purification. Curr. Dev. Biotechnol. Bioeng. 2023, 121–147. doi:10.1016/B978-0-323-91873-2.00014-5
Zhang, S., Wang, L., Ma, F., Zhang, X., and Fu, D. (2016). Reducing nitrogen runoff from paddy fields with arbuscular mycorrhizal fungi under different fertilizer regimes. J. Environ. Sci. 46, 92–100. doi:10.1016/j.jes.2015.12.024
Zhang, S., Wang, L., Ma, F., Zhang, X., Li, Z., Li, S., et al. (2015). Can arbuscular mycorrhiza and fertilizer management reduce phosphorus runoff from paddy fields? J. Environ. Sci. 33, 211–218. doi:10.1016/j.jes.2015.01.016
Zhang, X., Wang, H., He, L., Lu, K., Sarmah, A., Li, J., et al. (2013). Using biochar for remediation of soils contaminated with heavy metals and organic pollutants. Environ. Sci. Pollut. Res. 20 (12), 8472–8483. doi:10.1007/s11356-013-1659-0
Zhou, Y., Zhao, S., Suenaga, T., Kuroiwa, M., Riya, S., and Terada, A. (2022). Nitrous oxide-sink capability of denitrifying bacteria impacted by nitrite and pH. Chem. Eng. J. 428, 132402. doi:10.1016/j.cej.2021.132402
Keywords: arbuscular mycorrhizal fungi, phosphate solubilising bacteria, biochar, mycophytoremediation, nutrient runoff, nitrogen, phosphorus, eutrophication
Citation: Quill L, Ferreira D, Joyce B, Coleman G, Harper C, Martins M, Hodkinson T, Trimble D, Gill L and O’Connell DW (2024) An integrated mitigation approach to diffuse agricultural water pollution–a scoping review. Front. Environ. Sci. 12:1340565. doi: 10.3389/fenvs.2024.1340565
Received: 18 November 2023; Accepted: 21 March 2024;
Published: 12 September 2024.
Edited by:
Buddhi Wijesiri, Queensland University of Technology, AustraliaReviewed by:
Shamshad Ahmad, District Water Testing Laboratory Jal Nigam, IndiaAlaa El Din Mahmoud, Alexandria University, Egypt
Chris Adegoke Fayose, Obafemi Awolowo University, Nigeria
Kannan Nadarajah, Other, Sri Lanka
Copyright © 2024 Quill, Ferreira, Joyce, Coleman, Harper, Martins, Hodkinson, Trimble, Gill and O’Connell. This is an open-access article distributed under the terms of the Creative Commons Attribution License (CC BY). The use, distribution or reproduction in other forums is permitted, provided the original author(s) and the copyright owner(s) are credited and that the original publication in this journal is cited, in accordance with accepted academic practice. No use, distribution or reproduction is permitted which does not comply with these terms.
*Correspondence: David W. O’Connell, RGF2aWQub2Nvbm5lbGxAdGNkLmll
†These authors have contributed equally to this work