- 1Teagasc, Animal & Grassland Research and Innovation Centre, Moorepark Fermoy, Cork, Ireland
- 2College of Science and Engineering, Civil Engineering and Ryan Institute, University of Galway, Galway, Ireland
- 3Teagasc, Crops, Environment and Land Use Centre, Wexford, Ireland
Introduction: On dairy farms with poorly drained soils and high rainfall, open ditches receive nutrients from different sources along different pathways which are delivered to surface water. Recently, open ditches were ranked in terms of their hydrologic connectivity risk for phosphorus (P) along the open ditch network. However, the connectivity risk for nitrogen (N) was not considered in that analysis, and there remains a knowledge gap. In addition, the P connectivity classification system assumes all source–pathway interactions within open ditches are active, but this may not be the case for N. The objective of the current study, conducted across seven dairy farms, was to create an integrated connectivity risk ranking for P and N simultaneously to better inform where and which potential mitigation management strategies could be considered.
Methods: First, a conceptual figure of known N open ditch source–pathway connections, developed using both the literature and observations in the field, was used to identify water grab sampling locations on the farms. During fieldwork, all open ditch networks were digitally mapped, divided into ditch sections, and classified in terms of the existing P connectivity classification system.
Results and Discussion: The results showed that not all source–pathway connections were present across ditch categories for all species of N. This information was used to develop an improved open ditch connectivity classification system. Farmyard-connected ditches were the riskiest for potential point source losses, and outlet ditches had the highest connectivity risk among the other ditch categories associated with diffuse sources. Tailored mitigation options for P and N speciation were identified for these locations to intercept nutrients before reaching receiving waters. In ditches associated with diffuse sources, nitrate was introduced by subsurface sources (i.e., in-field drains and groundwater interactions from springs, seepage, and upwelling) and ammonium was introduced through surface connectivity pathways (i.e., runoff from internal roadways). On similar dairy farms where open ditches are prevalent, the integrated classification system and mapping procedure presented herein will enable a targeted and nutrient-specific mitigation plan to be developed. The same methodology may be applied to develop a bespoke integrated connectivity risk ranking for P and N along agricultural open ditches in other areas.
1 Introduction
Open ditch networks, also referred to as “surface ditch network,” are installed in poorly drained soils to remove excess water, control the water table, and aid with grass production and utilization (Tuohy et al., 2016; Hertzberger et al., 2019). These networks comprise a series of connected and unconnected sections that receive nutrients from a variety of surface and subsurface pathways, all of which can then be transported to other sections or associated waterbodies (Kröger et al., 2007; Herzon and Helenius, 2008; Moloney et al., 2020). Connectivity is defined as the transfer of energy and matter across two landscape zones, whereas disconnectivity is the isolation of these zones (Chorley and Kennedy, 1971). Identifying the connectivity of these systems enables mitigation strategies to be implemented at optimal locations where nutrients can be reduced or restrained (e.g., intercepting the pathway, slowing the flow, or removing some of the nutrients in the water) to minimize the impact on the receiving waterbody (Fenton et al., 2021). Research continues to help farmers optimize farm management practices (baseline) and engineering solutions (above baseline) (Moore et al., 2010; Schoumans et al., 2014; Carstensen et al., 2020). Many studies on open ditches have focused on nutrient dynamics (Sukias et al., 2003), sediment attenuation capacity (Ezzati et al., 2020; Mattila and Ezzati, 2022), nutrient loss attenuation potential by vegetation (Soana et al., 2017; Zhang et al., 2020), dissolved organic carbon dynamics (Tiemeyer and Kahle, 2014), organic matter composition (Hunting et al., 2016), ditch management (Dollinger et al., 2015; Hertzberger et al., 2019), and indirect greenhouse gas emissions (Hyvönen et al., 2013; Clagnan et al., 2019). However, few studies have investigated the role played by open ditch connectivity in the transfer of nutrients from the source to the receptor. Such studies may provide vital information to ascertain the positioning of ditch mitigation option and the dominant nutrient species it is required to target. Moreover, there is a poor understanding of processes leading to the immobilization and transformation of nutrients within soil and drainage systems along the hydrological pathways into ditches (Deelstra et al., 2014). For efficient mitigation of nutrient loss from open ditch networks, a conceptual understanding of how nutrient sources and their pathways connect to the open ditch system must be established.
The general trend and pathways of agricultural pollutants have been well-documented and are summarized in Figure 1. In summary, nutrient entry into ditches is predominantly from diffuse sources and often through the complex surface and subsurface pathways determined by soil type, climate, landscape position, farm management, and nutrient input sources (manure or fertilizer type) (Granger et al., 2010; Monaghan et al., 2016; Gramlich et al., 2018). These factors regulate the hydrology, the primary driver of nutrient transfer, and the terrestrial and aquatic biogeochemistry that defines the type and form/species of nutrients entering open ditches and subsequently discharging to associated waterbodies (Sukias et al., 2003). Conceptually, phosphorus (P), either as particulate P (PP) or dissolved reactive phosphorus (DRP), and nitrogen (N), as ammonium (NH4+) or nitrate (NO3−), are transported from fields or hard surfaces like roadways through surface flow pathways into open ditches (Figure 1).
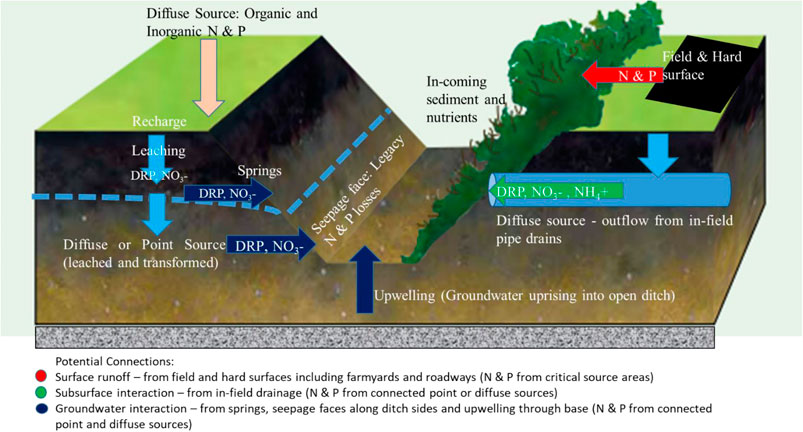
FIGURE 1. Conceptual figure of an open ditch showing all potential nitrogen and phosphorus sources (point and diffuse), pathways, and discharge connections [modified from Teagasc (2022) and Simpson et al. (2011)].
As shown in Figure 1, any groundwater-to-open ditch water connection represents a subsurface interaction distinct from in-field drain connections. In this scenario, typically, P is in the form of DRP, and NO3− represents mineralized N that has become mobilized due to infiltrating water. This N is primarily lost from diffuse sources in fields due to fertilization and grazing of animals. Clagnan et al. (2018) have shown the conversion of N to NH4+ in poorly drained soils, which can be discharged in waters from in-field drains within the groundwater-to-open ditch water connections (Needelman et al., 2007; Valbuena-Parralejo et al., 2019). The presence of NO3− in open ditch networks suggests more permeable connectivity pathways that eventually seep into open ditches along seepage faces or upwell as the water table rises, whereas the presence of NH4+ suggests less permeable routes before discharge occurs. Groundwater springs represent a distinct groundwater storage component that protrudes onto fields, which are often drained by the installation of an intersecting pipe into an open ditch below the spring. This creates a direct discharge point within the open ditch (Figure 1). The presence of this discharge may change during dry periods as the water level decreases below the base of the open ditch.
Moloney et al. (2020) used this concept to rank the connectivity risk (from highest to lowest) for P along agricultural open ditches. The riskiest open ditches were those directly connected to farmyards (farmyard connection ditches) and watercourses (outlet ditches), while the least risky open ditches included secondary and outflow ditches (disconnected ditches did not pose any risk of connectivity). The system devised by Moloney et al. (2020) conceptualized P sources and pathways with the aim of disconnecting P losses before discharge to associated waterbodies. The current study takes the same approach but creates an integrated connectivity risk ranking that considers both N, which discharges into the open ditch network via surface and subsurface pathways (Figure 1), and P. Such integration necessitates a thorough understanding of N and P biogeochemical cycles, how sources are connected along different surface and subsurface pathways to the open ditch network, and how this network is connected and delivered to the adjoining aquatic system (e.g., river). Accounting for attenuation along the pathway and within the open ditch network is a constraint within the current conceptual framework. Therefore, there is a need to integrate N into the connectivity risk ranking so that a more holistic mitigation management strategy may be designed (i.e., source protection on the farm and “right measure, right place” in the open ditch).
The objective of this study was to derive a farm-scale integrated open ditch risk ranking for both P and N loss risk based on connectivity to inform future mitigation management on heavy textured, grassland dairy farms. To fulfil this objective, seven farms were selected with open ditch networks on heavy textured soils. A conceptual figure illustrating the trends and pathways of agricultural pollutants for an open ditch is presented. The open ditch networks were mapped during a ground survey, and a qualitative water sampling campaign was conducted (based on the conceptual figure) to validate the presence or absence of pathways for N and P. This enabled an integrated classification of an open ditch network ranking to be developed. Mitigation options for each ditch class are presented.
2 Materials and methods
2.1 Site selection and characteristics
Seven grassland dairy farms on poorly drained soils geographically located across the SW and NE of Ireland were selected to represent a variety of agronomic dairy production systems and biophysical settings (Table 1). As per the Ireland EPA soil and subsoil maps (Fealy et al., 2009), the soil types on these farms varied from organic to mineral soils. The majority of these farm fields were imperfectly or poorly drained, necessitating an ad hoc network of artificial drainage installations on the farms. The grazing area of each farm ranged from 28 to 45 ha. Intensive dairy farm management practices were observed on all farms. Morgan’s extractable soil P test (Wall and Plunkett, 2020) was used to determine the agronomic excesses and deficiencies in plant available P for fields of each farm. The farms in this study were located in high-rainfall areas with an average rainfall of 1092.5 mm. The average farm slope was measured on all seven farms, as it could influence open ditch connectivity.
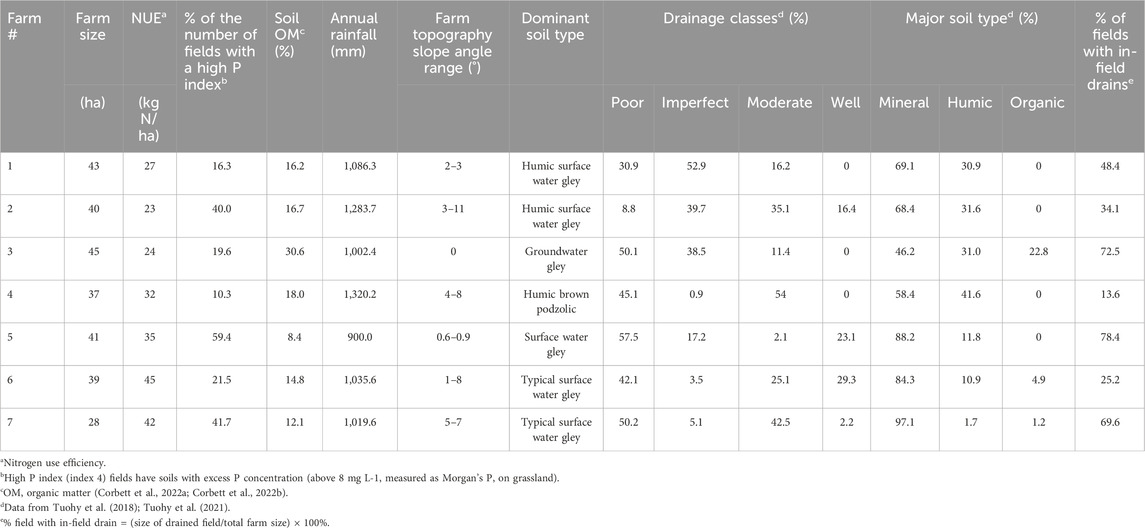
TABLE 1. Summary of agronomic and soil data and associated in-field drainage percentages across case study farms.
2.2 Ground survey and mapping connectivity pathways for N into P connectivity risk ditch categories
A ground survey was carried out on all the farms during winter (November 2021 to March 2022) to characterize the field boundaries and surface and subsurface networks on each farm. This period was selected following multiple field visits carried out across all seasons in the previous year. This period was identified as the best hydrological period when connectivity pathways were active for grab sampling. Drainage network features such as open ditches connected to the farmyard and the proximity of the open ditch to waterbodies were noted on each farm during the ground survey. In addition, the connectivity pathways for N into open ditches from in-field drains, farm roadways, groundwater springs, seepage, and upwelling as per the conceptual figure (Figure 1) throughout the drainage network were noted during this time. During the ground survey, all drainage network data, such as of drain locations, flows and connections, and sampling locations, were recorded by using an electronic device with ESRI ArcGIS Field Maps mobile software (ESRI, 2024).
Open ditches were identified as manmade open drains usually sited along the field edges to carry excess water from the field and farm. Surface waterbodies (1st- and 2nd-order streams) in and around each farm, defined as those appearing on the national ordnance survey maps (6-inch maps) (osi.ie), were mapped onto each farm map before each ground survey.
Information from the ground survey observations and qualitative interviews with farmers on drainage networks were used to digitize and map farm and field boundaries and the open ditch network (open ditches, sub-surface in-field drains, and drainage outlets) and associated connectivity pathways for N (Figure 2). For the open ditch network within each farm, each ditch was assigned a ditch category using their connection to a farmyard, watercourse, neighboring farm, other ditches on the same farm, and also their non-connection to any other part of the open ditch network after Moloney et al. (2020) (Table 2). These categories are as follows: (1) farmyard connection ditch, (2) outlet ditch, (3) outflow ditch, (4) secondary ditch, and (5) disconnected ditch (Figure 2) using ArcMap GIS software (version 10.5).
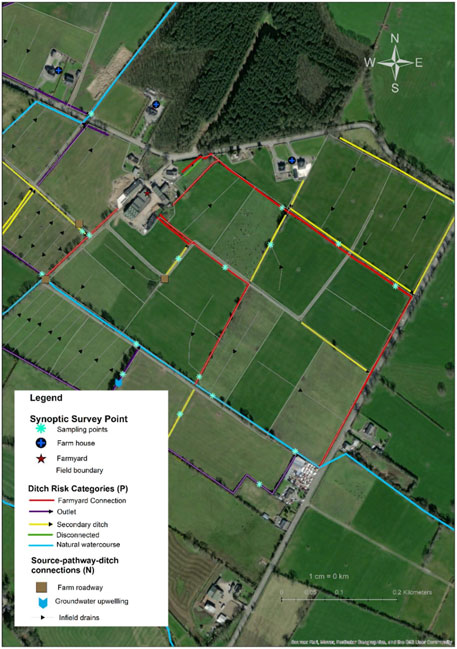
FIGURE 2. Example of a farm output map (for farm 5) showing the ranked classification risk along the open ditch network for P (color-coded into categories of connectivity risk) and all conceptualized N open ditch connectivity pathways to individual open ditch sections. For in-field drains, arrows indicate fall and flow direction toward open ditch sections, with a particular P risk indicated by the existing color-coding scheme of Moloney et al. (2020).
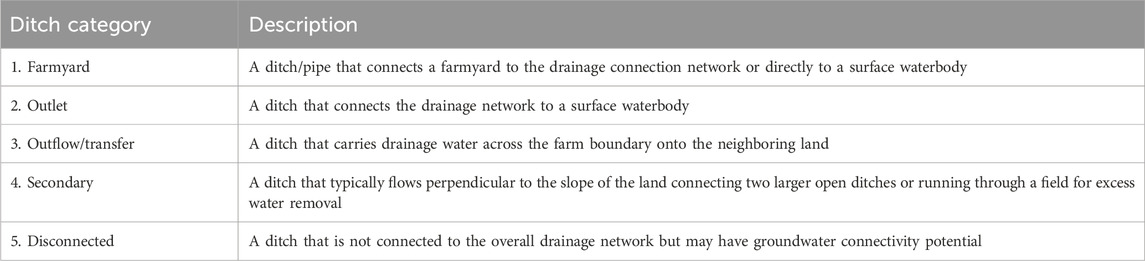
TABLE 2. Definition and description of open ditch categories for the P classification system of Moloney et al. (2020).
On each assigned ditch category, the connectivity pathways for N (Table 3), where present, were mapped within this open ditch network using the conceptual figure (Figure 1) as a guide during fieldwork to integrate the N connectivity pathway risk into the P connectivity risk open ditch categories. To identify the connectivity pathways, landscape position was taken into account, especially for assessing the interaction of groundwater with an open ditch section. Groundwater seeping through open ditch bank sides and groundwater upwelling through the base of the open ditch were identified as groundwater seepage and upwelling, respectively (Table 3), and were classified together as one connectivity pathway. Roadways were identified as a connectivity pathway when there were site observations of water flow and eroded/gully surface (due to continuous past water flows) from the farm roads into a nearby open ditch. Groundwater springs were identified as high-flow groundwater purging out into open ditches either over the surface or through pipes. Subsurface in-field drains were all piped drains directed into ditches but were differentiated from piped springs with their low and intermittent flows into the open ditches.
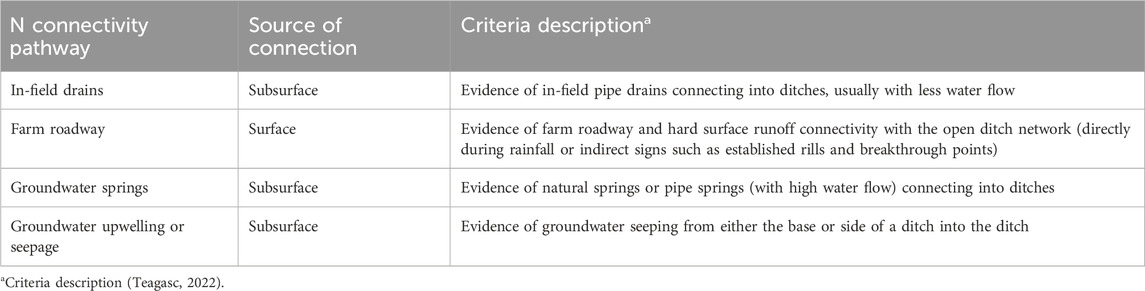
TABLE 3. Criteria for identifying N connectivity pathways on open ditch categories and associated source of connection.
The length of the open ditches and farm and field boundaries were measured in ArcGIS and compared for each farm, as shown in Table 4. In addition, the occurrence of a particular N connectivity pathway was calculated as a percentage of the total number of N connectivity pathways observed for each farm and for each open ditch category.
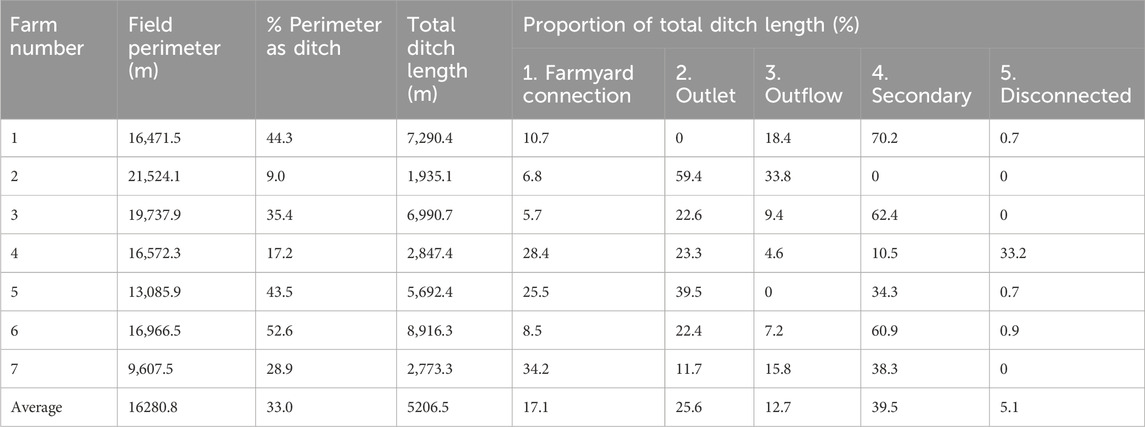
TABLE 4. Summary of open ditch data including the proportion of the open ditch network accounted for by different P open ditch categories for each case-study farm.
2.3 Grab water sampling campaign to assess integrated nutrient connectivity pathways
Water quality parameters change over time, depending on the local climatic conditions and farming practices (Huebsch et al., 2013). In the present study, the objective was to establish a link or connection (see Figure 1) between the source and pathway to the open ditch network. Therefore, “snapshot” sampling in spring (March) presented a good opportunity to collect qualitative data.
In spring (March) 2022, a total of 210 water samples were collected directly from 105 sampling sites in open ditches throughout the drainage network across all farms during a one-time sampling event following the procedure of Moloney et al. (2020). These sampling sites reflected connectivity pathways presented in Figure 1. March was selected for sampling because this month is hydrologically active in Ireland and all pathways interact with the open ditch network (e.g., groundwater upwelling, seepage, and springs), as observed from the previous year’s field visits. As this study aimed to validate established connectivity risk (water and the presence or absence of N and P) between open ditch types and adjoining surface waterbodies and did not aim to elucidate the load or impact of this connection, a temporal water sampling survey was not required. It is acknowledged that the connectivity level at the time of sampling water is influenced by the precipitation level (both antecedent and current). Therefore, sampling was undertaken when both surface and subsurface pathways were most active, and such data were used to validate the source and hydrologic connectivity with the open ditch network.
The number of samples collected was dictated mainly by the observations of connectivity pathways on open ditches during the initial fieldwork campaign. As such, open ditches that had surface or subsurface connectivity pathways (Table 3) noted in the earlier survey were prioritized for sampling. These observations were used to validate surface, subsurface, and groundwater flows that entered open ditches on the case study farms. However, some sampling points had no N connectivity pathways. Only four ditch categories from Table 2 (farmyard connection, outlet, outflow, and secondary ditches) were sampled for water across the seven case study farms. Shallow disconnected ditches (category 5 in Table 2) were dry, which indicated no N connectivity with perched or true water tables at the time of sampling. These acted as storage and recharge areas for groundwater during rainfall periods. At each water sampling location, two 50-ml samples (filtered on-site using 0.45-μm filter paper and unfiltered) were collected for dissolved and total P analyses, respectively. Grab water sampling was carried out in the mapped ditch categories on each farm, provided water was present in the open ditch. The grab water sampling taken directly from an open ditch was conducted within 1 m downstream of in-field drain outlets, farm roadways, groundwater springs, and groundwater seepage/upwelling, where present, in the open ditch categories. All water samples were kept in an ice box during sampling and transportation and then tested within 1 day of sample collection.
Filtered water samples were analyzed for DRP and total dissolved phosphorus (TDP) using a Gallery discrete analyzer (Gallery reference manual, 2016) and a Hach Ganimede P analyzer, respectively. The total dissolved phosphorus (TDP) was measured by acid persulfate oxidation under high temperature and pressure. The unfiltered water samples were analyzed for nitrite (NO2-N), NH4-N, total oxidized nitrogen (TON), and total reactive phosphorus (TRP) using the Gallery analyzer. Total phosphorus (TP) was analyzed using the Ganimede P analyzer. Phosphorus was measured colorimetrically by the ascorbic acid reduction method (Askew and Smith, 2005), where the 12-molybdophosphoric acid complex is formed by the reaction of orthophosphate ions with ammonium molybdate and antimony potassium tartrate (catalyst) and reduced ascorbic acid. All samples, reagent blanks, and check standards were analyzed at the Teagasc Johnstown laboratory following the Standard Methods (APHA, 2005). All quality control (QC) samples/check standards are prepared from certified stock standards from a different source than calibration standards. Quality control samples were analyzed at the beginning and end of every batch, for every 10 samples within a batch, and if the QC fell outside limits, samples were repeated back to the last correct QC. Blanks were included in every batch, and approximately 10% of samples were repeated. Tolerances range up to a maximum of ±7.5% of the nominal value. All instruments used were calibrated in line with the manufacturers’ recommendations. Nitrate-N was calculated by subtracting NO2-N from TON, particulate phosphorus (PP) was calculated by the difference between TP and TDP, and dissolved unreactive phosphorus (DUP) was calculated by the difference between TDP and DRP.
2.4 Data analysis
To validate the link between the conceptualized connectivity source–pathways and their introduction of N and P into the open ditch system, data from the spring season synoptic survey were analyzed statistically to differentiate the nutrient concentrations for the various open ditch categories and also for the various connectivities to ascertain if they varied from each other. As the data for each water quality parameter were not normally distributed, Kruskal–Wallis analysis was undertaken to find out the significant differences between farmyard connection, outlet, outflow, and secondary ditch categories and also between the conceptualized N connectivity pathways (in-field drains, internal roadways, springs, and seepage/upwelling) within and across the outlet, outflow, and secondary ditch categories for all the water quality parameters (NH4-N, NO3-N, TN, DRP, DUP, TP, and PP). Data were analyzed using R studio software version 4.0.2 (2020). Where significant differences were observed using an alpha level of 0.05 (95% confidence level), the pairwise Wilcoxon rank-sum test was further used to find the differences between the means of the pairs. Microsoft Excel software version 16.0 (2016) was used to find a correlation between the number of occurrences of in-field drains and the percentage of drained fields on poorly draining soil farms.
3 Results
3.1 Analysis of the open ditch networks
All five ditch categories, classified by Moloney et al. (2020), were identified using the criteria outlined in that work. The average percentage of the total ditch network in all farms was 17.1%, 25.6%, 12.7%, 39.5%, and 5.1% for farmyard connection, outlet, outflow, secondary, and disconnected ditches, respectively (Table 4). Farm 2 contained the fewest drainage categories (3 out of 5).
3.2 Observations relating to conceptualized N connections within the open ditch networks
Based on the criteria for identifying N connectivity pathways (Table 3), 52% of all the open ditch network sampling points were observed to have N connectivity pathways interacting with them. The N connectivity pathways to open ditches considered in this study were mainly connected to secondary ditches, followed by farmyard connection, outflow, and outlet ditches, with no N connectivity pathway to disconnected ditches (Supplementary Table S1). For each ditch category (Table 2) sampled in this study, the percentages of the occurrence of different N connectivity pathways are shown in Figure 3. Among these N connectivity pathways across all ditch categories, in-field drains were the most common (representing 64%), followed by groundwater springs, internal roadways, and groundwater upwelling/seepage, respectively, representing 20%, 11%, and 5% of the sampling points (Supplementary Table S1). The occurrence of observed in-field drains was positively correlated to the percentage of drained fields on case study farms (R2 = 0.35).
Farms 2 and 4, which had the lowest percentage of in-field drained fields (Table 1), had relatively high connectivity of groundwater springs to open ditches (Supplementary Table S1). Aside from farm roadway connectivity pathways to open ditches on Farm 2, roadway connectivity pathways to open ditches were found to be highest on farms with a flat topography, particularly farms 3 and 5. Groundwater upwelling/seepage connectivity to ditches was uncommon. There was an absence of groundwater upwelling and seepage connectivity pathways on outflow and farmyard connection ditches and roadway connectivity pathways on outlet ditches across all farms. In addition, there was evidence of multiple N connectivity pathways to individual ditches on some farms.
3.3 Validation of N connectivity pathway using the synoptic survey
The average TN and TP concentrations were significantly higher in farmyard connection ditches (Figure 4) than in outlet, outflow, and secondary ditches (p < 0.01). Across the outlet, outflow, and secondary ditch categories, NO3-N was the dominant N species, contributing on average to 44.7% of TN at sampling points near N connectivity. Only 10.6% of TN comprised NH4-N within these ditch categories. The highest average NO3-N across these ditch categories was observed in groundwater springs (1.90 mg L-1), followed by in-field drains (0.75 mg L-1), groundwater upwelling (0.65 mg L-1), and roadways (0.17 mg L-1) (Supplementary Table S1). In addition, NO3-N at groundwater springs was dissimilar (p < 0.05) to NO3-N at roadways and in-field drains (Figure 5A). High concentrations of NO3-N were also measured on roadways, where NH4-N is conceptualized as being dominant (Figure 1), on secondary ditches. However, NH4-N dominated TN across these ditches at sample points near roadways, with 25.3% composition as opposed to 6.9% of NO3-N. Ammonium-N concentrations across these ditch categories were not statistically significant (p > 0.05).
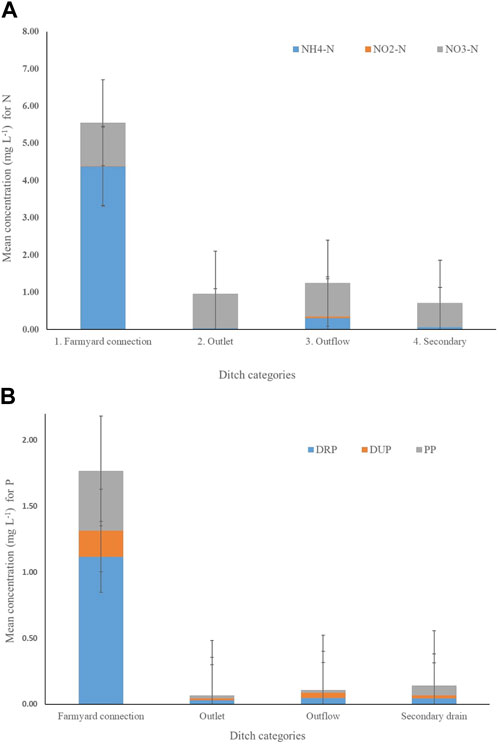
FIGURE 4. (A) Nitrogen (N) and (B) phosphorus (P) mean + standard error (SE) concentrations within the open ditch categories across case study farms.
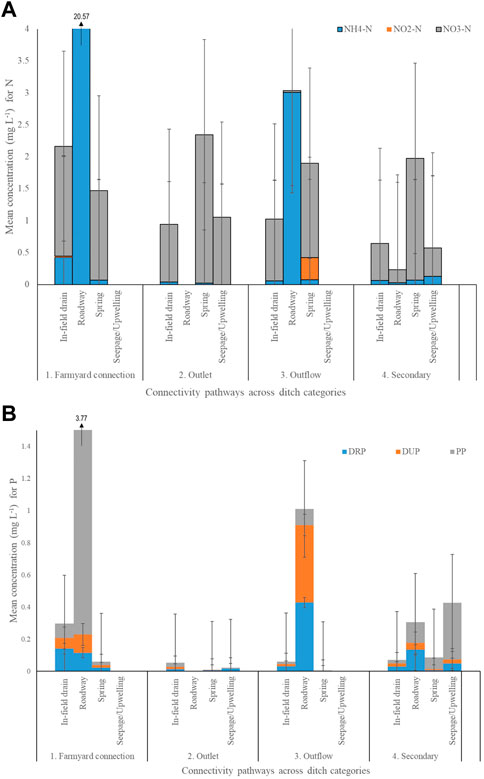
FIGURE 5. (A) Nitrogen (N) and (B) phosphorus (P) mean + standard error (SE) concentrations within associated connectivity pathways in sampled open ditch categories across case study farms.
No consistent trends in species of TP were observed across the outlet, outflow, and secondary ditch categories. Among these ditch categories, TP concentrations were relatively high in secondary ditches, in which PP was predominant (Figure 5B). Across the outlet, outflow, and secondary ditch categories, PP was statistically significant (p < 0.05), particularly between in-field drains and roadway connectivity pathways, and DRP was statistically significant (p < 0.01), particularly between roadways and groundwater springs. Comparing P species for each N connectivity pathway, average PP concentrations were found to be the highest in groundwater upwelling/seepage (0.24 mg L-1), followed by roadways (0.12 mg L-1), groundwater springs (0.04 mg L-1), and in-field drain (0.02 mg L-1) connectivity pathways, whereas average DRP concentrations were the highest in roadways (0.19 mg L-1), followed by groundwater upwelling/seepage (0.04 mg L-1), in-field drains (0.03 mg L-1), and groundwater springs (0.01 mg L-1).
4 Discussion
4.1 Observations on ditch categories and associated N connectivity pathways
Of the seven farms surveyed, disconnected and secondary ditches comprised the lowest and highest average percentages of the total ditch length, respectively. This result is consistent with that of Moloney et al. (2020), who recorded similarly low and high average percentages for total ditch length on varying soil grasslands in Ireland. Disconnected ditches are ineffective for excess field water removal within the drainage system and exist either as blocked normal ditches or as created disconnecting ditches that remove field runoff or precipitation water by infiltration or evaporation. Disconnected ditches, when wet, may hold water with vegetation and potentially provide denitrification or create pollution swapping by the release of greenhouse gases such as nitrous oxide (N2O) or nitric oxide (NO).
Secondary ditches, as the most prevalent ditch category, had the most N connectivity pathways, of which in-field drains were the most prevalent (Figure 3). Secondary ditches connect to other ditch categories from the central farm fields, and due to the farm slopes in that areas, frequent shallow water table (Clagnan et al., 2018) for potential for connectivity pathways may occur. As the majority of the farms in this study contained poorly drained soils (Table 1), a positive, albeit weak, correlation (R2 = 0.35) between the number of occurrences of in-field drains (Supplementary Table S1) and the percentage of drained fields (Table 1) on poorly draining soil farms was observed. Both the number of occurrences of in-field drains and the percentage of drained fields help in regulating water table levels and supporting grass growth functionality, so they were positively correlated.
4.2 Hydrochemistry across P ditch categories and consideration of N connectivity pathways
Higher TN and TP average concentrations were measured in farmyard connection ditches relative to the other ditch categories, which were similar to the findings of Moloney et al. (2020), Harrison et al. (2019), and Ezzati et al. (2020). In the farmyard connection ditches, the TN and TP concentrations were nearly 3 times higher than the TN standard limits of 2.5 mg L-1 set by the European Union for estuarine waters (Wuijts et al., 2022) and 15 times higher than TP standards of 0.1 mg L-1, as proposed by Wetzel (2001). While both Edwards et al. (2008) and Mockler et al. (2017) identified farmyards as point sources for high nutrient loss, the former argued that runoff from farmyards has been overlooked and not duly considered a major nutrient loss hotspot. Such runoff may lead to high nutrient concentration fields near the farmyard relative to fields further away (Fu et al., 2010), and these potentially may enter open ditches near the farmyard to create major downstream water quality problems. Unlike ditches (associated with point sources), the lower TP and TN concentrations in outlet, outflow, and secondary ditch categories may be associated with diffuse nutrient sources. Studies have shown that diffuse sources, relative to point sources, have lower TN and TP concentrations (Pieterse et al., 2003; Edwards and Withers, 2008). Management of some of these diffuse sources is problematic as they are difficult to locate in a landscape (Harrison et al., 2019). However, their impact on the deterioration of receiving waterbodies is substantial, and therefore needs to be managed (Andersen et al., 2014; Bradley et al., 2015). Diffuse sources depend on landscape and other management factors, which influence diffuse N and P mobilization, transformation, and delivery into the ditches (Granger et al., 2010; Schoumans et al., 2014). However, notable among these factors are the hydrological conditions on which diffuse nutrient release strongly depends (Edwards and Withers, 2008; Chen et al., 2013). This, coupled with biogeochemical factors, which may vary within a landscape, influences the spatial and temporal distribution patterns of diffuse N and P, including the pathways by which they enter and leave farms (Clagnan et al., 2019; Grenon et al., 2021). Nutrient losses from the diffuse sources are delivered into open ditches along surface and subsurface pathways, creating hotspots of nutrient loss in certain open ditch categories, which need to be characterized and potentially mitigated. Climatic, landscape, and management factors all have a role to play in when and where impacts occur. These could have contributed to the higher TN concentrations in water samples that were measured near N connectivity pathways than at locations with no N connectivity pathways within the outlet, outflow, and secondary ditch categories, and also for TP in the outflow ditch category. This observation aligns with those of the reported works of Ibrahim et al. (2013) and Valbuena-Parralejo et al. (2019) on in-field drains, Fenton et al. (2021) and Rice et al. (2022) on roadways, Soana et al. (2017) on groundwater springs, and O’Callaghan et al. (2018) on groundwater upwelling/seepage.
Nitrate was the dominating N species in in-field drains, groundwater springs, and upwelling connectivity pathways in outlet, outflow, and secondary ditch categories (Figure 5A). This may be attributed to their connection to a subsurface N source, which comprises leached N from animal excreta and fertilizer that may have been nitrified to NO3-N (Necpalova et al., 2012). In poorly drained grasslands, nitrification may have been increased by the high in-field drainage density (Table 1), which enhanced N preferential flow (Van Der Grift et al., 2016) and limited potential N attenuation (Clagnan et al., 2019; Valbuena-Parralejo et al., 2019). The average NO3-N concentration was highest in groundwater springs and in-field drains. Factors such as the presence of these N connectivity pathways within the shallow subsurface region, nearness to the soil surface (where farm management mostly occurs), and exposure to N sources at the groundwater–ground surface intersection spots (particularly for groundwater springs; Infusino et al., 2022) could have contributed to the high NO3-N concentrations in these locations. In contrast, NH4-N was the most dominating N species measured for roadway connectivity pathways across the outlet, outflow, and secondary ditch categories, especially where animal excreta were observed. This observation aligns with that of Fenton et al. (2021), who observed that roadways draw surface nutrient sources, high in NH4-N, as runoff from soil and animal excreta into nearby ditches and streams. Although important, redox reactions were not considered in the present study.
For TP concentrations across outlet, outflow, and secondary ditch categories, P concentrations were relatively low compared to those in the farmyard connection ditch category. However, such TP concentrations in the outlet, outflow, and secondary ditch categories were still high enough to cause eutrophication downstream, if undiluted. High TP concentrations measured in secondary ditches may be related to the impacts of farm management activities including grazing and farm machinery movement, which is intense within the central fields of most farms where secondary ditches lie as connecting ditch links. These contribute to the erosion of ditch sides and associated deposition of soils in the secondary ditches, as reflected in the higher PP concentrations observed. High TP concentrations measured near roadways on outflow ditches may be due to animal excreta, run-on deposits from farmyards, fields, and poached surfaces as a result of animal and machinery movement (Fenton et al., 2021). Both PP and DRP can trigger eutrophication in waterbodies and may pose a risk to downstream waterbodies. However, this depends on their closeness, connection, and mitigation along the pathway to water sources within agricultural landscapes.
Such information from the study provides an additional insight into the source, connection, and presence (and transformation process) of N in ditch categories from a previous study by Moloney et al. (2020), who observed high NH4+ and NO3− concentrations in all ditch categories, except for the outlet ditch, where high NO3− and low NH4+ were measured, and disconnected ditches, where NO3− concentration was found to be dominant. The risk ranking of connectivity along the open ditch for N and P does not determine the impact of the nutrients being lost to the associated waterbody; it simply establishes the N connectivity pathway if it is present.
4.3 Deriving a connectivity risk for N into P agricultural open ditch categories
The evidence of N concentrations in the ditch water chemistry from Moloney et al. (2020) and the current study informs an improved ditch connectivity risk category system (Table 5). This is a valuable information tool for environmental sustainability officers to enhance water quality management and mitigation options for N and P losses on dairy grassland farms with heavy textured soils in high-rainfall areas. It considers both the connectivity pathways, through which N can be introduced to a ditch network, and their associated N species.
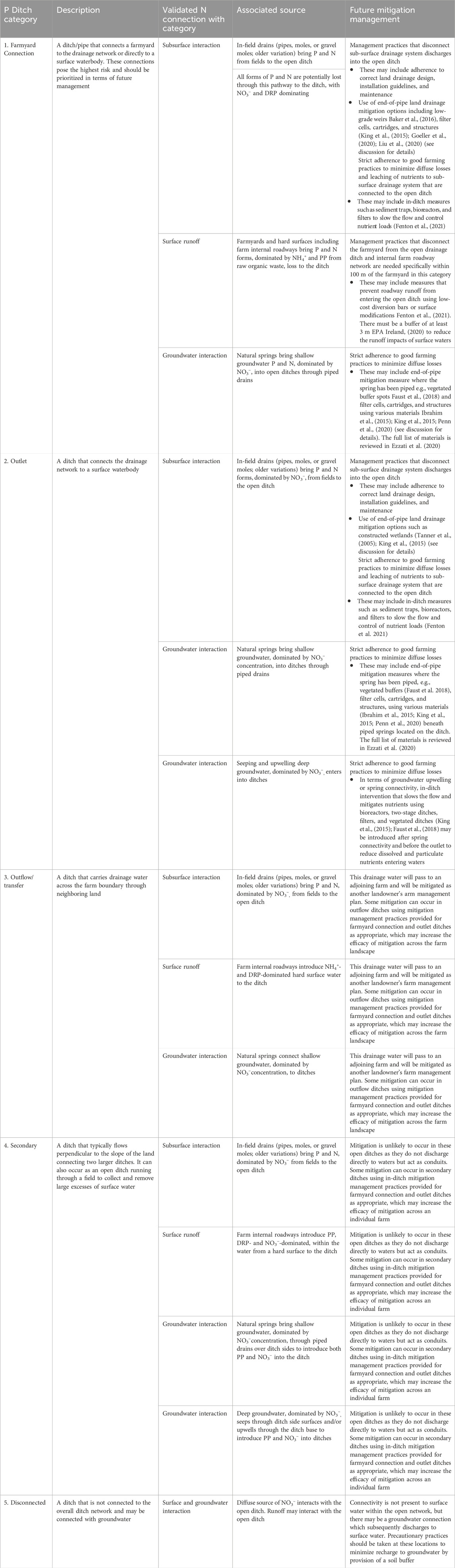
TABLE 5. An updated integrated ditch connectivity ranking that considers both phosphorus and nitrogen coupled with suggested strategies to reduce nutrients from ditches on dairy farms.
In the current study, all of the conceptualized N connectivity pathways (Figure 1) established from the literature were present, but not in all of the sampled P risk ditch categories developed by Moloney et al. (2020) (Supplementary Table S1). For instance, the established general trends and connectivity pathways of groundwater seepage and upwelling were not present on farmyard connection and outflow ditches. Moreover, the grab water sampling data results validated all the conceptualized N connectivity pathways present in ditches (Figure 5A), except groundwater seepage and upwelling. The dominance of high NO3-N concentrations at in-field drains and springs and high NH4-N concentrations at roadways within farmyard connection ditches indicated a point source of pollution arising from their connection to the farmyard aside from the hydrology-induced N concentrations. Farmyards pose the greatest nutrient loss risk on farms due to high nutrient concentration in discharges (Vedder, 2020), and like other point sources, they are independent of hydrology (Edwards and Withers, 2008). As such, primarily managing the farmyard wastewater before discharge into connecting ditches for mitigating nutrient connectivity to water sources is essential (NFGWS, 2020) before deployment along/within ditch interventions.
For the other sampled outlet, outflow, and secondary ditch categories, all N conceptualized pathways were observed, except for internal farm roadway on outlet ditches and groundwater seepage and upwelling on outflow ditches (Supplementary Table S1). In outlet, outflow, and secondary ditch categories, the ditch water synoptic data validated the conceptualized NO3-N and NH4-N for all the observed N connectivity pathways, except farm roadway connection on secondary ditches (which was invalid with NO3-N dominance over conceptualized NH4-N from hard field surface flow pathways). Nitrate dominated in-field drains, groundwater springs, upwelling, and seepage connectivity pathways, and NH4-N dominated farm roadways across the outlet, outflow, and secondary ditch categories, as conceptualized in Figure 1.
Assessment of the N connectivity pathway within ditch category 5 could not be included in the study due to the unavailability of water samples in this ditch for validating conceptualized N connectivity pathways. Moloney et al. (2020) showed that disconnected ditches pose relatively less risk for nutrient loss among the ditch categories, and therefore, merit less focus during nutrient loss mitigation for surface water. However, such low nutrient concentrations could be leached into groundwater, and therefore may require mitigation interventions to prevent leaching.
To apply this research in practice, once open ditches are investigated and mapped, a category should be assigned for an individual open ditch, after which the available N connections for that ditch are noted. All of these connections, in combination, will aid in the future mitigation management strategy. It is unlikely, for example, that more than one mitigation option will be installed in a single open ditch. Therefore, the information gathered from Table 5 can be used to ensure that correct nutrients and their speciation are targeted for mitigation in the open ditch. Mitigation options may be a combination of those that limit diffuse and point sources. For example, with respect to diffuse sources, strict adherence to action programs to reduce losses is important (e.g., Good Agricultural Practice Regulations, in line with the Nitrates Directive (91/676/EEC)). With respect to roadway runoff, NH4+ mitigation options are available and have been outlined in Fenton et al. (2021) and Rice et al. (2022) (e.g., diversion bars to move runoff to a buffer area of at least 1.5 m, cambering farm roadways, and directing flow onto adjacent fields). Adopting a two-stage ditch design may reduce high PP concentrations (King et al., 2015; Hodaj et al., 2017; Faust et al., 2018). With respect to the subsurface N connectivity pathways (in-field drains, groundwater springs, upwelling, and seepage), in-ditch management practices may control the flow and the nutrient content leaving the open ditch. These may include sediment traps (Wilkinson et al., 2014), vegetated ditches (Kröger et al., 2008; Soana et al., 2017; Faust et al., 2018), or in-ditch filters or bioreactors (King et al., 2015; Goeller et al., 2020; Liu et al., 2020). Nutrient filtering through vegetation (Moeder et al., 2017) or use of a medium (Ezzati et al., 2020) can only aim to mitigate a small amount of overall nutrients leaving the ditch due to hydraulic retention times needed and bypass flow during high storm events. Furthermore, mitigation practices including the construction of wetlands (Tanner et al., 2005), vegetated buffer zones (Faust et al., 2018), and low-grade weirs (Kröger et al., 2012; Littlejohn et al., 2014; Baker et al., 2016) that may be placed at the end of ditches after the connectivity pathways, especially for farmyard connection and outlet ditch categories, would help limit nutrient loss from these farms. Therefore, all measures need to be considered a package and not in isolation when trying to minimize nutrient and sediment loads leaving an open ditch system. It is worth noting that cooperation at the local level is needed to prevent other mitigation-related problems (such as the polluter pays principle regarding outflow ditches between neighboring farmers) to ensure mitigation occurs before waters are impacted.
5 Conclusion
Distinctly different from a P-only classification system, the integrated connectivity risk classification system for N and P showed that not all source–pathway interactions within open ditches are active. This is a valuable information tool that enables a much more specific and targeted nutrient-specific mitigation approach to be implemented on open ditches in heavy textured grassland dairy farms in high-rainfall areas. The new system avoids the pitfalls of a P-only classification system (i.e., mitigating for P but allowing N to affect water quality unabated). The findings of this study are limited to these field sites and may (or may not) differ in other geographic areas with different soils, climates, agricultural practices, etc. However, the same methodology may be applied to other areas to develop a bespoke integrated connectivity risk ranking for P and N along agricultural open ditches to inform targeted and specific mitigation strategies on those farms. Further assessment of the temporal and spatial variability of soil, weather, drainage system, and general hydrogeochemistry, which influences nutrient connectivity, may be needed to rank the N and P risk in each ditch category.
Data availability statement
The raw data supporting the conclusion of this article will be made available by the authors, without undue reservation.
Author contribution
DO: conceptualization, data curation, formal analysis, investigation, methodology, validation, visualization, writing–original draft, and writing–review and editing. MH: conceptualization, funding acquisition, investigation, methodology, software, supervision, validation, visualization, and writing–review and editing. OF: conceptualization, funding acquisition, investigation, methodology, software, supervision, validation, visualization, and writing–review and editing. KD: conceptualization, investigation, methodology, validation, visualization, and writing–review and editing. TC: funding acquisition, methodology, and writing–review and editing. PT: conceptualization, funding acquisition, investigation, methodology, project administration, resources, software, supervision, validation, visualization, and writing–review and editing.
Funding
The author(s) declare that financial support was received for the research, authorship, and/or publication of this article. The authors are grateful to Teagasc for the award of a Walsh Scholarship to the first author (grant number: RMIS-1381) to conduct this research.
Acknowledgments
The authors are grateful to Simon Leach, Asaf Shnel, and Denis Brennan for GIS, fieldwork, and laboratory assistance provided.
Conflict of interest
The authors declare that the research was conducted in the absence of any commercial or financial relationships that could be construed as a potential conflict of interest.
Publisher’s note
All claims expressed in this article are solely those of the authors and do not necessarily represent those of their affiliated organizations, or those of the publisher, the editors, and the reviewers. Any product that may be evaluated in this article, or claim that may be made by its manufacturer, is not guaranteed or endorsed by the publisher.
Supplementary material
The Supplementary Material for this article can be found online at: https://www.frontiersin.org/articles/10.3389/fenvs.2024.1337857/full#supplementary-material
References
Andersen, H. E., Blicher-Mathiesen, G., Bechmann, M., Povilaitis, A., Iital, A., Lagzdins, A., et al. (2014). Reprint of Mitigating diffuse nitrogen losses in the Nordic-Baltic countries. Agric. Ecosyst. Environ. 198, 127–134. doi:10.1016/j.agee.2014.05.023
APHA (2005). Standard methods for the examination of water and wastewater. Washington, DC: American Public Health Association (APHA).
Askew, F. E., and Smith, R. K. (2005). “Inorganic non metallic constituents; Phosphorus; Method 4500-P F. Automated ascorbic acid reduction method,” in Standard Methods for the Examination of Waters and Waste Water. 21st edn, Editor A. Bryman (Washington, DC: American Public Health Association), 4–155.
Baker, B. H., Kröger, R., Prevost, J. D., Pierce, T., Ramirez-Avila, J. J., Czarnecki, J. M. P., et al. (2016). A field-scale investigation of nutrient and sediment reduction efficiencies of a low-technology best management practice: low-grade weirs. Ecol. Eng. 91, 240–248. doi:10.1016/j.ecoleng.2016.02.038
Bradley, C., Byrne, C., Craig, M., and Free, G. (2015). Water quality in Ireland 2010 - 2012 | executive summary report. Available at: http://www.epa.ie/pubs/reports/water/waterqua/wqr20102012/WaterQualityReport.pdf.
Carstensen, M. V., Hashemi, F., Hoffmann, C. C., Zak, D., Audet, J., and Kronvang, B. (2020). Efficiency of mitigation measures targeting nutrient losses from agricultural drainage systems: a review. Ambio 49 (11), 1820–1837. doi:10.1007/s13280-020-01345-5
Chen, D., Dahlgren, R. A., and Lu, J. (2013). A modified load apportionment model for identifying point and diffuse source nutrient inputs to rivers from stream monitoring data. J. Hydrology 501, 25–34. doi:10.1016/j.jhydrol.2013.07.034
Chorley, R. J., and Kennedy, B. A. (1971). Physical geography: a systems Approach. London: Prentice-Hall International.
Clagnan, E., Thornton, S. F., Rolfe, S. A., Wells, N. S., Knoeller, K., and Fenton, O. (2018). Investigating “net” provenance, N source, transformation and fate within hydrologically isolated grassland plots. Agric. Water Manag. 203 (February), 1–8. doi:10.1016/j.agwat.2018.02.031
Clagnan, E., Thornton, S. F., Rolfe, S. A., Wells, N. S., Knoeller, K., Murphy, J., et al. (2019). An integrated assessment of nitrogen source, transformation and fate within an intensive dairy system to inform management change. PLoS ONE 14 (7), 1–22. doi:10.1371/journal.pone.0219479
Corbett, D., Lynch, B., Wall, D. P., and Tuohy, P. (2022a). The response of finely textured and organic soils to lime and phosphorus application: results from an incubation experiment. Soil Use Manag. 39, 368–384. doi:10.1111/sum.12825
Corbett, D., Wall, D. P., Lynch, M. B., and Tuohy, P. (2022b). The influence of phosphorus application and varying soil pH on soil and herbage properties across a range of grassland soils with impeded drainage. J. Agric. Sci. 160, 516–527. doi:10.1017/s0021859622000363
Daly, K., Tuohy, P., Peyton, D., Wall, D. P., and Fenton, O. (2017). Field soil and ditch sediment phosphorus dynamics from two artificially drained fields on poorly drained soils. Agric. Water Manag. 192, 115–125. doi:10.1016/j.agwat.2017.07.005
Deelstra, J., Iital, A., Povilaitis, A., Kyllmar, K., Greipsland, I., Blicher-Mathiesen, G., et al. (2014). Reprint of “Hydrological pathways and nitrogen runoff in agricultural dominated catchments in Nordic and Baltic countries.”. Agric. Ecosyst. Environ. 198, 65–73. doi:10.1016/j.agee.2014.06.032
Dollinger, J., Dagès, C., Bailly, J. S., Lagacherie, P., and Voltz, M. (2015). Managing ditches for agroecological engineering of landscape. A review. Agron. Sustain. Dev. 35 (3), 999–1020. doi:10.1007/s13593-015-0301-6
Edwards, A. C., Kay, D., McDonald, A. T., Francis, C., Watkins, J., Wilkinson, J. R., et al. (2008). Farmyards, an overlooked source for highly contaminated runoff. J. Environ. Manag. 87(4), 551–559. doi:10.1016/j.jenvman.2006.06.027
Edwards, A. C., and Withers, P. J. A. (2008). Transport and delivery of suspended solids, nitrogen and phosphorus from various sources to freshwaters in the UK. J. Hydrology 350 (3–4), 144–153. doi:10.1016/j.jhydrol.2007.10.053
EPA Ireland. (2020). Council Directive of 12 December 1991 concerning the protection of waters against pollution caused by nitrates from agricultural sources (91/676/EEC) Article 10 Report for Ireland for the Period 2016-2019: Vol. 375/1.
ESRI (2024). ArcGIS field maps mobile. Software version 21.4.0 https://www.esri.com/en-us/arcgis/products/arcgis-field-maps).
Ezzati, G., Fenton, O., Healy, M. G., Christianson, L., Feyereisen, G. W., Thornton, S., et al. (2020). Impact of P inputs on source-sink P dynamics of sediment along an agricultural ditch network. J. Environ. Manag. 257 (March), 109988. doi:10.1016/j.jenvman.2019.109988
Faust, D. R., Kröger, R., Moore, M. T., and Rush, S. A. (2018). Management practices used in agricultural drainage ditches to reduce gulf of Mexico hypoxia. Bull. Environ. Contam. Toxicol. 100 (1), 32–40. doi:10.1007/s00128-017-2231-2
Fealy, R., Green, S., Loftus, M., Meehan, R., Radford, T., Cronin, C., et al. (2009). Teagasc EPA soils and subsoils mapping Project final report.
Fenton, O., Tuohy, P., Daly, K., Moloney, T., Rice, P., and Murnane, J. G. (2021). A review of on-farm roadway runoff characterisation and potential management options for Ireland. Water Air Soil Pollut. 232 (3), 89. doi:10.1007/s11270-021-05027-0
Fu, W., Tunney, H., and Zhang, C. (2010). Spatial variation of soil nutrients in a dairy farm and its implications for site-specific fertilizer application. Soil Tillage Res. 106 (2), 185–193. doi:10.1016/j.still.2009.12.001
Goeller, B. C., Febria, C. M., McKergow, L. A., Harding, J. S., Matheson, F. E., Tanner, C. C., et al. (2020). Combining tools from edge-of-field to in-stream to attenuate reactive Nitrogen along small agricultural water ways. WaterSwitzerl. 12 (2), 383. doi:10.3390/w12020383
Granger, S. J., Bol, R., Anthony, S., Owens, P. N., White, S. M., and Haygarth, P. M. (2010). “Towards a holistic classification of diffuse agricultural water pollution from intensively managed grasslands on heavy soils,” in Advances in agronomy (Elsevier Inc). 105. doi:10.1016/S0065-2113(10)05003-0
Gramlich, A., Stoll, S., Stamm, C., Walter, T., and Prasuhn, V. (2018). Effects of artificial land drainage on hydrology, nutrient and pesticide fluxes from agricultural fields—a review Agriculture, Ecosystems and Environment 266, 84–99. doi:10.1016/j.agee.2018.04.005
Grenon, G., Singh, B., de Sena, A., Madramootoo, C. A., von Sperber, C., Goyal, M. K., et al. (2021). Phosphorus fate, transport and management on subsurface drained agricultural organic soils: a review. Environ. Res. Lett. 16 (1), 013004. doi:10.1088/1748-9326/abce81
Harrison, S., McAree, C., Mulville, W., and Sullivan, T. (2019). The problem of agricultural ‘diffuse’ pollution: getting to the point. Sci. Total Environ. 677, 700–717. doi:10.1016/j.scitotenv.2019.04.169
Hertzberger, A., Pittelkow, C. M., Harmel, R. D., and Christianson, L. E. (2019). The MANAGE Drain Concentration database: a new tool compiling North American drainage nutrient concentrations. Agric. Water Manag. 216, 113–117. doi:10.1016/j.agwat.2019.01.021
Herzon, I., and Helenius, J. (2008). Agricultural drainage ditches, their biological importance and functioning. Biol. Conserv. 141 (5), 1171–1183. doi:10.1016/j.biocon.2008.03.005
Hodaj, A., Bowling, L. C., Frankenberger, J. R., and Chaubey, I. (2017). Impact of a two-stage ditch on channel water quality. Agric. Water Manag. 192, 126–137. doi:10.1016/j.agwat.2017.07.006
Huebsch, M., Horan, B., Blum, P., Richards, K. G., Grant, J., and Fenton, O. (2013). Impact of agronomic practices of an intensive dairy farm on nitrogen concentrations in a karst aquifer in Ireland. Agric. Ecosyst. Environ. 179, 187–199. doi:10.1016/j.agee.2013.08.021
Hunting, E. R., Vonk, J. A., Musters, C. J. M., Kraak, M. H. S., and Vijver, M. G. (2016). Effects of agricultural practices on organic matter degradation in ditches. Sci. Rep. 6 (February), 21474–21479. doi:10.1038/srep21474
Hyvönen, N. P., Huttunen, J. T., Shurpali, N. J., Lind, S. E., Marushchak, M. E., Heitto, L., et al. (2013). The role of drainage ditches in greenhouse gas emissions and surface leaching losses from a cutaway peatland cultivated with a perennial bioenergy crop. Boreal Environ. Res. 18 (2), 109–126. https://helda.helsinki.fi/server/api/core/bitstreams/24802e65-d3ee-4965-9532-e479cad10fa6/content
Ibrahim, T. G., Fenton, O., Richards, K. G., Fealy, R. M., and Healy, M. G. (2013). Spatial and temporal variations of nutrient loads in overland flow and subsurface drainage from a marginal land site in south-east Ireland. Biol. Environ. 113 B (2), 1–18. doi:10.3318/BIOE.2013.13
Ibrahim, T. G., Goutelle, A., Healy, M. G., Brennan, R., Tuohy, P., Humphreys, J., et al. (2015). Mixed agricultural pollutant mitigation using woodchip/pea gravel and woodchip/zeolite permeable reactive interceptors. doi:10.1007/s11270-015-2335-4
Infusino, E., Guagliardi, I., Gaglioti, S., and Caloiero, T. (2022). Vulnerability to nitrate occurrence in the spring waters of the sila massif (calabria, southern Italy). Toxics 10 (3), 137. doi:10.3390/toxics10030137
King, K. W., Williams, M. R., Macrae, M. L., Fausey, N. R., Frankenberger, J., Smith, D. R., et al. (2015). Phosphorus transport in agricultural subsurface drainage: a review. J. Environ. Qual. 44 (2), 467–485. doi:10.2134/jeq2014.04.0163
Kröger, R., Holland, M. M., Moore, M. T., and Cooper, C. M. (2007). Hydrological variability and agricultural drainage ditch inorganic nitrogen reduction capacity. J. Environ. Qual. 36 (6), 1646–1652. doi:10.2134/jeq2006.0506
Kröger, R., Holland, M. M., Moore, M. T., and Cooper, C. M. (2008). Agricultural drainage ditches mitigate phosphorus loads as a function of hydrological variability. J. Environ. Qual. 37 (1), 107–113. doi:10.2134/jeq2006.0505
Kröger, R., Pierce, S. C., Littlejohn, K. A., Moore, M. T., and Farris, J. L. (2012). Decreasing nitrate-N loads to coastal ecosystems with innovative drainage management strategies in agricultural landscapes: an experimental approach. Agric. Water Manag. 103, 162–166. doi:10.1016/j.agwat.2011.11.009
Littlejohn, K. A., Poganski, B. H., Kröger, R., and Ramirez-Avila, J. J. (2014). Effectiveness of low-grade weirs for nutrient removal in an agricultural landscape in the Lower Mississippi Alluvial Valley. Agric. Water Manag. 131, 79–86. doi:10.1016/j.agwat.2013.09.001
Liu, W., Youssef, M. A., Birgand, F. P., Chescheir, G. M., Tian, S., and Maxwell, B. M. (2020). Processes and mechanisms controlling nitrate dynamics in an artificially drained field: insights from high-frequency water quality measurements. Agric. Water Manag. 232 (January), 106032. doi:10.1016/j.agwat.2020.106032
Mattila, T. J., and Ezzati, G. (2022). A common agricultural soil test can identify legacy P hotspots in a drainage ditch network. J. Environ. Manag. 302, 113876. doi:10.1016/j.jenvman.2021.113876
Mockler, E. M., Deakin, J., Archbold, M., Gill, L., Daly, D., and Bruen, M. (2017). Sources of nitrogen and phosphorus emissions to Irish rivers and coastal waters: estimates from a nutrient load apportionment framework. Sci. Total Environ. 601–602, 326–339. doi:10.1016/j.scitotenv.2017.05.186
Moeder, M., Carranza-Diaz, O., López-Angulo, G., Vega-Aviña, R., Chávez-Durán, F. A., Jomaa, S., et al. (2017). Potential of vegetated ditches to manage organic pollutants derived from agricultural runoff and domestic sewage: a case study in Sinaloa (Mexico). Sci. Total Environ. 598, 1106–1115. doi:10.1016/j.scitotenv.2017.04.149
Moloney, T., Fenton, O., and Daly, K. (2020). Ranking connectivity risk for phosphorus loss along agricultural drainage ditches. Sci. Total Environ. 703, 134556. doi:10.1016/j.scitotenv.2019.134556
Monaghan, R. M., Smith, L. C., and Muirhead, R. W. (2016). Pathways of contaminant transfers to water from an artificially-drained soil under intensive grazing by dairy cows. Agric. Ecosyst. Environ. 220, 76–88. doi:10.1016/j.agee.2015.12.024
Moore, M. T., Kröger, R., Locke, M. A., Cullum, R. F., Steinriede, R. W., Testa, S., et al. (2010). Nutrient mitigation capacity in Mississippi Delta, USA drainage ditches. Environ. Pollut. 158 (1), 175–184. doi:10.1016/j.envpol.2009.07.024
Necpalova, M., Fenton, O., Casey, I., and Humphreys, J. (2012). N leaching to groundwater from dairy production involving grazing over the winter on a clay-loam soil. Sci. Total Environ. 432, 159–172. doi:10.1016/j.scitotenv.2012.05.091
Needelman, B. A., Kleinman, P. J. A., Strock, J. S., and Allen, A. L. (2007). Improved management of agricultural drainage ditches for water quality protection: an overview. J. soil water conservation 62. https://link.gale.com/apps/doc/A168739819/AONE?u=anon∼1f1952a2sid=googleScholarxid=1474fa52
O’Callaghan, P., Kelly-Quinn, M., Jennings, E., Antunes, P., O’Sullivan, M., and Fenton, O. (2018). Impact of Cattle Access to Watercourses : Literature Review on Behalf of the COSAINT Project. Environmental Protection Agency. Issue 260.
Paul, O. C., Kelly-Quinn, M., Jennings, E., Antunes, P., Matt, O. S., and hUallacháin, D. O. (2018). Impact of cattle access to watercourses: literature review on behalf of the COSAINT Project. Environ. Prot. Agency 260, 60. Available at: www.epa.ie/publications/research/land-use-soils-and-transport/Research_Report_260.pdf
Penn, C., Livingston, S., Shedekar, V., King, K., and Williams, M. (2020). Performance of field-scale phosphorus removal structures utilizing steel slag for treatment of subsurface drainage. WaterSwitzerl. 12 (2), 443. doi:10.3390/w12020443
Pieterse, N. M., Bleuten, W., and Jørgensen, S. E. (2003). Contribution of point sources and diffuse sources to nitrogen and phosphorus loads in lowland river tributaries. J. Hydrology 271 (1–4), 213–225. doi:10.1016/S0022-1694(02)00350-5
Rice, P., Daly, K., Tuohy, P., Murnane, J. G., Nag, R., and Fenton, O. (2022). Evaluating connectivity risk of farm roadway runoff with waters - development and sensitivity analysis of a semi quantitative risk model. Sci. Total Environ. 851, 158114. doi:10.1016/j.scitotenv.2022.158114
Schoumans, O. F., Chardon, W. J., Bechmann, M. E., Gascuel-Odoux, C., Hofman, G., Kronvang, B., et al. (2014). Mitigation options to reduce phosphorus losses from the agricultural sector and improve surface water quality: a review. Sci. Total Environ., 1255–1266. doi:10.1016/j.scitotenv.2013.08.061
Sherriff, S. C., Rowan, J. S., Fenton, O., Jordan, P., and Ó hUallacháin, D. (2018). Sediment fingerprinting as a tool to identify temporal and spatial variability of sediment sources and transport pathways in agricultural catchments. Agric. Ecosyst. Environ. 267 (September), 188–200. doi:10.1016/j.agee.2018.08.023
Soana, E., Balestrini, R., Vincenzi, F., Bartoli, M., and Castaldelli, G. (2017). Mitigation of nitrogen pollution in vegetated ditches fed by nitrate-rich spring waters. Agric. Ecosyst. Environ. 243, 74–82. doi:10.1016/j.agee.2017.04.004
Sukias, J., Nguyen, L., Nagels, J., and Reeves, P. (2003). Drainage ditches as sinks for attenuating n and p pollutants from dairy farms. Diffuse Pollut. Conf. Dublin, 26–30. Agriculture. https://www.ucd.ie/dipcon/docs/theme03/theme03_05.PDF
Tanner, C. C., Nguyen, M. L., and Sukias, J. P. S. (2005). Nutrient removal by a constructed wetland treating subsurface drainage from grazed dairy pasture 105, 145–162. doi:10.1016/j.agee.2004.05.008
Teagasc (2022). “Teagasc Manual on Drainage and soil management,” in A best practice manual for Ireland’s Farmers. 2nd Edn. Editors P. Tuohy, O. Fenton, and M. Moore (Carlow: Teagasc).
Tiemeyer, B., and Kahle, P. (2014). Nitrogen and dissolved organic carbon (DOC) losses from an artificially drained grassland on organic soils. Biogeosciences 11 (15), 4123–4137. doi:10.5194/bg-11-4123-2014
Tuohy, P., Humphreys, J., Holden, N. M., and Fenton, O. (2016). Runoff and subsurface drain response from mole and gravel mole drainage across episodic rainfall events. Agric. Water Manag. 169, 129–139. doi:10.1016/j.agwat.2016.02.020
Tuohy, P., O’ Loughlin, J., Peyton, D., and Fenton, O. (2018). The performance and behavior of land drainage systems and their impact on field scale hydrology in an increasingly volatile climate. Agric. Water Manag. 210 (August), 96–107. doi:10.1016/j.agwat.2018.07.033
Tuohy, P., O’Sullivan, L., and Fenton, O. (2021). Field scale estimates of soil carbon stocks on ten heavy textured farms across Ireland. J. Environ. Manag. 281 (December 2020), 111903. doi:10.1016/j.jenvman.2020.111903
Valbuena-Parralejo, N., Fenton, O., Tuohy, P., Williams, M., Lanigan, G. J., and Humphreys, J. (2019). Phosphorus and nitrogen losses from temperate permanent grassland on clay-loam soil after the installation of artificial mole and gravel mole drainage. Sci. Total Environ. 659, 1428–1436. doi:10.1016/j.scitotenv.2018.12.173
Van Der Grift, B., Broers, H. P., Berendrecht, W., Rozemeijer, J., Osté, L., and Griffioen, J. (2016). High-frequency monitoring reveals nutrient sources and transport processes in an agriculture-dominated lowland water system. Hydrology Earth Syst. Sci. 20 (5), 1851–1868. doi:10.5194/hess-20-1851-2016
Vedder, M. (2020). Bovine faecal contamination in an Irish agricultural catchment: sources and pathways. [University College Cork] http://hdl.handle.net/10468/11269.
Wall, D., and Plunkett, M. (2020). Major and micro nutrient advice for productive agricultural crops. Wexford, Ireland: Teagasc. 180.
Wilkinson, M. E., Quinn, P. F., Barber, N. J., and Jonczyk, J. (2014). A framework for managing runoff and pollution in the rural landscape using a Catchment Systems Engineering approach. Sci. Total Environ. 468–469, 1245–1254. doi:10.1016/j.scitotenv.2013.07.055
Wuijts, S., Frathers, R., Sandra, B., and Van Duijnen, R. (2022). Monitoring of nitrogen in water in the EU. http://www.europarl.europa.eu/supporting-analyses%0AACKNOWLEDGEMENT.
Keywords: water quality, nutrient loss, grassland, drainage management, connectivity pathways, North Atlantic Europe, agricultural ditches
Citation: Opoku DG, Healy MG, Fenton O, Daly K, Condon T and Tuohy P (2024) An integrated connectivity risk ranking for phosphorus and nitrogen along agricultural open ditches to inform targeted and specific mitigation management. Front. Environ. Sci. 12:1337857. doi: 10.3389/fenvs.2024.1337857
Received: 13 November 2023; Accepted: 23 January 2024;
Published: 19 February 2024.
Edited by:
Alan Steinman, Annis Water Resources Institute, United StatesReviewed by:
Adam Canning, James Cook University, AustraliaKatelyn Lawson, Auburn University, United States
Copyright © 2024 Opoku, Healy, Fenton, Daly, Condon and Tuohy. This is an open-access article distributed under the terms of the Creative Commons Attribution License (CC BY). The use, distribution or reproduction in other forums is permitted, provided the original author(s) and the copyright owner(s) are credited and that the original publication in this journal is cited, in accordance with accepted academic practice. No use, distribution or reproduction is permitted which does not comply with these terms.
*Correspondence: P. Tuohy, cGF0cmljay5UdW9oeUB0ZWFnYXNjLmll