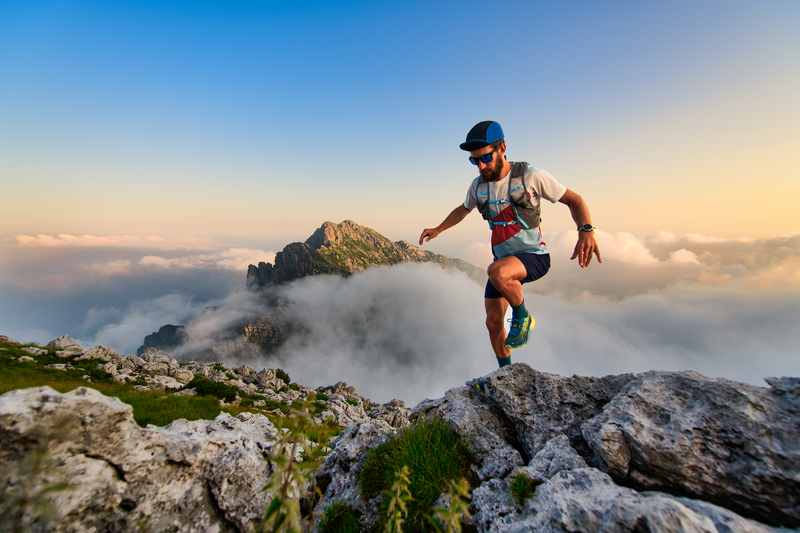
94% of researchers rate our articles as excellent or good
Learn more about the work of our research integrity team to safeguard the quality of each article we publish.
Find out more
ORIGINAL RESEARCH article
Front. Environ. Sci. , 22 December 2023
Sec. Soil Processes
Volume 11 - 2023 | https://doi.org/10.3389/fenvs.2023.1309169
This article is part of the Research Topic Advanced Geochemical Mapping and Geochemical Background/Baseline: An Environmental Perspective View all 5 articles
Lead in yard soils has been recognized as the principal source of excess lead absorption among young children. The hazard imposed by soil lead is dependent on the geochemical forms of lead in soils. Soil properties such as pH, soil organic matter, clay, and carbonate content influence the geochemical forms of lead in soil. This study was conducted to investigate the correlation between soil properties and the geochemical speciation of lead in lead paint-contaminated residential soils from three major US cities. A comprehensive field survey was conducted, involving the collection of soils from ten houses in each of the cities: Baltimore, San Antonio, and Detroit. The influence of soil properties on geochemical speciation was analyzed to identify effective immobilization amendments for each soil type. Results showed that soils collected from San Antonio were slightly alkaline, whereas those from Baltimore were slightly acidic. Soils collected from Detroit were neutral to mildly alkaline in pH. San Antonio soils had relatively high soil salinity, high clay content, moderate to high soil organic matter (SOM), and high total carbon (TC). In contrast, soils collected from Baltimore had lower salinity and clay content, low SOM, and total carbon. Soils from Detroit exhibited relatively high salinity, clay, SOM, and TC contents. The average total soil lead concentrations were as follows; San Antonio 4,073 mg/kg, Baltimore 2,706 mg/kg, and Detroit 850 mg/kg. Geochemical speciation studies revealed significant differences in lead distribution among the studied soils. San Antonio soils exhibited high carbonate-bound and organic matter-bound fractions, while Baltimore soils had elevated soluble + exchangeable fractions. Detroit soils showed substantial lead in organic matter-bound fractions. Correlation analysis showed that the soil properties influencing exchangeable lead, were pH, total Al, and total Ca for San Antonio soils; pH and total P for Baltimore soils; and SOM and total Al for Detroit soils. Correlation analysis showed that there is a significant negative correlation (p < 0.05) between exchangeable lead and total Al (r = −0.653), and total Ca (r = −0.438) for San Antonio soils; pH (r = −0.286) and total p (r = −0.314) for Baltimore soils; and SOM (r = −0.628) and total Al (r = −0.408) for Detroit soils. Based on these results, the best potential immobilization amendments for each of these cities were predicted.
Several studies have documented the harmful health effects of exposure to lead. In residential areas, one of the primary sources of lead absorption in young children is lead present in soils, originating mainly from deteriorating lead-based paints in homes constructed before the 1978 ban on such paints by the US Consumer Product Safety Commission (Clark and Knudsen, 2013). Before 1955, white paint contained up to 50% lead. Federal law lowered the amount of lead allowable in paint to 1% in 1971. In 1977, the Consumer Products Safety Commission limited lead in most paints to 0.06%. Since 2009, the lead allowable in most paints is 0.009%. (Clark et al., 1991; LeBlanc et al., 2022). The deterioration of lead paint from inside and outside these older homes can result in dangerously high concentrations of lead in house dust and the soil.
Lead in peeling paint chips, soil, and dust pose a risk to human health, especially to children (Jacobs et al., 2002). Lead contamination from lead-based paint can result in soil lead concentrations much greater than the US Environmental Protection Agency (USEPA) lead hazard cutoff value of 400 mg/kg (USEPA, 2001). Urban children are at risk of increased blood lead levels (BLL) due to contaminated soils in their backyards (Laidlaw and Filippelli, 2008; Burki, 2020). Many studies reported that lead concentrations in urban residential soil are highly correlated with elevated BLL in children (Canfield et al., 2003; Brown and Margolis, 2012; Egan et al., 2021). Despite the efforts made by the government to reduce residential exposure to lead, there exists a significant number of houses in every city in the U.S. that were built before the 1980s, that contain lead-based paint. For example, in San Antonio, TX, 52% of all owner-occupied housing units and 49% of all renter-occupied units were built before 1980, which translates to 141,426 owner units and 97,843 renter units (Saminathan et al., 2010). Several studies have reported that remediation of yard soil in lead paint-contaminated homes results in a substantial lowering of (BLL) in children (Laidlaw et al., 2017).
Traditional soil lead cleanup is achieved by soil removal for off-site disposal. Excavation of residential yards is disruptive to the residents and the neighborhood, and also very expensive. Hence, in situ lead immobilization techniques are considered more practical. These techniques aim to reduce the mobility and bioavailability of lead in contaminated soils, thereby minimizing its adverse health effects at a low cost. Immobilization can be achieved using inexpensive amendments that could be organic (e.g., biochar, compost), inorganic (e.g., phosphate-based compounds, lime), or soil stabilizers (e.g., iron oxides, clay minerals) (Bardy et al., 2003; Martin et al., 2008; Mahar, et al., 2015; Gomes et al., 2022). Adding phosphorus (p) to lead-contaminated soils from various sources has become a widespread practice to precipitate lead as stable compounds with low solubility. However, there is an ecological risk associated with this process. Although lead-phosphates (e.g., pyromorphites) are typically stable, some of the exchangeable p species can migrate out of the soil and cause eutrophication of surrounding water bodies (Guo et al., 2006). More sustainable amendments are being studied to immobilize lead in lead paint-contaminated soils.
The success of such amendments in mitigating lead mobility and bioavailability is governed by multiple factors, such as the extent of lead contamination, soil physicochemical characteristics, and geochemical speciation of lead in soil. Determining the total lead concentration alone is insufficient to fully assess lead availability, as soil properties play a crucial role in determining the mobility and potential health effects of lead (Manceau et al., 1996). Lead speciation, solubility, adsorption-desorption processes, availability for uptake by plants, and human bioavailability are influenced by soil properties, such as pH, organic matter content, cation exchange capacity, clay mineralogy, and redox potential (Saminathan et al., 2010). The determination of the concentrations of various geochemical forms of lead is thus central to risk assessment.
Despite its significance, there have been fewer studies characterizing geochemical lead speciation in residential soils (McBride et al., 1997; Andra et al., 2006; Zhang et al., 2022). Identifying the key soil properties that influence lead bioavailability can help design targeted soil management approaches for specific contaminated sites, ensuring the effective immobilization and long-term stability of lead in the soil matrix. Thus, a thorough understanding of the relationship between soil properties and the mobility of lead is crucial for the selection of effective soil management and remediation strategies. By elucidating these relationships, the most suitable soil immobilization amendments can be identified, and site-specific, targeted approaches for mitigating the environmental and health risks associated with lead-contaminated residential soils can be developed.
The objectives of the present study were to 1) Evaluate the concentrations and the geochemical speciation of lead in contaminated residential soils from Baltimore, Maryland; San Antonio, Texas; and Detroit, Michigan, in relation to their varying physico-chemical properties, and 2) Investigate the relationships between different soil properties and lead mobility and soluble and exchangeable lead fractionsin these contaminated soils. The results can guide the development of targeted remediation strategies in each city and facilitate the development of area-specific soil management techniques. Furthermore, the results from the present study can serve as a foundation for further research to explore the long-term effectiveness of soil amendments in lead immobilization, offering sustainable solutions for lead-contaminated soils in residential areas.
Ten houses each in the cities of Baltimore, San Antonio, and Detroit were selected, and a detailed grid survey of lead concentrations was conducted using a handheld portable X-Ray Fluorescence Analyzer (pXRF, Thermo Fisher Scientific, Niton XL3t) in 4 ft2 grids. Based on the pXRF analysis, at each housing unit, soil samples were collected from lead hotspots. These samples were collected at a depth of 0–15 cm from five distinct locations within each housing unit. Subsequently, a composite sample was generated by homogenizing these five individual samples. In the laboratory, soil samples were air-dried and passed through a 2 mm sieve before characterization.
The soils were characterized for texture, pH, salinity (EC), Cation Exchange Capacity (CEC), total lead, iron (Fe), aluminum (Al), calcium (Ca), magnesium (Mg), and P, Soil organic matter (SOM), and total carbon (TC). The standard protocols outlined in the Soil Science Society of America Handbook for Chemical and Mineralogical Analysis described in Sparks et al. (2020) were followed for soil characterization. Soil Organic Matter in samples was determined using the loss on ignition (LOI) method described in Schulte and Hopkins (1996). Total elemental concentrations were determined by Inductively Coupled Plasma-Optical Emission Spectroscopy (ICP-OES, Agilent Technologies 5100). Total metals were determined after acid digestion with HNO3 and H2O2 following USEPA Method 3050B (USEPA, 1996a). Lead concentrations obtained using pXRF were compared with those obtained using ICP-OES to assess pXRF reliability. All extractions and analyses were done in triplicate. The Quality Assurance/Quality Control protocols in Soil Sampling Quality Assurance User’s Guide (U.S. EPA, 2023; Barth et al., 1989) and Acid Digestion of Sediments, Sludges, and Soils (USEPA, 1996a) were followed for soil sampling and analysis in this study.
Soil samples were sequentially extracted following the scheme established by Tessier et al. (1979) and modified by Carbonell-Barrachina et al. (1999). Five geochemical fractions of Pb (i.e., F-1 Soluble + Exchangeable; F-2 carbonate-bound; F-3 oxides-bound; F-4 organic matter-bound; and F-5 residual silicate-bound) in all soil fractions were determined. Briefly, (1) 1 g oven-dried soil sample was extracted soil at room temperature (25°C) for 30 min with 8 mL 0.5M Mg (NO3)2, adjusted to a pH of 7.0, to obtain the F-1 fraction. (2) The residual soil was extracted using 8 mL of 1M NaOAc, adjusted to pH 5.0 with acetic acid for the carbonate-bound Pb fraction (F-2). (3) Extraction of the F-3 fraction from the residual soil from step 2 was done at 96°C for 6 h with 20 mL 0.08M NH2OH.HCl in 25% acetic acid, adjusted to pH 5.0 with acetic acid. (4) The Organic matter-bound Pb fraction (F-4) was extracted from the residual soil obtained from the previous step at 85°C for 5 h with 3 mL 0.02M HNO3 and 5 mL 30% H2O2 (adjusted to pH 2.0 with HNO3). After cooling, 5 mL of 3.2M CH3COONH4 in 20% (v/v) HNO3 was added. (5) The residual silicate bound Pb (F-5) was then obtained by digestion of the residual soil sample from step 4 with 25 mL concentrated HNO3 at 105°C. The mixture from each step were centrifuged for 20 min at 3,500 x g to separate the supernatant from the residuals. The supernatant was collected and filtered for ICP-OES analysis for lead. The residual soil from each step were weighed and used for the next step in the sequential extraction procedure.
Pearson’s correlation analysis was employed to identify the relationship between various soil properties and lead fractions. The significance level of correlations was determined at a 95% confidence interval (p < 0.05). Parametric statistical tests necessitate data to follow a normal distribution, but frequently, data from polluted sites exhibit a positive skewness (Davies, 1997). Therefore, Shapiro–Wilks’ W test for normality (Shapiro and Wilk, 1965) was conducted on the data of all soil properties for collected soils, and data were log-transformed as needed, using the natural log(ln) to attain normal distribution. Regression equations were developed to compare lead concentrations obtained by field pXRF and laboratory ICP-OES. Data analysis was performed using Microsoft Excel (Version 2018) and the JMP statistical software package (JMP 14, SAS Institute Inc.).
The physicochemical properties of lead-contaminated San Antonio, Baltimore, and Detroit soils are summarized in Table 1, Table 2, and Table 3 respectively. Soils collected from San Antonio were slightly alkaline (pH ranging from 7.43 to 7.87), whereas those from Baltimore were slightly acidic (pH ranging from 5.16 to 6.25). Soils collected from Detroit were characterized by neutral to mildly alkaline pH (ranging from 6.28 to 7.88). The alkaline nature of San Antonio soils is the result of both geological and climatic influences. San Antonio is situated in an area abundant in limestone bedrock, and this geological feature significantly impacts the soil properties (Ewing, 1996). The weathering of the underlying limestone is a primary mechanism responsible for elevating the pH of many San Antonio soils. Furthermore, the semi-arid to arid climate experienced in San Antonio, characterized by limited rainfall, plays a vital role in maintaining the alkalinity of the soils (Hernandez and Uddameri, 2014). Baltimore’s geological heritage is significantly influenced by its location within the Piedmont geological province (Yesilonis et al., 2008; Hunt, 1967). Soils found in the Piedmont region are typically underlain by acidic rocks, and the nature of these rocks significantly influences soil acidity (Levin and Griffin, 1998).
TABLE 1. Selected physical and chemical characteristics of lead-contaminated residential soils collected from San Antonio. Values are mean (±standard deviation) of three replicates.
TABLE 2. Selected physical and chemical characteristics of lead-contaminated residential soils collected from Baltimore. Values are mean (±standard deviation) of three replicates.
TABLE 3. Selected physical and chemical characteristics of Pb-contaminated residential soils collected from Detroit. Values are mean (±standard deviation) of three replicates.
Generally, the San Antonio soils had relatively high soil salinity; the EC values ranged between 217 and 11,051 μS/cm. These soils also had relatively high clay content with values reaching up to 65.22% and characterized by moderate to high SOM ranging from (5.23%–12.89%). Also, San Antonio soils were characterized by high TC (ranging from 32% to 47%), and relatively high CEC (12–42 meq/100 g) (Table 1). In contrast to the San Antonio soils, soils collected from Baltimore were characterized by lower salinity with EC ranging from 97 to 715 μS/cm, and lower clay content (2.1%–5.2%). Soils collected from Baltimore had relatively low SOM that ranged from 0.64% to 2.27% and low TC ranging from 4.5% to 5.5% (Table 2). Compared to San Antonio soils, soils collected from Baltimore had lower CEC ranging from 5 to 10 meq/100 g. Soils with high clay content or high organic matter content have been reported to have higher CEC values (Peter et al., 1994; Jeon et al., 2023). Soils collected from residential properties in Detroit were characterized by relatively high salinity (EC ranging from 301–780 μS/cm) and relatively high clay content ranging from 6.77% to 19.98%. These soils had high SOM content with values up to 20% and relatively high TC content with values up to 37%. The CEC of Detroit soils was high ranging from 12 to 54 meq/100 g (Table 3).
Total concentrations of Al, Fe, p, Ca, and Mg in the study soils from San Antonio, Baltimore, and Detroit are shown in Tables 1–3 respectively. Generally, total Al was higher in Detroit soils compared with soils collected from San Antonio and Baltimore, whereas Baltimore soils contain the highest concentrations of total Fe, p, and Mg. The total concentration of Ca was higher in San Antonio soils compared with Baltimore and Detroit soils.
The total lead concentration in the soils collected from San Antonio, Baltimore, and Detroit is presented in Figures 1A, C, E. A wide variation in total lead content was observed in the San Antonio soils, which ranged from 361–7,786 mg/kg (Figure 1A). Soils from all sites, except for SA4, exceeded the maximum permissible level (MPL) of lead in soils (400 mg/kg) prescribed by the U.S. Environmental Protection Agency (USEPA, 1996b). Previous research reported a considerable range in total lead concentrations within residential soils in San Antonio. This range was evident in a previous study, where lead levels ranged from 306 to 4,182 mg/kg (Andra et al., 2006). Furthermore, an even broader range of lead concentrations was reported, with the highest recorded value reaching 14,721 mg/kg in sites contaminated with Pb-based paint in San Antonio (Zhang et al., 2022).
FIGURE 1. Total lead concentrations in lead-contaminated residential soils of San Antonio (A), Baltimore (C), and Detroit (E). Data are shown as mean (n = 3) ± standard deviation. Simple linear regression of soil Pb concentrations (mg/kg) measured by pXRF against ICP-OES in residential soils of San Antonio (B); Baltimore (D), Detroit (F) soils.
A wider variation of lead concentrations was observed in the soils collected from Baltimore which ranged between 39–5,373 mg/kg (Figure 1C). About 70% of tested Baltimore soils exceeded the USEPA’s MPL of 400 mg/kg for soil lead (USEPA, 1996b). Previous studies in Baltimore have documented maximum concentrations of 5,650 mg/kg (Yesilonis et al., 2008) and 2,165 mg/kg (Sarkar et al., 2008) in residential soils. The overall lead concentrations in Detroit samples were lower than those of San Antonio and Baltimore (Figure 1E). The lead concentration in the Detroit soils ranged from 124–1,575 mg/kg with about 70% of the collected samples exceeding the USEPA’s MPL of 400 mg/kg for soil lead. Earlier studies have recorded average lead levels of 910 mg/kg (Mielke et al., 2020) and 1,147 mg/kg (Bickel, 2010) in Detroit soils.
The performance of pXRF for field soil lead measurement was assessed by comparing pXRF data with ICP-OES data. The average lead concentrations of triplicated samples measured by both methods are shown in Figure 1 for San Antonio (B), Baltimore (D), and Detroit (F). Regression equations were developed to compare both techniques. The obtained scatter plots represented a linear regression relation with the coefficient of determination of (R2 = 0.98, R2 = 0.97, R2 = 0.97) for San Antonio, Baltimore, and Detroit samples respectively. This result indicates a strong positive correlation between both techniques. These R2 values consistently displayed a stronger correlation, outperforming the reported value of 0.89 in Maliki et al. (2017). Previous studies have used pXRF analysis to assess metal contamination in a variety of soils (Zhang et al., 2022). Reports indicate that the accuracy of pXRF measurements is variable, based on the source of the metals, soil moisture, organic matter content, and the types of metals being analyzed (Caporale et al., 2018; Ravansari et al., 2020; Barzingi, 2023). In the current study, the data from all sites showed good correlations indicating the differences in soil properties between San Antonio, Baltimore and Detroit did not significantly affect the accuracy of pXRF measurements.
Although total lead concentration in soil is an important parameter from the regulatory standpoint, various other soil properties play a significant role in determining what fraction of the lead can be considered bioavailable (Ruby et al., 1993; Saminathan et al., 2010; Kastury et al., 2023). The distribution of lead in the geochemical fractions of the residential soils collected from San Antonio, Baltimore, and Detroit are presented in Figure 2. The mean values of lead recovery were 97.27% for San Antonio, 98.09% for Baltimore, and 97.14% for Detroit soils.
FIGURE 2. Geochemical fractionation of lead in residential soils collected from San Antonio (A), Baltimore (B), and Detroit (C). F-1 Soluble + Exchangeable; F-2 carbonate-bound; F-3 oxides-bound; F-4 organic matter-bound; and F-5 residual silicate-bound.
The results of San Antonio soils indicate that most of the lead existed within the carbonate-bound (F2) (average percentage of 32% ± 6.5%) and organic matter-bound (F4) fractions (average percentage of 25% ± 2.3%) of the soils (Figure 2A), which can be explained by the higher pH and the higher organic matter content in these soils respectively (Table 1). In contrast, in Baltimore soils, the majority of lead was in the soluble + exchangeable fraction (F1) (average percentage of 42% ± 3.8) and Fe/Mn oxide-bound fractions (F3) (average percentage of 26% ± 2.5%) (Figure 2B). This result could be attributed to the acidic nature of the soils and their high Al and Fe content respectively (Table 2). These results are consistent with those obtained from our previous study on residential soils of San Antonio and Baltimore (Sarkar et al., 2008). Lead adsorption on soil components is typically controlled by soil pH, which controls the surface charge of the adsorbent, as well as the degree of ionization and speciation of metal cations (Elliott and Huang, 1981; Andra et al., 2006). Despite the higher levels of total lead concentration in San Antonio soils compared to Baltimore soils, F1 fraction in San Antonio soils had lower lead levels (Figures 2A, B). The results of the geochemical speciation of lead in Detroit soils revealed that the highest percentage of lead was located within F4 fractions (average percentage of 49% ± 4.6%) and F3 fractions (average percentage of 21% ± 2%) (Figure 2C). The abundance of organic-bound lead in these soils can be explained by their high organic matter content while the high concentrations Al and Fe may contribute to the high percentage of oxide-bound lead (Table 3). The lowest lead content in the F1 fraction in San Antonio (average of 9%) and Detroit soils (average 6%) could be attributed to their alkaline nature. In contrast, the lowest lead concentration in Baltimore soils residing in the silicate-bound fraction (F5) (average 6%) could be attributed to their low clay content.
According to these results, soil pH, SOM, and clay content have the most influence on lead distribution in soil geochemical fractions. This coincides with the results of several previous studies (Covelo et al., 2007; Vega et al., 2008; Vega et al., 2010; Cerqueira et al., 2011; Luo et al., 2024). The results obtained in this study highlight the importance of considering the influence of soil physicochemical properties on lead dynamics while developing suitable remediation strategies for the cleanup and proper management of lead-contaminated soils.
Soil properties such as pH, SOM, carbonate levels, and clay content can affect the equilibrium between the exchangeable and immobilized lead pools. The water soluble + exchangeable lead fractions are considered the most bioavailable, followed by the carbonate-bound form. The reducible form bound to Fe/Al oxides and oxidizable form bound to SOM is believed to be potentially bioavailable, while the residual form is not bioavailable (Rinklebe and Shaheen, 2014). Physical and chemical properties of soil affect how much of the total lead present is extracted as each of the fractions (Finzgar et al., 2007; Yan et al., 2019).
The exchangeable fraction consists of metal ions that are weakly bonded or sorbed to the solid phase, which are easily mobilizable and, hence, are available for the biota (Haque et al., 2021). The exchangeable fraction of lead determined from sequential extractions was correlated with the soil properties to identify which soil properties exert the maximum influence on determining the exchangeable form of lead that is more likely to become bioavailable. Hence, Pearson’s correlation analysis was performed to understand the relationship between the physicochemical properties of soil samples and the exchangeable fraction of lead in lead paint-contaminated residential soils of San Antonio, Baltimore, and Detroit (Table 4). Shapiro–Wilks’ W test for normality (Shapiro and Wilk, 1965) was conducted on the data of all soil properties. The arithmetic values for clay%, EC, and CEC in San Antonio; pH, EC, and TC% in Baltimore; clay %, pH, and total Al, in Detroit soils yielded a probability value of less than 0.05 from Shapiro–Wilks’ W test. This suggested that these data were not normally distributed and hence we transformed those data into logarithmic values before running the correlation analysis.
TABLE 4. Pearson’s correlation coefficients (r) between the exchangeable Pb and various soil physicochemical properties of Pb-contaminated residential soils of San Antonio, Baltimore, and Detroit.
The exchangeable fraction of lead in San Antonio soils is influenced by the total amount of Al and Ca which is represented by the statistically significant negative correlation with total Al (r = −0.653) and total Ca (r = −0.438) (Table 4). Generally, the correlation coefficient between SOM and exchangeable lead is reported to be higher in alkaline soils compared to acidic soils (Sauve et al., 1998). Our results on San Antonio soils (alkaline) and Baltimore soils (acidic) support the same observation that the greater the amount of organic matter, the greater the extent of soluble lead in the alkaline San Antonio soils (r = 0.360, p < 0.05). These results coincide with the results obtained from our previous study (Sarkar et al., 2008). Soil pH is negatively correlated with the exchangeable lead forms in the San Antonio, Baltimore, and Detroit soils. Soil acidity favors the mobilization of lead from insoluble to soluble pools and increases the free- and solvated ions and ion pairs of lead by about two orders of magnitude with each unit drop of soil pH (Jin et al., 2005; Sarkar et al., 2008). For Baltimore soils, the exchangeable fraction is governed by the pH and total p levels in the soil as demonstrated by the significant negative correlations between exchangeable lead fraction and pH (r = −0.286, p < 0.05) and total p (r = −0.314). Solubility of lead generally increases with decreasing soil pH; hence, lead is likely to be more available for plant uptake in the Baltimore soils, which are slightly acidic. There were also negative correlations between lead mobility in Baltimore soils and clay content, EC, SOM, TC, and total Al content but they were not significant (Table 4). For Detroit soils, the exchangeable fraction of lead was significantly influenced by the total Al and SOM levels in the soil as represented by the significantly negative correlations between exchangeable lead and total Al (r = − 0.628, p < 0.05) and SOM (r = −0.408). A negative non-significant correlation between exchangeable lead and clay content, pH, EC, and TC was also observed in Detroit soils (Table 4).
Knowledge of the various soil properties that influence the geochemical forms of lead in soils is essential to develop remediation technologies for lead immobilization. This knowledge represents the key to understanding the potentially bioavailable fractions of lead, as well as the extent of lead that might be potentially remobilized under certain environmental conditions. This will result in an improved efficiency of a given remediation technique geared towards lowering soil lead-induced environmental and human health risks.
Based on the correlation analysis results (Table 4) in which the exchangeable fraction of lead in soil was correlated against several physicochemical soil properties, we recommend potential amendments for each soil type for lead immobilization. Considering the significant negative correlation of exchangeable lead in San Antonio soils with the total amount of Ca, calcium sulfate (gypsum) could represent a potential sustainable immobilization amendment for this type of soil. Gypsum is a cheap source of Ca, and its application could improve the physical and chemical properties of soils, aid in plant growth, and minimize the transport of dissolved organic carbon (DOC). The relatively high SOM content of San Antonio soils (Table 1), and the significantly positive correlation between SOM and mobilized lead (Table 4), SOM may decompose with time releasing all the associated lead content. High levels of DOC in the soil could enhance the complexation of lead by organic ligands, thereby increasing lead mobility in the soil (Almås et al., 2000; Weng et al., 2002; Cao et al., 2003; Kim et al., 2018). Gypsum can act as a bridge between negatively charged DOC and soil particles, assisting DOC coagulation and resulting in decreased soil DOC concentrations (Udeigwe et al., 2011).
Since Baltimore soils showed a significant negative correlation of the exchangeable fraction of lead with pH and total p, applying high P-containing biochar represents a good sustainable amendment for lead immobilization in these soils (Larson, et al., 2005). High P-containing biochars (e.g., pistachio shell biochar, soybean stover biochar, or bone char) can lead to the precipitation of lead as chloropyromorphite (Mashyekhi et al., 2020; Awad et al., 2021). Additionally, biochar has been recognized as a liming material. Its liming effects have been proven to successfully immobilize lead (Yang et al., 2016; O’Connor et al., 2018). That is an additional beneficial property concerning the significant negative correlation of mobilized lead with Baltimore soil pH.
Similar to San Antonio soils, the exchangeable fraction of lead in Detroit soils was significantly correlated with total Al content (Table 4). Aluminum sulfate (Alum) could be one of the best immobilization amendments for this type of soil containing relatively high SOM (Table 3). Aluminum is known to bind readily with SOM and such Al–OM complexes could lead to the reduction of DOC concentration (Udeigwe et al., 2011). This is generally beneficial since high DOC concentrations increase lead bioavailability. Regarding the significant negative correlation of exchangeable lead with SOM, biochar could also potentially be used as a sustainable immobilization technique for Detroit soils. Immobilization of lead by complexation or precipitation with high SOM has an advantage over adsorption approaches because organic matter could suppress the sorption of contaminants by blocking the micropores (Zhang et al., 2013).
These suggested sustainable amendments offer an environmentally friendly and economically feasible approach and align with the principles of sustainability. Biochar, for instance, is a byproduct of biomass pyrolysis, contributing to carbon sequestration while simultaneously aiding in lead immobilization. Gypsum, a naturally occurring mineral that can be sourced from industrial byproducts, not only minimizes lead mobility but also enhances soil structure, promoting long-term soil health. Similarly, alum, a common water treatment additive, exhibits dual benefits by reducing lead bioavailability and improving soil water-holding capacity.
This study investigated the concentrations and geochemical fractionation of lead in paint-contaminated residential soils with a wide range of physicochemical characteristics across three major U.S. cities. It aimed to identify the most suitable immobilization amendments based on soil-specific features and their correlation with mobile lead fractions. Field surveys at 10 residential sites each in San Antonio, Baltimore, and Detroit found that 77% of soil samples exceeded the EPA’s 400 mg/kg lead hazard threshold. Portable X-ray fluorescence (pXRF) analysis provided a cost-effective, rapid method for on-site lead level assessment, correlating with laboratory results. Geochemical lead distribution was influenced primarily by soil pH, organic matter (SOM), and clay content. In San Antonio, total Ca and Al content played a role, while pH and total p content influenced Baltimore soils, and SOM and total Al were key factors in Detroit. Recommended amendments include gypsum for San Antonio, biochar for Baltimore, and alum for Detroit soils, offering effective lead immobilization. This study emphasizes the significance of soil physicochemical properties in lead dynamics for developing efficient remediation strategies. Ongoing laboratory and greenhouse studies are assessing these the effectiveness of these amendments in reducing lead mobility and bioavailability in paint-contaminated soils.
The original contributions presented in the study are included in the article/Supplementary material, further inquiries can be directed to the corresponding author.
HS: Data curation, Formal Analysis, Investigation, Methodology, Validation, Writing–original draft. DS: Conceptualization, Funding acquisition, Project administration, Resources, Supervision, Writing–review and editing. WB: Data curation, Investigation, Visualization, Writing–review and editing. SL: Writing–review and editing. RD: Conceptualization, Funding acquisition, Project administration, Writing–review and editing.
The author(s) declare financial support was received for the research, authorship, and/or publication of this article. This study was funded by the Lead Technical Studies program of the U.S. Housing and Urban Development (grant# MILTS0023-21).
We acknowledge Brendan Brown and Chevelle Bash, Green and Healthy Homes Initiatives, Willie G. Villarreal, Jr of the Neighborhood and Housing Services Department of the City of San Antonio, and Anna Pinter, Housing and Revitalization Department, City of Detroit for liaison with homeowners. We thank Zhiming Zhang, Madeline English, and Benjamin Agnello for help with soil collection. We also thank Khalid Mostafa and Vijay Persaud at Stevens Institute of Technology for their technical help. We are grateful to the homeowners for their cooperation in providing soil samples for this study.
The authors declare that the research was conducted in the absence of any commercial or financial relationships that could be construed as a potential conflict of interest.
The author(s) declared that they were an editorial board member of Frontiers, at the time of submission. This had no impact on the peer review process and the final decision.
All claims expressed in this article are solely those of the authors and do not necessarily represent those of their affiliated organizations, or those of the publisher, the editors and the reviewers. Any product that may be evaluated in this article, or claim that may be made by its manufacturer, is not guaranteed or endorsed by the publisher.
Almås, Å. R., McBride, M. B., and Singh, B. R. (2000). Solubility and lability of cadmium and zinc in two soils treated with organic matter. Soil Sci. 165 (3), 250–259. doi:10.1097/00010694-200003000-00007
Andra, S. S., Sarkar, D., Datta, R., and Saminathan, S. (2006). Lead in soils in paint contaminated residential sites at San Antonio, Texas, and Baltimore, Maryland. Bull. Environ. Contam. Toxicol. 77 (5), 643–650. doi:10.1007/s00128-006-1111-y
Awad, M., Liu, Z., Skalicky, M., Dessoky, E. S., Brestic, M., Mbarki, S., et al. (2021). Fractionation of heavy metals in multi-contaminated soil treated with biochar using the sequential extraction procedure. Biomolecules 11 (3), 448. doi:10.3390/biom11030448
Bardy, B. A., Bricka, R. M., and Larson, S. L. (2003). Chemical stabilization of lead in small arms firing range soils–environmental quality and technology program. ERDC/ELTR-03-20. Vicksburg, Mississippi: U.S. Army Corps of Engineers, 1–67.
Barzingi, A. (2023). Comparative study ofP-OES, μ-XRF and P-XRF of trace elements analysis in various Sasanian and Parthian artworks found in the Garmian region of Iraq. AIP Conf. Proc. 2475 (1). doi:10.1063/5.0102500
Bickel, M. J. (2010). Spatial and temporal relationships between blood lead and soil lead concentrations in Detroit. Michigan: Wayne State University thesis. Available at: https://digitalcommons.wayne.edu/oa_theses/47/.
Brown, M. J., and Margolis, S. (2012). Lead in drinking water and human blood lead levels in the United States. MMWR Suppl. 61 (4), 1–9.
Burki, T. (2020). Report says 815 million children have high blood lead levels. Lancet 396 (10248), 370. doi:10.1016/s0140-6736(20)31684-6
Canfield, R. L., Henderson, C. R., Cory-Slechta, D. A., Cox, C., Jusko, T. A., and Lanphear, B. P. (2003). Intellectual impairment in children with blood lead concentrations below 10 μg per deciliter. N. Engl. J. Med. 348 (16), 1517–1526. doi:10.1056/nejmoa022848
Cao, X., Ma, L. Q., Chen, M., Hardison, D. W., and Harris, W. G. (2003). Weathering of lead bullets and their environmental effects at outdoor shooting ranges. J. Environ. Qual. 32 (2), 526–534. doi:10.2134/jeq2003.5260
Caporale, A. G., Adamo, P., Capozzi, F., Langella, G., Terribile, F., and Vingiani, S. (2018). Monitoring metal pollution in soils using portable-XRF and conventional laboratory-based techniques: evaluation of the performance and limitations according to metal properties and sources. Sci. Total Environ. 643, 516–526. doi:10.1016/j.scitotenv.2018.06.178
Carbonell-Barrachina, A., Jugsujinda, A., DeLaune, R. D., Patrick, W. H., Barlo, F., Sirisukhodom, S., et al. (1999). The influence of redox chemistry and pH on chemically active forms of arsenic in sewage sludge-amended soil. Environ. Internatl. 25, 613–618. doi:10.1016/s0160-4120(99)00027-6
Cerqueira, B., Covelo, E. F., Andrade, M. L., and Vega, F. A. (2011). The influence of soil properties on the individual and competitive sorption and desorption of Cu and Cd. Geoderma 162, 20–26. doi:10.1016/j.geoderma.2010.08.013
Clark, J. J., and Knudsen, A. C. (2013). Extent, characterization, and sources of soil lead contamination in small-urban residential neighborhoods. J. Environ. Qual. 42, 1498–1506. doi:10.2134/jeq2013.03.0100
Clark, S., Bornschein, R., Succop, P., Roda, S., and Peace, B. (1991). Urban lead exposures of children in Cincinnati, Ohio. Ohio. Chem. Speciat. Bioavail. 3, 163–171. doi:10.1080/09542299.1991.11083167
Covelo, E. F., Vega, F. A., and Andrade, M. L. (2007). Competitive sorption and desorption of heavy metals by individual soil components. J. Hazard. Mater. 140, 308–315. doi:10.1016/j.jhazmat.2006.09.018
Davies, B. E. (1997). Heavy metal contaminated soils in an old industrial area of Wales, Great Britain: source identification through statistical data interpretation. Water Air Soil Pollut. 94, 85–98. doi:10.1007/bf02407095
Egan, K. B., Cornwell, C. R., Courtney, J. G., and Ettinger, A. S. (2021). Blood lead levels in US children ages 1–11 years, 1976–2016. Environ. health Perspect. 129 (3), 037003. doi:10.1289/ehp7932
Elliott, H. A., and Huang, C. P. (1981). Adsorption characteristics of some Cu (II) complexes on aluminosilicates. Water Res. 15 (7), 849–855. doi:10.1016/0043-1354(81)90139-1
Ewing, T. E. (1996). “Summary of the geology of the san Antonio area,” in Rocks landscapes and man: urban geology of the san Antonio area. second edition, 6–54.
Finzgar, N., Tlustos, P., and Lestan, D. (2007). Relationship of soil properties to fractionation, bioavailability and mobility of lead and zinc in soil. Plant Soil Environ. 53 (5), 225–238. doi:10.17221/2201-pse
Gomes, F. P., Barreto, M. S. C., Amoozegar, A., and Alleoni, L. R. F. (2022). Immobilization of lead by amendments in a mine-waste impacted soil: assessing Pb retention with desorption kinetic, sequential extraction and XANES spectroscopy. Sci.Total Environ. 807, 150711. doi:10.1016/j.scitotenv.2021.150711
Guo, G., Zhou, Q., and Ma, L. (2006). Availability and assessment of fixing additives for the in-situ remediation of heavy metal contaminated soils: a review. Environ. Monit. Assess. 116 (1−3), 513–528. doi:10.1007/s10661-006-7668-4
Haque, E., Thorne, P. S., Nghiem, A. A., Yip, C. S., and Bostick, B. C. (2021). Lead (Pb)concentrations and speciation in residential soils from an urban community impacted by multiple legacy sources. J. Hazard. Mat. 416, 125886. doi:10.1016/j.jhazmat.2021.125886
Hernandez, E. A., and Uddameri, V. (2014). Standardized precipitation evaporation index (SPEI)-based drought assessment in semi-arid south Texas. Environ. Earth Sci. 71, 2491–2501. doi:10.1007/s12665-013-2897-7
Jacobs, D. E., Clickner, R. P., Zhou, J. Y., Viet, S. M., Marker, D. A., Rogers, J. W., et al. (2002). The prevalence of lead-based paint hazards in US housing. Environ. Health Perspect. 110 (10), A599–A606. doi:10.1289/ehp.021100599
Jeon, I., Chung, H., Kim, S. H., and Nam, K. (2023). Use of clay and organic matter contents to predict soil pH vulnerability in response to acid or alkali spills. Heliyon 9, e17044. doi:10.1016/j.heliyon.2023.e17044
Jin, C. W., Zheng, S. J., He, Y. F., Di Zhou, G., and Zhou, Z. X. (2005). Lead contamination in tea garden soils and factors affecting its bioavailability. Chemosphere 59 (8), 1151–1159. doi:10.1016/j.chemosphere.2004.11.058
Kastury, F., Li, H., Karna, R., Betts, A., Scheckel, K. G., Ma, L. Q., et al. (2023). Opportunities and challenges associated with bioavailability-based remediation strategies for lead-contaminated soil with arsenic as a Co-Contaminant—a critical review. Curr. Pollut. Rep. 9, 213–225. doi:10.1007/s40726-023-00252-z
Kim, H. S., Seo, B. H., Kuppusamy, S., Lee, Y. B., Lee, J. H., Yang, J. E., et al. (2018). A DOC coagulant, gypsum treatment can simultaneously reduce As, Cd and Pb uptake by medicinal plants grown in contaminated soil. Ecotoxicol. Environ. Saf. 148, 615–619. doi:10.1016/j.ecoenv.2017.10.067
Laidlaw, M. A., and Filippelli, G. M. (2008). Resuspension of urban soils as a persistent source of lead poisoning in children: a review and new directions. Appl. Geochem. 23 (8), 2021–2039. doi:10.1016/j.apgeochem.2008.05.009
Laidlaw, M. A., Filippelli, G. M., Brown, S., Paz-Ferreiro, J., Reichman, S. M., Netherway, P., et al. (2017). Case studies and evidence-based approaches to addressing urban soil lead contamination. Appl. Geochem. 83, 14–30. doi:10.1016/j.apgeochem.2017.02.015
Larson, S. L., Tardy, B., Rainwater, K., and Tingle, J. S. (2005). Rainfall lysimeter evaluation of leachability and surface transport of heavy metals from six soils with and without phosphate amendment.” ERDC TR-05-9. Vicksburg, MS: Engineering and Research Development Center.
LeBlanc, T. T., Svendsen, E. R., and Allwood, P. B. (2022). Ubiquitous lead: risks, prevention–mitigation programs, and emerging sources of exposure: introduction and contents of the issue. Amer. J. Public Health 112 (S7), S630–S631. doi:10.2105/ajph.2022.307083
Levin, M. J., and Griffin, T. M. (1998). Soil survey of city of Baltimore, Maryland. United States: Natural Resources Conservation Service.
Luo, X., Xiang, C., Wu, C., Gao, W., Ke, W., Zeng, J., et al. (2024). Geochemical fractionation and potential release behaviour of heavy metals in lead‒zinc smelting soils. J. Environ. Sci. 139, 1–11. doi:10.1016/j.jes.2023.05.022
Mahar, A., Ping, W. A. N. G., Ronghua, L. I., and Zhang, Z. (2015). Immobilization of lead and cadmium in contaminated soil using amendments: a review. Pedosphere 25 (4), 555–568. doi:10.1016/s1002-0160(15)30036-9
Maliki, A., Al-lami, A. K., Hussain, H. M., and Al-Ansari, N. (2017). Comparison between inductively coupled plasma and X-ray fluorescence performance for Pb analysis in environmental soil samples. Environ. Earth Sci. 76, 433. doi:10.1007/s12665-017-6753-z
Manceau, A., Boisset, M. C., Sarret, G., Hazemann, J. L., Mench, M., Cambier, P., et al. (1996). Direct determination of lead speciation in contaminated soils by EXAFS spectroscopy. Environ. Sci. Technol. 30 (5), 1540–1552. doi:10.1021/es9505154
Martin, W. A., Larson, S. L., Felt, D. R., Wright, J., Griggs, C. S., Thompson, M., et al. (2008). The effect of organics on lead sorption onto Apatite II™. Appl. Geochem. 23 (1), 34–43. doi:10.1016/j.apgeochem.2007.08.005
Mashyekhi, R., Emami, H., and Naghizade Asl, F. (2020). The influence of pistachio shell biochar and barley residues on soil properties. Pol. J. Soil Sci. 53 (1), 21–40. doi:10.17951/pjss.2020.53.1.21
McBride, M., Sauve, S., and Hendershot, W. (1997). Solubility control of Cu, Zn, Cd and Pb in contaminated soils. Eur. J. Soil Sci. 48 (2), 337–346. doi:10.1111/j.1365-2389.1997.tb00554.x
Mielke, H. W., Gonzales, C. R., Powell, E. T., Shah, A., Berry, K. J., and Richter, D. D. (2020). Spatial-temporal association of soil Pb and children’s blood Pb in the Detroit Tri-County Area of Michigan (USA). Environ. Res. 191, 110112. doi:10.1016/j.envres.2020.110112
O’Connor, D., Peng, T., Zhang, J., Tsang, D. C., Alessi, D. S., Shen, Z., et al. (2018). Biochar application for the remediation of heavy metal polluted land: a review of in situ field trials. Sci. Total Environ. 619, 815–826. doi:10.1016/j.scitotenv.2017.11.132
Peter, J., Wujcik, W. J., and Loncar, A. F. (1994). Remediation technologies screening matrix and reference guide. Second edition. Final report.
Ravansari, R., Wilson, S. C., and Tighe, M. (2020). Portable X-ray fluorescence for environmental assessment of soils: not just a point and shoot method. Environ. Int. 134, 105250. doi:10.1016/j.envint.2019.105250
Rinklebe, J., and Shaheen, S. M. (2014). Assessing the mobilization of cadmium, lead, and nickel using a seven-step sequential extraction technique in contaminated floodplain soil profiles along the central elbe river, Germany. Water Air Soil Pollut. 225, 2039. doi:10.1007/s11270-014-2039-1
Ruby, M. V., Davis, A., Link, T. E., Schoof, R., Chaney, R. L., Freeman, G. B., et al. (1993). Development of an in vitro screening test to evaluate the in vivo bioaccessibility of ingested mine-waste lead. Environ. Sci. Technol. 27 (13), 2870–2877. doi:10.1021/es00049a030
Saminathan, S. K., Sarkar, D., Andra, S. S., and Datta, R. (2010). Lead fractionation and bioaccessibility in contaminated soils with variable chemical properties. Chem. Speciat. Bioavail. 22 (4), 215–225. doi:10.3184/095422910x12892425424203
Sarkar, D., Andra, S. S., Saminathan, S. K., and Datta, R. (2008). Chelant-aided enhancement of lead mobilization in residential soils. Environ. Pollut. 156 (3), 1139–1148. doi:10.1016/j.envpol.2008.04.004
Sauve, S., McBride, M., and Hendershot, W. (1998). Soil solution speciation of lead (II): effects of organic matter and pH. Soil Sci. Soc. amer. J. 62 (3), 618–621. doi:10.2136/sssaj1998.03615995006200030010x
Schulte, E. E., and Hopkins, B. G. (1996). Estimation of soil organic matter by weight loss-on-ignition. Soil Org. matter Analysis interpretation 46, 21–31. doi:10.2136/sssaspecpub46.c3
Shapiro, S. S., and Wilk, M. B. (1965). An analysis of variance test for normality (complete samples). Biometrika 52, 591–611. doi:10.2307/2333709
D. L. Sparks, A. L. Page, P. A. Helmke, and R. H. Loeppert (2020). Methods of soil analysis, part 3: chemical methods, Vol. 14 (Madison,WI: John).
Tessier, A. P. G. C., Campbell, P. G., and Bisson, M. J. A. C. (1979). Sequential extraction procedure for the speciation of particulate trace metals. Anal. Chem. 51 (7), 844–851. doi:10.1021/ac50043a017
Udeigwe, T. K., Eze, P. N., Teboh, J. M., and Stietiya, M. H. (2011). Application, chemistry, and environmental implications of contaminant-immobilization amendments on agricultural soil and water quality. Environ. Internatl. 37 (1), 258–267. doi:10.1016/j.envint.2010.08.008
USEPA (2001). Lead: identification of dangerous levels of lead. Final rule. Fed. Regist. 66, 1206–1240.
U.S. EPA (2023). Assurance user's guide. EPA 600/8-89/046. Las Vegas, NV: U.S. EPA Environmental Monitoring Systems Lab.
USEPA (United States Environmental Protection Agency) (1996a). Method 3050B: acid digestion of Sediments, Sludges, and soils, revision 2. Washington, DC: United States Environmental Protection Agency.
USEPA (United States Environmental Protection Agency) (1996b). Soil screening guidance: user’s guidance. Vol. EPA 540/R-96/018. Washington, DC: Office of Solid and Emergency Response.
Vega, F. A., Covelo, E. F., and Andrade, M. L. (2008). A versatile parameter for comparing the capacities of soils for sorption and retention of heavy metals dumped individually or together: results for cadmium, copper and lead in twenty soil horizons. J. Colloid Interface Sci. 327, 275–286. doi:10.1016/j.jcis.2008.08.027
Vega, F. A., Covelo, E. F., and Andrade, M. L. (2010). Influence of soil properties on the sorption and retention of cadmium, copper and lead, separately and together, by 20 soil horizons: comparison of linear regression and tree regression analyses. J. Hazard. Mater. 174, 522–533. doi:10.1016/j.jhazmat.2009.09.083
Weng, L., Temminghoff, E. J., Lofts, S., Tipping, E., and Van Riemsdijk, W. H. (2002). Complexation with dissolved organic matter and solubility control of heavy metals in a sandy soil. Environ. Sci. Technol. 36 (22), 4804–4810. doi:10.1021/es0200084
Yan, K., Dong, Z., Wijayawardena, M. A., Liu, Y., Li, Y., and Naidu, R. (2019). The source of lead determines the relationship between soil properties and lead bioaccessibility. Environ. Pollut. 246, 53–59. doi:10.1016/j.envpol.2018.11.104
Yang, Z., Fang, Z., Zheng, L., Cheng, W., Tsang, P. E., Fang, J., et al. (2016). Remediation of lead contaminated soil by biochar-supported nano-hydroxyapatite. Ecotoxicol. Environ. Saf. 132, 224–230. doi:10.1016/j.ecoenv.2016.06.008
Yesilonis, I. D., Pouyat, R. V., and Neerchal, N. K. (2008). Spatial distribution of metals in soils in Baltimore, Maryland: role of native parent material, proximity to major roads, housing age and screening guidelines. Environ. Pollut. 156 (03), 723–731. doi:10.1016/j.envpol.2008.06.010
Zhang, X., Wang, H., He, L., Lu, K., Sarmah, A., Li, J., et al. (2013). Using biochar for remediation of soils contaminated with heavy metals and organic pollutants. Environ. Sci. Pollut. Res. 20, 8472–8483. doi:10.1007/s11356-013-1659-0
Keywords: lead, residential soils, soil physicochemical properties, geochemical speciation, bioaccessibility, immobilization, soil amendment
Citation: Saleh H, Sarkar D, Braida W, Larson S and Datta R (2023) Lead in paint-contaminated residential soils with varying physicochemical properties from three large US cities: assessment of geochemical forms and recommendations on amendments for immobilization. Front. Environ. Sci. 11:1309169. doi: 10.3389/fenvs.2023.1309169
Received: 07 October 2023; Accepted: 07 December 2023;
Published: 22 December 2023.
Edited by:
Prafulla Kumar Sahoo, Central University of Punjab, IndiaReviewed by:
Rakesh Kumar, Auburn University, United StatesCopyright © 2023 Saleh, Sarkar, Braida, Larson and Datta. This is an open-access article distributed under the terms of the Creative Commons Attribution License (CC BY). The use, distribution or reproduction in other forums is permitted, provided the original author(s) and the copyright owner(s) are credited and that the original publication in this journal is cited, in accordance with accepted academic practice. No use, distribution or reproduction is permitted which does not comply with these terms.
*Correspondence: Dibyendu Sarkar, ZHNhcmthckBzdGV2ZW5zLmVkdQ==
Disclaimer: All claims expressed in this article are solely those of the authors and do not necessarily represent those of their affiliated organizations, or those of the publisher, the editors and the reviewers. Any product that may be evaluated in this article or claim that may be made by its manufacturer is not guaranteed or endorsed by the publisher.
Research integrity at Frontiers
Learn more about the work of our research integrity team to safeguard the quality of each article we publish.