- 1Division of Applied Life Science (BK21+Program), Gyeongsang National University, Jinju, Republic of Korea
- 2Institute of Agriculture and Life Sciences, Gyeongsang National University, Jinju, Republic of Korea
- 3Department of Soil Science, College of Agriculture, Central Mindanao University, Maramag, Philippines
- 4POSCO Co., Ltd., Pohang, Republic of Korea
Iron slag-based silicate fertilizer (SF) has been utilized as a soil amendment in rice paddy fields for over 50 years. SF, which contains electron acceptors such as oxidized iron (Fe3+) compounds, is known to reduce methane (CH4) emissions, which have a global warming potential (GWP) of 23, higher than that of carbon dioxide (CO2). However, the dynamics of nitrous oxide (N2O), which has a GWP of 265, were questionable. Since the reduced Fe (Fe2+) can react as an electron donor, SF application might suppress N2O emissions by progressing N2O into nitrogen gas (N2) during the denitrification process. To verify the influence of SF application on two major greenhouse gas (GHG) dynamics during rice cultivation, three different kinds of SF were prepared by mixing iron rust (>99%, Fe2O3) as an electron acceptor with different ratios (0, 2.5, and 5%) and applied at the recommended level (1.5 Mg ha−1) for rice cultivation. SF application was effective in decreasing CH4 emissions in the earlier rice cropping season, and seasonal CH4 flux was more highly decreased with increasing the mixing ratio of iron rust from an average of 19% to 38%. Different from CH4 emissions, approximately 70% of seasonal N2O flux was released after drainage for rice harvesting. However, SF incorporation was very effective in decreasing N2O emissions by approximately 40% over the control. Reduced Fe2+ can be simultaneously oxidized into Fe3+ by releasing free electrons. The increased electron availability might develop more denitrification processes into N2 gas rather than NO and N2O and then decrease N2O emissions in the late rice cultivation season. We could find evidence of a more suppressed N2O flux by applying the electron acceptor-added SFs (SF2.5 and SF5.0) to a 49%–56% decrease over the control. The SF application was effective in increasing rice productivity, which showed a negative-quadratic response to the available silicate (SiO2) concentration in the soil at the harvesting stage. Grain yield was maximized at approximately 183 mg kg−1 of the available SiO2 concentration in the Korean rice paddy, with a 16% increase over no-SF application. Consequently, SF has an attractive potential as a soil amendment in rice paddy to decrease GHG emission impacts and increase rice productivity.
1 Introduction
Iron slag-based silicate fertilizer (SF) has been utilized as a soil amendment for >50 years to improve soil productivity in East Asian rice paddies (Ohta et al., 1953; Park, 2001; Makabe-Sasaki et al., 2014). SF, which is made from blast furnace slag (BFS), a byproduct from iron-making factories, primarily consists of silicate (SiO2) and calcium oxide (CaO) derived from iron ore and added lime, respectively (Horii et al., 2015; Piatak et al., 2015). In Korea and Japan, SF has been subsidized by the government to improve soil quality and rice productivity (Park, 2001; Makabe-Sasaki et al., 2014).
SF application can improve plant resistance against climatic vulnerabilities and abiotic–biotic stresses (Bokor et al., 2021). In particular, rice uptakes SiO2 by approximately 10% of its own biomass (Luyckx et al., 2017). Since SiO2 accumulates in the epidermal cell, it is known to protect itself against pathogens and lodging damage (Ma et al., 2006; Meharg and Meharg, 2015). On the other hand, the accumulated SiO2 can improve rice plant erectness and then enhance photosynthetic activity (Cooke and Leishman, 2011; Dorairaj et al., 2017). In a 28-year field experiment in Korea, 1.5 Mg ha−1 of periodic SF application enhanced rice grain productivity by an average of 14% over no amendment (Lim et al., 2022).
SF also contains high contents of oxidized iron (Fe3+) and was known to decrease methane (CH4) emissions during the rice cropping period (Ali et al., 2008; Wang et al., 2019; Galgo et al., 2022). CH4 is biologically formed via the decomposition of organic matter and the reaction of carbon dioxide (CO2) and hydrogen (H2) by methanogens under strongly anaerobic conditions (less than minus 200 mV of soil Eh value) (Christy et al., 2014). The activities of electron acceptors and donors can play a dynamic role in regulating CH4 production and consumption (Madsen, 2011). The oxidized ion can accept free electrons under extremely reduced soil conditions and suppress methanogenesis. However, its acceptability has higher power in the order of O2, NO3, Mn4+, Fe3+, SO42+, and CO2 (Keller et al., 2009; Gao et al., 2019). Overall, the electron acceptors, which have higher acceptability than CO2, can compete with methanogens and then suppress methanogenesis (Frenzel et al., 1999; Kumaraswamy et al., 2001).
Nitrous oxide (N2O) is biologically formed by denitrification from nitrite (NO2−), which is an electron-donating process (Schreiber et al., 2012; Heil et al., 2016). NO2− can be denitrified into nitrogen oxide (NO), N2O, and nitrogen gas (N2); however, with higher amounts of free electrons, NO2− might be converted into N2 gas rather than NO and N2O (Chandran et al., 2011). This implies that N2O flux can theoretically be determined by electron availability (Kool et al., 2011). Fe3+, which was added by SF, might react as an electron acceptor and then suppress methanogenesis by consuming free electrons, especially during the earlier rice cropping season. However, floodwater is generally drained 1 month before harvesting in rice paddy, and subsequently, the reduced soil is oxidized at the later cropping season. We hypothesized that reduced Fe (Fe2+) can be simultaneously oxidized, which can donate more free electrons to the denitrification process. This process can develop a stronger denitrification process into N2 gas rather than NO and N2O (Wang et al., 2016; Wang et al., 2020). Therefore, SF addition might decrease N2O flux in rice paddy, especially during the later rice cropping season. Since N2O has 265 times higher GWP than CO2, a small reduction in N2O flux can strongly influence mitigating global warming. However, the effect of SF on N2O flux was not properly verified during rice cropping season.
In these 2-year field studies, to investigate the influence of Fe3+ as an electron acceptor on two major GHG (CH4 and N2O) dynamics, three different kinds (SF0, SF2.5, and SF5.0) of SF were prepared by mixing the different rates (0, 2.5, and 5%, respectively) of iron rust (>99% Fe2O3) as an electron acceptor, and two GHG fluxes were characterized under the recommended level (1.5 Mg ha−1) of SF application.
2 Materials and methods
2.1 Experimental plot installation and rice cultivation
In the agricultural experimental station (35.15° N, 128.10° E) at Gyeongsang National University, Jinju, South Korea, the experimental field was prepared in a typical mono-rice paddy. In the selected rice paddy, only rice was cultivated for over 5 decades during the warm season, but the soil was not managed during the cold fallow season. The field was poorly drained with a silty clay loam (SiCL) texture under the USDA taxonomy classification. Before the experiment, the surface soil (0–15 cm) had a neutral pH (6.1) but low fertility with 16.7 g kg−1 of organic matter, 0.88 g kg−1 of total nitrogen (N), and 154 mg kg−1 of available SiO2.
SF and no-SF applications were prepared as the main treatment. SF was made from BFS, which primarily consisted of CaO (44%), SiO2 (35%), and Al2O3 (14%), with a high material pH (12.8). In the SF treatment, three different kinds of SFs (SF0, SF2.5, and SF5) were added as sub-treatments. These SFs were prepared by adding iron rust as an electron acceptor with rates of 0, 2.5, and 5.0% (wt wt−1), respectively. Iron rust had a neutral pH (6.7) and was mainly composed of Fe2O3 (>99%) (Galgo et al., 2022). A total of twelve plots (four treatments × three replications) were randomly placed in the selected field. Each plot had a size of 100 m2 (10 m × 10 m). In the SF treatments, the recommended level (1.5 Mg ha−1) of SF was applied 1 week before rice transplanting and then mechanically plowed within the surface soil (RDA, 2019).
In early June 2020 and 2021, 21 -day-old rice seedlings (Japonica type, Ilmi cultivar) were manually transplanted with a 30 cm × 15 cm planting distance. Except for the SF application, all plots were controlled under the same practices. The recommended levels of chemical fertilizers for rice cultivation were applied with the levels of N–P2O5–K2O = 90–45–57 kg ha−1, respectively. Throughout the rice cropping season, the soil was steadily flooded using groundwater to a depth of 5–10 cm and drained 1 month before rice harvesting. In mid-October of the same years, the aboveground biomass of rice plants was harvested to investigate yield properties.
2.2 Greenhouse gas flux measurement
To evaluate GHG (CH4 and N2O) fluxes, the static chamber method was used (Rolston, 1986). During rice cultivation, three sets of rectangular parallelepiped acrylic chambers (H. 120 cm x W. 60 cm x L. 60 cm) were installed in each plot. A thermometer and two electrical fans were equipped to record the temperature and homogenize the gas inside the chamber, respectively. A total of eight rice plants were transplanted into the chamber with the same planting distance on the outside. The chambers were kept open during the entire investigation period, except for gas sampling.
Before the start of regular gas samplings, the research protocol was established to set sampling conditions such as sampling hours, intervals, and times. In the preliminary measurement, 30 min was selected as the chamber lid closing hour to collect gas samples since the positive linear relationships between gas concentration and chamber closing hour (0, 10-, 20-, 30-, and 60-min chamber closing) were found.
Gas samples were collected weekly between 10:00 and 11:00 a.m., at 0 and 30 min after the chamber closing. Gases were sampled using an air-tight plastic syringe (30 mL) and then directly transferred into 20-mL vacuumed glass vials for analysis. Gas chromatography (GC) was used to quantify CH4 and N2O concentrations. A ShinCarbon ST micropacked column and a flame ionization detector (FID) with a methanizer were used to analyze CH4 concentration. A 63Ni electron capture detector (ECD) and capillary column (HP-PLOT/A capillary column) were selected to evaluate the N2O concentration. The column, injector, and detector were programmed with a constant temperature of 35, 200, and 200 C, respectively.
The increased concentration was used to calculate gas emission rates (Rolston, 1986).
where ∆C (mL m−3) and ∆t denote the increased gas concentration in the chamber headspace and the chamber closing hour (0.5), respectively. V (m3) and A (m2) indicate the volume and surface area of the chamber, respectively. ρ (kg m−3) and T (oK) indicate the gas density (standard condition) and absolute temperature inside the chamber during gas sampling, respectively.
Daily emission rates were integrated to calculate seasonal gas fluxes (Singh et al., 1999).
where Fi is the daily gas emission rate (g m−2 day−1) at the ith sampling; Di is the interval days between the ith and (i−1)th samplings; and n means the total number of gas samplings.
2.3 Net global warming potential and greenhouse gas intensity
Two GHG (CH4 and N2O) fluxes were integrated to calculate the net GWP with the CO2 equivalent (Mosier et al., 2006).
GHG intensity (GHGI) was calculated using the net GWP per grain productivity to compare the yield scale GHG emission impact among the treatments (Shang et al., 2011).
2.4 Investigation of soil and water properties and rice yield components
Soil temperature and redox potential were continuously monitored using electric sensors and electrodes in the field during rice cultivation. The sensors and electrodes were installed at a 5–10 cm soil depth and connected to a battery-powered data logger and Eh meter, respectively.
Soil samples (0–15 cm) were collected before rice transplanting and after harvesting, air-dried, and meshed (<2 mm) to analyze chemical properties. Soil pH was measured using a water suspension (1:5 with H2O). Organic C and total N contents were quantified using the Walkley–Black and Kjeldahl digestion method, respectively. The available P2O5 concentration was measured using the Lancaster method (RDA, 2019). Exchangeable Ca2+, Mg2+, and K+ concentrations were analyzed using an ICP spectrometer (PerkinElmer) after 1 M NH4-acetate (pH 7) extraction. The available SiO2 content was analyzed using the molybdenum blue method after 1 N Na-acetate extraction (pH 4) (Yoshida et al., 1959).
Soil and floodwaters were periodically sampled during rice cultivation to monitor the changes in soluble Fe3+ and Fe2+ concentrations. Floodwater samples were simultaneously collected with gas sampling, but the soil was sampled at monthly intervals. To assay soluble Fe3+ and Fe2+ concentrations in the soil, fresh soil was extracted using distilled water. The extracted water and collected floodwater were filtered. The Fe2+ concentration was analyzed using a 1,10 phenanthroline reagent in dark conditions to prevent photoreduction of Fe3+ at 510 nm (UV spectrophotometer) (Loeppert and Inskeep, 1996). The total Fe concentration was measured through the reduction of Fe3+ to Fe2+ by adding 10% hydroxylamine hydrochloride as a reducing agent. The Fe3+ concentrations were estimated using the difference between the total Fe and Fe2+ concentrations (Olson and Ellis, 1982).
Rice plants were harvested at the maturity stage. Ten representative rice plants were collected manually from each plot. The harvested rice was air-dried, and the grain and straw were separated. Rice yield components such as tiller number, panicles per hill, 1,000 grain weight, and ripened ratio were measured according to the Korean standard for investigating rice yield properties (RDA, 2019).
2.5 Statistical analysis
IBM SPSS version 20 (SPSS Inc., Chicago, IL, United States) was selected for analyzing statistical characteristics. Variance (ANOVA) analysis was applied to all datasets, and the differences among treatments were compared using the honestly significant difference (HSD) test at a p < 0.05 probability level. Regression analyses between GHG fluxes and soluble Fe3+ and Fe2+ concentrations were conducted using SigmaPlot10.
3 Results
3.1 Changes in temperatures and soil Eh values
Soil temperature similarly fluctuated with air temperature changes (Figure 1). However, soil temperatures were not different among treatments. It gradually increased after rice transplanting, peaked at the reproductive stage between 56 and 77 days after transplanting, and thereafter gradually decreased. However, air and soil temperatures were slightly higher in the second year than in the first year. The cumulative air temperature was 2,913°C and 2,942 C in the first and second years of rice cultivation, respectively. The higher temperature in the second year might influence better rice development and higher productivity.
Soil Eh values changed with a typical pattern in the rice cropping environment. These values sharply decreased to minus 150–260 mV within 2 weeks after flooding (Figure 1). The extremely reduced soil condition, having less than minus 200 mV of soil Eh value, was maintained until water drainage for rice harvesting practices. However, soil Eh values were not different among the treatments.
3.2 Changes in methane and nitrous oxide fluxes
Regardless of soil amendment management, CH4 emission rates sharply increased after flooding for rice transplanting, peaked at the panicle initiation stage, and thereafter slowly decreased to the background level at the maturity stage (Figure 2). In the control treatment, an average of 12.53 Mg CO2-eq. ha−1 of seasonal CH4 fluxes was observed. This flux was mostly released in the early stage of rice cultivation, with approximately 80% coverage of the total flux. The conventional SF (SF0) application significantly reduced the seasonal CH4 flux by an average of 19% over the control, but CH4 emission was mostly suppressed at the early rice growing season. The electron acceptor-added SFs (SF2.5 and SF5.0), which incorporated 2.5% and 5% of iron rust into BFS, were more effective in suppressing CH4 fluxes. These two SFs increased the suppression effect of seasonal CH4 flux by approximately 36%–38% over the control, but there was no big difference between SF2.5 and SF5.0.
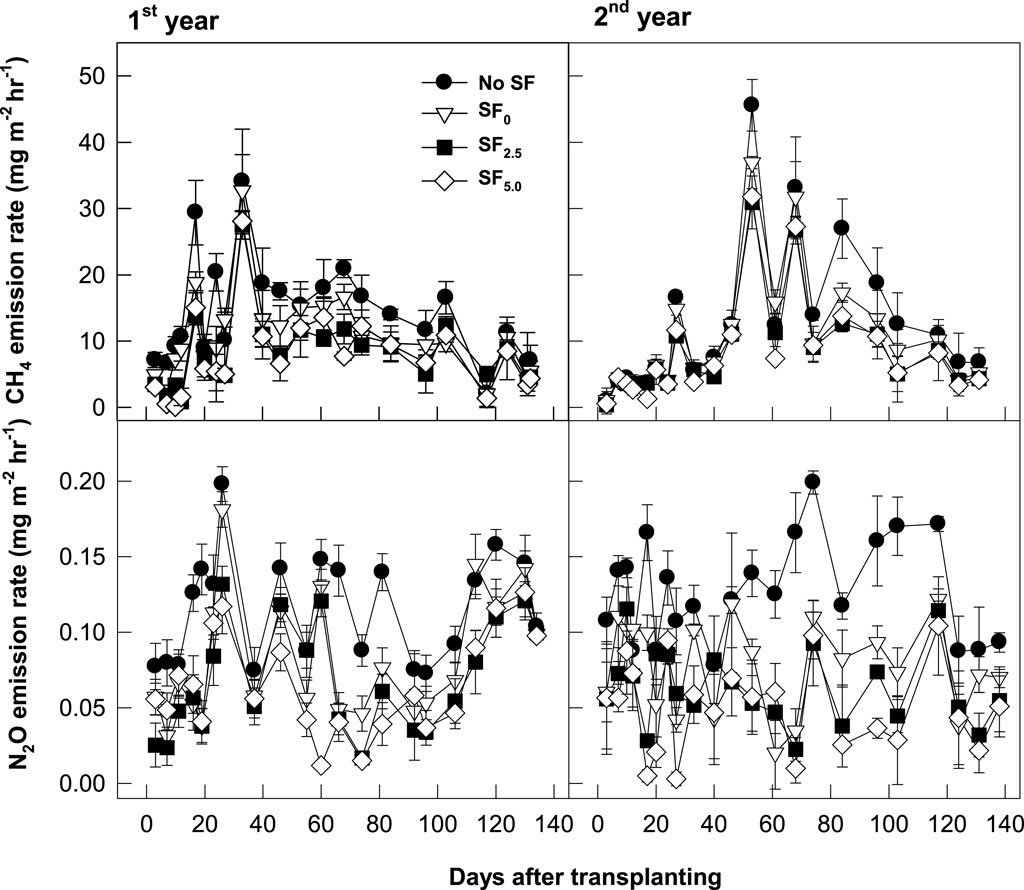
FIGURE 2. Changes in greenhouse gas (CH4 and N2O) emission rates in rice paddy soils amended with silicate fertilizer.
Different from CH4 emission changes, N2O was emitted without any specific patterns (Figure 2). In comparison, more N2O was emitted after drainage for rice harvesting. In all treatments, approximately 30% of seasonal N2O flux was observed in the early rice cropping season, but 70% of total N2O flux appeared after drainage for rice harvesting. In the control, approximately 1.0 Mg CO2-eq. ha−1 of N2O was emitted during the rice cultivation period, but the conventional SF (SF0) application significantly decreased this flux by approximately 40%. The electron acceptor-added SFs (SF2.5 and SF5.0) were more effective in decreasing seasonal N2O flux by 48%–56% over the control.
3.3 Changes in iron concentration in floodwater and soil
The changes in soluble Fe3+ and Fe2+ concentrations were monitored in the floodwater during rice cultivation (Figure 3). The soluble Fe3+ and Fe2+ concentrations fluctuated in a different pattern throughout the investigation period. Irrespective of the SF application background, the soluble Fe2+ concentration rapidly increased after irrigation, peaked at the tillering stage on 27–30 days after transplanting, and then slowly decreased to background levels after drainage for rice harvesting. In comparison, the soluble Fe3+ concentration rapidly decreased at the early stage of the rice growing season but increased again in the later stage. The conventional SF (SF0) application clearly increased Fe2+ and Fe3+ concentrations in the floodwater. However, the electron acceptor-added SFs (SF2.5 and SF5.0) more highly increased these two different forms of Fe concentrations in floodwater.
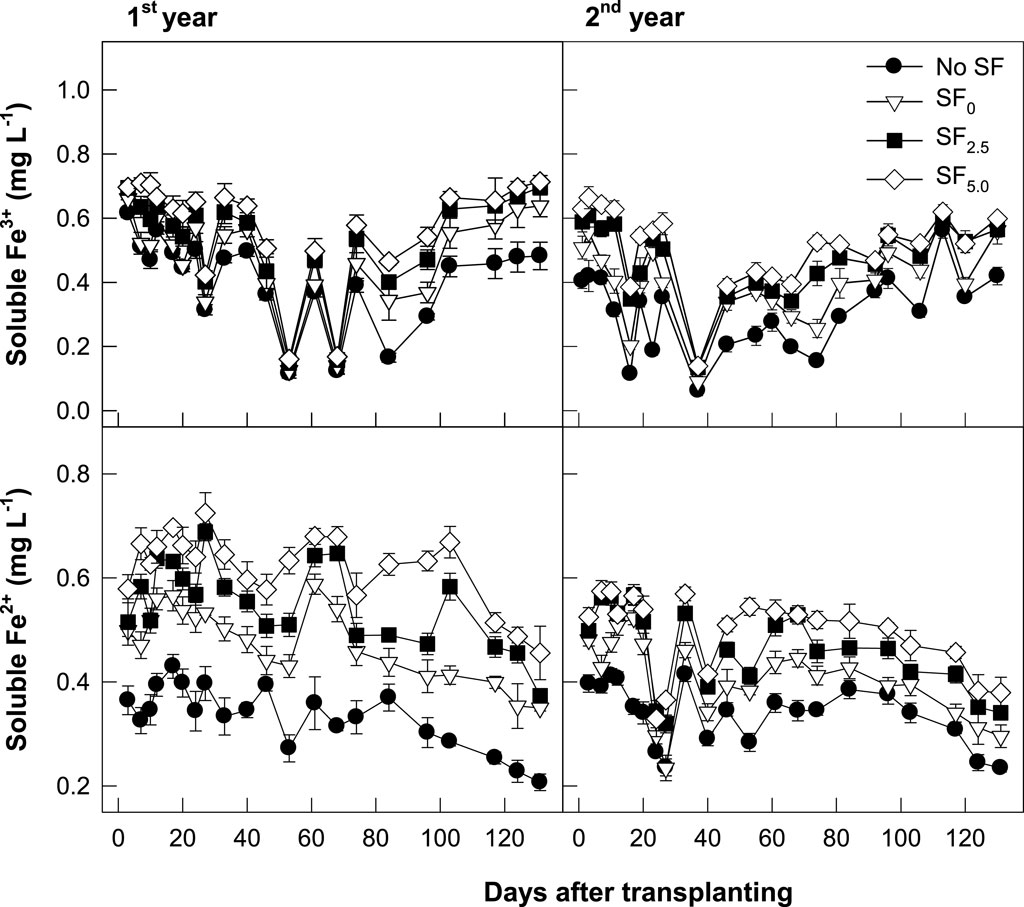
FIGURE 3. Changes in soluble Fe3+ and Fe2+ concentrations in the floodwater during the rice cropping season.
Water-soluble Fe3+ and Fe2+ concentrations in the soil were analyzed at a 1-month interval (Figure 4). Two different forms of Fe concentration were reversely changed during rice cultivation. Water-soluble Fe3+ concentration in soils decreased with decreasing soil Eh value at the early rice growing season but increased after water drainage at the late rice cultivation season. In contrast, the soluble Fe2+ concentration was highly increased with developing anoxic soil conditions, peaked at approximately 70–80 days after transplanting, but thereafter, rapidly decreased to the background level. However, soluble Fe3+ and Fe2+ concentrations entirely increased with SF application and correspondingly responded to iron rust addition.
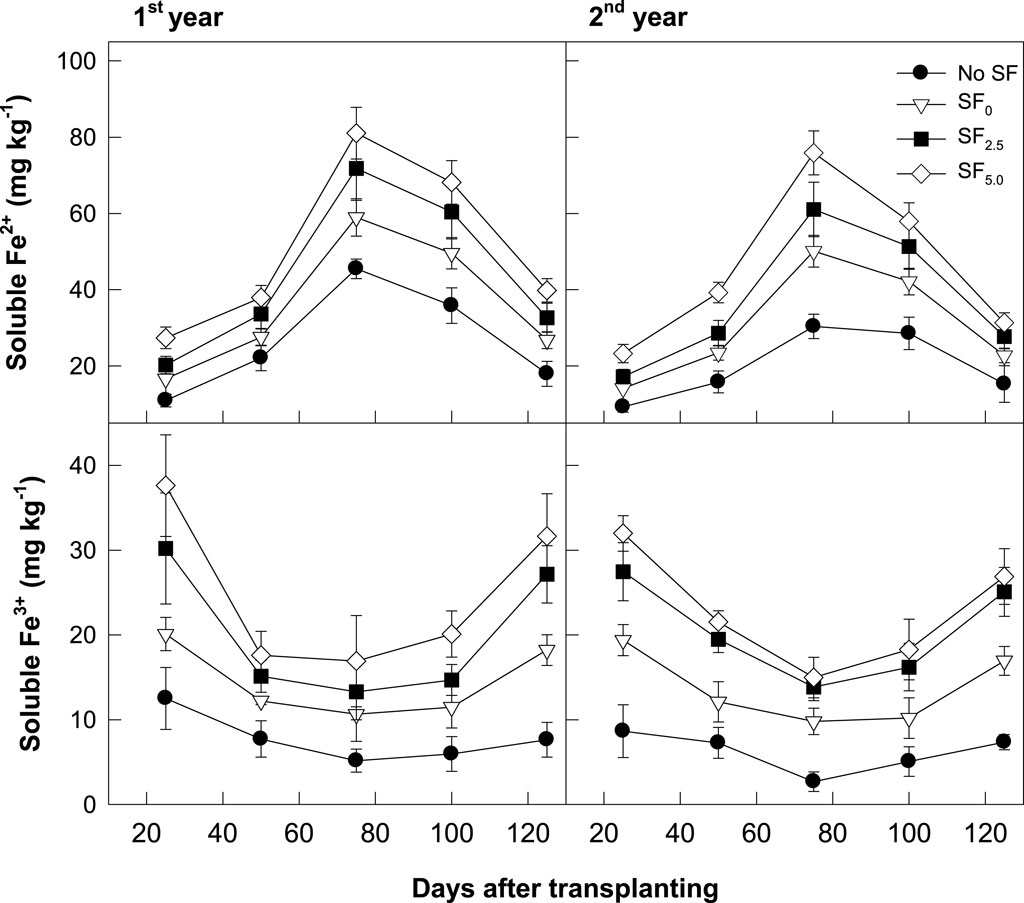
FIGURE 4. Changes in soluble Fe3+ and Fe2+ concentrations in the soil during the rice cropping season.
3.4 Global warming potential
SF application was effective in suppressing two major GHG (CH4 and N2O) fluxes during rice cultivation. Since CH4 and N2O have very big GWP values (23 and 265) over a 100-year time horizon, respectively, the net effect of SFs on mitigating global warming was compared using the net GWP, which was integrated by two GHG fluxes with CO2 equivalent. In the control treatment, total GWP was approximately 13.6 Mg CO2-eq ha−1, which was weighed by 92% and 8% of CH4 and N2O fluxes, respectively (Figure 5). However, the net GWP was slightly higher in the second year than the first year, mainly due to a higher CH4 flux.
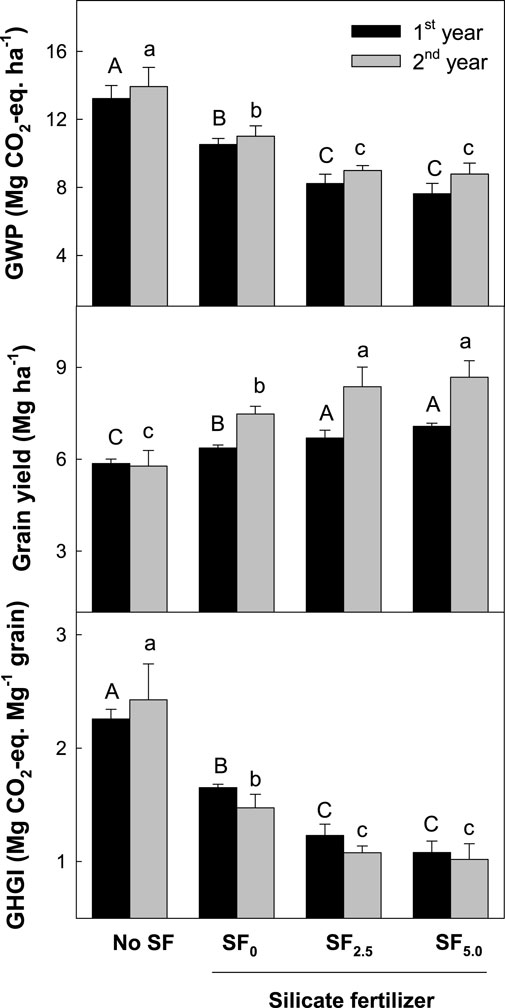
FIGURE 5. Global warming potential, grain yield, and greenhouse gas intensity in rice paddy soils applied with silicate fertilizer. The different letters above the bar indicate a significant difference between treatments (p < 0.05, Tukey test).
The conventional SF (SF0) application significantly decreased the net GWP by 21% over the control. SF0 decreased 19% and 38% of seasonal CH4 and N2O fluxes, respectively, but the reduction in net GWP was mainly affected by decreasing CH4 flux with 94% coverage. In comparison, the electron acceptor-added SFs (SF2.5 and SF5) were more effective in decreasing the net GWP by 37%–40% over the control. These SFs reduced the seasonal CH4 and N2O fluxes by 36%–38% and 49%–56% over the control, respectively. However, the decreased CH4 flux mainly influenced the significant decrease in net GWP with 94% coverage.
3.5 Rice productivity and greenhouse gas intensity
The conventional SF (SF0) application significantly increased grain yield by 16% over the control due to the increased grains per panicle and the improved 1,000 grain weight (Table 1). SF application increased grains per panicle and 1,000 grain weight by an average of 3% and 4%, respectively, but there was no change in other yield properties. The electron acceptor-added SFs (SF2.5 and SF5.0) were more effective in increasing grain productivity by 22%–25% over the control, but there was no big difference between SF2.5 and SF5.0. This yield increase was also influenced by improving grains per panicle, ripened ratio, and 1,000 grain weight. Iron rust-added SF2.5 and SF5.0 enhanced grains per panicle, ripened ratio, and 1,000 grain weight by an average of 4, 8, and 11% over the control, respectively.
GHGI, which indicates the net GWP per grain productivity, was 2.3 Mg CO2-eq Mg−1 grain in the control treatment (Figure 5). Conventional SF (SF0) significantly decreased GHGI by approximately 33% over the control due to a 16% increase in grain yield but a 21% decrease in the net GWP. In comparison, the iron rust-added SF2.5 and SF5.0 were more effective in decreasing GHGI by approximately 51%–55%. This reduction was affected by a highly reduced net GWP rather than a small yield increase.
4 Discussion
Iron slag-based SF, which contains electron acceptors such as oxidized Fe and Mn compounds, was known to decrease CH4 emissions during flooded rice cultivation (Ali et al., 2008, Huang et al., 2009; Yang and Lu, 2022). Electron acceptors play an important role in regulating CH4 production and consumption (Watanabe and Kimura, 1999; Sahrawat, 2004; Karri et al., 2005). CO2 can accept a free electron under an extremely reduced condition (less than minus 200 mV of the soil Eh value) and then produce CH4 by methanogens (Chapman et al., 1996). The oxidized ions can react as electron acceptors in anaerobic soils (Keller et al., 2009; Gao et al., 2019). The reduction of electron acceptors follows a sequence in the order of O2 > NO3− > Mn4+ > Fe3+ > SO42+ > CO2 (Keller et al., 2009; Gao et al., 2019). Generally, the electron acceptors, which have higher acceptability than CO2, can compete with methanogens and then suppress CH4 production (Frenzel et al., 1999; Kumaraswamy et al., 2001).
In this field study, conventional SF (SF0), which contains 5.4% and 0.4% of Fe and Mn oxides (Galgo et al., 2022), decreased seasonal CH4 flux by an average of 19% over the control (Figure 2). Since most of CH4 is emitted during the rice vegetative growth stage, SF mostly suppressed CH4 emissions at this early rice growing season. As an electron acceptor, a small addition (2.5%–5.0%, wt wt−1) of iron rust, which is a byproduct of the steel processing industry and contains over 99% of Fe2O3 on the iron slag-based SF, highly enhanced the suppression of CH4 emissions by 36%–38%. We also found a highly negative correlation between CH4 emission rates and soluble Fe3+ concentrations in the floodwater (Figure 6). This implies that a small addition of electron acceptors, which have higher electron acceptability than CO2, can be a soil management strategy to suppress CH4 production in rice paddy.
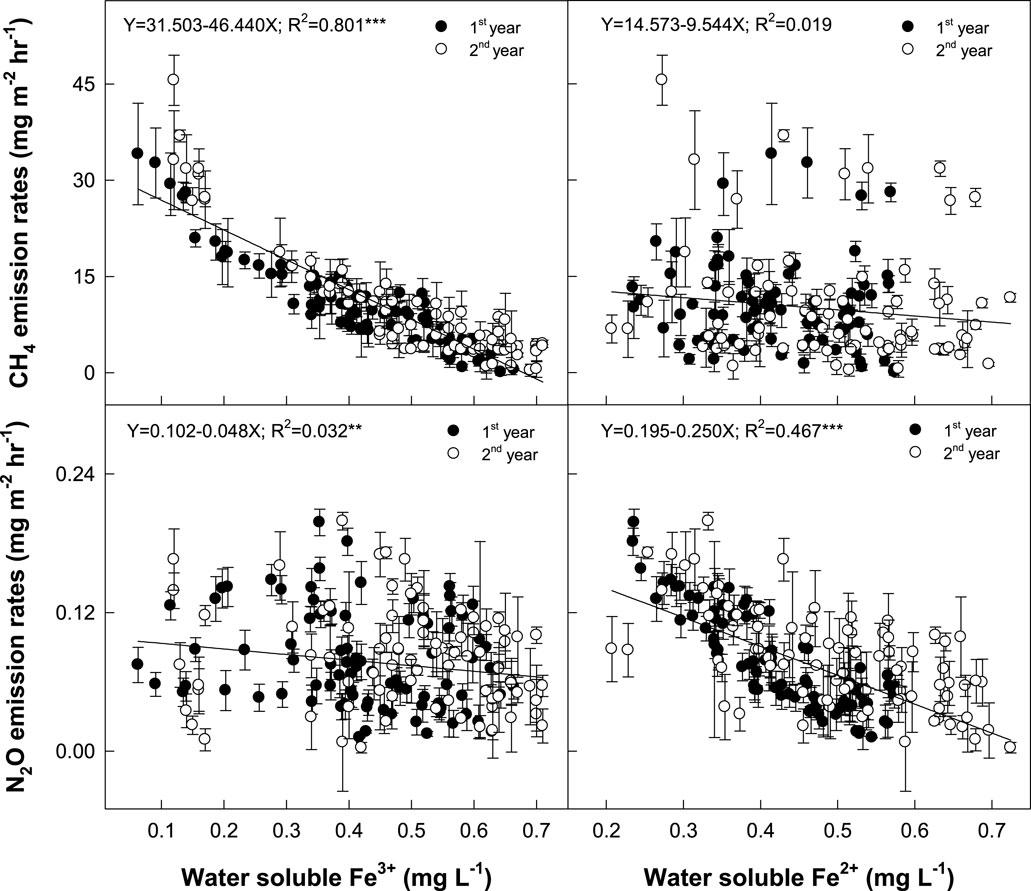
FIGURE 6. Relationship between CH4 and N2O emissions rates and soluble Fe3+ and Fe2+ in the floodwater.
Different from the general CH4 emission pattern, in which most of CH4 is emitted during the vegetative growth stage (Canatoy et al., 2023; Song and Kim, 2023), over 70% of seasonal N2O flux was emitted during the rice maturity stage (Figure 2). During the denitrification process, NO2− can progress from NO to N2O and more to N2 gas with less O2 supply (Khalil et al., 2004). Under the extremely reduced soil condition, which has less than minus 200 mV of soil Eh value and can activate methanogenesis, like during the early rice growing season, NO2− can be converted into N2 gas rather than NO and N2O. Therefore, rice paddy might have a very low N2O emission factor, which is not comparable with upland soils. The Intergovernmental Panel on Climate Change (IPCC) suggested only 0.3% of the added N source as the default value of N2O flux in the irrigated rice paddy, while 1% of the added N was emitted into N2O in the upland soils (IPCC, 2023).
SF application significantly decreased seasonal N2O flux by 38% over the control (Figure 2). N2O is biologically formed via electron donation from NO2− and NO. NO2− might be converted into N2 gas rather than NO and N2O with more free electrons (Pan et al., 2013; Young et al., 2022). Fe was known as a key factor in regulating N2O flux (Carlson et al., 2013; Zhu et al., 2013). Fe3+, which was supplied by SF application, might react as an electron acceptor and then suppress methanogenesis by consuming free electrons, especially in the earlier rice cropping season. However, water is drained 1 month before rice harvesting, and then the reduced soil is oxidized at the later cropping season. Fe2+ can be simultaneously oxidized into Fe3+ by releasing free electrons. The increased electron availability might develop more denitrification processes into N2 gas, rather than NO and N2O, and then decrease N2O emissions in the late rice cultivation season. We could find a clue from the more suppressed N2O flux by adding the electron acceptor-added SFs (49%–56% decrease over the control by SF2.5 and SF5.0, respectively) (Figure 2). The N2O emission rates were negatively correlated with soluble Fe2+ concentrations in the floodwater (Figure 6).
In rice fields, the net GWP, which was integrated by seasonal CH4 and N2O fluxes with CO2 equivalent (IPCC, 2023), was mostly determined by CH4 flux and not N2O flux. In the control, the net GWP was 13.58 Mg CO2-eq. ha−1, which was contributed by 93% and 7% of seasonal CH4 and N2O fluxes, respectively (Figure 5). The conventional SF (SF0) application decreased the net GWP by 20% over the control. This reduction was mainly attributed to a significant reduction in CH4 flux with 94% coverage of the net GWP. The SF application decreased seasonal N2O flux by 38%, but this reduction did not significantly change the net GWP. The electron acceptor-added SFs (SF2.5 and SF5.0) more effectively decreased net GWP by 37%–40% over the control, but this decrease came from the big suppression of CH4 emissions, with 93%–94% of the contribution to the net GWP. This suggests that in rice paddies, a big reduction in CH4 emissions might contribute to decreasing the impact of global warming.
SF application was very effective in increasing rice productivity and improving rice quality (Table 1). The conventional SF (SF0) increased rice grain productivity by approximately 16% over the control. This was mainly caused by the increase in grains per panicle and 1,000 grain weight. Rice accumulated SiO2 in approximately 10% of its own biomass (Luyckx et al., 2017). This accumulated SiO2 can enhance photosynthetic activity and then increase rice productivity (Cheng, 1982; Cooke and Leishman, 2011; Dorairaj et al., 2017; Galgo et al., 2022). It was known for SF to improve plant resistance against lodging damage and pathogen attack (Ma and Yamaji, 2006; Meharg and Meharg, 2015). In this study, SF application increased grains per panicle and 1,000 grain weight by an average of 3% and 4%, respectively, but there was no change in other yield properties. In addition, the iron rust-added SFs (SF2.5 and SF5.0) increased rice productivity by 22%–25% over the control. Iron, as an essential element, can enhance plant photosynthesis capacity and then increase productivity (Yadavalli et al., 2012). A high concentration of soluble Fe may lead to Fe toxicity, while Fe toxic levels range from 10 to 1,000 mg Fe kg−1 in soils with poor nutrient conditions (Becker and Asch, 2005; Zahra et al., 2021). However, this toxicity was rarely found in rice fields due to Fe precipitation by O2 released from rice roots (Atulba et al., 2015). In addition, in our long-term study for 28 years, we found that the long-term SF application improves soil properties and rice productivity and quality without any toxicity (Lim et al., 2022).
In the rice cropping area, iron slag-based SF has been used as a soil amendment only in Korea and Japan. Two countries have subsidized SF, but the management standard is slightly different between nations. In Korean and Japanese rice paddies, the SF application level is basically determined by multiplying the constant and difference between the optimum and present levels of the available SiO2 concentration in soils (RDA, 2019). The constant was determined using the relationship between rice grain productivity and the available SiO2 concentration in soils after rice harvesting. However, to maximize rice productivity, Korea and Japan recommended 157 and 150–300 mg kg−1 of the available SiO2 concentration as the optimum levels, respectively (NARO, 2009; RDA, 2019). In this study, we analyzed the responses of grain yield index to the available SiO2 concentration in soils using RDA (Song et al., 2007) and our investigation data (Supplementary Tables S1, S2). The rice grain yield index was negatively and quadratically changed by the available SiO2 concentration in soils, with a very high correlation coefficient (R2 = 0.573***) (Figure 7). Rice grain yield increased with the increasing available SiO2 concentration in soils and maximized at 183 mg kg−1 of the available SiO2 concentration, with a 16% yield increase over no-SF application. This implies that the optimum level of available SiO2 should be enhanced from the present level (157 mg SiO2 kg−1) to approximately 183 mg SiO2 kg−1 for maximizing rice grain productivity in the Korean rice paddy.
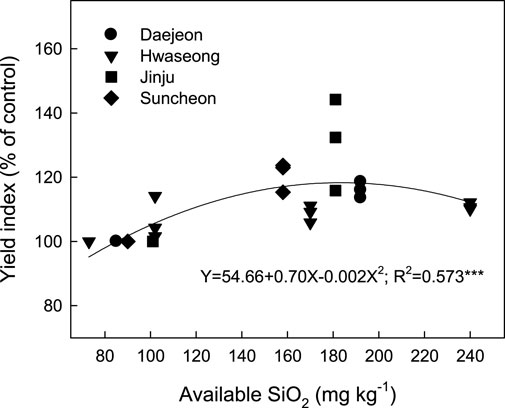
FIGURE 7. Relationship between the grain yield index and available SiO2 concentration in the soil at the harvesting stage.
GHGI, which indicates net GWP per crop productivity (Mosier et al., 2006), was utilized as a standard to compare the net influence of agricultural practices on GHG emission density under the same crop productivity (IPCC, 2023). The conventional SF (SF0) application significantly decreased GHGI by 33% over the control, mainly due to a highly decreased net GWP rather than a yield increase. In comparison, the iron rust-added SFs (SF2.5 and SF5.0) were more effective in decreasing GHGI by 51%–55% over the control. This suggests that GHG emission impacts can be significantly decreased using effective soil amendments like SF while improving soil quality and crop productivity in rice paddies.
5 Conclusion
A prospective soil amendment like SF can significantly decrease GHG emission impacts in rice paddies, while also improving soil quality and rice productivity. SF, which is made from iron slag and contains high amounts of Fe and Mn oxides as electron acceptors, was very effective in suppressing CH4 emissions during the early rice growing season as well as mitigating N2O emissions during the late rice developing stage. The functionality of SF in decreasing GHG emissions and increasing rice productivity could be highly improved by adding small amounts of electron acceptors like iron rust, which is a byproduct of the steel-making process and contains over 99% of Fe2O3. SF was very effective in increasing rice productivity and quality, but the optimum level of available SiO2 concentration could be better enhanced from the present level (154 mg SiO2 kg−1) to approximately 183 mg SiO2 kg−1 in the Korean rice paddy for maximizing rice productivity. In conclusion, the functionalized SF could be a prospective soil amendment to mitigate GHG emission impacts in rice paddy and improve rice productivity.
Data availability statement
The original contributions presented in the study are included in the article/Supplementary Material; further inquiries can be directed to the corresponding author.
Author contributions
SG: data curation, investigation, and writing–original draft. RC: data curation and writing–review and editing. JL: data curation and writing–review and editing. HP: data curation and writing–review and editing. PK: conceptualization, supervision, and writing–review and editing.
Funding
The author(s) declare that financial support was received for the research, authorship, and/or publication of this article. This study received funding from POSCO (Pohang Iron and Steel Co., Ltd.) (No. 2020Z065) and the National Research Foundation of Korea (NRF) through Basis Science Research Programs, which are funded by the Ministry of Science and ICT (NRF-2023R1A2C3004842). Author SJG was supported by scholarships from the BK21+ program of the Ministry of Education and Human Resources Development, Korea. The funders were not involved in the study design, collection, analysis, or interpretation of data, the writing of this article, or the decision to submit it for publication.
Acknowledgments
The authors thankfully acknowledge the Gyeongsang National University for granting necessary permissions and providing the facilities to carry out the work.
Conflict of interest
Author HP was employed by POSCO Co., Ltd.
The remaining authors declare that the research was conducted in the absence of any commercial or financial relationships that could be construed as a potential conflict of interest.
Publisher’s note
All claims expressed in this article are solely those of the authors and do not necessarily represent those of their affiliated organizations, or those of the publisher, the editors, and the reviewers. Any product that may be evaluated in this article, or claim that may be made by its manufacturer, is not guaranteed or endorsed by the publisher.
Supplementary material
The Supplementary Material for this article can be found online at: https://www.frontiersin.org/articles/10.3389/fenvs.2024.1290969/full#supplementary-material
References
Ali, M. A., Lee, C. H., and Kim, P. J. (2008). Effect of silicate fertilizer on reducing methane emission during rice cultivation. Biol. Fertil. Soils 44, 597–604. doi:10.1007/s00374-007-0243-5
Atulba, S. L., Gutierrez, J., Kim, G. W., Kim, S. Y., Khan, M. I., Lee, Y. B., et al. (2015). Evaluation of rice root oxidizing potential using digital image analysis. J. Korean Soc. Appl. Biol. Chem. 58, 463–471. doi:10.1007/s13765-015-0042-x
Becker, M., and Asch, F. (2005). Iron toxicity in rice—conditions and management concepts. J. Plant Nutr. Soil Sci. 168, 558–573. doi:10.1002/jpln.200520504
Bokor, B., Santos, C. S., Kostolani, D., Machado, J., Da Silva, M. N., Carvalho, S. M., et al. (2021). Mitigation of climate change and environmental hazards in plants: potential role of the beneficial metalloid silicon. J. Hazard. Mater. 416, 126193. doi:10.1016/j.jhazmat.2021.126193
Canatoy, R. C., Jeong, S. T., Cho, S. R., Galgo, S. J. C., and Kim, P. J. (2023). Importance of biochar as a key amendment to convert rice paddy into carbon negative. Sci. Total Environ. 873, 162331. doi:10.1016/j.scitotenv.2023.162331
Carlson, H. K., Clark, I. C., Blazewicz, S. J., Iavarone, A. T., and Coates, J. D. (2013). Fe (II) oxidation is an innate capability of nitrate-reducing bacteria that involves abiotic and biotic reactions. J. Bacteriol. 195, 3260–3268. doi:10.1128/jb.00058-13
Chandran, K., Stein, L. Y., Klotz, M. G., and Van Loosdrecht, M. C. (2011). Nitrous oxide production by lithotrophic ammonia-oxidizing bacteria and implications for engineered nitrogen-removal systems. Biochem. Soc. Trans. 39, 1832–1837. doi:10.1042/bst20110717
Cheng, B. (1982). Some significant functions of silicon to higher plants. J. plant Nutr. 5, 1345–1353. doi:10.1080/01904168209363068
Christy, P. M., Gopinath, L., and Divya, D. (2014). A review on anaerobic decomposition and enhancement of biogas production through enzymes and microorganisms. Renew. Sustain. Energy Rev. 34, 167–173. doi:10.1016/j.rser.2014.03.010
Cooke, J., and Leishman, M. R. (2011). Is plant ecology more siliceous than we realise? Trends plant Sci. 16, 61–68. doi:10.1016/j.tplants.2010.10.003
Dorairaj, D., Ismail, M. R., Sinniah, U. R., and Kar Ban, T. (2017). Influence of silicon on growth, yield, and lodging resistance of MR219, a lowland rice of Malaysia. J. Plant Nutr. 40, 1111–1124. doi:10.1080/01904167.2016.1264420
Frenzel, P., Bosse, U., and Janssen, P. H. (1999). Rice roots and methanogenesis in a paddy soil: ferric iron as an alternative electron acceptor in the rooted soil. Soil Biol. Biochem. 31, 421–430. doi:10.1016/s0038-0717(98)00144-8
Galgo, S. J. C., Lim, J. Y., Canatoy, R. C., Ha, J. S., Sohn, K. M., and Kim, P. J. (2022). Improving methane mitigating functionality of blast furnace slag by adding electron acceptor. Sci. Total Environ. 845, 157296. doi:10.1016/j.scitotenv.2022.157296
Gao, C., Sander, M., Agethen, S., and Knorr, K.-H. (2019). Electron accepting capacity of dissolved and particulate organic matter control CO2 and CH4 formation in peat soils. Geochimica Cosmochimica Acta 245, 266–277. doi:10.1016/j.gca.2018.11.004
Heil, J., Vereecken, H., and Brüggemann, N. (2016). A review of chemical reactions of nitrification intermediates and their role in nitrogen cycling and nitrogen trace gas formation in soil. Eur. J. soil Sci. 67, 23–39. doi:10.1111/ejss.12306
Horii, K., Kato, T., Sugahara, K., Tsutsumi, N., and Kitano, Y. (2015). Overview of iron/steel slag application and development of new utilization technologies. Nippon Steel and Sumitomo Tech. Rep. 109, 5–11.
IPCC (2023). “Summary for policymakers,” in Climate change 2023: synthesis report. A report of the intergovernmental Panel on climate change. Contribution of working groups I, II and III to the sixth assessment report of the intergovernmental Panel on climate change core writing team. Editors H. Lee, and J. Romero (Geneva, Switzerland: IPCC).
Keller, J. K., Weisenhorn, P. B., and Megonigal, J. P. (2009). Humic acids as electron acceptors in wetland decomposition. Soil Biol. Biochem. 41, 1518–1522. doi:10.1016/j.soilbio.2009.04.008
Khalil, K., Mary, B., and Renault, P. (2004). Nitrous oxide production by nitrification and denitrification in soil aggregates as affected by O2 concentration. Soil Biol. Biochem. 36, 687–699. doi:10.1016/j.soilbio.2004.01.004
Kool, D. M., Dolfing, J., Wrage, N., and Van Groenigen, J. W. (2011). Nitrifier denitrification as a distinct and significant source of nitrous oxide from soil. Soil Biol. Biochem. 43, 174–178. doi:10.1016/j.soilbio.2010.09.030
Kumaraswamy, S., Ramakrishnan, B., and Sethunathan, N. (2001). Methane production and oxidation in an anoxic rice soil as influenced by inorganic redox species. J. Environ. Qual. 30, 2195–2201. doi:10.2134/jeq2001.2195
Lim, J. Y., Kang, Y. G., Sohn, K. M., Kim, P. J., and Galgo, S. J. C. (2022). Creating new value of blast furnace slag as soil amendment to mitigate methane emission and improve rice cropping environments. Sci. Total Environ. 806, 150961. doi:10.1016/j.scitotenv.2021.150961
Luyckx, M., Hausman, J.-F., Lutts, S., and Guerriero, G. (2017). Impact of silicon in plant biomass production: focus on bast fibres, hypotheses, and perspectives. Plants 6, 37. doi:10.3390/plants6030037
Ma, J. F., Tamai, K., Yamaji, N., Mitani, N., Konishi, S., Katsuhara, M., et al. (2006). A silicon transporter in rice. Nature 440, 688–691. doi:10.1038/nature04590
Ma, J. F., and Yamaji, N. (2006). Silicon uptake and accumulation in higher plants. Trends plant Sci. 11, 392–397. doi:10.1016/j.tplants.2006.06.007
Madsen, E. L. (2011). Microorganisms and their roles in fundamental biogeochemical cycles. Curr. Opin. Biotechnol. 22, 456–464. doi:10.1016/j.copbio.2011.01.008
Makabe-Sasaki, S., Kakuda, K.-I., Sasaki, Y., and Ando, H. (2014). Effects of slag silicate fertilizer on silicon content of rice plants grown in paddy fields on the Shounai Plain, Yamagata, Japan. Soil Sci. Plant Nutr. 60, 708–721. doi:10.1080/00380768.2014.936305
Meharg, C., and Meharg, A. A. (2015). Silicon, the silver bullet for mitigating biotic and abiotic stress, and improving grain quality, in rice? Environ. Exp. Bot. 120, 8–17. doi:10.1016/j.envexpbot.2015.07.001
Mosier, A. R., Halvorson, A. D., Reule, C. A., and Liu, X. J. (2006). Net global warming potential and greenhouse gas intensity in irrigated cropping systems in northeastern Colorado. J. Environ. Qual. 35, 1584–1598. doi:10.2134/jeq2005.0232
NARO (2009). Soil diagnosis guide (in Japanese). Tokyo: Japan Federation of Agricultural Cooperatives, Department of Fertilizer and Pesticides, 36.
Ohta, M., Kobayashi, K., and Kawaguchi, U. (1953). Studies on silicates for fertilizer use. Memoirs Fac. Lib. Arts Educ. 4, 351–358.
Pan, Y., Ni, B.-J., and Yuan, Z. (2013). Modeling electron competition among nitrogen oxides reduction and N2O accumulation in denitrification. Environ. Sci. Technol. 47, 11083–11091. doi:10.1021/es402348n
Park, C.-S. (2001). “Past and future advances in silicon research in the Republic of Korea,” in Studies in plant science (Amsterdam, Netherlands: Elsevier).
Piatak, N. M., Parsons, M. B., and Seal, R. R. (2015). Characteristics and environmental aspects of slag: a review. Appl. Geochem. 57, 236–266. doi:10.1016/j.apgeochem.2014.04.009
RDA (2019). Fertilization standard of crop plants. Gyeonggi-do, South Korea: National Institute of Agricultural Science and Technology Suwon.
Rolston, D. (1986). Gas flux. Methods Soil Analysis Part 1 Phys. Mineralogical Methods 5, 1103–1119.
Schreiber, F., Wunderlin, P., Udert, K. M., and Wells, G. F. (2012). Nitric oxide and nitrous oxide turnover in natural and engineered microbial communities: biological pathways, chemical reactions, and novel technologies. Front. Microbiol. 3, 372. doi:10.3389/fmicb.2012.00372
Shang, Q., Yang, X., Gao, C., Wu, P., Liu, J., Xu, Y., et al. (2011). Net annual global warming potential and greenhouse gas intensity in Chinese double rice-cropping systems: a 3-year field measurement in long-term fertilizer experiments. Glob. Change Biol. 17, 2196–2210. doi:10.1111/j.1365-2486.2010.02374.x
Singh, S., Singh, J., and Kashyap, A. (1999). Methane flux from irrigated rice fields in relation to crop growth and N-fertilization. Soil Biol. Biochem. 31, 1219–1228. doi:10.1016/s0038-0717(99)00027-9
Song, H. J., and Kim, P. J. (2023). Agricultural practices to improve soil carbon sequestration in rice paddy soils. Cambridge: Burleigh Dodds Science Publishing. doi:10.19103/AS.2022.0106.16
Song, Y.-S., Jun, H.-J., Jung, B.-G., Park, W.-K., Lee, K.-S., Kwak, H.-K., et al. (2007). Determination of optimum rate and interval of silicate fertilizer application for rice cultivation in Korea. Korean J. Soil Sci. Fertilizer 40, 354–363.
Wang, M., Hu, R., Ruser, R., Schmidt, C., and Kappler, A. (2019). Role of chemodenitrification for N2O emissions from nitrate reduction in rice paddy soils. ACS Earth Space Chem. 4, 122–132. doi:10.1021/acsearthspacechem.9b00296
Wang, M., Hu, R., Zhao, J., Kuzyakov, Y., and Liu, S. (2016). Iron oxidation affects nitrous oxide emissions via donating electrons to denitrification in paddy soils. Geoderma 271, 173–180. doi:10.1016/j.geoderma.2016.02.022
Wang, R., Xu, S.-Y., Zhang, M., Ghulam, A., Dai, C.-L., and Zheng, P. (2020). Iron as electron donor for denitrification: the efficiency, toxicity and mechanism. Ecotoxicol. Environ. Saf. 194, 110343. doi:10.1016/j.ecoenv.2020.110343
Yadavalli, V., Neelam, S., Rao, A. S., Reddy, A. R., and Subramanyam, R. (2012). Differential degradation of photosystem I subunits under iron deficiency in rice. J. plant physiology 169, 753–759. doi:10.1016/j.jplph.2012.02.008
Yoshida, S., Onishi, Y., and Kitagishi, K. (1959). The chemical nature of silicon in rice plant. Soil Sci. Plant Nutr. 5, 23–27. doi:10.1080/00380768.1959.10430890
Young, M. N., Boltz, J., Rittmann, B. E., Al-Omari, A., Jimenez, J. A., Takacs, I., et al. (2022). Thermodynamic analysis of intermediary metabolic steps and nitrous oxide production by ammonium-oxidizing bacteria. Environ. Sci. Technol. 56, 12532–12541. doi:10.1021/acs.est.1c08498
Zahra, N., Hafeez, M. B., Shaukat, K., Wahid, A., and Hasanuzzaman, M. (2021). Fe toxicity in plants: impacts and remediation. Physiol. Plant. 173, 201–222. doi:10.1111/ppl.13361
Keywords: silicate fertilizer, methane, nitrous oxide, electron transfer, iron
Citation: Galgo SJC, Canatoy RC, Lim JY, Park HC and Kim PJ (2024) A potential of iron slag-based soil amendment as a suppressor of greenhouse gas (CH4 and N2O) emissions in rice paddy. Front. Environ. Sci. 12:1290969. doi: 10.3389/fenvs.2024.1290969
Received: 08 September 2023; Accepted: 16 February 2024;
Published: 05 March 2024.
Edited by:
Mehmet Senbayram, Harran University, TürkiyeReviewed by:
Zhijun Wei, Chinese Academy of Sciences (CAS), ChinaRoland Bol, Helmholtz Association of German Research Centres (HZ), Germany
Copyright © 2024 Galgo, Canatoy, Lim, Park and Kim. This is an open-access article distributed under the terms of the Creative Commons Attribution License (CC BY). The use, distribution or reproduction in other forums is permitted, provided the original author(s) and the copyright owner(s) are credited and that the original publication in this journal is cited, in accordance with accepted academic practice. No use, distribution or reproduction is permitted which does not comply with these terms.
*Correspondence: Pil Joo Kim, cGpraW1AZ251LmFjLmty