- 1Environmental Horticulture Department, University of Florida, Gainesville, FL, United States
- 2Plant Molecular and Cellular Biology Graduate Program, University of Florida, Gainesville, FL, United States
Secondary metabolites such as antioxidants are critical components that protect seeds from stress damage during seed development, desiccation, and ex-situ storage. Antioxidants are essential determinants of seed quality, longevity, and persistence. Understanding the environmental factors that regulate the accumulation, content, and function of antioxidant pools in sea oat seeds is critical for gene banking and understanding the environmental impacts on seed quality. Germination, viability, and Trolox Equivalent Antioxidant Capacity (TEAC) were analyzed on seeds from 18 sea oat populations from the US Atlantic and Gulf of Mexico coasts. We first assessed baseline TEAC, followed by TEAC performed on imbibed seeds for 36 h at 35/25°C during the day and night, respectively. Then, we analyzed the relationship of the antioxidant pools from these 18 sea oat populations to sampling site environmental classifications. Higher baseline antioxidants were common in regions with extreme environmental conditions corresponding to sea oat populations growing at latitudinal extremes characterized by warmer and colder temperatures. Baseline antioxidants did not correlate with prevailing seed germination. However, higher concentrations of antioxidants following imbibition were associated with poor seed germination in warmer conditions. Our results indicate that climatic conditions and environmental components associated with temperatures and precipitation may largely influence the innate pool of antioxidants in sea oat seeds. Also, a high amount of antioxidants following sea oat seed imbibition suggest seed damage or poor viability influenced by environmental stress conditions during seed development.
Introduction
Uniola paniculata L. (sea oats [Poaceae]) is an ecological keystone species occurring in coastal dune systems throughout the Yucatan peninsula, Cuba, the Bahamas, and southeastern US coastlines (Subudhi et al., 2005; Lonard et al., 2011; Hodel and Gonzales, 2013). Sea oats are essential for their ecological services, such as sand accretion, preventing erosion, and forming and stabilizing coastal dunes. These services largely buffer the effect of extreme events such as storms and hurricanes prevalent along those coastlines (Lonard et al., 2011; Hacker et al., 2019). Consequently, sea oats are in high demand for habitat restoration and re-vegetation programs. However, the spatial extent of sea oats populations has diminished greatly due to habitat destruction, conversion, and fragmentation. Protecting remaining sea oats populations is therefore crucial for maintaining donor populations for seed-based conservation and restoration programs.
Sea oats face diverse extreme conditions in the coastal dune habitat including osmotic stress from aerosolized salts and saline soils, hypoxia due to flooding, high and low-temperature stress, high humidity stress, and physical damage from tidal and wave over-wash or winds caused by storms and hurricanes. Sea oats also face burial from shifting dune sands. Therefore, sea oats and their seeds need highly functional stress-relief systems, especially antioxidants, to survive such conditions. However, there is limited knowledge on the presence, environmental influence, and functional role of antioxidants in plants from coastal dune systems exposed to such wide-ranging stressors.
Antioxidants are a category of secondary metabolites synthesized by living organisms that have been associated with preventing oxidative damage to cells and tissues. These molecules function at relatively low concentrations during optimal and stress conditions (Halliwell et al., 1995; Begcy et al., 2012) directly impacting complex aspects of seed quality including nutritional components, seed size, water potential and dry matter content (Bailly, 2004, 2019; Corbineau, 2012). In seeds, antioxidants are synthesized throughout the developmental program (i.e., histodifferentiation, reserve accumulation, and late maturation) and are thought to protect against stresses by quenching reactive oxygen species (ROS) and free radicals (Bailly, 2004; Chen et al., 2016; Adetunji et al., 2021). For instance, antioxidants balance ROS and free radicals generated in hydration-associated active metabolism from the initiation of embryogenesis through germination by acting as free radical scavengers (Pehlivan, 2017). Seed-based antioxidants also influence the hydration process through the action of seed coat phenolics, which may offer a barrier to rapid imbibition (Ross et al., 2010). They also regulate cellular redox balance preventing oxidative damage during the imbibition process (Bailly, 2004). Antioxidants also substantially buffer the damage from ROS and other radicles generated by desiccation stress and lipid oxidation during seed storage (Groot et al., 2015; Carta et al., 2018; Adetunji et al., 2021; Stegner et al., 2022), Furthermore, antioxidants may be linked to maintaining seed persistence and viability in the soil seed bank (Long et al., 2015).
At an ecological level, plants adapt to prevailing environmental conditions such as precipitation, relative humidity, solar radiation, and temperatures, allowing normal plant development and reproductive success (Saatkamp et al., 2019). Such adaptions result in the development of fitness-related traits vital to surviving harsh conditions such as drought and cold stress (Capblancq et al., 2022; Eckert and Neale, 2022). Studies have indicated a strong correlation between environmental conditions and plant traits. For example, the influence of geographical location on seed germination has been observed in sea oats (Pérez and Kane, 2017). At the same time, environmental conditions during plant growth, flowering, and seed development has been found to influence subsequent seed germination and seedling response to oxidative stress (Nguyen et al., 2021).
Seed traits including seed dormancy and vigor as well as resilience to environmental stresses have been suggested to vary broadly across a latitudinal gradient (Baskin and Baskin, 2014; Pérez and Kane, 2017; Moreira et al., 2020; Zhou et al., 2021). Using sea oat populations distributed across the US Atlantic and Gulf Coastlines, we investigated the functional dynamics of antioxidant pools in sea oats seeds collected from a continental-scale spatial distribution. First, we measured their antioxidant levels hypothesizing possible interactive links of the environmental conditions to the antioxidant levels and seed quality. We compared antioxidant levels from each population to 10-year climate data (precipitation, and temperature) and various ecological classification systems of the study sites. We further tested the potential role of the antioxidant pool on seed quality by correlating antioxidant levels to germination ability.
Materials and methods
Plant material collection and seed lot processing
We collected one to three sea oats (Uniola paniculata L.) panicles from no less than 30 widely spaced plants across 18 sites along the US Atlantic and Gulf of Mexico (referred to hereafter as Gulf) coasts in October 2019 (Figure 1A, Table 1). We spread panicles in a single layer on tarps within a non-climate-controlled warehouse and circulated air over the panicles with an electric fan. We allowed panicles to dry for 3 days, then hand-stripped spikelets and threshed caryopses (referred to hereafter as seeds) from spikelets with a laboratory debearder (Heavy Duty Batch Debearder, DB3001-600, Mater Seed Equipment, Corvallis, OR). We conditioned seeds further by passing all lots through an air-screen cleaner (Clipper Office Tester, A.T. Ferrell Co. Inc., Corvallis, OR) then an air-column separator (Oregon Seed Blower, Hoffman Manufacturing Inc., Corvallis, OR). We stored remaining seeds in the lab for two and half years (∼23°C, 30%–50% RH).
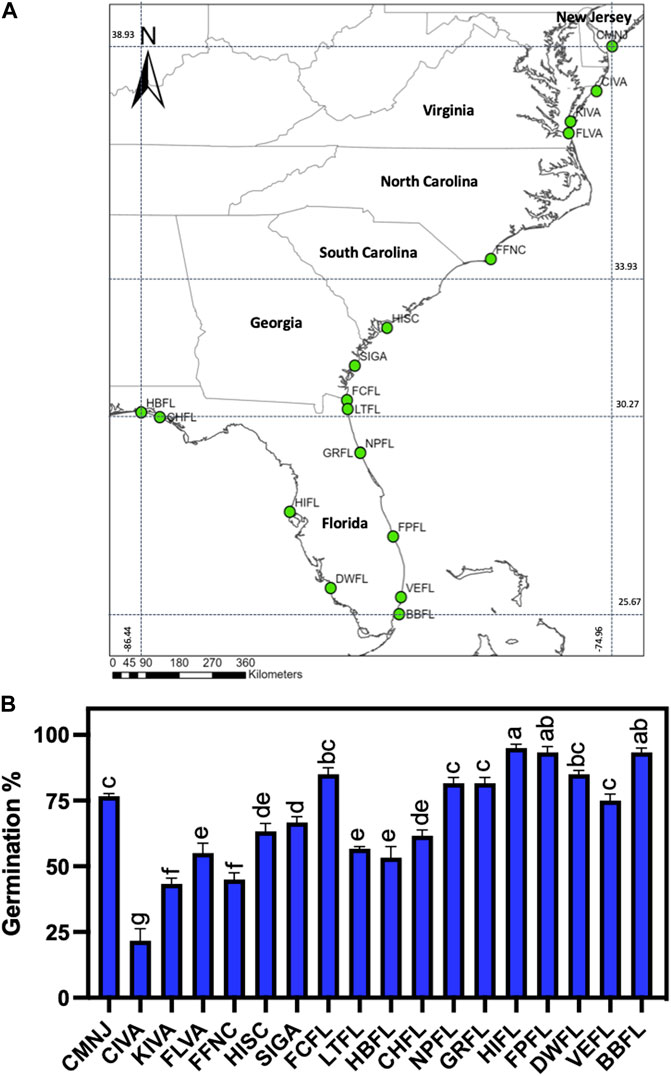
FIGURE 1. Sea oats seed collection sites and germination from 18 populations harvested in the US Atlantic and Gulf Coastlines. (A) Continental-scale map showing the collection sites representing different sea oat populations. CMNJ, Cape May Plant Materials Center; CIVA, Chincoteague National Wildlife Refuge; KIVA, Kiptopeke State Park; FLVA, First Landing State Park; FFNC, Fort Fisher State Recreation Area; HISC, Hunting Island State Park; SIGA, Sapelo Island National Estuarine Research Reserve; FCFL, Fort Clinch State Park; LTFL, Little Talbot Island State Park; NPFL, North Peninsula State Park; GRFL, Gamble Rogers State Park; FPFL, Fort Pierce Inlet State Park; VEFL, Dr. Von D. Mizell-Eula Johnson State Park; BBFL, Bill Baggs State Park; HBFL, Henderson Beach State Park; CHFL, Camp Helen State Park; HIFL, Honeymoon Island State Park and DWFL, Delnor-Wiggins State Park. North Peninsula State Park and Gamble Rogers State Park are close together and appear as a single population on the map. (B) Sea oat seed germination. Error bars indicate the standard error of the mean. Seed germination test was performed using 25 seeds in four independent replicates from a random sample of 200 seeds obtained from the seed stock of more than 500 seeds. Seed germination was based on the percentage of germinated seeds sown determined by the exposure of 2 mm of radicle and coleoptile emergence at 28 days. Collection sites are displayed left to right based on their latitudinal location.
Seed imbibition and germination assays
We randomly selected 200 seeds from each population for imbibition and germination assays. We surface sterilized tested seeds by placing them in 250 mL glass conical flasks with sufficient ethanol (50% v:v) to cover all the seeds (ca., 40 mL). The flasks were transferred to a benchtop shaker and agitated at 200 rpm for 10 min. This was followed by a double rinse (i.e., 3 min each) with 100 mL of sterilized distilled H2O followed by adding 40 mL of 25% sodium hypochlorite and incubation on a benchtop shaker at 200 rpm for 5 min, then another double rinse with 100 mL of sterilized, distilled H2O (i.e., 3 min each) before using them in imbibition and germination assays (Begcy et al., 2018).
We performed seed imbibition experiments by placing fifteen seeds on blotter paper (Steel Blue, Anchor Paper Co., St. Paul, MN) moistened with 30–50 mL distilled H2O containing 0.2% solution of a broad-spectrum biocide (Plant Preservative Mixture, Plant Cell Technologies, Washington, D.C.) contained within polystyrene germination boxes (156C, Hoffman Manufacturing, Corvallis, OR). We then transferred boxes to an incubator (I30-VL, Percival Scientific, Perry IA) set to 35/25°C (day/night) and a 12 h photoperiod. Illumination coincided with the day temperature. We allowed seeds to imbibe under these conditions for 36 h, after which we froze the seeds in liquid nitrogen and stored them at −80°C for use in the determination of Trolox Equivalent Antioxidant Capacity (TEAC) as described below.
We used three to four samples of 25 seeds in our germination experiments that were conducted under the same conditions described for the imbibition experiments. However, germination assays continued for 28 days. We inspected the germination boxes daily and counted germination when seeds exhibited 2 mm of radicle and coleoptile emergence (Begcy et al., 2018). We removed germinated seeds and any seeds showing signs of fungal contamination. We assessed the viability of any remaining, non-germinated seeds after day 28 using tetrazolium stain and analyzed embryo staining patterns at magnifications of 2.5–6.4× (Supplementary Figures S1A, B) (Peters and Lanham, 2000).
Seed water relations
We measured post-storage seed water potential, using five technical replicates, with a dewpoint potentiometer (WP4C, Decagon Devices, Pullman, WA). The potentiometer was calibrated before each measurement day according to manufacturer recommendations. We measured seed water potential at 20°C in precise mode. In this procedure, we used about 200 seeds to cover the bottom of the cuvette completely. Seed moisture content was calculated by recording the fresh mass on 20 seeds in five replicates for each of the 18 populations studied. We then dried these seeds in a forced-air oven (Blue M, Illinois, United States) at 103°C for 17 h and report water content on a dry mass basis (g H2O · g dry seed mass−1 [g g −1]).
Climate and ecological data
We obtained climate data for the 10 years before our seed sampling (2009–2019) using the PRISM application (Hart and Bell, 2015). The climate data used in this study included minimum (Tmin), mean (Tmean) and maximum (Tmax) temperatures, mean dew point temperature (Tdmean), total precipitation (ppt), and daily maximum vapor pressure deficit (Vpdmax). All data obtained were filtered at 4 km resolution using the location coordinates for sites corresponding to each of the 18 sea oat populations (Figure 1A, Supplementary Table S1). Data were extracted using the prism R package (Hart and Bell, 2015).
First, we explored seasonal precipitation and temperature patterns along the latitudinal gradient where the sea oats populations were collected. We organized our analysis into groups of 3 months, roughly representing the four seasons (Winter, Spring, Summer, and Autumn).
Ecological descriptors comprised the: U.S. Environmental Protection Agency (US EPA) ecoregion zoning (EPA3 and EPA4) (Supplementary Table S2), U.S. Department of Agriculture-Natural Resource Conservation Service (USDA-NRCs) and Food and Agriculture Organization of the United Nations (FAO) soil classification (Supplementary Table S3), American Horticulture Society (AHS) heat zoning (Supplementary Table S4), USDA plant hardiness zoning (Supplementary Table S5). We also used sea oat phylo-geography data as population grouping factors (Franks et al., 2004, Supplementary Table S6).
Antioxidant analysis
We estimated the levels of Trolox Equivalent Antioxidant Capacity (TEAC) in seeds using the modified ABTS/TEAC assay (Re et al., 1999). ABTS/TEAC determines the TEAC per gram of sample. ABTS (2,2′-Azino-bis (3-ethylbenzothiazoline-6-sulfonic acid) reagent was prepared by dissolving 2.74 mg of ABTS in 1 mL of sterile, distilled H2O for each reaction, including the standards. Then, 300 mg of MnO2 was added and mixed using a magnetic stirrer for 20 min. The mixture was filter sterilized through a 0.45 μm syringe filter. For calculations, a spectrophotometer (Scientific Genesys 10S UV-VIS Spectrophotometer, Thermo Scientific, Madison, United States) was zeroed at 734 nm with 100 μL PBS added to 1 mL of sterile distilled H2O. Absorbance readings were adjusted with 5 mM PBS to obtain a value of 0.700. We added 6.26 mg of Trolox (6-hydroxy-2,5,7,8-tetramethylchroman-2-carboxylic acid) to 50 mL of 5 mM PBS then mixed the reagents by sonication for 20 min to obtain 0.5 mM Trolox standard solution. Then, 1 mL of aliquots were prepared in cryovials and stored in a freezer at −20°C. During the assay, a 1 mL cryovial containing 0.5 mM Trolox was obtained from the freezer and allowed to thaw. We re-dissolved the solution by sonication for 10 min. Then, dilutions for five triplicate standards were obtained at 0, 11, 22, 33, and 44 μL of 5 mM Trolox corresponding to TEAC concentrations of 0, 5, 10, 15, and 20 μmol/L, respectively.
Antioxidants for TEAC estimation were extracted from seeds using a modified hydrophilic method (Re et al., 1999). Samples were finely ground using liquid nitrogen. Approximately 10 mg/sample was placed into 10 mm × 75 mm reaction vials. Triplicates were used per sample per reaction. TEAC was extracted by adding 5 mL of 75% aqueous methanol, followed by stirring under a nitrogen gas stream of 2.0 Pascals at 30°C for 60 min. Then, the obtained product was centrifugated at 2000 × g for 5 min to get a supernatant containing the extracts. A second extraction step was conducted by adding 2 mL of 75% aqueous methanol and covering the reaction vials. Then, each vial was vortexed and centrifugated at 2000 × g for 15 min. Supernatants of the first and second reactions were combined. Concurrently, 10–20 mg of finely ground samples were prepared in oven-drying foil pans and dried at 103°C for 17 h to obtain dry mass.
Extracts were re-suspended into 10 mL of 75% methanol. Each 100 μL of sample extract was mixed with 1 mL of 5 mM ABTS solution, vortexed for 2 min, and placed in the spectrophotometer cuvette before reading the absorbance at 734 nm. Three biological replicates and three technical replicates were used.
Data analysis
We subjected data on seed water potential, moisture content, TEAC, and germination percentage to an analysis of variance (ANOVA) to estimate differences among populations. We utilized linear and non-linear polynomial regressions to evaluate seed germination and TEAC levels against geographical, climatic, and ecological patterns. When linear regression analysis was used, we estimated the coefficient of determination (R2) using the Pearson correlation coefficients (r). For linear regression using ordinal data, we performed a non-parametric test for the significance of the correlation using the Spearman correlation to estimate the Spearman’s rho (rs) and the associated approximate p-value to test for the presence of monotonic relationships as previously described (Kvalseth, 1985). For non-linear regressions, the model selection was based on Akaike information criterion (AIC) involving the comparison of the best non-linear model to an alternative linear model. We tested the selected non-linear model by comparing to a “null model” for estimating the statistical significance using the “rcompanion” package in R software version 4.2.3 following the methods described previously (Mangiafico, 2015). We represent the overall p-values and the pseudo R2 (Nagelkerke R-squared) values (Mangiafico, 2015) for these non-linear models. Statistical analyses were performed using the R software/environment versions 4.1.1 and 4.2.3 (R Core Team, 2021). Differences were considered statistically significant at p-value < 0.05 (Kim et al., 2021).
Results
Impact of the latitudinal gradient on seed physiology in sea oat populations along the US Atlantic and Gulf coastlines
To test the hypothesis of seed related traits variation across a wide latitudinal gradient (Baskin and Baskin, 2014; Pérez and Kane, 2017; Moreira et al., 2020; Zhou et al., 2021), we collected and germinated eighteen sea oat populations distributed across the US Atlantic and Gulf Coastlines (Figure 1A). Seed germination ranged from 20% (CIVA) to 95% (HIFL) (Figure 1B). Seeds from 39% of the populations registered germination >80%, most of the populations displaying this level of germination were from Florida (Figure 1B). However, there were instances of poor germination (i.e., <50%) for other populations, especially from the northern latitudes of the Atlantic coastline (Figure 1B). Interestingly, seeds collected from populations in the southern US displayed germination percentages > 55% (Figure 1B).
To further understand differences in seed physiology that may influence the variation of germination, we quantified seed moisture content and water potential (Figures 2A, B). Seed moisture content was relatively similar across the latitudinal gradient and ranged from 0.1064 to 0.1129 g g−1. Seeds with lower moisture content (<0.1100 g g−1) comprised KIVA, FFNC, SIGA, GRFL, NPFL, and HBFL (Figure 2A). While seeds collected from LTFL and CIVA displayed the highest water contents observed of 0.1129 and 0.1130 g g−1, respectively. The seeds with the lowest moisture content were collected from Florida. They included GRFL and NPFL at 0.1066 and 0.1064 g g−1, respectively (Figure 2A). Sea oats seed water potential was also variable and ranged from −89.01 to −100.84 MPa (Figure 2B). Patterns of water potential did not necessarily track with patterns of water content as some populations displaying lower water content expressed higher water potential values. Conversely, other populations displaying higher water contents displayed lower water potentials (Figures 1B, 2A, B), and we did not find correlations between these two seed water relation traits with the seed germination traits (Supplementary Figure S2). Therefore, seed water relations could not explain germination variation across populations.
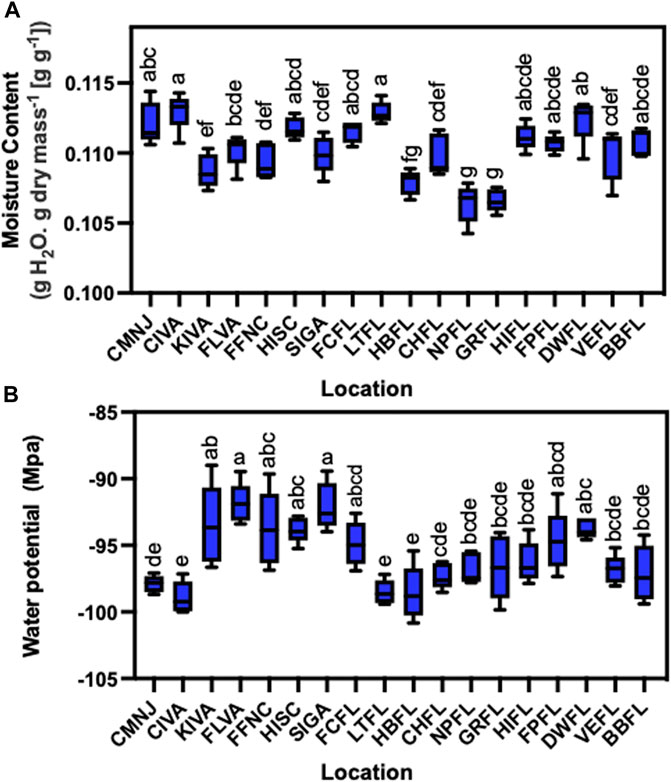
FIGURE 2. Limited variation on moisture content and water potential of sea oats seeds collected across the US Atlantic and Gulf Coastlines. Box plots showing (A) moisture content (g H2O g−1). The seed fresh mass was measured on 20 seeds in five independent replicates for each of the 18 populations studied and then dried in a forced-air oven (Blue M, Illinois, United States) at 103°C for 17 h and measured afterward to obtain the seed dry mass (n = 5). (B) Water potential (Mpa). Seed moisture content was calculated by obtaining the difference between fresh and dry mass and expressing the value on seed dry mass basis. Seed water potential was estimated from 200 randomly selected seeds from the seed stock covering the bottom of the cuvette completely and measured in precise mode using a dewpoint potentiometer (WP4C, Decagon Devices, Pullman, WA) in five independent replicates (n = 5). A different letter indicates significant differences based on one way analysis of variance (ANOVA) at an alpha of 0.05.
To characterize the extent to which location and environmental conditions influenced the germination of sea oats seeds, we examined how germination related to latitude and temperature zoning (Figure 3; Supplementary Tables S4, S5). We observed a latitudinal pattern of germination response, with regions closer to the equator having higher germination while populations located further from the equator displayed reduced germination capacity (R2 = 0.49), indicating a significant latitudinal influence on germination ability (Figure 3A). In addition, germination was lower in zones with fewer heat days above 30°C but increased as the number of heat days increased (Figure 3B). Similarly, germination was lowest in zones with lower average minimum winter temperatures but increased as this average rose (Figure 3C).
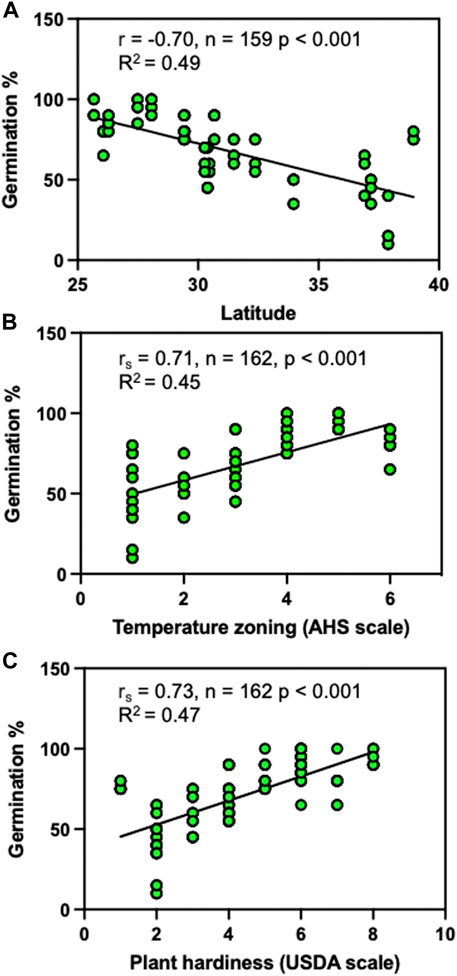
FIGURE 3. Influence of location and environmental zoning by temperature factors on the germination of sea oat seeds. (A) Latitude, (B) AHS temperature zoning, and (C) USDA plant hardiness zoning. The AHS temperature zoning is based on days at temperatures above 86°C, covering 45–60 days (1), 60–90 days (2), 90–120 days (3), 120–150 days (4), 150–180 days (5) and 180–210 days (6) per year. USDA plant hardiness scale is based on vegetation exposure to cold conditions in winter, at levels of 1(0–10F), 2(10–15F), 3(15–20F), 4(20–25F), 5(25–30F), 6(30–35F), 7(35–40F) and 8 (40–45F). For linear regressions, we estimated the coefficient of determination (R2) using the Pearson correlation coefficients (r) for continuous data (A) and performed a non-parametric test for the significance of the correlation using the Spearman correlation to estimate the Spearman’s rho (rs) and the associated approximate p-value to test for the presence of monotonic relationships for the analysis involving ordinal data (B,C).
Weather characteristics of sea oat seeds collection sites along the US Atlantic and Gulf coastlines
To explain which factors could have contributed to the observed differences in seed traits across all sea oats populations (Figures 1–3), we first looked at precipitation and seasonal mean temperature patterns for sea oats collection sites along the US Atlantic and Gulf coastlines in a 10-year period prior to seed sampling (2009–2019). Precipitation patterns across the latitudinal gradient were similar in winter and spring, averaging 50–150 mm (Figure 4A). However, in summer, southerly latitudes displayed higher precipitation (>150 mm) than northerly latitudes (<150 mm). In Autumn, the southerly latitudes maintained higher precipitation in September and October (>100 mm) before dropping below 100 mm in November. Interestingly, precipitation was much higher in southern than northern latitudes in September, while higher precipitation in the north was observed in October > ca.100 mm (Figure 4A).
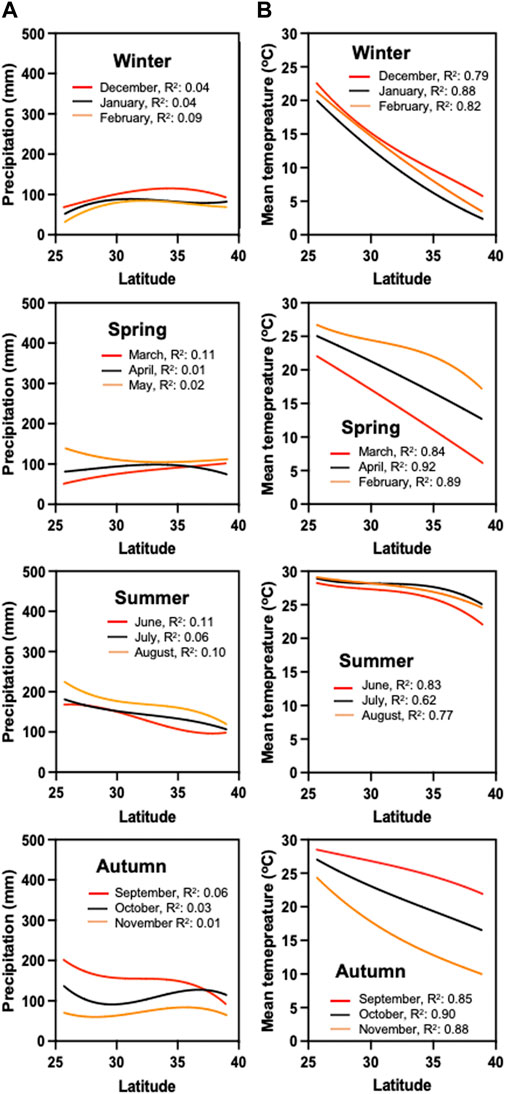
FIGURE 4. Environmental data patterns for the sites of sea oats collection from the US Atlantic and Gulf coastlines in a 10-year period prior to seed sampling (2009–2019). (A) Precipitation and (B) seasonal mean temperature. Model for precipitation and temperature patterns follow the function Y = B0 + B1 + B2x2 + B3x3. The coefficient of determination (R2) indicates the fitness of non-linear regression.
Temperature patterns were similar across all seasons (Figure 4B). However, higher temperatures were evident in southern latitudes compared to the northern ones during the Winter, Spring, and Autumn (Figure 4B). Summer temperatures varied less across all the latitudes in which the sea oats populations were analyzed (Figure 4B). Mean temperatures across all latitudes remained above 22°C throughout June, July, and August (Figure 4B). Both the maximum vapor pressure deficit and the mean dew point had a similar pattern, with higher values in the southern latitudes and lower values in the northern latitudes in most seasons (Winter, Spring, and Autumn), except in summer season in which there were no differences between southern and north latitudes (Supplementary Figures S3, S4).
Latitude-dependent environmental conditions influence TEAC concentration in sea oats seeds
Since our climate data pointed to precipitation and temperature as main drivers of germination differences across a latitudinal gradient except in Autumn (Supplementary Tables S7–S10), we used antioxidant levels in sea oats seeds as a proxy to explore a possible link of latitude-dependent environmental conditions with antioxidant accumulation and germination. First, we defined baseline TEAC values obtained from mature ungerminated seeds before imbibition across all sea oats populations. We found that TEAC levels from our collected seeds ranged from 0 to 12 μmol/g DW (Figure 5A). For instance, while seeds collected in CMNJ, CIVA, KIVA, VEFL, BBFL, HIFL DWFL and CHFL showed TEAC levels higher than 4 μmol/g, the remaining populations showed lower values than that (Figure 5A). Interestingly, in general, seeds from sea oat populations collected either from the most northern or southern locations showed higher antioxidant capacity than the ones collected in intermediate latitudes (Figure 5A). These results suggest an influence by extreme conditions experienced at northern or southern US latitudes. To further explore whether antioxidant capacity was maintained during the initiation of germination, we imbibed seeds for 36 h and quantified their TEAC levels (Figure 5B). Contrary to the earlier patterns of baseline antioxidant levels, there was wider population variability across the geographical distribution of sea oats (Figures 5A, B). The change in TEAC levels between dry and imbibed seeds showed considerable variation (Figure 5C). For instance, seeds from some latitudes displayed a drastic drop in TEAC levels, especially CMNJ and CIVA (Figure 5C), and those with significantly minimal changes comprised KIVA, FPFL, BBFL, DWFL, HBFL, and CHFL (Figure 5C). However, some seeds registered a significantly positive change in TEAC > 5 μmol/g DW comprised of FLVA, FFNC, HISC, SIGA, FCFL, LTFL, GRFL, NPFL and VEFL (Figure 5C).
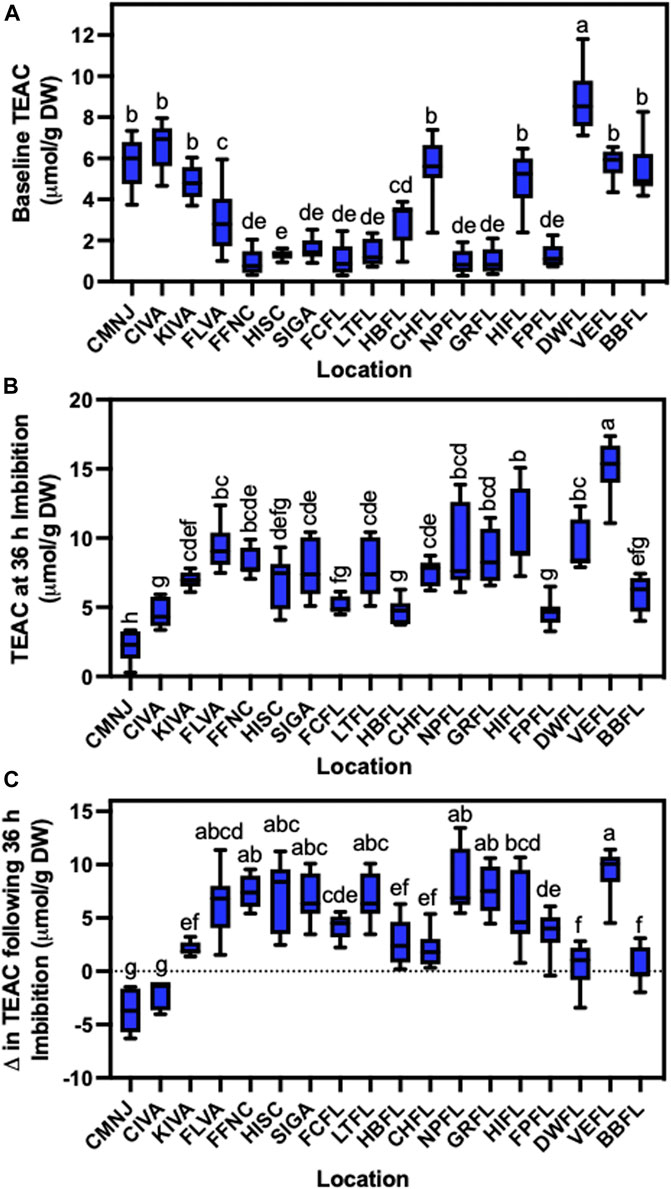
FIGURE 5. Baseline antioxidant levels of dry seeds and after 36 h of imbibition collected from a continental-scale spatial distribution. Baseline TEAC on (A) dry seeds, (B) after 36 h of imbibition, and the (C) difference in TEAC between dry seeds and after 36 h of imbibition. The Trolox Equivalent Antioxidant Capacity (TEAC) was extracted using the modified ABTS/TEAC assay in three biological replicates and three technical replicates. Different letters code based on one way analysis of variance (ANOVA) at an alpha of 0.05 indicate significant differences (n = 3).
Geographic and environmental patterns in relation to seed traits in sea oats from US Atlantic and Gulf coastlines
To assess the impact of latitude on antioxidant capacity, we used baseline seed TEAC concentrations and compared these with the latitudinal range where seeds were collected. In general, antioxidant levels in dry seeds displayed a concave parabolic pattern when evaluated against latitude, heat, and plant hardiness zones (Figures 6A–C). Then, we compared antioxidant capacity levels obtained 36 h after imbibition with the latitudinal zones (Figure 6D). We observed higher TEAC levels near the equator and lower levels in northern latitudes. Surprisingly, relationships between TEAC and AHS heat or USDA plant hardiness classifications showed similar patterns when TEAC data obtained from seeds imbibed after 36 h (Figures 6E, F). Zones with a greater number of hot days (Figure 6F) and less freezing conditions (Figure 6E) displayed higher TEAC values. We found a strong (R2: 0.50) latitudinal pattern for TEAC obtained from dry seeds compared against latitude (Figure 6A) and temperature zoning (AHS heat) (Figure 6B) as well as on temperature zoning in 36 h imbibed seeds (Figure 6E). We also found considerable patterns when TEAC obtained from dry and imbibed seeds were compared against USDA plant hardiness (Figures 6C, F) and imbibed seeds against latitude (Figure 6D).
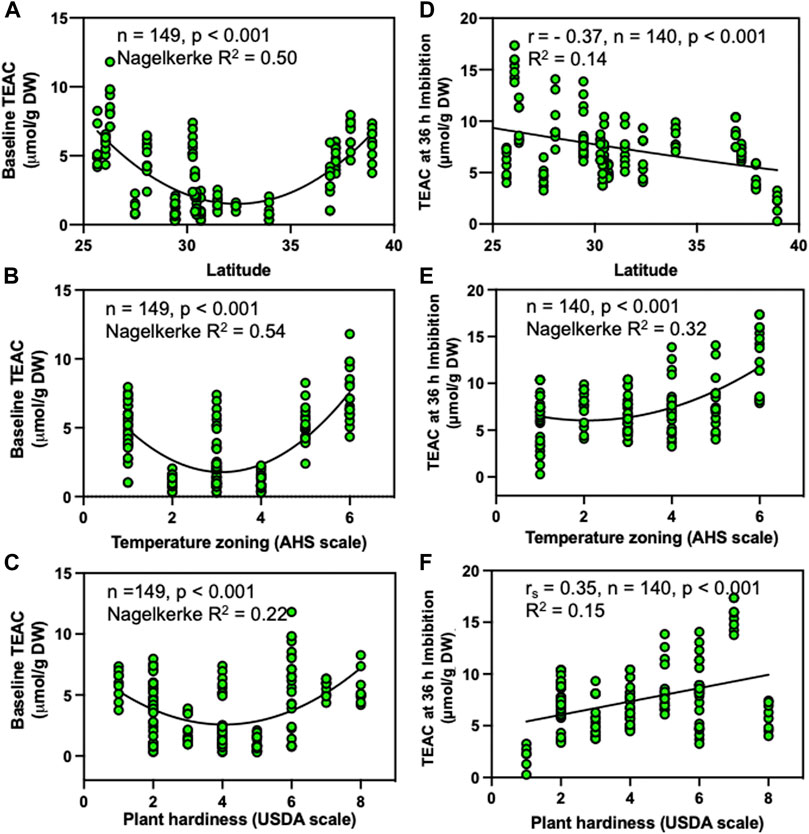
FIGURE 6. Influence of location and environmental zoning by temperature factors on antioxidant levels. Relationship of TEAC in dry seeds (A–C) and after 36 h of imbibition (D–F). (A–D) Latitude, (B–E) AHS temperature zoning, and (C–F) USDA plant hardiness zoning. The AHS temperature zoning is based on days at temperatures above 86°C, covering 45–60 days (1), 60–90 days (2), 90–120 days (3), 120–150 days (4), 150–180 days (5) and 180–210 days (6) per year, while the USDA plant hardiness scale is based on vegetation exposure to cold conditions in winter, at levels of 1(0–10F), 2(10–15F), 3(15–20F), 4(20–25F), 5(25–30F), 6(30–35F), 7(35–40F) and 8(40–45F). In the non-linear models (A–C,E), we represent the overall p values and the statistical significance (Nagelkerke R-squared values) obtained by comparing the non-linear model to a “null model” as described previously (Mangiafico, 2015). For linear regression with continuous data (D), we estimated the coefficient of determination (R2) using the Pearson correlation coefficients (r). For the linear regression using ordinal data (F), we used the Spearman correlation to estimate the Spearman’s rho (rs) and the associated approximate p-values.
Role of the environment and seed traits on the baseline germination and antioxidants in sea oats from the US Atlantic and Gulf coastlines
No clear relationships between germination and baseline TEAC or TEAC after 36 h of imbibition were evident when considering the geographical range of sea oats used in this study (Supplementary Figures S5A, B). Therefore, we analyzed relationships between germination and TEAC using subsets of data representing the extreme temperature regions where high TEAC levels had been observed. Similarly, no clear relationships existed between germination and TEAC after 36 h of imbibition for seeds originating from colder regions (>33N) (Figures 7A, C). However, seeds from warmer regions (<30N), where high seed quality was expected, exhibited a significant negative linear relationship of TEAC levels in imbibed seeds after 36 h (Figures 7B–D).
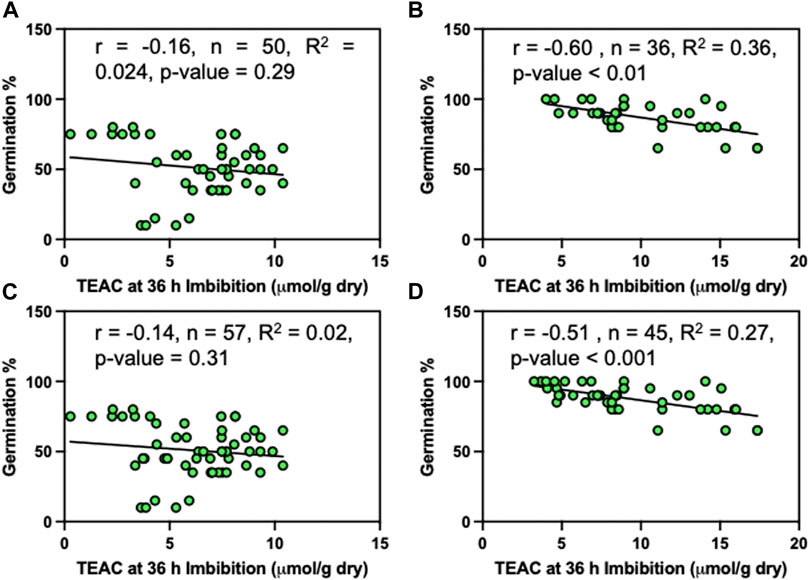
FIGURE 7. Influence of TEAC levels on germination of sea oats seed populations. (A) AHS Temperature zone ≤ 2; (B) AHS Temperature zone > 4; (C) USDA plant hardiness zone ≤ 3 and (D) zone ≥ 6. These zones correspond to latitudinal locations of >33N (A,C) and <30N (B,D). The AHS temperature zoning is based on days at temperatures above 86°C, covering 45–60 days (1), 60–90 days (2), 90–120 days (3), 120–150 days (4), 150–180 days (5) and 180–210 days (6) per year, while the USDA plant hardiness scale is based on vegetation exposure to cold conditions in winter, at levels of 1(0–10F), 2(10–15F), 3(15–20F), 4(20–25F), 5(25–30F), 6(30–35F), 7(35–40F) and 8 (40–45F).
Finally, we compared the influence of latitude and temperature patterns on TEAC levels on dry seeds and seeds imbibed for 36 h (Figure 8). We observed that the minimum and maximum temperatures during the seed development period (Spring and Summer) exhibited high TEAC levels in seeds collected from northern and south latitudes (Figure 8A). As such, the minimum and maximum temperatures exhibited latitudinal patterns of distribution (R2 of 0.21 and 0.20, respectively). Contrary, 36 h of imbibition resulted in lower TEAC levels on seeds collected on northern sites (Figure 8B). We further showed in a correlation matrix the intricate nature of likely interdependence and relations of climate variables with seed germination and seed TEAC characteristics (Supplementary Tables S7–S12), indicating that the environmental and climate conditions faced by sea oat populations substantially impact seed quality traits in terms of germination and antioxidant levels.
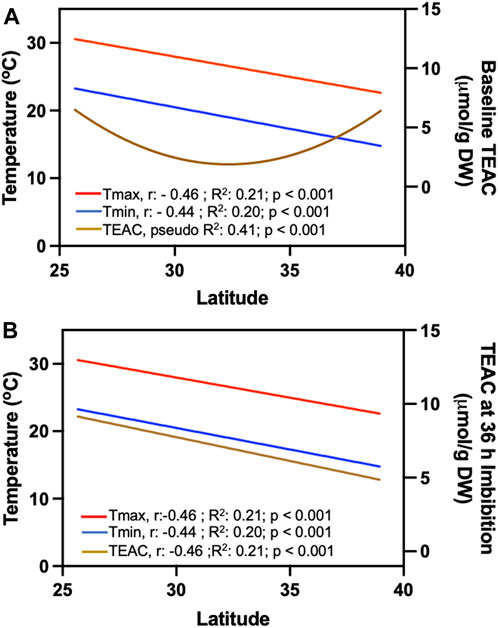
FIGURE 8. Influence of location and latitudinal temperature patterns on baseline antioxidants in sea oats. Tmax: maximum temperatures recorded during the day. Tmin: minimum temperatures recorded during the day in relation to (A) baseline TEAC. We represent the overall p values and the statistical significance (Nagelkerke R-squared values) obtained by comparing the non-linear model to a “null model” as described previously (Mangiafico, 2015). (B) After 36 h of imbibition. We estimated the coefficient of determination (R2) using the Pearson correlation coefficients (r) (n = 1,188).
Discussion
Sea oats are the primary native dune grass used to restore and stabilize beaches and dunes across the southeastern United States. Commercial nurseries have propagated sea oats using field-collected seeds to retain the genetic integrity of source populations. We investigated the effect of climate conditions on sea oats harvested across a wide latitudinal range that spanned more than 13° of latitude (Table 1). Our results show that sea oats seed germination was higher from the sites closer to the equator but decreased as the distance from the equator increased (Figure 1B). The observations of comparative germination with respect to distance from the equator indicated that the sea oat seeds are of high quality in terms of germination percentage from warmer tropical regions compared to seeds from colder areas. Similar patterns of seed germination were observed between populations located in Southern Italy in comparison with a Spanish core population of Lavandula multifida L., a plant species present in the Western Mediterranean Basin (Panuccio et al., 2018).
Environmental and climate influences on seed quality, antioxidants, and implications on seed germination
Antioxidant activity plays a key role in various events of seed life. Antioxidants are produced throughout the seed developmental program and during the germination process. These molecules occur within metabolically active cells, but also in dry tissues, with physiological activity depending on seed moisture status (Vertucci and Farrant, 1995; Walters et al., 2002; Ballesteros et al., 2020). Water potential measurements of dry seeds tested in this study suggest that seeds from all populations fall into the same hydration level (i.e., Hydration level II, ca. −15–190 MPa (Vertucci and Farrant, 1995; Walters et al., 2002; Walters et al., 2005) which was like previous studies (Pérez and Kane, 2017). Hydration level II represents a cellular physiological state characterized by enzyme-mediated catabolic reactions, oxidative and peroxidative processes, and formation of free radicals and reactive oxygen species. Existing pools of molecular antioxidants synthesized during the seed developmental program are thought to play a protective role by quenching reactive oxygen species and free radicals in dry seeds (Vertucci and Farrant, 1995; Walters et al., 2002; Walters et al., 2005). We did not observe a clear relationship between TEAC concentration and baseline seed water potential in this study. Nonetheless, seeds from all populations display antioxidant capacity that may be sufficient to mediate aging associated deteriorative reactions to some degree during storage under non-optimal conditions of temperature and relative humidity (Walters et al., 2005). Moreover, it is well documented that environmental conditions directly impact seed quality as well as their desiccation tolerance, nutrient level, storage potential and the accumulation of antioxidants and other related secondary metabolites (Bewley et al., 2013; Pehlivan, 2017; Ellis, 2019; Malovichko et al., 2021).
We observed differences in the accumulation of antioxidant levels in dry seeds compared to imbibed seeds across all populations (Figures 5, 6). Increased antioxidant activity during seed germination is vital in signaling and ROS homeostasis (Bailly, 2004; El-Maarouf-Bouteau and Bailly, 2008). High germination capacity associated with moderate changes in TEAC levels after initial hours of imbibition is expected to be a characteristic of good-quality seeds because it is linked to a low lipid peroxidation (Rogozhin et al., 2001). In our study, seeds from warmer regions with sharp increases in TEAC following imbibition had poor germination compared to those with limited fluctuations in TEAC. This rise in TEAC levels may be a physiological response to prevent seed deterioration and can also be an indicator of poor-quality seeds or seed damage. These results agree with the elevated antioxidant levels (α-tocopherol and tocotrienols) found in flax (Linum usitatissimum) (Oomah et al., 1997) and in barley (Hordeum vulgare) seeds after elevated temperatures (Roach et al., 2018). Similarly, a negative correlation between antioxidants (α-tocopherol and β-tocopherols) was associated with poor longevity for rice seeds from temperate regions (Lee et al., 2019). Therefore, the antioxidant variation may have been conditioned by environmental conditions as reported in other plant species.
Latitudinal gradients correlate with antioxidant accumulation
We aimed to answer whether latitudinal gradients have an impact on seed trait performance. Our results showed variation on germination and antioxidant accumulation along the latitudinal gradient (Figures 1B, 6). Interestingly, the latitude-associated geographic distribution of antioxidants in leaves of Bilberry (Vaccinium myrtillus L.) was linked to adaptions to prevailing conditions and prevailing abiotic stress associated with a given geographic location (Martz et al., 2010). Similarly, secondary metabolites such as anthocyanidin are strongly controlled by the latitude and geographic origin in V. myrtillus fruits (Åkerström et al., 2010). A similar case was reported for blueberries (Vaccinium ashei cv. “Brightwell”) in higher altitudes with higher concentrations of flavonoids, phenols, proanthocyanidins, and anthocyanins, among other compounds (Zeng et al., 2020). Our data shows that latitude-associated environmental variables, especially precipitation and temperatures, influence seed germination and antioxidant characteristics. However, other environmental variables that vary along the latitudinal gradient, such as relative humidity, may also impact seed quality hence further research to address such factors on sea oat seed traits will help to answer this question.
In summary, our results show a significant influence on seed physiological performance along a latitudinal gradient with varying environmental conditions in sea oats. Therefore, it is likely that environmental variables dictate antioxidant accumulation in ungerminated seeds and after imbibition. Antioxidants are among the seed storage components critical for persistence, longevity, storage quality, and viability (Long et al., 2015). Therefore, the involvement of the environment in accumulating these secondary metabolites alongside other storage reserves is critical in seed quality. Knowledge of the interaction of associated environmental factors and their interaction is crucial for plants. It can be helpful in guiding the collection of seed materials and seed quality control for storage and use in conservation efforts.
Data availability statement
The raw data supporting the conclusion of this article will be made available by the authors, without undue reservation.
Author contributions
AE: Data curation, Formal analysis, Investigation, Methodology, Visualization, Writing–review and editing. HP: Conceptualization, Funding acquisition, Supervision, Writing–review and editing. KB: Conceptualization, Funding acquisition, Supervision, Writing–original draft.
Funding
The authors declare financial support was received for the research, authorship, and/or publication of this article. This work was developed as part of the US Dept. of Comm. Sea Grant Program funding (Grant number SINERR-2018-8). This work was also partially supported by the USDA National Institute of Food and Agriculture, Hatch project FLA-ENH-005853.
Acknowledgments
We thank Dr. William Hammond for his insightful suggestions on the data analysis and Dr. Tia Tyler for the technical assistance during the seed collection.
Conflict of interest
The authors declare that the research was conducted in the absence of any commercial or financial relationships that could be construed as a potential conflict of interest.
Publisher’s note
All claims expressed in this article are solely those of the authors and do not necessarily represent those of their affiliated organizations, or those of the publisher, the editors and the reviewers. Any product that may be evaluated in this article, or claim that may be made by its manufacturer, is not guaranteed or endorsed by the publisher.
Supplementary material
The Supplementary Material for this article can be found online at: https://www.frontiersin.org/articles/10.3389/fenvs.2023.1263300/full#supplementary-material
References
Adetunji, A. E., Sershen Varghese, B., and Pammenter, N. (2021). Effects of exogenous application of five antioxidants on vigour, viability, oxidative metabolism and germination enzymes in aged cabbage and lettuce seeds. South Afr. J. Bot. 137, 85–97. doi:10.1016/j.sajb.2020.10.001
Åkerström, A., Jaakola, L., Bång, U., and Jäderlund, A. (2010). Effects of latitude-related factors and geographical origin on anthocyanidin concentrations in fruits of Vaccinium myrtillus L. (Bilberries). J. Agric. Food Chem. 58, 11939–11945. doi:10.1021/jf102407n
Bailly, C. (2004). Active oxygen species and antioxidants in seed biology. Seed Sci. Res. 14, 93–107. doi:10.1079/ssr2004159
Bailly, C. (2019). The signalling role of ROS in the regulation of seed germination and dormancy. Biochem. J. 476, 3019–3032. doi:10.1042/BCJ20190159
Ballesteros, D., Pritchard, H. W., and Walters, C. (2020). Dry architecture: towards the understanding of the variation of longevity in desiccation-tolerant germplasm. Seed Sci. Res. 30, 142–155. doi:10.1017/S0960258520000239
Baskin, C. C., and Baskin, J. M. (2014). Ecologically meaningful germination studies. Seeds 2014, 5–35. doi:10.1016/B978-0-12-416677-6.00002-0
Begcy, K., Mariano, E. D., Gentile, A., Lembke, C. G., Zingaretti, S. M., Souza, G. M., et al. (2012). A novel stress-induced sugarcane gene confers tolerance to drought, salt and oxidative stress in transgenic tobacco plants. PLoS ONE 7, e44697. doi:10.1371/journal.pone.0044697
Begcy, K., Sandhu, J., and Walia, H. (2018). Transient heat stress during early seed development primes germination and seedling establishment in rice. Front. Plant Sci. 9, 1768. doi:10.3389/fpls.2018.01768
Bewley, J. D., Bradford, K. J., Hilhorst, H. W. M., and Nonogaki, H. (2013). “Development and maturation,” in Seeds (New York, NY: Springer New York), 27–83. doi:10.1007/978-1-4614-4693-4_2
Capblancq, T., Lachmuth, S., Fitzpatrick, M. C., and Keller, S. R. (2022). From common gardens to candidate genes: exploring local adaptation to climate in red spruce. New Phytol. 237, 1590–1605. doi:10.1111/nph.18465
Carta, A., Bottega, S., and Spanò, C. (2018). Aerobic environment ensures viability and anti-oxidant capacity when seeds are wet with negative effect when moist: implications for persistence in the soil. Seed Sci. Res. 28, 16–23. doi:10.1017/S0960258517000307
Chen, D., Li, Y., Fang, T., Shi, X., and Chen, X. (2016). Specific roles of tocopherols and tocotrienols in seed longevity and germination tolerance to abiotic stress in transgenic rice. Plant Sci. 244, 31–39. doi:10.1016/j.plantsci.2015.12.005
Corbineau, F. (2012). Markers of seed quality: from present to future. Seed Sci. Res. 22, S61–S68. doi:10.1017/S0960258511000419
Eckert, A. J., and Neale, D. B. (2022). Probing the dark matter of environmental associations yields novel insights into the architecture of adaptation. New Phytol. 237, 1479–1482. doi:10.1111/nph.18639
Ellis, R. H. (2019). Temporal patterns of seed quality development, decline, and timing of maximum quality during seed development and maturation. Seed Sci. Res. 29, 135–142. doi:10.1017/S0960258519000102
El-Maarouf-Bouteau, H., and Bailly, C. (2008). Oxidative signaling in seed germination and dormancy. Plant Signal. Behav. 3, 175–182. doi:10.4161/psb.3.3.5539
Franks, S. J., Richards, C. L., Gonzales, E., Cousins, J. E., and Hamrick, J. L. (2004). Multi-scale genetic analysis of Uniola paniculata (Poaceae): a coastal species with a linear, fragmented distribution. Am. J. Bot. 91, 1345–1351. doi:10.3732/ajb.91.9.1345
Groot, S. P. C., de Groot, L., Kodde, J., and van Treuren, R. (2015). Prolonging the longevity of ex situ conserved seeds by storage under anoxia. Plant Genet. Resour. 13, 18–26. doi:10.1017/S1479262114000586
Hacker, S. D., Jay, K. R., Cohn, N., Goldstein, E. B., Hovenga, P. A., Itzkin, M., et al. (2019). Species-specific functional morphology of four US atlantic coast dune grasses: biogeographic implications for dune shape and coastal protection. Diversity 11, 82. doi:10.3390/d11050082
Halliwell, B., Aeschbach, R., Löliger, J., and Aruoma, O. I. (1995). The characterization of antioxidants. Food Chem. Toxicol. 33, 601–617. doi:10.1016/0278-6915(95)00024-V
Hart, E. M., and Bell, K. (2015). prism: download data from the Oregon prism project. Available at: https://github.com/ropensci/prism.
Hodel, R. G., and Gonzales, E. (2013). Phylogeography of sea oats (uniola paniculata), a dune-building coastal grass in southeastern north America. J. Hered. 104, 656–665. doi:10.1093/jhered/est035
Kim, T., Samraj, S., Jiménez, J., Gómez, C., Liu, T., and Begcy, K. (2021). Genome-wide identification of heat shock factors and heat shock proteins in response to UV and high intensity light stress in lettuce. BMC Plant Biol. 21, 185. doi:10.1186/s12870-021-02959-x
Lee, J. S., Kwak, J., Cho, J. H., Chebotarov, D., Yoon, M. R., Lee, J. S., et al. (2019). A high proportion of beta-tocopherol in vitamin E is associated with poor seed longevity in rice produced under temperate conditions. Plant Genet. Resour. Charact. Util. 17, 375–378. doi:10.1017/S147926211900008X
Lonard, R. I., Judd, F. W., and Stalter, R. (2011). Biological flora of coastal dunes and wetlands: uniola paniculata L. J. Coast. Res. 276, 984–993. doi:10.2112/JCOASTRES-D-10-00167.1
Long, R. L., Gorecki, M. J., Renton, M., Scott, J. K., Colville, L., Goggin, D. E., et al. (2015). The ecophysiology of seed persistence: a mechanistic view of the journey to germination or demise. Biol. Rev. 90, 31–59. doi:10.1111/brv.12095
Malovichko, Y. V., Shikov, A. E., Nizhnikov, A. A., and Antonets, K. S. (2021). Temporal control of seed development in dicots: molecular bases, ecological impact and possible evolutionary ramifications. Int. J. Mol. Sci. 22, 9252. doi:10.3390/ijms22179252
Mangiafico, S. S. (2015). An R companion for the handbook of biological statistics, version 1.3.9, revised 2023. Available at: rcompanion.org/rcompanion/.
Martz, F., Jaakola, L., Julkunen-Tiitto, R., and Stark, S. (2010). Phenolic composition and antioxidant capacity of Bilberry (vaccinium myrtillus) leaves in northern europe following foliar development and along environmental gradients. J. Chem. Ecol. 36, 1017–1028. doi:10.1007/s10886-010-9836-9
Moreira, X., Abdala-Roberts, L., Bruun, H. H., Covelo, F., De Frenne, P., Galmán, A., et al. (2020). Latitudinal variation in seed predation correlates with latitudinal variation in seed defensive and nutritional traits in a widespread oak species. Ann. Bot. 125, 881–890. doi:10.1093/aob/mcz207
Nguyen, C. D., Chen, J., Clark, D., Perez, H., Huo, H., and Alfred), (2021). Effects of maternal environment on seed germination and seedling vigor of petunia × hybrida under different abiotic stresses. Plants 10, 581. doi:10.3390/plants10030581
Oomah, B. D., Kenaschuk, E. O., and Mazza, G. (1997). Tocopherols in flaxseed. J. Agric. Food Chem. 45, 2076–2080. doi:10.1021/jf960735g
Panuccio, M. R., Fazio, A., Musarella, A. J., Mendoza-fernández, A. F., Mota, J. F., and Spampinato, G. (2018). Seed germination and antioxidant pattern in <b>Lavandula multifida</b> (<b>Lamiaceae</b>): a comparison between core and peripheral populations. Plant Biosyst. 152, 398–406. doi:10.1080/11263504.2017.1297333
Pehlivan, F. E. (2017). “Free radicals and antioxidant system in seed biology,” in Advances in seed biology. Editor J. C. Jimenez-Lopez (InTech). doi:10.5772/intechopen.70837
Pérez, H. E., and Kane, M. E. (2017). Different plant provenance same seed tolerance to abiotic stress: implications for ex situ germplasm conservation of a widely distributed coastal dune grass (Uniola paniculata L.). Plant Growth Regul. 82, 123–137. doi:10.1007/s10725-016-0244-1
Peters, J., and Lanham, B. (2000). Tetrazolium testing handbook, contribution No. 29 to the handbook on seed testing. New York, NY: Association of Official Seed Analysts.
R Core Team (2021). R: a language and environment for statistical computing. Available at: https://www.R-project.org/.
Re, R., Pellegrini, N., Proteggente, A., Pannala, A., Yang, M., and Rice-Evans, C. (1999). Antioxidant activity applying an improved ABTS radical cation decolorization assay. Free Radic. Biol. Med. 26, 1231–1237. doi:10.1016/S0891-5849(98)00315-3
Roach, T., Nagel, M., Börner, A., Eberle, C., and Kranner, I. (2018). Changes in tocochromanols and glutathione reveal differences in the mechanisms of seed ageing under seedbank conditions and controlled deterioration in barley. Environ. Exp. Bot. 156, 8–15. doi:10.1016/j.envexpbot.2018.08.027
Rogozhin, V. V., Verkhoturov, V. V., and Kurilyuk, T. T. (2001). The antioxidant system of wheat seeds during germination. Biol. Bull. Russ. Acad. Sci. 28, 126–133. doi:10.1023/a:1009454713659
Ross, K. A., Zhang, L., and Arntfield, S. D. (2010). Understanding water uptake from the induced changes occurred during processing: chemistry of pinto and navy bean seed coats. Int. J. Food Prop. 13, 631–647. doi:10.1080/10942910902718220
Saatkamp, A., Cochrane, A., Commander, L., Guja, L. K., Jimenez-Alfaro, B., Larson, J., et al. (2019). A research agenda for seed-trait functional ecology. New Phytol. 221, 1764–1775. doi:10.1111/nph.15502
Stegner, M., Wagner, J., and Roach, T. (2022). Antioxidant depletion during seed storage under ambient conditions. Seed Sci. Res. 32, 150–156. doi:10.1017/S0960258522000101
Subudhi, P. K., Parami, N. P., Harrison, S. A., Materne, M. D., Murphy, J. P., and Nash, D. (2005). An AFLP-based survey of genetic diversity among accessions of sea oats (Uniola paniculata, Poaceae) from the southeastern Atlantic and Gulf coast states of the United States. Theor. Appl. Genet. 111, 1632–1641. doi:10.1007/s00122-005-0096-y
Vertucci, C. W., and Farrant, J. M. (1995). “Acquisition and loss of desiccation torelance,” in Seed development and germination. Editors J. Kigel, and G. Galili (New York, NY: CRC Press Taylor & Francis Group), 237–271. doi:10.1201/9780203740071
Walters, C., Farrant, J. M., Pammenter, N. W., and Berjak, P. (2002). “Desiccation stress and damage,” in Desiccation and survival in plants: drying without dying. Editors M. Black, and H. W. Pritchard (Wallingford, Oxon, UK ; New York: CABI Pub).
Walters, C., Hill, L. M., and Wheeler, L. J. (2005). Dying while dry: kinetics and mechanisms of deterioration in desiccated organisms. Integr. Comp. Biol. 45, 751–758. doi:10.1093/icb/45.5.751
Zeng, Q., Dong, G., Tian, L., Wu, H., Ren, Y., Tamir, G., et al. (2020). High altitude is beneficial for antioxidant components and sweetness accumulation of rabbiteye blueberry. Front. Plant Sci. 11, 573531. doi:10.3389/fpls.2020.573531
Keywords: climate, environmental gradients, germination, latitude, seed ecophysiology
Citation: Egesa AO, Pérez HE and Begcy K (2023) Environmental conditions predetermine quality, germination, and innate antioxidants pool in sea oat (Uniola paniculata L.) seeds. Front. Environ. Sci. 11:1263300. doi: 10.3389/fenvs.2023.1263300
Received: 19 July 2023; Accepted: 17 October 2023;
Published: 06 November 2023.
Edited by:
Tao Yao, Oak Ridge National Laboratory, United StatesReviewed by:
Arnd G. Heyer, University of Stuttgart, GermanyRuchika Rajput, Oak Ridge National Laboratory (DOE), United States
Haihua Xiao, Sichuan Agricultural University, China
Copyright © 2023 Egesa, Pérez and Begcy. This is an open-access article distributed under the terms of the Creative Commons Attribution License (CC BY). The use, distribution or reproduction in other forums is permitted, provided the original author(s) and the copyright owner(s) are credited and that the original publication in this journal is cited, in accordance with accepted academic practice. No use, distribution or reproduction is permitted which does not comply with these terms.
*Correspondence: Kevin Begcy, a2JlZ2N5LnBhZGlsbGFAdWZsLmVkdQ==
†ORCID: Andrew Ogolla Egesa, https://orcid.org/0000-0002-9829-9009; Héctor E. Pérez, https://orcid.org/0000-0001-5609-2571; Kevin Begcy, https://orcid.org/0000-0002-5046-8029