- 1Australian Rivers Institute, Griffith University, Nathan, QLD, Australia
- 2Department of Forest Ecology and Management, Swedish University of Agricultural Sciences, Umeå, Sweden
Wetlands are increasingly valuable under climate change in terms of their ecological functions, ecosystem services, and biodiversity. Simultaneously, wetlands are hotspots for anthropogenic activity due to their high soil fertility and water supply, and have been subject to significant modification, degradation, and staggering losses. With climate change having increasing impacts on ecosystems globally, the need for wetland restoration is rapidly growing. Natural regeneration, whereby vegetation is allowed to regrow via propagules already present within the landscape, provides a cost-effective and large-scale approach to restoration for many, but not, all wetlands. This paper emphasises the importance of natural regeneration of wetland ecosystems as an effective restoration approach under climate change. We discuss drivers and barriers of natural regeneration of wetlands under climate change along with implications for management approaches. Drivers of wetland natural regeneration are depicted along with their interactions, displaying a range of abiotic and biotic factors that influence ecosystem change. Key adaption approaches to maintain and promote natural regeneration of wetlands under climate change include integrated land and water management, protecting and promoting key relevant biotic and abiotic processes within landscapes, and reconsidering current exotic species management strategies. Most importantly, however, natural regeneration should be recognised as an important and viable restoration approach under climate change in order to meet restoration demand and promote landscape resilience to changing conditions.
1 Introduction
Few wetland ecosystems in the world remain untouched by human activity (Foley et al., 2005). Anthropogenic impacts on wetlands are being further exacerbated by climate change (Fluet-Chouinard et al., 2023). Restoration and revegetation of wetlands is increasingly vital for creating resilient landscapes better able to respond to a changing climate without dramatic loss of biodiversity or ecosystem function (Erwin et al., 2009; Bastin et al., 2019). Wetland vegetation, both woody and non-woody, has disproportionately high value, supporting high levels of biodiversity, providing critical ecosystem functions to both terrestrial and aquatic organisms, and delivering a range of ecosystems services vital for human livelihoods (Capon et al., 2016). Key ecosystem services provided by wetlands include carbon sequestration, maintenance and creation of clean water and soil fertility, and regulation of water and climate (Dybala et a., 2019). Consequently, there is an urgent need to better understand and implement effective restoration management approaches for wetlands under climate change (Benayas et al., 2009; Tomscha et al., 2021).
Restoring wetlands can be achieved through a range of approaches spanning active, assisted and passive methods (Di Sacco et al., 2021). In any situation, the best restoration practice, however, will be unique to the set of circumstances at a local and landscape scale (Yang et al., 2020). Consequently, it is critical that we better understand the efficacy and drivers of a range of restoration approaches to ensure efficient use of limited resources at this critical time (Prach & Hobbs, 2008). We define wetlands in line with The Ramsar Convention as “areas of marsh, fen, peatland or water, whether natural or artificial, permanent or temporary, with water that is static or flowing, fresh, brackish or salt, including areas of marine water the depth of which at low tide does not exceed 6 m”. Although, within this paper we are also including floodplain and riparian areas but excluding marine wetlands. Overall, this paper seeks to emphasise the importance of natural regeneration of wetland ecosystems as an efficient and effective restoration approach under climate change. In this paper, we contrast different restoration approaches for wetland vegetation and highlighting situation where natural regeneration is most appropriate under climate change. We identify the key drivers and barriers of wetland regeneration, presenting a conceptual model to provide a better understand of ways to best promote natural regeneration. Finally, we explore why natural regeneration of wetlands is critical under climate change and how it can be best supported.
2 Wetland restoration approaches
Ecological restoration, revegetation, re-wilding, and regeneration are terms used to describe the recovery of an ecosystem following anthropogenic or natural disturbance. Traditionally, two broadly contrasting forms of restoration approaches are recognised: active and passive, the latter also often referred to as natural regeneration (Holl & Aide, 2011). Active restoration involves significant human intervention such as planting tube stock of seedlings or seeds to facilitate regeneration (Perrow & Davy, 2002). In contrast, natural regeneration requires minimal intervention with vegetation permitted to regrow via available plant propagules once a disturbance has ceased (Chazdon and Guariguata, 2016). Intermediate to active and passive regeneration, assisted natural regeneration requires the manipulation of some abiotic and biotic factors to encourage natural regeneration (Mälson et al., 2008). To determine which restoration approach is likely to be possible and effective in each situation depends at least partially on the level of wetland degradation experienced (Jackson & Hobbs, 2009). Natural regeneration, for instance, relies on the availability of suitable plant propagules and establishment sites existing within the landscape, as well as adequate regeneration conditions, including sufficient time (Prach et al., 2014). Where landscapes have been subjected to long-term intense agricultural pressure, significant erosion or are otherwise propagule limited, active restoration may be necessary due to a loss of suitable conditions (Holl & Aide, 2011).
Depending on the level of intervention required for active restoration, it can be associated with high initial costs and ongoing maintenance (Moreno-Mateos et al., 2015). The high costs are due to the acquisition of native seeds and tube stock and the intensive labour required to plant and maintain them, often restricting active restoration efforts to relatively small scales (Summers et al., 2015). Active restoration may be implemented to speed up regeneration, which otherwise would take long timeframes, although this has not always been found to be successful (Chimner et al., 2017; Jones et al., 2018). Active restoration attempts can also have a high risk of failure where abiotic conditions are unsuitable for the successful establishment of vegetation, these pressures are likely to increase under climate change (Clark et al., 2007). With more extreme weather events, higher temperatures and changed rainfall patterns, “climate-adjusted” provenancing strategies will be required for sourcing seed for restoration projects (Prober et al., 2015). Extra consideration should also be made when selecting species for restoration, as species historically native to the area, may not be adapted to the conditions which have arisen due to climate change (Harris et al., 2006). The increase investment required needed to maintain planted tube stock via active restoration and species selection may contribute to increase in failure of restoration attempts from active restoration.
In contrast to active restoration, passive restoration or natural regeneration requires minimal human intervention and is therefore typically associated with lower costs and can be applied over a larger scale (Chazdon and Guariguata, 2016). However, disturbances such as livestock grazing, fire, and cropping must often be controlled or stopped before natural regeneration can occur successfully (Prach & Hobbs, 2008), adding to the costs and potentially reducing the efficacy of natural regeneration approaches (Zahawi et al., 2014). In wetlands, such disturbances may also include alterations to hydrological regimes for water resources management and/or flood mitigation, as well as extreme flood events driven by climate change (Devkota et al., 2015; Parra et al., 2021). Natural regeneration is occurring within wetlands globally, although due to wetlands being poorly mapped due to inconsistences in wetland definition and limitation in modelling systems (Tootchi et al., 2019; Glanville et al., 2023), the extant and success of it is unknown in many areas.
In multiple meta-analyses in forest systems, natural regeneration has been found to have better restoration outcomes compared with active restoration (e.g., Crouzeilles et al., 2017; Huang et al., 2019; Shimamoto et al., 2018). Crouzeilles et al., 2017, found in their meta-analysis of 133 studies that natural regeneration surpassed active restoration attempts in tropical forests for all three biodiversity groups (plants, birds, and invertebrates) and five measures of vegetation structure. Another study done by Huang et al., 2019 conducted a meta-analysis on ecological restoration of terrestrial ecosystem collated data from 103 published studies and found that passive restoration performed better than active approaches for the recovery of biodiversity. Shimamoto et al., 2018 completed a meta-analysis from data obtained in 69 studies on the restoration of ecosystem services on tropical forests, found that natural regeneration was the most effective strategy. While none of these meta-analyses’ focused specifically on wetlands, they did demonstrate natural regeneration’s ability to restore vegetation successfully and, in some circumstances, better then active approaches. Meli et al., 2014, found in their meta-analysis on how restoration enhanced wetland biodiversity and ecosystems servicers, that only 3 out of 70 papers assessed used natural regeneration as their restoration approach. This highlights how underrepresented natural regeneration is for wetland restoration, despite its effectiveness clearly shown in forested ecosystems.
3 Drivers and barriers of wetland natural regeneration
In order to promote natural regeneration within wetlands under climate change, it is vital we understand barriers and drivers of vegetation regeneration (Figure 1) (Piana et al., 2020). Understanding key patterns and drivers of natural regeneration can help better prioritise wetlands which would most benefit from natural regeneration (Widis et al., 2015; Zivec et al., 2021). Key mechanisms driving natural regeneration can be classified into two broad categories: biotic and abiotic factors. Overall, for natural regeneration to occur, both adequate propagules, e.g., seeds (biotic) and suitable conditions for their successful germination and establishment (abiotic) must be present (Prach & Walker, 2011).
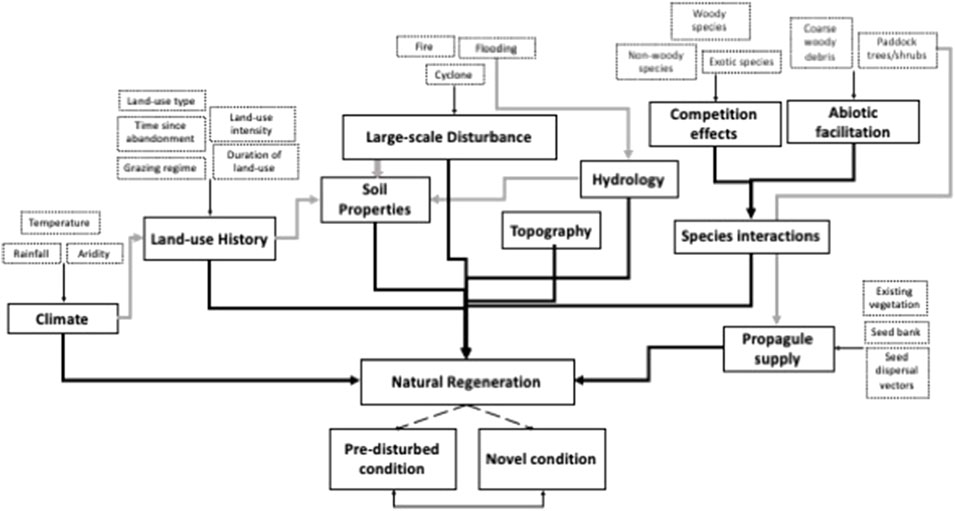
FIGURE 1. Conceptual model depicting drivers of natural regeneration within wetland ecosystems. General drivers of natural regeneration are represented in solid black boxes and characteristics of general drivers are represented in dotted line boxes. Solid black lines represent connections to naturally regenerating vegetation, while grey lines represented interconnected driver.
In wetland ecosystems, hydrology is a key driver of vegetation regeneration (Todd et al., 2010). By definition wetlands are habitats “that are inundated or saturated by water at a frequency and for a duration sufficient to support a prevalence of vegetation typically adapted for life in saturated soil conditions” (Mitsch and Gosselink, 2015). Therefore, wetland vegetation is more likely to have traits that are triggered by different hydrological cues (Rosbakh et al., 2020). The duration, frequency and timing of different hydrological events
shape vegetation characteristics within wetlands, particularly in floodplain and riparian ecosystems (Capon, 2005). Landscape topography underpins hydrological processes and will also drive patterns of natural regeneration within wetlands (Branton & Robinson, 2020). Wetlands within areas which are more likely to have overbank flow, water pooling or tidal influence will impact what vegetation species are able to regenerate (Grieger et al., 2019). Meso-climatic variation drive natural regeneration due to factors such as soil moisture availability and hydrology, which are strongly link to wetland vegetation. Regeneration of areas that are more benign (e.g., with balanced rainfall and potential evapotranspiration, fertile soils, etc.) is less restricted by abiotic factors and, therefore, natural regeneration often occurs in such places at faster rates (Cramer et al., 2008).
Propagule supply is a fundamental mechanism underpinning the successful occurrence of natural regeneration (Walker & Del Maral, 2003). Propagules, including seeds, tubers, plant fragments, etc., can be stored within existing vegetation, seed banks and transported throughout the landscape (Zivec et al., 2023). Hydrochory is a key process linked to propagule transportation and is responsible for large-scale patterns of species distribution within wetlands (Nilsson et al., 2010). Wetlands, particularly riparian zones, are often a hotspot for exotic species invasion due to the increase in disturbance from flooding and the transportation of seeds via hydrochory (Catford & Jansson, 2014). This risk is even more elevated in wetlands which have altered flow regimes (Catford et al., 2011). This interspecies competition, particularly exotic species, can affect the likelihood of regeneration occurring due to competition for light, nutrients, and resources, which can reduce wetland regeneration (Rehel & Olden, 2008). The presence of adequate existing vegetation as a seed source, however, is vital for regeneration (Houlahan et al., 2006). Existing trees, shrubs, and deadwood within disturbed areas are particularly important for abiotic facilitation for germination and establishment, providing microsites for regeneration (Blood & Titus, 2010).
In wetlands subjected to agricultural activity, natural regeneration may be impacted by legacies from previous land use. Wetlands are disproportionately impacted by grazing and cropping due to the close proximity to water and increased nutrients (Wiegman et al., 2022). Land-use history, including the type, frequency, duration, and intensity of previous agriculture, influences the vegetation assemblage that regrows via natural regeneration (Cramer et al., 2008). Changes in soil properties through the depletion or addition of nutrients and alteration of soil pH or salinity resulting from agricultural practices, for instance, can have a significant effect on the abiotic conditions needed for regeneration (Arbelo et al., 2006; Breen et al., 2015). Additionally, wetlands throughout the world have been drained of water by ditches to increase arable land, this has resulted in widespread wetland loss (Fluet-Chouinard et al., 2023) and the creation of new human-made wetlands (Kuglerova et al., 2017).
Climate change will influence natural regeneration through the alteration of abiotic and biotic conditions that drives vegetation to regrow (Pugnaire et al., 2019). First, climatic factors are strongly linked to abiotic factors that are vital for breaking seed dormancy and germination (Klupczynka & Pawlowski, 2021). Under climate change, fire regimes, rainfall patterns and temperature are all likely to shift, therefore altering which species may be able to successfully emerge from seed banks (Ooi, 2012; Kiss et al., 2018). Changes to soil temperature and rainfall patterns may impact seedbanks persistence and therefore a depletion of biotic components needed for vegetation regeneration (Ooi et al., 2012). Weedy, exotic and generalist species, that are adapted to altered or a wide range of conditions may then have the regenerative advantage under climate change (Hellmann et al., 2008). Therefore, there may be an increased risk of exotic plant invasions or dominance of generalist species in the future, with these species being “ecological winners” under climate change (Van der Putten et al., 2010). Such eventualities may cause shifts in species compositions and structure, leading to changes in wetlands character and condition, especially in naturally regenerated areas (Capon et al., 2021). Consequently, it’s likely we will increasingly see vegetation communities occurring in novel states, and trajectories, with altered species composition and structure (Capon & Pettit, 2018).
4 Discussion
Naturally regenerated wetlands can provide complimentary habitat to remnant wetlands for a low cost and with minimal intervention, offering the potential for much larger areas of wetlands to be rehabilitated then via active methods (De Steven et al., 2010; Holl & Aide, 2011). With respect to climate change adaptation, increasing the amount of wetland vegetation is vital for improving and creating new thermal refuges for biodiversity as the climate and weather events become more extreme (Nelson et al., 2009; Mandelik et al., 2012). Wetlands provide habitat connectivity throughout the landscape and between terrestrial and aquatic areas (Desta et al., 2012). Species will need to disperse in response to changing conditions and increased landscape vegetation achieved from natural regeneration will allow for safer and easier movements (Doerr et al., 2011).
Natural regeneration is of increasing importance under climate change due to demand for restoration, its resilience to altered climatic conditions and capacity to support ecosystem services (Jones et al., 2018). As vegetation regrows via the seed already within the landscape, those species will have local genetic adaptions and high functional diversity when compared to active restoration (Chazdon and Guariguatat, 2016). As nature selects which species will regenerate under natural regeneration, vegetation is likely to be more heterogeneous (Zhang et al., 2021). This combination of genetic and landscape heterogeneity can be expected to increase landscape resilience to future conditions under climate change (Moore & Schindler, 2022). Naturally regenerated vegetation may also alter local climatic conditions by increasing shade, or altering soil moisture and hydrology (McLaughlin et al., 2017). Newly regenerating vegetation areas may act as climate refuges for biodiversity and, in turn, support more vegetation regeneration by providing a seed source and favourable abiotic conditions for further plant establishment (Selwood and Zimmer, 2020; Pradhan et al., 2023).
There is a range of adaption approaches that can be implemented to promote natural regeneration of wetlands under climate change (Simenstad et al., 2006; Capon & Pettit., 2018). First, it is important to minimise landscape stresses such as altered flow regimes (McIver and Starr, 2001). The reintroduction of ecosystem engineers such as beavers, can be an important strategy in restoring natural flow regimes needed for successful establishment of some vegetation species (Orr et al., 2020). Coupling revegetation projects with environmental flows is a promising avenue for research that may lead to improved restoration outcomes in wetlands under climate change (Schlatter et al., 2017). Environmental flows are the quantity, timing, and quality of water flows that sustain freshwater ecosystems (Bunn and Arthington, 2002). Environmental flows may be implemented within systems with altered flow regimes to improve vegetation resilience and promote natural regeneration (Sims and Colloff, 2012). At the same time, a better understanding of how to implement environmental flows and grazing management may assist in reducing woody thickening within naturally regenerated wetlands (Good et al., 2012; Saintilan and Rogers, 2015).
It is vital to promote key abiotic and biotic processes needed for natural regeneration within wetlands on a landscape and local scale (Kentula, 2000). Within wetlands, hydrology is a major driver of biotic processes (i.e., hydrochory) and abiotic conditions (i.e., germination and establishment) (Bejarano et al., 2018). Restoring natural flow regimes can assist in promoting large scale natural regeneration (Chazdon et al., 2021). On a smaller scale, microsites from existing structural features provide shading, lower wind exposure, and reduce grass or ground cover competition, facilitating natural regeneration (Kikvidze & Callaway, 2009). Minimising woody weed removal in some circumstances may be beneficial in creating these microsites and thermal refuges under climate change (Pollen-Bankhead et al., 2009; Schlaepfer et al., 2011). The presence of existing vegetation throughout the landscape are important seed sources for wetlands, particularly higher up in the catchment to enable dispersal via hydrochory downstream (Houlahan et al., 2006; Kuglerová et al., 2019). Therefore, under climate change, it should be the highest management priority to protect any existing vegetation and restore hydrological regimes to promote local and landscape scale natural regeneration.
In conclusion, natural regeneration is critical for rehabilitating wetlands under climate change, due to its low-cost, large-scale application and resilience to changed conditions (Chazdon, 2017). Natural regeneration is spatially and temporally variable, due to it being shaped by abiotic and biotic drivers and constrains (Zivec et al., 2021; Zivec et al., 2023). This creates heterogeneous vegetation within the landscape which adds to catchment resilience due to its patch diversity, distribution, and climate adapted species (Prach & del Moral., 2015). Although, when regeneration barriers become too high, for example, from intensive agriculture or repeat disturbances, assisted or active restoration may need to occur (Zahawi et al., 2014). Natural regeneration of poses a huge opportunity to rehabilitate large areas of wetlands and should be a key restoration strategy under climate change (Whisenant, 1999; Capon et al., 2013; Chazdon and Guariguatat, 2016; Capon et al., 2021).
Data availability statement
The original contributions presented in the study are included in the article/supplementary material, further inquiries can be directed to the corresponding author.
Author contributions
PZ was involved in the writing, conceptualising, and structuring of the paper. While FS and SC were involved with the conceptualisation, editing, and theoretical application. All authors contributed to the article and approved the submitted version.
Funding
This research was finically supported by Griffith University.
Acknowledgments
I would like to thank the reviewers for their feedback which assisted in the improvement of this manuscript.
Conflict of interest
The authors declare that the research was conducted in the absence of any commercial or financial relationships that could be construed as a potential conflict of interest.
Publisher’s note
All claims expressed in this article are solely those of the authors and do not necessarily represent those of their affiliated organizations, or those of the publisher, the editors and the reviewers. Any product that may be evaluated in this article, or claim that may be made by its manufacturer, is not guaranteed or endorsed by the publisher.
References
Arbelo, C. D., Rodríguez-Rodríguez, A., Guerra, J. A., Mora, J. L., Notario, J. S., and Fuentes, F. (2006). Soil degradation processes and plant colonization in abandoned terraced fields overlying pumice tuffs. Land Degrad. Dev. 17 (6), 571–588. doi:10.1002/ldr.735
Bastin, J., Finegold, Y., Garcia, C., Mollicone, D., Rezende, M., Routh, D., et al. (2019). The global tree restoration potential. Science 365 (6448), 76–79. doi:10.1126/science.aax0848
Bejarano, M. D., Jansson, R., and Nilsson, C. (2018). The effects of hydropeaking on riverine plants: A review. Biol. Rev. 93 (1), 658–673. doi:10.1111/brv.12362
Benayas, J. R., Newton, A. C., Diaz, A., and Bullock, J. M. (2009). Enhancement of biodiversity and ecosystem services by ecological restoration: A meta-analysis. Science 325 (5944), 1121–1124. doi:10.1126/science.1172460
Blood, L. E., and Titus, J. H. (2010). Microsite effects on forest regeneration in a bottomland swamp in Western New York1. J. Torrey Botanical Soc. 137 (1), 88–102. doi:10.3159/08-ra-062.1
Branton, C., and Robinson, D. T. (2020). Quantifying topographic characteristics of wetlandscapes. Wetlands 40 (2), 433–449. doi:10.1007/s13157-019-01187-2
Breen, D. B., Beauchamp, V. B., Koontz, S. M., and Roberts, R. P. (2015). The influence of agricultural abandonment and the abiotic environment on the vegetation communities of a suburban deciduous forest. Castanea 80 (2), 103–121. doi:10.2179/15-055
Bunn, S. E., and Arthington, A. H. (2002). Basic principles and ecological consequences of altered flow regimes for aquatic biodiversity. Environ. Manage. 30, 492–507.
Capon, S., James, C., and Reid, M. (2016). in Vegetation of Australian riverine landscapes: Biology, ecology and management (CSIRO PUBLISHING: Clayton, Australia).
Capon, S. J. (2005). Flood variability and spatial variation in plant community composition and structure on a large arid floodplain. J. Arid Environ. 60 (2), 283–302. doi:10.1016/j.jaridenv.2004.04.004
Capon, S. J., Chambers, L. E., Mac Nally, R., Naiman, R. J., Davies, P., Marshall, N., et al. (2013). Riparian ecosystems in the 21st century: hotspots for climate change adaptation?. Ecosystems 16, 359–381.
Capon, S. J., and Pettit, N. E. (2018). Turquoise is the new green: Restoring and enhancing riparian function in the Anthropocene. Ecol. Manag. Restor. 19, 44–53. doi:10.1111/emr.12326
Capon, S. J., Stewart-Koster, B., and Bunn, S. E. (2021). Future of freshwater ecosystems in a 1.5 C warmer world. Front. Environ. Sci. 9, 596. doi:10.3389/fenvs.2021.784642
Catford, J. A., Downes, B. J., Gippel, C. J., and Vesk, P. A. (2011). Flow regulation reduces native plant cover and facilitates exotic invasion in riparian wetlands. J. Appl. Ecol. 48 (2), 432–442. doi:10.1111/j.1365-2664.2010.01945.x
Catford, J. A., and Jansson, R. (2014). Drowned, buried and carried away: Effects of plant traits on the distribution of native and alien species in riparian ecosystems. New Phytol. 204 (1), 19–36. doi:10.1111/nph.12951
Chazdon, R. L., Falk, D. A., Banin, L. F., Wagner, M., Wilson, S., Grabowski, R. C., et al. (2021). The intervention continuum in restoration ecology: Rethinking the active–passive dichotomy. Restor. Ecol., e13535. doi:10.1111/rec.13535
Chazdon, R. L., and Guariguata, M. R. (2016). Natural regeneration as a tool for large-scale forest restoration in the tropics: Prospects and challenges. Biotropica 48 (6), 716–730. doi:10.1111/btp.12381
Chazdon, R. L. (2017). Landscape restoration, natural regeneration, and the forests of the future. Ann. Mo. Botanical Gard. 102 (2), 251–257. doi:10.3417/2016035
Chimner, R. A., Cooper, D. J., Wurster, F. C., and Rochefort, L. (2017). An overview of peatland restoration in north America: Where are we after 25 years? Restor. Ecol. 25 (2), 283–292. doi:10.1111/rec.12434
Clark, C. J., Poulsen, J. R., Levey, D. J., and Osenberg, C. W. (2007). Are plant populations seed limited? A critique and meta-analysis of seed addition experiments. Am. Nat. 170 (1), 128–142. doi:10.1086/518565
Cramer, V. A., Hobbs, R. J., and Standish, R. J. (2008). What's new about old fields? Land abandonment and ecosystem assembly. Trends Ecol. Evol. 23 (2), 104–112. doi:10.1016/j.tree.2007.10.005
Crouzeilles, R., Ferreira, M. S., Chazdon, R. L., Lindenmayer, D. B., Sansevero, J. B., Monteiro, L., et al. (2017). Ecological restoration success is higher for natural regeneration than for active restoration in tropical forests. Sci. Adv. 3 (11), 1701345. doi:10.1126/sciadv.1701345
De Steven, D., Sharitz, R. R., and Barton, C. D. (2010). Ecological outcomes and evaluation of success in passively restored southeastern depressional wetlands. Wetlands 30, 1129–1140. doi:10.1007/s13157-010-0100-4
Desta, H., Lemma, B., and Fetene, A. (2012). Aspects of climate change and its associated impacts on wetland ecosystem functions: A review. J. Am. Sci. 8 (10), 582–596.
Devkota, L. P., and Gyawali, D. R. (2015). Impacts of climate change on hydrological regime and water resources management of the Koshi River Basin, Nepal. J. Hydrology Regional Stud. 4, 502–515. doi:10.1016/j.ejrh.2015.06.023
Di Sacco, A., Hardwick, K. A., Blakesley, D., Brancalion, P. H., Breman, E., Cecilio Rebola, L., et al. (2021). Ten golden rules for reforestation to optimize carbon sequestration, biodiversity recovery and livelihood benefits. Glob. Change Biol. 27 (7), 1328–1348. doi:10.1111/gcb.15498
Doerr, V. A., Barrett, T., and Doerr, E. D. (2011). Connectivity, dispersal behaviour and conservation under climate change: A response to hodgson et al. J. Appl. Ecol. 48 (1), 143–147. doi:10.1111/j.1365-2664.2010.01899.x
Dybala, K. E., Matzek, V., Gardali, T., and Seavy, N. E. (2019). Carbon sequestration in riparian forests: A global synthesis and meta-analysis. Glob. Change Biol. 25 (1), 57–67. doi:10.1111/gcb.14475
Erwin, K. L. (2009). Wetlands and global climate change: The role of wetland restoration in a changing world. Wetl. Ecol. Manag. 17 (1), 71–84. doi:10.1007/s11273-008-9119-1
Fluet-Chouinard, E., Stocker, B. D., Zhang, Z., Malhotra, A., Melton, J. R., Poulter, B., et al. (2023). Extensive global wetland loss over the past three centuries. Nature 614 (7947), 281–286. doi:10.1038/s41586-022-05572-6
Foley, J. A., DeFries, R., Asner, G. P., Barford, C., Bonan, G., Carpenter, S. R., et al. (2005). Global consequences of land use. Science 309 (5734), 570–574. doi:10.1126/science.1111772
Glanville, K., Perry, J., Ryan, T., Ronan, M., and Zivec, P. (2023). Applying a versatile, comprehensive, attribute-based waterhole classification scheme to ecosystem-based management challenges. Wildl. Res. doi:10.1071/wr22027
Good, M. K., Price, J. N., Clarke, P. J., and Reid, N. (2012). Dense regeneration of floodplain Eucalyptus coolabah: Invasive scrub or passive restoration of an endangered woodland community? Rangel. J. 34 (2), 219–230. doi:10.1071/rj12008
Grieger, R., Capon, S., and Hadwen, W. (2019). Resilience of coastal freshwater wetland vegetation of subtropical Australia to rising sea levels and altered hydrology. Reg. Environ. Change 19, 279–292. doi:10.1007/s10113-018-1399-2
Harris, J. A., Hobbs, R. J., Higgs, E., and Aronson, J. (2006). Ecological restoration and global climate change. Restor. Ecol. 14 (2), 170–176.
Hellmann, J. J., Byers, J. E., Bierwagen, B. G., and Dukes, J. S. (2008). Five potential consequences of climate change for invasive species. Conserv. Biol. 22 (3), 534–543. doi:10.1111/j.1523-1739.2008.00951.x
Holl, K. D., and Aide, T. M. (2011). When and where to actively restore ecosystems? For. Ecol. Manag. 261 (10), 1558–1563. doi:10.1016/j.foreco.2010.07.004
Houlahan, J. E., Keddy, P. A., Makkay, K., and Findlay, C. S. (2006). The effects of adjacent land use on wetland species richness and community composition. Wetlands 26 (1), 79–96. doi:10.1672/0277-5212(2006)26[79:teoalu]2.0.co;2
Huang, C., Zhou, Z., Peng, C., Teng, M., and Wang, P. (2019). How is biodiversity changing in response to ecological restoration in terrestrial ecosystems? A meta-analysis in China. Sci. Total Environ. 650, 1–9. doi:10.1016/j.scitotenv.2018.08.320
Jackson, S. T., and Hobbs, R. J. (2009). Ecological restoration in the light of ecological history. Science 325 (5940), 567–569. doi:10.1126/science.1172977
Jones, H. P., Jones, P. C., Barbier, E. B., Blackburn, R. C., Rey Benayas, J. M., Holl, K. D., et al. (2018). Restoration and repair of Earth's damaged ecosystems. Proc. R. Soc. B Biol. Sci. 285 (1873), 20172577. doi:10.1098/rspb.2017.2577
Kentula, M. E. (2000). Perspectives on setting success criteria for wetland restoration. Ecol. Eng. 15 (3-4), 199–209. doi:10.1016/s0925-8574(00)00076-8
Kikvidze, Z., and Callaway, R. M. (2009). Ecological facilitation may drive major evolutionary transitions. BioScience 59 (5), 399–404. doi:10.1525/bio.2009.59.5.7
Kiss, R., Deák, B., Török, P., Tóthmérész, B., and Valkó, O. (2018). Grassland seed bank and community resilience in a changing climate. Restor. Ecol. 26, S141–S150. doi:10.1111/rec.12694
Klupczyńska, E. A., and Pawłowski, T. A. (2021). Regulation of seed dormancy and germination mechanisms in a changing environment. Int. J. Mol. Sci. 22 (3), 1357. doi:10.3390/ijms22031357
Kuglerová, L., Hasselquist, E. M., Richardson, J. S., Sponseller, R. A., Kreutzweiser, D. P., and Laudon, H. (2017). Management perspectives on Aqua incognita: Connectivity and cumulative effects of small natural and artificial streams in boreal forests. Hydrol. Process. 31 (23), 4238–4244. doi:10.1002/hyp.11281
Kuglerová, L., Kielstra, B. W., Moore, R. D., and Richardson, J. S. (2019). Importance of scale, land-use, and stream network properties for riparian plant communities along an urban gradient. Freshw. Biol. 64 (3), 587–600. doi:10.1111/fwb.13244
Mälson, K., Backéus, I., and Rydin, H. (2008). Long-term effects of drainage and initial effects of hydrological restoration on rich fen vegetation. Appl. Veg. Sci. 11 (1), 99–106. doi:10.1111/j.1654-109x.2008.tb00208.x
Mandelik, Y., Winfree, R., Neeson, T., and Kremen, C. (2012). Complementary habitat use by wild bees in agro-natural landscapes. Ecol. Appl. 22 (5), 1535–1546. doi:10.1890/11-1299.1
McIver, J., and Starr, L. (2001). Restoration of degraded lands in the interior Columbia River basin: passive vs. active approaches. For. Ecol. Manag. 153 (1–3), 15–28.
McLaughlin, B. C., Ackerly, D. D., Klos, P. Z., Natali, J., Dawson, T. E., and Thompson, S. E. (2017). Hydrologic refugia, plants, and climate change. Glob. change Biol. 23 (8), 2941–2961. doi:10.1111/gcb.13629
Meli, P., Rey Benayas, J. M., Balvanera, P., and Martínez Ramos, M. (2014). Restoration enhances wetland biodiversity and ecosystem service supply, but results are context-dependent: A meta-analysis. PloS one 9 (4), e93507. doi:10.1371/journal.pone.0093507
Moore, J. W., and Schindler, D. E. (2022). Getting ahead of climate change for ecological adaptation and resilience. Science 376 (6600), 1421–1426. doi:10.1126/science.abo3608
Moreno-Mateos, D., Meli, P., Vara-Rodríguez, M. I., and Aronson, J. (2015). Ecosystem response to interventions: Lessons from restored and created wetland ecosystems. J. Appl. Ecol. 52 (6), 1528–1537. doi:10.1111/1365-2664.12518
Nelson, K. C., Palmer, M. A., Pizzuto, J. E., Moglen, G. E., Angermeier, P. L., Hilderbrand, R. H., et al. (2009). Forecasting the combined effects of urbanization and climate change on stream ecosystems: From impacts to management options. J. Appl. Ecol. 46 (1), 154–163. doi:10.1111/j.1365-2664.2008.01599.x
Nilsson, C., Brown, R. L., Jansson, R., and Merritt, D. M. (2010). The role of hydrochory in structuring riparian and wetland vegetation. Biol. Rev. 85 (4), 837–858. doi:10.1111/j.1469-185x.2010.00129.x
Ooi, M. K., Auld, T. D., and Denham, A. J. (2012). Projected soil temperature increase and seed dormancy response along an altitudinal gradient: Implications for seed bank persistence under climate change. Plant Soil 353, 289–303. doi:10.1007/s11104-011-1032-3
Ooi, M. K. (2012). Seed bank persistence and climate change. Seed Sci. Res. 22 (S1), S53–S60. doi:10.1017/s0960258511000407
Orr, M. R., Weber, N. P., Noone, W. N., Mooney, M. G., Oakes, T. M., and Broughton, H. M. (2020). Short-term stream and riparian responses to beaver dam analogs on a low-gradient channel lacking woody riparian vegetation. Northwest Sci. 93 (3–4), 171–184.
Parra, G., Guerrero, F., Armengol, J., Brendonck, L., Brucet, S., Finlayson, C. M., et al. (2021). The future of temporary wetlands in drylands under global change. Inland Waters 11 (4), 445–456. doi:10.1080/20442041.2021.1936865
Perrow, M. R., and Davy, A. J. (2002). Handbook of ecological restoration, 2. Cambridge University Press: Cambridge, England.
Piana, M. R., Hallett, R. A., Aronson, M. F., Conway, E., and Handel, S. N. (2021). Natural regeneration in urban forests is limited by early-establishment dynamics: Implications for management. Ecol. Appl. 31 (2), e02255. doi:10.1002/eap.2255
Pollen-Bankhead, N., Simon, A., Jaeger, K., and Wohl, E. (2009). Destabilization of streambanks by removal of invasive species in Canyon de Chelly National Monument, Arizona. Geomorphology 103 (3), 363–374. doi:10.1016/j.geomorph.2008.07.004
Prach, K., and del Moral, R. (2015). Passive restoration is often quite effective: Response to Zahawi et al. (2014). Restor. Ecol., 23(4), 344–346. doi:10.1111/rec.12224
Prach, K., and Hobbs, R. J. (2008). Spontaneous succession versus technical reclamation in the restoration of disturbed sites. Restor. Ecol. 16 (3), 363–366. doi:10.1111/j.1526-100x.2008.00412.x
Prach, K., Řehounková, K., Lencová, K., Jírová, A., Konvalinková, P., Mudrák, O., et al. (2014). Vegetation succession in restoration of disturbed sites in central europe: The direction of succession and species richness across 19 seres. Appl. Veg. Sci. 17 (2), 193–200. doi:10.1111/avsc.12064
Prach, K., and Walker, L. R. (2011). Four opportunities for studies of ecological succession. Trends Ecol. Evol. 26 (3), 119–123. doi:10.1016/j.tree.2010.12.007
Pradhan, K., Ettinger, A. K., Case, M. J., and Lambers, J. H. R. (2023). Applying climate change refugia to forest management and old-growth restoration. Glob. Change Biol.
Prober, S. M., Byrne, M., McLean, E. H., Steane, D. A., Potts, B. M., Vaillancourt, R. E., et al. (2015). Climate-adjusted provenancing: A strategy for climate-resilient ecological restoration. Front. Ecol. Evol. 3, 65. doi:10.3389/fevo.2015.00065
Pugnaire, F. I., Morillo, J. A., Peñuelas, J., Reich, P. B., Bardgett, R. D., Gaxiola, A., et al. (2019). Climate change effects on plant-soil feedbacks and consequences for biodiversity and functioning of terrestrial ecosystems. Sci. Adv. 5 (11), 1834. doi:10.1126/sciadv.aaz1834
Rahel, F. J., and Olden, J. D. (2008). Assessing the effects of climate change on aquatic invasive species. Conserv. Biol. 22 (3), 521–533. doi:10.1111/j.1523-1739.2008.00950.x
Rosbakh, S., Phartyal, S. S., and Poschlod, P. (2020). Seed germination traits shape community assembly along a hydroperiod gradient. Ann. Bot. 125 (1), 67–78. doi:10.1093/aob/mcz139
Saintilan, N., and Rogers, K. (2015). Woody plant encroachment of grasslands: A comparison of terrestrial and wetland settings. New Phytol. 205 (3), 1062–1070. doi:10.1111/nph.13147
Schlaepfer, M. A., Sax, D. F., and Olden, J. D. (2011). The potential conservation value of non-native species. Conserv. Biol. 25 (3), 428–437. doi:10.1111/j.1523-1739.2010.01646.x
Schlatter, K. J., Grabau, M. R., Shafroth, P. B., and Zamora-Arroyo, F. (2017). Integrating active restoration with environmental flows to improve native riparian tree establishment in the Colorado River Delta. Ecol. Eng. 106, 661–674.
Selwood, K. E., and Zimmer, H. C. (2020). Refuges for biodiversity conservation: A review of the evidence. Biol. Conserv. 245, 108502. doi:10.1016/j.biocon.2020.108502
Shimamoto, C. Y., Padial, A. A., da Rosa, C. M., and Marques, M. C. (2018). Restoration of ecosystem services in tropical forests: A global meta-analysis. PLoS One 13 (12), e0208523. doi:10.1371/journal.pone.0208523
Simenstad, C., Reed, D., and Ford, M. (2006). When is restoration not?: Incorporating landscape-scale processes to restore self-sustaining ecosystems in coastal wetland restoration. Ecol. Eng. 26 (1), 27–39. doi:10.1016/j.ecoleng.2005.09.007
Sims, N. C., and Colloff, M. J. (2012). Remote sensing of vegetation responses to flooding of a semi-arid floodplain: Implications for monitoring ecological effects of environmental flows. Ecol. Indic. 18, 387–391.
Summers, D. M., Bryan, B. A., Nolan, M., and Hobbs, T. J. (2015). The costs of reforestation: A spatial model of the costs of establishing environmental and carbon plantings. Land Use Policy 44, 110–121. doi:10.1016/j.landusepol.2014.12.002
Todd, M. J., Muneepeerakul, R., Pumo, D., Azaele, S., Miralles-Wilhelm, F., Rinaldo, A., et al. (2010). Hydrological drivers of wetland vegetation community distribution within Everglades National Park, Florida. Adv. Water Resour. 33 (10), 1279–1289. doi:10.1016/j.advwatres.2010.04.003
Tomscha, S., Bentley, S., Platzer, E., Jackson, B., de Roiste, M., Hartley, S., et al. (2021). Multiple methods confirm wetland restoration improves ecosystem services. Ecosyst. People 17 (1), 25–40. doi:10.1080/26395916.2020.1863266
Tootchi, A., Jost, A., and Ducharne, A. (2019). Multi-source global wetland maps combining surface water imagery and groundwater constraints. Earth Syst. Sci. Data 11 (1), 189–220. doi:10.5194/essd-11-189-2019
Van der Putten, W. H., Macel, M., and Visser, M. E. (2010). Predicting species distribution and abundance responses to climate change: Why it is essential to include biotic interactions across trophic levels. Philosophical Trans. R. Soc. B Biol. Sci. 365 (1549), 2025–2034. doi:10.1098/rstb.2010.0037
Walker, L. R., and Del Moral, R. (2003). Primary succession and ecosystem rehabilitation.(Cambridge University Press: Cambridge, England).
Whisenant, S. (1999). Repairing damaged wildlands: A process-orientated. in Landscape-Scale Approach (Cambridge University Press: Cambridge, England), 1.
Widis, D. C., BenDor, T. K., and Deegan, M. (2015). Prioritizing wetland restoration sites: A review and application to a large-scale coastal restoration program. Ecol. Restor. 33 (4), 358–377. doi:10.3368/er.33.4.358
Wiegman, A. R., Myers, G. H., Augustin, I. C., Kubow, M. L., Fein-Cole, M. J., Perillo, V. L., et al. (2022). Potential for soil legacy phosphorus release from restored riparian wetlands within an agricultural landscape. Biogeochemistry 161 (2), 137–156. doi:10.1007/s10533-022-00972-2
Yang, Y., Hobbie, S. E., Hernandez, R. R., Fargione, J., Grodsky, S. M., Tilman, D., et al. (2020). Restoring abandoned farmland to mitigate climate change on a full Earth. One Earth 3 (2), 176–186. doi:10.1016/j.oneear.2020.07.019
Zahawi, R. A., Reid, J. L., and Holl, K. D. (2014). Hidden costs of passive restoration. Restor. Ecol. 22 (3), 284–287. doi:10.1111/rec.12098
Zhang, Z., Bortolotti, L. E., Li, Z., Armstrong, L. M., Bell, T. W., and Li, Y. (2021). Heterogeneous changes to wetlands in the Canadian prairies under future climate. Water Resour. Res. 57 (7), e2020WR028727. doi:10.1029/2020wr028727
Zivec, P., Balcombe, S., McBroom, J., Sheldon, F., and Capon, S. J. (2021). Patterns and drivers of natural regeneration on old-fields in semi-arid floodplain ecosystems. Agric. Ecosyst. Environ. 316, 107466. doi:10.1016/j.agee.2021.107466
Keywords: restoration, revegetation, passive restoration, rehabilitation, global warming, spontaneous succession
Citation: Zivec P, Sheldon F and Capon SJ (2023) Natural regeneration of wetlands under climate change. Front. Environ. Sci. 11:989214. doi: 10.3389/fenvs.2023.989214
Received: 08 July 2022; Accepted: 19 May 2023;
Published: 05 June 2023.
Edited by:
Martin Siegert, University of Exeter, United KingdomReviewed by:
Anders Bryn, University of Oslo, NorwayCopyright © 2023 Zivec, Sheldon and Capon. This is an open-access article distributed under the terms of the Creative Commons Attribution License (CC BY). The use, distribution or reproduction in other forums is permitted, provided the original author(s) and the copyright owner(s) are credited and that the original publication in this journal is cited, in accordance with accepted academic practice. No use, distribution or reproduction is permitted which does not comply with these terms.
*Correspondence: Peta Zivec, cGV0YS5sLnppdmVjQGdtYWlsLmNvbQ==