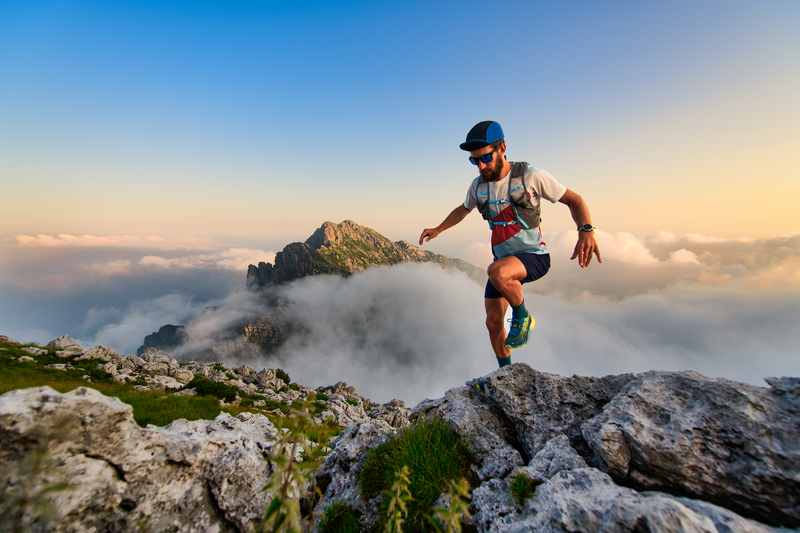
94% of researchers rate our articles as excellent or good
Learn more about the work of our research integrity team to safeguard the quality of each article we publish.
Find out more
REVIEW article
Front. Environ. Sci. , 20 November 2023
Sec. Toxicology, Pollution and the Environment
Volume 11 - 2023 | https://doi.org/10.3389/fenvs.2023.1277240
This article is part of the Research Topic By-product Amendments for the Remediation of Metal- and Metalloid-contaminated Soils View all 7 articles
Biochar production and application have become increasingly popular in the past 15 years. Biochar, derived from diverse biomass types, offers a rich carbon source created through thermal combustion. Biochar production primarily depends on pyrolysis conditions and feedstock type. This review focuses on the multifaceted aspects of biochar, encompassing hydrothermal carbonization, gasification, and pyrolysis temperatures in biochar production and its role in bioeconomy and soil remediation. Biochar has yielded valuable insights, notably in decreasing nutrient leaching, curbing greenhouse gas (GHG) emissions, reducing the bioavailability of environmental pollutants, sequestering carbon (C) in soils, and enhancing agricultural productivity. Consequently, it has emerged as a valuable commodity for the bioeconomy, which involves harnessing bioresources through bioengineering to create economically valuable products. As a marketable output, biochar finds application in energy, diverse biochar-based product manufacturing, and the agricultural sector. Thus, biochar production not only enhances soil quality but also unlocks additional revenue streams. This review underscores the critical role of feedstock selection and pyrolysis conditions in optimizing biochar production. Furthermore, it highlights biochar as a sustainable and effective tool for improving various soil types and remediating soil contamination caused by organic impurities, including persistent organic compounds and antibiotics.
Biochar is a carbon-rich, porous substance with a unique structure comprising carbon aromatic compounds, ample functional groups, high cation exchange capacity, negative surface charges, neutral to alkaline pH, and high specific surface area. As a soil conditioner, biochar significantly impacts soil quality by altering biological and physiochemical soil traits (Murtaza et al., 2021a; Murtaza et al., 2021b), which can increase soil quality and crop yields (Chen et al., 2022; Chen et al., 2023). The effectiveness of biochar depends on the feedstock used, production mechanism, crop type, and soil type (Issaka et al., 2022). Biochar is produced from various biomass sources, including paper mill byproducts, animal waste, and crop residues, offering a sustainable means of converting these residues into valuable products (Singh et al., 2020).
Biochar production can range from small-scale to large-scale operations using pyrolysis methods, a thermochemical process that transforms feedstock into bio-oil, biochar, and syngas within a temperature range of 300°C–750 °C. Gasification and pyrolysis are thermochemical processes that convert biomass into solid biochar (Kamali et al., 2022). These processes include fast and slow pyrolysis, with each method dependent on heating and residence time rates. Fast pyrolysis yields more liquids and oils, while slow pyrolysis generates more syngas. Slow pyrolysis also accelerates biochar formation more efficiently than gasification and fast pyrolysis (Mandal et al., 2021). Fast pyrolysis occurs at high temperatures with a brief residence time of approximately one second. The key distinction between fast and slow pyrolysis lies in their product yields. The biochar production process commences with feedstock drying, during which particles are heated to release volatile organic compounds (VOC) (Qian et al., 2023), such as hydrogen, methane, CO, CO2, and condensable compounds like methanol and acetic acid. Gas-phase polymerization and cracking reactions transform the overall product composition (Lu et al., 2022).
Gasification enhances the surface area, aromaticity, and porosity of biochar. In gasification, feedstock is converted into a gaseous form, leading to a limited amount of oxidizing agents (water and oxygen) at a higher temperature (Tauqeer et al., 2021). During biochar formation, the solid carbon product is the main result, with the release of volatile components and water evaporation contributing to the increase in stable C content in the solid. Polymerization of organic substances in gases and vapors results in a more compact char structure (Anae et al., 2021). Table 1 summarizes the reaction mechanisms involved in biochar production, and Table 2 presents information on the influence of pyrolysis temperature and feedstock on various biochar elemental compositions. Biochar typically consists of carbon and ash, with its elemental composition and properties varying based on the pyrolysis temperature and feedstock material. The structure of biochar primarily comprises carbon and minerals with varying pore sizes. Micropores contribute to the extensive surface area and sorption capacity of biochar, while mesopores facilitate liquid–solid sorption mechanisms, and macropores play a crucial role in soil structure, quality, aeration, hydrology, and root movement (Yin et al., 2021). The size and arrangement of these pores depend on the specific pyrolysis temperature and feedstock constituents used during production. Scanning electron microscopy (SEM) provides insight into the pore size distribution and morphology in biochar derived from different sources, as illustrated in Figure 1.
FIGURE 1. Effect of porosity and surface of biochar of various feedstock (A) Sugar beet tailing (Li et al., 2022) (B) Ricc hull (Rashid et al., 2020) (C) Wood-chips (Samoraj et al., 2022) (D) Fruit bunches (Liang et al., 2021). (E) Human waste (Vanapalli et al., 2021) (F) Wood bark (Yin et al., 2021) (G) Dairy residue (Lehmann et al., 2011) (H) Poultry waste (Zahed et al., 2021).
Shaheen et al. (2022) defined biochar as a porous, solid carbon substance produced through the thermochemical transformation of organic substances in an oxygen-depleted environment, resulting in ideal chemical and physical characteristics for long-lasting and safe carbon sequestration in the environment. However, biochar occurs in some types of charcoal but is obtained under fixed moderated circumstances, which allows carbon to be most stable and transformed to useable output (Rashid et al., 2020). The micropores in biochar enhance microbial activity and enable the sorption of dissolved organic substances, facilitating the remediation of organic contaminants in soil (Li et al., 2022). Biochar holds significant potential for deactivating pesticides in soil, retaining soil quality and fertility, and enhancing abiotic biodegradation. Consequently, biochar enhances phytoremediation techniques for removing various soil pollutants, complementing other remediation techniques. This review discusses i) the key physiochemical characteristics influenced by various soil amendments to acidic or alkaline soils and their preparation conditions, ii) the remediation potential of biochar in soils contaminated with organic pollutants and other environmental benefits such as gas remediation and greenhouse gas (GHG) mitigation, iii) the economic benefits of biochar, and iv) future research directions on biochar.
Biomass/feedstock is a complex organic/inorganic or biological solid material derived from plants and other living organisms (Reyhanitabar et al., 2020). It encompasses different types of residue and litter, including industrial waste, papermill residues, animal manure, and poultry litter (Behnam and Firouzi, 2022). Biomass/feedstock can be broadly categorized into woody and non-woody feedstock. Woody feedstock typically comprises waste from trees and forestry (Singh et al., 2022), characterized by low voidage, high bulk density, high calorific value, and low ash and moisture contents (Huang et al., 2021). Non-woody feedstock consists of industrial solid waste, urban waste, animal manure, and agricultural residues (Singh et al., 2022), characterized by high voidage, high ash and moisture contents, low bulk density, and low calorific value (Mukherjee et al., 2022). The moisture content within biomass significantly influences biochar production (Al-Rabaiai et al., 2022), with high moisture contents elevating the energy required to reach carbonization temperature, which hinders biochar production (Mukherjee et al., 2022). Lower moisture contents typically favor biochar production due to the significant reduction in heat energy and time required for carbonization, making the process more economically feasible than pyrolysis using feedstock with higher moisture contents (Behnam and Firouzi, 2022). Different moisture/water content levels in feedstock lead to biochar production with diverse physicochemical attributes (Huang et al., 2021). For instance, the moisture/water contents of softwood and hardwood bark significantly influence the surface chemistry of the resulting biochars (Xu et al., 2022). A low moisture content in maple bark results in a more graphite-like and polyaromatic char surface, possibly due to prolonged effective pyrolysis after water evaporation (Wani et al., 2022).
Biochar production comprises three main stages: i) pre-pyrolysis, ii) main-pyrolysis, and iii) the formation of carbonaceous soil products (Balmuk et al., 2023). The first stage (100°C–200°C) involves the evaporation of light volatiles and moisture. This moisture evaporation causes bond breakages and forms hydroperoxide, -CO, and -COOH groups (Dhar et al., 2022). The second stage (200°C–500°C) focuses on removing volatiles and decomposing cellulose and hemicelluloses at an accelerated rate (Balmuk et al., 2023). The third and final stage (>500 °C) involves the degradation of organic matter and lignin with strong chemical bonds (Greenough et al., 2021). The carbonization temperature strongly correlates with changes in the biochar’s physicochemical attributes and structure (Kalina et al., 2022). Table 3 presents data on these relationships. The pyrolysis temperature significantly affects the physicochemical characteristics of biochar, including functional groups, pH, and surface area, and its role as a soil amendment (Wani et al., 2022). Higher pyrolytic temperatures enhance volatile matter, pH, and carbonized fractions and reduce surface functional groups and cation exchange capacity (CEC).
Biochar addition accelerates the bioremediation process for organic compounds by promoting microbial activity that degrades saturated hydrocarbons in biochar-amended soils. Certain metalloids, such as boron, arsenic, and silicon, can not be removed from the soil but can be transformed, typically from higher to lower concentrations (Yang et al., 2021). When remediating metalloids, specific considerations must be taken into account, including the adsorption of metalloids by plants and bioenergy crops in contaminated cultivated land and feedstock and the conversion of metalloids into less harmful products (Azeem et al., 2022; Rizwan et al., 2024). Biochar properties conducive to soil remediation include a large surface area, high degradation resistance, and negative charges (Mazarji et al., 2021). A source refers to the site of the contaminant, while a receptor refers to the site that induces damage. The pathway is the route through which contaminants migrate from the source to the receptor. Contaminants that move from the source to the receptor in sufficient quantities to cause harm are considered pollutants (Murtaza et al., 2022). One of the most common methods to address polluted soil is to remove the receptor or the source (Zahed et al., 2021). However, these methods can be costly and impractical, especially in the case of widespread soil contamination. Contaminants can also transfer from sources to receptors through dissolution into solution via various mechanisms (Wang L et al., 2021). Biochar intervenes in the source route–receptor relationship by adsorbing contaminants on its surface and reducing their concentrations in soil solutions (Xu et al., 2021). Successful remediation occurs when biochar permanently eliminates contaminants from the soil solution, eliminating the pathway to receptors. After adsorption onto biochar surfaces, contaminants no longer pose a risk of harm. The large surface area of biochar plays a crucial role in its ability to remove metal ions and organic pollutants (Natasha et al., 2022).
Figure 2 illustrates the remediation of pollutants (inorganic and organic) through biochar in soil based on the source–pathway–receptor relationship, with Stage 1 showing a contaminated site in the absence of biochar and Stage 2 showing a pollution-free site after biochar application. Biochar derived from various biomasses exhibits robust adsorption abilities for various organic pollutants and pesticides (Table 4), which can surpass the natural soil organic matter by a factor ranging from 10 to 98. Various studies have reported significant reductions in organic contaminants in biochar-amended soils (Van Nguyen et al., 2022). According to Murtaza et al. (2022), the processes involved primarily include surface partitioning and adsorption. The adsorption of organic pollutants onto biochar occurs repeatedly due to partitioning (in uncarbonized fraction) in lower-temperature biochar and surface adsorption (in carbonized fraction) in higher-temperature biochar. Increasing the pyrolysis temperature enhances carbonization, increasing the biochar-specific surface area (SSA) by decreasing amorphous organic materials. Consequently, biochar targets the bioavailable fraction of organic pollutants. While this can be beneficial in reducing biopesticide residues in crops, it may also reduce the efficacy of biopesticides, necessitating higher application rates of these substances (Sun et al., 2021). The effectiveness of biochar on herbicides depends on the herbicide’s specific molecular properties and mode of action (Muhammad et al., 2020). Understanding the positive effects of biochar on pesticide remediation and its impact on pesticide effectiveness is crucial for pollutant remediation objectives and composite queries.
FIGURE 2. Biochar remediation of contaminants in the soil by a source pathway-receptor connection: Biochar application for soil remediation (Van Nguyen et al., 2022).
Biochar application to soil can increase negative charges on the soil surface by enhancing CEC and reducing zeta potential (Wei et al., 2023), stimulating electrostatic attraction between the soil and positively charged heavy metals. Due to the presence of various functional groups on the biochar surface, such as OH and COO, biochar forms complexes with heavy metals, reducing their bioavailability (Rizwan et al., 2020a; Rizwan et al., 2020b; Qiu et al., 2022). As a result, essential plant nutrients can become immobilized. While this can be advantageous in nutrient-rich conditions, it may be detrimental in nutrient-poor soils, leading to nutrient deficiencies. Pollutants and nutrients on the biochar surface help balance the immobilization of pollutants and nutrient deficiency in contaminated soil (Cara et al., 2022). Heavy metal precipitation, including copper, zinc, cadmium, and lead, can also increase soil pH, decreasing mobilization (Van Nguyen et al., 2022). Figure 3 illustrates different processes in biochar-amended soils, including improvements in biochar preparation in Malaysia, as presented at an international seminar on biochar (Pan et al., 2021).
The impact of biochar on soil characteristics depends on soil type and biochar composition, as illustrated in Figure 4, with quantitative effects detailed in Table 4.
The modification of soil traits depends on soil type, biochar properties, and biochar application rate (Kayiranga et al., 2023). Biochar addition to soil affects soil wettability, stability, aggregation, water retention, and water infiltration, contributing to mitigating drought, reducing nutrient losses, combating erosion, and improving groundwater quality.
Soil bulk density is an important physical trait of soil closely related to soil compaction (Xiao et al., 2023). Low soil bulk density improves soil structure, decreases soil compaction, and facilitates nutrient release and retention (Mazarji et al., 2023). Studies have shown that biochar application decreases soil bulk density compared to control samples. For instance, 25 g kg–1 biochar application reduced the soil bulk density of silt soil from 1.52 to 1.30 g cm–3 (Sun et al., 2022), with similar findings reported by Mazarji et al. (2022). Soil bulk density decreases with biochar incorporation by stimulating microbial activity and fungal growth, improving soil agglomeration, and increasing hyphae and root development (El-Naggar et al., 2021). Moreover, biochar addition to soil enhances total soil porosity (Gautam et al., 2021). The reduction in soil bulk density due to biochar application varies with soil type, biochar type, particle size, and application rate.
Soil pores provide oxygen and space for soil organisms and influence water utilization, storage, and transformation. Biochar particle size, pore distribution, and connectivity significantly affect soil pore structure (Burachevskaya et al., 2021). Sandy soil, which has poor water retention ability and large pores, experiences improved permeability and porosity after biochar addition (Gouma et al., 2022). Biochar application alters soil pore size distribution, shifting to smaller pore sizes that enhance crop growth (Baiamonte et al., 2019). For instance, adding biochar to medium- and coarse-textured soils altered soil porosity by 4–9 µm and 20 μm, increasing crop yields by 10% and 12%, respectively (Lu et al., 2023). Biochar addition to frozen soil enhanced soil porosity and pore content >0.30 mm and increased the structural stability index (Gorovtsov et al., 2020). Boguta et al. (2019) reported that adding straw biochar enhanced mesopore and macropore numbers in clay soils, and soil particles combined with biochar to form stable agglomerates. Thus, biochar addition increases soil porosity and distribution, enhances air and water circulation, improves water retention, and increases soil compaction, fertility, and productivity.
Biochar significantly influences soil aggregation (Xiao et al., 2023). Applying biochar derived from peanut hulls and corn straw at a rate of 7.80 t ha–1 enhanced the proportion of soil macroaggregates. However, the impact of biochar on aggregate stability depends on soil texture (Baiamonte et al., 2019). In one study, biochar-amended silt-loam soils exhibited increased aggregate stability, whereas sandy loam soils exhibited no substantial effect (Chen et al., 2023). In contrast, Gouma et al. (2022) reported reduced aggregate stability with biochar addition. The interaction between biochar and SOC, minerals, and microorganisms influences the impact of biochar on soil aggregation and its stability (Sun et al., 2022). Biochar provides a habitat for soil microbes, sheltering them from predators and desiccation. These microbes release polysaccharides that enhance soil aggregation (Kayiranga et al., 2023).
Soil moisture content is a critical factor in soil systems and is influenced by precipitation rates and soil texture (Hussain et al., 2020). Biochar’s high surface area and porosity reduce soil water permeability resistance, enhance soil water-holding capacity, alter water residence time (Wani et al., 2020), and change the flow path in soil (Gorovtsov et al., 2020). Biochar addition can enhance soil water-holding capacity in the field, with a more pronounced effect in sandy soils than clay soils due to sandy soil’s poor water retention ability (Bolan et al., 2022). Shafiq et al. (2023) reported that soil bulk density decreased after biochar addition (sandy soil > loamy soil), while soil water holding capacity increased. Sandy soil with 5% and 25% biochar addition retained 250% and 360% more water than control samples, respectively (Blanco-Canqui, 2021). However, the relationship between biochar application rate and water-holding capacity remains unclear. Thus, biochar can be an environmentally friendly tool for improving sandy soils in humid regions, with its effectiveness adjusted according to the biochar:soil ratio.
Biochar addition significantly influences soil pH (Bolan et al., 2022). Biochar pH typically ranges from 4 to 12, and its alkaline nature directly influences soil pH. Biochar can modulate soil pH and enhance base saturation. When added to soils, biochar can exchange with Al3+ and H+ ions in water, decreasing these ion concentrations in the soil (Ramezanzadeh et al., 2023). The presence of metal carbonates, hydroxides, and oxides in biochar increases soil pH, as negatively charged phenolic, hydroxyl, and carboxyl groups in biochar bind H+ ions from the soil solution, decreasing H+ ion activity and increasing soil pH (Patwa et al., 2021). However, there are cases where biochar addition can decrease alkaline soil pH, attributed to acid production during biochar oxidation (Khan et al., 2022). Na2CO3 and NaHCO3 contents in alkaline soils can convert CaCO3 and Ca (HCO3)2, decreasing soil pH (Blanco-Canqui, 2021). Studies have reported that acidic contents produced from soil organic matter decomposition decrease alkaline soil pH (Hussain et al., 2020). Kocsis et al. (2022) reported that biochar application increased calcareous soil pH. Similarly, biochar derived from steam activation and slow pyrolysis decreased calcareous soil pH by 0.2–0.5 units (Ji et al., 2022). Even at high biochar application rates, the soil’s buffering capacity prevented changes in the soil reaction (Zuo et al., 2022). Incorporating poultry manure-derived biochar increased alkaline soil pH, decreasing crop nutrient availability (Chen et al., 2023). In contrast to acidic soil, relatively few studies have investigated the effect of biochar on alkaline soil pH. Biochar addition to acidic soil increased soil pH to varying degrees: 4.30–4.60 (Osman et al., 2022), 4.80–6.30 (Albert et al., 2021), and 4.60–4.90 (Yadav and Bag, 2023). Thus, biochar addition can positively impact acidic and alkaline soils, depending on the specific soil conditions.
The CEC measures a soil’s capacity to retain, absorb, and exchange cations (Hossain et al., 2020). Increasing the number of soil cation exchange sites can enhance soil CEC. Soils with higher CEC are more likely to adsorb cations such as Mg2+, Ca2+, K+, and NH4+, which can improve nutrient utilization and decrease nutrient loss in soil (Egamberdieva et al., 2022). With its acidic aromatic carbon on the surface, biochar can create numerous functional groups, such as–COOH and–OH, which enhance the adsorption capacity for soil cations and, thus, CEC (Da Silva Mendes et al., 2021). Several studies have shown that biochar addition to soils enhances the total soil charge and CEC by approximately 25%–45% compared to control samples. Even small amounts of applied biochar can greatly increase soil nutrient and alkaline cation contents (Cui et al., 2022). Biochar addition to alkaline or acidic soils enhances soil CEC due to the high number of anions on the surface (Egamberdieva et al., 2022). However, biochar addition in highly organic soils may not increase soil CEC significantly, as soils with high organic matter already have a high CEC (Elkhlifi et al., 2021).
Biochar addition can increase the electrical conductivity of soil, primarily due to the release of soluble components (mineral and organic) when biochar reacts with water (Da Silva Mendes et al., 2021). For instance, 100 t ha–1 biochar enhanced soil electrical conductivity 15-fold (Farid et al., 2022). While biochar can enhance electrical conductivity in acidic soils (Abagandura et al., 2022), it may decrease it in salt-stressed soils due to physical entrapment of salts in biochar pores (Rombola et al., 2022). Biochar has been observed to alleviate salt stress effects. Under salinity stress, electrical conductivity decreased with increasing biochar application rates (Lee et al., 2022).
Biochar application can increase soil organic carbon content due to its high carbon content of recalcitrant nature (Adeniyi et al., 2022). Gross et al. (2022) reported 30%–40% higher soil organic carbon contents with varying biochar application rates. The increase in soil organic carbon with biochar addition is attributed to the cumulative effects of carbon from biochar, microbial activity, rhizosphere decomposition, and root exudates (Qianqian et al., 2022). For example, corn-derived biochar with lower volatile matter and higher ash content reduced carbon mineralization in clay soils (Bolan et al., 2022).
Biochar’s heterogeneous structure and surface properties, including basic, acidic, hydrophobic, and hydrophilic characteristics, enhance its ability to adsorb soil solution constituents, affecting fertilizer retention. Biochar can enhance nutrient retention through sorption processes. For example, bamboo-derived biochar produced at 900 °C adsorbed 1.3 mg g–1 nitrate (Enaime et al., 2020), while biochar derived from pepperwood and Arachis hulls at 600 °C decreased the total amounts of PO43–, NH4+, and NO3− in leachates by 39.1%, 14.4%, and 34.3% and 20.6%, 34.7%, and 34.0% respectively. Wood-derived biochar at 400 °C adsorbed approximately 250–430 mg g–1 phosphorus (Chen et al., 2023), and biochar derived from Spartina spartinae at 350 °C adsorbed approximately 0.5 mmol g–1 potassium. Thus, biochar can be used to decrease nutrient leaching in soil. Biochar can also enhance soil fertility by mitigating gaseous nitrogen losses (Teodoro et al., 2020). Ramadan and Abd-Elsalam (2020) reported that N2O emissions decreased by 70% after biochar application. Increased nutrient retention after biochar addition is also likely related to reduced bulk density, enhanced water storage capacity and porosity, and increased biological activities (Medha et al., 2021). The effectiveness of nutrient retention by biochar depends on application rate, pyrolysis temperature, and feedstock type.
Soil biological attributes, including microbial biomass and enzymatic activity, are vital soil health components that can be affected by biochar addition.
Biochar can directly and indirectly affect soil microbial populations (Mulabagal et al., 2022). Due to its higher aromatic hydrocarbon structure, biochar provides a conducive environment for soil microorganisms such as fungi, bacteria, and algae, which benefit from the nutrients and habitat created by biochar (Aboughaly and Fattah, 2023).
Biochar can alter the structural composition of soil microbes. Biochar surfaces contain partially soluble nitrogen (N) and carbon (C) sources suitable for microbial activities (Abukari et al., 2020). Biochar’s porous structure and high specific surface area (SSA) can retain nutrients and water, providing habitats for microbes (Manirakiza et al., 2019). Soil microorganisms can convert biochar into humus carbon, stimulating humus carbon development (Pathy et al., 2020).
While the increased amount of organic matter in soil increases microbial growth, biochar addition significantly reduces soil NO3−-N content (Mulabagal et al., 2022). Some studies have reported increases in soil microbial populations following biochar addition (Aboughaly and Fattah, 2023), while others have observed no significant change (Bamdad et al., 2022) or even a reduction (Saleem et al., 2022) in microbial populations. The effects of biochar on soil microorganisms are complex and depend on factors such as the experimental setting, biochar type, soil texture, fertility level, nutrient management, and land use patterns.
Biochar application can also influence soil enzyme activities. The interactions between biochar, enzymes, and substrates strongly affect soil enzyme activities (Sormo et al., 2021). Biochar can either limit or promote enzymatic activity based on the sorption of enzymes and substrates to different functional groups in the biochar (Krahn et al., 2023). Several studies have reported the positive impact of biochar on soil enzyme activities (Bamdad et al., 2022). Mulabagal et al. (2022) reported that biochar enhanced enzyme activities through increased organic substrates such as SOC, nitrogen pools, and microbial biomass carbon (Saleem et al., 2022). Rice husk-derived biochar at 12 t ha–1 significantly improved alkaline phosphatase, invertase, catalase, and urease activities (Krahn et al., 2023). In another study, soil invertase activity positively correlated with SOC, available phosphorus, and nitrogen (Bamdad et al., 2022). Pathy et al. (2020) reported increased phosphatase activity with biochar application, indicating an increased fraction of bioavailable P (Shikha et al., 2023). Aboughaly and Fattah (2023) showed that almond-derived biochar positively impacted urease and dehydrogenase activities. Baskar et al. (2022) found no significant relationship between SOC and enzyme activity. In another study, invertase activity positively correlated with NO3−-N and NH4+-N contents, whereas urease activity positively correlated with available phosphorus and nitrogen, attributed to stimulated root growth exuding these enzymes (Winchell et al., 2021). Dehydrogenase activity in soils incubated with biochar produced at 700 °C decreased by 48%, whereas biochar at 350 °C enhanced dehydrogenase activity by 70% (Sormo et al., 2023). Irrespective of soil type, biochar application increased alkaline phosphomonoesterase activity but inhibited acidic phosphomonoesterase activity (Qianqian et al., 2022). Lade (2023) reported that biochar treatment in alfisols increased phosphomonoesterase activity (alkaline and acidic). Biochar application to vermicompost from sewage sludge decreased heavy metal bioavailability and toxicity, enhancing earthworm growth and reproduction (Lu et al., 2023).
Biochar has gained recognition as an effective tool for eliminating various organic contaminants from soils, including pesticides, herbicides, fungicides, insecticides, industrial chemicals, aromatic dyes, VOC, and drugs/antibiotics (Dong et al., 2023), that can pose significant threats to the environment and human health.
Biochar enhances the adsorption and degradation of organic contaminants in soil. For example, the pesticide carbofuran decreased due to degradation and adsorption onto the surface of biochar particles during carbonization, increasing porosity and pesticide sorption (Lima et al., 2021). The sorption of pesticides onto biochar surfaces is likely related to the quality of phenolic and carboxylic functional groups (Panahi et al., 2020). Therefore, optimizing the quantity of biochar added to soil is crucial to enhance contaminant adsorption (Xiang et al., 2021). The processes involved in the removal of organic pollutants primarily revolve around physisorption and chemisorption mechanisms, such as repulsion/electrostatic attraction through π-π electron donor–acceptor interactions, pore diffusion, hydrophobic interactions, H bonding, and electrophilic interactions, especially with functional groups such as alcohols and carboxylic acids (Velusamy et al., 2021). Additional processes include chemical conversion and partitioning, ultimately leading to the mineralization of bonded pollutants through biodegradation (Gonzalez-Hourcade et al., 2022).
Several factors influence the interaction between biochar and organic pollutants, including the ratio of pollutants to biochar, feedstock type, pyrolysis temperature, and soil pH. Higher pyrolysis temperatures produce biochar with increased microporosity and surface area, making it particularly effective in removing non-polar carbon-based contaminants (Clurman et al., 2020). The physical properties of biochar, such as particle size and surface area, affect its sorption capabilities. Biochar with smaller particle sizes has higher SSA and, thus, better sorption effects, leading to shorter timeframes for removing contaminants (Lima et al., 2021). Moreover, soil conditions and specific biochar attributes contribute to pollutant degradation and adsorption. For instance, pesticide adsorption is more likely at lower pH (Panahi et al., 2020).
Biochar produced at temperatures exceeding 500 °C tends to have low acidity, reduced polarity, and increased aromaticity, which can degrade hydrogen- and oxygen-comprising functional groups, accelerating hydrophobic relations. Conversely, biochar produced at temperatures below 500 °C pyrolysis temperature tends to retain more hydrogen- and oxygen-bearing functional groups, making it more attractive to polar organic compounds (Tang et al., 2022). This temperature-dependent variation in biochar properties has implications for its effectiveness in removing pollutants from soil. For example, the removal of polar herbicide and insecticide compounds results from particular polar interactions and the mechanism by which various carbon-based pollutants are removed from soil using specific biochar types and their associated processes (Delgado-Moreno et al., 2021). Biochar can reduce the bioavailability of carbon-based pollutants in soil, limiting their uptake by microbes and plants (Grimm et al., 2022). Table 5 presents additional details on eliminating persistent carbon-based contaminants in soil using biochar.
Previous studies have revealed that biochar is effective in remediating contaminated soils (Tables 5, 6), particularly those polluted with persistent organic pollutants, petroleum hydrocarbons, polychlorinated biphenyls, polycyclic aromatic hydrocarbons, antibiotics, herbicides, and pesticides (Zheng et al., 2022; Zhao et al., 2023). After biochar is incorporated into polluted soil with thorough mixing, it rapidly interacts with organic pollutants and soil microbes. Organic pollutants are stabilized on the surface and within biochar pores and may be subsequently decomposed by soil microbes (Haider et al., 2022). Biochar’s abundant functional groups and porous surfaces can adsorb various organic contaminants through several mechanisms (Duwiejuah et al., 2020). As organic contaminants are adsorbed by biochar, their levels in soil water decrease, and their bio-accessibility to soil microbes diminishes. Furthermore, biochar improves soil quality and productivity by enhancing soil biological and physiochemical attributes (Qi et al., 2022; Zhao et al., 2023). Moreover, biochar introduces a substantial amount of biodegradable organic carbon (OC) as a microbial substrate while also supplying and retaining mineral nutrients (e.g., S, Mg, Ca, K, P, and N) (Yaashikaa et al., 2020). Consequently, biochar-treated soils exhibit improved microbial community structures and increased microbial activity, including microbial biomass, enzyme activity, and respiration rates (Qi et al., 2022; Rashid et al., 2022). This enhanced microbial activity leads to the microbial mineralization of various organic contaminants in soil.
Biochar alleviates/stabilizes organic pollutants through various physical/chemical sorption mechanisms (Figure 5), including London forces and electrostatic interactions between polar and non-polar molecules (Wang et al., 2023). Despite being relatively weak, London forces enhance the surface area of molecules and become more dominant as biochar particle size decreases (Chen K et al., 2022; Wu et al., 2023). Biochar contains mineral elements and functional groups (e.g., N, H, O, and C) (Table 7) that allow it to form H bonds with polar organic molecules (Figure 5). Zeng et al. (2022) reported that numerous functional groups in biochar become protonated at pH < pHZNC and dissociated at pH > pHZNC, resulting in biochar with positive and negative charges, respectively. The biochar’s surface charge facilitates the electrical attraction of ionized organic contaminants and polar organic molecules with counter-charges such as antibiotics, hormones, and pesticides. As conventionally denoted, this electrostatic interaction is usually stronger than H-bonding and dispersion force interactions (Zeghioud et al., 2022). Intensified electrostatic interactions may contribute to inner-sphere adsorption (specific surface interaction), in which ionized organic molecules react chemically with surface functional biochar groups (Zhen et al., 2023). Moreover, the aromatic carbon in biochar, which possesses excess π-electrons, can interact with electron-deficient organic compounds, particularly those containing Br, Cl, P, S, N, and O, via π-π electron donor–acceptor interactions (Hao et al., 2021).
Biochar sorbs non-ionic organic molecules through partitioning or surface adsorption. Smaller pore sizes in biochar have higher surface energy and tend to sorb organic contaminants in micropores first (Viggi et al., 2022). At low surface coverage (such as equilibrium solute amount to solute water solubility ratio ≤0.2), non-linear competitive adsorption of organic solutes into micropores in biochar’s carbonized porous surface is dominant; at higher surface coverage (i.e., equilibrium solute amount/water solubility >0.2), the adsorption shifts progressively to linear, non-competitive partition into biochar’s un-carbonized carbon moiety (Jing et al., 2022a). Pore filling is another mechanism by which biochar adsorbs organic pollutants (Figure 5). Studies have shown that the amount/degree of surface adsorption is correlated with biochar surface porosity, surface area, and aromaticity (Sanchez-Hernandez, 2021), while pore-filling adsorption relies on biochar ash and OC contents (Yi et al., 2021).
Moreover, the biochar surface may contain polyvalent metal elements, e.g., Mg, Ca, Al, and Fe (Zhang et al., 2023), and ionized or polar organic molecules can form complexes with these metal ions, leading to surface deposition. Kinetic sorption of various organic compounds onto biochars follows a pseudo-second-order pattern, suggesting the significance of surface precipitation and chemisorption (Figure 4) in biochar–organic material interactions (Viggi et al., 2022). Du et al. (2023) concluded that methyl violet sorption by two crop residue-derived biochars mainly occurred through surface precipitation and electrostatic attraction.
Biochar surface properties such as hydrophobicity, aromaticity, pore volume and size, polarity, and surface area influence its interactions with organic molecules (Sima and Jiang, 2020). Generally, biochars produced at high pyrolytic temperatures have greater surface area, hydrophobicity and aromaticity, and lower surface polarity than those produced at low temperatures due to the loss of hydrogen and oxygen-comprising functional groups (Pidlisnyuk et al., 2021). Vu and Mulligan (2023) reported that increasing pyrolysis temperatures from 200°C to 600 °C progressively increased the surface area of rice straw-derived biochar from 7.20 to 194 m2 g−1 and its sorption capacity for insecticide in the soil. The mineral ash content of biochar, particularly manure-derived biochar, can block micropores and modify its polarity, hydrophobicity, and SSA (Hao et al., 2021). Fagnano et al. (2020) reported that there was an increase in the SSA biochar derived from pig manure at 350°C and 700°C from 23.80 to 32.60 m2 g−1 and from 66.90 to 22.0 m2 g−1, respectively, but CEC level reduced from 97.0 to 113 cmolc kg−1 and from 5.60 to 8.70 cmolc kg−1, respectively. Consequently, the sorption capacities for pesticides atrazine and carbaryl of pig manure-derived biochars significantly increased (Zeghioud et al., 2022). Different feedstock-derived biochars have diverse adsorption capabilities and primary mechanisms for alleviating soil organic pollutants. Biochar mainly adsorbs hydrophobic and non-polar organic pollutants (e.g., petroleum hydrocarbons and PAHs) through the hydrophobic effect, partitioning, and pore filling. In contrast, biochar adsorbs ionized and polar organic contaminants, including antibiotics and pesticides, through surface precipitation, specific interactions, electrostatic attraction, and H bonding (Figure 5).
The efficacy of biochar in mitigating organic contaminants and facilitating their removal from soil varies with pollutant type, soil type, biochar source and type, particle size, and application rate (Table 6). For instance, biochars derived from rice hulls, soybean straw, peanut straw, and canola straw pyrolyzed at 350 °C exhibited varying sorption capabilities for methyl violet removal from soil (Beljin et al., 2023). In another study, a 5% application rate of biochars derived from wheat straw, coconut shell, and willow decreased the freely dissolved and bio-accessible fractions of PAHs in soils collected from different industrial contaminated sites by 30%–88% compared to control soils (Zhu et al., 2023). It is worth noting that biochars can contain PAHs but at relatively low and ecologically insignificant levels (∼6 0 mg kg−1) (Dai et al., 2022).
The efficiency of biochar treatment can also vary with soil type. Clay (OC = 2.4%, pH = 5.80) and sandy loam (OC = 0.55%, pH = 5.70) soils treated with 5% biochar derived from sugarcane (CEC 114 cmolc kg−1, pH 8.70; surface area 59 m2 g−1) had enhanced 17α-ethinylestradiol sorption and decreased microbial mineralization (Wang H et al., 2021). Moreover, the sandy loam soil had a notably higher adsorption efficiency for 17α-ethinylestradiol than clay, and biochar decreased the adsorption coefficient of the sandy loam soil but improved it in the clay soil (Wang L et al., 2021). In a study involving a phenanthrene-contaminated acidic soil (pH 3.20), the addition of 1% biochar (SSA = 3.50 m2 g−1; N = 2.1%) derived from burn nettle enhanced phenanthrene degradation by 40% (Tian et al., 2022). The biochar primarily acted to stabilize/alleviate metals and did not significantly adsorb the phenanthrene. However, the biochar improved soil quality and stimulated microbial activity. Valentina et al. (2021) reported that 2% rice biochar application to petroleum-contaminated soil improved microbial activity, increasing petroleum hydrocarbon biodegradation by 20%. Moreover, the biochar had a higher affinity for hydrophilic, polar organic contaminants than hydrophobic, non-polar organic molecules.
Nguyen et al. (2023) investigated the effect of biochar on biodegradation and herbicide (simazine) leaching in different soil types. Two particle size fractions of wood-derived biochar were examined: fine (<2 mm) and coarse (2–10 mm). For 0.5% and 5% biochar applications, both fractions inhibited simazine biodegradation and decreased its leaching in clay loam (OC = 3.50%, pH = 6.20) and loamy sand (OC = 1%, pH = 4.80) soils, with more notable impacts at the higher addition rate, in the clay loam soil, and for the fine particle size (Kou et al., 2023). Biochar particle size affects the uniformity of biochar distribution in soil, affecting its remediation efficiency (Nguyen et al., 2023).
Incorporating biochar into the soil through mechanical mixing achieved more uniform biochar distribution than shovel or hand mixing, increasing polychlorinated biphenyl retention in the soil by 2.80% and decreasing the bioaccumulation of polychlorinated biphenyls in pumpkin roots by 50% (Keerthanan et al., 2021).
Thus, biochars produced at higher pyrolysis temperatures from plant and wood wastes generally have higher aromaticity levels, porosity, and SSA than manure-derived biochars, making them preferable for sorbing organic pollutants. Over time, biochar enhances mineralization and ultimately removes organic pollutants from soils by increasing soil microbial activities (Tian et al., 2022).
Numerous studies have shown that biochar affects nutrient availability, with the potential as a moderate-release fertilizer in soils. Nutrient release from biochar depends on its desorption characteristics, with several factors affecting nutrient desorption from biochar (Issaka et al., 2022). For example, Zhang et al. (2022) reported that the NH4+ desorption ratio from Betula-derived biochar increased from 17% to 30% when the pyrolysis temperature decreased from 500 °C to 300°C, indicating that lower pyrolysis temperatures can increase nutrient desorption from biochar.
Brtnicky et al. (2021) studied P desorption from black or regur soil, reporting that the average desorbed P increased from 35% to 40% when the biochar addition rate increased from 0% to 10%. Approximately 70% of the P adsorbed by biochar was released at higher P loadings. These findings indicate that increasing the P loadings and biochar addition ratio can enhance P desorption from biochar. Furthermore, different feedstock types used to produce biochar can have varying effects on nutrient desorption. For instance, biochar derived from cacao shell desorbed 1,485 mg g–1 phosphate, while biochar derived from corn cobs desorbed 170 mg g–1 phosphate (Razzaq et al., 2022). Thus, the desorption properties of biochar depend on factors such as biochar application rate, feedstock type, and preparation temperature. Based on the research on desorption characteristics, it is possible to select different types of biochar for specific soil nutrient management purposes. Biochar can be applied to maintain various soil nutrients in the same soil or preferentially in different soils to optimize nutrient supply. This flexibility makes biochar a valuable tool for sustainable soil management and improving nutrient availability in agricultural soils.
Biochar is highly effective in the remediation of toxic components from gases. Various types of biochar, including those derived from pig manure, poultry manure, hardwood chips, camphor, sludge, bamboo, and rice hulls, have demonstrated efficient removal (>90%) of hydrogen sulfide (H2S) from biogas, with sorption capacities ranging from 100 to 380 mg H2S g–1 biochar (Das et al., 2020). The amount of H2S removal is influenced by biochar moisture content, chemical bonding, and surface area with functional groups. H2S reacts with the alkali-based biochar surface through ionic interactions with–COOH and–OH functional groups in water and oxygen to form Na2SO4 and K2SO4 (Gao et al., 2022). Biochars derived from soybean straw and peanut shells prepared at high pyrolysis temperature (700 °C) were more effective for trichloroethylene removal than biochars produced at low pyrolysis temperatures (300 °C) due to their increased surface area and hydrophobicity and decreased polarity (Ambika et al., 2022).
GHG emissions are a significant driver of climate change, with CO2 emissions contributing to over 70% of these emissions (Atilano-Camino et al., 2022). Reducing CO2 pollutants from agricultural soil is crucial for mitigating climate change. Biochar can reduce CH4 and N2O emissions and enhance soil C sequestration (Romero et al., 2021). However, its effects on soil GHG emissions can differ depending on the feedstock type, pyrolysis temperature, and water contents (Hua et al., 2021). While there are numerous studies on the effects of biochar on soil CO2 emissions, the outcomes are not always significant due to variations in methodologies and research materials. Reducing GHG emissions through biochar application is a multifaceted process and is becoming better understood through systematic research (Ginebra et al., 2022). One study reported that adding biochar to soil enhances the activity of microbes involved in reducing N2O to N (Sarauer et al., 2019). However, the alkaline nature of biochar can also enhance the activity of N2O-decreasing organisms. In cases where soil pH is increased, the disadvantages of biochar may lead to reduced soil emissions and acidity (Cui et al., 2021). Biochar provides ample sites for N and N2O removal due to its high SSA, decreasing the release of these gases from the soil environment (Levesque et al., 2020).
The physiochemical characteristics of biochars affect their adsorption capabilities. For example, enhanced acidic (e.g., hydroxyl and carboxyl) functional groups in biochar increase NH4+ sorption (Danesh et al., 2022). Biochar has numerous functional groups, high stability, and SSA, influenced by the pyrolysis process and feedstock type (Lopes and Astruc, 2021). Various feedstocks, including poultry manure, organic waste, wood chips, and plant residues, can be used to prepare biochar. Pyrolysis temperatures typically range from 250 °C to over 750 °C (Shan et al., 2020). Table 7 presents the effects of the pyrolysis process and feedstock type on biochar properties.
The CEC reflects the ability of biochar to adsorb cations, such as Ca2+ and NH4+, which are essential for plants. A high biochar CEC can reduce nutrient losses from soil leaching (Gabhane et al., 2020). CEC values of biochar vary based on factors such as feedstock type and pyrolysis temperature. For example, the CEC of biochar produced from Spartina increased from 7 to 45.4 cmo1c kg–1 and then decreased to 31 cmo1c kg–1 as the pyrolysis temperature increased from 250°C to 600 °C. Oatmeal pine biochars prepared at 200, 300, 350, and 600 °C had CEC values of 16.5, 16.9, 23.8, and 2.1 cmo1c kg–1, respectively (Patwardhan et al., 2022). In addition, sugarcane biochar had a CEC that increased from 6.45 cmo1c kg–1 (at 250 °C) to 8.99 cmo1c kg–1 (at 500 °C) and then decreased to 5.10 cmo1c kg–1 (at 600 °C) (Kamarudin et al., 2022). These findings suggest that biochars prepared at higher temperatures tend to have lower CEC values (Danesh et al., 2022). The reduction in CEC in biochar produced at higher temperatures is attributed to aromatization and the loss of functional groups (hydroxyl and carbonyl) (Kang et al., 2022). One study reported that biochar prepared at temperatures of up to 480 °C retained carboxyl and phenolic acid groups (acidic-oxygenated functional groups) (Li et al., 2022). Lu et al. (2022) demonstrated that biochar CEC depends on its surface distribution and oxygen-enrich functionalities, with negative charge sites on biochar surfaces accredited to phenolate and carboxylate functional groups (Kamali et al., 2022). Some studies have reported that biochars with higher SSA (produced at temperatures ≥600 °C) have enhanced CEC due to increased surface microporosity (Shaheen et al., 2022) despite the loss of volatile matter (Samoraj et al., 2022). Biochar CEC also depends on feedstock type. For instance, pig manure-derived biochar had a lower CEC (32 cmo1c kg–1) than chicken manure biochar (81 cmo1c kg–1) produced at 500 °C (Yaro et al., 2023). However, paper mill-derived biochar had a markedly lower CEC (9–18 cmo1c kg–1) than sugarcane-derived biochar (112 cmo1c kg–1) (Chai et al., 2021). This variation was attributed to higher ash contents in feedstock, resulting in biochar with greater CEC values (Behl et al., 2020).
The biochar preparation temperature significantly influences the surface functional groups of biochar, which affect the sorption properties of biochar (Bao et al., 2022). Pyrolysis temperatures ranging from 350°C to 650 °C rearrange and break chemical bonds in the feedstock, creating various functional groups (e.g., pyrrole, pyridone, pyridine, pyrone, ether, phenol, anhydride, chromene, quinine, lactol, lactone, and carboxyl) (Zhou et al., 2021). Generally, high pyrolysis temperatures decrease O and H contents and the molar H/C ratio, with a notable reduction in polar functional groups such as C-O and–OH (Fang et al., 2021). Fourier-transform infrared (FTIR) analysis of biochar often reveals the dominance of functional groups containing oxygenated hydrocarbons, reflecting the carbohydrate structure of hemicelluloses and cellulose (Kumari et al., 2023). For example, sawdust-derived biochar had a broad band between 3,000 cm−1 and 3,600 cm−1, with a smaller band between 2,700 cm−1 and 3,000 cm−1 (Vanapalli et al., 2021). The band centered at 3,339 cm−1 was accredited to the presence of phenolic and alcoholic functional groups (Yang et al., 2021), while the band at 2,907 cm−1 corresponded to alkyl C-H stretching. Another band arising at 1,600 cm−1 was associated with aromatic C–O and C–C stretching of conjugated quinones and ketones (Azeem et al., 2022), a band at 1,735 cm−1 was related to C=O stretching of esters, aldehydes, and ketones (Zahed et al., 2021), and a band arising at 1,238 cm−1 was attributed to the presence of C–O–C groups and phenolic, aryl ethers linked with lignin (Wang H et al., 2021).
Biochars produced at high temperatures (e.g., 600°C and 700 °C) exhibit hydrophobic natures with well-ordered carbon layers (Xu et al., 2021). However, they contain lower amounts of O- and H-comprising functional groups due to the deoxygenation and dehydration of biomass during pyrolysis (Natasha et al., 2022). These surface functional groups can function as electron acceptors and donors, creating co-existing zones with attributes ranging from hydrophilic to hydrophobic and acidic to basic (Van Nguyen et al., 2022). Consequently, biochar produced at high temperatures may have a lower ion exchange capability (Wang L et al., 2021). In contrast, biochar produced at lower temperatures (300°C and 400 °C) exhibits a more diverse organic nature due to cellulose and aliphatic-based structures. These biochars tend to have well-organized carbon layers and a higher content of surface functional groups than those produced at higher temperatures (Sun et al., 2021).
The pH of biochar is typically alkaline, with values ranging from 7 to 10, but variations in pH can occur due to pyrolysis temperature and feedstock type (Muhammad et al., 2020). For instance, biochars derived from soybean, peanut, and corn straw at 300 °C had pH values of 7.70, 8.60, and 9.40, respectively, while corn straw derived-biochar was acidic (Wei et al., 2023). Woody biomass-derived biochars had average pH values two units lower than non-woody biomass-derived biochars under similar carbonization conditions (Qiu et al., 2022). Biochars derived from non-woody biomass sources had higher pH values (around three units), attributed to salts like calcium and potassium chlorides and carbonates in the ash (Cara et al., 2022). Sugarcane-derived biochar had a lower pH (8.5) than rice husk-derived biochar (9.0), possibly due to its lower ash content (19.10% vs. 40%) (Kayiranga et al., 2023). The increase in pH values could be due to the concentration of non-pyrolyzed inorganic components and the decomposition of the organic matrix (Xiao et al., 2023). Biochar pH is likely associated with its cellulose, hemicellulose, and lignin contents and oxygen-based functional groups (Sun et al., 2022). El-Naggar et al. (2021) stated that the -O- and -COO groups and carbonate content in biochar were responsible for their alkaline nature. Gouma et al. (2022) reported that biochar pH positively correlated with oxygen content (R2 = 0.7), consistent with findings that oxygen-containing functionalities, including quinine, diketone, chromene, and pyrone, are responsible for the basicity of biochar (Kumari et al., 2023). The carbonization process during biochar production can lead to the formation of oxygen-rich functionalities (Burachevskaya et al., 2021), and this process is associated with the condensation reactions of aliphatic elements and the dehydration of feedstock (Bandara et al., 2020). Moreover, rice straw and rice bran-derived biochar pH values were negatively correlated with anomeric (O-C-O) and aliphatic O-alkylated carbons but positively correlated with aromatic C-O and fused ring aromatic structures (Zheng et al., 2022). Thus, numerous carboxylic groups in produced biochars that decrease during carbonization and acidic groups that turn into deprotonated to the conjugated bases result in a more alkaline pH of biochars (Venkatesh et al., 2022).
Biochar application can increase soil pH by increasing cation retention (e.g., K+, Mg2+, and Ca2+) (Armah et al., 2022). Biochars prepared at higher pyrolysis temperatures have greater pH due to the release of alkali salts from organic biomass substances (Kamarudin et al., 2022). For example, the pH value of maize straw biochar increased from 8 to 11.5 when the preparation temperature increased from 350°C to 550 °C (Kamali et al., 2022). Similarly, pig waste biochar prepared at 500°C and 700 °C had pH values of 8.55–10.53, respectively (Vijayaraghavan, 2019). Thus, biochar with a high pH and CEC can potentially preserve K+ and NH4+ fertilizers and enhance their effectiveness when applied to soil (Varjani et al., 2019).
Specific surface area is an essential biochar attribute influenced by pyrolysis conditions and feedstock/biomass type (Balajii and Niju, 2019). A high SSA helps biochar to adsorb substances, such as organic compounds and heavy metals (Atilano-Camino et al., 2022). Different feedstocks and pyrolysis temperatures can result in variations in SSA. For example, vine pruning and orange pomace-derived biochars with low ash contents had lower SSA values (8.10 and 1.20 m2 g–1, respectively) (Jing et al., 2022b), possibly indicating less porous biochar (Albalasmeh et al., 2020). Enhanced biochar porosity is attributed to lignin decomposition, rapid release of CH4 and H2, and reactions involving aromatic condensation as the pyrolysis temperature increases (Rizwan et al., 2020a; Amusat et al., 2021). For example, sugarcane-derived biochar had a larger SSA and pore size (253 and 0.1 m2 g–1, respectively) than coconut shell-derived biochar (25.80 and 0.1 m2 g–1, respectively) (Velusamy et al., 2021). Similarly, sugarcane-derived biochar had a higher SSA and larger pore size (185 and 0.1 m2 g–1, respectively) than rice husk-derived biochar (157 and 0.1 m2 g–1, respectively). (Gonzalez-Hourcade et al., 2022), reflecting differences in thermal degradation, cellulose, and lignin content (Sun et al., 2021). Biochar derived from peanut shells and pine cones had larger SSA values (2.0 and 1.8 m2 g–1, respectively) than biochar derived from corn stalks and cake (0.8 and 0.5 m2 g–1, respectively) (Tang et al., 2022), likely due to the large quantity of lignin in the peanut shells and pine cones (Kumar et al., 2023). Biochar derived from apricot stones, grapes, and cobnuts shells had higher SSA values (11.60, 14.5, and 14.70 m2 g–1, respectively), pore volumes (0.2, 0.2, and 0.1 cm g–1, respectively), and porosity values (all 0.1%) than their respective biomasses (SSA: 10.60, 5.80, and 10.50 m2 g–1, respectively; pore volume: all 0.1 cm g–1; and porosity: all 0.1%) (Jeyasubramanian et al., 2021), attributed to varying lignin and cellulose degradation (Goswami et al., 2022).
Increasing the pyrolysis temperature can enhance micropore formation and SSA in biochar. For example, the SSA of sugarcane-derived biochar increased from 0.55 to 13.2 m2 g–1 as the pyrolysis temperature increased from 300°C to 700 °C (Kumari et al., 2023). Similarly, soybean-derived biochar prepared at 650 °C had an SSA of 419 m2 g–1, much higher than that prepared at 400 °C (Danesh et al., 2022).
The choice of feedstock also plays a role in determining SSA. Biochar prepared from cocopeat had a lower SSA (13.7 m2 g–1) compared to that from bagasse (202 m2 g–1), attributed to the volatiles released from the biomass during pyrolysis (70% and 87%, respectively) (Kamarudin et al., 2022). The release of volatile substances, particularly those produced from hemicellulose and cellulose during pyrolysis, can enhance the formation of a vascular bundle structure in biochar and improve its pore structure and SSA (Armah et al., 2022). Reducing the volatile content within Euphorbia mammillaris and corncob biochar increased the surface area by 60.7–193.1 m2 g–1 (Bao et al., 2022).
Biochar stability helps determine its carbon sequestration ability (Nzediegwu et al., 2021). The pyrolysis temperature used in the carbonization process has been considered evidence of biochar stability (Nzediegwu et al., 2022). This proximate analysis is a traditional method used to assess the nature of the fixed carbon, ash, charcoal, moisture, and volatile matter in biochar. However, it requires high temperatures (around 900°C–950°C for volatile matter and 700°C–800°C for ash determination) for an extended period (Janu et al., 2021), which can overestimate carbon content and underestimate ash content (Da et al., 2022). Techniques for evaluating the stability of biochars can be divided into three classes: i) quantification of carbon structures, particularly those with aromatic characteristics as they are considered relatively stable and less prone to degradation; ii) quantification of stable carbon using thermochemical/thermal degradation and chemical oxidation; and iii) biochar incubation in soil and carbon mineralization modeling (Fu et al., 2019). Biochar stability is associated with the carbon structure within the biochar, which contains amorphous and crystalline phases (Bakshi et al., 2020; Islam et al., 2021).
Biochar stability can vary depending on the feedstock type and pyrolysis temperature (Petersen et al., 2023). Higher pyrolysis temperatures generally lead to more stable biochar (Gopal et al., 2020). For instance, increasing the pyrolysis temperature from 300°C to 600 °C significantly improved the stability of sugarcane-derived biochar (Gluckler et al., 2021). In contrast, biochar prepared at lower pyrolysis temperatures is more susceptible to degradation (Mai et al., 2019).
The elemental composition of biochar is a function of pyrolysis temperature and feedstock species (Almutairi et al., 2023). Higher pyrolysis temperatures typically increase the carbon and ash contents of biochar (Muigai et al., 2021). Moreover, different feedstock species contribute varying elemental amounts to the resulting biochar. For instance, chicken waste-derived biochar has higher Mg, Ca, K, P, and N contents than woodchip biochar, while woodchip biochar has a higher total carbon content (Choudhary et al., 2019; Rodriguez et al., 2021).
The nitrogen content of biochar can also be influenced by pyrolysis temperature, with lignocellulosic-rich biochar showing a moderate increase as the pyrolysis temperature increased, while biochar derived from sewage sludge and animal manure followed a downward trend (Stylianou et al., 2020). Pyrolysis conditions, including residence time and temperature, can affect the accumulation and release of specific elements. For example, a longer residence time (about 60 min) and higher temperature accumulated K and P, released Si, Mg, and Ca, and retained S, Mn, and Fe (Hassan et al., 2020).
Similarly, increased pyrolysis temperature and reaction time removed some unstable constituents (comprising O and H elements) of feedstock due to decarboxylation, dehydration, and deoxygenation reactions, resulting in VOC losses and decreased H/C and O/C ratios (Venkatesh et al., 2022), contributing to a more stable graphite structure, improved aromatic structure, and greater carbonization level in the biochar (Balajii and Niju, 2019). For instance, ∼400°C–500°C pyrolysis temperatures resulted in O/C ratios ranked as follows: Oryza sativa straw > O. sativa husk > apple tree > oak tree (Albalasmeh et al., 2020). Thus, wood-derived biochar (oak tree and apple tree), with higher lignin contents and slower mineralization rates, had lower O/C ratios than herb-derived biochar (rice husk and rice straw) and sewage sludge-derived biochar and thus greater stability (Jing et al., 2022a).
Biochar plays a crucial role in the bioeconomy, which involves the sustainable use of bioresources to create economically valuable bioproducts (Rex et al., 2023). Biomass (feedstock), as the primary bioresource, is transformed into various bioproducts, with biochar being one of the most significant (Han et al., 2021). The bioeconomy encompasses activities such as awareness promotion, marketing, commercialization, and production, all of which contribute to job creation, both indirectly and directly (Sri Shalini et al., 2021). In the context of the bioeconomy, the safety, quantity, and quality of bioproducts, including biochar, affect its success. Efficient biochar production has economic and agronomic advantages. For instance, using biochar in crop cultivation can increase yields and generate additional income, enhancing economic stability (Seow et al., 2022). The global energy demand continues to grow with the increasing global population (Hamidzadeh et al., 2023), making sustainable energy sources essential. Feedstock is one such energy source that can be converted using thermochemical, physical, biochemical, and mechanical methods. Thermochemical transformation is particularly effective, breaking chemical bonds in carbon-based substances to produce syngas, bio-oil, and biochar. Biochar, in particular, is gaining prominence due to its sustainability benefits, financial advantages, and growing demand in the energy and environmental sectors (Kwon et al., 2020).
While developed nations have progressed significantly in advancing their bioeconomies, many developing nations need to raise awareness and increase bioproduct production (Amalina et al., 2022). As a profitable and commercially viable material, biochar finds applications in energy, manufacturing, and agriculture. Biochar production can help improve soil quality, providing opportunities for additional profit. Using forest biomass-derived biochar as a soil amendment in agriculture can significantly impact the economy, especially in forest and agricultural sectors, and stimulate related industries, such as biochar equipment manufacturing and carbon sequestration (Anastopoulos et al., 2019). Studies have shown that CO2 sequestration remittance could enhance biochar efficiency (Kwon et al., 2020). Biochar’s potential application for enriching soils and enhancing crop production appears promising (Sieradzka et al., 2022), as it can improve water and soil retention, decrease irrigation and fertilizer costs, and rejuvenate depleted soils (Ghodake et al., 2021).
Feedstock pyrolysis produces two key byproducts: oil and syngas, both of which have applications in renewable energy and fuel (Schmidt et al., 2021). Syngas can be used as an input in the pyrolysis process and as a heating source in drying methods. Oil can be used as a standalone product, but emissions can be heavy in black carbon and particulates. Therefore, it is essential to refine oil into biodiesel for use as fuel in transportation (Song et al., 2021).
The economic significance of biochar production is influenced by various factors, with transportation playing a significant role. Campion et al. (2023) conducted an economic analysis of biochar production and transportation costs. They assessed the feasibility of mobile pyrolysis facilities by analyzing two types of biochar in three states with frequent transportation needs. Their analysis included shipping logistics based on GIS data and used a Monte Carlo financial simulation model. They found that the net present value of biochar production often increases when the mobile pyrolysis facility is located closer to the biomass source (Allohverdi et al., 2021). Figure 6 illustrates the economic benefits of biochar utilization.
FIGURE 6. Economic profits of biochars derived from various feedstocks. Source: sugar beet tailing (Ramanayaka et al., 2020) Rice shell (Scaria et al., 2022) wood-chips (Gouma et al., 2022) fruit bunches (Qiu et al., 2022) Human manure (Mazarji et al., 2022) wood barks (Xiao et al., 2023) dairy residue (Rex et al., 2023) poultry waste (Han et al., 2021).
Biochar production has garnered attention due to its potential in the environmental and energy sectors. Seow et al. (2022) presented economic assumptions for biochar production in Selangor, Malaysia, estimating annual costs at US$ 532 and total revenue from biochar sales at US$ 8,012 (Table 8). Consequently, the net value of biochar production demonstrated positive economic feasibility for biochar (Seow et al., 2022). The cost-effectiveness of biochar production also depends on factors such as selling price. Tang et al. (2022) identified a break-even selling price of approximately US$ 220 per ton for biochar produced at 300 °C and approximately US$ 280 per ton for biochar produced at 450 °C. Dong et al. (2023) reported promising net margins for biochar production using agricultural residues such as horse, cattle, and livestock manure, with net margins of US$ 69 and US$ 162 for high and low revenue scenarios of CO2, respectively. Thus, the economic viability of biochar production depends on various factors, including the costs associated with biomass collection, storage, transportation, carbonization, application, and processing (Jing et al., 2022b). Research suggests that the net margin of biochar production can be enhanced by using low-cost biomass sources and adopting efficient processing technologies (Venkatesh et al., 2022).
TABLE 8. Economic investigation of biochar production in three states of Malaysia (Rashid et al., 2022).
Biochar is a versatile product derived from various feedstock materials and pyrolyzed under different conditions, resulting in a wide range of chemical and physical properties. These properties make biochar a valuable tool for improving soil quality, enhancing carbon sequestration, mitigating soil erosion, promoting photosynthesis, reducing urban heat island effects, and decreasing GHG emissions. One of its key benefits is its ability to reduce the mobility and bioavailability of inorganic and organic contaminants in soils.
The specific pyrolysis conditions and the feedstock type strongly influence the quality and quantity of biochar. Therefore, it is crucial to consider these factors when using biochar in soil improvement and remediation efforts. Understanding how specific biochars immobilize contaminants over time as their adsorption sites interact with native soil organic matter and competing contaminants is essential for effective long-term application.
Biochar plays a significant role in the bioeconomy concept, which focuses on the sustainable exploitation and exploration of bioresources to create valuable products using biotechnology. As a commercially viable bio-product (biogas and bio-oil) with applications in energy, various industries, and agriculture, biochar production can benefit soils and provide opportunities for additional income. Furthermore, biochar offers advantages such as ease of transport, cost-effectiveness compared to traditional fertilizers, and long-lasting effects on soil improvement. Introducing strategies related to CO2 sequestration costs can further incentivize the use of biochar in various applications.
The future outlook for biochar holds significant promise and potential for further advances in environmental and agricultural applications. Several important areas of research and exploration should be considered to harness these benefits, including:
1. Risk assessment and mitigation: As biochar is produced from various feedstocks, some of which may be contaminated, it is crucial to conduct further studies to assess the associated risks.
2. In-depth characterization: Systematic research is needed to investigate the relationships between biomass types, carbonization conditions, and the physiochemical attributes of biochar, including biochar stability and structure and lignin, cellulose, and hemicellulose contents. Moreover, novel statistical analysis techniques, such as machine learning, could be adapted to help predict and optimize biochar properties for specific uses.
3. Innovative carbonization techniques: Experiments are needed to investigate the feasibility of combining novel carbonization techniques with modification approaches to generate more efficient biochars. These methods include co-pyrolysis and microwave-assisted modifications to develop modified biochars with enhanced properties, such as higher surface areas and reduced environmental risks.
4. Field validation: Most research on biochar effects has been conducted under controlled laboratory conditions; thus, validating these findings under real-world field conditions is essential. Field experiments should encompass diverse soil types and agro-climatic zones to better understand how biochar performs under varying environmental conditions.
MR: Original draft. GM: Original draft. FZ: Review and editing. AM: Review and editing. RI: Review and editing. ZA: Review and editing. SI: Review and editing. IK: Review and editing. TL: Review and editing. JC: Review and editing. MZ: Review and editing. KHMS: Resources and supervision. LL: Resources and supervision. HL: Resources and supervision.
The author(s) declare financial support was received for the research, authorship, and/or publication of this article. This work was supported by the Science and Technology Innovation Program of Hunan Province (2021RC4005) and the Open Project of Xiangjiang Laboratory (No. 22XJ03003).
The authors declare that the research was conducted in the absence of any commercial or financial relationships that could be construed as a potential conflict of interest.
All claims expressed in this article are solely those of the authors and do not necessarily represent those of their affiliated organizations, or those of the publisher, the editors and the reviewers. Any product that may be evaluated in this article, or claim that may be made by its manufacturer, is not guaranteed or endorsed by the publisher.
Abagandura, G. O., Bansal, S., Karsteter, A., and Kumar, S. (2022). Soil greenhouse gas emissions, organic carbon and crop yield following pinewood biochar and biochar–manure applications at eroded and depositional landscape positions: a field trial in South Dakota, USA. Soil Use Manag. 38 (1), 487–502. doi:10.1111/sum.12760
Aboughaly, M., and Fattah, I. M. R. (2023). Production of biochar from biomass pyrolysis for removal of PFAS from wastewater and biosolids. A Crit. Rev. 2023, 2023040309. doi:10.20944/preprints202304.0309.v1
Abukari, A., Abunyewa, A. A., and Yeboah, E. (2020). Influence of integrated soil fertility management on the vegetative growth parameters of Zea mays in the Guinea savanna eco-zone of Ghana. J. Agric. Sci. Belgr. 65 (2), 187–197. doi:10.2298/jas2002187a
Adeniyi, A. G., Abdulkareem, S. A., Iwuozor, K. O., Ogunniyi, S., Abdulkareem, M. T., Emenike, E. C., et al. (2022). Effect of salt impregnation on the properties of orange albedo biochar. Clean. Chem. Eng. 3, 100059. doi:10.1016/j.clce.2022.100059
Albalasmeh, A., Gharaibeh, M. A., Mohawesh, O., Alajlouni, M., Quzaih, M., Masad, M., et al. (2020). Characterization and Artificial Neural Networks Modelling of methylene blue adsorption of biochar derived from agricultural residues: effect of biomass type, pyrolysis temperature, particle size. J. Saud. Chem. Soc. 24 (11), 811–823. doi:10.1016/j.jscs.2020.07.005
Albert, H. A., Li, X., Jeyakumar, P., Wei, L., Huang, L., Huang, Q., et al. (2021). Influence of biochar and soil properties on soil and plant tissue concentrations of Cd and Pb: a meta-analysis. Sci. Total Environ. 755, 142582. doi:10.1016/j.scitotenv.2020.142582
Allohverdi, T., Mohanty, A. K., Roy, P., and Misra, M. (2021). A review on current status of biochar uses in agriculture. Molecules 26 (18), 5584. doi:10.3390/molecules26185584
Almutairi, A. A., Ahmad, M., Rafique, M. I., and Al-Wabel, M. I. (2023). Variations in composition and stability of biochars derived from different feedstock types at varying pyrolysis temperature. J. Saud. Soc. Agric. Sci. 22 (1), 25–34. doi:10.1016/j.jssas.2022.05.005
Al-Rabaiai, A., Menezes-Blackburn, D., Al-Ismaily, S., Janke, R., Pracejus, B., Al-Alawi, A., et al. (2022). Customized biochar for soil applications in arid land: effect of feedstock type and pyrolysis temperature on soil microbial enumeration and respiration. J. Anal. Appl. Pyrol. 168, 105693. doi:10.1016/j.jaap.2022.105693
Amalina, F., Abd Razak, A. S., Krishnan, S., Sulaiman, H., Zularisam, A. W., and Nasrullah, M. (2022). Biochar production techniques utilizing biomass waste-derived materials and environmental applications–A review. J. Hazard. Mat. Adv. 7, 100134. doi:10.1016/j.hazadv.2022.100134
Ambika, S., Kumar, M., Pisharody, L., Malhotra, M., Kumar, G., Sreedharan, V., et al. (2022). Modified biochar as a green adsorbent for removal of hexavalent chromium from various environmental matrices: mechanisms, methods, and prospects. Chem. Eng. J. 135716. doi:10.1016/j.cej.2022.135716
Amusat, S. O., Kebede, T. G., Dube, S., and Nindi, M. M. (2021). Ball-milling synthesis of biochar and biochar–based nanocomposites and prospects for removal of emerging contaminants: a review. J. Water Process Eng. 41, 101993. doi:10.1016/j.jwpe.2021.101993
Anae, J., Ahmad, N., Kumar, V., Thakur, V. K., Gutierrez, T., Yang, X. J., et al. (2021). Recent advances in biochar engineering for soil contaminated with complex chemical mixtures: remediation strategies and future perspectives. Sci. Total Environ. 767, 144351. doi:10.1016/j.scitotenv.2020.144351
Anastopoulos, I., Pashalidis, I., Hosseini-Bandegharaei, A., Giannakoudakis, D. A., Robalds, A., Usman, M., et al. (2019). Agricultural biomass/waste as adsorbents for toxic metal decontamination of aqueous solutions. J. Mol. Liq. 295, 111684. doi:10.1016/j.molliq.2019.111684
Armah, E. K., Chetty, M., Adedeji, J. A., Estrice, D. E., Mutsvene, B., Singh, N., et al. (2022). “Biochar: production, application and the future,” in Biochar-productive technologies, properties and application (Intech Open).
Atilano-Camino, M. M., Canizales Laborin, A. P., Ortega Juarez, A. M., Valenzuela Cantú, A. K., and Pat-Espadas, A. M. (2022). Impact of soil amendment with biochar on greenhouse gases emissions, metals availability and microbial activity: a meta-analysis. Sustainability 14 (23), 15648. doi:10.3390/su142315648
Azadi, N., and Raiesi, F. (2021). Biochar alleviates metal toxicity and improves microbial community functions in a soil co-contaminated with cadmium and lead. Biochar 3 (4), 485–498. doi:10.1007/s42773-021-00123-0
Azeem, M., Shaheen, S. M., Ali, A., Jeyasundar, P. G., Latif, A., Abdelrahman, H., et al. (2022). Removal of potentially toxic elements from contaminated soil and water using bone char compared to plant-and bone-derived biochars: a review. J. Hazard. Mat. 427, 128131. doi:10.1016/j.jhazmat.2021.128131
Baiamonte, G., Crescimanno, G., Parrino, F., and De Pasquale, C. (2019). Effect of biochar on the physical and structural properties of a sandy soil. Catena 175, 294–303. doi:10.1016/j.catena.2018.12.019
Bakshi, S., Banik, C., and Laird, D. A. (2020). Estimating the organic oxygen content of biochar. Sci. Rep. 10 (1), 13082–13112. doi:10.1038/s41598-020-69798-y
Balajii, M., and Niju, S. (2019). Biochar-derived heterogeneous catalysts for biodiesel production. Environ. Chem. Lett. 17 (4), 1447–1469. doi:10.1007/s10311-019-00885-x
Balmuk, G., Videgain, M., Manyà, J. J., Duman, G., and Yanik, J. (2023). Effects of pyrolysis temperature and pressure on agronomic properties of biochar. J. Anal. Appl. Pyrol. 169, 105858. doi:10.1016/j.jaap.2023.105858
Bamdad, H., Papari, S., Moreside, E., and Berruti, F. (2022). High-temperature pyrolysis for elimination of per-and polyfluoroalkyl substances (PFAS) from biosolids. Processes 10 (11), 2187. doi:10.3390/pr10112187
Bandara, T., Franks, A., Xu, J., Bolan, N., Wang, H., and Tang, C. (2020). Chemical and biological immobilization mechanisms of potentially toxic elements in biochar-amended soils. Crit. Rev. Environ. Sci. Technol. 50 (9), 903–978. doi:10.1080/10643389.2019.1642832
Bao, Z., Shi, C., Tu, W., Li, L., and Li, Q. (2022). Recent developments in modification of biochar and its application in soil pollution control and ecoregulation. Environ. Pollut. 313, 120184. doi:10.1016/j.envpol.2022.120184
Baskar, A. V., Bolan, N., Hoang, S. A., Sooriyakumar, P., Kumar, M., Singh, L., et al. (2022). Recovery, regeneration and sustainable management of spent adsorbents from wastewater treatment streams: a review. Sci. Total Environ. 822, 153555. doi:10.1016/j.scitotenv.2022.153555
Behl, K., SeshaCharan, P., Joshi, M., Sharma, M., Mathur, A., Kareya, M. S., et al. (2020). Multifaceted applications of isolated microalgae Chlamydomonas sp. TRC-1 in wastewater remediation, lipid production and bioelectricity generation. Bioresour. Technol. 304, 122993. doi:10.1016/j.biortech.2020.122993
Behnam, H., and Firouzi, A. F. (2022). Effects of synthesis method, feedstock type, and pyrolysis temperature on physicochemical properties of biochar nanoparticles. Biomass Conv. bioref. 13, 13859–13869. doi:10.1007/s13399-021-02108-2
Beljin, J., Isakovski, M. K., Zeremski, T., Đukanović, N., Apostolović, T., Rončević, S., et al. (2023). The efficiency of the hard wood origin biochar addition on the PAHs bioavailability and stability in sediment. J. Hazard. Mat. Adv. 10, 100276. doi:10.1016/j.hazadv.2023.100276
Blanco-Canqui, H. (2021). Does biochar improve all soil ecosystem services? GCB Bioenerg. 13 (2), 291–304. doi:10.1111/gcbb.12783
Boguta, P., Sokołowska, Z., Skic, K., and Tomczyk, A. (2019). Chemically engineered biochar–Effect of concentration and type of modifier on sorption and structural properties of biochar from wood waste. Fuel 256, 115893. doi:10.1016/j.fuel.2019.115893
Bolan, N., Hoang, S. A., Beiyuan, J., Gupta, S., Hou, D., Karakoti, A., et al. (2022). Multifunctional applications of biochar beyond carbon storage. Int. Mat. Rev. 67 (2), 150–200. doi:10.1080/09506608.2021.1922047
Brtnicky, M., Datta, R., Holatko, J., Bielska, L., Gusiatin, Z. M., Kucerik, J., et al. (2021). A critical review of the possible adverse effects of biochar in the soil environment. Sci. Total Environ. 796, 148756. doi:10.1016/j.scitotenv.2021.148756
Burachevskaya, M., Mandzhieva, S., Bauer, T., Minkina, T., Rajput, V., Chaplygin, V., et al. (2021). The effect of granular activated carbon and biochar on the availability of Cu and Zn to Hordeum sativum Distichum in contaminated soil. Plants 10 (5), 841. doi:10.3390/plants10050841
Campion, L., Bekchanova, M., Malina, R., and Kuppens, T. (2023). The costs and benefits of biochar production and use: a systematic review. J. Clean. Prod. 408, 137138. doi:10.1016/j.jclepro.2023.137138
Cara, I. G., Țopa, D., Puiu, I., and Jităreanu, G. (2022). Biochar a promising strategy for pesticide-contaminated soils. Agriculture 12 (10), 1579. doi:10.3390/agriculture12101579
Chai, W. S., Tan, W. G., Munawaroh, H. S. H., Gupta, V. K., Ho, S. H., and Show, P. L. (2021). Multifaceted roles of microalgae in the application of wastewater biotreatment: a review. Environ. Pollut. 269, 116236. doi:10.1016/j.envpol.2020.116236
Chen, K., Liang, J., Xu, X., Zhao, L., Qiu, H., Wang, X., et al. (2022). Roles of soil active constituents in the degradation of sulfamethoxazole by biochar/persulfate: contrasting effects of iron minerals and organic matter. Sci. Total Environ. 853, 158532. doi:10.1016/j.scitotenv.2022.158532
Chen, Q., Zhang, Z., Wang, Y., Mu, G., Wu, X., Liu, Y., et al. (2023). Remediation of soil contaminated by heavy metals using biochar: strategies and future prospects. Pol. J. Environ. Stud. 32 (1), 27–40. doi:10.15244/pjoes/153912
Chen, X., Lin, Q., Xiao, H., and Rizwan, M. (2022). Manganese-modified biochar promotes Cd accumulation in Sedum alfredii in an intercropping system. Environ. Pollut. 317, 120525. doi:10.1016/j.envpol.2022.120525
Choudhary, T. K., Khan, K. S., Hussain, Q., Ahmad, M., and Ashfaq, M. (2019). Feedstock-induced changes in composition and stability of biochar derived from different agricultural wastes. Arab. J. Geosci. 12, 617–713. doi:10.1007/s12517-019-4735-z
Clurman, A. M., Rodríguez-Narvaez, O. M., Jayarathne, A., De Silva, G., Ranasinghe, M. I., Goonetilleke, A., et al. (2020). Influence of surface hydrophobicity/hydrophilicity of biochar on the removal of emerging contaminants. Chem. Eng. J. 402, 126277. doi:10.1016/j.cej.2020.126277
Cui, J., Glatzel, S., Bruckman, V. J., Wang, B., and Lai, D. Y. (2021). Long-term effects of biochar application on greenhouse gas production and microbial community in temperate forest soils under increasing temperature. Sci. Total Environ. 767, 145021. doi:10.1016/j.scitotenv.2021.145021
Cui, L., Liu, Y., Yan, J., Hina, K., Hussain, Q., Qiu, T., et al. (2022). Revitalizing coastal saline-alkali soil with biochar application for improved crop growth. Ecol. Eng. 179, 106594. doi:10.1016/j.ecoleng.2022.106594
Da, T. X., Ren, H. K., He, W. K., Gong, S. Y., and Chen, T. (2022). Prediction of uranium adsorption capacity on biochar by machine learning methods. J. Environ. Chem. Eng. 10 (5), 108449. doi:10.1016/j.jece.2022.108449
Dai, C., Han, Y., Duan, Y., Lai, X., Fu, R., Liu, S., et al. (2022). Review on the contamination and remediation of polycyclic aromatic hydrocarbons (PAHs) in coastal soil and sediments. Environ. Res. 205, 112423. doi:10.1016/j.envres.2021.112423
Danesh, P., Niaparast, P., Ghorbannezhad, P., and Ali, I. (2022). Biochar production: recent developments, applications, and challenges. Fuel 337, 126889. doi:10.1016/j.fuel.2022.126889
Das, S. K., Ghosh, G. K., and Avasthe, R. (2020). Biochar application for environmental management and toxic pollutant remediation. Biomass Conv. bioref. 13, 555–566. doi:10.1007/s13399-020-01078-1
Da Silva Mendes, J., Fernandes, J. D., Chaves, L. H. G., Guerra, H. O. C., Tito, G. A., and de Brito Chaves, I. (2021). Chemical and physical changes of soil amended with biochar. Water Air, Soil Pollut. 232 (8), 338. doi:10.1007/s11270-021-05289-8
Delgado-Moreno, L., Bazhari, S., Gasco, G., Mendez, A., El Azzouzi, M., and Romero, E. (2021). New insights into the efficient removal of emerging contaminants by biochars and hydrochars derived from olive oil wastes. Sci. Total Environ. 752, 141838. doi:10.1016/j.scitotenv.2020.141838
Dhar, S. A., Sakib, T. U., and Hilary, L. N. (2022). Effects of pyrolysis temperature on production and physicochemical characterization of biochar derived from coconut fiber biomass through slow pyrolysis process. Biomass Conv. bioref. 12 (7), 2631–2647. doi:10.1007/s13399-020-01116-y
Dong, M., He, L., Jiang, M., Zhu, Y., Wang, J., Gustave, W., et al. (2023). Biochar for the removal of emerging pollutants from aquatic systems: a review. Int. J. Environ. Res. Public Health 20 (3), 1679. doi:10.3390/ijerph20031679
Du, C., Yang, S., Ding, D., Cai, T., and Chen, R. (2023). Origin of synergistic effect between Fe/Mn minerals and biochar for peroxymonosulfate activation. Chem. Eng. J. 453, 139899. doi:10.1016/j.cej.2022.139899
Duwiejuah, A. B., Abubakari, A. H., Quainoo, A. K., and Amadu, Y. (2020). Review of biochar properties and remediation of metal pollution of water and soil. J. Health Pollut. 10 (27), 200902. doi:10.5696/2156-9614-10.27.200902
Egamberdieva, D., Alaylar, B., Kistaubayeva, A., Wirth, S., and Bellingrath-Kimura, S. D. (2022). Biochar for improving soil biological properties and mitigating salt stress in plants on salt-affected soils. Commun. Soil Sci. Plant Anal. 53 (2), 140–152. doi:10.1080/00103624.2021.1993884
Elkhlifi, Z., Kamran, M., Maqbool, A., El-Naggar, A., Ifthikar, J., Parveen, A., et al. (2021). Phosphate-lanthanum coated sewage sludge biochar improved the soil properties and growth of ryegrass in an alkaline soil. Ecotoxicol. Environ. Saf. 216, 112173. doi:10.1016/j.ecoenv.2021.112173
El-Naggar, A., Ahmed, N., Mosa, A., Niazi, N. K., Yousaf, B., Sharma, A., et al. (2021). Nickel in soil and water: sources, biogeochemistry, and remediation using biochar. J. Hazard. Mater. 419, 126421. doi:10.1016/j.jhazmat.2021.126421
Enaime, G., Baçaoui, A., Yaacoubi, A., and Lübken, M. (2020). Biochar for wastewater treatment—conversion technologies and applications. Appl. Sci. 10 (10), 3492. doi:10.3390/app10103492
Fagnano, M., Visconti, D., and Fiorentino, N. (2020). Agronomic approaches for characterization, remediation, and monitoring of contaminated sites. Agronomy 10 (9), 1335. doi:10.3390/agronomy10091335
Fang, Q., Ye, S., Yang, H., Yang, K., Zhou, J., Gao, Y., et al. (2021). Application of layered double hydroxide-biochar composites in wastewater treatment: recent trends, modification strategies, and outlook. J. Hazard. Mat. 420, 126569. doi:10.1016/j.jhazmat.2021.126569
Farid, I. M., Siam, H. S., Abbas, M. H., Mohamed, I., Mahmoud, S. A., Tolba, M., et al. (2022). Co-composted biochar derived from rice straw and sugarcane bagasse improved soil properties, carbon balance, and zucchini growth in a sandy soil: a trial for enhancing the health of low fertile arid soils. Chemosphere 292, 133389. doi:10.1016/j.chemosphere.2021.133389
Fu, M. M., Mo, C. H., Li, H., Zhang, Y. N., Huang, W. X., and Wong, M. H. (2019). Comparison of physicochemical properties of biochars and hydrochars produced from food wastes. J. Clean. Prod. 236, 117637. doi:10.1016/j.jclepro.2019.117637
Gabhane, J. W., Bhange, V. P., Patil, P. D., Bankar, S. T., and Kumar, S. (2020). Recent trends in biochar production methods and its application as a soil health conditioner: a review. SN Appl. Sci. 2, 1307–1321. doi:10.1007/s42452-020-3121-5
Gao, N., Du, W., Zhang, M., Ling, G., and Zhang, P. (2022). Chitosan-modified biochar: preparation, modifications, mechanisms and applications. Int. J. Biol. Macromol. 209, 31–49. doi:10.1016/j.ijbiomac.2022.04.006
Gautam, R. K., Goswami, M., Mishra, R. K., Chaturvedi, P., Awashthi, M. K., Singh, R. S., et al. (2021). Biochar for remediation of agrochemicals and synthetic organic dyes from environmental samples: a review. Chemosphere 272, 129917. doi:10.1016/j.chemosphere.2021.129917
Ghodake, G. S., Shinde, S. K., Kadam, A. A., Saratale, R. G., Saratale, G. D., Kumar, M., et al. (2021). Review on biomass feedstocks, pyrolysis mechanism and physicochemical properties of biochar: state-of-the-art framework to speed up vision of circular bioeconomy. J. Clean. Prod. 297, 126645. doi:10.1016/j.jclepro.2021.126645
Ginebra, M., Muñoz, C., Calvelo-Pereira, R., Doussoulin, M., and Zagal, E. (2022). Biochar impacts on soil chemical properties, greenhouse gas emissions and forage productivity: a field experiment. Sci. Total Environ. 806, 150465. doi:10.1016/j.scitotenv.2021.150465
Gluckler, R., Herzschuh, U., Kruse, S., Andreev, A., Vyse, S. A., Winkler, B., et al. (2021). Wildfire history of the boreal forest of south-western Yakutia (Siberia) over the last two millennia documented by a lake-sediment charcoal record. Biogeosci 18 (13), 4185–4209. doi:10.5194/bg-18-4185-2021
Gonzalez-Hourcade, M., dos Reis, G. S., Grimm, A., Lima, E. C., Larsson, S. H., Gentili, F. G., et al. (2022). Microalgae biomass as a sustainable precursor to produce nitrogen-doped biochar for efficient removal of emerging pollutants from aqueous media. J. Clean. Prod. 348, 131280. doi:10.1016/j.jclepro.2022.131280
Gopal, M., Gupta, A., Shahul Hameed, K., Sathyaseelan, N., Khadeejath Rajeela, T. H., and Thomas, G. V. (2020). Biochars produced from coconut palm biomass residues can aid regenerative agriculture by improving soil properties and plant yield in humid tropics. Biochar 2, 211–226. doi:10.1007/s42773-020-00043-5
Gorovtsov, A. V., Minkina, T. M., Mandzhieva, S. S., Perelomov, L. V., Soja, G., Zamulina, I. V., et al. (2020). The mechanisms of biochar interactions with microorganisms in soil. Environ. Geochem. Health 42, 2495–2518. doi:10.1007/s10653-019-00412-5
Goswami, R. K., Mehariya, S., Karthikeyan, O. P., Gupta, V. K., and Verma, P. (2022). Multifaceted application of microalgal biomass integrated with carbon dioxide reduction and wastewater remediation: a flexible concept for sustainable environment. J. Clean. Prod. 339, 130654. doi:10.1016/j.jclepro.2022.130654
Gouma, V., Tziasiou, C., Pournara, A. D., and Giokas, D. L. (2022). A novel approach to sorbent-based remediation of soil impacted by organic micropollutants and heavy metals using granular biochar amendment and magnetic separation. J. Environ. Chem. Eng. 10 (2), 107316. doi:10.1016/j.jece.2022.107316
Greenough, S., Dumont, M. J., and Prasher, S. (2021). The physicochemical properties of biochar and its applicability as a filler in rubber composites: a review. Mat. Today Commun. 29, 102912. doi:10.1016/j.mtcomm.2021.102912
Grimm, A., dos Reis, G. S., Dinh, V. M., Larsson, S. H., Mikkola, J. P., Lima, E. C., et al. (2022). Hardwood spent mushroom substrate–based activated biochar as a sustainable bioresource for removal of emerging pollutants from wastewater. Biomass Conv. bioref., 1–17. doi:10.1007/s13399-022-02618-7
Gross, C. D., Bork, E. W., Carlyle, C. N., and Chang, S. X. (2022). Biochar and its manure-based feedstock have divergent effects on soil organic carbon and greenhouse gas emissions in croplands. Sci. Total Environ. 806, 151337. doi:10.1016/j.scitotenv.2021.151337
Haider, F. U., Wang, X., Farooq, M., Hussain, S., Cheema, S. A., Ul Ain, N., et al. (2022). Biochar application for the remediation of trace metals in contaminated soils: implications for stress tolerance and crop production. Ecotoxicol. Environ. Saf. 230, 113165. doi:10.1016/j.ecoenv.2022.113165
Hamidzadeh, Z., Ghorbannezhad, P., Ketabchi, M. R., and Yeganeh, B. (2023). Biomass-derived biochar and its application in agriculture. Fuel 341, 127701. doi:10.1016/j.fuel.2023.127701
Han, L., Sun, H., Sun, K., Yang, Y., Fang, L., and Xing, B. (2021). Effect of Fe and Al ions on the production of biochar from agricultural biomass: properties, stability and adsorption efficiency of biochar. Renew. Sustain. Energy Rev. 145, 111133. doi:10.1016/j.rser.2021.111133
Hao, Z., Wang, Q., Yan, Z., and Jiang, H. (2021). Novel magnetic loofah sponge biochar enhancing microbial responses for the remediation of polycyclic aromatic hydrocarbons-contaminated sediment. J. Hazard. Mat. 401, 123859. doi:10.1016/j.jhazmat.2020.123859
Hassan, M., Liu, Y., Naidu, R., Parikh, S. J., Du, J., Qi, F., et al. (2020). Influences of feedstock sources and pyrolysis temperature on the properties of biochar and functionality as adsorbents: a meta-analysis. Sci. Total Environ. 744, 140714. doi:10.1016/j.scitotenv.2020.140714
Hossain, M. Z., Bahar, M. M., Sarkar, B., Donne, S. W., Ok, Y. S., Palansooriya, K. N., et al. (2020). Biochar and its importance on nutrient dynamics in soil and plant. Biochar 2, 379–420. doi:10.1007/s42773-020-00065-z
Hua, B., Li, Z., Gao, W., Feng, H., Chen, N., Li, J., et al. (2021). Soil amendment in plastic greenhouse using modified biochar: soil bacterial diversity responses and microbial biomass carbon and nitrogen. Biotechnol. Lett. 43, 655–666. doi:10.1007/s10529-020-03046-1
Huang, H., Reddy, N. G., Huang, X., Chen, P., Wang, P., Zhang, Y., et al. (2021). Effects of pyrolysis temperature, feedstock type and compaction on water retention of biochar amended soil. Sci. Rep. 11 (1), 7419. doi:10.1038/s41598-021-86701-5
Hussain, R., Ravi, K., and Garg, A. (2020). Influence of biochar on the soil water retention characteristics (SWRC): potential application in geotechnical engineering structures. Soil till. Res. 204, 104713. doi:10.1016/j.still.2020.104713
Ippolito, J. A., Cui, L., Kammann, C., Wrage-Mönnig, N., Estavillo, J. M., Fuertes-Mendizabal, T., et al. (2020). Feedstock choice, pyrolysis temperature and type influence biochar characteristics: a comprehensive meta-data analysis review. Biochar 2, 421–438. doi:10.1007/s42773-020-00067-x
Islam, T., Li, Y., and Cheng, H. (2021). Biochars and engineered biochars for water and soil remediation: a review. Sustainability 13 (17), 9932. doi:10.3390/su13179932
Issaka, E., Fapohunda, F. O., Amu-Darko, J. N. O., Yeboah, L., Yakubu, S., Varjani, S., et al. (2022). Biochar-based composites for remediation of polluted wastewater and soil environments: challenges and prospects. Chemosphere 297, 134163. doi:10.1016/j.chemosphere.2022.134163
Janu, R., Mrlik, V., Ribitsch, D., Hofman, J., Sedláček, P., Bielská, L., et al. (2021). Biochar surface functional groups as affected by biomass feedstock, biochar composition and pyrolysis temperature. Carb. Resour. Conv. 4, 36–46. doi:10.1016/j.crcon.2021.01.003
Jeyasubramanian, K., Thangagiri, B., Sakthivel, A., Raja, J. D., Seenivasan, S., Vallinayagam, P., et al. (2021). A complete review on biochar: production, property, multifaceted applications, interaction mechanism and computational approach. Fuel 292, 120243. doi:10.1016/j.fuel.2021.120243
Ji, M., Wang, X., Usman, M., Liu, F., Dan, Y., Zhou, L., et al. (2022). Effects of different feedstocks-based biochar on soil remediation: a review. Environ. Pollut. 294, 118655. doi:10.1016/j.envpol.2021.118655
Jing, F., Guan, J., Tang, W., and Chen, J. (2022a). Mechanistic insight into adsorptive removal of ionic NOR and nonionic DEP organic contaminates by clay-biochar composites. Environ. Pollut. 310, 119881. doi:10.1016/j.envpol.2022.119881
Jing, F., Sun, Y., Liu, Y., Wan, Z., Chen, J., and Tsang, D. C. (2022b). Interactions between biochar and clay minerals in changing biochar carbon stability. Sci. Total Environ. 809, 151124. doi:10.1016/j.scitotenv.2021.151124
Kalina, M., Sovova, S., Svec, J., Trudicova, M., Hajzler, J., Kubikova, L., et al. (2022). The effect of pyrolysis temperature and the source biomass on the properties of biochar produced for the agronomical applications as the soil conditioner. Mater 15 (24), 8855. doi:10.3390/ma15248855
Kamali, M., Sweygers, N., Al-Salem, S., Appels, L., Aminabhavi, T. M., and Dewil, R. (2022). Biochar for soil applications-sustainability aspects, challenges and future prospects. Chem. Eng. J. 428, 131189. doi:10.1016/j.cej.2021.131189
Kamarudin, N. S., Dahalan, F. A., Hasan, M., An, O. S., Parmin, N. A., Ibrahim, N., et al. (2022). Biochar: a review of its history, characteristics, factors that influence its yield, methods of production, application in wastewater treatment and recent development. Bio interface Res. Appl. Chem. 12, 7914–7926. doi:10.33263/BRIAC126.79147926
Kang, K., Nanda, S., and Hu, Y. (2022). Current trends in biochar application for catalytic conversion of biomass to biofuels. Catal. Today 404, 3–18. doi:10.1016/j.cattod.2022.06.033
Kayiranga, A., Li, Z., Isabwe, A., Ke, X., Simbi, C. H., Ifon, B. E., et al. (2023). The effects of heavy metal pollution on collembola in urban soils and associated recovery using biochar remediation: a review. Int. J. Environ. Res. Public Health 20 (4), 3077. doi:10.3390/ijerph20043077
Keerthanan, S., Gunawardane, C., Somasundaram, T., Jayampathi, T., Jayasinghe, C., and Vithanage, M. (2021). Immobilization and retention of caffeine in soil amended with Ulva reticulata biochar. J. Environ. Manag. 281, 111852. doi:10.1016/j.jenvman.2020.111852
Khan, Z., Xianting, F., Khan, M. N., Khan, M. A., Zhang, K., Fu, Y., et al. (2022). The toxicity of heavy metals and plant signaling facilitated by biochar application: implications for stress mitigation and crop production. Chemosphere 308, 136466. doi:10.1016/j.chemosphere.2022.136466
Kocsis, T., Ringer, M., and Biró, B. (2022). Characteristics and applications of biochar in soil–plant systems: a short review of benefits and potential drawbacks. Appl. Sci. 12 (8), 4051. doi:10.3390/app12084051
Kou, L., Chen, H., Zhang, X., Liu, S., Zhang, B., Zhu, H., et al. (2023). Enhanced degradation of phthalate esters (PAEs) by biochar-sodium alginate immobilized Rhodococcus sp. KLW-1. Environ. Technol. (just-accepted), 1–35. doi:10.1080/09593330.2023.2215456
Krahn, K. M., Cornelissen, G., Castro, G., Arp, H. P. H., Asimakopoulos, A. G., Wolf, R., et al. (2023). Sewage sludge biochars as effective PFAS-sorbents. J. Hazard. Mat. 445, 130449. doi:10.1016/j.jhazmat.2022.130449
Kumar, A., Bhattacharya, T., Shaikh, W. A., Roy, A., Chakraborty, S., Vithanage, M., et al. (2023). Multifaceted applications of biochar in environmental management: a bibliometric profile. Biochar 5 (1), 11. doi:10.1007/s42773-023-00207-z
Kumari, K., Kumar, R., Bordoloi, N., Minkina, T., Keswani, C., and Bauddh, K. (2023). Unravelling the recent developments in the production technology and efficient applications of biochar for agro-ecosystems. Agriculture 13 (3), 512. doi:10.3390/agriculture13030512
Kwon, G., Bhatnagar, A., Wang, H., Kwon, E. E., and Song, H. (2020). A review of recent advancements in utilization of biomass and industrial wastes into engineered biochar. J. Hazard. Mat. 400, 123242. doi:10.1016/j.jhazmat.2020.123242
Lade, C. B. M. (2023). Stabilization of PFAS contaminated soil with waste-based biochar sorbents. Master's thesis. Norwegian University of Life Sciences.
Lee, J. M., Park, D. G., Kang, S. S., Choi, E. J., Gwon, H. S., Lee, H. S., et al. (2022). Short-term effect of biochar on soil organic carbon improvement and nitrous oxide emission reduction according to different soil characteristics in agricultural land: a laboratory experiment. Agronomy 12 (8), 1879. doi:10.3390/agronomy12081879
Lehmann, J., Rillig, J., Thies, C. A., Masiello, W. C., Hockaday, A., and Crowley, D. (2011). Biochar effects on soil biota—a review. Soil Biol. Biochem. 43 (2011), 1812–1836. doi:10.1016/j.soilbio.2011.04.022
Levesque, V., Rochette, P., Hogue, R., Jeanne, T., Ziadi, N., Chantigny, M. H., et al. (2020). Greenhouse gas emissions and soil bacterial community as affected by biochar amendments after periodic mineral fertilizer applications. Biol. Fertil. Soils 56, 907–925. doi:10.1007/s00374-020-01470-z
Li, A., Ge, W., Liu, L., and Qiu, G. (2022). Preparation, adsorption performance and mechanism of MgO-loaded biochar in wastewater treatment: a review. Environ. Res. 212, 113341. doi:10.1016/j.envres.2022.113341
Liang, M., Lu, L., He, H., Li, J., Zhu, Z., and Zhu, Y. (2021). Applications of biochar and modified biochar in heavy metal contaminated soil: a descriptive review. Sustainability 13 (24), 14041. doi:10.3390/su132414041
Lima, D. R., Lima, E. C., Thue, P. S., Dias, S. L., Machado, F. M., Seliem, M. K., et al. (2021). Comparison of acidic leaching using a conventional and ultrasound-assisted method for preparation of magnetic-activated biochar. J. Environ. Chem. Eng. 9 (5), 105865. doi:10.1016/j.jece.2021.105865
Lopes, R. P., and Astruc, D. (2021). Biochar as a support for nanocatalysts and other reagents: recent advances and applications. Coord. Chem. Rev. 426, 213585. doi:10.1016/j.ccr.2020.213585
Lu, Y., Cheng, J., Wang, J., Zhang, F., Tian, Y., Liu, C., et al. (2022). Efficient remediation of cadmium contamination in soil by functionalized biochar: recent advances, challenges, and future prospects. Processes 10 (8), 1627. doi:10.3390/pr10081627
Lu, Y., Gu, K., Shen, Z., Tang, C. S., Shi, B., and Zhou, Q. (2023). Biochar implications for the engineering properties of soils: a review. Sci. Total Environ. 888, 164185. doi:10.1016/j.scitotenv.2023.164185
Mai, N. T., Nguyen, N. H., Tsubota, T., Shinogi, Y., Dultz, S., and Nguyen, M. N. (2019). Fern Dicranopteris linearis-derived biochars: adjusting surface properties by direct processing of the silica phase. Colloids Surfaces A Physicochem. Eng. Aspects 583, 123937. doi:10.1016/j.colsurfa.2019.123937
Mandal, S., Pu, S., Adhikari, S., Ma, H., Kim, D. H., Bai, Y., et al. (2021). Progress and future prospects in biochar composites: application and reflection in the soil environment. Crit. Rev. Environ. Sci. Technol. 51 (3), 219–271. doi:10.1080/10643389.2020.1713030
Manirakiza, E., Ziadi, N., Luce, M. S., Hamel, C., Antoun, H., and Karam, A. (2019). Nitrogen mineralization and microbial biomass carbon and nitrogen in response to co-application of biochar and paper mill biosolids. Appl. Soil Ecol. 142, 90–98. doi:10.1016/j.apsoil.2019.04.025
Mazarji, M., Bayero, M. T., Minkina, T., Sushkova, S., Mandzhieva, S., Bauer, T. V., et al. (2023). Nanomaterials in biochar: review of their effectiveness in remediating heavy metal-contaminated soils. Sci. Total Environ. 880, 163330. doi:10.1016/j.scitotenv.2023.163330
Mazarji, M., Bayero, M. T., Minkina, T., Sushkova, S., Mandzhieva, S., Tereshchenko, A., et al. (2021). Realizing United Nations sustainable development goals for greener remediation of heavy metals-contaminated soils by biochar: emerging trends and future directions. Sustainability 13 (24), 13825. doi:10.3390/su132413825
Mazarji, M., Minkina, T., Sushkova, S., Mandzhieva, S., Bayero, M. T., Fedorenko, A., et al. (2022). Metal-organic frameworks (MIL-101) decorated biochar as a highly efficient bio-based composite for immobilization of polycyclic aromatic hydrocarbons and copper in real contaminated soil. J. Environ. Chem. Eng. 10 (6), 108821. doi:10.1016/j.jece.2022.108821
Medha, I., Chandra, S., Vanapalli, K. R., Samal, B., Bhattacharya, J., and Das, B. K. (2021). (3-Aminopropyl) triethoxysilane and iron rice straw biochar composites for the sorption of Cr (VI) and Zn (II) using the extract of heavy metals contaminated soil. Sci. Total Environ. 771, 144764. doi:10.1016/j.scitotenv.2020.144764
Muhammad, N., Nafees, M., Khan, M. H., Ge, L., and Lisak, G. (2020). Effect of biochars on bioaccumulation and human health risks of potentially toxic elements in wheat (Triticum aestivum L.) cultivated on industrially contaminated soil. Environ. Pollut. 260, 113887. doi:10.1016/j.envpol.2019.113887
Muigai, H. H., Bordoloi, U., Hussain, R., Ravi, K., Moholkar, V. S., and Kalita, P. (2021). A comparative study on synthesis and characterization of biochars derived from lignocellulosic biomass for their candidacy in agronomy and energy applications. Int. J. Energy Res. 45 (3), 4765–4781. doi:10.1002/er.6092
Mukherjee, A., Patra, B. R., Podder, J., and Dalai, A. K. (2022). Synthesis of biochar from lignocellulosic biomass for diverse industrial applications and energy harvesting: effects of pyrolysis conditions on the physicochemical properties of biochar. Front. Mat. 9, 870184. doi:10.3389/fmats.2022.870184
Mulabagal, V., Baah, D. A., Egiebor, N. O., Sajjadi, B., Chen, W. Y., Viticoski, R. L., et al. (2022). “Biochar from biomass: a strategy for carbon dioxide sequestration, soil amendment, power generation, CO2 utilization, and removal of perfluoroalkyl and polyfluoroalkyl substances (PFAS) in the environment,” in Handbook of climate change mitigation and adaptation (Cham: Springer International Publishing), 1023–1085.
Murtaza, G., Ahmed, Z., Dai, D. Q., Iqbal, R., Bawazeer, S., Usman, M., et al. (2022). A review of mechanism and adsorption capacities of biochar-based engineered composites for removing aquatic pollutants from contaminated water. Front. Environ. Sci. 10, 2155. doi:10.3389/fenvs.2022.1035865
Murtaza, G., Ahmed, Z., Eldin, S. M., Ali, I., Usman, M., Iqbal, R., et al. (2023). Biochar as a green sorbent for remediation of polluted soils and associated toxicity risks: a critical review. Separations 10 (3), 197. doi:10.3390/separations10030197
Murtaza, G., Ahmed, Z., Usman, M., Tariq, W., Ullah, Z., Shareef, M., et al. (2021a). Biochar induced modifications in soil properties and its impacts on crop growth and production. J. Plant Nutr. 44 (11), 1–15. doi:10.1080/01904167.2021.1871746
Murtaza, G., Ditta, A., Ullah, N., Usman, M., and Ahmed, Z. (2021b). Biochar for the management of nutrient impoverished and metal contaminated soils: preparation, applications, and prospects. J. Soil Sci. Plant Nut. 21, 2191–2213. doi:10.1007/s42729-021-00514-z
Natasha, N., Shahid, M., Khalid, S., Bibi, I., Naeem, M. A., Niazi, N. K., et al. (2022). Influence of biochar on trace element uptake, toxicity and detoxification in plants and associated health risks: a critical review. Crit. Rev. Environ. Sci. Technol. 52 (16), 2803–2843. doi:10.1080/10643389.2021.1894064
Nguyen, T. M., Chen, H. H., Chang, Y. C., Ning, T. C., and Chen, K. F. (2023). Remediation of groundwater contaminated with trichloroethylene (TCE) using a long-lasting persulfate/biochar barrier. Chemosphere 333, 138954. doi:10.1016/j.chemosphere.2023.138954
Nzediegwu, C., Naeth, M. A., and Chang, S. X. (2021). Elemental composition of biochars is affected by methods used for its determination. J. Anal. Appl. Pyrolysis 156, 105174. doi:10.1016/j.jaap.2021.105174
Nzediegwu, C., Naeth, M. A., and Chang, S. X. (2022). Feedstock type drives surface property, demineralization and element leaching of nitric acid-activated biochars more than pyrolysis temperature. Bioresour. Technol. 344, 126316. doi:10.1016/j.biortech.2021.126316
Osman, A. I., Fawzy, S., Farghali, M., El-Azazy, M., Elgarahy, A. M., Fahim, R. A., et al. (2022). Biochar for agronomy, animal farming, anaerobic digestion, composting, water treatment, soil remediation, construction, energy storage, and carbon sequestration: a review. Environ. Chem. Lett. 20 (4), 2385–2485. doi:10.1007/s10311-022-01424-x
Pan, H., Yang, X., Chen, H., Sarkar, B., Bolan, N., Shaheen, S. M., et al. (2021). Pristine and iron-engineered animal-and plant-derived biochars enhanced bacterial abundance and immobilized arsenic and lead in a contaminated soil. Sci. Total Environ. 763, 144218. doi:10.1016/j.scitotenv.2020.144218
Panahi, H. K. S., Dehhaghi, M., Ok, Y. S., Nizami, A. S., Khoshnevisan, B., Mussatto, S. I., et al. (2020). A comprehensive review of engineered biochar: production, characteristics, and environmental applications. J. Clean. Prod. 270, 122462. doi:10.1016/j.jclepro.2020.122462
Pathy, A., Ray, J., and Paramasivan, B. (2020). Biochar amendments and its impact on soil biota for sustainable agriculture. Biochar 2 (3), 287–305. doi:10.1007/s42773-020-00063-1
Patwa, D., Chandra, A., Ravi, K., and Sreedeep, S. (2021). Influence of biochar particle size fractions on thermal and mechanical properties of biochar-amended soil. J. Mat. Civ. Eng. 33 (9), 04021236. doi:10.1061/(asce)mt.1943-5533.0003915
Patwardhan, S. B., Pandit, S., Gupta, P. K., Jha, N. K., Rawat, J., Joshi, H. C., et al. (2022). Recent advances in the application of biochar in microbial electrochemical cells. Fuel 311, 122501. doi:10.1016/j.fuel.2021.122501
Petersen, H. I., Lassen, L., Rudra, A., Nguyen, L. X., Do, P. T. M., and Sanei, H. (2023). Carbon stability and morphotype composition of biochars from feedstocks in the Mekong Delta, Vietnam. Int. J. Coal Geol. 271, 104233. doi:10.1016/j.coal.2023.104233
Pidlisnyuk, V., Herts, A., Khomenchuk, V., Mamirova, A., Kononchuk, O., and Ust’ak, S. (2021). Dynamic of morphological and physiological parameters and variation of soil characteristics during miscanthus × giganteus cultivation in the diesel-contaminated land. Agronomy 11 (4), 798. doi:10.3390/agronomy11040798
Qi, X., Yin, H., Zhu, M., Yu, X., Shao, P., and Dang, Z. (2022). MgO-loaded nitrogen and phosphorus self-doped biochar: high-efficient adsorption of aquatic Cu2+, Cd2+, and Pb2+ and its remediation efficiency on heavy metal contaminated soil. Chemosphere 294, 133733. doi:10.1016/j.chemosphere.2022.133733
Qian, S., Zhou, X., Fu, Y., Song, B., Yan, H., Chen, Z., et al. (2023). Biochar-compost as a new option for soil improvement: application in various problem soils. Sci. Total Environ. 870, 162024. doi:10.1016/j.scitotenv.2023.162024
Qianqian, M., Haider, F. U., Farooq, M., Adeel, M., Shakoor, N., Jun, W., et al. (2022). Selenium treated Foliage and biochar treated soil for improved lettuce (Lactuca sativa L.) growth in Cd-polluted soil. J. Clean. Prod. 335, 130267. doi:10.1016/j.jclepro.2021.130267
Qiu, M., Liu, L., Ling, Q., Cai, Y., Yu, S., Wang, S., et al. (2022). Biochar for the removal of contaminants from soil and water: a review. Biochar 4 (1), 19. doi:10.1007/s42773-022-00146-1
Ramadan, M. M., and Abd-Elsalam, K. A. (2020). Micro/Nano biochar for sustainable plant health: present status and future prospects. Carbon Nanomater. Agri-Food Environ. Appl. 2020, 323–357. doi:10.1016/b978-0-12-819786-8.00016-5
Ramanayaka, S., Tsang, D. C., Hou, D., Ok, Y. S., and Vithanage, M. (2020). Green synthesis of graphitic nanobiochar for the removal of emerging contaminants in aqueous media. Sci. Total Environ. 706, 135725. doi:10.1016/j.scitotenv.2019.135725
Ramezanzadeh, H., Zarehaghi, D., Baybordi, A., Bouket, A. C., Oszako, T., Alenezi, F. N., et al. (2023). The impacts of biochar-assisted factors on the hydrophysical characteristics of amended soils: a review. Sustainability 15 (11), 8700. doi:10.3390/su15118700
Rashid, M., Hussain, Q., Khan, K. S., Al-Wabel, M. I., Afeng, Z., Akmal, M., et al. (2020). Prospects of biochar in alkaline soils to mitigate climate change. Environ. Clim. Plant Veg. Growth, 133–149. doi:10.1007/978-3-030-49732-3_7
Rashid, M. S., Liu, G., Yousaf, B., Hamid, Y., Rehman, A., Arif, M., et al. (2022). A critical review on biochar-assisted free radicals mediated redox reactions influencing transformation of potentially toxic metals: occurrence, formation, and environmental applications. Environ. Pollut. 315, 120335. doi:10.1016/j.envpol.2022.120335
Razzaq, S., Zhou, B., Zia-ur-Rehman, M., Aamer Maqsood, M., Hussain, S., Bakhsh, G., et al. (2022). Cadmium stabilization and redox transformation mechanism in maize using nanoscale zerovalent-iron-enriched biochar in cadmium-contaminated soil. Plants 11 (8), 1074. doi:10.3390/plants11081074
Rex, P., Mohammed Ismail, K. R., Meenakshisundaram, N., Barmavatu, P., and Sai Bharadwaj, A. V. S. L. (2023). Agricultural biomass waste to biochar: a review on biochar applications using machine learning approach and circular economy. Chem. Eng. 7 (3), 50. doi:10.3390/chemengineering7030050
Reyhanitabar, A., Frahadi, E., Ramezanzadeh, H., and Oustan, S. (2020). Effect of pyrolysis temperature and feedstock sources on physicochemical characteristics of biochar. J. Agric. Sci. Technol. 22 (2), 547–561.
Rizwan, M., Lin, Q., Chen, X., Adeel, M., Li, G., and Zhao, X. (2020b). Comparison of Pb2+ adsorption and desorption by several chemically modified biochars derived from steam exploded oil-rape straw. Appl. Eco. Environ. Res. 18 (5), 6181–6197. doi:10.15666/aeer/1805_61816197
Rizwan, M., Lin, Q., Chen, X., Li, Y., Li, G., Zhao, X., et al. (2020a). Synthesis, characterization and application of magnetic and acid modified biochars following alkaline pretreatment of rice and cotton straws. Sci. Total Environ. 714, 136532. doi:10.1016/j.scitotenv.2020.136532
Rizwan, M., Nawaz, A., Irshad, S., and Manoharadas, S. (2024). Exogenously applied melatonin enhanced chromium tolerance in pepper by up-regulating the photosynthetic apparatus and antioxidant machinery. Sci. Hortic. 323, 112468. doi:10.1016/j.scienta.2023.112468
Rodriguez, J. A., Lustosa Filho, J. F., Melo, L. C. A., de Assis, I. R., and de Oliveira, T. S. (2021). Co-pyrolysis of agricultural and industrial wastes changes the composition and stability of biochars and can improve their agricultural and environmental benefits. J. Anal. Appl. Pyrol. 155, 105036. doi:10.1016/j.jaap.2021.105036
Rombola, A. G., Torri, C., Vassura, I., Venturini, E., Reggiani, R., and Fabbri, D. (2022). Effect of biochar amendment on organic matter and dissolved organic matter composition of agricultural soils from a two-year field experiment. Sci. Total Environ. 812, 151422. doi:10.1016/j.scitotenv.2021.151422
Romero, C. M., Chunli, L. I., Owens, J., Ribeiro, G. O., Mcallister, T. A., Okine, E., et al. (2021). Nutrient cycling and greenhouse gas emissions from soil amended with biochar-manure mixtures. Pedosphere 31 (2), 289–302. doi:10.1016/s1002-0160(20)60071-6
Saleem, A., Zulfiqar, A., Arshed, M. Z., Hussain, S., Khan, M. T., Zivcak, M., et al. (2022). The impact of newly synthesized sulfonamides on soil microbial population and respiration in rhizospheric soil of wheat (Triticum aestivum L.). PLOS ONE 17 (4), e0264476. doi:10.1371/journal.pone.0264476
Samoraj, M., Mironiuk, M., Witek-Krowiak, A., Izydorczyk, G., Skrzypczak, D., Mikula, K., et al. (2022). Biochar in environmental friendly fertilizers-Prospects of development products and technologies. Chemosphere 296, 133975. doi:10.1016/j.chemosphere.2022.133975
Sanchez-Hernandez, J. C. (2021). “Vermiremediation of pharmaceutical-contaminated soils and organic amendments,” in Interaction and fate of pharmaceuticals in soil-crop systems: the impact of reclaimed wastewater, 339–375.
Sarauer, J. L., Page-Dumroese, D. S., and Coleman, M. D. (2019). Soil greenhouse gas, carbon content, and tree growth response to biochar amendment in western United States forests. GCB Bioenerg. 11 (5), 660–671. doi:10.1111/gcbb.12595
Scaria, J., Gopinath, A., Ranjith, N., Ravindran, V., Ummar, S., Nidheesh, P. V., et al. (2022). Carbonaceous materials as effective adsorbents and catalysts for the removal of emerging contaminants from water. J. Clean. Prod. 350, 131319. doi:10.1016/j.jclepro.2022.131319
Schmidt, H. P., Kammann, C., Hagemann, N., Leifeld, J., Bucheli, T. D., Sánchez Monedero, M. A., et al. (2021). Biochar in agriculture–A systematic review of 26 global meta-analyses. GCB Bioenerg. 13 (11), 1708–1730. doi:10.1111/gcbb.12889
Seow, Y. X., Tan, Y. H., Mubarak, N. M., Kansedo, J., Khalid, M., Ibrahim, M. L., et al. (2022). A review on biochar production from different biomass wastes by recent carbonization technologies and its sustainable applications. J. Environ. Chem. Eng. 10 (1), 107017. doi:10.1016/j.jece.2021.107017
Shafiq, F., Anwar, S., Zhang, L., and Ashraf, M. (2023). Nano-biochar: properties and prospects for sustainable agriculture. Land Degrad. Dev. 34 (9), 2445–2463. doi:10.1002/ldr.4620
Shaheen, S. M., Antoniadis, V., Shahid, M., Yang, Y., Abdelrahman, H., Zhang, T., et al. (2022). Sustainable applications of rice feedstock in agro-environmental and construction sectors: a global perspective. Renew. Sustain. Energy Rev. 153, 111791. doi:10.1016/j.rser.2021.111791
Shan, R., Han, J., Gu, J., Yuan, H., Luo, B., and Chen, Y. (2020). A review of recent developments in catalytic applications of biochar-based materials. Resour. Conserv. Recycl. 162, 105036. doi:10.1016/j.resconrec.2020.105036
Shikha, F. S., Rahman, M. M., Sultana, N., Mottalib, M. A., and Yasmin, M. (2023). Effects of biochar and biofertilizer on groundnut production: a perspective for environmental sustainability in Bangladesh. Carbon Res. 2 (1), 10. doi:10.1007/s44246-023-00043-7
Sieradzka, M., Kirczuk, C., Kalemba-Rec, I., Mlonka-Mędrala, A., and Magdziarz, A. (2022). Pyrolysis of biomass wastes into carbon materials. Energies 15 (5), 1941. doi:10.3390/en15051941
Sima, X. F., and Jiang, H. (2020). “Remediation of contaminated soil by biochar,” in A handbook of environmental toxicology: human disorders and ecotoxicology (Wallingford UK: CABI), 545–558.
Singh, H., Northup, B. K., Rice, C. W., and Prasad, P. V. (2022). Biochar applications influence soil physical and chemical properties, microbial diversity, and crop productivity: a meta-analysis. Biochar 4 (1), 8. doi:10.1007/s42773-022-00138-1
Singh, S., Kumar, V., Dhanjal, D. S., Datta, S., Bhatia, D., Dhiman, J., et al. (2020). A sustainable paradigm of sewage sludge biochar: valorization, opportunities, challenges and future prospects. J. Clean. Prod. 269, 122259. doi:10.1016/j.jclepro.2020.122259
Song, J., Wang, Y., Zhang, S., Song, Y., Xue, S., Liu, L., et al. (2021). Coupling biochar with anaerobic digestion in a circular economy perspective: a promising way to promote sustainable energy, environment and agriculture development in China. Renew. Sustain. Energy Rev. 144, 110973. doi:10.1016/j.rser.2021.110973
Sormo, E., Castro, G., Hubert, M., Licul-Kucera, V., Quintanilla, M., Asimakopoulos, A. G., et al. (2023). The decomposition and emission factors of a wide range of PFAS in diverse, contaminated organic waste fractions undergoing dry pyrolysis. J. Hazard. Mat. 454, 131447. doi:10.1016/j.jhazmat.2023.131447
Sormo, E., Silvani, L., Bjerkli, N., Hagemann, N., Zimmerman, A. R., Hale, S. E., et al. (2021). Stabilization of PFAS-contaminated soil with activated biochar. Sci. Total Environ. 763, 144034. doi:10.1016/j.scitotenv.2020.144034
Sri Shalini, S., Palanivelu, K., Ramachandran, A., and Raghavan, V. (2021). Biochar from biomass waste as a renewable carbon material for climate change mitigation in reducing greenhouse gas emissions—a review. Biomass Convers. Biorefinery 11, 2247–2267. doi:10.1007/s13399-020-00604-5
Stylianou, M., Christou, A., Dalias, P., Polycarpou, P., Michael, C., Agapiou, A., et al. (2020). Physicochemical and structural characterization of biochar derived from the pyrolysis of biosolids, cattle manure and spent coffee grounds. J. Energy Inst. 93 (5), 2063–2073. doi:10.1016/j.joei.2020.05.002
Sun, Y., Wang, T., Bai, L., Han, C., and Sun, X. (2022). Application of biochar-based materials for remediation of arsenic contaminated soil and water: preparation, modification, and mechanisms. J. Environ. Chem. Eng. 10, 108292. doi:10.1016/j.jece.2022.108292
Sun, Y., Xiong, X., He, M., Xu, Z., Hou, D., Zhang, W., et al. (2021). Roles of biochar-derived dissolved organic matter in soil amendment and environmental remediation: a critical review. Chem. Eng. J. 424, 130387. doi:10.1016/j.cej.2021.130387
Tang, Y., Li, Y., Zhan, L., Wu, D., Zhang, S., Pang, R., et al. (2022). Removal of emerging contaminants (bisphenol A and antibiotics) from kitchen wastewater by alkali-modified biochar. Sci. Total Environ. 805, 150158. doi:10.1016/j.scitotenv.2021.150158
Tauqeer, H. M., Fatima, M., Rashid, A., Shahbaz, A. K., Ramzani, P. M. A., Farhad, M., et al. (2021). The current scenario and prospects of immobilization remediation technique for the management of heavy metals contaminated soils. Approaches Remediat. Inorg. Pollut., 155–185. doi:10.1007/978-981-15-6221-1_8
Teodoro, M., Trakal, L., Gallagher, B. N., Šimek, P., Soudek, P., Pohořelý, M., et al. (2020). Application of co-composted biochar significantly improved plant-growth relevant physical/chemical properties of a metal contaminated soil. Chemosphere 242, 125255. doi:10.1016/j.chemosphere.2019.125255
Tian, W., Wu, H., Wu, L., Liu, C., Yue, C., and Zhou, J. (2022). Enhanced microwave remediation of organic contaminated soil: a new way of utilization of bluecoke powder. Int. J. Environ. Sci. Technol. 19 (6), 5451–5460. doi:10.1007/s13762-021-03439-3
Valentina, P., Volodymyr, K., Aigerim, M., Oleksandr, K., and Sergey, U. A. (2021). Dynamic of morphological and physiological parameters and variation of soil characteristics during miscanthus x giganteus cultivation in the diesel-contaminated land.
Vanapalli, K. R., Samal, B., Dubey, B. K., and Bhattacharya, J. (2021). “Biochar for sustainable agriculture: prospects and implications,” in Advances in chemical pollution, environmental management and protection (Elsevier), 7, 221–262.
Van Nguyen, T. T., Phan, A. N., Nguyen, T. A., Nguyen, T. K., Nguyen, S. T., Pugazhendhi, A., et al. (2022). Valorization of agriculture waste biomass as biochar: as first-rate biosorbent for remediation of contaminated soil. Chemosphere 307, 135834. doi:10.1016/j.chemosphere.2022.135834
Varjani, S., Kumar, G., and Rene, E. R. (2019). Developments in biochar application for pesticide remediation: current knowledge and future research directions. J. Environ. Manage. 232, 505–513. doi:10.1016/j.jenvman.2018.11.043
Velusamy, K., Periyasamy, S., Kumar, P. S., Jayaraj, T., Krishnasamy, R., Sindhu, J., et al. (2021). Analysis on the removal of emerging contaminant from aqueous solution using biochar derived from soap nut seeds. Environ. Pollut. 287, 117632. doi:10.1016/j.envpol.2021.117632
Venkatesh, G., Gopinath, K. A., Reddy, K. S., Reddy, B. S., Prabhakar, M., Srinivasarao, C., et al. (2022). Characterization of biochar derived from crop residues for soil amendment, carbon sequestration and energy use. Sustainability 14 (4), 2295. doi:10.3390/su14042295
Viggi, C. C., Tucci, M., Resitano, M., Matturro, B., Crognale, S., Feigl, V., et al. (2022). Passive electro bioremediation approaches for enhancing hydrocarbons biodegradation in contaminated soils. Sci. Total Environ. 845, 157325. doi:10.1016/j.scitotenv.2022.157325
Vijayaraghavan, K. (2019). Recent advancements in biochar preparation, feedstocks, modification, characterization and future applications. Environ. Technol. Rev. 8 (1), 47–64. doi:10.1080/21622515.2019.1631393
Vu, K. A., and Mulligan, C. N. (2023). Remediation of organic contaminated soil by Fe-based nanoparticles and surfactants: a review. Environ. Technol. Rev. 12 (1), 60–82. doi:10.1080/21622515.2023.2177200
Wang, H., Xing, L., Zhang, H., Gui, C., Jin, S., Lin, H., et al. (2021). Key factors to enhance soil remediation by bio electrochemical systems (BESs): a review. Chem. Eng. J. 419, 129600. doi:10.1016/j.cej.2021.129600
Wang, L., Rinklebe, J., Tack, F. M., and Hou, D. (2021). A review of green remediation strategies for heavy metal contaminated soil. Soil Use Manage 37 (4), 936–963. doi:10.1111/sum.12717
Wang, Z., Han, Y., Peng, Q., Jiang, C., and Wang, H. (2023). The intermolecular interactions of methanol diluted in protic and aprotic solvent probed by polarized Raman spectroscopy and HNMR. J. Mol. Liq. 387, 122658. doi:10.1016/j.molliq.2023.122658
Wani, I., Kushvaha, V., Garg, A., Kumar, R., Naik, S., and Sharma, P. (2022). Review on effect of biochar on soil strength: towards exploring usage of biochar in geo-engineering infrastructure. Biomass Conv. bioref., 1–32. doi:10.1007/s13399-022-02795-5
Wani, I., Sharma, A., Kushvaha, V., Madhushri, P., and Peng, L. (2020). Effect of pH, volatile content, and pyrolysis conditions on surface area and O/C and H/C ratios of biochar: towards understanding performance of biochar using simplified approach. J. Hazard. Toxic. Radioact. Waste 24 (4), 04020048. doi:10.1061/(asce)hz.2153-5515.0000545
Wei, Z., Wei, Y., Liu, Y., Niu, S., Xu, Y., Park, J. H., et al. (2023). Biochar-based materials as remediation strategy in petroleum hydrocarbon-contaminated soil and water: Performances, mechanisms, and environmental impact. J. Environ. Sci. 138, 350–372. doi:10.1016/j.jes.2023.04.008
Winchell, L. J., Ross, J. J., Wells, M. J., Fonoll, X., Norton, J. W., and Bell, K. Y. (2021). Per-and polyfluoroalkyl substances thermal destruction at water resource recovery facilities: a state of the science review. Water Environ. Res. 93 (6), 826–843. doi:10.1002/wer.1483
Wu, P., Singh, B. P., Wang, H., Jia, Z., Wang, Y., and Chen, W. (2023). Bibliometric analysis of biochar research in 2021: a critical review for development, hotspots and trend directions. Biochar 5 (1), 6. doi:10.1007/s42773-023-00204-2
Xiang, L., Liu, S., Ye, S., Yang, H., Song, B., Qin, F., et al. (2021). Potential hazards of biochar: the negative environmental impacts of biochar applications. J. Hazard. Mat. 420, 126611. doi:10.1016/j.jhazmat.2021.126611
Xiao, B., Jia, J., Wang, W., Zhang, B., Ming, H., Ma, S., et al. (2023). A Review on magnetic biochar for the removal of heavy metals from contaminated soils: preparation, application, and microbial response. J. Hazard. Mat. Adv. 100254, 100254. doi:10.1016/j.hazadv.2023.100254
Xu, D. M., Fu, R. B., Wang, J. X., Shi, Y. X., and Guo, X. P. (2021). Chemical stabilization remediation for heavy metals in contaminated soils on the latest decade: available stabilizing materials and associated evaluation methods–A critical review. J. Clean. Prod. 321, 128730. doi:10.1016/j.jclepro.2021.128730
Xu, X., Wu, Y., Wu, X., Sun, Y., Huang, Z., Li, H., et al. (2022). Effect of physicochemical properties of biochar from different feedstock on remediation of heavy metal contaminated soil in mining area. Surfaces Interfaces 32, 102058. doi:10.1016/j.surfin.2022.102058
Yaashikaa, P. R., Kumar, P. S., Varjani, S., and Saravanan, A. (2020). A critical review on the biochar production techniques, characterization, stability and applications for circular bioeconomy. Biotechnol. Rep. 28, e00570. doi:10.1016/j.btre.2020.e00570
Yadav, S. K., and Bag, R. (2023). Effect of Bamboo biochar on strength and water retention properties of low plastic clay and silty sand. Sci. Rep. 13 (1), 6201. doi:10.1038/s41598-023-33466-8
Yang, Q., Wang, Y., and Zhong, H. (2021). Remediation of mercury-contaminated soils and sediments using biochar: a critical review. Biochar 3, 23–35. doi:10.1007/s42773-021-00087-1
Yaro, N. S. A., Sutanto, M. H., Habib, N. Z., Usman, A., Kaura, J. M., Murana, A. A., et al. (2023). A comprehensive review of biochar utilization for low-carbon flexible asphalt pavements. Sustainability 15 (8), 6729. doi:10.3390/su15086729
Yi, Y., Kou, F., Tsang, P. E., and Fang, Z. (2021). Highly efficient remediation of decabromodiphenyl ether-contaminated soil using mechanochemistry in the presence of additive and its mechanism. J. Environ. Manage. 299, 113595. doi:10.1016/j.jenvman.2021.113595
Yin, D., Li, H., Wang, H., Guo, X., Wang, Z., Lv, Y., et al. (2021). Impact of different biochars on microbial community structure in the rhizospheric soil of rice grown in albic soil. Molecules 26 (16), 4783. doi:10.3390/molecules26164783
Zahed, M. A., Salehi, S., Madadi, R., and Hejabi, F. (2021). Biochar as a sustainable product for remediation of petroleum contaminated soil. Curr. Res. Green Sustain. Chem. 4, 100055. doi:10.1016/j.crgsc.2021.100055
Zeghioud, H., Fryda, L., Djelal, H., Assadi, A., and Kane, A. (2022). A comprehensive review of biochar in removal of organic pollutants from wastewater: characterization, toxicity, activation/functionalization and influencing treatment factors. J. Water Process Eng. 47, 102801. doi:10.1016/j.jwpe.2022.102801
Zeng, Y., Li, T., Ding, Y., Fang, G., Wang, X., Ye, B., et al. (2022). Biochar-supported nano-scale zerovalent iron activated persulfate for remediation of aromatic hydrocarbon-contaminated soil: an in-situ pilot-scale study. Biochar 4 (1), 64. doi:10.1007/s42773-022-00188-5
Zhang, X., Wells, M., Niazi, N. K., Bolan, N., Shaheen, S., Hou, D., et al. (2022). Nanobiochar-rhizosphere interactions: implications for the remediation of heavy-metal contaminated soils. Environ. Pollut. 299, 118810. doi:10.1016/j.envpol.2022.118810
Zhang, Y., Zhang, H., Zhang, A., Héroux, P., Sun, Z., and Liu, Y. (2023). Remediation of atrazine-polluted soil using dielectric barrier discharge plasma and biochar sequential batch experimental technology. Chem. Eng. J. 458, 141406. doi:10.1016/j.cej.2023.141406
Zhao, H., Wang, L., Bai, Y., Li, Y., Tang, T., Liang, H., et al. (2023). Immobilized enzyme with sustainable chestnut biochar to remediate polycyclic aromatic hydrocarbons contaminated soils. Environ. Technol., 1–11. doi:10.1080/09593330.2022.2164221
Zhen, Z., Luo, S., Chen, Y., Li, G., Li, H., Wei, T., et al. (2023). Performance and mechanisms of biochar-assisted vermicomposting in accelerating di-(2-ethylhexyl) phthalate biodegradation in farmland soil. J. Hazard. Mater. 443, 130330. doi:10.1016/j.jhazmat.2022.130330
Zheng, X., Xu, W., Dong, J., Yang, T., Shangguan, Z., Qu, J., et al. (2022). The effects of biochar and its applications in the microbial remediation of contaminated soil: a review. J. Hazard. Mater. 438, 129557. doi:10.1016/j.jhazmat.2022.129557
Zhou, Y., Qin, S., Verma, S., Sar, T., Sarsaiya, S., Ravindran, B., et al. (2021). Production and beneficial impact of biochar for environmental application: a comprehensive review. Bioresour. Technol. 337, 125451. doi:10.1016/j.biortech.2021.125451
Zhu, X., Sun, W., Li, C., Wang, Z., Cui, X., Li, B., et al. (2023). Generation, activation, and recycling of ammonium persulfate for the remediation of organic-contaminated soil: system construction, process, and mechanisms. Chem. Eng. J. 471, 144482. doi:10.1016/j.cej.2023.144482
Keywords: feedstock, bio-economy, biochar, emission, remediation, pollutants, pyrolysis
Citation: Rizwan M, Murtaza G, Zulfiqar F, Moosa A, Iqbal R, Ahmed Z, Irshad S, Khan I, Li T, Chen J, Zhang M, Siddique KHM, Leng L and Li H (2023) Sustainable manufacture and application of biochar to improve soil properties and remediate soil contaminated with organic impurities: a systematic review. Front. Environ. Sci. 11:1277240. doi: 10.3389/fenvs.2023.1277240
Received: 14 August 2023; Accepted: 24 October 2023;
Published: 20 November 2023.
Edited by:
Rubén Forján, University of Oviedo, SpainReviewed by:
Leonard Lim, University of Malaysia Sarawak, MalaysiaCopyright © 2023 Rizwan, Murtaza, Zulfiqar, Moosa, Iqbal, Ahmed, Irshad, Khan, Li, Chen, Zhang, Siddique, Leng and Li. This is an open-access article distributed under the terms of the Creative Commons Attribution License (CC BY). The use, distribution or reproduction in other forums is permitted, provided the original author(s) and the copyright owner(s) are credited and that the original publication in this journal is cited, in accordance with accepted academic practice. No use, distribution or reproduction is permitted which does not comply with these terms.
*Correspondence: Faisal Zulfiqar, Y2guZmFpc2FsLnp1bGZpcWFyQGdtYWlsLmNvbQ==; Kadambot H. M. Siddique, a2FkYW1ib3Quc2lkZGlxdWVAdXdhLmVkdS5hdQ==; Lijian Leng, bC5sZW5nMjAxOUBjc3UuZWR1LmNu, bGxqY2hzQDEyNi5jb20=; Hailong Li, aGFpbG9uZ2xpQGNzdS5lZHUuY24=, aGFpbG9uZ2xpMThAZ21haWwuY29t
Disclaimer: All claims expressed in this article are solely those of the authors and do not necessarily represent those of their affiliated organizations, or those of the publisher, the editors and the reviewers. Any product that may be evaluated in this article or claim that may be made by its manufacturer is not guaranteed or endorsed by the publisher.
Research integrity at Frontiers
Learn more about the work of our research integrity team to safeguard the quality of each article we publish.