- Environmental Biogeochemistry and Ecotoxicology, Department F.-A. Forel for Environmental and Aquatic Sciences, Faculty of Sciences, University of Geneva, Geneva, Switzerland
Based on the up-to-date knowledge we critically discuss the current understanding of the influence of the compounds secreted by phytoplankton species on the fate of metal-containing engineered nanoparticles (ENPs) in aquatic settings. Different biomolecules, such as extracellular polymeric substances (EPS) and exometabolites play important, yet to elucidate, role in the dissolution, colloidal stability, transformations and biouptake of the ENPs and thus shape their behavior within the phycosphere. Phytoplankton secretions can also mediate the synthesis of ENPs from dissolved ions by reducing the metals ions and capping the newly formed ENPs. However, the environmental significance of this process remains to be demonstrated. Exposure to ENPs triggers changes in the secretion of the biomolecules. An improved understanding of the regulatory mechanism and exometabolite changes due to ENP exposure is essential for deciphering the ENPs-phytoplankton interactions. Unveiling the significance of secreted biomolecules in modulating the behavior of the metal-containing ENPs is central for understudying the phytoplankton-ENPs feedbacks, drivers of transformations of ENPs and their mechanisms in the aquatic environment.
1 Introduction
Engineered nanoparticles (ENPs) have dimensions ranging from 1 to 100 nm (Vert et al., 2012), and possess unique properties which make them highly valuable for various applications in technology, medicine, environment and consumer products (Zhang et al., 2016; Mitchell et al., 2021; Keller et al., 2023). When released into freshwater environment, ENPs can undergo a variety of physical and chemical transformations (Levard et al., 2012; Batley et al., 2013; Yu et al., 2018; Wheeler et al., 2021; Liu et al., 2022; Rex M et al., 2023). The interconnected transformation processes determine collectively the environmental behavior and effects of ENPs on aquatic biota (French et al., 2009; Fernando and Zhou, 2019; Khort et al., 2022).
In the present review paper, we focus on the role of the phytoplankton secretions in the fate of the metal-containing ENPs in the freshwater environment. Phytoplankton play a central role in the global biogeochemical cycles of various nutrients (C, O, N, P, and Si) (Falkowski, 1994; Litchman et al., 2015), essential (Sunda, 2012) and toxic (Gregoire and Poulain, 2014; Cossart et al., 2022) trace metals and ENPs (Slaveykova, 2023). For example, the phytoplankton species have been shown to influence the fate of the ENPs by i) secreting various exometabolites and extracellular polymeric substances (EPS); ii) accumulating the ENPs and transforming them via different cellular processes; iii) synthesizing the ENPs inside the cells and/or on cell surfaces from dissolved metal species (Slaveykova, 2023).
The phytoplankton secretions contain various exometabolites and EPS, which form its “secretome” and play a key role for the processes in the phycosphere (Seymour et al., 2017; Mühlenbruch et al., 2018). The EPS are known to represent up to 25% of natural organic matter (NOM) in freshwaters, especially during algal blooms (Wilkinson et al., 1997) and to be predominant fraction of marine NOM (Corsi et al., 2020). The composition, types, and properties of microalgal and cyanobacterial EPS vary with the phytoplankton species and environmental conditions, and most often are dominated by polysaccharides and proteins as reviewed (Naveed et al., 2019).
The phytoplankton are source of exometabolites, such as carbohydrates, amino- and carboxylic acids, thiols, fatty acids etc. However, up to now, these exudate components have received limited attention in the research literature. The exudates of different strains of green algae, diatoms, red algae and cyanobacteria have been shown to contain multiples monosaccharides, uronic acid, pyruvate, glucosamine, glucuronic and galacturonic acids (Xiao and Zheng, 2016; Babiak and Krzemińska, 2021; Laroche, 2022). Amino acids have been measured in the secretions of the diatom Skeletonema costatum (Hosny et al., 2022), thiols in the secretions of green algae and diatoms (Mangal and Guéguen, 2015; Mangal et al., 2020), as well as different marine microalgae (Yang et al., 2022). They are present in nanomolar concentration in freshwater environment (Mangal and Guéguen, 2015; Rasheduzzaman et al., 2018). Moreover, it has been demonstrated that various microalgae and cyanobacteria release various allelochemicals, serving for communication, defense, and adaptation purposes (Śliwińska-Wilczewska et al., 2021). Microalgal allelochemicals contain alkaloids, fatty acids and derivatives, polyketides, peptides, phenolics, terpenoids and other volatile organic compounds (Chaïb et al., 2021; Casanova et al., 2023). Lipids have also been found in the exudate (Tambiev et al., 1989), together with other non-polar exometabolites, such as di-tri peptides, lumichrome and prostaglandin-like substances (Brisson et al., 2021). Different constituents of secretome contain divers cationic (e.g., –NH4+, –RNH2+) or anionic (e.g., –SH, –NH2, –COOH, –PO4 3−) functional groups, which confer them metal binding properties (Xu et al., 2013), and play key role in the biomolecule adsorption to ENPs (Chetwynd and Lynch, 2020; Liu et al., 2022).
Drawing upon the latest advancements in current state of the research, the aim of the present review paper is to thoroughly discuss the impact of biomolecules produced by phytoplankton species on the fate of the ENPs in the aquatic environment. Our critical analysis delves into how phytoplankton secretions influence: i) the colloidal stability of the ENPs, the formation of biomolecular corona around them; ii) the chemical transformations, such as dissolution and sulfidation, and iii) the formation of the ENPs from dissolved ions (Figure 1). Furthermore, the influence of the ENP exposure on the secretion of exometabolites and EPS by different phytoplankton species is also considered. The role of the secreted biomolecules on the uptake of ENPs to phytoplankton species and thus their cellular transformations is also briefly discussed. The discussion is illustrated by selected recent examples (Tables 1, 2) and sheds light on the complex interplay between phytoplankton, their secretions, and the behavior of ENPs in aquatic ecosystems.
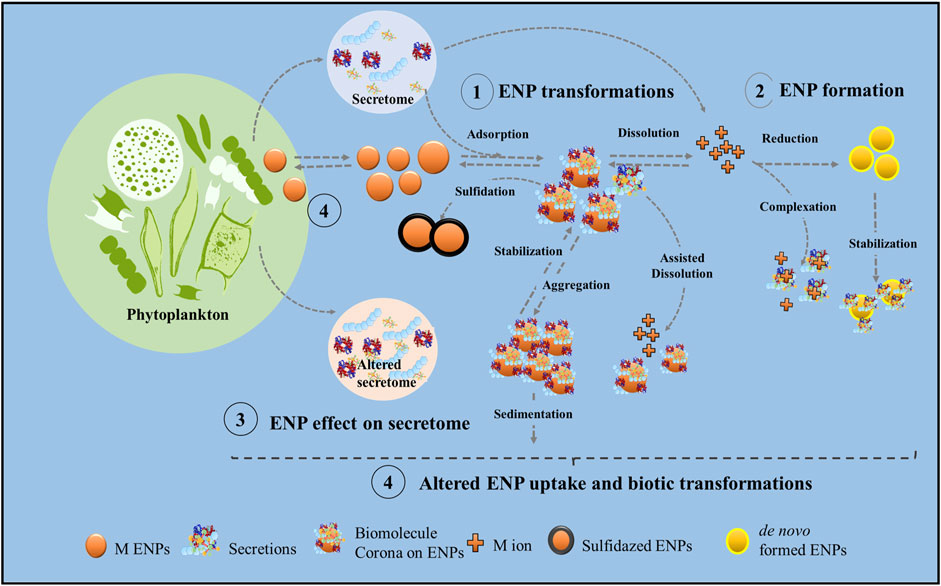
FIGURE 1. Conceptual view of the main physical and chemical processes involved in the interactions between phytoplankton secretions and metal-containing ENPs in the aquatic ecosystems, subject of this review paper.
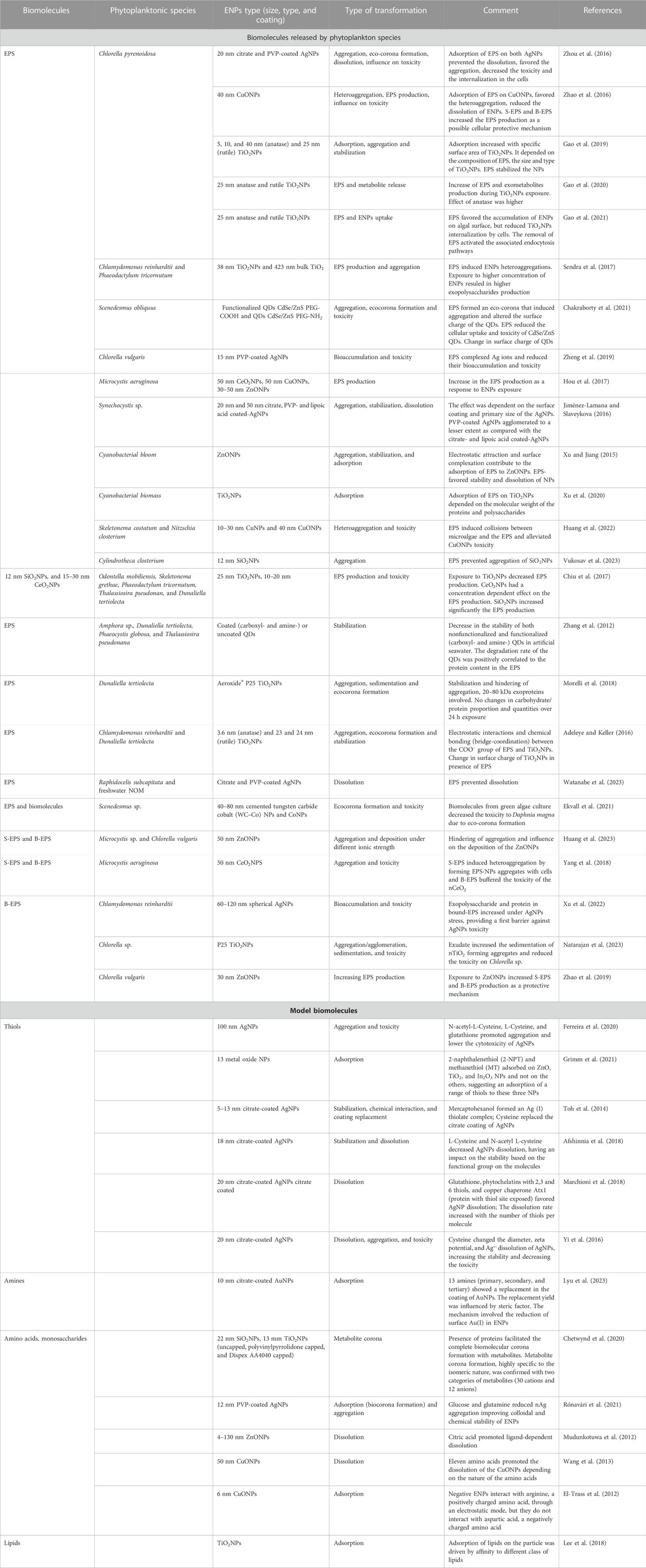
TABLE 1. Selected examples illustrating the interaction between biomolecules and metal-containing ENPs.
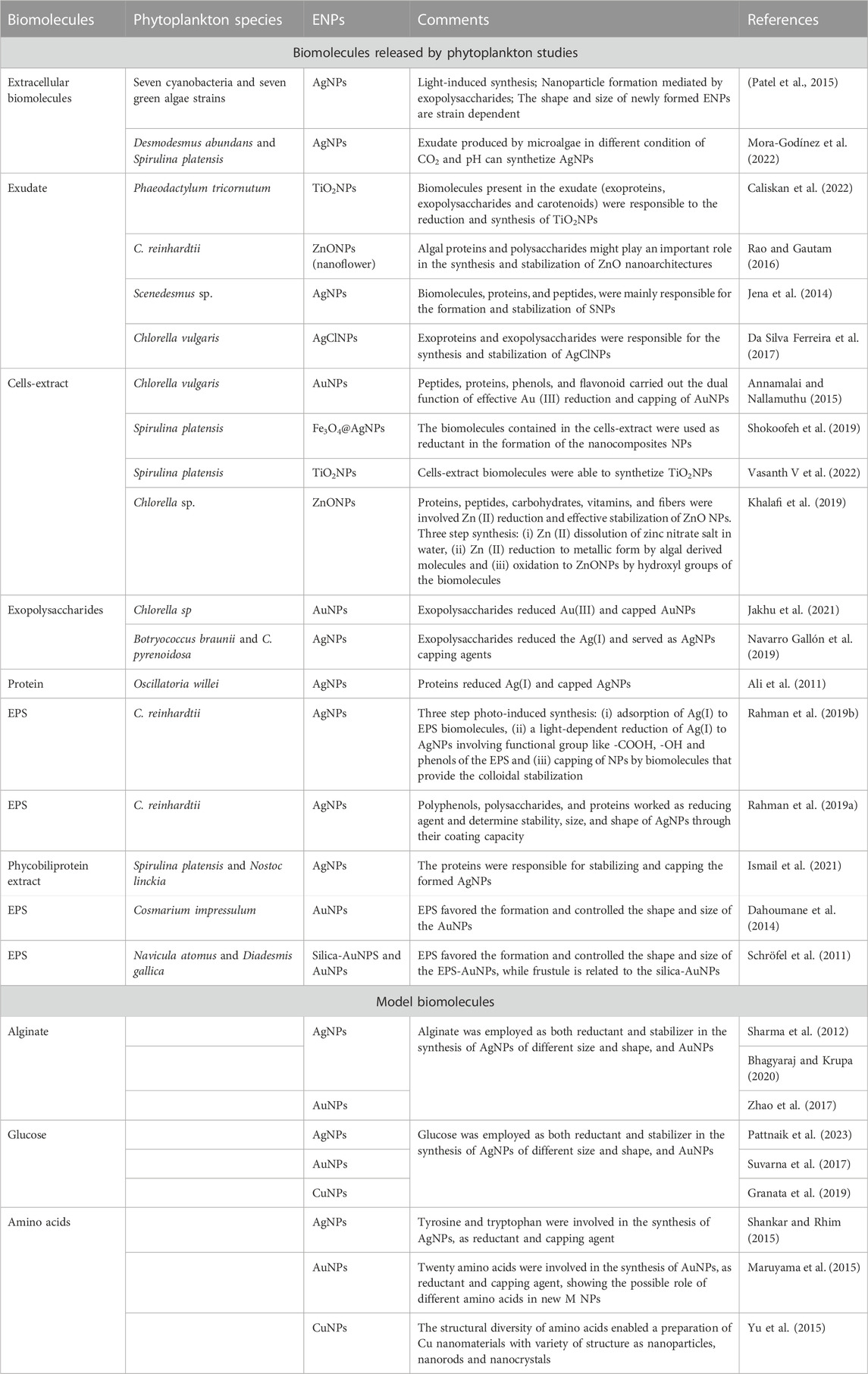
TABLE 2. Selected examples illustrating the involvement of phytoplankton secretions in metal-containing ENPs formation from dissolved metals.
2 Phytoplankton secretions and colloidal stability of ENPs
Most of the available knowledge has been obtained from the studies with EPS produced by cultures of marine and freshwater phytoplankton. It reveals that EPS are able to form biomolecular corona on different ENPs which alters the colloidal stability, transformations (Zheng et al., 2019; Zhang et al, 2020b; Junaid and Wang, 2021; Liu et al., 2022; Slaveykova, 2023) and the uptake (and toxicity) of the ENPs by phytoplankton (Zhou et al., 2016; Natarajan et al., 2023). Key transformations involving ENPs in freshwaters, the role of the NOM in these transformation (Hedberg et al., 2019; Abbas et al., 2020; Milosevic et al., 2020; Spurgeon et al., 2020) and the uptake and biological effects have been comprehensively reviewed (Xu et al., 2020). The EPS released by the major phytoplankton groups, such as cyanobacteria, green algae and diatoms were shown to increase the stability of the ENPs, although examples of enhanced aggregation exist too (Table 1; Figure 1. ①).
The EPS isolated from bloom-forming cyanobacteria has been demonstrated to stabilize the ZnONPs via electrostatic attraction and surface complexation (Xu and Jiang, 2015). Interestingly, the binding affinity of EPS to ZnONPs differs for the fluorophore families identified in the EPS and decreased in the order humic-like > tryptophan-like > fulvic-like components (Xu and Jiang, 2015). Rich in proteins soluble and bound EPS extracted from cyanobacterium Microcystis sp. have been shown to hinder the aggregation of 50 nm ZnONPs in NaCl and low CaCl2 media due to the adsorption of the proteins to the surface of the ENPs for the soluble EPS and through steric repulsion for the bound EPS. However, at higher Ca2+ concentration, soluble EPS promoted the aggregation, whereas the bound EPS stabilized ZnONPs through steric repulsion (Huang et al., 2023). The EPS from C. vulgaris prevented the aggregation of ZnONPs likely due to the electrostatic interactions (Huang et al., 2023). Hydroxyl, carboxyl and amide groups in the EPS have been shown to participate in the adsorption of dissolved and bound EPS of C. vulgaris on the ZnONPs in addition to the interaction of the tryptophan-like components in the soluble EPS with ZnONPs (Zhao et al., 2019). Hydrogen bounding involving the hydroxyl, carboxyl and the amine groups of the EPS have also been shown to play a role in the EPS interaction with ZnONPs (Chen et al., 2012).
The adsorption of the EPS with high molecular weight (HMW, 1 kDa-0.45 μm) isolated from lake cyanobacteria on the TiO2NPs has been found to be more important than that of low MW EPS (LMW, <1 kDa) (Xu et al., 2020). The EPS secreted by green alga Chlorella pyrenoidosa decreased significantly the aggregation rate of TiO2NPs (Lin et al., 2016). Interestingly, a selective adsorption of aromatic components of the EPS from the same alga onto four types of TiO2NPs (5, 10, and 40 nm anatase and 25 nm rutile) and an increase of the adsorption (and stabilization effect) with the specific surface area of the ENPs have been observed (Gao et al., 2019). Comparative study of the soluble EPS from C. reinhardtii and D. tertiolecta has revealed that the EPS adsorption to three commercial TiO2NPs with different coatings depends on the particle surface area, surface charge and hydrophobicity. The interactions between EPS and TiO2NPs have been shown to be driven by electrostatic interactions and chemical bonding between the COO- group of EPS and TiO2NPs (Adeleye and Keller, 2016). The exoproteins (MW of 20–80 kDa) produced by green alga Dunaliella tertiolecta prevent the aggregation of the TiO2NPs in marine water by forming an eco-corona (Morelli et al., 2018; Corsi et al., 2020).
The EPS produced by diatom Cylindrotheca closterium stabilized 12 nm SiO2NPs in marine environment due to molecular and nanoscale interactions (Vukosav et al., 2023). However, the EPS isolated from four other diatoms Amphora sp., D. tertiolecta, Phaeocystis globosa, and Thalassiosira pseudonana decrease the stability of both nonfunctionalized and functionalized (carboxyl- and amine-) quantum dots (QDs) in artificial seawater with a rate positively correlated to the protein fraction of the EPS (Zhang et al., 2012). Similarly the EPS from green alga Scenedesmus obliquus induced the aggregation of both PEG-COOH and PEG-NH2 functionalized QDs, forming an eco-corona that changes the surface charge of the ENPs (Chakraborty et al., 2021). A steric stabilization of sulfide/silica-modified zero valent iron (ZVI) NPs by organic matter released from C. reinhardtii at different growth stages, demonstrating that the feedback from algae may play important roles in the environmental implications of ENPs (Adeleye et al., 2016).
The EPS (<1 kDa MW) released by cyanobacterium Synechocystis sp. stabilized AgNPs with primary size of 20 nm and 50 nm, and three different coatings (Jiménez-Lamana and Slaveykova, 2016). However the stabilization was dependent on the primary surface coating as the effect was significant for citrate-, and lipoic acid-coated AgNPs, but minor on the PVP-coated AgNPs (Jiménez-Lamana and Slaveykova, 2016). EPS from green alga C. vulgaris stabilized both PVP and citrate coated AgNPs (Zhou et al., 2016).
Opposite to the studies with the EPS, very limited knowledge is available concerning the effect of the exometabolites on the stability of the ENPs. Some examples from rather scarce literature are provided below. The adsorption of glucose and glutamine on PVP-coated AgNPs resulted in a formation of biomolecular corona, decreasing their zeta potential and aggregation (Rónavári et al., 2021). A recent study on the interaction between citrate-coated AuNPs and 13 different primary, secondary, and tertiary amines has revealed that the exchange of coating is influenced by steric factors and by the presence of oxidized Au (I) on the surface of ENPs allowing the reduction of Au (I) by amines involved also in the protein corona formation (Lyu et al., 2023). Positively charged arginine adsorbs on the negatively charged CuONPs, but this was not the case of negative charged aspartic acid, demonstrating the importance of the electrostatic interactions (El-Trass et al., 2012). A pilot study has shown the formation of a metabolite corona on SiO2NPs and three different TiO2NPs by 28 cationic metabolites and 12 anionic metabolites, which was highly specific to the isomeric nature of the metabolites (Chetwynd et al., 2020). Thiols such as 2-naphthalenethiol and methanethiol have been also demonstrated to form a corona on the ZnONPs, TiO2NPs, and In2O3NPs (Grimm et al., 2021). Cysteine, but not serine, stabilized ZnS- and HgSNPs, showing the importance of the chemical nature of the exometabolites in the interactions with ENPs (Gondikas et al., 2010). By contrast, the presence of cysteine destabilized the citrate-coated AgNPs, whereas the addition of N-acetyl L-cysteine stabilized the same NPs. This contrasting effect has been attributed to the different functional groups in these two molecules: negatively charged carboxylic groups and positively charged amine group in the cysteine favor bridging interactions (Afshinnia et al., 2018). The addition of small thiols (e.g., cysteine) mitigated the aggregation kinetics of AgNPs in the presence of Suwannee River fulvic acid (Afshinnia et al., 2018), pointing out the complexity in determining the ENPs stability in complex environmental settings. Different classes of lipids formed corona on the TiO2NPs, with LysoPL exhibited higher affinity to TiO2NPs than other lipid categories (Lee et al., 2018).
3 Phytoplankton secretion and ENPs dissolution and transformations
Studies have demonstrated both increase and decrease of the dissolution of metal-containing ENPs in the presence of phytoplankton secretions (Table 1; Figure 1. ①). The EPS from haptophyta Isochrysis galbana increase the dissolution of CuONPs, CuNPs and Cu (OH)2NPs (Kocide) (Adeleye et al., 2014). This is in agreement with early literature showing that the EPS released by various phytoplankton species, including D. tertiolecta (Gonzalez-Davila et al., 1995), Chlorella sp. (Kaplan et al., 1987), C. reinhardii (Xue et al., 1988), Phaeodactylum tricornutum, and T. weissflogii (Gonzalez-Davila et al., 2000) complexed different ions that can be released from the ENPs, such as Cu (II).
The EPS from Chlorella sp. (Chen et al., 2012) and lake cyanobacteria (Xu and Jiang, 2015) decreased the dissolution of the ZnONPs. Moreover, EPS isolated from C. pyrenoidosa reduced the dissolution of citrate- or PVP-coated 20 nm AgNPs by complexing the Ag (I) ions (Zhou et al., 2016). Similarly the EPS from Raphidocelis subcapitata prevented the dissolution of both citrate- and PVP-coated AgNPs (Watanabe et al., 2023). High concentrations of the alginate, an extracellular polysaccharide released from cyanobacterium M. aeruginosa, adsorbed to 20 nm citrate-coated AgNPs and reduced their dissolution rate (Ostermeyer et al., 2013).
Studies on model exometabolites have shown an increase, decrease or no effect on the dissolution of the ENPs. For example, cysteine adsorbed to the surface of AgNPs via SH-group and increased the release of Ag (I) (Gondikas et al., 2012; Wang et al., 2013), however decreased the dissolution and reduced the toxicity of AgNPs to Phanerochaete chrysosporium (Yi et al., 2016). Cysteine has been reported to replace the citrate coating in AgNPs and to increase their stability, however mercaptohexanol formed a silver (I) thiolate complex and favored the AgNPs dissolution (Toh et al., 2014). Cysteine and N-acetyl L-cysteine (NAL-cys) decreased citrate-coated AgNPs dissolution at high cysteine and NAL-cys concentrations (Afshinnia et al., 2018). Given the high affinity of Ag to sulfur and nitrogen groups, it is expected that sulfur- and nitrogen-rich molecules will adsorb more strongly on AgNPs and result in a decrease in the dissolution rate of AgNPs and in an increase of stability (Gunsolus et al., 2015). Glutathione and phytochelatins with 2, 3 or 6 thiols favored AgNPs dissolution into Ag (I) with a rate that increases with the number of thiols per molecule (Marchioni et al., 2018). Citrate enhanced the dissolution of the ZnONPs by ligand-induced dissolution (Mudunkotuwa et al., 2012). Eleven amino-acids Cys, Met, Arg, Glu, Lys, Val, GSH, Thr, Asp, Gln, and His significantly promoted the dissolution of the CuONPs, but the effect was dependent on the nature of the amino acids (Wang et al., 2013).
Much less studies dealt with the role of phytoplankton secretions in other transformation processes, for example, sulfidation. AgNPs, CdNPs, CuNPs, and ZnNPs have been shown to undergo sulfidation with reduced sulfur species of different biomolecules (Levard et al., 2012; Liu et al., 2012). The formation of Ag2SNPs under various environmental scenarios have been reviewed from thermodynamic and kinetic perspectives (He et al., 2019). Dissolution–precipitation mechanism for the sulfidation of ZnONPs (Ma et al., 2013; Banerjee and Jain, 2018) and CuONPs (Wang et al., 2013) was proposed. Sulfidation of metallic ENPs is considered as a major transformation process in urban water sewage systems (Kaegi et al., 2013) and sulphur-rich environment (Thalmann et al., 2014; Liu et al., 2018; He et al., 2019; Zhang et al., 2019).
4 Phytoplankton secretions and ENP formation from dissolved metals
Phytoplankton secretions have been found to mediate the formation of the metal-containing ENPs via reduction of dissolved metal ions (Figure 1. ②; Table 2). Indeed, different EPS components contain reducing functional groups, such as aldehydes, hydroxyls, and phenolic groups (Sheng et al., 2010), tyrosine and tryptophan (Si and Mandal, 2007). Indeed, proteins, peptides, amino acids, polysaccharides, lipids, and nucleic acids and some aromatic compounds are among the compounds reported to guide the formation from dissolved ions, crystal growth and stabilization of metal and metal oxide ENPs (Siddiqi and Husen, 2016). Selected recent examples (Table 2) illustrated the major interested for the synthesis of AgNPs from Ag (I). For example, the carbohydrates from green alga Scenedesmus sp. have been used to synthesize and stabilize the AgNPs (Patel et al., 2015). The proteases, β-D-glucosidases, chitinases, alkaline phosphatases have been also shown to participate in the reduction of Ag (I) to AgNPs (Naveed et al., 2019). In addition, several oxidoreductive proteins produced by C. reinhardtii, including histone H4, superoxide dismutase, and carbonic anhydrase, have been demonstrated to be involved in biosynthesis and stabilization of AgNPs (Barwal et al., 2011). The exopolysaccharides isolated from two green microalgae Botryococcus braunii and C. pyrenoidosa were used to synthetize AgNPs with antibacterial capacity (Navarro Gallón et al., 2019). Similarly the exudates from another green alga Desmodesmus abundans, have been found to synthetize AgNPs under different condition of CO2 and pH (Mora-Godínez et al., 2022). The exoproteins and exopolysaccharides extracted from C. vulgaris, have been shown to be responsible for the reduction of Ag (I) and the consecutive formation and stabilization of AgNPs (Da Silva Ferreira et al., 2017), as it was the case for the exoproteins of the cyanobacterium Oscillatoria willei (Ali et al., 2011) and the phycobiliproteins produced by Spirulina platensis and Nostoc linckia, (Ismail et al., 2021). A three-step mechanism for photo-induced synthesis of AgNPs by EPS released from green alga C. reinhardtii has been proposed including: i) adsorption of Ag(I) to EPS biomolecules, ii) a light-dependent reaction that reduce the Ag (I) for the formation of AgNPs involving functional group like -COOH, -OH and phenols and iii) capping by biomolecules that provide the colloidal stabilization of NPs (Rahman et al., 2019b). Moreover, the polyphenols, polysaccharides and proteins and their nanoparticle-capping capacity determined the different stability, size, and shape of AgNPs produced by C. reinhardtii (Rahman et al., 2019a). A recent study with EPS from Chlorella sp. isolated from a brackish water high-altitude lake of the Northern-Western Himalayas demonstrated that exopolysaccharides synthesized polysaccharide-capped AuNPs that are stable in extended range of pH and salinity (Jakhu et al., 2021). The EPS from chlorophyceae Cosmarium impressulum (Dahoumane et al., 2014), diatoms Navicula atomus and Diadesmis gallica (Schröfel et al., 2011) favored the formation and controlled the shape and size of the AuNPs. Alginate has also reduced Ag (I) forming hetero-shaped AgNPs (Sharma et al., 2012; Bhagyaraj and Krupa, 2020) and Au (I) into AuNPs (Zhao et al., 2017). Similar results have been obtained for the synthesis of AuNPs by C. vulgaris cell extract: peptides, proteins, phenols, and flavonoid carried out the reduction and the capping of AuNPs from Au ions (Annamalai and Nallamuthu, 2015).
The exoproteins, exopolysaccharides, and carotenoids from diatom P. tricornutum have been identified as the agent responsible for the synthesis of TiO2NPs (Caliskan et al., 2022). Similarly, the exoproteins and exopolysaccharides produced by C. reinhardtii played a role in reduction of Zn (II), consecutive formation and stabilization of ZnO nanoflowers (Rao and Gautam, 2016). The proteins, peptides, carbohydrates, vitamins, and fibers of Chlorella sp. have been found to play a role in both reduction of Zn (II) and effective stabilization of ZnONPs in a three steps process: (i) initial activation step involving Zn (II) dissolution from zinc nitrate salt in water, (ii) Zn (II) reduction to metallic form by algal derived biomolecules and (iii) immediate oxidation to ZnONPs during air-drying process by hydroxyl groups present in the biomolecules (Khalafi et al., 2019). Secretions containing polyphenols, alkaloids, proteins, flavonoids, sugars, vitamins terpenoids, steroids, tannins, carboxylic acids and amines, have been demonstrated to synthesize CuONPs (Mallakpour et al., 2020). The proposed reaction mechanism an innitial interaction between Cu (II) polyphenols, leading to a sequential reduction to Cu (I) and to Cu (0), which is further converted to CuONPs.
Glucose was used as reducing and capping reagent in the synthesis of AgNPs (Pattnaik et al., 2023), AuNPs (Suvarna et al., 2017), and CuNPs (Granata et al., 2019). Amino acids, tyrosine and tryptophan, have been shown to be involved in the formation and capping process of AgNPs (Shankar and Rhim, 2015). Similarly twenty amino acids contributed to the formation of the AuNPs (Maruyama et al., 2015). Furthermore, the structural diversity of amino acids enabled a preparation of Cu nanomaterials with variety of structure as nanoparticles, nanorods and nanocrystals (Yu et al., 2015).
5 Influence of ENPs on the release of phytoplankton secretions
Different ENPs have been shown to alter the concentration and composition of the secreted biomolecules by various phytoplankton species (Figure 1. ③). For example, the exposure of the green alga C. pyrenoidosa to anatase and rutile TiO2NPs resulted in an increase of the EPS secretion, which was more pronounced in the case of anatase (Gao et al., 2020). The authors have demonstrated that the effect of anatase on EPS secretion depended mainly on the cellular ROS production, whereas the rutile altered the Ca2+ signaling pathway (Gao et al., 2020). Exposure to CeO2NPs induced ENPs-concentration dependent increase in the production of the EPS by O. mobiliensis, P. tricornutum, D. tertiolecta (Chiu et al., 2017). SiO2NPs significantly increased the secretion of the EPS by T. pseudonana (around 600%) and S. grethae (around 1,000%–1,500%) but not by the O. mobiliensis, P. tricornutum, D. tertiolecta (Chiu et al., 2017). By contrast, the exposure of four different diatom species Odontella mobiliensis, Skeletonema grethae, P. tricornutum, T. pseudonana and green alga D. tertiolecta to TiO2NPs of comparable size demonstrated a decrease of the amount of the released biomolecules (Chiu et al., 2017). AgNPs enhanced the exopolysaccharides and proteins production in bound EPS of C. reinhardtii (Xu et al., 2022). The exposure to TiO2NPs increased in the protein content in the EPS of both C. reinhardtii and marine diatom P. tricornutum (Sendra et al., 2017) Likewise, the exposure to 30 nm ZnONPs resulted in an increase of the protein fraction of the EPS as a protective response of C. vulgaris (Zhao et al., 2019). These findings pointed out that the effect of the ENPs on the release of the EPS by phytoplankton species was dependent on both the type of the phytoplankton species and the type and primary size of the ENPs.
Exposure to 50 nm CeO2NPs, 50 nm CuONPs or 30–50 nm ZnONPs has led to an increase of both tightly and loosely bound EPS of cyanobacterium M. aeruginosa (Hou et al., 2017). It has been suggested that the enhanced EPS production by phytoplankton species when exposed to ENPs lowered collisions between microalgae and the ENPs by decreasing the specific contact area, and thus alleviated CuONPs toxicity in two diatoms S. costatum and N. closterium (Huang et al., 2022). CuONPs enhanced the production of both soluble and cell bounded EPS of C. pyrenoidosa, that consequentially enhance the hetero-aggregation of the ENPs (Zhao et al., 2016).
Most of the published literature has demonstrated that the exposure to ENPs resulted in an increase of the EPS produced by the phytoplankton species, which can be considered as a cellular defense mechanism (Hou et al., 2017). To date no studies have explored the effect of ENPs on the release of exometabolites. However, a recent study revealed an increase in intracellular microcystin concentrations, which was subsequently released in the extracellular environment due to cell death triggered by either ENPs exposure or environmental factors (Zhang et al, 2020a). Despite the current advancements, the underlying regulatory mechanisms of the EPS and exometabolite secretion and ENPs-induced effects are not well known and need to be further explored. In addition to the direct exposure of phytoplankton species to ENPs, the secretion of biomolecules could be affected indirectly via the interaction of the released metal ions with cells (Naveed et al., 2019).
6 Phytoplankton secretions, ENPs uptake and biotic transformations
Both intracellular and cell surface-mediated physical and chemical transformations of ENPs by phytoplankton species have been reported (Chen et al., 2019; Spurgeon et al., 2020). They include heteroaggregation of ENPs and cells, cellular dissolution, sulfidation, redox transformations etc. However, the influence of the phytoplankton secretions on these processes is overlooked. Few examples found in the literature demonstrate that the EPS can reduce the uptake of the ENPs by phytoplankton species (and thus ENPs cellular transformations). The exudates secreted by S. obliquus reduced cellular uptake of the eco-coronated amine and carboxyl functionalized CdSe/ZnS QDs inducing aggregation and altering the surface charge of the QDs (Chakraborty et al., 2021). The EPS of C. pyrenoidosa reduced the amount of the internalized anatase and rutile TiO2NPs (Gao et al., 2021). The EPS from green alga Scenedesmus sp. formed corona on cemented tungsten carbide cobalt (WC–Co) NPs and CoNPs and decreased the toxicity of these ENPs on Daphnia magna (Ekvall et al., 2021). Bound EPS have shown a barrier effect on the toxicity of AgNPs nanoparticles relative to the released Ag (I) ions. However, they did not exhibit a distinct effect on the silver accumulation by AgNPs and Ag (I) in C. vulgaris (Zheng et al., 2019). Nevertheless, both soluble and bound EPS have been shown to modify the attachments of the ENPs to the phytoplankton cells. This alteration occurs through modification of the steric and electrostatic interactions between the ENPs and the cells (Natarajan et al., 2021). Similarly, the intact cells of C. pyrenoidosa with EPS was characterized with greater adsorption of both citrate and PVP coated AgNPs in comparison with cells with removed EPS (Zhou et al., 2016). Interestingly, the importance of the EPS thickness on cells of C. reinhardtii in AgNPs internalization have been demonstrated (Yan et al., 2021). The presence of EPS on the C. pyrenoidosa cell surface promoted the heteroaggregation between anatase and rutile TiO2NPs with primary size of 25 nm, and algal cells. The amount of the TiO2NPs accumulated on cell surface was higher in comparison to the algal cells with removed EPS (Gao et al., 2021). Moreover, the EPS from Chlorella sp. increased the sedimentation of TiO2NPs in mixture with polystyrene NPs thus contributing to the reduction of the number of ENPs interacting with the organism and consequently reducing the biouptake the TiO2NPs (Natarajan et al., 2023). The presence of soluble and bound EPS induced heteroaggregation by forming EPS-NPs aggregates with cells of cyanobacterium Microcystis aeruginosa (Yang et al., 2018). To the best of our knowledge, no study has reported the role of the phytoplankton secretions in the cellular transformations of metal-containing ENPs, including processes like cellular dissolution, sulfidation, redox transformations exist.
7 Conclusions and research gaps
An examination of the current state of the research highlights that different constituents of the phytoplankton secretions play an important, yet incompletely understood, role in shaping the behavior of metal-containing ENPs in aquatic environment. These secretions interact with and adsorb on the ENPs, forming a biomolecular corona and altering their behavior. The formation of biomolecular corona on ENPs involves hydrodynamic, electrodynamic, electrostatic, steric, and bridging interactions as previously comprehensively reviewed for eco-corona formed by NOM on the ENPs (Pulido-Reyes et al., 2017). In most cases the adsorbed secretions, in particularly the EPS have been shown to stabilize the ENPs. As for the other components of the NOM, the adsorption and effect on the colloidal stability are dependent on the i) chemical composition, primary surface coating, surface charge, surface area and hydrophobicity; ii) chemical composition of the secretions, molecular weight and aromaticity, isomeric nature, which in turn are dependent on the phytoplankton species, algal growth stage and environmental parameters. The current body of literature primarily focusses on the interactions between TiO2NPs or ZnONPs, and EPS isolated from green algae, diatoms and cyanobacteria. There is a limited amount of literature concerning the influence of exometabolites on the stability of the ENPs, despite their presumably important role in the phycosphere. Further attention and research are warranded in this area to better understand their impact.
Some discrepancy in the information exists concerning the role of the phytoplankton secretions in the ENPs dissolution, showcasing both decrease and increase in the dissolution rates. Limited studies have explored the role of phytoplankton secretions in other transformation processes, such as sulfidation. Further deep investigations are essential to understand how phytoplankton secretions contribute to both the dissolution and sulfidation of ENPs within aquatic environment.
Most of the up-to-date knowledge largely steems from the experiments involving whole EPS, isolated polysaccharides, or proteins from specific phytoplankton species and model compounds. As a result, the role of some EPS components, including nucleic acids, lipids, uronic acid, and inorganic compounds, all part of the phytoplankton secretome, remains unexplored, despite their likely influence on the stability, dissolution and transformation of metal-containing ENPs. In addition, major secretome components such as thiols and amino acids exist within concentrations ranging from ng L−1 to μg L−1 in surface water. Their significance in modulating the aforementioned processes in the presence of the EPS or humic-like components of NOM (typically present in few mgL−1) still requires a conclusive demonstartion.
Phytoplankton secretions serve mediators in the synthesis of metal-containing nanoparticles from the dissolved ions. Nevertheless, the majority these studies are conducted within biotechnology context using high metal concentration than those found in the aquatic environment. Thus their contribution to the metal reduction in the natural environment is to be explored. In addition, the role of the EPS and exometabolites released by algae, as well as the importance of reductive mechanism for ENPs formation from metal ions, its environmental significance for the ENP biogeochemistry is to be further explored. It is currently unclear if the phytoplankton could be considered as a “source” of ENPs in metal contaminated environment and what will be the environmental relevance in the nanoparticle production. Further studies are, thus, necessary to understand the underlying mechanisms and their contribution in natural environment, where ENPs are present in quite low concentrations is still to demonstrate.
Exposure of phytoplankton species to ENPs leads to changes in the concentration and composition of secreted biomolecules. These alterations might act as a detoxification response and defensive pathway. To deepen our understanding it is crucial to explore the underlying regulatory mechanisms, encompassing changes in exometabolomics of phytoplankton due to ENP exposure.
In conclusion, the lack of understanding on the influence of the phytoplankton secretions on the destiny of the ENPs is a major knowledge gap in the aquatic nanoscience. Considering the feedback of aquatic organisms and the control they exert in shaping the fate (and impact) of ENPs in the aquatic environment is necessary for: i) better understanding of drivers of environmental transformations of ENPs and their mechanisms and ii) reduction of the uncertainties and the improved assessment of the effect of the ENPs on the phytoplankton cells, given that the chemical conditions and ENPs stability will be strongly affected by these secretions in the microenvironment surrounding phytoplankton cells in comparison with bulk waters.
Author contributions
RG: Conceptualization, Writing–original draft, Writing–review and editing. VS: Conceptualization, Funding acquisition, Investigation, Resources, Supervision, Writing–original draft, Writing–review and editing.
Funding
The author(s) declare financial support was received for the research, authorship, and/or publication of this article. Authors acknowledge the financial support of Swiss National Science Foundation Projects No. 204174.
Conflict of interest
The authors declare that the research was conducted in the absence of any commercial or financial relationships that could be construed as a potential conflict of interest.
The author(s) declared that they were an editorial board member of Frontiers, at the time of submission. This had no impact on the peer review process and the final decision.
Publisher’s note
All claims expressed in this article are solely those of the authors and do not necessarily represent those of their affiliated organizations, or those of the publisher, the editors and the reviewers. Any product that may be evaluated in this article, or claim that may be made by its manufacturer, is not guaranteed or endorsed by the publisher.
References
Abbas, Q., Yousaf, B., Amina Ali, M. U., Munir, M. A. M., El-Naggar, A., Rinklebe, J., et al. (2020). Transformation pathways and fate of engineered nanoparticles (ENPs) in distinct interactive environmental compartments: a review. Environ. Int. 138 (18), 105646. doi:10.1016/j.envint.2020.105646
Adeleye, A. S., Conway, J. R., Perez, T., Rutten, P., and Keller, A. A. (2014). Influence of extracellular polymeric substances on the long-term fate, dissolution, and speciation of copper-based nanoparticles. Environ. Sci. Technol. 48 (21), 12561–12568. doi:10.1021/es5033426
Adeleye, A. S., and Keller, A. A. (2016). Interactions between algal extracellular polymeric substances and commercial TiO2 nanoparticles in aqueous media. Environ. Sci. Technol. 50 (22), 12258–12265. doi:10.1021/acs.est.6b03684
Adeleye, A. S., Stevenson, L. M., Su, Y. M., Nisbet, R. M., Zhang, Y. L., and Keller, A. A. (2016). Influence of phytoplankton on fate and effects of modified zerovalent iron nanoparticles. Environ. Sci. Technol. 50 (11), 5597–5605. doi:10.1021/acs.est.5b06251
Afshinnia, K., Marrone, B., and Baalousha, M. (2018). Potential impact of natural organic ligands on the colloidal stability of silver nanoparticles. Sci. Total Environ. 625, 1518–1526. doi:10.1016/j.scitotenv.2017.12.299
Ali, D. M., Sasikala, M., Gunasekaran, M., and Tajuddin, N. (2011). Biosynthesis and characterization of silver nanoparticles using marine cyanobacterium, Oscillatoria willei NTDM01. Dig. J. Nanomater. Biostructures 6 (2), 385–390.
Annamalai, J., and Nallamuthu, T. (2015). Characterization of biosynthesized gold nanoparticles from aqueous extract of Chlorella vulgaris and their anti-pathogenic properties. Appl. Nanosci. 5 (5), 603–607. doi:10.1007/s13204-014-0353-y
Babiak, W., and Krzemińska, I. (2021). Extracellular polymeric substances (EPS) as microalgal bioproducts: a review of factors affecting EPS synthesis and application in flocculation processes. Energies 14 (13), 4007. doi:10.3390/en14134007
Banerjee, P., and Jain, P. K. (2018). Mechanism of sulfidation of small zinc oxide nanoparticles. RSC Adv. 8 (60), 34476–34482. doi:10.1039/c8ra06949b
Barwal, I., Ranjan, P., Kateriya, S., and Yadav, S. C. (2011). Cellular oxido-reductive proteins of Chlamydomonas reinhardtii control the biosynthesis of silver nanoparticles. J. Nanobiotechnology 9 (1), 56. doi:10.1186/1477-3155-9-56
Batley, G. E., Kirby, J. K., and McLaughlin, M. J. (2013). Fate and risks of nanomaterials in aquatic and terrestrial environments. Accounts Chem. Res. 46 (3), 854–862. doi:10.1021/ar2003368
Bhagyaraj, S., and Krupa, I. (2020). Alginate-mediated synthesis of hetero-shaped silver nanoparticles and their hydrogen peroxide sensing ability. Molecules 25 (3), 435. doi:10.3390/molecules25030435
Brisson, V., Mayali, X., Bowen, B., Golini, A., Thelen, M., Stuart, R. K., et al. (2021). Identification of effector metabolites using exometabolite profiling of diverse microalgae. Microalgae 6 (6), e0083521. doi:10.1128/msystems.00835-21
Caliskan, G., Mutaf, T., Agba, H. C., and Elibol, M. (2022). Green synthesis and characterization of titanium nanoparticles using microalga, Phaeodactylum tricornutum. Geomicrobiol. J. 39 (1), 83–96. doi:10.1080/01490451.2021.2008549
Casanova, L. M., Macrae, A., De Souza, J. E., Neves Junior, A., and Vermelho, A. B. (2023). The potential of allelochemicals from microalgae for biopesticides. Plants 12 (9), 1896. doi:10.3390/plants12091896
Chaïb, S., Pistevos, J. C. A., Bertrand, C., and Bonnard, I. (2021). Allelopathy and allelochemicals from microalgae: an innovative source for bio-herbicidal compounds and biocontrol research. Algal Res. 54, 102213. doi:10.1016/j.algal.2021.102213
Chakraborty, D., Ethiraj, K. R., Chandrasekaran, N., and Mukherjee, A. (2021). Mitigating the toxic effects of CdSe quantum dots towards freshwater alga Scenedesmus obliquus: role of eco-corona. Environ. Pollut. 270, 116049. doi:10.1016/j.envpol.2020.116049
Chen, F. R., Xiao, Z. G., Yue, L., Wang, J., Feng, Y., Zhu, X. S., et al. (2019). Algae response to engineered nanoparticles: current understanding, mechanisms and implications. Environ. Science-Nano 6 (4), 1026–1042. doi:10.1039/c8en01368c
Chen, P., Powell, B. A., Mortimer, M., and Ke, P. C. (2012). Adaptive interactions between zinc oxide nanoparticles and Chlorella sp. Environ. Sci. Technol. 46 (21), 12178–12185. doi:10.1021/es303303g
Chetwynd, A. J., and Lynch, I. (2020). The rise of the nanomaterial metabolite corona, and emergence of the complete corona. Environ. Sci. Nano 7 (4), 1041–1060. doi:10.1039/c9en00938h
Chetwynd, A. J., Zhang, W., Thorn, J. A., Lynch, I., and Ramautar, R. (2020). The nanomaterial metabolite corona determined using a quantitative metabolomics approach: a pilot study. Small 16 (21), 2000295. doi:10.1002/smll.202000295
Chiu, M.-H., Khan, Z. A., Garcia, S. G., Le, A. D., Kagiri, A., Ramos, J., et al. (2017). Effect of engineered nanoparticles on exopolymeric substances release from marine phytoplankton. Nanoscale Res. Lett. 12 (1), 620. doi:10.1186/s11671-017-2397-x
Corsi, I., Bergami, E., and Grassi, G. (2020). Behavior and bio-interactions of anthropogenic particles in marine environment for a more realistic ecological risk assessment. Front. Environ. Sci. 8 (21), 60. doi:10.3389/fenvs.2020.00060
Cossart, T., Garcia-Calleja, J., Santos, J. P., Kalahroodi, E. L., Worms, I. A. M., Pedrero, Z., et al. (2022). Role of phytoplankton in aquatic mercury speciation and transformations. Environ. Chem. 19, 104–115. doi:10.1071/en22045
Dahoumane, S. A., Yepremian, C., Djediat, C., Coute, A., Fievet, F., Coradin, T., et al. (2014). A global approach of the mechanism involved in the biosynthesis of gold colloids using micro-algae. J. Nanoparticle Res. 16 (10), 2607. doi:10.1007/s11051-014-2607-8
Da Silva Ferreira, V., ConzFerreira, M. E., Lima, L. M. T. R., Frasés, S., De Souza, W., and Sant’Anna, C. (2017). Green production of microalgae-based silver chloride nanoparticles with antimicrobial activity against pathogenic bacteria. Enzyme Microb. Technol. 97, 114–121. doi:10.1016/j.enzmictec.2016.10.018
Ekvall, M. T., Hedberg, J., Odnevall Wallinder, I., Malmendal, A., Hansson, L.-A., and Cedervall, T. (2021). Adsorption of bio-organic eco-corona molecules reduces the toxic response to metallic nanoparticles in Daphnia magna. Sci. Rep. 11 (1), 10784. doi:10.1038/s41598-021-90053-5
El-Trass, A., ElShamy, H., El-Mehasseb, I., and El-Kemary, M. (2012). CuO nanoparticles: synthesis, characterization, optical properties and interaction with amino acids. Appl. Surf. Sci. 258 (7), 2997–3001. doi:10.1016/j.apsusc.2011.11.025
Falkowski, P. G. (1994). The role of phytoplankton photosynthesis in global biogeochemical cycles. Photosynth. Res. 39 (3), 235–258. doi:10.1007/bf00014586
Fernando, I., and Zhou, Y. (2019). Impact of pH on the stability, dissolution and aggregation kinetics of silver nanoparticles. Chemosphere 216, 297–305. doi:10.1016/j.chemosphere.2018.10.122
Ferreira, L. A. B., Dos Reis, S. B., Do Nascimento Da Silva, E., Cadore, S., Bernardes, J. D. S., Durán, N., et al. (2020). Thiol-antioxidants interfere with assessing silver nanoparticle cytotoxicity. Nanomedicine Nanotechnol. Biol. Med. 24, 102130. doi:10.1016/j.nano.2019.102130
French, R. A., Jacobson, A. R., Kim, B., Isley, S. L., Penn, R. L., and Baveye, P. C. (2009). Influence of ionic strength, pH, and cation valence on aggregation kinetics of titanium dioxide nanoparticles. Environ. Sci. Technol. 43 (5), 1354–1359. doi:10.1021/es802628n
Gao, X., Deng, R., and Lin, D. H. (2020). Insights into the regulation mechanisms of algal extracellular polymeric substances secretion upon the exposures to anatase and rutile TiO2 nanoparticles. Environ. Pollut. 263, 114608. doi:10.1016/j.envpol.2020.114608
Gao, X., Middepogu, A., Deng, R., Liu, J. F., Hao, Z. N., and Lin, D. H. (2019). Adsorption of extracellular polymeric substances from two microbes by TiO2 nanoparticles. Sci. Total Environ. 694, 133778. doi:10.1016/j.scitotenv.2019.133778
Gao, X., Yang, K., and Lin, D. (2021). Influence of extracellular polymeric substance on the interaction between titanium dioxide nanoparticles and Chlorella pyrenoidosa cells. Sci. Total Environ. 778, 146446. doi:10.1016/j.scitotenv.2021.146446
Gondikas, A. P., Jang, E. K., and Hsu-Kim, H. (2010). Influence of amino acids cysteine and serine on aggregation kinetics of zinc and mercury sulfide colloids. J. Colloid Interface Sci. 347 (2), 167–171. doi:10.1016/j.jcis.2010.03.051
Gondikas, A. P., Morris, A., Reinsch, B. C., Marinakos, S. M., Lowry, G. V., and Hsu-Kim, H. (2012). Cysteine-induced modifications of zero-valent silver nanomaterials: implications for particle surface chemistry, aggregation, dissolution, and silver speciation. Environ. Sci. Technol. 46 (13), 7037–7045. doi:10.1021/es3001757
Gonzalez-Davila, M., Santana-Casiano, I. M., Perez-Pena, J., and Millero, F. J. (1995). Binding of Cu(II) to the surface and exudates of the alga Dunaliella tertiolecta in seawater. Environ. Sci. Technol. 29 (2), 289–301. doi:10.1021/es00002a004
Gonzalez-Davila, M., Santana-Casiano, J. M., and Laglera, L. M. (2000). Copper adsorption in diatom cultures. Mar. Chem. 70 (1-3), 161–170. doi:10.1016/s0304-4203(00)00020-7
Granata, G., Onoguchi, A., and Tokoro, C. (2019). Preparation of copper nanoparticles for metal-metal bonding by aqueous reduction with d-glucose and PVP. Chem. Eng. Sci. 209, 115210. doi:10.1016/j.ces.2019.115210
Gregoire, D. S., and Poulain, A. J. (2014). A little bit of light goes a long way: the role of phototrophs on mercury cycling. Metallomics 6 (3), 396–407. doi:10.1039/c3mt00312d
Grimm, O. C., Somaratne, R. M. D. S., Wang, Y., Kim, S., and Whitten, J. E. (2021). Thiol adsorption on metal oxide nanoparticles. Phys. Chem. Chem. Phys. 23 (14), 8309–8317. doi:10.1039/d1cp00506e
Gunsolus, I. L., Mousavi, M. P. S., Hussein, K., Bühlmann, P., and Haynes, C. L. (2015). Effects of humic and fulvic acids on silver nanoparticle stability, dissolution, and toxicity. Environ. Sci. Technol. 49 (13), 8078–8086. doi:10.1021/acs.est.5b01496
He, D., Garg, S., Wang, Z., Li, L., Rong, H., Ma, X., et al. (2019). Silver sulfide nanoparticles in aqueous environments: formation, transformation and toxicity. Environ. Sci. Nano 6 (6), 1674–1687. doi:10.1039/c9en00138g
Hedberg, J., Blomberg, E., and Odnevall Wallinder, I. (2019). In the search for nanospecific effects of dissolution of metallic nanoparticles at freshwater-like conditions: a critical review. Environ. Sci. Technol. 53 (8), 4030–4044. doi:10.1021/acs.est.8b05012
Hosny, S., El-Sheekh, M., and Labib, W. (2022). Intercellular and extracellular amino acids of different bloom species in the Mediterranean Sea. Oceanol. Hydrobiological Stud. 51 (2), 178–188. doi:10.26881/oandhs-2022.2.06
Hou, J., Yang, Y., Wang, P., Wang, C., Miao, L., Wang, X., et al. (2017). Effects of CeO2, CuO, and ZnO nanoparticles on physiological features of Microcystis aeruginosa and the production and composition of extracellular polymeric substances. Environ. Sci. Pollut. Res. Int. 24 (1), 226–235. doi:10.1007/s11356-016-7387-5
Huang, R., Han, Z., Ma, C., Liu, H., and Huangfu, X. (2023). Stability and mobility of zinc oxide nanoparticles in aquatic environment: influence of extracellular polymeric substances from cyanobacteria and microalgae. J. Environ. Chem. Eng. 11 (1), 109069. doi:10.1016/j.jece.2022.109069
Huang, W., Zhou, Y., Zhao, T., Tan, L., and Wang, J. (2022). The effects of copper ions and copper nanomaterials on the output of amino acids from marine microalgae. Environ. Sci. Pollut. Res. 29 (7), 9780–9791. doi:10.1007/s11356-021-16347-3
Ismail, G. A., El-Sheekh, M. M., Samy, R. M., and Gheda, S. F. (2021). Antimicrobial, antioxidant, and antiviral activities of biosynthesized silver nanoparticles by phycobiliprotein crude extract of the cyanobacteria Spirulina platensis and Nostoc linckia. BioNanoScience 11 (2), 355–370. doi:10.1007/s12668-021-00828-3
Jakhu, S., Sharma, Y., Sharma, K., Vaid, K., Dhar, H., Kumar, V., et al. (2021). Production and characterization of microalgal exopolysaccharide as a reducing and stabilizing agent for green synthesis of gold-nanoparticle: a case study with a Chlorella sp. from Himalayan high-altitude psychrophilic habitat. J. Appl. Phycol. 33 (6), 3899–3914. doi:10.1007/s10811-021-02580-3
Jena, J., Pradhan, N., Nayak, R. R., Dash, B. P., Sukla, L. B., Panda, P. K., et al. (2014). Microalga Scenedesmus sp.: a potential low-cost green machine for silver nanoparticle synthesis. J. Microbiol. Biotechnol. 24 (4), 522–533. doi:10.4014/jmb.1306.06014
Jiménez-Lamana, J., and Slaveykova, V. I. (2016). Silver nanoparticle behaviour in lake water depends on their surface coating. Sci. Total Environ. 573, 946–953. doi:10.1016/j.scitotenv.2016.08.181
Junaid, M., and Wang, J. (2021). Interaction of nanoplastics with extracellular polymeric substances (EPS) in the aquatic environment: a special reference to eco-corona formation and associated impacts. Water Res. 201, 117319. doi:10.1016/j.watres.2021.117319
Kaegi, R., Voegelin, A., Ort, C., Sinnet, B., Thalmann, B., Krismer, J., et al. (2013). Fate and transformation of silver nanoparticles in urban wastewater systems. Water Res. 47 (12), 3866–3877. doi:10.1016/j.watres.2012.11.060
Kaplan, D., Christiaen, D., and Arad, S. M. (1987). Chelating properties of extracellular polysaccharides from Chlorella Spp. Appl. Environ. Microbiol. 53 (12), 2953–2956. doi:10.1128/aem.53.12.2953-2956.1987
Keller, A. A., Ehrens, A., Zheng, Y., and Nowack, B. (2023). Developing trends in nanomaterials and their environmental implications. Nat. Nanotechnol. 18, 834–837. doi:10.1038/s41565-023-01409-z
Khalafi, T., Buazar, F., and Ghanemi, K. (2019). Phycosynthesis and enhanced photocatalytic activity of zinc oxide nanoparticles toward organosulfur pollutants. Sci. Rep. 9 (1), 6866. doi:10.1038/s41598-019-43368-3
Khort, A., Brookman-Amissah, M., Hedberg, J., Chang, T., Mei, N., Lundberg, A., et al. (2022). Influence of natural organic matter on the transformation of metal and metal oxide nanoparticles and their ecotoxic potency in vitro. NanoImpact 25, 100386. doi:10.1016/j.impact.2022.100386
Laroche, C. (2022). Exopolysaccharides from microalgae and cyanobacteria: diversity of strains, production strategies, and applications. Mar. Drugs 20 (5), 336. doi:10.3390/md20050336
Lee, J. Y., Wang, H., Pyrgiotakis, G., DeLoid, G. M., Zhang, Z., Beltran-Huarac, J., et al. (2018). Analysis of lipid adsorption on nanoparticles by nanoflow liquid chromatography-tandem mass spectrometry. Anal. Bioanal. Chem. 410 (24), 6155–6164. doi:10.1007/s00216-018-1145-0
Levard, C., Hotze, E. M., Lowry, G. V., and Brown, G. E. (2012). Environmental transformations of silver nanoparticles: impact on stability and toxicity. Environ. Sci. Technol. 46 (13), 6900–6914. doi:10.1021/es2037405
Lin, D., Story, S. D., Walker, S. L., Huang, Q. Y., and Cai, P. (2016). Influence of extracellular polymeric substances on the aggregation kinetics of TiO2 nanoparticles. Water Res. 104, 381–388. doi:10.1016/j.watres.2016.08.044
Litchman, E., de Tezanos Pinto, P., Edwards, K. F., Klausmeier, C. A., Kremer, C. T., and Thomas, M. K. (2015). Global biogeochemical impacts of phytoplankton: a trait-based perspective. J. Ecol. 103 (6), 1384–1396. doi:10.1111/1365-2745.12438
Liu, J., Wang, Z., Liu, F. D., Kane, A. B., and Hurt, R. H. (2012). Chemical transformations of nanosilver in biological environments. ACS Nano 6 (11), 9887–9899. doi:10.1021/nn303449n
Liu, J. Y., Zhang, F., Allen, A. J., Johnston-Peck, A. C., and Pettibone, J. M. (2018). Comparing sulfidation kinetics of silver nanoparticles in simulated media using direct and indirect measurement methods. Nanoscale 10 (47), 22270–22279. doi:10.1039/c8nr06668j
Liu, W., Worms, I. A. M., Jakšić, Ž., and Slaveykova, V. I. (2022). Aquatic organisms modulate the bioreactivity of engineered nanoparticles: focus on biomolecular corona. Front. Toxicol. 4, 933186. doi:10.3389/ftox.2022.933186
Lyu, Y., Becerril, L. M., Vanzan, M., Corni, S., Cattelan, M., Granozzi, G., et al. (2023). The interaction of amines with gold nanoparticles. Adv. Mater., 2211624. doi:10.1002/adma.202211624
Ma, R., Levard, C., Michel, F. M., Brown, G. E., and Lowry, G. V. (2013). Sulfidation mechanism for zinc oxide nanoparticles and the effect of sulfidation on their solubility. Environ. Sci. Technol. 47 (6), 2527–2534. doi:10.1021/es3035347
Mallakpour, S., Azadi, E., and Hussain, C. M. (2020). Environmentally benign production of cupric oxide nanoparticles and various utilizations of their polymeric hybrids in different technologies. Coord. Chem. Rev. 419, 213378. doi:10.1016/j.ccr.2020.213378
Mangal, V., and Guéguen, C. (2015). Examining concentrations and molecular weights of thiols in microorganism cultures and in Churchill River (Manitoba) using a fluorescent-labeling method coupled to asymmetrical flow field-flow fractionation. Anal. Bioanal. Chem. 407 (15), 4305–4313. doi:10.1007/s00216-015-8599-0
Mangal, V., Phung, T., and Guéguen, C. (2020). An estimation of sulfur concentrations released by three algae (Chlorella vulgaris, Chlamydomonas reinhardtii, Scenedesmus obliquus) in response to variable growth photoperiods. Environ. Sci. Pollut. Res. 27 (11), 12491–12498. doi:10.1007/s11356-020-07812-6
Marchioni, M., Gallon, T., Worms, I., Jouneau, P.-H., Lebrun, C., Veronesi, G., et al. (2018). Insights into polythiol-assisted AgNP dissolution induced by bio-relevant molecules. Environ. Sci. Nano 5 (8), 1911–1920. doi:10.1039/c8en00340h
Maruyama, T., Fujimoto, Y., and Maekawa, T. (2015). Synthesis of gold nanoparticles using various amino acids. J. Colloid Interface Sci. 447, 254–257. doi:10.1016/j.jcis.2014.12.046
Milosevic, A., Romeo, D., and Wick, P. (2020). Understanding nanomaterial biotransformation: an unmet challenge to achieving predictive nanotoxicology. Small 16 (36), 1907650. doi:10.1002/smll.201907650
Mitchell, M. J., Billingsley, M. M., Haley, R. M., Wechsler, M. E., Peppas, N. A., and Langer, R. (2021). Engineering precision nanoparticles for drug delivery. Nat. Rev. Drug Discov. 20 (2), 101–124. doi:10.1038/s41573-020-0090-8
Mora-Godínez, S., Abril-Martínez, F., and Pacheco, A. (2022). Green synthesis of silver nanoparticles using microalgae acclimated to high CO2. Mater. Today Proc. 48, 5–9. doi:10.1016/j.matpr.2020.04.761
Morelli, E., Gabellieri, E., Bonomini, A., Tognotti, D., Grassi, G., and Corsi, I. (2018). TiO2 nanoparticles in seawater: aggregation and interactions with the green alga Dunaliella tertiolecta. Ecotoxicol. Environ. Saf. 148, 184–193. doi:10.1016/j.ecoenv.2017.10.024
Mudunkotuwa, I. A., Rupasinghe, T., Wu, C.-M., and Grassian, V. H. (2012). Dissolution of ZnO nanoparticles at circumneutral pH: a study of size effects in the presence and absence of citric acid. Langmuir 28 (1), 396–403. doi:10.1021/la203542x
Mühlenbruch, M., Grossart, H. P., Eigemann, F., and Voss, M. (2018). Mini-review: phytoplankton-derived polysaccharides in the marine environment and their interactions with heterotrophic bacteria. Environ. Microbiol. 20 (8), 2671–2685. doi:10.1111/1462-2920.14302
Natarajan, L., Annie Jenifer, M., Peijnenburg, W. J. G. M., and Mukherjee, A. (2023). Algal extracellular polymeric substances (algal-EPS) for mitigating the combined toxic effects of polystyrene nanoplastics and nano-TiO2 in Chlorella. Nanotoxicology 17 (2), 143–156. doi:10.1080/17435390.2023.2179438
Natarajan, L., Jenifer, M. A., and Mukherjee, A. (2021). Eco-corona formation on the nanomaterials in the aquatic systems lessens their toxic impact: a comprehensive review. Environ. Res. 194, 110669. doi:10.1016/j.envres.2020.110669
Navarro Gallón, S. M., Alpaslan, E., Wang, M., Larese-Casanova, P., Londoño, M. E., Atehortúa, L., et al. (2019). Characterization and study of the antibacterial mechanisms of silver nanoparticles prepared with microalgal exopolysaccharides. Mater. Sci. Eng. C 99, 685–695. doi:10.1016/j.msec.2019.01.134
Naveed, S., Li, C. H., Lu, X. D., Chen, S. S., Yin, B., Zhang, C. H., et al. (2019). Microalgal extracellular polymeric substances and their interactions with metal(loid)s: a review. Crit. Rev. Environ. Sci. Technol. 49 (19), 1769–1802. doi:10.1080/10643389.2019.1583052
Ostermeyer, A.-K., Kostigen Mumuper, C., Semprini, L., and Radniecki, T. (2013). Influence of bovine serum albumin and alginate on silver nanoparticle dissolution and toxicity to Nitrosomonas europaea. Environ. Sci. Technol. 47 (24), 14403–14410. doi:10.1021/es4033106
Patel, V., Berthold, D., Puranik, P., and Gantar, M. (2015). Screening of cyanobacteria and microalgae for their ability to synthesize silver nanoparticles with antibacterial activity. Biotechnol. Rep. 5, 112–119. doi:10.1016/j.btre.2014.12.001
Pattnaik, C., Mishra, R., Sahu, A. K., Sahoo, L. N., Sahoo, N. K., Tripathy, S. K., et al. (2023). Green synthesis of glucose-capped stable silver nanoparticles: a cost-effective sensor for the selective detection of Hg 2+ions in aqueous solutions. Sensors Diagnostics 2 (3), 647–656. doi:10.1039/d3sd00019b
Pulido-Reyes, G., Leganes, F., Fernández-Piñas, F., and Rosal, R. (2017). Bio-nano interface and environment: a critical review. Environ. Toxicol. Chem. 36, 3181–3193. doi:10.1002/etc.3924
Rahman, A., Kumar, S., Bafana, A., Dahoumane, S., and Jeffryes, C. (2019a). Individual and combined effects of extracellular polymeric substances and whole cell components of Chlamydomonas reinhardtii on silver nanoparticle synthesis and stability. Molecules 24 (5), 956. doi:10.3390/molecules24050956
Rahman, A., Kumar, S., Bafana, A., Lin, J., Dahoumane, S. A., and Jeffryes, C. (2019b). A mechanistic view of the light-induced synthesis of silver nanoparticles using extracellular polymeric substances of Chlamydomonas reinhardtii. Molecules 24 (19), 3506. doi:10.3390/molecules24193506
Rao, M. D., and Gautam, P. (2016). Synthesis and characterization of ZnO nanoflowers using Chlamydomonas reinhardtii: a green approach. Environ. Prog. Sustain. Energy 35 (4), 1020–1026. doi:10.1002/ep.12315
Rasheduzzaman, M., Kawaguchi, M., Obata, H., and Maruo, M. (2018). Determination of dissolved and particulate thiols in Lake Biwa water and extracted fulvic acids by solid phase extraction followed by HPLC with fluorescence detection. Limnology 19 (3), 299–309. doi:10.1007/s10201-018-0547-1
Rex, M. C., Anand, S., Rai, P. K., and Mukherjee, A. (2023). Engineered nanoparticles (ENPs) in the aquatic environment: an overview of their fate and transformations. Water, Air, & Soil Pollut. 234 (7), 462. doi:10.1007/s11270-023-06488-1
Rónavári, A., Bélteky, P., Boka, E., Zakupszky, D., Igaz, N., Szerencsés, B., et al. (2021). Polyvinyl-pyrrolidone-coated silver nanoparticles—the colloidal, chemical, and biological consequences of steric stabilization under biorelevant conditions. Int. J. Mol. Sci. 22 (16), 8673. doi:10.3390/ijms22168673
Schröfel, A., Kratošová, G., Bohunická, M., Dobročka, E., and Vávra, I. (2011). Biosynthesis of gold nanoparticles using diatoms—silica-gold and EPS-gold bionanocomposite formation. J. Nanoparticle Res. 13 (8), 3207–3216. doi:10.1007/s11051-011-0221-6
Sendra, M., Moreno-Garrido, I., Yeste, M. P., Gatica, J. M., and Blasco, J. (2017). Toxicity of TiO2, in nanoparticle or bulk form to freshwater and marine microalgae under visible light and UV-A radiation. Environ. Pollut. 227, 39–48. doi:10.1016/j.envpol.2017.04.053
Seymour, J. R., Amin, S. A., Raina, J.-B., and Stocker, R. (2017). Zooming in on the phycosphere: the ecological interface for phytoplankton–bacteria relationships. Nat. Microbiol. 2 (7), 17065. doi:10.1038/nmicrobiol.2017.65
Shankar, S., and Rhim, J.-W. (2015). Amino acid mediated synthesis of silver nanoparticles and preparation of antimicrobial agar/silver nanoparticles composite films. Carbohydr. Polym. 130, 353–363. doi:10.1016/j.carbpol.2015.05.018
Sharma, S., Sanpui, P., Chattopadhyay, A., and Ghosh, S. S. (2012). Fabrication of antibacterial silver nanoparticle—sodium alginate–chitosan composite films. RSC Adv. 2 (13), 5837. doi:10.1039/c2ra00006g
Sheng, G.-P., Yu, H.-Q., and Li, X.-Y. (2010). Extracellular polymeric substances (EPS) of microbial aggregates in biological wastewater treatment systems: a review. Biotechnol. Adv. 28 (6), 882–894. doi:10.1016/j.biotechadv.2010.08.001
Shokoofeh, N., Moradi-Shoeili, Z., Naeemi, A. S., Jalali, A., Hedayati, M., and Salehzadeh, A. (2019). Biosynthesis of Fe3O4@Ag nanocomposite and evaluation of its performance on expression of norA and norB efflux pump genes in ciprofloxacin-resistant Staphylococcus aureus. Biol. Trace Elem. Res. 191 (2), 522–530. doi:10.1007/s12011-019-1632-y
Si, S., and Mandal, T. K. (2007). Tryptophan-Based peptides to synthesize gold and silver nanoparticles: a mechanistic and kinetic study. Chem. – A Eur. J. 13 (11), 3160–3168. doi:10.1002/chem.200601492
Siddiqi, K. S., and Husen, A. (2016). Fabrication of metal and metal oxide nanoparticles by algae and their toxic effects. Nanoscale Res. Lett. 11 (1), 363. doi:10.1186/s11671-016-1580-9
Slaveykova, V. (2023). “Phytoplankton controls on the transformations of metal-containing nanoparticles in aquatic environment,” in Environmental nanoparzicles: sources, occurrence, analysis and fate. Editors J. Jimenez Lamana, and J. Szpunar (London, United Kingdom: Royal Society of Chemistry), 5, 113–131. doi:10.1039/9781839166570-00113
Śliwińska-Wilczewska, S., Wiśniewska, K., Konarzewska, Z., Cieszyńska, A., Barreiro Felpeto, A., Lewandowska, A. U., et al. (2021). The current state of knowledge on taxonomy, modulating factors, ecological roles, and mode of action of phytoplankton allelochemicals. Sci. Total Environ. 773, 145681. doi:10.1016/j.scitotenv.2021.145681
Spurgeon, D. J., Lahive, E., and Schultz, C. L. (2020). Nanomaterial transformations in the environment: effects of changing exposure forms on bioaccumulation and toxicity. Small 16, 2000618. doi:10.1002/smll.202000618
Sunda, W. G. (2012). Feedback interactions between trace metal nutrients and phytoplankton in the ocean. Front. Microbiol. 3, 204. doi:10.3389/fmicb.2012.00204
Suvarna, S., Das, U., Kc, S., Mishra, S., Sudarshan, M., Saha, K. D., et al. (2017). Synthesis of a novel glucose capped gold nanoparticle as a better theranostic candidate. PLOS ONE 12 (6), e0178202. doi:10.1371/journal.pone.0178202
Tambiev, A. H., Shelyastina, N. N., and Kirikova, N. N. (1989). Exometabolites of lipid nature from two species of marine microalgae. Funct. Ecol. 3 (2), 245. doi:10.2307/2389307
Thalmann, B., Voegelin, A., Sinnet, B., Morgenroth, E., and Kaegi, R. (2014). Sulfidation kinetics of silver nanoparticles reacted with metal sulfides. Environ. Sci. Technol. 48 (9), 4885–4892. doi:10.1021/es5003378
Toh, H. S., Batchelor-McAuley, C., Tschulik, K., and Compton, R. G. (2014). Chemical interactions between silver nanoparticles and thiols: a comparison of mercaptohexanol against cysteine. Sci. China Chem. 57 (9), 1199–1210. doi:10.1007/s11426-014-5141-8
Vasanth, V., Murugesh, Ka, and Susikaran, S. (2022). Synthesis of titanium dioxide nanoparticles using Spirulina platensis algae extract. Pharma Innovation 11 (7S), 266–269. doi:10.22271/tpi.2022.v11.i7sd.13643
Vert, M., Doi, Y., Hellwich, K.-H., Hess, M., Hodge, P., Kubisa, P., et al. (2012). Terminology for biorelated polymers and applications (IUPAC Recommendations 2012). Pure Appl. Chem. 84 (2), 377–410. doi:10.1351/pac-rec-10-12-04
Vukosav, P., Pašalić, L., Bakarić, D., Domazet Jurašin, D., and Mišić Radić, T. (2023). Interaction of silica nanoparticles with microalgal extracellular polymers. Water 15 (3), 519. doi:10.3390/w15030519
Wang, Z., von dem Bussche, A., Kabadi, P. K., Kane, A. B., and Hurt, R. H. (2013). Biological and environmental transformations of copper-based nanomaterials. ACS Nano 7 (10), 8715–8727. doi:10.1021/nn403080y
Watanabe, C. H., Domingos, R. F., Benedetti, M. F., and Rosa, A. H. (2023). Dissolution and fate of silver nanoparticles in the presence of natural aquatic organic matter. J. Environ. Expo. Assess. 2 (1), 6. doi:10.20517/jeea.2022.24
Wheeler, K. E., Chetwynd, A. J., Fahy, K. M., Hong, B. S., Tochihuitl, J. A., Foster, L. A., et al. (2021). Environmental dimensions of the protein corona. Nat. Nanotechnol. 16 (6), 617–629. doi:10.1038/s41565-021-00924-1
Wilkinson, K. J., Joz-Roland, A., and Buffle, J. (1997). Different roles of pedogenic fulvic acids and aquagenic biopolymers on colloid aggregation and stability in freshwaters. Limnol. Oceanogr. 42 (8), 1714–1724. doi:10.4319/lo.1997.42.8.1714
Xiao, R., and Zheng, Y. (2016). Overview of microalgal extracellular polymeric substances (EPS) and their applications. Biotechnol. Adv. 34 (7), 1225–1244. doi:10.1016/j.biotechadv.2016.08.004
Xu, H., and Jiang, H. (2015). Effects of cyanobacterial extracellular polymeric substances on the stability of ZnO nanoparticles in eutrophic shallow lakes. Environ. Pollut. 197, 231–239. doi:10.1016/j.envpol.2014.10.031
Xu, H. C., Yan, Z. S., Cai, H. Y., Yu, G. H., Yang, L. Y., and Jiang, H. L. (2013). Heterogeneity in metal binding by individual fluorescent components in a eutrophic algae-rich lake. Ecotoxicol. Environ. Saf. 98, 266–272. doi:10.1016/j.ecoenv.2013.09.008
Xu, L., Xu, M., Wang, R., Yin, Y., Lynch, I., and Liu, S. (2020). The crucial role of environmental coronas in determining the biological effects of engineered nanomaterials. Small 16 (36), 2003691. doi:10.1002/smll.202003691
Xu, L., Zhao, Z., Yan, Z., Zhou, G., Zhang, W., Wang, Y., et al. (2022). Defense pathways of Chlamydomonas reinhardtii under silver nanoparticle stress: extracellular biosorption, internalization and antioxidant genes. Chemosphere 291, 132764. doi:10.1016/j.chemosphere.2021.132764
Xue, H.-B., Stumm, W., and Sigg, L. (1988). The binding of heavy metals to algal surfaces. Water Res. 22 (7), 917–926. doi:10.1016/0043-1354(88)90029-2
Yan, N., Tang, B. Z., and Wang, W.-X. (2021). Cell cycle control of nanoplastics internalization in phytoplankton. ACS Nano 15 (7), 12237–12248. doi:10.1021/acsnano.1c03879
Yang, Q.-Q., Li, P.-F., Duan, S.-S., Han, L., Gao, P.-P., Liu, C.-Y., et al. (2022). Production of dimethylsulfoniopropionate, dimethylsulfide and acrylic acid from marine microalgae. J. Sea Res. 190, 102299. doi:10.1016/j.seares.2022.102299
Yang, Y., Hou, J., Wang, P., Wang, C., Wang, X., and You, G. (2018). Influence of extracellular polymeric substances on cell-NPs heteroaggregation process and toxicity of cerium dioxide NPs to Microcystis aeruginosa. Environ. Pollut. 242, 1206–1216. doi:10.1016/j.envpol.2018.08.005
Yi, F., Chen, G., Zeng, G., Guo, Z., Liu, W., Huang, Z., et al. (2016). Influence of cysteine and bovine serum albumin on silver nanoparticle stability, dissolution, and toxicity to Phanerochaete chrysosporium. RSC Adv. 6 (108), 106177–106185. doi:10.1039/c6ra23675h
Yu, J.-C., Zhao, F.-G., Shao, W., Ge, C.-W., and Li, W.-S. (2015). Shape-controllable and versatile synthesis of copper nanocrystals with amino acids as capping agents. Nanoscale 7 (19), 8811–8818. doi:10.1039/c5nr00146c
Yu, S., Liu, J., Yin, Y., and Shen, M. (2018). Interactions between engineered nanoparticles and dissolved organic matter: a review on mechanisms and environmental effects. J. Environ. Sci. 63, 198–217. doi:10.1016/j.jes.2017.06.021
Zhang, F., Allen, A. J., Johnston-Peck, A. C., Liu, J. Y., and Pettibone, J. M. (2019). Transformation of engineered nanomaterials through the prism of silver sulfidation. Nanoscale Adv. 1 (1), 241–253. doi:10.1039/c8na00103k
Zhang, J., Jiang, L., Wu, D., Yin, Y., and Guo, H. (2020a). Effects of environmental factors on the growth and microcystin production of Microcystis aeruginosa under TiO2 nanoparticles stress. Sci. Total Environ. 734, 139443. doi:10.1016/j.scitotenv.2020.139443
Zhang, S., Jiang, Y., Chen, C.-S., Spurgin, J., Schwehr, K. A., Quigg, A., et al. (2012). Aggregation, dissolution, and stability of quantum dots in marine environments: importance of extracellular polymeric substances. Environ. Sci. Technol. 46 (16), 8764–8772. doi:10.1021/es301000m
Zhang, X.-F., Liu, Z.-G., Shen, W., and Gurunathan, S. (2016). Silver nanoparticles: synthesis, characterization, properties, applications, and therapeutic approaches. Int. J. Mol. Sci. 17 (9), 1534. doi:10.3390/ijms17091534
Zhang, Z., Si, R., Lv, J., Ji, Y., Chen, W., Guan, W., et al. (2020b). Effects of extracellular polymeric substances on the formation and methylation of mercury sulfide nanoparticles. Environ. Sci. Technol. 54 (13), 8061–8071. doi:10.1021/acs.est.0c01456
Zhao, J., Cao, X., Liu, X., Wang, Z., Zhang, C., White, J. C., et al. (2016). Interactions of CuO nanoparticles with the algae Chlorella pyrenoidosa: adhesion, uptake, and toxicity. Nanotoxicology 10 (9), 1297–1305. doi:10.1080/17435390.2016.1206149
Zhao, J., Liu, S., Liu, N., Zhang, H., Zhou, Q., and Ge, F. (2019). Accelerated productions and physicochemical characterizations of different extracellular polymeric substances from Chlorella vulgaris with nano-ZnO. Sci. Total Environ. 658, 582–589. doi:10.1016/j.scitotenv.2018.12.019
Zhao, X., Li, Z., Deng, Y., Zhao, Z., Li, X., and Xia, Y. (2017). Facile synthesis of gold nanoparticles with alginate and its catalytic activity for reduction of 4-nitrophenol and H2O2 detection. Materials 10 (5), 557. doi:10.3390/ma10050557
Zheng, S., Zhou, Q., Chen, C., Yang, F., Cai, Z., Li, D., et al. (2019). Role of extracellular polymeric substances on the behavior and toxicity of silver nanoparticles and ions to green algae Chlorella vulgaris. Sci. Total Environ. 660, 1182–1190. doi:10.1016/j.scitotenv.2019.01.067
Keywords: nanoparticles, phytoplankton, secretome, exudates, EPS, eco-corona, dissolution, aggregation
Citation: Gasco R and Slaveykova VI (2024) Unraveling the impact of phytoplankton secretions on the behavior of metal-containing engineered nanoparticles in aquatic environment. Front. Environ. Sci. 11:1285752. doi: 10.3389/fenvs.2023.1285752
Received: 30 August 2023; Accepted: 08 December 2023;
Published: 04 January 2024.
Edited by:
Dengjun Wang, Auburn University, United StatesReviewed by:
Islam Radwan, Auburn University, United StatesJing Fang, Zhejiang University of Science and Technology, China
Copyright © 2024 Gasco and Slaveykova. This is an open-access article distributed under the terms of the Creative Commons Attribution License (CC BY). The use, distribution or reproduction in other forums is permitted, provided the original author(s) and the copyright owner(s) are credited and that the original publication in this journal is cited, in accordance with accepted academic practice. No use, distribution or reproduction is permitted which does not comply with these terms.
*Correspondence: Vera I. Slaveykova, dmVyYS5zbGF2ZXlrb3ZhQHVuaWdlLmNo