- 1Department of Farm Power and Machinery, Bangladesh Agricultural University, Mymensingh, Bangladesh
- 2Department of Civil and Environmental Engineering, Hanyang University, Seoul, Republic of Korea
- 3Department of Earth Resources and Environmental Engineering, Hanyang University, Seoul, Republic of Korea
Biochar is a versatile and sustainable tool for agricultural and environmental remediation due to its unique physicochemical properties in terms of soil fertility, nutrient retention, and water holding capacity. As a stable carbon-rich material, biochar promotes plant growth and increases crop yields by enhancing microbial activity. It can also be used as a sorbent for removing pollutants such as heavy metals, organic contaminants, and nutrients from soil and water systems. However, the utility of biochar in soil and its ecological impact can be affected by the combined effects of many variables. This paper discusses the effects of biochar application on soil properties and its potential to mitigate various environmental challenges by enhancing soil composition, augmenting water accessibility, and removing pollutants as part of efforts to promote sustainable agriculture based on recent findings. These findings are expected to improve the utility of biochar in farming while contributing to the mitigation of climate change in diverse routes (e.g., by sequestering atmospheric carbon, improving soil quality, and reducing greenhouse gas emissions). This paper offers a promising opportunity to help harness the power of biochar and to pave the way for a more sustainable and resilient future.
Highlights
• Biochar has valuable soil amendment benefits to improve soil properties
• Biochar has positive impact on plant growth and crop yields, particularly in coarse-textured soils
• It has a wide range of environmental benefits specially on greenhouse gas mitigation
• The risks that may arise from the use of biochar need to be carefully evaluated
1 Introduction
Climate change, soil degradation, soil contamination, scarcity of water, and food security pose serious risks to the environment and civilization (Alkharabsheh et al., 2021). Among the tools available to address such concerns, biochar is a promising option with multiple advantageous properties, such as high porosity and surface area; the ability to sequester carbon, reduce nutrient leaching and soil acidity; and a role in mitigating climate change (Brtnicky et al., 2021). Biochar is a charcoal-like product generated by pyrolysis in a low-oxygen environment at temperatures between 300 and 1,000°C using organic biomass (e.g., agriculture residues, animal manure, and municipal wastes) as feedstock (Diatta et al., 2020). In addition to its primary components (carbon, hydrogen, and oxygen), biochar may also contain macronutrients such as nitrogen, phosphorus, and potassium (Alkharabsheh et al., 2021). However, the overall composition and characteristics of biochar depend on the choice of feedstock and the production process (i.e., the type of thermal conversion and temperatures applied) (Wang et al., 2020).
The potential benefits of biochar in the effort to mitigate climate change are difficult to estimate due to its ability to sequester carbon in a resistant form, reduce the emissions of the strong greenhouse gases such as nitrous oxide and methane, increase crop yields and fertilizer efficiency, restore degraded lands, and offset water pollution by removing organic contaminants (such as pesticides, herbicides, dyes, pharmaceutical/personal care products, perfluorooctane sulfonate, humic substances, and N-nitrosomodimethylamine) (Ayaz et al., 2021). The application of biochar to soil has been demonstrated to enhance soil structure, boost nutrient retention, foster the growth of microorganisms, and increase plant nutrient uptake (Arif et al., 2021). As a refractory form of carbon that breaks down slowly in soil, it may take many years for biochar to disintegrate completely (de Freitas et al., 2020).
Depending on the composition of biochar, it can also act as a source of pollutants such as heavy metals, volatile organic compounds (VOCs), polycyclic aromatic hydrocarbons (PAHs), and dissolved organic carbon (Brtnicky et al., 2021). In addition, crop production may decrease due to sorption of water and nutrients by biochar (Allohverdi et al., 2021). Most relevant studies have been conducted under laboratory conditions over the short term (<2 years). Despite a large and growing body of research on biochar in recent years, relatively little is known about the economic and logistical feasibility of biochar application at larger scales under field conditions. As the use of biochar has both benefits and drawbacks, this review focuses on the following: the impact of biochar on soil characteristics, the leaching of nutrients, ecological advantages, and potential hazards to the environment and human health associated with feedstocks, production, and application. This review is intended to help raise the awareness of the potential benefits of biochar through its adoption in agriculture along with the discussions on the challenges associated with biochar (Refer to a tree diagram for the main concepts of this review as provided in Figure 1.) As such, it is expected to helpfarmers and scientists develop and select suitable biochar technologies to enhance agriculture and environmental sustainability without damaging crop production.
2 Physical structure of biochar
Biochar is black, highly porous, lightweight, fine-grained material with large surface area values. Biochar characteristics, such as microbial activity, mineral and nutrient binding, and soil water holding capacity (WHC), depend on the physical structure, pore size, and surface area of the type of feedstock used to produce the biochar (Tomczyk et al., 2020). For example, biochar produced from coconut shells with a particle diameter of 1.55 mm at a reaction temperature of 850 °C and a retention time of 1.5 h in a fluidized bed reactor was found to have a surface area of 1,400 m2 g-1 (Khawkomol et al., 2021). The surface area of biochar determines its potential to retain water, hold nutrients, and interact with microbes and pollutants in the environment. A larger surface area provides more sites for adsorption reactions and microbial colonization (Wyn et al., 2020). In comparison with biochar made from woody biomass, biochar made from other sources (e.g., manure, seaweed, and agricultural residue) typically has greater nutrient content, a higher pH, and less stable carbon (Rawat et al., 2019). The porosity of biochar governs its ability to retain water and nutrients while facilitating microbial activity (Khawkomol et al., 2021).
The surface area of biochar typically ranges from 8 to 132 m2 g-1, while total pore volume can range from 0.016 to 0.083 cm3 g-1 (Leng et al., 2021). However, under controlled conditions (i.e., with a suitable precursor and optimal pyrolysis parameters), the surface area and total pore volume of biochar can be as high as 491 m2 g-1 and 5.31 cm3 g−1, respectively (Liu et al., 2016; Khawkomol et al., 2021; Yang et al., 2021) Liu et al. (2016) found that effective post-treatment such as KOH activation can result in biochar with a surface area of up to 3,263 m2 g-1 and a total pore volume of 1.77 cm3 g-1. The surface area of biochar produced from lotus stems (1,610 m2 g-1) is reportedly significantly larger than that derived from lotus leaves (1,039 m2 g-1), which reflects the variation in the content of metal ions, particular potassium (Zhang et al., 2017b). According to (Sun et al., 2013), a high organic carbon content stimulates the formation of larger micropore surface areas (r = 0.97; p 0.01), whereas a high ash concentration leads to a decrease in micropore surface areas (r = −0.94; p 0.01). This is primarily because the formation of pores is strongly affected by the release of volatile substances. However, the presence of ash frequently obstructs pores, resulting in a smaller surface area. Among various types of biochar made from 12 different feedstocks, sawdust with the lowest ash content (2.8 wt%) produced the maximum surface area (203 m2 g-1), whereas waterweeds and cow dung with the highest ash content (approximately 38%) produced the smaller surface area (3–22 m2 g-1) (Zhao et al., 2013). Figure 2 depicts scanning electron microscopic images of biomass and biochar (pyrolyzed at 400–550°C for 15–120 min) produced from poplar biomass, sewage sludge, and corn (Zhao et al., 2019).
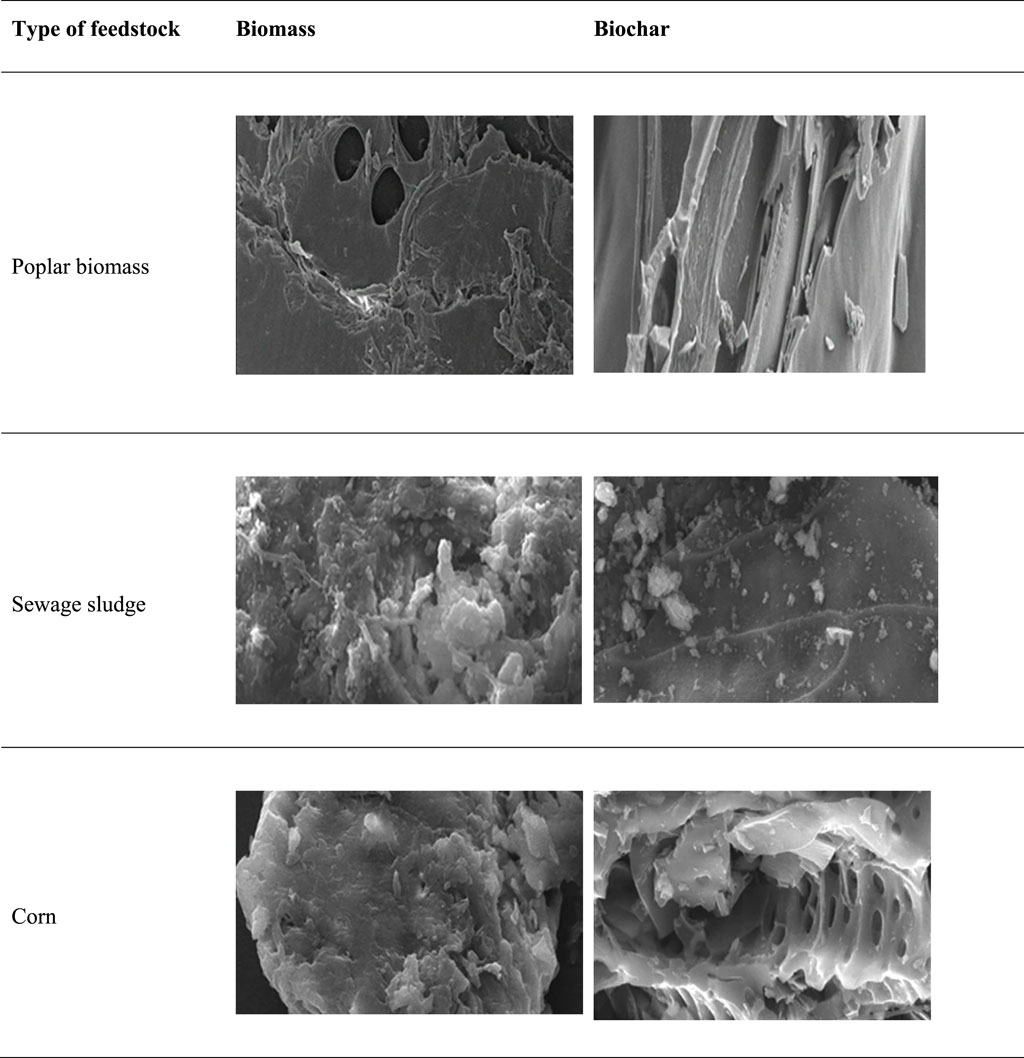
FIGURE 2. Scanning electron microscopic image of various feedstocks (Zhao et al., 2019).
Activation, and chemical activation in particular, is the most widely used and effective approach to enhancing the surface area and porosity of biochar (Alkharabsheh et al., 2021). Other treatment methods such as carbonaceous material coating, ball milling, and templating can also be used to achieve further enhancement. The combined effects of both the removal of water through dehydration during the pyrolysis of biomass and the release of volatile components from the carbon matrix can play a crucial role in the formation of biochar’s pore structure. According to the International Union of Pure and Applied Chemistry, biochar can be divided into three pore-size categories: micropores (<2 nm), mesopores (2–50 nm), and macropores (>50 nm) (Khawkomol et al., 2021). Changes in the surface area and porosity of biochar at various temperatures are directly related to changes in the biochemical content of the biomass. Devolatilization of biochar occurs to a modest extent above 500°C, which leads to the production of additional pores through breaking (Collard and Blin, 2014). However, the increased ash content due to rising temperatures may reduce the growth rates of surface area and total pore volume (Luo et al., 2015).
Another element that has a strong correlation with biochar’s porosity and surface area is the heating rate. In most cases, there is an initial increase in surface area and total pore volume, which is then followed by a drop. The surface area and total pore volume of biochar reportedly increased from 296 to 384 m2 g-1 and from 0.166 to 0.219 cm3 g-1, respectively, when the heating rate was increased from 1°C to 20°C min-1, respectively (Zhao et al., 2018). High heating rates are more likely to allow biochar particles to form smoother surfaces through melting (Luo et al., 2015). However (Chen et al., 2016), found that the surface area and total pore volume decreased from 411 to 385 m2 g-1 and 0.182 to 0.161 cm3 g-1, respectively, as heating rates were increased from 30°C to 50 °C min-1. A similar trend was also observed for residence time. For example, when the residence time was prolonged from 10 to 60 min, the surface area increased from 46.7 to 98.4 m2 g-1; however, the surface area was reduced to 91.4 m2 g-1 as the residence time was raised to 100 min (Zhao et al., 2018). An increase in the flow rate of the carrier gas (nitrogen) increased biochar’s surface area and total pore volume. If the carrier gas flow rate exceeds the desired level, it can produce a detrimental effect. For example, the surface area and total pore volume increased 36–352 m2 g-1 and 0.012–0.125 cm3 g-1, respectively, when the nitrogen flow rate was increased from 50 to 150 cm-3 min-1 (Bouchelta et al., 2012). However, as the nitrogen flow rate was increased further, the biochar processing temperature dropped as a result of the high carrier gas flow rate, reducing both the reaction rate and the release of volatile matter. As a result, the surface area and total pore volume were reduced from 164 to 136 m2 g-1 and 0.058 to 0.048 cm3 g-1, respectively, as the nitrogen flow rate was increased from 200 to 400 cm3 min-1 (Bouchelta et al., 2012).
The specific chemical properties of biochar can vary depending on the production process and the source material used. Biochars with high content carbon were reported to range from 60% to 95% (Brtnicky et al., 2021). The remaining percentage consists of nitrogen, hydrogen, and oxygen among other elements. For instance, the concentration of nitrogen (N) in hardwood and the poultry litter biochar were found as 0.71% and 3.57%, respectively (Egamberdieva et al., 2021). The phosphorus (P) concentration of switchgrass biochar had a relatively low P concentration of 4.49 mg L−1 while the mixed hardwoods biochar exhibited a much higher P values of 125.9 mg L−1 (Evans et al., 2017). Further, the mixed hardwoods biochar had the lowest potassium (K) concentration at 1985 mg L−1, while the poultry litter biochar had the highest K concentration at 18,391 mg L−1 (Evans et al., 2017). Physicochemical properties of biochar are depicted in Figure 3.
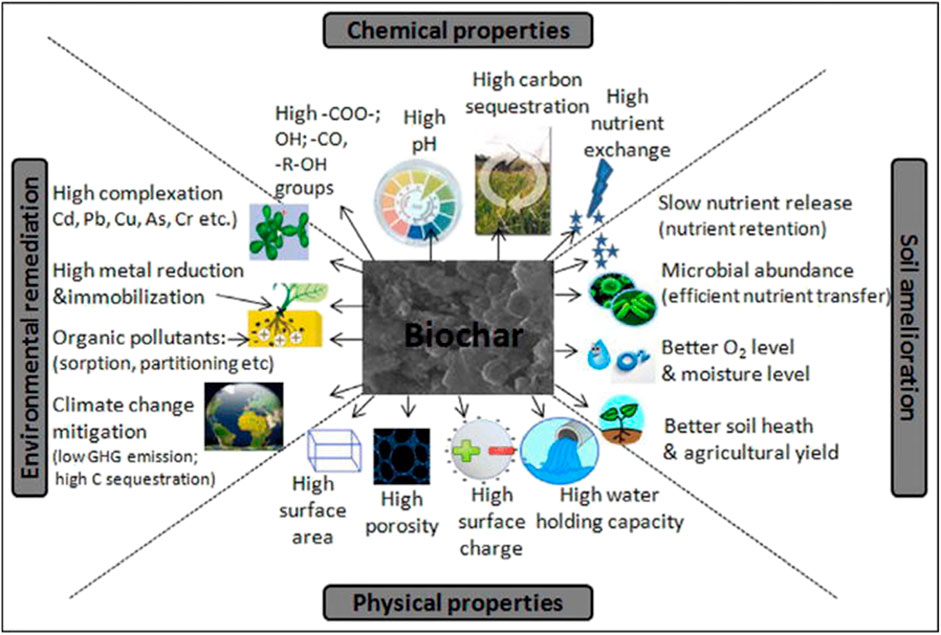
FIGURE 3. Physicochemical properties of biochar (Shreya et al., 2021).
3 Effects of biochar on soil properties
Applying biochar to soil can create a favorable environment for the growth of plants and uptake of nutrients by improving both the physiochemical and biological properties of the soil, such as porosity, water infiltration, WHC, aggregate stability, bulk density, soil hardness, pH, cation exchange capacity, and nutrient cycling (Kavitha et al., 2018; Adekiya et al., 2020).
3.1 Soil porosity
Soil porosity is a key variable determining the suitability of soil for various agricultural and environmental applications. A high porosity can improve water retention for plant growth and overall soil health (Purakayastha et al., 2019). Biochar has been found to increase soil porosity by creating channels and spaces in the soil for air and water, promoting nutrient uptake and overall crop productivity (Lu et al., 2023). Porous soil can absorb and retain pollutants while sequestering CO2 from the atmosphere (Blanco-Canqui, 2017). Biochar also reportedly increases capillary and overall soil porosity by 23% and 24%, respectively (Zhang et al., 2017b). Conducted A 2-year field experiments on sandy loam alfisol soil found containing four concentrations of hardwood biochar at: 0 t ha−1 (control), 10 t ha−1, 20 t ha−1, and 30 t ha−1 (Adekiya et al., 2020). A noticeable increase in soil porosity of 65% (compared with the untreated control) was recorded at the application rate of 30 t ha−1. Blanco-Canqui (2017), who conducted a meta-analysis of 104 studies, found that biochar application typically reduced soil bulk density by 3%–31%, while simultaneously increasing porosity by 14%–64%. Similarly, the addition of biochar was also found to enhance soil porosity by 8.4% (Omondi et al., 2016). However, the effects of biochar on soil porosity depend largely on the soil type. Table 1 shows the effects of different types of biochar on soil porosity.
3.2 Soil water holding capacity
Water holding capacity (WHC) plays a vital role in agriculture as it directly affects plant growth and overall crop productivity. Biochar can improve soil WHC by increasing the porosity of the soil, providing a surface for water to adhere to (Razzaghi et al., 2020). The larger surface area of biochar allows it to hold more water per unit volume of soil while the porous structure of biochar helps improve water retention (Mclennon et al., 2020). The pores in biochar provide a network of water-filled channels through which water can move more easily (Liu et al., 2017). By reducing water runoff and erosion, biochar is particularly beneficial in regions with limited water resources or extended dry periods and limited irrigation. Biochar’s porous structure also creates microhabitats that help promote beneficial microbial activity. These microorganisms can improve nutrient cycling and root development while boosting moisture retention. Biochar-based amendment of WHC complies with sustainable farming practices by reducing reliance on synthetic fertilizers and/or excessive irrigation methods. As such, it can serve as an environmentally friendly solution for maintaining soil health and conserving water resources.
The amount of water that biochar can hold depends on the type of biochar, pyrolysis conditions, and soil type. In general, biochar can increase the WHC of soil by 10%–30% (Adekiya et al., 2020). Likewise, the addition of biochar to sandy soil reportedly increased the WHC by 20% (Li et al., 2021), while adding it to clay soil increased the WHC by 30% (Liu et al., 2023a). Biochar made from rice husks can hold up to 2.7 times as much water relative to its weight (Asadi et al., 2021). Also, a 10.8% increase in soil moisture content was attained by applying sawdust and rice-husk biochar at a rate of 5–10 Mg ha-1 compared with a group to which no biochar was added (Ndor et al., 2015). It was also observed that applying poultry-litter biochar to sandy-loam soil increased the moisture content by 33% (Are, 2019). In a long-term columnar study, biochar-supplemented Clarion soil retained up to 15% more water compared with a control (Laird et al., 2010). Similarly, the addition of 1% biochar (by mass) to gray soil (with an initial moisture content of 13%) boosted its WHC capacity by 26%–33% depending on the type of biochar used (coffee husk or rice husk) (Duong et al., 2017). Aging biochar for a minimum of 1 year reportedly improves the WHC while decreasing hydrophobicity (Adhikari et al., 2022). Table 2 lists the impacts of biochar on WHC.
3.3 Soil organic carbon content
Soil organic carbon (SOC) is a crucial component of soil health and fertility due to its vital role in regulating soil structure, water retention, nutrient cycling, and carbon sequestration (Gross et al., 2021). However, the effects of biochar application on SOC depend on the type of biochar, application rate, and soil type. For example, it took 2 years to observe a notable increase in SOC (from 4.0% to 26.7%) after the introduction of straw biochar (Tao et al., 2020). Over a period of 4 years, application of biochar at rates of 6 and 12 t ha⁻1 resulted in an increase in SOC by 11.02% and 22.13%, respectively (Zheng et al., 2022). After applying biochar at 1–100 Mg ha−1, an average 13.0 Mg ha-1 (29%) increase in SOC was recorded. However, in a pot-and-incubation experiment, applying biochar at 5–200 g kg−1 over a period of 3.5 years increased SOC by an average of 6.3 g kg−1 (75%) (Gross et al., 2021). Applying straw biochar for 5 days increased the SOC content from 34.5% to 38.0% (Jing et al., 2020). Likewise, the SOC rose from 3.1 to 4.9 mg kg-1 after adding biochar (Zhang et al., 2017a). The increase in SOC likely reflected the combined effects of the stability of biochar, its ability to improve soil aggregation, and the presence of conditions necessary for microorganism growth.
3.4 Soil bulk density
Soil bulk density is a crucial parameter that determines soil health. Soil with high bulk density may develop high penetration resistance for plant roots (Omondi et al., 2016). Biochar has been shown to have a positive impact on soil bulk density. However, the effect of biochar on soil bulk density also depends on the type of biochar, the amount of biochar added to the soil, and the properties of the soil. As biochar has a lower bulk density compared with soil (0.6 g cm⁻³ vs. 1.25 g cm⁻³), adding biochar helps reduce the overall bulk density of soil (Blanco-Canqui, 2017). According to a literature review by (Omondi et al., 2016), it the addition of biochar resulted in a reduction in soil bulk density of 3%–31%. Similarly, it was found that the incorporation of 30 Mg ha-1 of biochar led to a reduction in soil bulk density of up to 75% when compared with soil without biochar (Adekiya et al., 2020). The effect of biochar on soil bulk density can be influenced by the texture of the soil. Biochar has greater impact on reducing soil bulk density in coarse-textured soils compared with fine-textured soils (Alghamdi, 2018). Based on a series of laboratory investigations, declines in soil bulk density peaked in sandy soil (approximately 31%) followed by coarse soil (14.2%) and fine-texture soil (9.2%) after applying biochar (Liu et al., 2017). In another study, the use of biochar reduced the bulk density of coarse and finely textured soil by 14.2% and 9.2%, respectively (Blanco-Canqui, 2017).
3.5 Soil pH
Soil pH is an important factor controlling the growth and health of plants. Acidic soils can have a number of negative effects on plant growth, such as reduced nutrient availability, increased susceptibility to pests and diseases, and stunted growth (El-Naggar et al., 2018). Biochar can neutralize acidity and/or raise the pH of soil by releasing alkaline compounds such as potassium carbonate and calcium carbonate (El-Naggar et al., 2018). In addition, biochar can help soil efficiently buffer changes in pH. However, biochar can also have a negative impact on soil pH if it is applied to alkaline soils (Chathurika et al., 2016). The effect of biochar on soil pH is dependent on the type of feedstock, pyrolysis conditions, and soil type (Tian et al., 2017).
Soil pH was observed to increase by an average of 0.29 units after cacao-shell biochar was applied to acidic soils (Martinsen et al., 2015). The increase in pH was most pronounced in soils with a low initial pH. For example, the pH of soil increased from 4.5 to 5.0 after the application of biochar. In sandy soil, the pH values exhibited a significant increase of 51.1%, 86.3%, and 46.8% upon treatment with silvergrass, paddy-straw, and umbrella-tree biochar, respectively, compared with untreated soil (control) (El-Naggar et al., 2018). It was also found that the pH of clay-loam soil increased by 0.46 pH units after 70 days of applying Acer-woodchip biochar at 20 g kg−1, while the pH of the loamy-sand soil only increased by 0.23 pH units (Chathurika et al., 2016). However, the same research team also reported that the application of synthetic fertilizer reduced the soil pH of the clay-loam and loamy-sand soil by 0.13 and 0.07 pH units, respectively (Chathurika et al., 2016). This is because synthetic fertilizers typically contain nitrogen, phosphorus, and potassium. As nitrogen and phosphorus are acidic components of soil in the form of nitrate (NO3-) and phosphate (PO43-), respectively, they can lower the pH of soil by releasing hydrogen ions (H+) into the soil solution. Likewise, no increase in the pH of clay soil was seen after applying 10 Mg ha-1 of biochar produced from ponderosa pine, wood residues, maize stover, and switchgrass (Sandhu et al., 2017). This outcome may be attributable to the combined effects of the buffering capacity of the clay soil and the low biochar application rate. Sandu et al. suggested that the buffering effect of switchgrass biochar on clay soils may limit the ability of biochar to increase soil pH (Sandhu et al., 2017). However, it is also possible that the low application rate was not sufficient to cause a significant increase in pH.
3.6 Cation exchange capacity
The cation exchange capacity (CEC) of biochar is an important variable that controls its potential for soil amendment. CEC refers to the ability of biochar to attract and retain positively charged ions (e.g., calcium, magnesium, sodium, and potassium) that are essential for plant growth (Karimi et al., 2020). The CEC of biochar is determined by the number of negatively charged functional groups on the surface of the biochar particles. These functional groups can attract and hold cations, which improve the nutrient-holding capacity of the soil. The CEC of biochar can depend on the feedstock used to make the biochar, the pyrolysis temperature, and the post-treatment of the biochar (Adhikari et al., 2022). In general, biochar made from agricultural residues, such as rice husks and corncobs, has a higher CEC compared with biochar made from wood. The CEC of biochar can also be raised by post-treatment methods such as ozonization or alkaline activation. Differences in the CEC values are apparent for biochar made from rice husks (20–40 cmol(c) kg-1), corncobs (15–30 cmol(c) kg-1), and wood (10–20 cmol(c) kg-1) and ozonized biochar (50–100 cmol(c) kg-1) (Munera-Echeverri et al., 2018).
In a greenhouse of sandy-loam soil, a significant (21%) increase in the CEC was seen after the application of rice-husk biochar, in comparison with unamended soil (control) (Zhang et al., 2017a). Likewise, biochars derived from rice straw, silvergrass residues, and umbrella trees were also applied to sandy soils (El-Naggar et al., 2018), resulting in significant rises in the CEC of 906%, 180%, and 130%, respectively, compared with unamended soil. In another field study, the application of biochar (made from cashew-tree wood and pyrolyzed at 500 °C) at 30 Mg ha-1 to sandy-loam soil led to a 65% increase in the CEC of the soil (Adekiya et al., 2020). Further, the application of 5 Mg ha-1 of biochar produced from rice husks and sawdust resulted in a 21% increase in the CEC in both cases (Ndor et al., 2015). Also, 44% and 57% increases in CEC were observed, respectively, when 10 Mg ha-1 of each biochar was applied compared with non-biochar application (Ndor et al., 2015). The results of many other studies consistently show that biochar application can be increase the CEC of soil.
3.7 Microbiome
Biochar can affect the soil microbiome in a number of ways. For example, as biochar can provide a surface for microorganisms to attach to, it can increase the availability of nutrients, while suppressing the growth of harmful microorganisms (Li et al., 2020). Biochar can also increase the diversity of the soil microbiome (Lv et al., 2022). A diverse microbiome is more resilient to environmental changes, promoting nutrient cycling and plant growth. Biochar application reportedly increased the soil abundance of beneficial bacteria such as Bacillus and Pseudomonas by up to 100% (Ajema, 2018). These bacteria play a role in promoting plant growth and nutrient cycling. The same study found that biochar application decreased the abundance of harmful fungi such as Fusarium and Phytophthora by up to 50%. In a scientific report covering 964 data points from 72 papers published between 2007 and 2020, biochar was reported to substantially improve soil microbial biomass carbon by 21.7%, urease activity by 23.1%, alkaline phosphatase activity by 25.4%, and dehydrogenase activity by 19.8% (Pokharel et al., 2020). In addition, no significant negative effects of biochar were reported on any of the enzymes. Another study found that biochar application at rates of 20,000 and 40,000 kg ha-1 significantly altered soil microbial community composition, with a shift in favor of bacterial over fungal populations (Chen et al., 2013). A meta-analysis found that biochar is more effective in increasing microbial biomass in acidic and sandy soils and in soils with low soil organic carbon content (Li et al., 2020). Biochar produced at pyrolysis temperatures of 350°C–550 °C with a low C/N ratio (<50) were also more effective in increasing microbial biomass (Li et al., 2020). Table 3 shows the effect of biochar on soil biological properties.
4 Biochar effects on nutrient leaching
4.1 Nutrient availability
The nutrient content of biochar varies depending on the pyrolysis temperature and the feedstock used. Although biochar can be a valuable source of nitrogen, it can also help improve the availability of phosphorus and potassium. In addition to its effects on soil properties, biochar can also improve nutrient use and nutrient uptake efficiency by plants. This is because biochar can help retain nutrients in the soil by preventing them from leaching or being lost to the atmosphere. The nutrient content of biochar, except for nitrogen content, is positively correlated with pyrolysis temperature (Hossain et al., 2020). Because nitrogen is a volatile element that is more likely to be lost during pyrolysis at higher temperatures, biochar produced from manure and wastes at low temperatures (≤400 °C) is a good source of nitrogen (Hossain et al., 2020). By comparison, because phosphorus, potassium, and other nutrients are less volatile, they are more likely to be retained in biochar even at higher temperatures. This is why manure-and-waste biochar has a higher level of such nutrients compared with biochar produced from crop residues and woody biomass (Hossain et al., 2020). A previous study found that the coarse fraction of biochar derived from husks and paper-fiber feedstock had a higher concentration of total nitrogen, phosphorus, and potassium (Prasad et al., 2019). These authors concluded that the availability of calcium and magnesium nutrients in biochar is greatly influenced by both the feedstock and particle size. In another study, the release of carboxylates from barley roots was increased by up to 300% by the application of biochar amended with phosphorus (Honvault et al., 2022). Carboxylates, organic acids that help solubilize nutrients in the soil, can play a crucial role in the solubilization of nutrients in the soil. In one study, application of biochar reportedly enhanced the amount of nutrients available in the rhizosphere, possibly leading to longer roots and more root hairs (Prendergast-Miller et al., 2014).
In comparison with unaltered soil, the addition of maize-residue biochar at 1%–2% (w/w) enhanced total nitrogen, phosphorus, potassium, manganese, iron, zinc, and copper by 41%, 165%, 160%, 21%, 17%, 42%, and 10%, respectively (Sahin et al., 2017). The incorporation of biochar into calcareous soil was observed to facilitate the decomposition of calcium carbonate and release nutrients and increase their availability to plants. Accordingly, acid-modified biochar was found to be superior to unmodified biochar in enhancing the availability of soil nutrients (Sahin et al., 2017). However, the choice of feedstock materials and C:N ratio are crucial factors affecting both the amount of nutrients in the biochar and how quickly the nutrients are released into the soil. For example, biochar made from sewage sludge at 350 °C had a greater nitrogen content (3.17%) than did biochar made from sugarcane (1.4%) or eucalyptus trash (0.4%) (Figueredo et al., 2017). The elevated nitrogen content in biochar derived from sewage sludge can be attributed to the high-nitrogen composition of the sludge. Sewage sludge, which is typically produced in wastewater treatment plants, contains a variety of organic matter, including proteins, carbohydrates, and fats. These organic compounds contain nitrogen, which can be released during pyrolysis. Moreover, the effects of biochar amendment are most conspicuous in sandy soils, succeeded by sandy loam, and subsequently fine-textured soils (Blanco-Canqui, 2017). The incorporation of rice-straw biochar to sandy soil resulted in a significant increase in the total nitrogen content of 125%, but only 22% in sandy-loam soil (El-Naggar et al., 2018). The benefits of biochar amendment are pronounced in sandy soil, which has less organic matter compared with finely textured soils.
4.2 Nutrient leaching from soil
Nutrient leaching is a significant issue in agriculture, leading to soil degradation and reduced crop yields. Biochar has been observed to help mitigate nutrient leaching by improving soil structure and increasing nutrient retention (Karimi et al., 2020). The high CEC of biochar allows it to bind to nutrients and prevent them from being leached away by water (Rubin et al., 2020). As biochar can also improve soil structure, the soil becomes more porous, which allows water to drain more slowly. This gives the plants more time to absorb nutrients before they are leached away. Biochar can physically trap nutrients, particularly anions such as nitrate and phosphate, in its pores. Biochar can also increase water retention, which can keep nutrients in the soil. Biochar reportedly reduced nitrate leaching by up to 37% in a sandy-loam soil (Jiang et al., 2022). Likewise, rich-husk biochar was able to reduce phosphate leaching by up to 20% in a clay-loam soil (Pratiwi et al., 2016). Further, the cumulative leaching of phosphorus (37.7%) and NH4+ (50.2) was greatly reduced by the application of biochar at 10% (w/w) (Rubin et al., 2020). The leaching of NO3-, NH4+, and total nitrogen in loamy-sand soil was reduced by 23%, 18%, and 19%, respectively, when biochar was added, compared with unamended soil (Xu et al., 2016). As such, biochar can significantly reduce nitrogen leaching from soil by boosting soil nitrogen retention, reducing ammonia volatilization, or transforming it to nitrate through nitrification in saline soil (Sun et al., 2017). However, the effectiveness of biochar in reducing nutrient leaching varies depending on the type of biochar used, soil type, and environmental conditions. For example, biochar made from wood enhances phosphate adsorption more effectively than does biochar made from leftover straw (Rubin et al., 2020).
5 Environmental benefit of biochar
5.1 Carbon sequestration
Climate change is a major threat to the environment and human livelihoods. The excessive emission of CO2 and other greenhouse gases (GHGs) into the atmosphere has had a dramatic on effect on the global climate. Elevated CO2 levels have also been linked to soil carbon loss (Chen et al., 2020). Atmospheric CO2 levels have been rising rapidly, reaching 412 ppmv in 2020 (Friedlingstein et al., 2022). It is projected that the emissions of carbon dioxide could reach approximately 54–56 gigatonne (Gt) in 2030 surpassing the threshold of 42 Gt required to potentially restrict global warming to 2°C (Mahato et al., 2022). Carbon sequestration involves the long-term storage of CO2 (or other forms of carbon) to help mitigate or defer global warming (Yadav et al., 2017).
Biochar is a promising tool for carbon sequestration due to its ability to remain stable in the soil for hundreds or even thousands of years (Yin et al., 2022). In fact, biochar produced from agricultural waste was estimated to sequester up to 1.2 t C ha-1 year-1 (Yrjälä et al., 2022). Likewise, biochar produced from manure could sequester up to 0.6 t C ha-1 year-1 (Rehman et al., 2020). Similar results were also found such that biochar could sequester up to 0.8 t C ha-1 year-1 (Lal et al., 2018). According to a literature review, the mean capacity of biochar to capture carbon was approximately 0.7 t C ha-1 year-1 (range = 0.1–2) (Majumder et al., 2019). Biochar particles smaller than 0.5–2 mm are associated with lower CO2 and N2O emission rates compared with larger particles (5–10 mm) (Weng et al., 2020). As smaller particles have a higher surface area–to–volume ratio, they can be more accessible to microbes and are therefore more likely to decompose. Biochar application can have both positive and negative effects on carbon sequestration. Positive priming effects can lead to carbon mineralization, while negative priming effects can lead to carbon stabilization (Majumder et al., 2019). The type of priming effect that occurs depends on the pyrolysis temperature used to produce the biochar. Biochar produced at low temperatures (250°C–400 °C) typically has a positive priming effect, while biochar produced at high temperatures (525°C–650 °C) typically has a negative priming effect (Zimmerman et al., 2011). However, the carbon footprint of biochar is a complex matter that is contingent on various factors. Biochar can nonetheless be considered a carbon-negative product as it can sequester a greater amount of carbon than it emits.
5.2 Nitrous oxide emissions
Nitrous oxide (N2O) is a greenhouse gas that is approximately 300 times more effective at trapping heat compared with CO2 over a 100-year period (Hoekman, 2020). It is released primarily through agricultural practices such as the use of nitrogen fertilizers and livestock manure management. Biochar can help reduce N2O emissions from agricultural soils by increasing soil pH, reducing soil compaction, and enhancing soil microbial activity. Biochar application significantly reduced N2O emissions from sandy-loam soil (Niu et al., 2017). The reductions were as high as 50% which was consistent across a range of biochar application rates and soil-moisture conditions (Liu et al., 2014). According to a meta-analysis study, biochar application was able to reduce N2O emissions by an average of 26% (Shakoor et al., 2021). The reductions were more pronounced in soils with high levels of available nitrogen. Biochar application can affect soil N2O emissions in different ways, depending on the edaphic conditions. Biochar can increase nitrification, leading to enhanced N2O emissions when soil ammonium concentrations are high due to fertilizer application (Edwards et al., 2018). Biochar can also prevent significant spikes in N2O emissions after a heavy rainfall (Edwards et al., 2018). A previous study reported that the application of animal manure resulted in a 17.7% increase in N2O emissions, while the use of biochar amendments significantly reduced N2O emissions by 19.7% (Shakoor et al., 2021). In another experiment conducted in flooded and non-flooded soils during freeze-thaw cycles, the addition of biochar led to a noteworthy decrease in N2O emissions, with reductions of up to 67% (Yang et al., 2022b).
The magnitude of the N2O emissions reduction by biochar depends on the biochar application rate and C:N ratio (Kaur et al., 2023). The effects of biochar application on soil N2O emissions are also time-dependent (Kaur et al., 2023). Biochar application can suppress warming-induced stimulation typical of N2O emissions. The outcomes of the trials with a substantial biochar addition (25 Mg ha−1) demonstrated that N2O emissions at 40 °C were equivalent to or less than those recorded at 20 °C (Rittl et al., 2021). Another study revealed that the addition of biochar can significantly augment denitrification, leading to a reduction of up to 98% in N2O accumulation (Zhang et al., 2021). The study demonstrated that the stimulating effect of biochar is primarily attributable to the bulk particles rather than the released soluble compounds (Zhang et al., 2021). These findings suggest that biochar can enhance denitrification by establishing a physical or chemical environment conducive to the growth and activity of denitrifying bacteria. However, the efficacy of biochar in mitigating N2O emissions is contingent on the choice of feedstock and pyrolysis temperature. For example, maize-stover biochar achieved a 17% greater reduction in N2O emissions than eucalyptus biochar (Fungo et al., 2014). Additionally, biochar pyrolyzed at 350 °C demonstrated a 3% superior reduction in N2O emissions compared with biochar pyrolyzed at 550 °C (Fungo et al., 2014).
5.3 Methane emissions
The utilization of biochar as a means of mitigating methane (CH4) emissions has also attracted attention in recent years. Biochar has been demonstrated to effectively reduce CH4 emissions from various sources such as livestock manure, rice fields, and landfills. The mechanism behind this reduction is believed to be the adsorption of CH4 onto the biochar surface, which subsequently undergoes microbial oxidation. One previous study found that spruce-wood biochar, applied at a 10 t ha-1 for 7 years, dramatically reduced CH4 gas emissions by up to 43% (Kalu et al., 2022). A meta-analysis comprising 43 papers has also revealed that the application of biochar has resulted in a significant reduction of CH4 emissions by an average of 37.9% in East Asian rice fields (Lee et al., 2023). Biochar could reduce CH4 emissions from landfills by up to 60% (Yaghoubi et al., 2014). Likewise, biochar amendment to landfill cover soil significantly reduced emissions by up to 80% (Yargicoglu and Reddy, 2017). This reduction was likely achieved by increasing the abundance of methanotrophs, as they consume CH4 efficiently. Biochar also improved soil aeration and drainage to further reduce CH4 emissions. Hence, hydrophobic biochar-amended landfill cover soil can reduce CH4 emissions from landfills more effectively than can biochar-amended landfill cover soil (Qin et al., 2022).
Rice straw–derived biochar reportedly reduced CH4 emissions from paddy soil at elevated temperatures and CO2 concentrations (Han et al., 2016). This reduction in CH4 emissions was mainly attributable to the decreased activity of methanogens along with the increased CH4 oxidation activity and pmoA gene abundance of methanotrophs. Furthermore, aged biochar was seen to be more effective than fresh biochar in promoting CH4 oxidation (Wu et al., 2019). This is likely because aged biochar has a higher surface area and more pores then fresh biochar, which can provide more surface area for methanotrophs to colonize. Biochar produced at a pyrolysis temperature of 300 °C reportedly increased CH4 emissions from paddy soil by an average of 38%. (Ji et al., 2020). This was due to the presence of easily biodegradable organics in the biochar, as they can server as a more effective substrate for CH4 production. Conversely, biochar produced at higher pyrolysis temperatures of 700 °C has been found to reduce CH4 emissions by approximately 18.2% (Ji et al., 2020) due to the higher concentration of aromatic compounds in the biochar, which are less biodegradable and therefore inhibit or suppress CH4 production. These findings have important implications for the use of biochar in agricultural practices.
Efforts have been made to compare CH4 emissions resulting from biochar application at different applications rates, such as 2.8 t ha−1 annually for 3 years versus a one-time application of 22.5 t ha−1 in the first year (Nan et al., 2020b). The findings indicated decreases in emissions of 43%, 31%, and 30% over three consecutive years in the first case (Nan et al., 2020b). In the second case, the reductions were 52%, 22%, and 14% over three successive years (Nan et al., 2020b). Similarly, an annual low-rate approach resulted in a 41% decrease in CH4 emissions over a 4-year period, while the high single-biochar returning method led to a 38.3% reduction in CH4 emissions over the same period (Nan et al., 2020a). A consistent supply of fresh biochar to the soil stimulated the growth of methanotrophs. Therefore, annual small-scale applications of biochar represent a sustainable and efficacious approach to enhancing soil health as opposed to a single high-dose application.
5.4 Sorption of chemicals and heavy metals
The sorption of chemicals and heavy metals by biochar has won significant attention due to its potential for environmental remediation, wastewater treatment, soil improvement, and more. The type and concentration of functional groups (e.g., carboxyl, carbonyl, and hydroxyl), the surface area, and the pore-size distribution of biochar all affect its ability to adsorb heavy metals. Biochar with a high surface area and a large pore-size distribution is typically more effective at adsorbing heavy metals (Sizmur et al., 2017). Negative charges on the biochar surface formed by the presence of functional groups can attract positively charged heavy metal ions and hold them in place on the biochar surface. In a controlled pot experiment (i.e., artificial contamination of soil with 50 mg kg−1 of cadmium), the application of 5% rice-straw biochar was found to significantly reduce cadmium in soil by 19.3% in contrast with bamboo biochar, which achieved a reduction of 8.6% (Liu et al., 2018).
Biochar and digestate mixtures can also be used as novel sorbents for biopurification systems. In one study, the addition of digestate to biochar resulted in a substantial improvement in pesticide sorption. For bentazone, the sorption coefficient was enhanced by a factor of 55.2 when 5% biochar and 30% digestates were mixed (Mukherjee et al., 2016). This means that the biomixtures were able to sorb more pesticides than could biochar alone. Likewise, biochar loaded with nanoparticles had a maximum adsorption capacity of 147 mg g-1 relative to unmodified biochar, which had a capacity of 67.8 mg g-1 (Wang and Wang, 2018). This is because the nanoparticles increased the surface area and the number of functional groups on the biochar surface. The application of a KOH modification on biochar also resulted in a significant (e.g., 2.4 times) increase in specific surface area, along with a more than 50% enhancement of the adsorption capacity for Cd2+ and Cu2+(Regmi et al., 2012). Unmodified biochar had a specific surface area of 189 m2 g-1, while that of KOH-modified biochar was 455 m2 g-1 (Regmi et al., 2012). Unaltered biochar achieved a Cd2+ adsorption capacity of 4.48 m2 g-1, while KOH-treated biochar had a capacity of 6.81 m2 g-1, a 50.7% increase (Regmi et al., 2012). Similarly, unmodified biochar had a Cu2+ adsorption capacity of 2.64 m2 g-1, while the KOH-modified biochar had a much enhanced capacity of 4.03 m2 g-1 (Regmi et al., 2012). The maximum sorption capacity of atrazine by maize-stalk biochar could be increased from 21.9 to 35.8 mg g−1 by increasing the pyrolysis temperature from 250°C to 850 °C (Tao et al., 2020). This is because, biochar has a larger surface area at higher pyrolysis temperatures, and can increase the number and types of functional groups on the biochar surface. In addition to dosage, pH, and temperature, several other factors can affect the removal of heavy metals by biochar. It was found that the initial removal rate of Cu2+ increased with the dosage of pig-manure biochar up to 5 g L-1 (Meng et al., 2014). However, at 10 g L-1, the removal rate did not change due to saturation of the adsorption sites on the biochar surface and the competitive adsorption of Cu2+ ions with other ions in the solution. The study also found that the removal rate of Cu2+ was the highest at pH 5.0°C and 25 °C (Meng et al., 2014). The half-life value of flubendiamide is 165 days. The half-life decreased to 103 and 117 days when the soil was amended with biochar at 5% and 10%, respectively (Das and Mukherjee, 2020). This is because the biochar can sorb flubendiamide molecules, making them unavailable for biodegradation by microorganisms. The environmental impact of biochar is listed at Table 4.
6 Potential risks
Biochar has attracted significant attention due to its numerous agronomic and environmental benefits. Nevertheless certain risks are associated with its use, such as the possibility of toxicant contamination, heavy metal retention, and lowering of pesticide efficacy (Hussain et al., 2017).
6.1 Potential source of toxicants
Biochar can contain a variety of toxicants, such as heavy metals, PAHs, polychlorinated dibenzodioxins, polychlorinated dibenzofurans, and VOCs. These toxicants can be present in the biomass used to produce biochar or they can form during pyrolysis. The aforementioned hazardous substances are generated through catalytic encounters with dioxin structures comprising O2, carbon, and chlorine at temperatures ranging between 300°C and 325 °C through the post-combustion process (Godlewska et al., 2021). PAHs can also form through direct carbonization and aromatization at temperatures below 500°C, at which the feedstock is converted into carbonaceous materials. At temperatures above 500°C, PAHs form through a free radical pathway, followed by pyrosynthesis of larger aromatic structures (Gelardi et al., 2019). Dioxins are formed by a precursor through a de novo pathway in the presence of both oxygen and solid carbon at temperatures ranging from 200°C to 400 °C (Gelardi et al., 2019). Biochar can increase soil-dust emissions of particle matter <10 μm in size (PM10), which can be inhaled deep into the lungs (Gelardi et al., 2019). The International Biochar Initiative (IBI) set a maximum permissible concentration of 300 mg kg-1 for Σ16 PAHs in biochar (Godlewska et al., 2021). Σ16 PAHs refers to a group of 16 PAHs that are considered the most important from a toxicological perspective. In an investigation of biochars derived from 23 different substrates (at temperatures ranging from 250°C to 900 °C), the concentrations of bioavailable PAHs varied between 0.17 and 10.0 ng L-1 (Zielińska and Oleszczuk, 2016). Biochar derived from pine wood had the lowest bioavailable PAH content (0.17 ng L-1), while biochar derived from food waste had the highest (10.0 ng L-1) (Zielińska and Oleszczuk, 2016). The study also revealed that the bioavailable PAH content in biochar decreased as the pyrolysis temperature increased because higher temperatures break down PAH molecules (Zielińska and Oleszczuk, 2016). In a previous study, certain patterns were observed in the occurrence of VOCs, depending on the temperature range: at 350°C, benzene, toluene, ethylbenzene, and xylene (BTEX) were seen; at 450°C, indenes, benzonitrile, benzofurans, aldehydes were seen; and at 650°C, ketones from corn stalk biochar formed (Ghidotti et al., 2017). Depending on the nature of the biomass and the thermal processing, VOC content in biochar can reach 40% (Saletnik et al., 2019). For example, biochar made from biomass high in VOCs, such as manure or food waste, is more likely to have a higher VOC content compared with biochar made from biomass that is low in VOCs, such as wood (Saletnik et al., 2019). Likewise, biochar produced from various sources, including plant biomass, municipal solid waste, compost, and coal refuse exhibited a range of concentrations for chromium (5.88–69.4), nickel (4.60–33.4), copper (3.96–40.3), zinc (9.61–138), lead (1.71–36), cadmium (0.12–0.17), and arsenic (0.12–1.53) mg kg-1 (Kujawska, 2023). However, these heavy metals did not exceed the guidelines set by the IBI. It was also found that biochar derived from plant biomass held the lowest concentrations of heavy metals, while coal-refuse biochar displayed the highest concentrations (Kujawska, 2023). For example, the recorded concentrations of cadmium, chromium, copper, nickel, lead, and zinc in plant biomass were 0.03, 10.3, 19.7, 10.5, 10.9, and 55.5 mg kg-1, respectively, while those in coal-refuse biochar were 0.25, 16.9, 34.1, 14.3, 15.1, and 71.3 mg kg-1, respectively (Kujawska, 2023).
6.2 Efficacy of pesticides
The porous structure and high surface area of biochar means it can readily adsorb various compounds including pesticides. This property, while potentially beneficial for reducing pesticide leaching, can also sequester pesticides away from their intended target to reduce their efficacy. As biochar can coat the roots of plants, it can prevent the pesticides from reaching plant tissues. Biochar can also slow down the degradation of pesticides to increase the residual life of pesticides in soil. As such, pesticides are more likely to persist in the environment and pose a risk to human health and the environment.
Biochar’s impact on soil pH can potentially alter the chemical properties of pesticides and affect their effectiveness (Alkharabsheh et al., 2021). It was found that adding biochar to soil to a level of 0.5% resulted in 52.3%, 27.4%, and 11.6% increases in the sorption of acetamiprid in red, paddy, and black soils, respectively (Yu et al., 2011). This outcome is likely attributable to the interactive relationships between key variables, such as organic matter, clay content, and pH. It was also found that 58%–68% of the pesticides were lost in control soil, while soil amended with 1.0% biochar lost only 34% of chlorpyrifos and 32% of fipronil (Yang et al., 2010). The results of a meta-analysis of 14 studies showed that biochar can reduce the concentrations of a variety of pesticides, such as chlorpyrifos, fipronil, atrazine, glyphosate, triclopyr, in soil by an average of 81% (with a 95% confidence interval of 75%–88%) (Holanda et al., 2023).
6.3 Ecotoxicological effect on soil organisms
Certain compounds present in biochar, such as PAHs, formaldehyde, cresols, xylenols, acrolein, and other noxious carbonyl compounds (contingent upon pyrolysis conditions), may interrupt bactericidal or fungicidal actions (Ajema, 2018). These toxins can be harmful to microbes in a number of ways. They can disrupt the metabolism of microbes by damaging DNA or even kill the microbe (Ajema, 2018). As a result, the presence of toxins in biochar can inhibit microbial activity and reduce the ability of microbes to degrade organic matter and other pollutants. Acidity or the existence of toxic substances in biochar may render it unsuitable for earthworms. In the laboratory, three soil samples spiked with pentachlorophenol (PCP) and one field-contaminated soil sample amended with 2% biochar were tested (Zhu et al., 2018). The results showed that biochar amendment significantly reduced the bioavailability and bioaccumulation factor of PCP in the soil. Alkaline biochar (made from wood ash or straw) has a high pH that can disrupt earthworm digestion and impede nutrient absorption (Weyers and Spokas, 2011). Such a biochar can elevate the electrical conductivity of soil, impeding earthworm respiration and mobility in the soil (Weyers and Spokas, 2011). As biochar can adsorb pesticides, it can reduce the availability of pesticides to microorganisms for degradation (Holanda et al., 2023). This can result in elevated levels of pesticides in the soil, posing a threat to soil organisms and other forms of life.
7 Future perspectives of biochar
Biochar applications in soil have the potential to promote sustainable agriculture while mitigating climate change. However, there are still some challenges and barriers that need to be addressed to realize this potential. More research is needed to better understand the long-term effects of biochar application on soil properties, crop yields, and environmental quality for optimal biochar type and application rate for different soil types and cropping systems as well as its long-term impacts on soil microbial communities and greenhouse gas emissions. New and improved biochar production technologies should also be developed to reduce energy and production cost from a wider range of feedstocks so as to make biochar more affordable and accessible to farmers.
Policies and programs need to be developed to support the adoption of biochar in agriculture by many types of incentives such as tax breaks for farmers to use biochar and research and development funding for new biochar technologies and their applications. Investigation should also be made to facilitate the use of biochar in combination with other sustainable farming practices such as cover cropping and crop rotation. Moreover, the potential of biochar should be researched to cover from soil resilience to climate change stressors such as drought and flooding. It is also important to develop biochar-based carbon capture and storage (CCS) technologies to help offset greenhouse gas emissions from agriculture and other sectors.
Farmers should consider using biochar as part of a holistic soil management strategy. This could involve combining biochar application with other practices such as crop rotation, cover cropping, and reduced tillage. Farmers should also select the right biochar type and application rate for their specific soil type and cropping system. As there are a variety of biochar products available, it is important to choose one that is well-suited to the individual needs. Farmers should also monitor the effects of biochar application on their soil and crops over time. This will help ensure that they are gaining the most out of their biochar investment to build a knowledge base on the long-term effects of biochar application. In addition, proper training and support should be provided for farmers and other stakeholders to promote the best practices for biochar production and application. Hence, through workshops, field demonstrations, and extension programs, the use of biochar in sustainable agriculture and climate change mitigation programs can increase public awareness of its benefits in various respects.
8 Conclusion
Biochar holds great promise as a medium for soil amendment due to its potential to enhance nutrient retention, improve WHC, and sequester carbon. The numerous positive effects of biochar emphasize its potential to contribute to sustainable agriculture and mitigate climate change. However, the impacts of biochar are highly context-dependent and the outcomes of biochar application can be affected by the combined effects of diverse factors such as biochar feedstock, production methods, application rates, and soil characteristics.
Careful consideration and tailored approaches are necessary to maximize the benefits of biochar application and to minimize potential negative consequences. The majority of studies conducted to date suggest biochar has an overall positive effect on soil properties and crop yields, while a subset of articles highlight the importance of proper application strategies. Excessive application of biochar or the selection of inappropriate feedstocks can lead to unintended consequences, such as nutrient imbalances, altered pH levels, and disruptions to soil microbial communities. To harness the full potential of biochar, future research should focus on refining production techniques, optimizing application protocols for different soil types and crops, and assessing its long-term effects on soil health and ecosystem sustainability. Comprehensive life-cycle assessments are also needed to evaluate the environmental and economic impacts of large-scale biochar implementation.
Author contributions
EK: Writing–original draft, Writing–review and editing. K-HK: Conceptualization, Supervision, Writing–review and editing. EK: Supervision, Visualization, Writing–review and editing.
Funding
The author(s) declare financial support was received for the research, authorship, and/or publication of this article. This work was supported by a grant from the National Research Foundation of Korea funded by the Ministry of Science and ICT of the Korean government (Grant Nos. 2021R1A3B1068304).
Conflict of interest
The authors declare that the research was conducted in the absence of any commercial or financial relationships that could be construed as a potential conflict of interest.
Publisher’s note
All claims expressed in this article are solely those of the authors and do not necessarily represent those of their affiliated organizations, or those of the publisher, the editors and the reviewers. Any product that may be evaluated in this article, or claim that may be made by its manufacturer, is not guaranteed or endorsed by the publisher.
References
Adekiya, A. O., Agbede, T. M., Olayanju, A., Ejue, W. S., Adekanye, T. A., Adenusi, T. T., et al. (2020). Effect of biochar on soil properties, soil loss, and cocoyam yield on a tropical sandy loam Alfisol. Scie World J. 2020, 1–9. doi:10.1155/2020/9391630
Adhikari, S., Timms, W., and Mahmud, M. P. (2022). Optimising water holding capacity and hydrophobicity of biochar for soil amendment–A review. Sci. Total Environ. 851, 158043. doi:10.1016/j.scitotenv.2022.158043
Ajema, L. (2018). Effects of biochar application on beneficial soil organism. Int. J. Res. Stud. Sci. Eng. Technol. 5 (5), 9–18.
Alghamdi, A. G. (2018). Biochar as a potential soil additive for improving soil physical properties—a review. Arab. J. Geosci. 11 (24), 766. doi:10.1007/s12517-018-4056-7
Alkharabsheh, H. M., Seleiman, M. F., Battaglia, M. L., Shami, A., Jalal, R. S., Alhammad, B. A., et al. (2021). Biochar and its broad impacts in soil quality and fertility, nutrient leaching and crop productivity: a review. Agronomy 11 (5), 993. doi:10.3390/agronomy11050993
Allohverdi, T., Mohanty, A. K., Roy, P., and Misra, M. (2021). A review on current status of biochar uses in agriculture. Molecules 26 (18), 5584. doi:10.3390/molecules26185584
Andrenelli, M., Maienza, A., Genesio, L., Miglietta, F., Pellegrini, S., Vaccari, F., et al. (2016). Field application of pelletized biochar: short term effect on the hydrological properties of a silty clay loam soil. Agric. Water Manag. 163, 190–196. doi:10.1016/j.agwat.2015.09.017
Are, K. S. (2019). “Biochar and soil physical health,” in An imperative amendment for soil and the environment. Editors V. Abrol, and P. Sharma (Berlin, Germany: Springer), 21–33.
Arif, M., Ali, S., Ilyas, M., Riaz, M., Akhtar, K., Ali, K., et al. (2021). Enhancing phosphorus availability, soil organic carbon, maize productivity and farm profitability through biochar and organic–inorganic fertilizers in an irrigated maize agroecosystem under semi-arid climate. Soil Use Manag. 37 (1), 104–119. doi:10.1111/sum.12661
Asadi, H., Ghorbani, M., Rezaei-Rashti, M., Abrishamkesh, S., Amirahmadi, E., Chengrong, C., et al. (2021). Application of rice husk biochar for achieving sustainable agriculture and environment. Rice Sci. 28 (4), 325–343. doi:10.1016/j.rsci.2021.05.004
Ayaz, M., Feizienė, D., Tilvikienė, V., Akhtar, K., Stulpinaitė, U., and Iqbal, R. (2021). Biochar role in the sustainability of agriculture and environment. Sustainability 13 (3), 1330. doi:10.3390/su13031330
Blanco-Canqui, H. (2017). Biochar and soil physical properties. Soil Sci. Soc. Am. J. 81 (4), 687–711. doi:10.2136/sssaj2017.01.0017
Bouchelta, C., Medjram, M. S., Zoubida, M., Chekkat, F. A., Ramdane, N., and Bellat, J.-P. (2012). Effects of pyrolysis conditions on the porous structure development of date pits activated carbon. J. Anal. Appl. Pyrolysis 94, 215–222. doi:10.1016/j.jaap.2011.12.014
Brtnicky, M., Datta, R., Holatko, J., Bielska, L., Gusiatin, Z. M., Kucerik, J., et al. (2021). A critical review of the possible adverse effects of biochar in the soil environment. Sci. Total Environ. 796, 148756. doi:10.1016/j.scitotenv.2021.148756
Chathurika, J. S., Kumaragamage, D., Zvomuya, F., Akinremi, O. O., Flaten, D. N., Indraratne, S. P., et al. (2016). Woodchip biochar with or without synthetic fertilizers affects soil properties and available phosphorus in two alkaline, chernozemic soils. Can. J. Soil Sci. 96 (4), 472–484. doi:10.1139/cjss-2015-0094
Chen, D., Chen, X., Sun, J., Zheng, Z., and Fu, K. (2016). Pyrolysis polygeneration of pine nut shell: quality of pyrolysis products and study on the preparation of activated carbon from biochar. Bioresour. Technol. 216, 629–636. doi:10.1016/j.biortech.2016.05.107
Chen, J., Elsgaard, L., Van Groenigen, K. J., Olesen, J. E., Liang, Z., Jiang, Y., et al. (2020). Soil carbon loss with warming: new evidence from carbon-degrading enzymes. Glob. Chang. Biol. 26 (4), 1944–1952. doi:10.1111/gcb.14986
Chen, J., Liu, X., Zheng, J., Zhang, B., Lu, H., Chi, Z., et al. (2013). Biochar soil amendment increased bacterial but decreased fungal gene abundance with shifts in community structure in a slightly acid rice paddy from Southwest China. Appl. Soil Ecol. 71, 33–44. doi:10.1016/j.apsoil.2013.05.003
Clay, S. A., Krack, K. K., Bruggeman, S. A., Papiernik, S., and Schumacher, T. E. (2016). Maize, switchgrass, and ponderosa pine biochar added to soil increased herbicide sorption and decreased herbicide efficacy. J. Environ. Sci. Health-B 51 (8), 497–507. doi:10.1080/03601234.2016.1170540
Collard, F.-X., and Blin, J. (2014). A review on pyrolysis of biomass constituents: mechanisms and composition of the products obtained from the conversion of cellulose, hemicelluloses and lignin. Renew. Sust. Energ Rev. 38, 594–608. doi:10.1016/j.rser.2014.06.013
Das, S. K., and Mukherjee, I. (2020). Low cost biomass derived biochar amendment on persistence and sorption behaviour of flubendiamide in soil. Bull. Environ. Contam. Toxicol. 105, 261–269. doi:10.1007/s00128-020-02936-4
De Freitas, M. I., and Lucas, A. T.Gonzaga MIS 16 (2020). 'Biochar and its impact on soil properties and on the growth of Okra plants. Colloq. Agrar., 1809–8215.
Diatta, A. A., Fike, J. H., Battaglia, M. L., Galbraith, J. M., and Baig, M. B. (2020). Effects of biochar on soil fertility and crop productivity in arid regions: a review. Arab. J. Geosci. 13, 595–617. doi:10.1007/s12517-020-05586-2
Du, Z., Xiao, Y., Qi, X., Liu, Y., Fan, X., and Li, Z. (2018). Peanut-shell biochar and biogas slurry improve soil properties in the North China Plain: a four-year field study. Sci. Rep. 8 (1), 13724. doi:10.1038/s41598-018-31942-0
Duong, V. T., Khanh, N. M., Nguyen, N. T. H., Phi, N. N., Duc, N. T., and Xo, D. H. (2017). Impact of biochar on the water holding capacity and moisture of basalt and grey soil. Ho Chi Minh City Open Uni J. Sci. - Eng. Tech. 7 (1), 36–43.
Edwards, J. D., Pittelkow, C. M., Kent, A. D., and Yang, W. H. (2018). Dynamic biochar effects on soil nitrous oxide emissions and underlying microbial processes during the maize growing season. Soil Biol. Biochem. 122, 81–90. doi:10.1016/j.soilbio.2018.04.008
Egamberdieva, D., Ma, H., Reckling, M., Omari, R. A., Wirth, S., and Bellingrath-Kimura, S. D. (2021). Interactive effects of biochar, nitrogen, and phosphorous on the symbiotic performance, growth, and nutrient uptake of soybean (Glycine max L.). Agronomy 12 (1), 27. doi:10.3390/agronomy12010027
El-Naggar, A., Lee, S. S., Awad, Y. M., Yang, X., Ryu, C., Rizwan, M., et al. (2018). Influence of soil properties and feedstocks on biochar potential for carbon mineralization and improvement of infertile soils. Geoderma 332, 100–108. doi:10.1016/j.geoderma.2018.06.017
Evans, M. R., Jackson, B. E., Popp, M., and Sadaka, S. (2017). Chemical properties of biochar materials manufactured from agricultural products common to the Southeast United States. Horttechnology 27 (1), 16–23. doi:10.21273/horttech03481-16
Figueredo, N. D., Costa, L. M. D., Melo, L. C. A., Siebeneichlerd, E. A., and Tronto, J. (2017). Characterization of biochars from different sources and evaluation of release of nutrients and contaminants. Rev. Cienc. Agron. 48, 483–403. doi:10.5935/1806-6690.20170046
Friedlingstein, P., Jones, M. W., O'sullivan, M., Andrew, R. M., Bakker, D. C., Hauck, J., et al. (2022). Global carbon budget 2021. Earth Sys Sci. Data 14 (4), 1917–2005. doi:10.5194/essd-14-1917-2022
Fungo, B., Guerena, D., Thiongo, M., Lehmann, J., Neufeldt, H., and Kalbitz, K. (2014). N2O and CH4 emission from soil amended with steam-activated biochar. J. Plant Nutr. Soil Sci. 177 (1), 34–38. doi:10.1002/jpln.201300495
Gamage, D. V., Mapa, R., Dharmakeerthi, R., and Biswas, A. (2016). Effect of rice-husk biochar on selected soil properties in tropical Alfisols. Soil Res. 54 (3), 302–310. doi:10.1071/sr15102
Gelardi, D. L., Li, C., and Parikh, S. J. (2019). An emerging environmental concern: biochar-induced dust emissions and their potentially toxic properties. Sci. Total Environ. 678, 813–820. doi:10.1016/j.scitotenv.2019.05.007
Ghidotti, M., Fabbri, D., and Hornung, A. (2017). Profiles of volatile organic compounds in biochar: insights into process conditions and quality assessment. ACS Sustain Chem. Eng. 5 (1), 510–517. doi:10.1021/acssuschemeng.6b01869
Głąb, T., Palmowska, J., Zaleski, T., and Gondek, K. (2015). Effect of biochar application on soil hydrological properties and physical quality of sandy soil. Geoderma 281, 11–20.
Godlewska, P., Ok, Y. S., and Oleszczuk, P. (2021). The dark side of black gold: Ecotoxicological aspects of biochar and biochar-amended soils. J. Hazard Mater 403, 123833. doi:10.1016/j.jhazmat.2020.123833
Gross, A., Bromm, T., and Glaser, B. (2021). Soil organic carbon sequestration after biochar application: a global meta-analysis. Agronomy 11 (12), 2474. doi:10.3390/agronomy11122474
Hagner, M., Kemppainen, R., Jauhiainen, L., Tiilikkala, K., and Setälä, H. (2016). The effects of birch (Betula spp.) biochar and pyrolysis temperature on soil properties and plant growth. Soil Tillage Res. 163, 224–234. doi:10.1016/j.still.2016.06.006
Han, X., Sun, X., Wang, C., Wu, M., Dong, D., Zhong, T., et al. (2016). Mitigating methane emission from paddy soil with rice-straw biochar amendment under projected climate change. Sci. Rep. 6 (1), 24731. doi:10.1038/srep24731
Hoekman, S. K. (2020). Review of nitrous oxide (N2O) emissions from motor vehicles. SAE Int. J. Fuels Lubr. 13 (1), 79–98. doi:10.4271/04-13-01-0005
Holanda, M. A. S., Menezes, J. M. C., Coutinho, H. D. M., and Teixeira, R. N. P. (2023). Effectiveness of biochar as an adsorbent for pesticides: systematic review and meta-analysis. J. Environ. Manage 10. doi:10.16/j.jenvman.2023.118719
Honvault, N., Nobile, C., Faucon, M. P., Firmin, S., and Houben, D. (2022). Direct and indirect interactions between biochar properties, plant belowground traits, and plant performance. GCB Bioener 14 (12), 1254–1265. doi:10.1111/gcbb.12993
Hossain, M. Z., Bahar, M. M., Sarkar, B., Donne, S. W., Ok, Y. S., Palansooriya, K. N., et al. (2020). Biochar and its importance on nutrient dynamics in soil and plant. Biochar 2, 379–420. doi:10.1007/s42773-020-00065-z
Hussain, M., Farooq, M., Nawaz, A., Al-Sadi, A. M., Solaiman, Z. M., Alghamdi, S. S., et al. (2017). Biochar for crop production: potential benefits and risks. J. Soils Sediments 17, 685–716. doi:10.1007/s11368-016-1360-2
Hussain, R., Ghosh, K. K., and Ravi, K. (2021). Impact of biochar produced from hardwood of mesquite on the hydraulic and physical properties of compacted soils for potential application in engineered structures. Geoderma 385, 114836. doi:10.1016/j.geoderma.2020.114836
Igalavithana, A. D., Lee, S.-E., Lee, Y. H., Tsang, D. C., Rinklebe, J., Kwon, E. E., et al. (2017). Heavy metal immobilization and microbial community abundance by vegetable waste and pine cone biochar of agricultural soils. Chemosp 174, 593–603. doi:10.1016/j.chemosphere.2017.01.148
Ji, M., Zhou, L., Zhang, S., Luo, G., and Sang, W. (2020). Effects of biochar on methane emission from paddy soil: focusing on DOM and microbial communities. Sci. Total Environ. 743, 140725. doi:10.1016/j.scitotenv.2020.140725
Jiang, Z.-X., Cui, S., Zhang, X., Xi, M., and Sun, D.-M. (2022). Influence of biochar application on soil nitrate leaching and phosphate retention: a synthetic meta-analysis. Huan Jing ke Xue 43 (10), 4658–4668. doi:10.13227/j.hjkx.202112314
Jing, Y., Zhang, Y., Han, I., Wang, P., Mei, Q., and Huang, Y. (2020). Effects of different straw biochars on soil organic carbon, nitrogen, available phosphorus, and enzyme activity in paddy soil. Sci. Rep. 10 (1), 8837. doi:10.1038/s41598-020-65796-2
Kalu, S., Kulmala, L., Zrim, J., Peltokangas, K., Tammeorg, P., Rasa, K., et al. (2022). Potential of biochar to reduce greenhouse gas emissions and increase nitrogen use efficiency in boreal arable soils in the long-term. Front. Environ. Sci. 10, 914766. doi:10.3389/fenvs.2022.914766
Karimi, A., Moezzi, A., Chorom, M., and Enayatizamir, N. (2020). Application of biochar changed the status of nutrients and biological activity in a calcareous soil. J. Soil Sci. Plant Nutr. 20, 20450–20459. doi:10.1007/s42729-019-00129-5
Kaur, N., Kieffer, C., Ren, W., and Hui, D. (2023). How much is soil nitrous oxide emission reduced with biochar application? An evaluation of meta-analyses. GCB Bioenergy 15 (1), 24–37. doi:10.1111/gcbb.13003
Kavitha, B., Reddy, P. V. L., Kim, B., Lee, S. S., Pandey, S. K., and Kim, K.-H. (2018). Benefits and limitations of biochar amendment in agricultural soils: a review. J. Environ. Manage 227, 146–154. doi:10.1016/j.jenvman.2018.08.082
Khawkomol, S., Neamchan, R., Thongsamer, T., Vinitnantharat, S., Panpradit, B., Sohsalam, P., et al. (2021). Potential of biochar derived from agricultural residues for sustainable management. Sustainability 13 (15), 8147. doi:10.3390/su13158147
Kujawska, J. (2023). Content of heavy metals in various biochar and assessment environmental risk. J. Ecol. Eng. 24 (8), 287–295. doi:10.12911/22998993/166557
Laird, D. A., Fleming, P., Davis, D. D., Horton, R., Wang, B., and Karlen, D. L. (2010). Impact of biochar amendments on the quality of a typical Midwestern agricultural soil. Geoderma 158 (3-4), 443–449. doi:10.1016/j.geoderma.2010.05.013
Lal, R., Smith, P., Jungkunst, H. F., Mitsch, W. J., Lehmann, J., Nair, P. R., et al. (2018). The carbon sequestration potential of terrestrial ecosystems. J. Soils Water Conserv. 73 (6), 145A–152A. doi:10.2489/jswc.73.6.145a
Lee, J.-M., Jeong, H.-C., Gwon, H.-S., Lee, H.-S., Park, H.-R., Kim, G.-S., et al. (2023). Effects of biochar on methane emissions and crop yields in East Asian paddy fields: a regional scale meta-analysis. Sustainability 15 (12), 9200. doi:10.3390/su15129200
Leng, L., Xiong, Q., Yang, L., Li, H., Zhou, Y., Zhang, W., et al. (2021). An overview on engineering the surface area and porosity of biochar. Sci. Total Environ. 763, 144204. doi:10.1016/j.scitotenv.2020.144204
Li, L., Zhang, Y.-J., Novak, A., Yang, Y., and Wang, J. (2021). Role of biochar in improving sandy soil water retention and resilience to drought. Water 13 (4), 407. doi:10.3390/w13040407
Li, X., Wang, T., Chang, S. X., Jiang, X., and Song, Y. (2020). Biochar increases soil microbial biomass but has variable effects on microbial diversity: a meta-analysis. Sci. Total Environ. 749, 141593. doi:10.1016/j.scitotenv.2020.141593
Liu, D., Sun, W., Kong, Y., and Zhang, S. (2023a). Effect of dry and Wet cycles on the Strength characteristics of biochar–clay mixture. Processes 11 (3), 970. doi:10.3390/pr11030970
Liu, D., Zhang, W., Lin, H., Li, Y., Lu, H., and Wang, Y. (2016). A green technology for the preparation of high capacitance rice husk-based activated carbon. J. Clean. Prod. 112, 1190–1198. doi:10.1016/j.jclepro.2015.07.005
Liu, L., Shen, G., Sun, M., Cao, X., Shang, G., and Chen, P. (2014). Effect of biochar on nitrous oxide emission and its potential mechanisms. J. Air Waste Manag. Assoc. 64 (8), 894–902. doi:10.1080/10962247.2014.899937
Liu, Y., Wang, Y., Lu, H., Lonappan, L., Brar, S. K., He, L., et al. (2018). Biochar application as a soil amendment for decreasing cadmium availability in soil and accumulation in Brassica chinensis. J. Soils Sediments 18, 2511–2519. doi:10.1007/s11368-018-1927-1
Liu, Z., Dugan, B., Masiello, C. A., and Gonnermann, H. M. (2017). Biochar particle size, shape, and porosity act together to influence soil water properties. Plos one 12 (6), e0179079. doi:10.1371/journal.pone.0179079
Liu, Z., Jia, M., Li, Q., Lu, S., Zhou, D., Feng, L., et al. (2023b). Comparative analysis of the properties of biochars produced from different pecan feedstocks and pyrolysis temperatures. Ind. Crops Prod. 197, 116638. doi:10.1016/j.indcrop.2023.116638
Lu, Y., Gu, K., Shen, Z., Tang, C.-S., Shi, B., and Zhou, Q. (2023). Biochar implications for the engineering properties of soils: a review. Sci. Total Environ. 888, 164185. doi:10.1016/j.scitotenv.2023.164185
Luo, L., Xu, C., Chen, Z., and Zhang, S. (2015). Properties of biomass-derived biochars: combined effects of operating conditions and biomass types. Bioresour. Technol. 192, 83–89. doi:10.1016/j.biortech.2015.05.054
Lv, Y., Xu, L., Guo, X., Liu, J., Zou, B., Guo, Y., et al. (2022). Effect of biochar on soil physiochemical properties and bacterial diversity in dry direct-seeded rice paddy fields. Agronomy 13 (1), 4. doi:10.3390/agronomy13010004
Mahato, M., Nam, S., Tabassian, R., Oh, S., Nguyen, V. H., and Oh, I. K. (2022). Electronically conjugated multifunctional covalent triazine Framework for unprecedented CO2 selectivity and high-power flexible supercapacitor. Adv. Funct. Mater 32 (5), 2107442. doi:10.1002/adfm.202107442
Majumder, S., Neogi, S., Dutta, T., Powel, M. A., and Banik, P. (2019). The impact of biochar on soil carbon sequestration: meta-analytical approach to evaluating environmental and economic advantages. J. Environ. Manage. 250, 109466. doi:10.1016/j.jenvman.2019.109466
Manso, E. F., Nartey, E., Adjadeh, T., Darko, D., Lawson, I., and Amoatey, C. (2019). Use of corn cob and rice husk biochar as liming materials in acid soils. West Afr. J. Appl. Ecol. 27 (2), 32–50.
Martinsen, V., Alling, V., Nurida, N., Mulder, J., Hale, S., Ritz, C., et al. (2015). pH effects of the addition of three biochars to acidic Indonesian mineral soils. Soil Sci. Plant Nutr. 61 (5), 821–834. doi:10.1080/00380768.2015.1052985
Mclennon, E., Solomon, J. K., Neupane, D., and Davison, J. (2020). Biochar and nitrogen application rates effect on phosphorus removal from a mixed grass sward irrigated with reclaimed wastewater. Sci. Total Environ. 715, 137012. doi:10.1016/j.scitotenv.2020.137012
Meng, J., Feng, X., Dai, Z., Liu, X., Wu, J., and Xu, J. (2014). Adsorption characteristics of Cu (II) from aqueous solution onto biochar derived from swine manure. Environ. Sci. Pollut. Res. 21, 7035–7046. doi:10.1007/s11356-014-2627-z
Mukherjee, S., Weihermüller, L., Tappe, W., Hofmann, D., Köppchen, S., Laabs, V., et al. (2016). Sorption–desorption behaviour of bentazone, boscalid and pyrimethanil in biochar and digestate based soil mixtures for biopurification systems. Sci. Total Environ. 559, 63–73. doi:10.1016/j.scitotenv.2016.03.145
Munera-Echeverri, J. L., Martinsen, V., Strand, L. T., Zivanovic, V., Cornelissen, G., and Mulder, J. (2018). Cation exchange capacity of biochar: an urgent method modification. Sci. Total Environ. 642, 190–197. doi:10.1016/j.scitotenv.2018.06.017
Nan, Q., Wang, C., Wang, H., Yi, Q., and Wu, W. (2020a). Mitigating methane emission via annual biochar amendment pyrolyzed with rice straw from the same paddy field. Sci. Total Environ. 746, 141351. doi:10.1016/j.scitotenv.2020.141351
Nan, Q., Yi, Q., Zhang, L., Ping, F., Thies, J. E., Wu, W., et al. (2020b). Biochar amendment pyrolysed with rice straw increases rice production and mitigates methane emission over successive three years. Waste Manag. 118, 1–8. doi:10.1016/j.wasman.2020.08.013
Ndede, E. O., Kurebito, S., Idowu, O., Tokunari, T., and Jindo, K. (2022). The potential of biochar to enhance the water retention properties of sandy agricultural soils. Agronomy 12 (2), 311. doi:10.3390/agronomy12020311
Ndor, E., Jayeoba, O., and Asadu, C. (2015). Effect of biochar soil amendment on soil properties and yield of sesame varieties in Lafia, Nigeria. Am. J. Exp. Agric. 9 (4), 1–8. doi:10.9734/ajea/2015/19637
Niu, Y., Chen, Z., Müller, C., Zaman, M. M., Kim, D., Yu, H., et al. (2017). Yield-scaled N2O emissions were effectively reduced by biochar amendment of sandy loam soil under maize-wheat rotation in the North China Plain. Atmos. Environ. 170, 58–70. doi:10.1016/j.atmosenv.2017.09.050
Omondi, M. O., Xia, X., Nahayo, A., Liu, X., Korai, P. K., and Pan, G. (2016). Quantification of biochar effects on soil hydrological properties using meta-analysis of literature data. Geoderma 274, 28–34. doi:10.1016/j.geoderma.2016.03.029
Pokharel, P., Ma, Z., and Chang, S. X. (2020). Biochar increases soil microbial biomass with changes in extra-and intracellular enzyme activities: a global meta-analysis. Biochar 2, 65–79. doi:10.1007/s42773-020-00039-1
Prasad, M., Chrysargyris, A., Mcdaniel, N., Kavanagh, A., Gruda, N. S., and Tzortzakis, N. (2019). Plant nutrient availability and pH of biochars and their fractions, with the possible use as a component in a growing media. Agronomy 10 (1), 10. doi:10.3390/agronomy10010010
Pratiwi, E. P. A., Hillary, A. K., Fukuda, T., and Shinogi, Y. (2016). The effects of rice husk char on ammonium, nitrate and phosphate retention and leaching in loamy soil. Geoderma 27, 761–768. doi:10.1016/j.geoderma.2016.05.006
Prendergast-Miller, M. T., Duvall, M., and Sohi, S. P. (2014). Biochar–root interactions are mediated by biochar nutrient content and impacts on soil nutrient availability. Eur. J. Soil Sci. 65, 173–185.
Purakayastha, T., Bera, T., Bhaduri, D., Sarkar, B., Mandal, S., Wade, P., et al. (2019). A review on biochar modulated soil condition improvements and nutrient dynamics concerning crop yields: Pathways to climate change mitigation and global food security. Chemosphere 227, 345–365. doi:10.1016/j.chemosphere.2019.03.170
Qin, Y., Xi, B., Sun, X., Zhang, H., Xue, C., and Wu, B. (2022). Methane emission reduction and biological characteristics of landfill cover soil amended with hydrophobic biochar. Front. Bioeng. Biotechnol. 10, 905466. doi:10.3389/fbioe.2022.905466
Randolph, P., Bansode, R., Hassan, O., Rehrah, D., Ravella, R., Reddy, M., et al. (2017). Effect of biochars produced from solid organic municipal waste on soil quality parameters. J. Environ. Manage 192, 271–280. doi:10.1016/j.jenvman.2017.01.061
Rawat, J., Saxena, J., and Sanwal, P. (2019). “Biochar: a sustainable approach for improving plant growth and soil properties,” in Biochar-an imperative amendment for soil and the environment (London, UK: Intechopen), 1–17. doi:10.5772/intechopen.82151
Razzaghi, F., Obour, P. B., and Arthur, E. (2020). Does biochar improve soil water retention? A systematic review and meta-analysis. Geoderma 361, 114055. doi:10.1016/j.geoderma.2019.114055
Regmi, P., Moscoso, J. L. G., Kumar, S., Cao, X., Mao, J., and Schafran, G. (2012). Removal of copper and cadmium from aqueous solution using switchgrass biochar produced via hydrothermal carbonization process. J. Environ. Manage 109, 61–69. doi:10.1016/j.jenvman.2012.04.047
Rehman, A., Nawaz, S., Alghamdi, H. A., Alrumman, S., Yan, W., and Nawaz, M. Z. (2020). Effects of manure-based biochar on uptake of nutrients and water holding capacity of different types of soils. Case Stud. Chem. Environ. Eng. 2, 100036. doi:10.1016/j.cscee.2020.100036
Rittl, T. F., Oliveira, D. M., Canisares, L. P., Sagrilo, E., Butterbach-Bahl, K., Dannenmann, M., et al. (2021). High application rates of biochar to mitigate N2O emissions from a N-fertilized tropical soil under warming conditions. Front. Environ. Sci. 8. doi:10.3389/fenvs.2020.611873
Rubin, R. L., Anderson, T. R., and Ballantine, K. A. (2020). Biochar simultaneously reduces nutrient leaching and greenhouse gas emissions in restored wetland soils. Wetlands 40, 1981–1991. doi:10.1007/s13157-020-01380-8
Sahin, O., Taskin, M., Kaya, E., Atakol, O., Emir, E., Inal, A., et al. (2017). Effect of acid modification of biochar on nutrient availability and maize growth in a calcareous soil. Soil Use Manag. 33 (3), 447–456. doi:10.1111/sum.12360
Saletnik, B., Zaguła, G., Bajcar, M., Tarapatskyy, M., Bobula, G., and Puchalski, C. (2019). Biochar as a multifunctional component of the environment—a review. App Sci. 9 (6), 1139. doi:10.3390/app9061139
Sandhu, S. S., Ussiri, D. A., Kumar, S., Chintala, R., Papiernik, S. K., Malo, D. D., et al. (2017). Analyzing the impacts of three types of biochar on soil carbon fractions and physiochemical properties in a corn-soybean rotation. Chemosphere 184, 473–481. doi:10.1016/j.chemosphere.2017.05.165
Shakoor, A., Shahzad, S. M., Chatterjee, N., Arif, M. S., Farooq, T. H., Altaf, M. M., et al. (2021). Nitrous oxide emission from agricultural soils: application of animal manure or biochar? A global meta-analysis. J. Environ. Manage 285, 112170. doi:10.1016/j.jenvman.2021.112170
Shreya, D., Samanyita, M., Gayatri, S., Mausami, R., and Kiran, P. (2021). “Biochar: a sustainable approach for improving soil health and environment,” in Soil erosion - current challenges and future perspectives in a changing World (London, UK: Intechopen). doi:10.5772/intechopen.97136
Sizmur, T., Fresno, T., Akgül, G., Frost, H., and Moreno-Jiménez, E. (2017). Biochar modification to enhance sorption of inorganics from water. Bioresour. Technol. 246, 34–47. doi:10.1016/j.biortech.2017.07.082
Sun, H., Lu, H., Chu, L., Shao, H., and Shi, W. (2017). Biochar applied with appropriate rates can reduce N leaching, keep N retention and not increase NH3 volatilization in a coastal saline soil. Sci. Total Environ. 575, 820–825. doi:10.1016/j.scitotenv.2016.09.137
Sun, K., Kang, M., Zhang, Z., Jin, J., Wang, Z., Pan, Z., et al. (2013). Impact of deashing treatment on biochar structural properties and potential sorption mechanisms of phenanthrene. Environ. Sci. Technol. 47 (20), 11473–11481. doi:10.1021/es4026744
Tao, Y., Han, S., Zhang, Q., Yang, Y., Shi, H., Akindolie, M. S., et al. (2020). Application of biochar with functional microorganisms for enhanced atrazine removal and phosphorus utilization. J. Clean. Prod. 257, 120535. doi:10.1016/j.jclepro.2020.120535
Tian, S., Tan, Z., Kasiulienė, A., and Ai, P. (2017). Transformation mechanism of nutrient elements in the process of biochar preparation for returning biochar to soil. Chin. J. Chem. Eng. 25 (4), 477–486. doi:10.1016/j.cjche.2016.09.009
Tomczyk, A., Sokołowska, Z., and Boguta, P. (2020). Biochar physicochemical properties: pyrolysis temperature and feedstock kind effects. Rev. Environ. Sci. Bio/Technol 19, 191–215. doi:10.1007/s11157-020-09523-3
Wang, C., and Wang, H. (2018). Pb (II) sorption from aqueous solution by novel biochar loaded with nano-particles. Chemosphere 192, 1–4. doi:10.1016/j.chemosphere.2017.10.125
Wang, D., Jiang, P., Zhang, H., and Yuan, W. (2020). Biochar production and applications in agro and forestry systems: a review. Sci. Total Environ. 723, 137775. doi:10.1016/j.scitotenv.2020.137775
Weng, Z. H., Liu, X., Eldridge, S., Wang, H., Rose, T., Rose, M., et al. (2020). Priming of soil organic carbon induced by sugarcane residues and its biochar control the source of nitrogen for plant uptake: a dual 13C and 15N isotope three-source-partitioning study. Soil Biol. Biochem. 146, 107792. doi:10.1016/j.soilbio.2020.107792
Weyers, S. L., and Spokas, K. A. (2011). Impact of biochar on earthworm populations: a review. App Environ. Soil Sci. 1687, 1–12. doi:10.1155/2011/541592
Wu, Z., Song, Y., Shen, H., Jiang, X., Li, B., and Xiong, Z. (2019). Biochar can mitigate methane emissions by improving methanotrophs for prolonged period in fertilized paddy soils. Environ. Poll. 253, 1038–1046. doi:10.1016/j.envpol.2019.07.073
Wyn, H. K., Zárate, S., Carrascal, J., and Yermán, L. (2020). A novel approach to the production of biochar with improved fuel characteristics from biomass waste. Waste Biomass Valorization 11, 6467–6481. doi:10.1007/s12649-019-00909-1
Xu, N., Tan, G., Wang, H., and Gai, X. (2016). Effect of biochar additions to soil on nitrogen leaching, microbial biomass and bacterial community structure. Eur. J. Soil Biol. 74, 1–8. doi:10.1016/j.ejsobi.2016.02.004
Yadav, R., Yadav, M., Kumar, R., Parihar, C., Yadav, N., Bajiya, R., et al. (2017). Role of biochar in mitigation of climate change through carbon sequestration. Int. J. Curr. Microbiol. App Sci. 6 (4), 859–866. doi:10.20546/ijcmas.2017.604.107
Yaghoubi, P., Yargicoglu, E. N., and Reddy, K. R. (2014). “Effects of biochar-amendment to landfill cover soil on microbial methane oxidation: initial results,” in Proceedings of the 2014: Geo-characterization and Modeling for Sustainability 1849-1858, February 2014 (United States: Geo-Congress). doi:10.1061/9780784413272.181
Yang, C., Liu, J., and Lu, S. (2021). Pyrolysis temperature affects pore characteristics of rice straw and canola stalk biochars and biochar-amended soils. Geoderma 397, 115097. doi:10.1016/j.geoderma.2021.115097
Yang, X., Sun, Q., Yuan, J., Fu, S., Lan, Y., Jiang, X., et al. (2022a). Successive corn stover and biochar applications mitigate N2O emissions by altering soil physicochemical properties and N-cycling-related enzyme activities: a five-year field study in Northeast China. Agric. Ecosyst. Environ. 340, 108183. doi:10.1016/j.agee.2022.108183
Yang, X.-B., Ying, G.-G., Peng, P.-A., Wang, L., Zhao, J.-L., Zhang, L.-J., et al. (2010). Influence of biochars on plant uptake and dissipation of two pesticides in an agricultural soil. J. Agric. Food Chem. 58 (13), 7915–7921. doi:10.1021/jf1011352
Yang, Z., She, R., Hu, L., Yu, Y., and Yao, H. (2022b). Effects of biochar addition on nitrous oxide emission during soil freeze–thaw cycles. Front. Microbiol. 13, 1033210. doi:10.3389/fmicb.2022.1033210
Yargicoglu, E. N., and Reddy, K. R. (2017). Microbial abundance and activity in biochar-amended landfill cover soils: evidence from large-scale column and field experiments. J. Environ. Eng. 143 (9), 04017058. doi:10.1061/(ASCE)EE.1943-7870.0001254
Yin, J., Zhao, L., Xu, X., Li, D., Qiu, H., and Cao, X. (2022). Evaluation of long-term carbon sequestration of biochar in soil with biogeochemical field model. Sci. Total Environ. 822, 153576. doi:10.1016/j.scitotenv.2022.153576
Yrjälä, K., Ramakrishnan, M., and Salo, E. (2022). Agricultural waste streams as resource in circular economy for biochar production towards carbon neutrality. Curr. Opin. Environ. Sci. Health 26, 100339. doi:10.1016/j.coesh.2022.100339
Yu, O.-Y., Raichle, B., and Sink, S. (2013). Impact of biochar on the water holding capacity of loamy sand soil. Int. J. Energy Environ. Eng. 4, 44–49. doi:10.1186/2251-6832-4-44
Yu, X.-Y., Mu, C.-L., Gu, C., Liu, C., and Liu, X.-J. (2011). Impact of woodchip biochar amendment on the sorption and dissipation of pesticide acetamiprid in agricultural soils. Chemosphere 85 (8), 1284–1289. doi:10.1016/j.chemosphere.2011.07.031
Zhang, C., Lin, Y., Tian, X., Xu, Q., Chen, Z., and Lin, W. (2017a). Tobacco bacterial wilt suppression with biochar soil addition associates to improved soil physiochemical properties and increased rhizosphere bacteria abundance. App Soil Ecol. 112, 90–96. doi:10.1016/j.apsoil.2016.12.005
Zhang, Y., Liu, S., Zheng, X., Wang, X., Xu, Y., Tang, H., et al. (2017b). Biomass organs control the porosity of their pyrolyzed carbon. Adv. Funct. Mater 27 (3), 1604687. doi:10.1002/adfm.201604687
Zhang, Y., Zhang, Z., and Chen, Y. (2021). Biochar mitigates N2O emission of microbial denitrification through modulating carbon metabolism and allocation of reducing power. Environ. Sci. Technol. 55 (12), 8068–8078. doi:10.1021/acs.est.1c01976
Zhao, B., O'connor, D., Zhang, J., Peng, T., Shen, Z., Tsang, D. C., et al. (2018). Effect of pyrolysis temperature, heating rate, and residence time on rapeseed stem derived biochar. J. Clean. Prod. 174, 977–987. doi:10.1016/j.jclepro.2017.11.013
Zhao, J., Shen, X.-J., Domene, X., Alcañiz, J.-M., Liao, X., and Palet, C. (2019). Comparison of biochars derived from different types of feedstock and their potential for heavy metal removal in multiple-metal solutions. Sci. Rep. 9 (1), 9869. doi:10.1038/s41598-019-46234-4
Zhao, L., Cao, X., Mašek, O., and Zimmerman, A. (2013). Heterogeneity of biochar properties as a function of feedstock sources and production temperatures. J. Hazard Mater 256, 1–9. doi:10.1016/j.jhazmat.2013.04.015
Zheng, H., Liu, D., Liao, X., Miao, Y., Li, Y., Li, J., et al. (2022). Field-aged biochar enhances soil organic carbon by increasing recalcitrant organic carbon fractions and making microbial communities more conducive to carbon sequestration. Agric. Ecosyst. Environ. 340, 108177. doi:10.1016/j.agee.2022.108177
Zhu, M., Zhang, L., Zheng, L., Zhuo, Y., Xu, J., and He, Y. (2018). Typical soil redox processes in pentachlorophenol polluted soil following biochar addition. Front. Microbiol. 9, 579. doi:10.3389/fmicb.2018.00579
Zielińska, A., and Oleszczuk, P. (2016). Effect of pyrolysis temperatures on freely dissolved polycyclic aromatic hydrocarbon (PAH) concentrations in sewage sludge-derived biochars. Chemosphere 153, 68–74. doi:10.1016/j.chemosphere.2016.02.118
Keywords: biochar, sustainable agriculture, environmental remediation, soil fertility, carbon sequestration, water filtration
Citation: Kabir E, Kim K-H and Kwon EE (2023) Biochar as a tool for the improvement of soil and environment. Front. Environ. Sci. 11:1324533. doi: 10.3389/fenvs.2023.1324533
Received: 19 October 2023; Accepted: 06 December 2023;
Published: 18 December 2023.
Edited by:
Yaqin Wang, University of Helsinki, FinlandReviewed by:
Claudio Ciavatta, University of Bologna, ItalyRakesh Kumar, Auburn University, United States
Jaya Nepal, University of Florida, United States
Copyright © 2023 Kabir, Kim and Kwon. This is an open-access article distributed under the terms of the Creative Commons Attribution License (CC BY). The use, distribution or reproduction in other forums is permitted, provided the original author(s) and the copyright owner(s) are credited and that the original publication in this journal is cited, in accordance with accepted academic practice. No use, distribution or reproduction is permitted which does not comply with these terms.
*Correspondence: Ki-Hyun Kim, a2tpbTYxQGhhbnlhbmcuYWMua3I=; Eilhann E. Kwon, ZWsyMTQ4QGhhbnlhbmcuYWMua3I=