- 1Northwest Institute of Eco-Environment and Resources, Chinese Academy of Sciences, Lanzhou, China
- 2Key Laboratory of Desert and Desertification, Chinese Academy of Sciences, Lanzhou, China
- 3School of Resources and Environment, University of Chinese Academy of Sciences, Beijing, China
- 4Naiman Desertification Research Station, Northwest Institute of Eco-Environment and Resources, Chinese Academy of Sciences, Tongliao, China
Soil extracellular enzyme activity (EEA) and enzyme stoichiometry are indicators of soil nutrient availability and microbial substrate limitation. However, the patterns in soil enzyme activities and stoichiometry, and their key drivers remain unclear during vegetation restoration in degraded sandy land. Here, soil ecosystems at five restoration stages of degraded sandy land were selected for investigation and we quantified the microbial nutrient limitation using EEA stoichiometry. The average C:N:P ratio for the nutrient-acquisition enzymes was 1:0.88:0.86, which differed from the global ratio of 1:1:1, indicating stronger microbial C limitation than N or P limitation. With vegetation restoration, the enzyme N:P ratio decreased, but the angle term used in vector analysis increased, indicating that the restored grassland transitioned from N-restricted (angle < 45°) to P-restricted (angle > 45°). Plant C inputs increased the soil nutrient content and significantly decreased the microbial C and N limitation but increased P limitation due to nutrient competition between plants and soil microorganisms. Decreased soil water levels caused by vegetation transpiration may have shifted the microbial limitation from N to P. The decreased fungi:bacteria ratio strengthened the microbial C and N or P limitations. On the basis of these findings, as measures to alleviate the associated nutrient limitations, we would recommend supplementation with phosphorus in the potential and slight stages of rocky desertification and the supplementary application of nitrogen in the moderate and severe stages during the restoration of degraded sandy ecosystems.
1 Introduction
Mineralization of this soil organic matter (SOM) by heterotrophic microorganisms has the potential to affect the cycling of elements such as carbon (C), nitrogen (N), and phosphorus (P), as well as plant production and the atmosphere’s composition on a global scale (Sinsabaugh et al., 2008). Soil extracellular enzymes are the proximate agents of SOM decomposition, as they catalyze a series of chemical reactions related to SOM degradation while also obtaining the required energy and nutrient resources for enzyme producers (Sinsabaugh et al., 2009). Changes in soil extracellular enzyme activity (EEA) levels result from changes in cellular metabolism during C, N, and P cycling (Peng and Wang, 2016), reflecting the biogeochemical balance between the microbial community’s metabolism and nutrient requirements and a site’s nutrient availability (Moorhead et al., 2013; Fanin et al., 2016).
Soil EEA stoichiometry is applied to predict nutrient availability in the environment and microorganism metabolic activity using EEA ratios and stoichiometric invariance (Sinsabaugh et al., 2009; Moorhead et al., 2013; 2016). Sinsabaugh et al. (2008) proposed that the metabolic and stoichiometric needs of soil microorganisms are reflected by the production of enzymes that target the principal C, N, and P sources. The most widely measured enzyme activities usually include C-acquiring enzymes: β-1,4-glucosidase (BG) and β-D-cellobiohydrolase (CBH); N-acquiring enzymes: β-1,4-N-acetylglucosaminidase (NAG) and leucine aminopeptidase (LAP); and P-acquiring enzymes: alkaline phosphatase (AP) (Sinsabaugh et al., 2008; Burns et al., 2013; Cenini et al., 2016; Mori, 2020).
Since these enzyme activities are higher than but correlated with the activities of other related enzymes (Sinsabaugh, 1994), these extracellular enzymes can be used as proxies for the allocation of microbial resources acquired by C, N, and P (Schimel and Weintraub, 2003; Fanin et al., 2016). Sinsabaugh et al. (2008) suggested that the EEA ratios, including BG:NAG (or BG [NAG + LAP]), BG:AP, and NAG:AP (or [NAG + LAP]:AP), reflect the relative activities of the enzymes that acquire carbon (C) vs. nitrogen (N), C vs. P, and N vs. P. Alternatively, if the ratios of BG:NAG (or BG [NAG LAP]), BG:AP, and NAG:AP (or [NAG + LAP]:AP) all rise, it indicates a microbial C limitation or shortage relative to N, a C limitation or a shortage relative to P, and an N limitation or shortage relative to P (Moorhead et al., 2016; Chen et al., 2018). In this context, Moorhead et al. (2016) developed a vector analysis method to calculate the “vector length” and “vector angle” that represent the enzymatic activity of the microbial community by connecting the origin of the plot to a point represented by the ratio of C:P to C:N. This approach assumes that the acquisition of relatively restricted elements by microorganisms is accomplished through the secretion of corresponding extracellular enzymes (Sinsabaugh and Follstad Shah, 2012). It is not affected by a single enzyme, and can comprehensively reflect the relative resources (C, N and P) of microbial metabolism as a whole. In addition, it can identify whether microbial metabolism in a given environment is limited by N or P alone, rather than by both together (Moorhead et al., 2016). This approach quantifies the acquisition of C, N, and P as vector lengths and the relative P and N acquisition as vector angles. Yang et al. (2020) adopted this method to confirm a shift from P limitation to N limitation of microorganisms during grassland restoration on the Loess Plateau in northwestern China. Vector analysis also showed that all temperate grassland sites in northern China are nutrient-limited, with the most serious limitation being P (Deng et al., 2022). Thus, EEA stoichiometry can help us better understand the metabolic constraints of soil microorganisms. This knowledge can provide insights into the fate of SOC and other nutrients.
EEA responses to soil resource availability are primarily regulated by soil microbes to meet their nutrient requirements (Sinsabaugh et al., 2009; Burns et al., 2013; Fanin et al., 2016); that is, EEA modifies enzymatic allocations to extract energy (C) and nutrients (N, P) from the soil as soil nutrient availability changes (Deng et al., 2019b). The EEA is generally affected by the quality of the available organic matter and the nutrient demands of the microbial biomass (Sinsabaugh et al., 2015). Litter input is the primary source of soil organic matter, and changes in its quantity and quality can affect the soil C content as well as the N and P stoichiometry (Cross and Schlesinger, 2001; Córdova et al., 2018). Changes in C and nutrient requirements, as well as changes in organic matter decomposition, can alter the composition and activity of microorganisms, leading to corresponding changes in EEA (Kaiser et al., 2014; Li et al., 2019). Recent studies have widely reported that the EEA is highly responsive to abiotic factors such as pH and nutrient availability (Allison and Vitousek, 2005; Manzoni et al., 2012; Bowles et al., 2014; Stark et al., 2014; Dong et al., 2019; Yang et al., 2020). EEA also responds to soil texture and moisture availability (Cui et al., 2018), precipitation (Zheng et al., 2020), temperature and the C:N ratio (Zhou et al., 2020).
However, the impact of biotic factors on the EEA cannot be ignored. Given the relatively limited data on the C:N:P ratio of microbial biomass (Cleveland and Liptzin, 2007), enzymatic activity is expected to change to increase the availability of the most limited nutrients to meet microbial metabolic needs (Bowles et al., 2014). Fungi have lower nutrient requirements than bacteria and higher carbon-use efficiency in poor-quality substrates (Keiblinger et al., 2010; McGuire et al., 2010), whereas bacteria require more N and P to maintain a high concentration of these elements during biomass turnover (Elser et al., 2003). As a result, changes in soil nutrients can affect the soil microbial community composition (Schneider et al., 2012; Kaiser et al., 2014). Soil microbial communities adjust their physiological metabolism accordingly, which is reflected in varying degrees of exoenzyme expression (Sinsabaugh et al., 2009). However, the impact of the soil microbial community on EEA stoichiometry has received little attention, and their relative contributions to stoichiometry and the driving mechanisms have received little attention.
In many places around the world, restoring vegetation in degraded landscapes is an effective way to improve soil quality (Paul et al., 2002; Berthrong et al., 2009; Li et al., 2013; 2015; 2017; Deng and Shangguan, 2017; Zhu et al., 2018). On the other hand, soil quality changes relatively slowly, whereas soil biological characteristics, such as the soil EEA, are sensitive to changes in the soil’s internal and external environments (Burns et al., 2013; Ren et al., 2016). As a result, soil EEA is frequently used as an indicator to assess soil recovery in various degraded ecosystems (Lino et al., 2016; Ren et al., 2016; Raiesi and Salek-Gilani, 2018; Deng et al., 2019a). Vegetation restoration can improve soil quality (Zhu et al., 2018; Deng et al., 2019a) by creating a favorable growth environment for soil microorganisms, thereby increasing soil microbial biomass and EEA (Ren et al., 2016; Raiesi and Salek-Gilani, 2018). Deng et al. (2019b) found that in the process of vegetation restoration in the Loess Plateau of China, soil microbial community was mainly limited by C and P, especially in the early stage of vegetation restoration (<13 years) soil microbial metabolism was mainly limited by C, while in the later stage of vegetation restoration (>13 years) soil microbial metabolism was mainly limited by P. Yang et al. (2020) found that with the increase of restoration years of degraded grassland, ln (BG):ln (NAG + LAP):ln (AP) gradually increased, while ln (NAG + LAP): LN (AP) gradually decreased. Soil microorganisms were jointly limited by N and P, but gradually changed from P limitation to N limitation. Moreover, the intensity of N limitation increases with the increase of recovery years. However, the regulation of limitations on soil microbial metabolism, as well as the mechanisms of metabolic responses to biotic and abiotic factors during the restoration of degraded ecosystems, remain poorly understood because of the complexity of soil microbial metabolic limitations.
The Horqin Sandy Land in northern China was formerly a grassland with abundant water and lush vegetation dominated by palatable grass species and sparsely scattered woody species. However, desertification began at the end of the 19th century, when the area was reclaimed for agriculture and livestock during the Qing Dynasty (1,644–1912), which resulted in a population boom that drove widespread grazing and crop cultivation (Zhou et al., 2008). Especially since the 1950s–1970s, more severe desertification has occurred due to a combination of the region’s fragile ecology and inappropriate human activities, such as overgrazing, large-scale intensive farming, firewood cutting, and excessive groundwater extraction (Li et al., 2014).
To control desertification, a variety of restoration practices have been implemented since the 1980s, primarily through the establishment of grazing prohibition and returning degraded farmland to forests and grasslands. Desertification has gradually reversed since 2000 as land’s degraded vegetation has recovered, resulting in a variety of landscape types (Figure 1). These sites illustrate a landscape evolution gradient: 1) mobile dunes with successional pioneer species, which gradually recover to 2) semimobile dunes colonized primarily by small sand-fixing shrubs, 3) semifixed dunes dominated by annual herbaceous species, 4) fixed dunes dominated by perennial and annual herbs, and 5) sparse forest meadows, which represent the climax community in the Horqin Sandy Land (Zhang et al., 2005; Zuo et al., 2012; Lv et al., 2021). This region’s unique features provide an ideal platform for studying microbial nutrient limitations after vegetation restoration. However, most previous studies focused on soil nutrients (Li et al., 2015; Zuo et al., 2015; Lv et al., 2021), soil microbial composition (Wang et al., 2016), or plant functional traits (Zuo et al., 2016a; b) along a gradient of degraded land restoration, with little information on microbial metabolic limitations or the combined effects of soil elements (e.g., C, N, P), extracellular enzymes, and microorganisms along a sandy land restoration gradient.
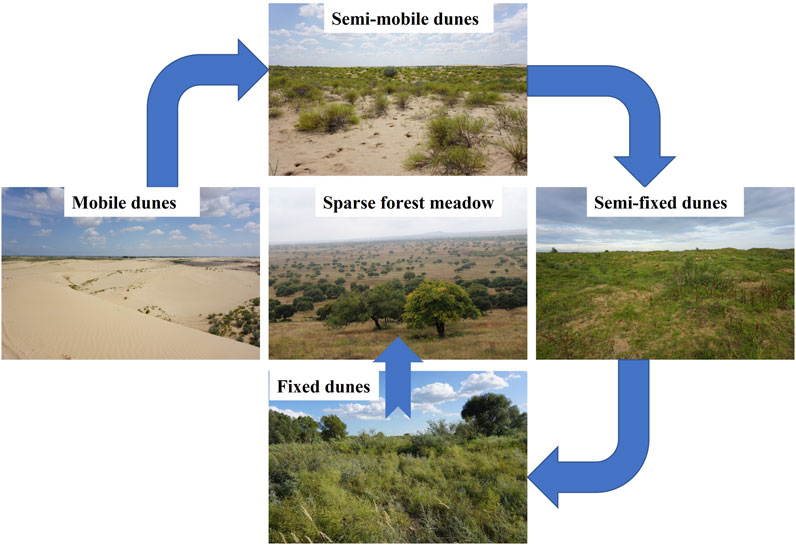
FIGURE 1. Five landscape types in different restoration stages in the Horqin Sandy Land, including (1) mobile dunes with successional pioneer species, which gradually recover to (2) semi-mobile dunes colonized primarily by small sand-fixing shrubs, (3) semi-fixed dunes dominated by annual dominant herbaceous species, (4) fixed dunes dominated by perennial and annual herbs, and (5) sparse forest meadows, which represent the climax community in the study area. The blue arrows represent the direction of vegetation restoration.
We treated each succession stage as a separate landscape type and investigated soil microbial nutrient limitations and their influencing mechanisms during grassland restoration in northern China’s Horqin Sandy Land. The goals of the study were to test three hypotheses. We hypothesized that (1) the activities of C-, N-, and P-acquiring enzymes would increase as nutrient demand increases along the vegetation restoration gradient and that increased EEA would accelerate nutrient cycling to meet the increasing nutrient demand; (2) the microbial C and N limitation would decrease in response to increasing plant C input with vegetation restoration; and (3) variations in soil EEA stoichiometry would result from differences in the vegetation, soil, microorganism communities, and other factors.
2 Materials and methods
2.1 Study area
The Horqin Sandy Land study area is a typical semiarid sandy land in northern China (Figure 2A). It is a temperate steppe of the Central Asian steppe ecosystem, with a continental semiarid monsoon temperate climate. The elevation ranges from 90 m to 1,625 m above sea level. The mean annual temperature ranges from 3°C to 7°C. Monthly mean temperatures range from −12.6°C to −16.8°C in January and 20.3°C–23.5°C in July. The frost-free period lasts 140–160 days per year. The annual average precipitation is 350–500 mm, with the maximum precipitation during the summer, from June to August (Duan et al., 2014).
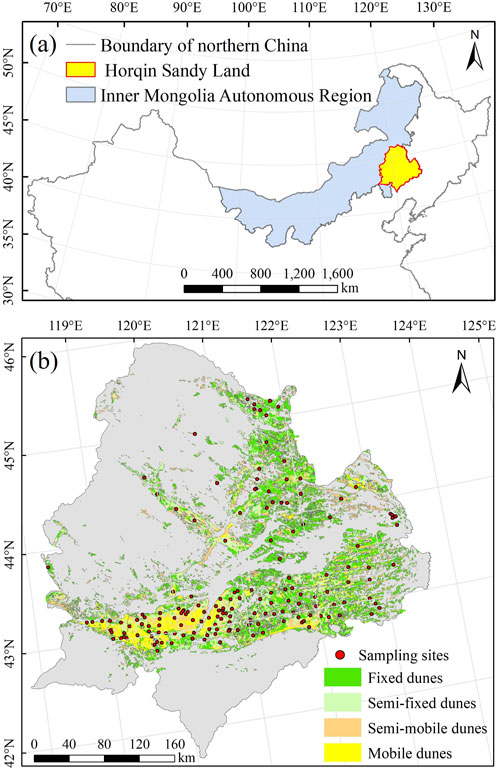
FIGURE 2. (A) Location of the Horqin Sandy Land in the Inner Mongolia Autonomous Region of northern China. (B) Locations of the sampling sites in the Horqin Sandy Land.
The zonal soils are classified as Kastanozems, Chernozems, and Luvisols according to the Food and Agriculture Organization of the United Nations (FAO, 2006). However, due to desertification, Arenosols are currently the dominant soil. The native vegetation are sparse forest meadows, mainly dominated by meso-xerophytes, which include delicate herbs and sparse woody plants (Li et al., 2017). However, extensive desertification has severely degraded the vegetation community over the past century because of a combination of climate change and unsustainable land use. As a result, xerophytes and psammophytes have come to dominate the area (Liu et al., 1996). Supplementary Table S1 presents the key species in each stage of restoration (Yu and Chen, 2007).
2.2 Experimental design and sampling
We used the “space for time” method to investigate the evolution of vegetation restoration. This chronosequence scheme has been proposed for application in vegetation restoration research to monitor plants and soils under similar climatic conditions by following the sequence of vegetation development (Bhojvaid and Timmer, 1998). We selected five landscape types (mobile dunes, semimobile dunes, semifixed dunes, fixed dunes, and sparse forest meadows) to represent different restoration stages of the Horqin Sandy Land (Figure 1). Based on a classification map for the degree of desertification of the Horqin Sandy Land, we defined the specific classification criteria for desertification shown in Supplementary Table S1. We obtained RGB images of the UAV plots by using a Sony EXMOR Sensor mounted on a DJI Phantom 3 Professional (DJI Innovation Company Inc., Shenzheng, China) and used ImageJ software to measure vegetation cover (Ricotta et al., 2014; Lian et al., 2022). The samples of the surface soil (0–10 cm) were collected at 163 sites (to a depth of 10 cm) from July to August 2021 (Figure 2B). At each site, we first established a 10 m × 10 m plot, removed surface debris and then collected the soil samples randomly at 15 sampling points within each plot using a 5-cm diameter soil auger. The soils collected from each plot were combined to form a single composite sample. The collected soil samples were stored at 4°C in a portable refrigerator during field sampling. When sent to the lab we used a 2 mm screen to remove roots and other debris from all samples. Then the samples were separated into two subsamples: One subsample was used to measure soil microbial and enzyme activity, and the other was air-dried at room temperature to determine soil physical and chemical properties. To estimate the dry bulk density, we obtained three undisturbed soil cores using a soil bulk density sampler with a stainless steel cylinder (100 cm3 volume) at each site, which we dried for 48 h at 105°C for the bulk density calculation.
In addition, five quadrats (1 m × 1 m) were separately selected in each of the four corners and center of the plot (10 m × 10 m). In each quadrat, all the aboveground parts of the green plants were chopped off, gathered from the ground, placed in envelopes, and marked with tags. Because the biomass samples were large, they were weighed fresh, and a part of each sample was dried and weighed. The ratio of dry weight to fresh weight was multiplied by the fresh weight to determine the samples’ aboveground biomass (AGB).
2.3 Environmental variables
A suite of 9 environmental variables were collected to clarify the regional-scale factors controlling the spatial pattern of soil microbial metabolic limitations in the sandy ecosystems. Environmental variables include meteorological factors, topographic factors and vegetation index, specifically include the mean annual temperature (MAT) and mean annual precipitation (MAP) data with a spatial resolution of 1 km for 2021, the normalized difference vegetation index (NDVI) dataset during the growing season (April to September) in 2021, and the corresponding elevation, slope, ground roughness, and profile curvature. The source of the above dataset can be found in our previous article (Li et al., 2023; Wang et al., 2023). All the environmental variables mentioned above were uniformly converted into raster data with a spatial resolution of 500 m and extracted to our actual sampling points using the Extract Multi Values to Points geoprocessing tool, which extracts cell values from one or more rasters to a point feature class, in version 10.3 of ArcMap GIS software (http://www.esri.com).
2.4 Soil physical and chemical properties
The Walkley–Black method (Nelson and Sommers, 1982), Kjeldahl method (Bremner, 1996) and Olsen method (Olsen and Sommers, 1982) was used to determine the SOC, total N (TN) and total P content (TP), respectively. We determined the soil pH and electric conductivity (EC) at soil:water ratios of 1:2.5 v/v and 1:5 v/v, respectively, using pH and EC meters (PHS-3C, Puchun, Shanghai, China). The bulk soil samples were dried at 105°C for 24 h to determine the bulk density (BD) (Blake and Hartge, 1986). Wet sieving was used to separate the soil sample into four fractions: coarse sand (>0.25 mm), fine sand (0.10–0.25 mm), very fine sand (0.05–0.10 mm), and clay and silt (<0.05 mm) (Li et al., 2012). We used the chloroform fumigation–extraction method to determine the C, N, and P contents of the microbial biomass (Brookes et al., 1982; 1985; Vance et al., 1987). The amounts of bacteria and fungi were determined by the quantitative real-time polymerase chain reaction (qPCR) amplification technique to identify the abundance and diversity of soil community. The abundance and diversity calculations of soil microbial communities are described in our previous study (Duan et al., 2022).
2.5 Measurement of soil enzyme activity
Using 7-amino-4 methylcoumarin and 4-methylumbelliferone as fluorescent substrates, and standard fluorometric technique was used to measure the activities of the soil C-acquiring enzymes (BG and CBH), N-acquiring enzymes (NAG and LAP), and P-acquiring enzyme (AP) (Saiya-Cork et al., 2002; Sinsabaugh et al., 2008; 2009; Steinweg et al., 2012). The enzymatic activity was expressed in units of nmol activity g-1 dry soil h-1, and the ratios of C-, N-, and P-acquiring enzymes were calculated to express the soil EEA stoichiometry (Sinsabaugh et al., 2008):
2.6 Microbial metabolic limitation
Sinsabaugh et al. (2008) noted that the relative activities of C- vs. P-acquiring enzymes and C- vs. N-acquiring enzymes may reflect soil microbial nutrient limitations (N or P). Vector analysis of the soil EEA stoichiometry was proposed by Moorhead et al. (2016) to evaluate microbial nutrient limitations. In this method, the vector length quantifies relative C vs. N or P nutrient limitation, and the angle quantifies the relative P vs. N limitation. The vector length and angle were calculated as follows:
where x represents the relative C- vs. P-acquiring enzyme activities (i.e., [BG + CBH]/AP) and y represents the relative C- vs. N-acquiring activities (i.e., [BG + CBH]/[LAP + NAG]). Soil microbial C limitation is thought to increase with increasing vector lengths, whereas soil microbial N or P limitation is represented by vector angles: angles less than 45° indicate N limitation, and angles greater than 45° indicate P limitation (Moorhead et al., 2013). The P limitation increases as the angle increases, whereas the N limitation increases as the angle decreases (Moorhead et al., 2016).
2.7 Statistical analysis
First, we tested for heterogeneity of variance, and if necessary, we log-transformed or standardized the data (using z scores) before statistical analysis. We used one-way analysis of variance to test for significant differences in soil enzyme activities and enzymatic stoichiometry among the sandy landscapes, followed by least-significance-difference (LSD) tests for multiple comparisons (p < 0.05). We used Pearson’s correlation coefficient (r) to evaluate the relationships among the influencing factors and soil EEA. The redundancy analysis (RDA) was applied to determine the relative contributions of the influencing factors to the microbial metabolic limitation. RDA analysis was performed in version 5.0 of Canoco software (http://www.canoco5.com/).
3 Results
3.1 Soil C, N, and P content and microbial biomass C, N, and P
The SOC, TN, TP, and the soil microbial biomass C, N, and P (MBC, MBN, and MBP, respectively) increased significantly (p < 0.05) along the landscape evolution gradient from mobile dunes to sparse forest meadows (Table 1), whereas the soil C:N decreased significantly (p < 0.05). In addition, the C:P and N:P ratios increased significantly from mobile dunes to fixed dunes and then decreased (but not significantly) from fixed dunes to sparse forest meadows. Compared with mobile dunes, SOC content in semi-mobile dunes, semi-fixed dunes, fixed dunes and sparse forest meadows was increased by 49.40%, 149.40%, 268.07% and 437.95%, respectively. MBC content was increased by 285.94%, 552.11%, 1,174.17% and 2,330.33%, respectively. In addition, the MBC:MBN, MBC:MBP, and MBN:MBP ratios were lowest in mobile dunes and highest in sparse forest meadows.
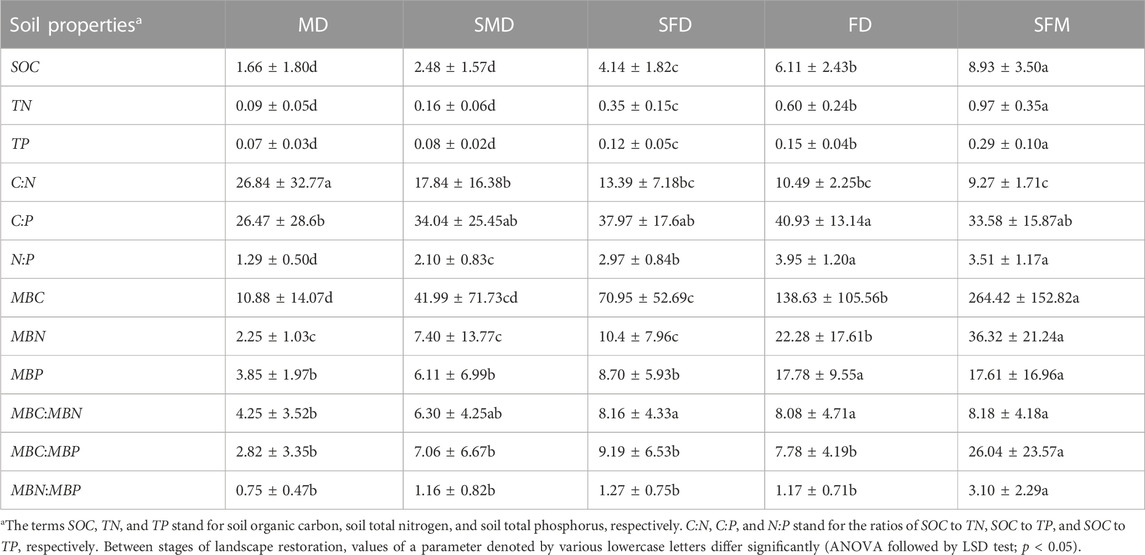
TABLE 1. Changes in soil characteristics brought on by vegetation restoration during the evolution of a landscape. Land types: MD, mobile dunes; SMD, semi-mobile dunes; SFD, semi-fixed dunes; FD, fixed dunes; SFM, sparse forest meadows.
3.2 Variations in soil EEA and EEA stoichiometry along the landscape restoration gradient
Figure 3 shows that the restoration of sandy land significantly (ANOVA followed by LSD test, p < 0.05) affected soil EEA (BG + CBH, NAG + LAP, AP). With increasing vegetation restoration, the activities of the C-acquiring enzymes (BG + CBH) increased significantly from mobile dunes (131.31 nmol g−1 h−1, mean ± SE) to sparse forest meadows (1992.01 nmol g−1 h−1). The N-acquiring enzymes (NAG + LAP) and P-acquiring enzyme (AP) followed the same pattern. The activity of the N-acquiring enzymes (NAG + LAP) was lowest in mobile dunes (243.39 nmol g−1 h−1) and highest in sparse forest meadows (724.33 nmol g−1 h−1). The activity of the P-acquiring enzyme (AP) was lowest in mobile dunes (15.57 nmol g−1 h−1) and highest in sparse forest meadows (1,036.86 nmol g−1 h−1). The enzyme C:N ratio calculated by ln (BG + CBH)/ln (NAG + LAP) was significantly lower in mobile dunes than at the other stages of restoration, which did not differ significantly. The enzyme C:N ratio in mobile dunes was less than 1 versus values greater than 1 at the other stages. In contrast, both the enzyme C:P ratio calculated by ln (BG + CBH)/ln (AP) and the N:P ratio calculated by ln (NAG + LAP)/ln (AP) were highest in the mobile dunes.
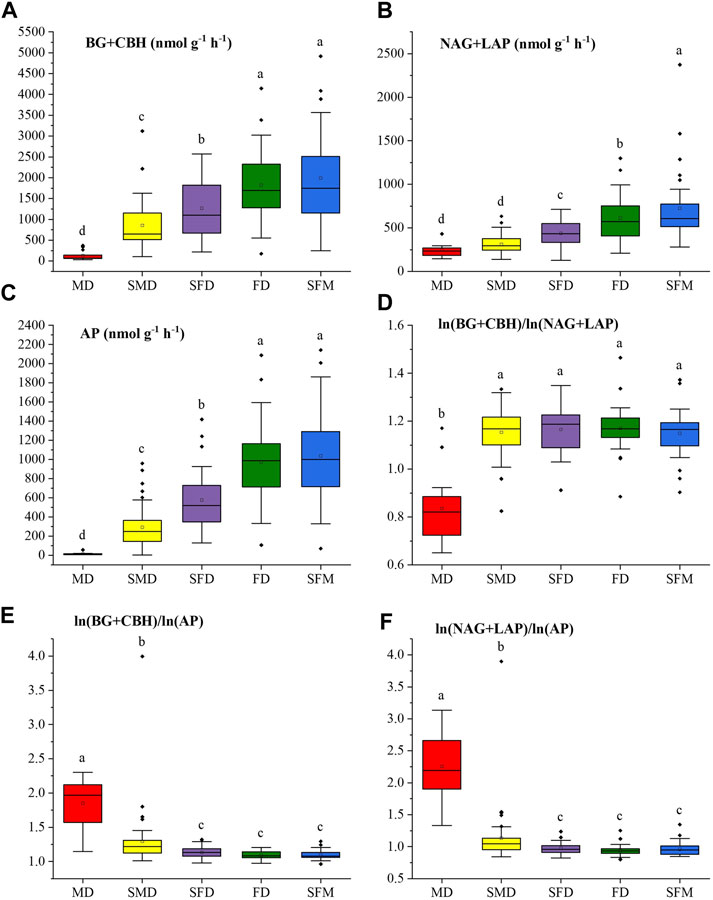
FIGURE 3. Variations of soil enzymatic activity and enzymatic stoichiometry at different stages of vegetation restoration: (A) C-acquiring enzymes (BG + CBH); (B) N-acquiring enzymes (NAG + LAP); (C) the P-acquiring enzyme (AP); and the logarithmic ratios (D) (BG + CBH)/(NAG + LAP); (E) (BG + CBH)/AP; (F) (NAG + LAP)/AP. Values of a parameter followed by different lowercase letters differ significantly among landscape restoration stages (ANOVA followed by LSD test; p < 0.05).
3.3 Effects of vegetation restoration on soil microbial metabolic limitations
We used vector analysis (vector length and vector angle) of the soil EEA stoichiometry data to evaluate microbial nutrient limitations. The microbial C limitation was represented by the vector length, with the microbial C limitation decreasing significantly (ANOVA followed by LSD test; p < 0.05) along the vegetation restoration gradient (Figure 4A). The vector angles in mobile dunes and semimobile dunes were less than 45°, indicating an N deficiency, but more than 45° in semifixed dunes, fixed dunes, and sparse forest meadows, indicating a P deficiency. Thus, during vegetation restoration in the Horqin Sandy Land, N limitation gradually weakened and P limitation gradually increased. Soil microorganisms suffer from N limitation in the initial stages of vegetation restoration (mobile dunes and semimobile dunes), but soil microorganisms in semifixed dunes, fixed dunes, and sparse forest meadows were mainly limited by P. That is, there was a transition from N limitation to P limitation for microorganisms during vegetation restoration in the Horqin Sandy Land.
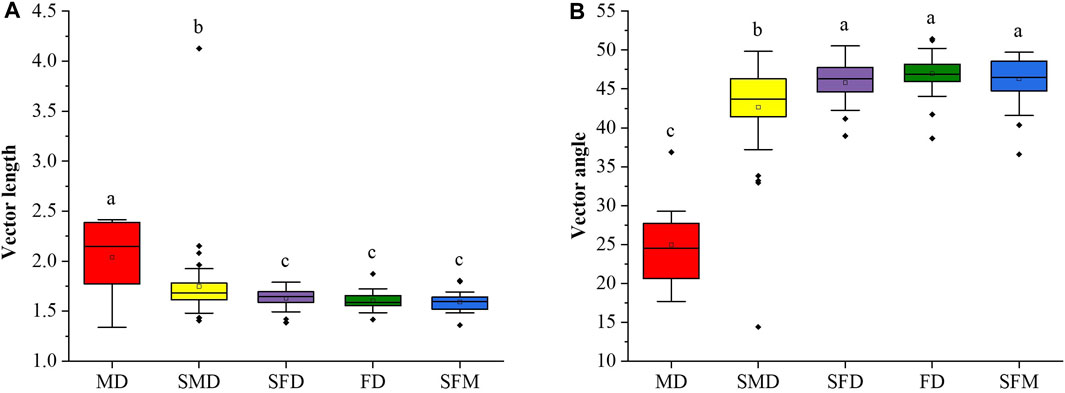
FIGURE 4. Variations of the (A) soil microbial C limitation and (B) soil microbial N or P limitation at different stages of vegetation restoration. Soil microbial C limitation is represented by vector length, and the strength of the limitation increases with increasing length. Soil microbial N or P limitation is represented by the vector angle: angles <45° represent N limitation and angles >45° represent P limitation. Values of a parameter followed by different lowercase letters differ significantly among landscape restoration stages (ANOVA followed by LSD test; p < 0.05).
Similarly, the majority of the mobile dune and semimobile dune study sites appeared on the lower right side of the graph of the ratios of C to N enzyme activities to the ratios of C to P enzyme activities (Figure 5); that is, the angles are <45°, indicating that soil microbial activity was primarily limited by N rather than P during these restoration stages. In contrast, most of the semifixed dune, fixed dune, and sparse forest meadow study sites appeared on the upper left side of the graph, at angles >45°, indicating that soil microbial activities were primarily limited by P rather than N during these restoration stages. Furthermore, we found a statistically significant negative correlation (r = −0.64, p < 0.001) between the two ratios; that is, as the soil microbial C limit increased, the soil microbial N or P limit decreased (Figure 6).
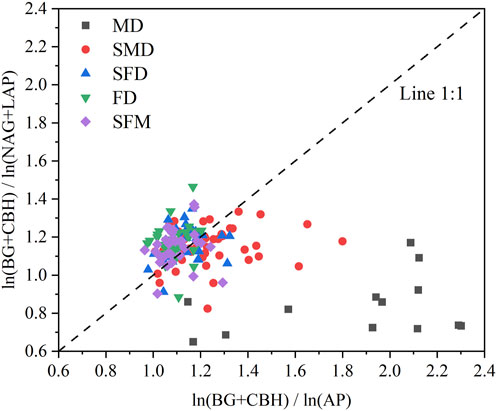
FIGURE 5. The pattern of microbial resource limitation illustrated by a scatterplot of the soil enzymatic stoichiometries. Soil microbial C limitation is represented by vector length. Vector angles represent soil microbial N or P limitation: angles less than 45° represent N limitation, and angles greater than 45° represent P limitation.
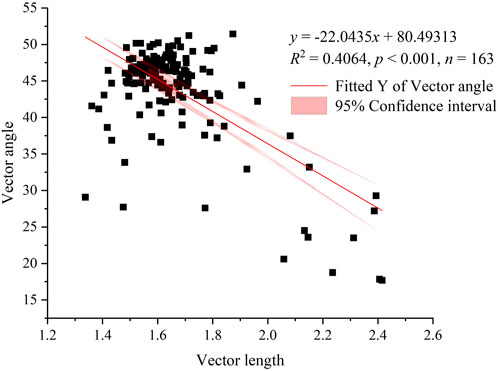
FIGURE 6. Linear regression results for the relationship between soil microbial C limitation (vector length) and microbial N or P limitation (vector angle). Note: the red line indicates the results of linear regression, and the pink shading represents the 95% confidence interval of the linear model.
4 Discussion
4.1 Changes in soil enzyme activities during vegetation restoration
Soil extracellular enzymes are thought to be sensitive and reliable indicators of vegetation restoration (Yu et al., 2019). In our study, the activities of C-acquiring enzymes (BG + CBH), N-acquiring enzymes (NAG + LAP), and the P-acquiring enzyme (AP) increased during the restoration of the sandy grassland from mobile dunes to sparse forest meadows, which supports our first hypothesis that the activities of the C-, N-, and P-acquiring enzymes would increase as the nutrient demand increases with increasing vegetation restoration. This finding is consistent with previous findings for grasslands (Peng and Wang, 2016; Zhang et al., 2016; He et al., 2020; Wang et al., 2020), abandoned farmland (Cui et al., 2018; Cui et al., 2019 Y.; Yang et al., 2020), and desert ecosystems (Liu et al., 2017). The soil at our sites had low levels of nutrients and microbial biomass in the early stages of grassland restoration (i.e., mobile and semimobile dunes) (Table 1), and the majority of the vegetation was annual herbaceous plants with shallow roots. As a result of the limited availability of an organic C substrate, microorganisms’ ability to perform exoenzymatic synthesis was low, ultimately limiting soil EEA (Liu et al., 2018; Li et al., 2020). However, as vegetation restoration progressed, plant biomass increased significantly and increasingly competed with microorganisms for soil resources (Bardgett et al., 2005). Furthermore, fungal activity increased significantly, necessitating more nutrients to meet fungal growth requirements (Fanin et al., 2016). As a result, soil microorganisms began to secrete more C-, N-, and P-acquiring enzymes.
The C:N:P ratios of soil enzymes represent the biochemical balance between soil microbial nutrient allocation and resource availability (Xiao et al., 2018). A meta-analysis of data from 40 ecosystems around the world confirmed that the ratio of C:N:P acquisition enzymes in the topsoil was close to 1:1:1 (Sinsabaugh et al., 2009), indicating that microorganisms have a broad pattern of maintaining stoichiometric homeostasis of C, N, and P across a range of ecosystems. However, due to the wide variation in ecosystems and regional environmental conditions, this equilibrium pattern is easily disrupted (Xiao et al., 2020; Zhou et al., 2020). For instance, the average C:N:P ratio of soil enzymes was approximately 1:1:1 in forest ecosystems along the north–south transect in eastern China (Xu et al., 2017), but subsequent national-scale analysis results revealed that the ratio of C:N:P acquisition enzymes in Chinese forests averaged 1:1.4:2.6, deviating from the 1:1:1 ratio (Sinsabaugh et al., 2009; Zhou et al., 2020). The ratios of C:N:P acquisition enzymes were 1:1.2:1.4 and 1:1.08:1.28 in temperate grassland ecosystems of northern China and the Loess Plateau of China (Peng and Wang, 2016; Yang et al., 2020), respectively, indicating that C-acquiring enzyme activities were lower than N- and P-acquiring enzyme activities. In the present study, the average ratio of C:N:P acquisition enzymes was 1:0.88:0.86, suggesting (1) higher C-acquiring enzyme activities than N- or P-acquiring enzyme activities and (2) lower P-acquiring enzyme activity than that of N- or C-acquiring enzymes, which is inconsistent with the abovementioned results.
4.2 Microbial nutrient limitations revealed by soil enzyme stoichiometry
We found that the vector length decreased with vegetation restoration from mobile dunes to sparse forest meadows, indicating that microbial C limitation decreased during grassland restoration, whereas the activity of C-acquiring enzymes (BG + CBH) increased significantly with increasing vegetation restoration, which suggests that there was an opposite trend between microbial C limitation and the activity of soil C-acquiring enzymes. This is mainly due to increasing plant C inputs to the soil (e.g., litter, roots), which mitigated the microbial C limitation that developed during vegetation restoration (Ruiz-Jaén and Aide, 2005; Deng et al., 2013) but did not reduce the demand for soil carbon. In addition, when soil C availability is severely limited, soil microorganisms are likely to be dormant until C limitation is alleviated, implying that soil microorganisms generally do not produce more extracellular enzymes associated with C acquisition until the limitation is alleviated (Sinsabaugh and Follstad Shah, 2012; Blagodatskaya and Kuzyakov, 2013).
In addition, mobile dunes had a significantly lower vector angle than the other restoration stages (Figure 4B), which suggests that soil microorganisms are more highly limited by N in the mobile dunes. The vector angles were less than 45° in the mobile and semimobile dunes but greater than 45° in the semifixed dunes, fixed dunes, and sparse forest meadows, which supports our second hypothesis that grassland restoration would reduce the microbial C and N limitation but cause a shift from N limitation to P limitation from semi-mobile dunes to semi-fixed dunes. It is worth noting that the shift from N limitation to P limitation of microorganisms between semimobile dunes and semifixed dunes indicates that vegetation restoration requires more P than N at this stage, which leads to the secretion of more P-acquiring enzymes than N-acquiring enzymes, resulting in a reduction in the enzyme N:P ratio as vegetation restoration progresses in sandy land (Figure 2).
Due to the sparse vegetation cover of mobile and semimobile dunes and the dominance of annual herbaceous plants with shallow roots in their vegetation communities, fewer exogenous nutrients were available for microorganisms. In contrast, plants growing on semifixed and fixed dunes or in sparse forest meadows have relatively large root biomass, which provides more exogenous resources for microorganisms (Cui et al., 2018; Cui et al., 2019 YX.). This is reflected by increasing soil C and N contents with increasing vegetation restoration (Table 1), but this increase was not synchronous; that is, the increase in soil C lagged behind the increase in soil N, resulting in a continuous decrease in the soil C:N ratio. This may increase soil N availability (Waring et al., 2014; Zhao et al., 2018; Liu et al., 2019; Rosinger et al., 2019; Zhang et al., 2019; Zhou et al., 2020), eventually leading to weakening of the soil microbial nitrogen limitation in the later stages of vegetation restoration in sandy land.
On the other hand, as restoration proceeded from semimobile dunes to sparse forest meadows, the soil P content increased significantly (Table 1), and the soil microbial biomass significantly improved (Table 1) along with the obvious increase in plant biomass accumulation, which increased the competition for soil P. Soil microbial activity increases nutrient requirements to sustain the high growth rates (Sterner and Elser, 2002), but the limited input of soil P due to the relatively low plant biomass resulted in an increased microbial P limitation. Previous research has also found increased P limitation in soils during the late stages of vegetation restoration on the Loess Plateau (Jiao et al., 2013; Ren et al., 2016; Deng et al., 2019b), particularly in areas where soil erosion has accelerated soil P loss (Feng et al., 2013). Based on the discussion in this section, our results demonstrate a shift from N to P limitation during vegetation restoration in sandy land.
4.3 Factors affecting soil microbial metabolic limitation during vegetation restoration
Both aboveground biomass and the normalized difference vegetation index (NDVI) were significantly negatively correlated with microbial C limitation but significantly positively correlated with microbial N or P limitation (Supplementary Table S2), mainly due to improvement of the soil C pool by increased plant C inputs, such as litter and root turnover, during vegetation restoration, thus reducing the C demand of the soil microorganisms (Zhang et al., 2011; Deng and Shangguan, 2017; Deng et al., 2018). Similarly, the SOC, TN, and TP exhibited a significant negative correlation with microbial C limitation but a significant positive correlation with microbial N or P limitation, confirming that vegetation restoration can provide more C and nutrient sources for soil microorganisms (Zhu et al., 2010), thereby reducing the microbial communities’ C limitation (Cui YX. et al., 2019).
In addition, the soil microbial community structure and composition significantly affected microbial metabolic limitations. We found that MBC, MBN, and MBP were significantly negatively correlated with microbial C limitation but significantly positively correlated with microbial N or P limitation (Supplementary Table S2). According to the growth rate hypothesis (Sterner and Elser, 2002), the increase in soil microbial activity is related to the high demand for P to support the synthesis of ribosomal RNA, so MBP had a strong direct positive impact on microbial phosphorus limitation. Previous research has also confirmed that the relative abundance of soil microorganisms is highly sensitive to changes in the soil C:N:P ratio (Turner and Haygarth, 2005; van der Heijden et al., 2008). Fundamental differences in bacterial and fungal growth forms and living strategies can influence C:N:P demand, and the ratio responds to changes in microbial community diversity (Güsewell and Gessner, 2009; Strickland and Rousk, 2010). The physiological metabolisms of these microorganisms can be further altered by the secretion of extracellular enzymes to acquire nutrients to support the organism’s growth (Sinsabaugh et al., 2009; Schneider et al., 2012). For instance, EEA may be higher in soils with larger fungal populations due to the long hyphal lengths (Burke et al., 2011; Kivlin and Treseder, 2014). In our study, we discovered that both bacterial community diversity indices were significantly negatively correlated with C limitation but significantly positively correlated with N or P limitation, whereas the fungal community diversity index was only significantly negatively correlated with N or P limitation (Supplementary Table S2). It is unknown whether the production of some enzymes is differentially controlled by different groups of soil microorganisms, and answering this question is critical to understanding the role of the microbial community structure in ecosystem functioning (Fanin et al., 2016); this will require additional research.
Figure 7 shows the RDA ordination results of the soil microbial C limitation and microbial N or P limitation and the influencing factors. The cumulative percentage of the variance for the dependent variables, including the soil microbial C limitation (MCL) and microbial N or P limitation (MNPL), explained by the influencing factors totaled 44.6% for the first two axes. Soil microbial N or P limitation was positively associated with N:P, the soil microbial factors (i.e., MBN, MBC:MBN and MBC:MBP) and NDVI. The soil microbial C limitation exhibited the opposite relationships. Both MCL and MNPL were negatively correlated with the Chao1 richness estimator for fungi, and the fungus-to-bacteria ratio (F/B) was positively associated with MCL but negatively correlated with MNPL. Furthermore, we used a partial Monte Carlo permutation test to evaluate the contributions of each factor to the variation in MCL and MNPL (Table 2). N:P explained the largest proportion of the variation in soil microbial metabolic limitations (18.0%) and had the highest contribution rate (35.7%). The second-most important factor was MBC:MBN, which explained 5.5% of the variation and contributed 11.0%. The influences of the other factors were statistically significant but much smaller (≤5% of the variation explained). This indicates that N:P was the dominant driver for the soil microbial metabolic limitation in the semiarid sandy study area.
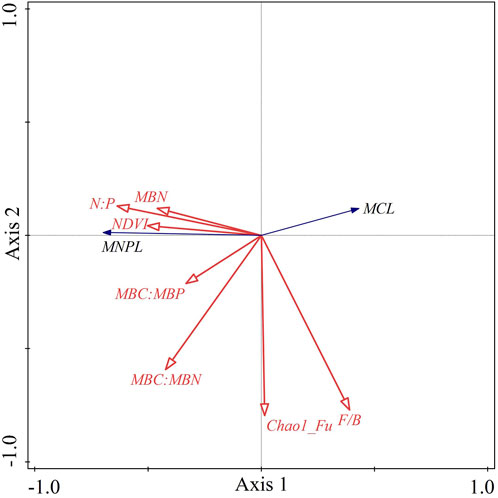
FIGURE 7. Redundancy analysis ordination diagram for soil microbial C limitation (MCL) and soil microbial N or P limitation (MNPL) and the associated influence factors. Note: N:P, the ratio of total nitrogen to total phosphorus; MBN, microbial biomass nitrogen; MBC:MBN, the ratio of microbial biomass carbon to microbial biomass nitrogen; MBC:MBP, the ratio of microbial biomass carbon to microbial biomass phosphorus; NDVI, normalized-difference vegetation index; Chao1_Fu, the Chao1 richness estimator for fungi; F/B, fungus-to-bacteria ratio.
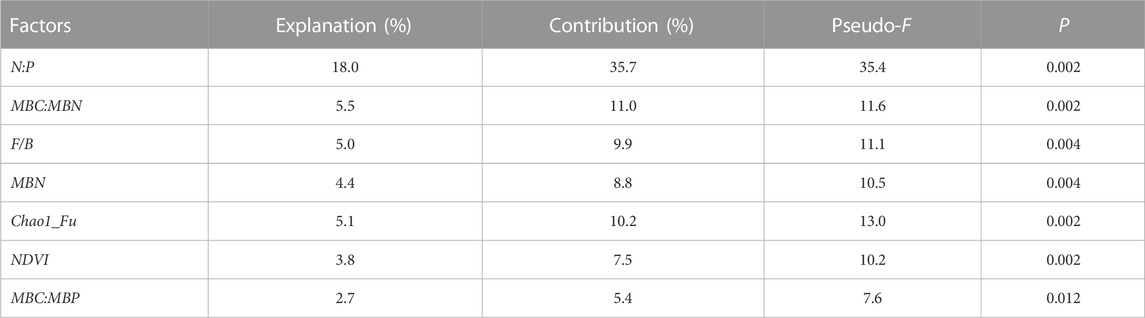
TABLE 2. The percentages of variation explained (the explanation rate) and contributions (based on redundancy analysis) of the influencing factors to variation of the soil microbial N or P constraint as well as soil microbial C limitation in the redundancy analysis. Abbreviations: N:P stands for the total nitrogen to total phosphorus ratio; MBC, MBN, and MBP stand for microbial biomass carbon, nitrogen, and phosphorus, respectively; NDVI, the normalized-difference vegetation index; Chao1_Fu, the Chao1 richness estimator for fungi; F/B, fungus-to-bacteria ratio.
5 Conclusion
Through analyses of microbial metabolism and plant–microbe nutrient competition, we confirmed that during vegetation restoration in the Horqin Sandy land, the soil microbial C limitation gradually decreased. In contrast, soil microbial N or P limitation increased over time, and the limitation shifted from N limitation to P limitation as the P limitation increased in strength from mobile to semimobile dunes. The effect of vegetation restoration on microbial C limitation and N or P limitation resulted from a combination of effects induced by plants, soils, extracellular enzymes, and microorganisms. Plant C input significantly increased the soil nutrient content and decreased the microbial C limitation but increased the microbial N or P limitation due to competition between plants and soil microorganisms for nutrients. In addition, the decrease in the fungi:bacteria ratio (to a value less than 1) strengthened the microbial N or P limitation. This suggests that the number of fungi could be increased artificially in future restoration projects to alleviate microbial nutrient limitations during vegetation restoration. Our results provide an indirect theoretical basis for the sustainable management of ecological restoration. In the process of vegetation restoration in semiarid sandy land, it is necessary to replenish vegetation C input in the mobile dune stage. Due to N limitation in semimobile dunes, attention should be given to nitrogen fertilizer supplementation in this stage. To accelerate and maintain the vegetation restoration of semifixed dunes and fixed dunes, phosphorus fertilizer should be added in a timely manner. However, our study will be replicated in a different area of sandy land in subsequent research to confirm whether the results are widely applicable.
Data availability statement
The original contributions presented in the study are included in the article/Supplementary Material, further inquiries can be directed to the corresponding authors.
Author contributions
BY: Formal Analysis, Writing–original draft, Data curation, Investigation, Methodology, Software, Visualization. XW: Methodology, Writing–original draft, Data curation, Formal Analysis, Funding acquisition, Investigation, Project administration, Visualization. YgL: Funding acquisition, Methodology, Writing–review and editing. JL: Data curation, Methodology, Writing–review and editing. YnL: Data curation, Writing–review and editing. YLuo: Funding acquisition, Methodology, Writing–review and editing. YlL: Methodology, Writing–review and editing.
Funding
The author(s) declare financial support was received for the research, authorship, and/or publication of this article. This research was supported by the National Natural Science Foundation of China (grants 32001214, 31971466 and 42275132) and the Youth Innovation Promotion Association of Chinese Academy of Sciences (2023449).
Conflict of interest
The authors declare that the research was conducted in the absence of any commercial or financial relationships that could be construed as a potential conflict of interest.
Publisher’s note
All claims expressed in this article are solely those of the authors and do not necessarily represent those of their affiliated organizations, or those of the publisher, the editors and the reviewers. Any product that may be evaluated in this article, or claim that may be made by its manufacturer, is not guaranteed or endorsed by the publisher.
Supplementary material
The Supplementary Material for this article can be found online at: https://www.frontiersin.org/articles/10.3389/fenvs.2023.1298027/full#supplementary-material
References
Allison, S. D., and Vitousek, P. M. (2005). Responses of extracellular enzymes to simple and complex nutrient inputs. Soil Biol. Biochem. 37, 937–944. doi:10.1016/j.soilbio.2004.09.014
Bardgett, R. D., Bowman, W. D., Kaufmann, R., and Schmidt, S. K. (2005). A temporal approach to linking aboveground and belowground ecology. Trends Ecol. Evol. 20, 634–641. doi:10.1016/j.tree.2005.08.005
Berthrong, S. T., Jobbágy, E. G., and Jackson, R. B. (2009). A global meta-analysis of soil exchangeable cations, pH, carbon, and nitrogen with afforestation. Ecol. Appl. 19, 2228–2241. doi:10.1890/08-1730.1
Bhojvaid, P. P., and Timmer, V. R. (1998). Soil dynamics in an age sequence of Prosopis juliflora planted for sodic soil restoration in India. For Ecol Manag 106, 181–193. doi:10.1016/s0378-1127(97)00310-1
Blagodatskaya, E., and Kuzyakov, Y. (2013). Active microorganisms in soil: critical review of estimation criteria and approaches. Soil Biol. Biochem. 67, 192–211. doi:10.1016/j.soilbio.2013.08.024
Blake, G., and Hartge, K. (1986). in Bulk density. Methods of soil analysis Part I: physical and mineralogical methods. Editor A. Klute (Madison, WI, USA: Soil Science Society of America), 463–478.
Bowles, T. M., Acosta-Martinez, V., Calderon, F., and Jackson, L. E. (2014). Soil enzyme activities, microbial communities, and carbon and nitrogen availability in organic agroecosystems across an intensively-managed agricultural landscape. Soil Biol. Biochem. 68, 252–262. doi:10.1016/j.soilbio.2013.10.004
Bremner, J. (1996). “Nitrogen-total,” in Methods soil anal. Part, 3. Editors D. L. Sparks, A. L. Page, P. A. Helmke, R. H. Loeppert, P. N. Soltanpour, M. A. Tabatabaiet al. (Madison, WI, USA: Soil Science Society of America), 1085–1121.
Brookes, P. C., Landman, A., Pruden, G., and Jenkinson, D. S. (1985). Chloroform fumigation and the release of soil nitrogen: a rapid direct extraction method to measure microbial biomass nitrogen in soil. Soil Biol. Biochem. 17, 837–842. doi:10.1016/0038-0717(85)90144-0
Brookes, P. C., Powlson, D. S., and Jenkinson, D. S. (1982). Measurement of microbial biomass phosphorus in soil. Soil Biol. Biochem. 14, 319–329. doi:10.1016/0038-0717(82)90001-3
Burke, D. J., Weintraub, M. N., Hewins, C. R., and Kalisz, S. (2011). Relationship between soil enzyme activities, nutrient cycling and soil fungal communities in a northern hardwood forest. Soil Biol. Biochem. 43, 795–803. doi:10.1016/j.soilbio.2010.12.014
Burns, R. G., DeForest, J. L., Marxsen, J., Sinsabaugh, R. L., Stromberger, M. E., Wallenstein, M. D., et al. (2013). Soil enzymes in a changing environment: current knowledge and future directions. Soil Biol. Biochem. 58, 216–234. doi:10.1016/j.soilbio.2012.11.009
Cenini, V. L., Fornara, D. A., McMullan, G., Ternan, N., Carolan, R., Crawley, M. J., et al. (2016). Linkages between extracellular enzyme activities and the carbon and nitrogen content of grassland soils. Soil Biol. Biochem. 96, 198–206. doi:10.1016/j.soilbio.2016.02.015
Chen, H., Li, D., Zhao, J., Zhang, W., Xiao, K., and Wang, K. (2018). Nitrogen addition aggravates microbial carbon limitation: evidence from ecoenzymatic stoichiometry. Geoderma 329, 61–64. doi:10.1016/j.geoderma.2018.05.019
Cleveland, C. C., and Liptzin, D. (2007). C: N:P stoichiometry in soil: is there a “Redfield ratio” for the microbial biomass? Biogeochemistry 85, 235–252. doi:10.1007/s10533-007-9132-0
Córdova, S. C., Olk, D. C., Dietzel, R. N., Mueller, K. E., Archontouilis, S. V., and Castellano, M. J. (2018). Plant litter quality affects the accumulation rate, composition, and stability of mineral-associated soil organic matter. Soil Biol. Biochem. 125, 115–124. doi:10.1016/j.soilbio.2018.07.010
Cross, A. F., and Schlesinger, W. H. (2001). Biological and geochemical controls on phosphorus fractions in semiarid soils. Biogeochemistry 52, 155–172. doi:10.1023/a:1006437504494
Cui, Y., Bing, H., Fang, L., Jiang, M., Shen, G., Yu, J., et al. (2019a). Extracellular enzyme stoichiometry reveals the carbon and phosphorus limitations of microbial metabolisms in the rhizosphere and bulk soils in alpine ecosystems. Plant Soil 393, 7–20. doi:10.1007/s11104-019-04159-x
Cui, Y., Fang, L., Guo, X., Wang, X., Zhang, Y., Li, P., et al. (2018). Ecoenzymatic stoichiometry and microbial nutrient limitation in rhizosphere soil in the arid area of the northern Loess Plateau, China. Soil Biol. Biochem. 116, 11–21. doi:10.1016/j.soilbio.2017.09.025
Cui, Y. X., Fang, L. C., Guo, X. B., Han, F., Ju, W. L., Ye, L. P., et al. (2019b). Natural grassland as the optimal pattern of vegetation restoration in arid and semi-arid regions: evidence from nutrient limitation of soil microbes. Sci. Total Environ. 648, 388–397. doi:10.1016/j.scitotenv.2018.08.173
Deng, L., Kim, D. G., Li, M. Y., Huang, C. B., Liu, Q. Y., Cheng, M., et al. (2019a). Land-use changes driven by “Grain for Green” program reduced carbon loss induced by soil erosion on the Loess Plateau of China. Glob. Planet Chang. 177, 101–115. doi:10.1016/j.gloplacha.2019.03.017
Deng, L., Kim, D. G., Peng, C. H., and Shangguan, Z. P. (2018). Controls of soil and aggregate-associated organic carbon variations following natural vegetation restoration on the Loess Plateau in China. Land Degrad. Dev. 29, 3974–3984. doi:10.1002/ldr.3142
Deng, L., Peng, C., Huang, C., Wang, K., Shangguan, Z., Liu, Y., et al. (2019b). Drivers of soil microbial metabolic limitation changes along a vegetation restoration gradient on the Loess Plateau, China. Geoderma 353, 188–200. doi:10.1016/j.geoderma.2019.06.037
Deng, L., and Shangguan, Z. P. (2017). Afforestation drives soil carbon and nitrogen changes in China. Land Degrad. Dev. 28, 151–165. doi:10.1002/ldr.2537
Deng, L., Shangguan, Z. P., and Sweeney, S. (2013). Changes in soil carbon and nitrogen following land abandonment of farmland on the Loess Plateau, China. PLoS One 8, e71923. doi:10.1371/journal.pone.0071923
Deng, Y., Chen, X., Yao, X., Dong, L., Zhang, H., Zeng, H., et al. (2022). No thermal adaptation in soil extracellular enzymes across a temperate grassland region. Soil Biol. Biochem. 165, 108540. doi:10.1016/j.soilbio.2021.108540
Dong, C., Wang, W., Liu, H., Xu, X., and Zeng, H. (2019). Temperate grassland shifted from nitrogen to phosphorus limitation induced by degradation and nitrogen deposition: evidence from soil extracellular enzyme stoichiometry. Ecol. Indic. 101, 453–464. doi:10.1016/j.ecolind.2019.01.046
Duan, H. C., Wang, T., Xue, X., Liu, S. L., and Guo, J. (2014). Dynamics of aeolian desertification and its driving forces in the Horqin sandy land, northern China. Environ. Monit. Assess. 186, 6083–6096. doi:10.1007/s10661-014-3841-3
Duan, Y. L., Wang, X. Y., Wang, L. L., Lian, J., Wang, W. F., Wu, F. S., et al. (2022). Biogeographic patterns of soil microbe communities in the deserts of the Hexi Corridor, northern China. Catena 211, 106026. doi:10.1016/j.catena.2022.106026
Elser, J., Acharya, K., Kyle, M., Cotner, J., Makino, W., Markow, T., et al. (2003). Growth rate-stoichiometry couplings in diverse biota. Ecol. Lett. 6, 936–943. doi:10.1046/j.1461-0248.2003.00518.x
Fanin, N., Moorhead, D., and Bertrand, I. (2016). Eco-enzymatic stoichiometry and enzymatic vectors reveal differential C, N, P dynamics in decaying litter along a land-use gradient. Biogeochemistry 129, 21–36. doi:10.1007/s10533-016-0217-5
FAO (FAO/IUSS Working Group WRB) (2006). “World reference base for soil resources 2006,” in World soil resources report (Rome, Italy: FAO), 103.
Feng, X. M., Fu, B. J., Lu, N., Zeng, Y., and Wu, B. F. (2013). How ecological restoration alters ecosystem services: an analysis of carbon sequestration in China’s Loess Plateau. Sci. Rep. 3, 2846. doi:10.1038/srep02846
Güsewell, S., and Gessner, M. O. (2009). N:P ratios influence litter decomposition and colonization by fungi and bacteria in microcosms. Funct. Ecol. 23, 211–219. doi:10.1111/j.1365-2435.2008.01478.x
He, Q. Q., Wu, Y. H., Bing, H. J., Zhou, J., and Wang, J. P. (2020). Vegetation type rather than climate modulates the variation in soil enzyme activities and stoichiometry in subalpine forests in the eastern Tibetan Plateau. Geoderma 374, 114424. doi:10.1016/j.geoderma.2020.114424
Jiao Fwen, Z. M., An, S. S., and Yuan, Z. Y. (2013). Successional changes in soil stoichiometry after land abandonment in Loess Plateau, China. Ecol. Eng. 58, 249–254. doi:10.1016/j.ecoleng.2013.06.036
Kaiser, C., Franklin, O., Dieckmann, U., and Richter, A. (2014). Microbial community dynamics alleviate stoichiometric constraints during litter decay. Ecol. Lett. 17, 680–690. doi:10.1111/ele.12269
Keiblinger, K., Hall, E., Wanek, W., Szukics, U., Hämmerle, I., Ellersdorfer, G., et al. (2010). The effect of resource quantity and resource stoichiometry on microbial carbon-use-efficiency. FEMS Microbiol. Ecol. 73, 430–440. doi:10.1111/j.1574-6941.2010.00912.x
Kivlin, S. N., and Treseder, K. K. (2014). Soil extracellular enzyme activities correspond with abiotic factors more than fungal community composition. Biogeochemistry 117, 23–37. doi:10.1007/s10533-013-9852-2
Li, J., Shangguan, Z., and Deng, L. (2020). Dynamics of soil microbial metabolic activity during grassland succession after farmland abandonment. Geoderma 363, 114167. doi:10.1016/j.geoderma.2019.114167
Li, Y., Bezemer, T. M., Yang, J., Lü, X., Li, X., Liang, W., et al. (2019). Changes in litter quality induced by N deposition alter soil microbial communities. Soil Biol. Biochem. 130, 33–42. doi:10.1016/j.soilbio.2018.11.025
Li, Y., Brandle, J., Awada, T., Chen, Y., Han, J., Zhang, F., et al. (2013). Accumulation of carbon and nitrogen in the plant–soil system after afforestation of active sand dunes in China's Horqin Sandy Land. Agr. Ecosyst. Environ. 177, 75–84. doi:10.1016/j.agee.2013.06.007
Li, Y., Chen, Y., Wang, X., Niu, Y., and Lian, J. (2017). Improvements in soil carbon and nitrogen capacities after shrub planting to stabilize sand dunes in China’s Horqin Sandy Land. Sustainability 9, 662. doi:10.3390/su9040662
Li, Y., Han, J., Wang, S., Brandle, J., Lian, J., Luo, Y., et al. (2014). Soil organic carbon and total nitrogen storage under different land uses in the Naiman Banner, a semiarid degraded region of northern China. Can. J. Soil Sci. 94, 9–20. doi:10.4141/cjss2013-074
Li, Y., Wang, X., Chen, Y., Gong, X., Yao, C., Cao, W., et al. (2023). Application of predictor variables to support regression kriging for the spatial distribution of soil organic carbon stocks in native temperate grasslands. J. Soil Sediment. 23, 700–717. doi:10.1007/s11368-022-03370-1
Li, Y., Zhao, X., Zhang, F., Awada, T., Wang, S., Zhao, H., et al. (2015). Accumulation of soil organic carbon during natural restoration of desertified grassland in China's Horqin Sandy Land. J. Arid. Land 7, 328–340. doi:10.1007/s40333-014-0045-1
Li, Y. Q., Zhao, X. Y., Chen, Y. P., Luo, Y. Q., and Wang, S. K. (2012). Effects of grazing exclusion on carbon sequestration and the associated vegetation and soil characteristics at a semi-arid desertified sandy site in Inner Mongolia, northern China. Can. J. Soil Sci. 92, 807–819. doi:10.4141/cjss2012-030
Lian, J., Gong, X., Wang, X., Wang, X. Y., Zhao, X., Li, X., et al. (2022). Mapping of soil organic carbon stocks based on aerial photography in a fragmented desertification landscape. Remote Sens. 14, 2829. doi:10.3390/rs14122829
Lino, I. A. N., dos Santos, V. M., Escobar, I. E. C., da Silva, D. K. A., de Araújo, A. S. F., and Maia, L. C. (2016). Soil enzymatic activity in Eucalyptus grandis plantations of different ages. Land Degrad. Dev. 27, 77–82. doi:10.1002/ldr.2454
Liu, H., Mi, Z., Lin, L. I., Wang, Y., Zhang, Z., Zhang, F., et al. (2018). Shifting plant species composition in response to climate change stabilizes grassland primary production. P Natl. Acad. Sci. U. S. A. 115, 4051–4056. doi:10.1073/pnas.1700299114
Liu, L. C., Liu, Y. B., Hui, R., and Xie, M. (2017). Recovery of microbial community structure of biological soil crusts in successional stages of Shapotou desert revegetation, northwest China. Soil Biol. Biochem. 107, 125–128. doi:10.1016/j.soilbio.2016.12.030
Liu, Q., Xu, X., Wang, H., Blagodatskaya, E., and Kuzyakov, Y. (2019). Dominant extracellular enzymes in priming of SOM decomposition depend on temperature. Geoderma 343, 187–195. doi:10.1016/j.geoderma.2019.02.006
Liu, X. M., Zhao, H. L., and Zhao, A. F. (1996). Characteristics of Sandy Environment And Vegetation In the Horqin Sandy Land. Beijing, China: Science Press. (in Chinese with English abstract).
Lv, P., Sun, S., Medina-Roldán, E., Zhao, S., Zuo, X., Guo, A., et al. (2021). Soil net nitrogen transformation rates are co-determined by multiple factors during the landscape evolution in Horqin Sandy Land. Catena 206, 105576. doi:10.1016/j.catena.2021.105576
Manzoni, S., Taylor, P., Richter, A., Porporato, A., and Ågren, G. I. (2012). Environmental and stoichiometric controls on microbial carbon-use efficiency in soils. New Phytol. 196, 79–91. doi:10.1111/j.1469-8137.2012.04225.x
McGuire, K. L., Bent, E., Borneman, J., Majumder, A., Allison, S. D., and Treseder, K. K. (2010). Functional diversity in resource use by fungi. Ecology 91, 2324–2332. doi:10.1890/09-0654.1
Moorhead, D. L., Rinkes, Z. L., Sinsabaugh, R. L., and Weintraub, M. N. (2013). Dynamic relationships between microbial biomass, respiration, inorganic nutrients and enzyme activities: informing enzyme-based decomposition models. Front. Microbiol. 4, 223. doi:10.3389/fmicb.2013.00223
Moorhead, D. L., Sinsabaugh, R. L., Hill, B. H., and Weintraub, M. N. (2016). Vector analysis of ecoenzyme activities reveal constraints on coupled C, N and P dynamics. Soil Biol. Biochem. 93, 1–7. doi:10.1016/j.soilbio.2015.10.019
Mori, T. (2020). Does ecoenzymatic stoichiometry really determine microbial nutrient limitations? Soil Biol. Biochem. 146, 107816. doi:10.1016/j.soilbio.2020.107816
Nelson, D. W., and Sommers, L. E. (1982). “Total carbon, organic carbon and organic matter,” in Methods of soil analysis, Part 2. Editors A. L. Page, R. H. Miller, and D. R. Keeney 2nd (Madison, WI, USA: American Society of Agronomy), 539–577.
Olsen, S. R., and Sommers, L. E. (1982). “Phosphorous,”. Methods of soil analysis, Part 2, chemical and microbial properties. Agronomy monograph. Editors A. L. Page, R. H. Miller, and D. R. Keeney (Madison, Wisconsin: Agronomy Society of America), 403–430.
Paul, K. I., Polglase, P. J., Nyakuengama, J. G., and Khanna, P. K. (2002). Change in soil carbon following afforestation. For Ecol Manag 168, 241–257. doi:10.1016/s0378-1127(01)00740-x
Peng, X. Q., and Wang, W. (2016). Stoichiometry of soil extracellular enzyme activity along a climatic transect in temperate grasslands of northern China. Soil Biol. Biochem. 98, 74–84. doi:10.1016/j.soilbio.2016.04.008
Raiesi, F., and Salek-Gilani, S. (2018). The potential activity of soil extracellular enzymes as an indicator for ecological restoration of rangeland soils after agricultural abandonment. Appl. Soil Ecol. 126, 140–147. doi:10.1016/j.apsoil.2018.02.022
Ren, C. J., Kang, D., Wu, J. P., Zhao, F. Z., Yang, G. H., Han, X. H., et al. (2016). Temporal variation in soil enzyme activities after afforestation in the Loess Plateau, China. Geoderma 282, 103–111. doi:10.1016/j.geoderma.2016.07.018
Ricotta, E. E., Frese, S. A., Choobwe, C., Louis, T. A., and Shiff, C. J. (2014). Evaluating local vegetation cover as a risk factor for malaria transmission: a new analytical approach using ImageJ. Malar. J. 13, 94. doi:10.1186/1475-2875-13-94
Rosinger, C., Rousk, J., and Sandén, H. (2019). Can enzymatic stoichiometry be used to determine growth-limiting nutrients for microorganisms? A critical assessment in two subtropical soils. Soil Biol. Biochem. 128, 115–126. doi:10.1016/j.soilbio.2018.10.011
Ruiz-Jaén, M. C., and Aide, T. M. (2005). Vegetation structure, species diversity, and ecosystem processes as measures of restoration success. For Ecol Manag 218, 159–173. doi:10.1016/j.foreco.2005.07.008
Saiya-Cork, K. R., Sinsabaugh, R. L., and Zak, D. R. (2002). The effects of long term nitrogen deposition on extracellular enzyme activity in an Acer saccharum forest soil. Soil Biol. Biochem. 34, 1309–1315. doi:10.1016/s0038-0717(02)00074-3
Schimel, J. P., and Weintraub, M. N. (2003). The implications of exoenzyme activity on microbial carbon and nitrogen limitation in soil: a theoretical model. Soil Biol. Biochem. 35, 549–563. doi:10.1016/s0038-0717(03)00015-4
Schneider, T., Keiblinger, K. M., Schmid, E., Sterflinger-Gleixner, K., Ellersdorfer, G., Roschitzki, B., et al. (2012). Who is who in litter decomposition? Metaproteomics reveals major microbial players and their biogeochemical functions. ISME J. 6, 1749–1762. doi:10.1038/ismej.2012.11
Sinsabaugh, R. L. (1994). Enzymic analysis of microbial pattern and process. Biol. Fertil. Soils 17, 69–74. doi:10.1007/bf00418675
Sinsabaugh, R. L., and Follstad Shah, J. J. (2012). Ecoenzymatic stoichiometry and ecological theory. Annu. Rev. Ecol. Evol. Syst. 43, 313–343. doi:10.1146/annurev-ecolsys-071112-124414
Sinsabaugh, R. L., Hill, B. H., and Follstad Shah, J. J. (2009). Ecoenzymatic stoichiometry of microbial organic nutrient acquisition in soil and sediment. Nature 462, 795–798. doi:10.1038/nature08632
Sinsabaugh, R. L., Lauber, C. L., Weintraub, M. N., Ahmed, B., Zeglin, L. H., Crenshaw, C., et al. (2008). Stoichiometry of soil enzyme activity at global scale. Ecol. Lett. 11, 1252–1264. doi:10.1111/j.1461-0248.2008.01245.x
Sinsabaugh, R. L., Shah, J. J. F., Findlay, S. G., Kuehn, K. A., and Moorhead, D. L. (2015). Scaling microbial biomass, metabolism and resource supply. Biogeochemistry 122, 175–190. doi:10.1007/s10533-014-0058-z
Stark, S., Mäannistö, M. K., and Eskelinen, A. (2014). Nutrient availability and pH jointly constrain microbial extracellular enzyme activities in nutrient-poor tundra soils. Plant Soil 383, 373–385. doi:10.1007/s11104-014-2181-y
Steinweg, J. M., Dukes, J. S., and Wallenstein, M. D. (2012). Modeling the effects of temperature and moisture on soil enzyme activity: linking laboratory assays to continuous field data. Soil Biol. Biochem. 55, 85–92. doi:10.1016/j.soilbio.2012.06.015
Sterner, R. W., and Elser, J. J. (2002). Ecological stoichiometry: the biology of elements from molecules to the biosphere. Princeton, NJ, USA: Princeton University Press.
Strickland, M. S., and Rousk, J. (2010). Considering fungal:bacterial dominance in soils–methods, controls, and ecosystem implications. Soil Biol. Biochem. 42, 1385–1395. doi:10.1016/j.soilbio.2010.05.007
Turner, B. L., and Haygarth, P. M. (2005). Phosphatase activity in temperate pasture soils: potential regulation of labile organic phosphorus turnover by phosphodiesterase activity. Sci. Total Environ. 344, 27–36. doi:10.1016/j.scitotenv.2005.02.003
van der Heijden, M. G. A., Bardgett, R. D., and van Straalen, N. M. (2008). The unseen majority: soil microbes as drivers of plant diversity and productivity in terrestrial ecosystems. Ecol. Lett. 11, 296–310. doi:10.1111/j.1461-0248.2007.01139.x
Vance, E. D., Brookes, P. C., and Jenkinson, D. S. (1987). An extraction method for measuring soil microbial biomass C. Soil Biol. Biochem. 19, 703–707. doi:10.1016/0038-0717(87)90052-6
Wang, J., Wang, X., Liu, G., Wang, G., Wu, Y., and Zhang, C. (2020). Fencing as an effective approach for restoration of alpine meadows: evidence from nutrient limitation of soil microbes. Geoderma 363, 114148. doi:10.1016/j.geoderma.2019.114148
Wang, S. K., Zuo, X. A., Zhao, X. Y., Li, Y. Q., Zhou, X., Lv, P., et al. (2016). Responses of soil fungal community to the sandy grassland restoration in Horqin Handy Land, northern China. Environ. Monit. Assess. 188, 1–13. doi:10.1007/s10661-015-5031-3
Wang, X., Li, Y., Wang, L., Duan, Y., Yao, B., Chen, Y., et al. (2023). Soil extracellular enzyme stoichiometry reflects microbial metabolic limitations in different desert types of northwestern China. Sci. Total Environ. 874, 162504. doi:10.1016/j.scitotenv.2023.162504
Waring, B. G., Weintraub, S. R., and Sinsabaugh, R. L. (2014). Ecoenzymatic stoichiometry of microbial nutrient acquisition in tropical soils. Biogeochemistry 117, 101–113. doi:10.1007/s10533-013-9849-x
Xiao, L., Liu, G. B., Li, P., Li, Q., and Xue, S. (2020). Ecoenzymatic stoichiometry and microbial nutrient limitation during secondary succession of natural grassland on the Loess Plateau, China. Soil Tillage Res. 200, 104605. doi:10.1016/j.still.2020.104605
Xiao, W., Chen, X., Jing, X., and Zhu, B. (2018). A meta-analysis of soil extracellular enzyme activities in response to global change. Soil Biol. Biochem. 123, 21–32. doi:10.1016/j.soilbio.2018.05.001
Xu, Z., Yu, G., Zhang, X., He, N., Wang, Q., Wang, S., et al. (2017). Soil enzyme activity and stoichiometry in forest ecosystems along the North-South Transect in eastern China (NSTEC). Soil Biol. Biochem. 104, 152–163. doi:10.1016/j.soilbio.2016.10.020
Yang, Y., Liang, C., Li, T., Cheng, H., An, S., and Chang, S. X. (2020). Soil extracellular enzyme stoichiometry reflects the shift from P- to N-limitation of microorganisms with grassland restoration. Soil Biol. Biochem. 149, 107928. doi:10.1016/j.soilbio.2020.107928
Yu, P. J., Tang, X. G., Zhang, A. C., Fan, G. H., and Liu, S. W. (2019). Responses of soil specific enzyme activities to short-term land use conversions in a salt-affected region, northeastern China. Sci. Total Environ. 687, 939–945. doi:10.1016/j.scitotenv.2019.06.171
Yu, S. L., and Chen, H. W. (2007). Characteristics and formation causes of temperate sparse forest grassland ecosystem in Inner Mongolia Plateau. Chin. J. Ecol. 26, 549–554. (in Chinese with English abstract). doi:10.1371/journal.pone.0002332
Zhang, C., Liu, G., Xue, S., and Wang, G. (2016). Soil bacterial community dynamics reflect changes in plant community and soil properties during the secondary succession of abandoned farmland in the Loess Plateau. Soil Biol. Biochem. 97, 40–49. doi:10.1016/j.soilbio.2016.02.013
Zhang, C., Xue, S., Liu, G. B., and Song, Z. L. (2011). A comparison of soil qualities of different revegetation types in the Loess Plateau, China. Plant Soil 347, 163–178. doi:10.1007/s11104-011-0836-5
Zhang, J., Zhao, H., Zhang, T., Zhao, X., and Drake, S. (2005). Community succession along a chronosequence of vegetation restoration on sand dunes in Horqin Sandy Land. J. Arid. Environ. 62, 555–566. doi:10.1016/j.jaridenv.2005.01.016
Zhang, W., Xu, Y., Gao, D., Wang, X., Liu, W., Deng, J., et al. (2019). Ecoenzymatic stoichiometry and nutrient dynamics along a revegetation chronosequence in the soils of abandoned land and Robinia pseudoacacia plantation on the Loess Plateau, China. Soil Biol. Biochem. 134, 1–14. doi:10.1016/j.soilbio.2019.03.017
Zhao, F. Z., Ren, C., Han, X., Yang, G., Wang, J., and Doughty, R. B. (2018). Changes of soil microbial and enzyme activities are linked to soil C, N and P stoichiometry in afforested ecosystems. For Ecol Manag 15, 289–295. doi:10.1016/j.foreco.2018.06.011
Zheng, H., Liu, Y., Chen, Y., Zhang, J., Li, H., Wang, L., et al. (2020). Short-term warming shifts microbial nutrient limitation without changing the bacterial community structure in an alpine timberline of the eastern Tibetan Plateau. Geoderma 360, 113985. doi:10.1016/j.geoderma.2019.113985
Zhou, L., Liu, S., Shen, H., Zhao, M., Xu, L., Xing, A., et al. (2020). Soil extracellular enzyme activity and stoichiometry in China’s forests. Funct. Ecol. 34, 1461–1471. doi:10.1111/1365-2435.13555
Zhou, R. L., Li, Y. Q., Zhao, H. L., and Drake, S. (2008). Desertification effects on C and N content of sandy soils under grassland in Horqin, northern China. Geoderma 145, 370–375. doi:10.1016/j.geoderma.2008.04.003
Zhu, B., Li, Z., Li, P., Liu, G., and Xue, S. (2010). Soil erodibility, microbial biomass, and physical–chemical property changes during long-term natural vegetation restoration: a case study in the Loess Plateau, China. Ecol. Res. 25, 531–541. doi:10.1007/s11284-009-0683-5
Zhu, G. Y., Deng, L., and Shangguan, Z. P. (2018). Effects of soil aggregate stability on soil N following land use changes under erodible environment. Agric. Ecosyst. Environ. 262, 18–28. doi:10.1016/j.agee.2018.04.012
Zuo, X., Zhang, J., Lv, P., Zhou, X., Lin, Y., Luo, Y., et al. (2016a). Plant functional diversity mediates the effects of vegetation and soil properties on community-level plant nitrogen use in the restoration of semiarid sandy grassland. Ecol. Indic. 64, 272–280. doi:10.1016/j.ecolind.2016.01.012
Zuo, X., Zhang, J., Zhou, X., Zhao, X., Wang, S., Lian, J., et al. (2015). Changes in carbon and nitrogen storage along a restoration gradient in a semiarid sandy grassland. Acta Oecol 69, 1–8. doi:10.1016/j.actao.2015.08.004
Zuo, X., Zhou, X., Lv, P., Zhao, X., Zhang, J., Wang, S., et al. (2016b). Testing associations of plant functional diversity with carbon and nitrogen storage along a restoration gradient of sandy grassland. Front. Plant Sci. 7, 189. doi:10.3389/fpls.2016.00189
Keywords: sandy ecosystem, soil extracellular enzyme stoichiometry, nutrient limitation, microbial biomass, microbial community
Citation: Yao B, Wang X, Li Y, Lian J, Li Y, Luo Y and Li Y (2023) Soil extracellular enzyme activity reflects the change of nitrogen to phosphorus limitation of microorganisms during vegetation restoration in semi-arid sandy land of northern China. Front. Environ. Sci. 11:1298027. doi: 10.3389/fenvs.2023.1298027
Received: 21 September 2023; Accepted: 12 October 2023;
Published: 27 October 2023.
Edited by:
Jun’E. Liu, Shaanxi Normal University, ChinaCopyright © 2023 Yao, Wang, Li, Lian, Li, Luo and Li. This is an open-access article distributed under the terms of the Creative Commons Attribution License (CC BY). The use, distribution or reproduction in other forums is permitted, provided the original author(s) and the copyright owner(s) are credited and that the original publication in this journal is cited, in accordance with accepted academic practice. No use, distribution or reproduction is permitted which does not comply with these terms.
*Correspondence: Xuyang Wang, eHV5YW5nd2FuZ0BsemIuYWMuY24=; Yulin Li, bGl5bEBsemIuYWMuY24=