- 1College of Environment and Surveying and Mapping Engineering, Suzhou University, Suzhou, Anhui, China
- 2Faculty of Horticulture and Landscape Engineering, Institute of Landscape Engineering, Slovak University of Agriculture in Nitra, Nitra, Slovakia
Phytoremediation is one of the cheapest and most widely used technologies for stabilizing and extracting pollutants from contaminated sites. Recently, a variety of solutions, such as the use of different elements, compost, nanoparticles, microorganisms, etc., have been explored for improving and accelerating the phytoremediation process. Biochar has also gained attention for its affordability, abundance, ability to improve soil structure and plant morpho-physiology and biochemistry, lack of environmental hazards, etc. As a first step, this study aimed to provide an overview of biochar’s properties, and operation by identifying the method of production and examining the differences between different types of biochar. Following that, by examining various factors that pollute the environment, the influence of different types of biochar on phytoremediation efficiency was explored. Also, in this study, an attempt has been made to examine the effect of the combination of biochar with other factors in improving the phytoremediation of pollutants, as well as the use of the residues of phytoremediation for the production of biochar, so that future research can be planned based on the results obtained.
1 Introduction
Biochar is a stable carbon-rich product that is made by decomposing animal or plant residues under anaerobic (or low oxygen) and heat conditions (Lehmann and Joseph, 2015; Nguyen et al., 2017; Tomczyk et al., 2020). This porous material, which contains more than 60% carbon, along with oil and gas, is one of the products of biomass decomposition by heat (Lebrun et al., 2021a), and based on research, thousands-year-old biochar particles have been found in the soil of Amazon’s tropical and humid regions (Narayanan and Ma, 2022). Since it has a special properties, this cheap material has a very high application and efficiency in improving different soil ecosystems (Ghosh and Maiti, 2021). In general, Biochar is obtained from the decomposition of various biomass such as urban waste, industrial waste, agricultural waste (sugarcane, sugar beet, corn, weeds, etc.), poultry litter, animal manure, forestry waste (waste of trees, wood, bamboo, etc.) and sludge (Khiari et al., 2019; Kiran and Prasad, 2019; Maroušek et al., 2019; Albalasmeh et al., 2020; Simiele et al., 2020) (Figure 1).
To prepare Biochar, biomass is heated to between 400°C and 700°C under limited oxygen conditions (decomposition by pyrolysis method) (Yavari et al., 2015; Simiele et al., 2020), and biochar’s physicochemical properties are directly related to its raw material and pyrolysis conditions (Benavente et al., 2018; Albalasmeh et al., 2020) (Table 1), and to the extent that biochar derived from animal manure has more nutrients than biochar derived from plants (Narayanan and Ma, 2022). Researchers also found that changing the temperature of pyrolysis from 400°C to 500°C increases the content (mg/kg) of magnesium (Mg), potassium (K), phosphate (P), Carbon (C), nitrogen (N), oxygen (O), hydrogen (H), and calcium (Ca) in the biochar produced, and this increase in temperature also increases the mineralization of raw materials (Ma et al., 2015; Brassard et al., 2017; Ferjani et al., 2019). Additionally, the cost of raw materials, labor costs, and production technologies affect the cost of biochar production, and the average cost varies from one hundred to five thousand US dollars per ton in different countries (Maroušek et al., 2019) (Figure 2).
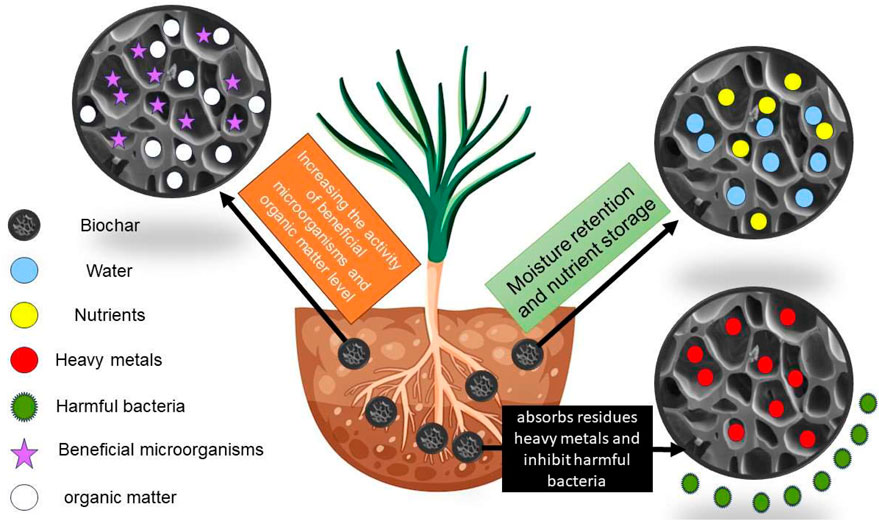
FIGURE 2. An analysis of the soil components affected by biochar. The results are taken from Chukwuka et al. (2020).
Biochar can be widely used in agriculture and the environment due to its unique characteristics, which include its ability to improve soil fertility and adsorption of various pollutants (Xie et al., 2015; Zanganeh et al., 2022). The special characteristics of biochar include high porosity, high cation exchange capacity (CEC), alkaline pH, low density, abundant functional groups (aromatic and heterocyclic carbons such as C=N, COOH, OH), high water holding capacity, high specific surface area and adsorption capacity (Czekała et al., 2016; Du et al., 2019a; Simiele et al., 2020; Tu et al., 2020; Li et al., 2021a; Gonzaga et al., 2022). According to Ahmad et al., biochar is an alkaline material with pH 8-11, a specific surface area of 10–1,400 m2·g−1, a porosity of 0–1.32 cm3·g−1, and CEC of 25–485 cmol (þ) kg−1 (Ahmad et al., 2014). Furthermore, while biochar has a very low mineralization rate, its particle size affects its interactions with soil, changing its porosity and ions adsorption and exchange capacity (Williams et al., 2019; Li et al., 2021a).
Biochar is a low-cost and environmentally friendly amendment that can cause long-term positive effects in soil ecosystems by improving biogeochemical processes and physicochemical properties (Gul et al., 2015; Zhang et al., 2018). Biochar’s higher content of polycyclic aromatic hydrocarbons (PAHs) than other organic carbons makes it more compatible with soils and contributes to climate change mitigation through improving soil ventilation, carbon sequestration, and reduction of greenhouse gases such as Nitrous oxide (N2O) (Rizwan et al., 2016; Kolton et al., 2017; Lian and Xing, 2017; Kiran and Prasad, 2019). Biochar increases soil fertility by improving water and nutrient availability and increasing the content of organic matter (Lebrun et al., 2018; Lebrun et al., 2021a; Ali et al., 2021), and by softening soil, it improves water retention capacity, reduces nutrient leaching, and improves nutrient cycling in soil (Zhang et al., 2018; Li et al., 2021a; Gholizadeh and Hu, 2021). Additionally, biochar in soil regulates pH, CEC, EC, and ash content as well as increasing catalase, urease, and dehydrogenase activity, and increasing microbial activities, especially indigenous microbes (Hossain et al., 2010; Binti Ab Aziz et al., 2015; Chen et al., 2017; Zhang et al., 2019b; Gong et al., 2021) (Figure 2).
Furthermore, biochar increases plant growth, biomass, and yield by improving soil conditions, improving water quality, providing nutrients, increasing root colonization by microbes, and improving the propagation and surface of roots (Paz-Ferreiro et al., 2014; Doan et al., 2015; Rees et al., 2016; Herman and Resigia, 2018; Zhang et al., 2019a; Zhou et al., 2020). Nonetheless, dissolved organic matter in biochar (aqueous biochar extract) contains a considerable amount of organic compounds, including organic acids with low molecular weight, humic organic molecules, and complex sugars, which have significant effects on plant growth (Lou et al., 2016; Bian et al., 2018). Enriching biochar with elements such as silicon, iron, selenium, calcium, etc., in addition to increasing biochar availability in soil, can improve the positive properties of biochar (Rajkovich et al., 2012; Simiele et al., 2020), further, other methods for modifying biochar have been considered, including ultraviolet (UV) radiation, which increases the specific surface area of biochar and can introduce oxygen-containing functional groups (Peng et al., 2019). Several studies have been conducted in recent years on the pyrolysis of lignocellulosic wastes to produce biochar, and if this technology becomes common, it can be seen as a promising method for reducing greenhouse gas emissions and other environmental pollution in addition to producing added value (Liu et al., 2015b; Du et al., 2019a; Du et al., 2019b). In this study, various pollutants in the soil ecosystem were investigated, as well as how phytoremediation reduces these pollutants, and how biochar affect in the process of phytoremediation.
2 Soil pollution problem
In general, HMs consist of elements with a high atomic mass and weight, and a specific density exceeding 5 g/cm3, and are classified into two groups, essentials, and non-essentials (Buha et al., 2014; Farooq et al., 2016). Essential elements (micronutrients) are necessary in very small amounts for plants and animals, such as copper (Cu), iron (Fe), manganese (Mn), cobalt (Co), zinc (Zn), and chromium (Cr), which in high concentrations can cause soil pollution and harm to organisms (Naja and Volesky, 2017; Awad et al., 2021; Rathika et al., 2021); However, non-essential elements [including cadmium (Cd), mercury, (Hg) arsenic (As), and lead (Pb)] are very dangerous and can lead to severe soil contamination (Huang et al., 2012; Rathika et al., 2021; Haider et al., 2022; Boorboori, 2023). In contrast to organic pollutants, HMs are resistant to degradation and remain in soil and sediment for a long time, for instance, it has been reported that HMs contamination can persist in soil for more than 5,000 years (Lu et al., 2015; Gong et al., 2018b; Li et al., 2021b), as a result, long-term persistence in polluted sites facilitates their entry into the food cycle and ultimately causes severe environmental damage (Xu et al., 2018b; Zhao et al., 2019; Zanganeh et al., 2022) (Table 2).
3 Phytoremediation of metal(iod)s
Considering the growing trend of combined pollution in soil, it seems necessary to find strategies for cleaning contaminated soil (Zhen et al., 2019; Zhang et al., 2021; Fernández-Braña et al., 2023). There have been several physical and chemical methods also are available for the remediation of soils contaminated with HMs, including encapsulation, soil washing, freezing, electrokinetic remediation, membrane filtration, reverse osmosis (RO), 3 electrodialytic remediation (EDR), landfilling, nanomaterials, immobilization, and surface capping (Barati et al., 2018; Liu et al., 2018; Kiran and Prasad, 2019; Shah and Daverey, 2020). However, all the aforementioned techniques have several limitations, including high economic costs, creating secondary pollution for the environment, inapplicability in wide areas, high energy consumption, and extensive chemical needs (Paz-Alberto and Sigua, 2013; Gkorezis et al., 2016; Lin et al., 2019; Yrjälä and Lopez-Echartea, 2021), therefore, finding cheaper and more effective methods can be a remedial to reduce pollution in soils contaminated with HMs.
During the past few decades, plants have been used to decompose, convert, stabilize, or remove pollutants in sediments, underground and surface water, soil, and the atmosphere (Reddy and Chirakkara, 2013; Ratnasari et al., 2020), and this green biological technique based on hyperaccumulators or non-hyperaccumulators plant species and their associated microorganisms is called phytoremediation (Ma et al., 2016; Jha et al., 2017; Rathika et al., 2021; Boorboori and Zhang, 2022a). The low cost of phytoremediation (about 25–100 US dollars per ton of soil) makes it a cost-effective technology that, in addition to being environmentally friendly, is highly effective and simple to use (Wang et al., 2017a; Gao et al., 2018; Logeshwaran et al., 2018). As a result of its general acceptance, no disruption to soil fertility and biodiversity, prevention of soil erosion, carbon sequestration, extensive application, and use in sites with multiple types of pollutants, this method has become increasingly subject for potted and field studies (Lebrun et al., 2018; Cárdenas-Aguiar et al., 2020; Zanganeh et al., 2022).
Phytoremediation which can be used alone or in conjunction with other soil remediation methods improves soils contaminated with organic and inorganic compounds by various routes such as extraction, evaporation, transpiration, stabilization, Rhizofiltration, and transformation (Forján et al., 2018; Fancello et al., 2019; Shah and Daverey, 2020) (Table 3). Plant extraction and plant stabilization are the most important phytoremediation processes (Mahar et al., 2016), in the plant extraction method, environmental pollution is absorbed into the roots and transferred to the plant’s aerial organs, which are then harvested and removed (Lebrun et al., 2021a). Although plant extraction offers many advantages, including safety and high efficiency, it faces a problem in disposing of contaminated biomass, which incurs additional costs (Boominathan et al., 2004; Lebrun et al., 2021a).
As the process of extracting plants from highly polluted lands takes tens of years, and because falling leaves and branches also are responsible for returning pollution to the ground, plant stabilization may be a suitable alternative in the contaminated environment (Lucchini et al., 2014; Laghlimi et al., 2015). In this method, which aims to reduce the mobility and bioavailability of pollutants, resistant plants with extensive root systems can be used along with soil amendment compounds, provided that the plants used do not transfer the pollutants from the roots to the aerial organs, but stabilize it on the surface and inside the roots through the complexation with root secretions (Kumpiene et al., 2008; Acosta et al., 2018; Lebrun et al., 2021a). Since HMs do not decompose, plant stabilization is more effective for them and prevents them from transferring to the surrounding environments, and the efficiency of this method depends on several factors, including the hydrological regime, pollution type, soil geochemistry, etc., (Chehregani et al., 2009; Forján et al., 2018; Kiran and Prasad, 2019). Despite all the benefits of phytoremediation, it also has weaknesses, including a long remediation period, non-implementation at high pollution sites, production of contaminated biomass, secondary pollution, slow growth of plants, limited plant resistance to unfavorable physical and chemical conditions found in polluted soils, and plants’ limited capacity to accumulate pollution (Yang et al., 2017b; Cárdenas-Aguiar et al., 2020; Gu et al., 2020; Manori et al., 2021).
4 Phytoremediation efficiency, plant type, and other factors
In the phytoremediation process, plants are used to remove and stabilization of soil pollution without negatively affecting the structure, quality, and biological activities of the soil, so it is necessary to select plants that are not only tolerant of pollution but also fast-growing and provide maximum root surface (Claus et al., 2007; Barati et al., 2018; Li et al., 2021b). Also, phytoremediation can be used as a first step to creating wildlife habitats, which will only be possible if native plant species are used that are well adapted to the local climate and soil (Roohi et al., 2020), because native plants will have better growth, survival, and reproduction under environmental stress than non-native plants (Barati et al., 2018) (Table 3). There has been a high degree of focus in recent years on woody plant species in phytoremediation because these plant species, in addition to concentrating soil pollution in their tissues, can be used for various types of pollution as well as be economically viable due to their efficiency in various industries (Pandey et al., 2009; Fancello et al., 2019). Plants belonging to the Salicaceae family (Salix and Picea) are among the most important woody species used in phytoremediation (Evlard et al., 2014), which are suitable for growing in abandoned agricultural lands and polluted marginal lands because of their rapid growth, extensive root system, high biomass production rate, ability to support microbial communities, high transpiration rate, capability to absorb nutrients, tolerance to a wide range of organic and inorganic pollutants, high antioxidant activities, and easy reproduction (Wu et al., 2010; Cloutier-Hurteau et al., 2014; Salam et al., 2019; Lebrun et al., 2021b; Li et al., 2021b).
Among other woody plants, Populus x euramericana clones could potentially be used for the phytoremediation of polluted lands due to their high growth, biomass, and ability to extract various types of HMs (Borghi et al., 2007; Redovniković et al., 2017; Simiele et al., 2020). In contrast, herbaceous species with extensive fibrous roots also are very beneficial for remediating polluted soils (APHA, 2003), especially those species known as hyperaccumulators, which are capable of absorbing large amounts of HMs and transferring them to their aerial organs, such as Sedum alfredii and Thlaspi caerulescens (Lebrun et al., 2021b). Symphytum officinale also is a perennial herbaceous plant that, due to its high biomass, rapid growth, and high accumulation of HMs, can be widely spread across large areas and improve phytoremediation (Wang et al., 2017b; Xiao et al., 2018). However, one of the most ideal plants for polluted environments is Solanum nigrum, which is relatively easy to adapt to different conditions and highly tolerant (Liang et al., 2017). This plant has a strong antioxidant system and the ability to produce macrophage proteins and various organic acids in different organs involved in the transport and accumulation of HMs, which makes it capable of extracting several types of HMs (such as Cr, As, Pb, Cd, and Ni) as well as carbon tetrachloride, polycyclic aromatic hydrocarbons, etc., (Liang et al., 2017; Ur Rehman et al., 2017). Among other herbaceous plants with a high ability to heavy metals accumulate and phytoremediation are Beta vulgaris var. cicla and Potentilla griffithii Hook. f. var. velutina Card (Ali et al., 2013; Gu et al., 2020).
The use of economic crops such as rice, cotton, sunflower, etc., which have high environmental compatibility and biomass production potential, has attracted the attention of researchers for the phytoremediation of contaminated land on a large scale (Bhardwaj et al., 2014; Lebrun et al., 2021b; Gusmini et al., 2021). Alfalfa (Medicago sativa L.) is another crop that can be used to remediate contaminated soils, as it has high yields, deep roots, and drought tolerance that distinguish it from other plants used in the phytoremediation process (Agegnehu et al., 2016). Another crop suitable for phytoremediation is corn, which, in addition to its economic importance, can provide a biological indicator of pollution (Khan et al., 2018). With its fibrous root system, global availability, ability to grow in diverse climates, and fast germination rate, corn has special advantages over other crops for phytoremediation (Khan et al., 2018; Lebrun et al., 2021b). In addition, there has been a recent focus on using a periodic system of oil crops to improve polluted soils, which has economic benefits as well as the effect on crop production (Yang et al., 2017b; Gong et al., 2018b).
On the other hand, in the stabilization process, certain plants are used in polluted soils that are not capable of transferring pollutants to their aerial organs, but in addition to reducing the bioavailability and mobility of pollutants in soil, they prevent them from entering underground water and the food chain (Fancello et al., 2019; Roohi et al., 2020). Therefore, plants should be selected that are capable of stabilizing pollutants on the epidermis of their roots or rhizosphere, Among the most important of these plants are Euphorbia Pithyulentum, Spartina anglica, Solanum lycopersicum, Juncus acutus, Lycopersicon esculentum, Phragmites australis, Vallisneria americana, and Bromus tomentellus (Fancello et al., 2019; Zhen et al., 2019; Roohi et al., 2020). In addition, plants of Salicaceae family are also potent stabilizers due to their extensive root systems, abundant biomass, high transpiration rates, ability to grow in polluted environments, high water-use efficiency, and high contaminant accumulation at the roots (Evlard et al., 2014; Redovniković et al., 2017; Lebrun et al., 2018).
In addition to the selection of suitable plants, phytoremediation’s efficiency is highly influenced by the chemical and physical properties of soil, including the quantity and nature of organic matter and nutrients, mineralogy, texture, structure, and pH (Kayser et al., 2000; Zhao et al., 2019). Therefore, modifying polluted environments with mineral and organic modifiers improves phytoremediation efficiency (Cheng et al., 2020). Several studies have demonstrated that a wide range of microbial communities, including bacteria, algae, and fungi, enhance the effectiveness of phytoremediation by influencing the soil’s physical and chemical properties (Koul and Taak, 2018; Cui et al., 2020). A microbial community produces different types of enzymes to regulate degradation pathways through various oxidation reactions that result in the production of CO2 and H2O (Ali et al., 2021). Additionally, microbial communities can improve the morpho-physiological structures of plants through ACC-deaminase activity, phosphorus dissolution, siderophores production, and ethylene stress reduction, thereby increasing phytoremediation efficiency (Liu et al., 2015a; Fatima et al., 2018; Afzal et al., 2020). The microbes also play a major role in changing HMs’ chemical behavior in the rhizosphere, and in addition to influencing plant growth during phytoremediation, that effect at improving HMs extraction, bioavailability, and accumulation in plants as well (Li et al., 2019b; Fancello et al., 2019; Farias et al., 2020).
The use of organic and inorganic modifiers in phytoremediation has gained popularity in recent years (Lebrun et al., 2021a; Manori et al., 2021). Several studies have shown that the addition of these soil modifiers through increasing the stabilization of HMs in the soil, reducing the toxicity of HMs in plants, increasing the cation exchange capacity (CEC) of the soil, improving soil structure, enhancing soil ventilation, increasing nutrient availability and content, and improving soil water holding capacity, increases the efficiency of phytoremediation in polluted environments (Bashir et al., 2018; Roohi et al., 2020; Lebrun et al., 2021a). Furthermore, these organic and inorganic modifiers increase phytoremediation efficiency by stimulating microorganisms’ activities, increasing plant growth and biomass production (Clemente et al., 2012; Barati et al., 2018; Roohi et al., 2020; Boorboori and Zhang, 2022b). The following are some of the most important modifiers used in recent years: poultry droppings, cow dung, biochar, rice husk, compost, technocell, steel slag, carbon materials (CMs), and nanomaterials (NMs) (Singh and Lee, 2016; Forján et al., 2018; Lebrun et al., 2021a; Deebika et al., 2021), and in the following, biochar’s role in phytoremediation of HMs will be discussed in more detail.
5 Biochar and phytoremediation
Since contaminated soil is not an ideal environment for plant growth, phytoremediation is greatly reduced in efficiency (Fellet et al., 2014). Therefore, the use of modifiers like Biochar can, in addition to improving soil structure, promote the growth of plants used for phytoremediation (Simiele et al., 2020; Li et al., 2021b). Moreover, biochar has attracted researchers’ attention due to its cost-effectiveness and ability to clean environments contaminated with organic and inorganic pollutants (Byrne et al., 2018; Ding et al., 2020). Phytoremediation can be improved with biochar due to its long-term stability compared to other organic amendments as well as its effective role in improving the soil’s physical, chemical, and biological properties (Cárdenas-Aguiar et al., 2019; Ratnasari et al., 2020). By improving soil pH, carbon content, soil aeration, soil fertility, water retention capacity, microbial diversity, and plant growth, biochar is highly effective at cleaning environments contaminated with HMs (Forján et al., 2018; Gong et al., 2019; Deebika et al., 2021). Furthermore, Its high specific surface area, porous structure (macropores and micropores), cation exchange capacity, and active functional groups make biochar an effective tool for stabilizing soil pollution and reducing HMs bioavailability for organisms (Song et al., 2016; Yang et al., 2017a; Zhang et al., 2019a). It is important to note that all the benefits mentioned above depend on the particle size, intrinsic properties, application method, and the amount of biochar applied to the soil (Li et al., 2021a), and in the following, it will be discussed the details how biochar can improve the phytoremediation process of HMs (Figure 3).
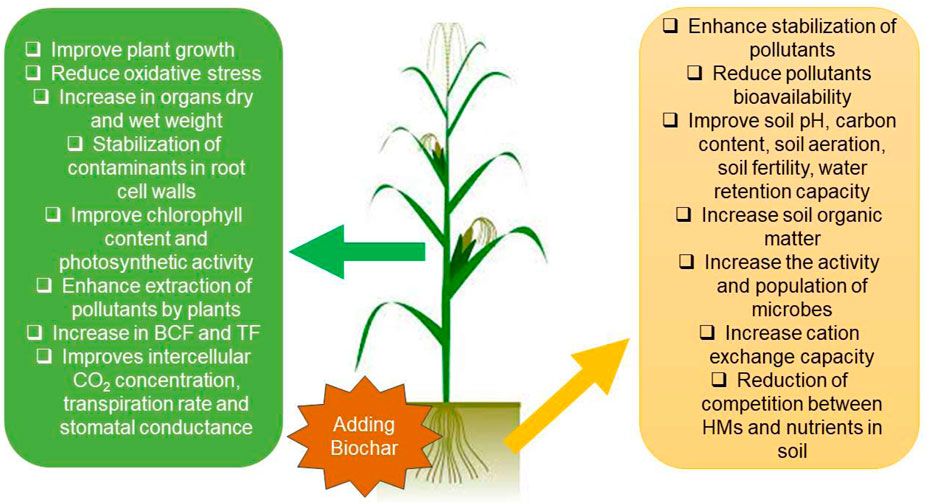
FIGURE 3. The effect of adding biochar on soil and plants in the phytoremediation process. BCF, Bioconcentration factor; TF, Transfer factor; HMs, Heavy metals.
Studies have shown that adding biochar to the culture medium can significantly increase the growth of plants, and this growth may be heterogeneous across different organs of the plant, but it generally results in a 10% increase in overall growth (Paz-Ferreiro et al., 2014; Burachevskaya et al., 2021). Biochar causes morphological changes in plant roots, as well as affects a plant’s ability to absorb pollutants (Rees et al., 2016; Gu et al., 2020); however, the extent of reduction in pollutant absorption depends on the species of plants and the speciation characteristics of environmental pollutants (Gu et al., 2020). On the other hand, the cell walls of roots contain molecules such as pectin, proteins, and dissolved phosphates that stabilize pollutants in the root space and prevent their transfer to aerial organs, and biochar increases the efficiency of these physiological structures in plants’ roots (Lebrun et al., 2017; Gu et al., 2020). Previous studies confirm that amended soils by biochar increase plant tolerance to environmental pollution by improving parameters such as chlorophyll pigment content, intercellular CO2 concentration, transpiration rate, photosynthetic rate, and stomatal conductance, as well as reducing the efficiency of HMs transport in plants (Farias et al., 2020; Li et al., 2021b; Lyu et al., 2021) (Figure 3).
According to a study conducted on Brassica juncea in a copper-contaminated environment, by increasing the rate of Holm oak biochar (BH), plant growth increased and Cu absorption decreased (Rodríguez-Vila et al., 2014). Furthermore, a study conducted on Bidens pilosa grown in Cd-contaminated soil found that pine needle biochar had a positive effect on root growth, and at the same time decreased chlorophyll concentrations and increased proline concentrations, which revealed an increase in Cd toxicity in plant tissues (Manori et al., 2021); therefore it can be concluded that pine needle biochar can be used as a soil amendment to increase plant HMs extraction. In a study conducted by Li et al. (2021b) on Salix psammophila grown in Cu, Zn, and Cd-contaminated environments, it was shown that bamboo biochar, in addition to a slight increase in plant biomass, improved Bioconcentration Factor (BCF) and Translocation Factor (TF), which indicate that bamboo biochar improves the efficiency of HMs extraction and phytoremediation. Additionally, the study on Boehmeria nivea demonstrated that tea waste biochar reduced Cd toxicity and increased its accumulation and displacement in seedlings by changing the intracellular distribution of Cd, reducing oxidative stress, and improving plant growth (Gong et al., 2019) (Table 4).
An investigation of the potential of Ipomoea reptans Poir for phytoremediation of Cd-contaminated soils revealed that adding corn biochar in addition to increasing Cd absorption by seedlings, increased the dry and fresh weight of shoots, the dry and fresh weight of roots, chlorophyll content, the leaf area, the number of leaves and plant height (Ratnasari et al., 2020). In the study conducted on coconut husk and orange bagasse biochars, it was demonstrated that their use for B. juncea under Cu stress with low concentration, increased root and shoot mass, photosynthetic activity, chlorophyll content, Cu absorption from the soil, and Cu concentration in the aerial parts of plants (Gonzaga et al., 2022), which showed that these biochars increased effectiveness of B. juncea in phytoremediation. Zhang et al. (2019b) found that adding rice straw biochar to alfalfa (M. sativa L.) culture medium improved the plant’s ability to extract Cd from contaminated soils as well as produce the seedlings’ biomass. Another study that was conducted on B. vulgaris grown in a Cd-contaminated environment found that cornstalk biochar, in addition to producing more root biomass, increased Cd extraction from the soil environment, as well as Cd absorption into the roots’ cell walls (Gu et al., 2020), therefore, cornstalk biochar, in addition to helping the phytoremediation process, can also enhance B. vulgaris’ resistance to pollutions. Similar results have been reported using straw biochar in improving Cd absorption and root morphology of Sedum plumbizincicola in acidic soils (Li et al., 2018) (Figure 3; Table 4).
As well as influencing the morpho-physiological structure of plants involved in phytoremediation, biochar also helps stabilize pollutants and reduce their bioavailability in the soil through its effects on soil structures (Ghosh and Maiti, 2021; Gonzaga et al., 2022). Since HMs are both cationic and anionic, they interact with the anionic and cationic ions on biochar and directly absorb on the biochar surface, therefore reducing their bioavailability in soil (Rees et al., 2014; Fijałkowska et al., 2021). Biochar Also contains functional groups on its surface that can form complexes with soil pollutants and stabilize them (Ali et al., 2021; Gupta et al., 2021). According to Gupta et al. (2021), when biochar is combined with the soil surface, the biochar particles with cation charged can absorb anionic HMs, such as arsenate and arsenite, while negatively charged particles of biochar stabilize soil positive components, including Pb and Cd (Figure 3).
Rodríguez-Vila et al. (2014) found that Holm oak biochar reduced the bioavailability of Cu in contaminated mine soils, and at the same time, Puga et al. (2015) showed that sugar cane biochar reduced Zn, Pb, and Cd bioavailability in contaminated mineral soils. A study conducted on soils contaminated with Pb and Cd showed that bamboo biochar could stabilize and reduce the bioavailability of these HMs, possibly due to the absorption of HMs on the surface of biochar (Xu et al., 2016). A greenhouse experiment showed that the addition of pig manure biochar reduced Pb leaching by 71% in soils with low organic carbon (Kiran and Prasad, 2019), while Fellet et al. (2014) demonstrated in their studies that garden pruning residues biochar reduces Pb, Cd, and Zn bioavailability in the soil. Moreover, biochar can convert Cr (VI) into less mobile Cr (III) by continuously transferring electrons, which connect with oxygen-containing functional groups on its surface (Dong et al., 2017). A study conducted on soils contaminated with Cd, Pb, Mn, Cu, and Fe showed that date palm waste biochar can significantly reduce the concentration of extractable HMs in the soil (Al-Wabel et al., 2015). Various studies have also shown that miscanthus, poplar wood, and rice straw biochars can significantly stabilize HMs in polluted soils, reducing their bioavailability to living organisms (Houben et al., 2013; Jones et al., 2016; Chen et al., 2018). According to Narayanan and Ma (2022) study, the type of biochar used plays an important role in the amount of Ni and Cd stabilization.
In addition to being capable of stabilizing HMs, biochar can also cause the absorption of soil nutrients, reducing the competition between HMs and nutrients in the soil, which, in addition to the accumulation of soil nutrients, increases the absorption of HMs in plants used in the phytoremediation and extraction process (Rees et al., 2015; Li et al., 2021b). Studies have shown that adding biochar to the environment causes a deficiency of nitrogen, phosphorus, and calcium in plants, and in addition, biochar with different functional groups (carboxylic, lactonic, and organic nitrogen) and inorganic impurities (metal oxides and Ash) increases the capacity of soil to absorb carbon materials (Agrafioti et al., 2014; Rees et al., 2016; Roohi et al., 2020). Aside from competing for absorption sites on biochar, nutrients and HMs also compete for transporters and entry channels into plants (Farhangi-Abriz and Torabian, 2017). As a result of adding bamboo biochar to soil, Li et al. (2021b) found that the soil content of potassium and organic matter increased, and it decreased the accumulation of Zn, Cd, and Cu in the soil, while biochar increased the accumulation of these HMs in S. psammophila, which was used in the experiment (Figure 3).
The addition of biochar to soil changes soil pH, water holding capacity, and dissolved organic content (DOC) (Abdelaal et al., 2021; Narayanan and Ma, 2022). In general, biochar creates a liming effect and increases the pH of the soil, which is due to the increase of ash content in the soil (Simiele et al., 2020), and since pH and DOC have a significant effect on HMs removal and absorption in soil (Wang et al., 2020), thus, adding biochar to the soil increases the mobility of anionic HMs like Selenium (Se), As, Cu, Cr, and Antimony (Sb), among which Cu mobility heavily depends on DOC content of biochar (Sun et al., 2018; Gupta et al., 2021; Narayanan and Ma, 2022). Furthermore, some studies have shown that increasing the pH of soil through biochar addition reduces the bioavailability of some heavy metals (such as Pb, Zn, and Cd), and reduces their concentration in soil pore water (Rees et al., 2015; Lebrun et al., 2017; Mehmood et al., 2018; Zhang et al., 2021) (Figures 3, 4).
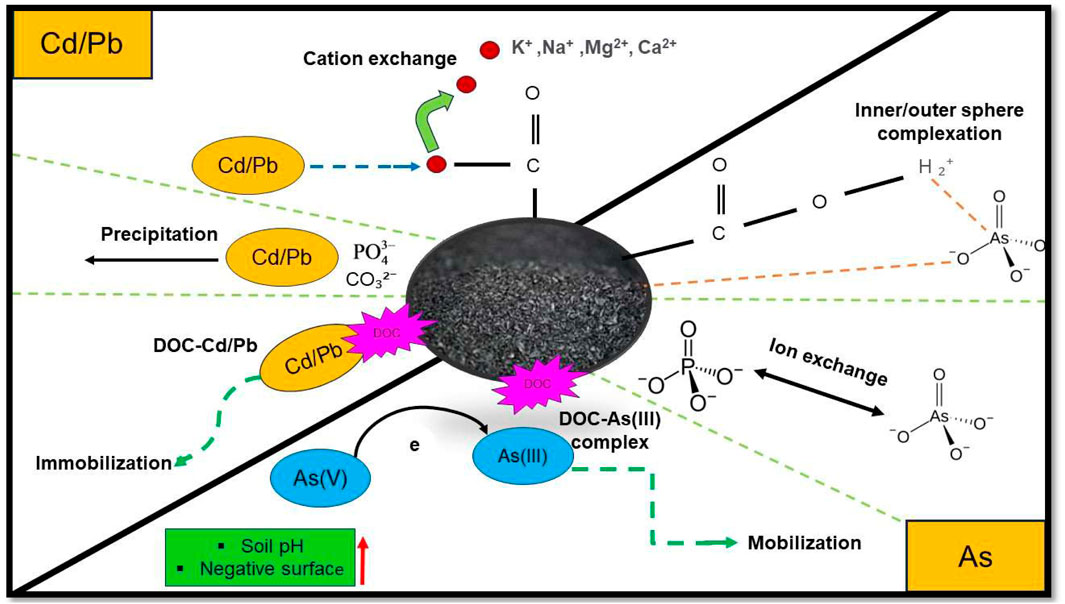
FIGURE 4. The sorption of Cd, Pb, and As by biochars in soil. The results are taken from Shaheen et al. (2023).
Biochar treatment efficiency in the phytoremediation process is also influenced by several factors, such as pyrolysis temperature, heating rate, raw materials, aging, and particle size (Lu et al., 2014; Gasco et al., 2016; Rathnayake et al., 2021) (Figure 5). Although the addition of biochar improves the absorption and stabilization of various pollutants, in general, the temperature of the pyrolysis for the preparation of biochar plays an effective role in improving phytoremediation in addition to soil type, plant type, and target pollutant (Cárdenas-Aguiar et al., 2020). Biochar obtained from biomass at high temperatures is more stable in the environment and can be used for carbon sequestration (Gasco et al., 2016), while biochar made at low temperatures and from raw materials high in nutrients (such as sewage sludge or manure waste) is good for soil improvement (CEC and water retention capacity) and can be used to replace commercial fertilizers in soils contaminated with HMs (Cárdenas-Aguiar et al., 2020). Due to the risk of the re-release of pollutants stabilized by biochar over time (Gu et al., 2020), researchers have been exploring whether the aging of biochar increases the release of pollutants. Based on the limited research that has been done on biochar aging, it has been shown that during the aging process, a wide range of functional groups, including carboxylic, hydroxyl, and phenolic, are formed on the surface of biochar (Narayanan and Ma, 2022), and the aging process does not affect the ability of biochar to stabilize HMs, especially those with a positive charge (Ghosh and Maiti, 2021; Narayanan and Ma, 2022), In studies, aged biochar produced at high pyrolysis temperatures more effectively stabilized pollutants in contaminated soils than aged biochar produced at lower pyrolysis temperatures (Rathnayake et al., 2021), however, this process needs more studies.
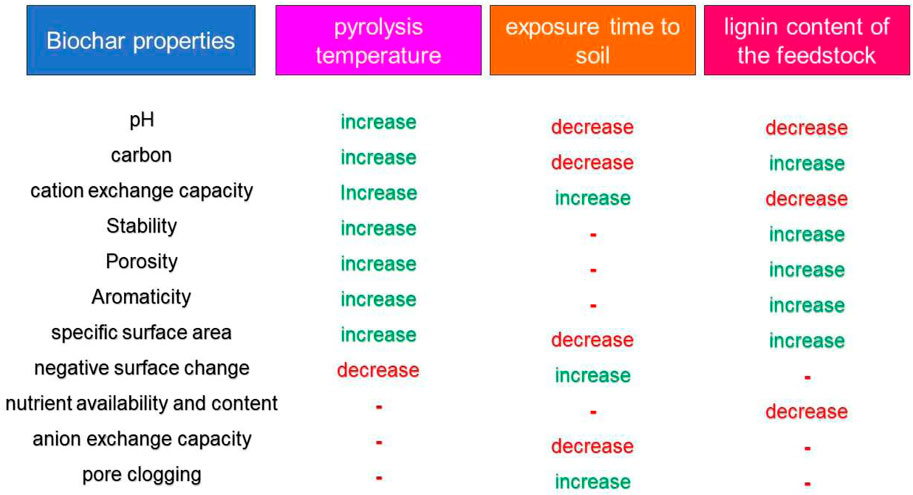
FIGURE 5. The effects of an increase in pyrolysis temperature, soil exposure time, and lignin content of the feedstock on the properties of biochar. The results are taken from Beusch (2021).
On the other hand, studies conducted on the effect of biochar particle size on phytoremediation efficiency have indicated that biochar particle size plays a role in plants’ response to polluted culture environments (Lebrun et al., 2021b). Biochar particle size, which is divided into large (>4 mm) and small (<20 µm) particles, is not expected to play a significant role in nutrient uptake, but it can alter biochar surface properties that affect contaminant stabilization (Lebrun et al., 2021a; Lebrun et al., 2021b). There have been limited studies on the effect of biochar particle size on the absorption and stabilization of HMs (Zhang et al., 2017; Li et al., 2021a; Lebrun et al., 2021b). In their study of S. psammophila treated with different particle sizes of bamboo biochar (P1<0.15 mm, 0.15 mm < P2 < 0.25 mm and 0.25 mm < P3 < 0.50 mm), Li et al. (2021a) found that treatment P2 had the greatest effect on Cd and Zn accumulation as well as plant growth. Furthermore, Zhang et al. (2017) showed that small particles of corn biochar increased Cd transfer from roots to shoots of Brassica chinensis L. Studies have also shown that treating rice and S. plumbizincicola with small particle size biochar reduces Zn concentrations in their aerial organs (Zheng et al., 2012; Lu et al., 2014).
The researchers found that biochar increases the activity and population of microbes (fungi and bacteria) in HMs-contaminated environments, whether there are plants present or not (Karppinen et al., 2017; Zhang et al., 2018; Gong et al., 2019). Additionally, biochar reduces the bioavailability of pollutants in soils by improving the physical and chemical properties of contaminated soils and creating conducive conditions for soil microorganisms, thus increasing the efficiency of phytoremediation in contaminated environments (Liu et al., 2017a; Kiran and Prasad, 2019; Arif et al., 2020). According to a study conducted on the effect of wheat straw biochar on cadmium remediation by M. sativa L, the addition of biochar improved soil enzyme productions (chitinase and protease), and fungal community growth (Zhang et al., 2018). In Gong et al. (2019)’s study on the effect of biochar derived from tea waste in reducing the toxicity caused by Cd in polluted sediments, it was shown that biochar improved the activity of microbes producing catalase, phosphatase, and urease and decreased the Cd toxicity to ramie and microbes. Mackie et al. (2015) also found in their study in copper-contaminated vineyards that biochar increases the activity and abundance of microbes and their ecosystem services. In another study, it was found that biochar derived from sugarcane bagasse, in addition to increasing the population of Actinomycetales and bacteria, increased the activity of soil enzymes in soils contaminated with Pb, Cu, and Cd (Nie et al., 2018) (Figure 3).
6 Phytoremediation with biochar in combination with other factors
Using biochar simultaneously with other compounds and amendment materials, in addition to overlapping their defects, enhances phytoremediation and pollution stabilization and removal (Forján et al., 2018; Gong et al., 2021), therefore, researchers have been focusing on how biochar can be combined with nanoparticles, compost, lime, sulfur, metal oxides, etc., to improve phytoremediation in recent decades (Xu et al., 2018a; Ding et al., 2020; Roohi et al., 2020; Gogoi et al., 2021; Narayanan and Ma, 2022). In a study conducted on B. juncea for lead extraction, it was found that the simultaneous application of Neem tree wood logs biochar and EDTA significantly increased the biomass and total chlorophyll content of plants and increased lead absorption by the seedlings compared to the application of each treatment separately (Rathika et al., 2021). Several studies have shown that the combination of iron sulfate and biochar can be an effective strategy for increasing the stabilization and reducing the bioavailability of As in polluted environments through absorption or surface precipitation mechanisms (Hartley and Lepp, 2008; Lebrun et al., 2020; Simiele et al., 2020), and besides improving soil fertility, this combination can also boost plant growth and survival (Hartley et al., 2009; Simiele et al., 2020).
A study conducted by Li et al. (2019b) on S. nigrum L. treated with biochar and attapulgite showed that the simultaneous application of these treatments improved the fresh weight and length of the seedlings, while also increasing the absorption of Zn, Mn, Hg, Cu, Cd, and Pb in the plant roots, which demonstrates the positive effect of this compound in improving the stabilization of HMs. Furthermore, adding lime and sulfur to biochar can reduce HM bioavailability and improve plant growth in the process of phytoremediation (Xu et al., 2018a; Narayanan and Ma, 2022). Studies have also been conducted on the effectiveness of compost and biochar combination in phytoremediation of soil contaminated with HMs (Roohi et al., 2020; Deebika et al., 2021), some of which are listed below. In a study that was conducted to evaluate the potential of B. tomentellus for phytoremediation of soils contaminated with Cr and Zn with the help of biochar treatment and urban waste compost, it was shown that by combining these treatments, HMs absorption by seedlings significantly increased (Roohi et al., 2020). In addition, Forján et al. (2018) showed that B. juncea L. + compost + biochar is the best combination for reducing Zn, Pb, Ni, and Cu concentrations in polluted soils. In another study, Liu et al. (2017b) found that the combination of compost and biochar reduces the bioavailability of HMs such as Cd, Cr, Zn, As, Cu, Ni, and Pb due to the higher levels of extractable minerals (such as nitrogen, phosphorus, calcium, magnesium, and potassium).
In a study that was conducted to evaluate the effectiveness of rice husk ash and Prosopis biochar in phytoremediation of Pb by Ricinus communis, it was demonstrated that the simultaneous use of these treatments improves soil pH, seedling tolerance to Pb, antioxidant activity, and nutrient uptake, while it reduced the availability of Pb in the soil, and a detailed analysis of the infrared spectra confirmed that oxygenated (ester and ether), amide and phosphate functional groups absorbed Pb and reduced its toxicity for plants (Kiran and Prasad, 2019). Similarly, a study conducted with rice straw and bamboo biochar found that the combination of these treatments reduced the extractable concentration of Zn, Pb, Cu, and Cd in contaminated soils (Lu et al., 2017). A positive effect on plant growth factors was observed following the addition of the above-mentioned treatments to the cultivation environment due to the improvement of physical and biological properties and the enhancement of soil nutrients (Isitekhale et al., 2013; Barati et al., 2018).
It has also been shown that the simultaneous treatment of microbes and biochar can improve the phytoremediation of HMs and increase the resistance of plants to these pollutants (Farias et al., 2020). Alternatively, heavy metal toxicity could affect the microorganisms that are added to soil environments to improve phytoremediation, and their efficiency may decrease (Dzionek et al., 2016; Yao et al., 2017). Thus, cell stabilization is an effective way of increasing microorganism resistance to pollution and restoring native soil microflora, and biochar, as a good carrier material for cell stabilization, can increase microorganisms’ resistance to pollutants’ toxicity (Dzionek et al., 2016; Chuaphasuk and Prapagdee, 2019), and It is clear that, in this case, a more in-depth research is required. In an experiment conducted on Plant extraction of Cd by Chlorophytum laxum R.Br, it was shown that bacteria (Arthrobacter sp. and Micrococcus sp.) stabilized with biochar increased the accumulation of Cd in the roots and shoots of seedlings and the extraction of Cd from contaminated soils (Chuaphasuk and Prapagdee, 2019). Another study conducted on Portulaca oleracea L. under Zn, Al, Cr (III), Cr (VI), and Fe stress demonstrated that by using biochar combined with Oscillatoria sp, in addition to increasing root and shoot lengths, organic carbon, nitrogen content, chlorophyll content, and stabilizing HMs in the soil, the accumulation of HMs in plant organs decreased (Zanganeh et al., 2022). In a study on the role of Jacaranda mimosifolia in the phytoremediation of Zn, Mn, and Cu under the treatment of biochar and spore suspension of five fungal isolates (Metarhizium anisopliae, Trichoderma asperella, Beauveria bassiana, Purpureocillium lilacinum, and Pochonia chlamydosporia), it was found that the extraction rate of HMs and their concentration in plant tissues increased noticeably, reducing the risk of HMs leaching in the soil (Farias et al., 2020) (Figure 6).
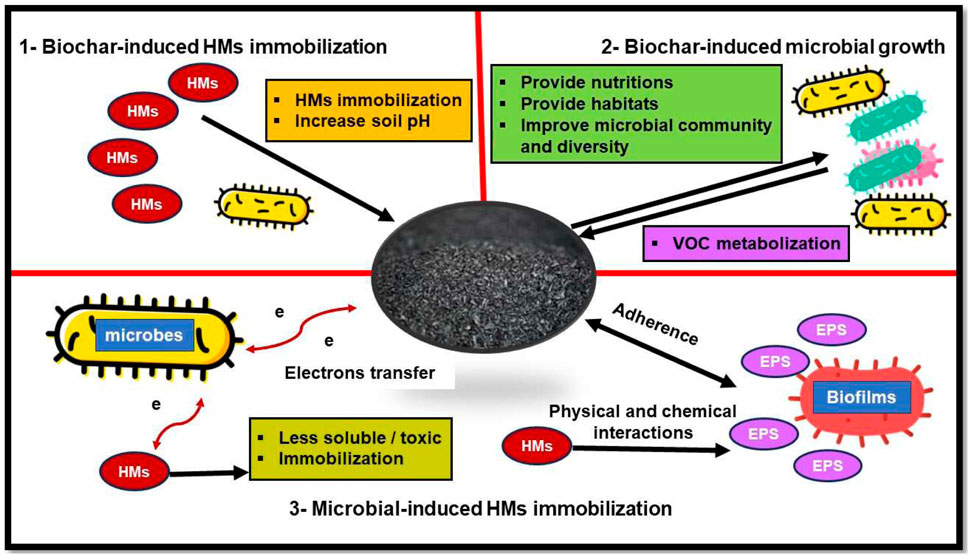
FIGURE 6. Mechanisms of immobilization of metal(iod)s (HMs) by soil microorganisms in biocharamended soil; EPS (Extracellular polymeric substances). The results are taken from Bandara et al. (2020).
7 Pyrolysis of phytoremediation residues
As phytoremediation plays an increasingly important role in cleaning polluted environments, disposing of plants used in this process has become an urgent issue (Gong et al., 2018b; Zhou et al., 2020). If contaminated biomass is returned directly to the soil after phytoremediation, it can cause secondary pollution in the soil and underground water, which can have irreparable effects on the ecosystem, animals, and humans (Huang et al., 2015; Özkan et al., 2016; Gong et al., 2017). Therefore, in recent years, several solutions have been proposed for recycling plants grown in polluted environments, including making furniture, burning, and composting, though each mentioned method has obvious disadvantages (Chalot et al., 2012; Li et al., 2020a; Ghosh and Maiti, 2021). For example, only wooden plants can be used to make household furniture, while herbaceous plants resulting from the phytoremediation process are not useful (Ghosh and Maiti, 2021); On the other hand, burning or composting contaminated biomass can release pollutants into the air, water, and soil (Zhang et al., 2021).
One of the best ways to utilize phytoremediation residues and produce biochar is through pyrolysis, which offers many advantages such as reducing contaminated biomass volume and mass, stabilizing (reducing bioavailability) and decomposing organic and inorganic pollutants, producing valuable pyrolytic products (such as coal, biofuel, etc.), and improving soil quality (carbon sequestration, stabilization of metals in polluted soil and increasing soil fertility) (Lievens et al., 2008; Xiao et al., 2015; Bert et al., 2017; Niu et al., 2017; Du et al., 2019b; Palansooriya et al., 2020; Zhang et al., 2020). A key factor in producing biochar from phytoremediation residues is the pyrolysis temperature, which determines the distribution of heavy metals in biochar (Huang et al., 2018). For instance, Cd evaporates at a temperature above 600°C, while Hg completely evaporates at 350°C (Gong et al., 2018a). Compared to high pyrolysis temperatures, low pyrolysis temperatures increase the accumulation of heavy metals and humic substances in biochar, while reducing the amount of low molecular weight acids (Bian et al., 2018). On the other hand, HMs species present in phytoremediation plants can influence the properties of biochar, for instance, Fe can enhance the graphicization of biochar, while Zn can increase the porosity of biochar (Ding et al., 2020).
In their studies on S. officinale L. used in phytoremediation, Du et al. (2019b) found that pyrolysis temperatures above 550°C stabilize HMs and greatly reduce their leaching into biochar, and improve biochar environmental safety. In another study by Zhang et al. (2020) on pyrolysis of Lolium perenne L., Pennisetum sinese, and Brassica napus L. residues obtained from phytoremediation, they found that a temperature higher than 600°C makes the Cd toxicity in biochar acceptable to the environment and its leaching rate in biochar is reduced to an acceptable level. A study on Silphium perfoliatum L. obtained from a phytoremediation process and containing HMs found that pyrolysis at 750°C reduces the long-term leaching of HMs, increases oxidation resistance, and prevents the release of HMs attached to organic materials (Du et al., 2019a). The study by Zhou et al. (2020) found that biochar obtained from Helianthus annuus L. containing Cd, Zn, Pb, and Cu can be used as a fertilizer to improve soil quality. Researchers found that converting cobalt-containing Siberian iris into biochar through one-step pyrolysis improved mercury phytoremediation of polluted soils and waters (Li et al., 2020b). The results of another study conducted on ramie after the phytoremediation process showed that pyrolysis can enhance the stabilization of Pb, Cu, Zn, Cd, and Cr in plant residues and increase the possibility of reusing phytoremediation residues (Gong et al., 2018b). Moreover, Zhang et al. (2021) found that biochar produced from cadmium-contaminated L. perenne L. and B. napus L. residues at high pyrolysis temperatures, due to the increase in Cd stabilization in biochar, had a greatly decreased risk of environmental contamination. However, to demonstrate the effectiveness of phytoremediation residues as biochar, more studies are necessary about environmental toxicity, washability, stability, environmental hazards, and its chemical forms (Han et al., 2018; Du et al., 2019a).
8 Conclusion
The results of the present study demonstrated that using biochar in the phytoremediation process can improve the extraction and stabilization of HMs pollution, as well as increase plant resistance under stress conditions. On the other hand, owing to the variety of pollutions, plants suitable for phytoremediation, biochars, and other factors that enhance the phytoremediation process, the positive effects of different types of biochar on reducing all pollution in the environment cannot be mentioned with absolute certainty. Therefore, in future studies, a complex investigation of the interplay between different factors (biochar, plants, pollutants, etc.) is required to improve the efficiency of phytoremediation. It is also suggested that future studies should focus on field experiments so that by obtaining more practical information, an effective step can be taken toward reducing pollution and creating a healthier environment for future generations.
Author contributions
MB and LL wrote and edited the manuscript. All authors contributed to the article and approved the submitted version.
Funding
This paper was funded by the project: VEGA 1/0186/23 Windbreaks in the agricultural landscape—ecological, environmental, and economic value of multifunctional structures acting as soil degradation measures.
Conflict of interest
The authors declare that the research was conducted in the absence of any commercial or financial relationships that could be construed as a potential conflict of interest.
Publisher’s note
All claims expressed in this article are solely those of the authors and do not necessarily represent those of their affiliated organizations, or those of the publisher, the editors and the reviewers. Any product that may be evaluated in this article, or claim that may be made by its manufacturer, is not guaranteed or endorsed by the publisher.
References
Abdelaal, A., Pradhan, S., Alnouss, A., Tong, Y., Al-Ansari, T., Mckay, G., et al. (2021). The impact of pyrolysis conditions on orange peel biochar physicochemical properties for sandy soil. Waste Manag. Res. 39, 995–1004. doi:10.1177/0734242x20978456
Acosta, J., Abbaspour, A., Martínez, G., Martínez-Martínez, S., Zornoza, R., Gabarrón, M., et al. (2018). Phytoremediation of mine tailings with Atriplex halimus and organic/inorganic amendments: a five-year field case study. Chemosphere 204, 71–78. doi:10.1016/j.chemosphere.2018.04.027
Afzal, M. J., Khan, M. I., Cheema, S. A., Hussain, S., Anwar-Ul-Haq, M., Ali, M. H., et al. (2020). Combined application of Bacillus sp. MN-54 and phosphorus improved growth and reduced lead uptake by maize in the lead-contaminated soil. Environ. Sci. Pollut. Res. 27, 44528–44539. doi:10.1007/s11356-020-10372-4
Agegnehu, G., Bass, A. M., Nelson, P. N., and Bird, M. I. (2016). Benefits of biochar, compost and biochar–compost for soil quality, maize yield and greenhouse gas emissions in a tropical agricultural soil. Sci. Total Environ. 543, 295–306. doi:10.1016/j.scitotenv.2015.11.054
Agrafioti, E., Kalderis, D., and Diamadopoulos, E. (2014). Ca and Fe modified biochars as adsorbents of arsenic and chromium in aqueous solutions. J. Environ. Manag. 146, 444–450. doi:10.1016/j.jenvman.2014.07.029
Ahmad, M., Rajapaksha, A. U., Lim, J. E., Zhang, M., Bolan, N., Mohan, D., et al. (2014). Biochar as a sorbent for contaminant management in soil and water: a review. Chemosphere 99, 19–33. doi:10.1016/j.chemosphere.2013.10.071
Al-Wabel, M. I., Usman, A. R., El-Naggar, A. H., Aly, A. A., Ibrahim, H. M., Elmaghraby, S., et al. (2015). Conocarpus biochar as a soil amendment for reducing heavy metal availability and uptake by maize plants. Saudi J. Biol. Sci. 22, 503–511. doi:10.1016/j.sjbs.2014.12.003
Albalasmeh, A., Gharaibeh, M. A., Mohawesh, O., Alajlouni, M., Quzaih, M., Masad, M., et al. (2020). Characterization and Artificial Neural Networks Modelling of methylene blue adsorption of biochar derived from agricultural residues: effect of biomass type, pyrolysis temperature, particle size. J. Saudi Chem. Soc. 24, 811–823. doi:10.1016/j.jscs.2020.07.005
Ali, H., Khan, E., and Sajad, M. A. (2013). Phytoremediation of heavy metals—concepts and applications. Chemosphere 91, 869–881. doi:10.1016/j.chemosphere.2013.01.075
Ali, M. H., Khan, M. I., Bashir, S., Azam, M., Naveed, M., Qadri, R., et al. (2021). Biochar and Bacillus sp. MN54 assisted phytoremediation of diesel and plant growth promotion of maize in hydrocarbons contaminated soil. Agronomy 11, 1795. doi:10.3390/agronomy11091795
APHA (2003). Standard methods for the examination of water and waste water for the examination of water and wastewater “Part 5210B: 5-day Biochemical Oxygen Demand. Berlin, Germany: Springer.
Arif, I., Batool, M., and Schenk, P. M. (2020). Plant microbiome engineering: expected benefits for improved crop growth and resilience. Trends Biotechnol. 38, 1385–1396. doi:10.1016/j.tibtech.2020.04.015
Awad, M., Liu, Z., Skalicky, M., Dessoky, E. S., Brestic, M., Mbarki, S., et al. (2021). Fractionation of heavy metals in multi-contaminated soil treated with biochar using the sequential extraction procedure. Biomolecules 11, 448. doi:10.3390/biom11030448
Bandara, T., Franks, A., Xu, J., Bolan, N., Wang, H., and Tang, C. (2020). Chemical and biological immobilization mechanisms of potentially toxic elements in biochar-amended soils. Crit. Rev. Environ. Sci. Technol. 50, 903–978. doi:10.1080/10643389.2019.1642832
Bandara, T., Herath, I., Kumarathilaka, P., Seneviratne, M., Seneviratne, G., Rajakaruna, N., et al. (2017). Role of woody biochar and fungal-bacterial co-inoculation on enzyme activity and metal immobilization in serpentine soil. J. Soils Sediments 17, 665–673. doi:10.1007/s11368-015-1243-y
Barati, M., Bakhtiari, F., Mowla, D., and Safarzadeh, S. (2018). Comparison of the effects of poultry manure and its biochar on barley growth in petroleum-contaminated soils. Int. J. phytoremediation 20, 98–103. doi:10.1080/15226514.2017.1337069
Bashir, S., Shaaban, M., Mehmood, S., Zhu, J., Fu, Q., and Hu, H. (2018). Efficiency of C3 and C4 plant derived-biochar for Cd mobility, nutrient cycling and microbial biomass in contaminated soil. Bull. Environ. Contam. Toxicol. 100, 834–838. doi:10.1007/s00128-018-2332-6
Beesley, L., and Marmiroli, M. (2011). The immobilisation and retention of soluble arsenic, cadmium and zinc by biochar. Environ. Pollut. 159, 474–480. doi:10.1016/j.envpol.2010.10.016
Benavente, I., Gasco, G., Plaza, C., Paz-Ferreiro, J., and Mendez, A. (2018). Choice of pyrolysis parameters for urban wastes affects soil enzymes and plant germination in a Mediterranean soil. Sci. Total Environ. 634, 1308–1314. doi:10.1016/j.scitotenv.2018.04.120
Bert, V., Allemon, J., Sajet, P., Dieu, S., Papin, A., Collet, S., et al. (2017). Torrefaction and pyrolysis of metal-enriched poplars from phytotechnologies: effect of temperature and biomass chlorine content on metal distribution in end-products and valorization options. Biomass bioenergy 96, 1–11. doi:10.1016/j.biombioe.2016.11.003
Beusch, C. (2021). Biochar as a soil ameliorant: how biochar properties benefit soil fertility—a review. J. Geoscience Environ. Prot. 9, 28–46. doi:10.4236/gep.2021.910003
Bhardwaj, R., Sharma, R., Handa, N., Kaur, H., Kaur, R., Sirhindi, G., et al. (2014). Prospects of field crops for phytoremediation of contaminants. Emerging technologies and management of crop stress tolerance. Netherlands: Elsevier.
Bian, R., Li, L., Shi, W., Ma, B., Joseph, S., Li, L., et al. (2018). Pyrolysis of contaminated wheat straw to stabilize toxic metals in biochar but recycle the extract for agricultural use. Biomass Bioenergy 118, 32–39. doi:10.1016/j.biombioe.2018.08.003
Binti Ab Aziz, N. S., Bin Mohd Nor, M. A., and Hamzah, F. (2015). Suitability of biochar produced from biomass waste as soil amendment. Procedia-Social Behav. Sci. 195, 2457–2465. doi:10.1016/j.sbspro.2015.06.288
Boominathan, R., Saha-Chaudhury, N., Sahajwalla, V., and Doran, P. M. (2004). Production of nickel bio-ore from hyperaccumulator plant biomass: applications in phytomining. Biotechnol. Bioeng. 86, 243–250. doi:10.1002/bit.10795
Boorboori, M. R. (2023). Investigating the role of silicon in reducing the risk of arsenic, cadmium, drought and salinity stresses in wheat (Triticum aestivum L). J. Crop Sci. Biotechnol. 26, 387–404. doi:10.1007/s12892-022-00191-z
Boorboori, M. R., and Zhang, H.-Y. (2022a). Arbuscular mycorrhizal fungi are an influential factor in improving the phytoremediation of arsenic, cadmium, lead, and chromium. J. Fungi 8, 176. doi:10.3390/jof8020176
Boorboori, M. R., and Zhang, H.-Y. (2022b). The role of Serendipita indica (Piriformospora indica) in improving plant resistance to drought and salinity stresses. Biology 11, 952. doi:10.3390/biology11070952
Borghi, M., Tognetti, R., Monteforti, G., and Sebastiani, L. (2007). Responses of Populus× euramericana (P. deltoides× P. nigra) clone Adda to increasing copper concentrations. Environ. Exp. Bot. 61, 66–73. doi:10.1016/j.envexpbot.2007.03.001
Brassard, P., Godbout, S., and Raghavan, V. (2017). Pyrolysis in auger reactors for biochar and bio-oil production: a review. Biosyst. Eng. 161, 80–92. doi:10.1016/j.biosystemseng.2017.06.020
Buha, J., Mueller, N., Nowack, B., Ulrich, A., Losert, S., and Wang, J. (2014). Physical and chemical characterization of fly ashes from Swiss waste incineration plants and determination of the ash fraction in the nanometer range. Environ. Sci. Technol. 48, 4765–4773. doi:10.1021/es4047582
Burachevskaya, M., Mandzhieva, S., Bauer, T., Minkina, T., Rajput, V., Chaplygin, V., et al. (2021). The effect of granular activated carbon and biochar on the availability of Cu and Zn to Hordeum sativum Distichum in contaminated soil. Plants 10, 841. doi:10.3390/plants10050841
Byrne, G., Dimitrov, D., Monostori, L., Teti, R., Van Houten, F., and Wertheim, R. (2018). Biologicalisation: biological transformation in manufacturing. CIRP J. Manuf. Sci. Technol. 21, 1–32. doi:10.1016/j.cirpj.2018.03.003
Cárdenas-Aguiar, E., Gasco, G., Paz-Ferreiro, J., and Mendez, A. (2019). Thermogravimetric analysis and carbon stability of chars produced from slow pyrolysis and hydrothermal carbonization of manure waste. J. Anal. Appl. pyrolysis 140, 434–443. doi:10.1016/j.jaap.2019.04.026
Cárdenas-Aguiar, E., Ruiz, B., Fuente, E., Gascó, G., and Méndez, A. (2020). Improving mining soil phytoremediation with Sinapis alba by addition of hydrochars and biochar from manure wastes. Waste Biomass Valorization 11, 5197–5210. doi:10.1007/s12649-020-00999-2
Chalot, M., Blaudez, D., Rogaume, Y., Provent, A.-S., and Pascual, C. (2012). Fate of trace elements during the combustion of phytoremediation wood. Environ. Sci. Technol. 46, 13361–13369. doi:10.1021/es3017478
Chehregani, A., Noori, M., and Yazdi, H. L. (2009). Phytoremediation of heavy-metal-polluted soils: screening for new accumulator plants in Angouran mine (Iran) and evaluation of removal ability. Ecotoxicol. Environ. Saf. 72, 1349–1353. doi:10.1016/j.ecoenv.2009.02.012
Chen, D., Liu, X., Bian, R., Cheng, K., Zhang, X., Zheng, J., et al. (2018). Effects of biochar on availability and plant uptake of heavy metals–A meta-analysis. J. Environ. Manag. 222, 76–85. doi:10.1016/j.jenvman.2018.05.004
Chen, M., Wang, D., Yang, F., Xu, X., Xu, N., and Cao, X. (2017). Transport and retention of biochar nanoparticles in a paddy soil under environmentally-relevant solution chemistry conditions. Environ. Pollut. 230, 540–549. doi:10.1016/j.envpol.2017.06.101
Cheng, S., Chen, T., Xu, W., Huang, J., Jiang, S., and Yan, B. (2020). Application research of biochar for the remediation of soil heavy metals contamination: a review. Molecules 25, 3167. doi:10.3390/molecules25143167
Chuaphasuk, C., and Prapagdee, B. (2019). Effects of biochar-immobilized bacteria on phytoremediation of cadmium-polluted soil. Environ. Sci. Pollut. Res. 26, 23679–23688. doi:10.1007/s11356-019-05661-6
Chukwuka, K. S., Akanmu, A. O., Umukoro, O., Asemoloye, M. D., and Odebode, A. C. (2020). Biochar: a vital source for sustainable agriculture. Biostimulants Plant Sci. 20, 51–74. doi:10.5772/intechopen.86568
Claus, D., Dietze, H., Gerth, A., Grosser, W., and Hebner, A. (2007). Application of agronomic practice improves phytoextraction on a multipolluted site. J. Environ. Eng. Landsc. Manag. 15, 208–212. doi:10.3846/16486897.2007.9636932
Clemente, R., Walker, D. J., Pardo, T., Martínez-Fernández, D., and Bernal, M. P. (2012). The use of a halophytic plant species and organic amendments for the remediation of a trace elements-contaminated soil under semi-arid conditions. J. Hazard. Mater. 223, 63–71. doi:10.1016/j.jhazmat.2012.04.048
Cloutier-Hurteau, B., Turmel, M.-C., Mercier, C., and Courchesne, F. (2014). The sequestration of trace elements by willow (Salix purpurea)—which soil properties favor uptake and accumulation? Environ. Sci. Pollut. Res. 21, 4759–4771. doi:10.1007/s11356-013-2450-y
Cui, J.-Q., He, Q.-S., Liu, M.-H., Chen, H., Sun, M.-B., and Wen, J.-P. (2020). Comparative study on different remediation strategies applied in petroleum-contaminated soils. Int. J. Environ. Res. public health 17, 1606. doi:10.3390/ijerph17051606
Czekała, W., Malińska, K., Cáceres, R., Janczak, D., Dach, J., and Lewicki, A. (2016). Co-composting of poultry manure mixtures amended with biochar–The effect of biochar on temperature and C-CO2 emission. Bioresour. Technol. 200, 921–927. doi:10.1016/j.biortech.2015.11.019
Deebika, P., Merline Sheela, A., and Ilamathi, R. (2021). Biochar and compost-based phytoremediation of crude oil contaminated soil. Indian J. Sci. Technol. 14, 220–228. doi:10.17485/ijst/v14i3.1178
Ding, D., Zhou, L., Kang, F., Yang, S., Chen, R., Cai, T., et al. (2020). Synergistic adsorption and oxidation of ciprofloxacin by biochar derived from metal-enriched phytoremediation plants: experimental and computational insights. ACS Appl. Mater. Interfaces 12, 53788–53798. doi:10.1021/acsami.0c15861
Doan, T. T., Henry-Des-Tureaux, T., Rumpel, C., Janeau, J.-L., and Jouquet, P. (2015). Impact of compost, vermicompost and biochar on soil fertility, maize yield and soil erosion in Northern Vietnam: a three year mesocosm experiment. Sci. Total Environ. 514, 147–154. doi:10.1016/j.scitotenv.2015.02.005
Dong, H., Deng, J., Xie, Y., Zhang, C., Jiang, Z., Cheng, Y., et al. (2017). Stabilization of nanoscale zero-valent iron (nZVI) with modified biochar for Cr (VI) removal from aqueous solution. J. Hazard. Mater. 332, 79–86. doi:10.1016/j.jhazmat.2017.03.002
Du, J., Zhang, L., Ali, A., Li, R., Xiao, R., Guo, D., et al. (2019a). Research on thermal disposal of phytoremediation plant waste: stability of potentially toxic metals (PTMs) and oxidation resistance of biochars. Process Saf. Environ. Prot. 125, 260–268. doi:10.1016/j.psep.2019.03.035
Du, J., Zhang, L., Liu, T., Xiao, R., Li, R., Guo, D., et al. (2019b). Thermal conversion of a promising phytoremediation plant (Symphytum officinale L.) into biochar: dynamic of potentially toxic elements and environmental acceptability assessment of the biochar. Bioresour. Technol. 274, 73–82. doi:10.1016/j.biortech.2018.11.077
Dzionek, A., Wojcieszyńska, D., and Guzik, U. (2016). Natural carriers in bioremediation: a review. Electron. J. Biotechnol. 23, 28–36. doi:10.1016/j.ejbt.2016.07.003
Evlard, A., Sergeant, K., Ferrandis, S., Printz, B., Renaut, J., Guignard, C., et al. (2014). Physiological and proteomic responses of different willow clones (Salix fragilis X alba) exposed to dredged sediment contaminated by heavy metals. Int. J. phytoremediation 16, 1148–1169. doi:10.1080/15226514.2013.821448
Fancello, D., Scalco, J., Medas, D., Rodeghero, E., Martucci, A., Meneghini, C., et al. (2019). XRD-thermal combined analyses: an approach to evaluate the potential of phytoremediation, phytomining, and biochar production. Int. J. Environ. Res. public health 16, 1976. doi:10.3390/ijerph16111976
Farhangi-Abriz, S., and Torabian, S. (2017). Antioxidant enzyme and osmotic adjustment changes in bean seedlings as affected by biochar under salt stress. Ecotoxicol. Environ. Saf. 137, 64–70. doi:10.1016/j.ecoenv.2016.11.029
Farias, C. P., Alves, G. S., Oliveira, D. C., De Melo, E. I., and Azevedo, L. C. B. (2020). A consortium of fungal isolates and biochar improved the phytoremediation potential of Jacaranda mimosifolia D. Don and reduced copper, manganese, and zinc leaching. J. Soils Sediments 20, 260–271. doi:10.1007/s11368-019-02414-3
Farooq, M. A., Islam, F., Ali, B., Najeeb, U., Mao, B., Gill, R. A., et al. (2016). Arsenic toxicity in plants: cellular and molecular mechanisms of its transport and metabolism. Environ. Exp. Bot. 132, 42–52. doi:10.1016/j.envexpbot.2016.08.004
Fatima, K., Imran, A., Amin, I., Khan, Q. M., and Afzal, M. (2018). Successful phytoremediation of crude-oil contaminated soil at an oil exploration and production company by plants-bacterial synergism. Int. J. phytoremediation 20, 675–681. doi:10.1080/15226514.2017.1413331
Fellet, G., Marmiroli, M., and Marchiol, L. (2014). Elements uptake by metal accumulator species grown on mine tailings amended with three types of biochar. Sci. Total Environ. 468, 598–608. doi:10.1016/j.scitotenv.2013.08.072
Ferjani, A. I., Jeguirim, M., Jellali, S., Limousy, L., Courson, C., Akrout, H., et al. (2019). The use of exhausted grape marc to produce biofuels and biofertilizers: effect of pyrolysis temperatures on biochars properties. Renew. Sustain. Energy Rev. 107, 425–433. doi:10.1016/j.rser.2019.03.034
Fernández-Braña, A., Salgado, L., Gallego, J. L. R., Afif, E., Boente, C., and Forján, R. (2023). Phytoremediation potential depends on the degree of soil pollution: a case study in an urban brownfield. Environ. Sci. Pollut. Res. 30, 67708–67719. doi:10.1007/s11356-023-26968-5
Fijałkowska, G., Wiśniewska, M., Szewczuk-Karpisz, K., Jędruchniewicz, K., and Oleszczuk, P. (2021). Comparison of lead (II) ions accumulation and bioavailability on the montmorillonite and kaolinite surfaces in the presence of polyacrylamide soil flocculant. Chemosphere 276, 130088. doi:10.1016/j.chemosphere.2021.130088
Forján, R., Rodríguez-Vila, A., Cerqueira, B., and Covelo, E. (2018). Comparison of compost with biochar versus technosol with biochar in the reduction of metal pore water concentrations in a mine soil. J. Geochem. Explor. 192, 103–111. doi:10.1016/j.gexplo.2018.06.007
Gao, L., Deng, J., Tang, G., Huang, X., Cai, K., Cai, Y., et al. (2018). Adsorption characteristics and mechanism of Cd2+ on biochar with different pyrolysis temperatures produced from eucalyptus leaves. China Environ. Sci. 38, 1001–1009.
Gasco, G., Paz-Ferreiro, J., Cely, P., Plaza, C., and Mendez, A. (2016). Influence of pig manure and its biochar on soil CO2 emissions and soil enzymes. Ecol. Eng. 95, 19–24. doi:10.1016/j.ecoleng.2016.06.039
Gholizadeh, M., and Hu, X. (2021). Removal of heavy metals from soil with biochar composite: a critical review of the mechanism. J. Environ. Chem. Eng. 9, 105830. doi:10.1016/j.jece.2021.105830
Ghosh, D., and Maiti, S. K. (2021). Biochar assisted phytoremediation and biomass disposal in heavy metal contaminated mine soils: a review. Int. J. phytoremediation 23, 559–576. doi:10.1080/15226514.2020.1840510
Gkorezis, P., Daghio, M., Franzetti, A., Van Hamme, J. D., Sillen, W., and Vangronsveld, J. (2016). The interaction between plants and bacteria in the remediation of petroleum hydrocarbons: an environmental perspective. Front. Microbiol. 7, 1836. doi:10.3389/fmicb.2016.01836
Gogoi, L., Narzari, R., Chutia, R. S., Borkotoki, B., Gogoi, N., and Kataki, R. (2021). “Remediation of heavy metal contaminated soil: role of biochar,” in Advances in chemical pollution, environmental management and protection (Netherlands: Elsevier).
Gong, X., Huang, D., Liu, Y., Peng, Z., Zeng, G., Xu, P., et al. (2018a). Remediation of contaminated soils by biotechnology with nanomaterials: bio-behavior, applications, and perspectives. Crit. Rev. Biotechnol. 38, 455–468. doi:10.1080/07388551.2017.1368446
Gong, X., Huang, D., Liu, Y., Zeng, G., Chen, S., Wang, R., et al. (2019). Biochar facilitated the phytoremediation of cadmium contaminated sediments: metal behavior, plant toxicity, and microbial activity. Sci. Total Environ. 666, 1126–1133. doi:10.1016/j.scitotenv.2019.02.215
Gong, X., Huang, D., Liu, Y., Zeng, G., Wang, R., Wan, J., et al. (2017). Stabilized nanoscale zerovalent iron mediated cadmium accumulation and oxidative damage of Boehmeria nivea (L.) Gaudich cultivated in cadmium contaminated sediments. Environ. Sci. Technol. 51, 11308–11316. doi:10.1021/acs.est.7b03164
Gong, X., Huang, D., Liu, Y., Zeng, G., Wang, R., Wei, J., et al. (2018b). Pyrolysis and reutilization of plant residues after phytoremediation of heavy metals contaminated sediments: for heavy metals stabilization and dye adsorption. Bioresour. Technol. 253, 64–71. doi:10.1016/j.biortech.2018.01.018
Gong, X., Huang, D., Liu, Y., Zou, D., Hu, X., Zhou, L., et al. (2021). Nanoscale zerovalent iron, carbon nanotubes and biochar facilitated the phytoremediation of cadmium contaminated sediments by changing cadmium fractions, sediments properties and bacterial community structure. Ecotoxicol. Environ. Saf. 208, 111510. doi:10.1016/j.ecoenv.2020.111510
Gonzaga, M. I. S., De Jesus Santos, J. C., Ganassali Junior, L. F., Fontes, P. T. N., Araujo, J. D. S., and Gonzaga, T. A. S. (2022). Copper uptake, physiological response, and phytoremediation potential of Brassica juncea under biochar application. Int. J. Phytoremediation 24, 474–482. doi:10.1080/15226514.2021.1954875
Gu, P., Zhang, Y., Xie, H., Wei, J., Zhang, X., Huang, X., et al. (2020). Effect of cornstalk biochar on phytoremediation of Cd-contaminated soil by Beta vulgaris var. cicla L. Ecotoxicol. Environ. Saf. 205, 111144. doi:10.1016/j.ecoenv.2020.111144
Gul, S., Whalen, J. K., Thomas, B. W., Sachdeva, V., and Deng, H. (2015). Physico-chemical properties and microbial responses in biochar-amended soils: mechanisms and future directions. Agric. Ecosyst. Environ. 206, 46–59. doi:10.1016/j.agee.2015.03.015
Gupta, A., Sharma, V., Sharma, K., Kumar, V., Choudhary, S., Mankotia, P., et al. (2021). A review of adsorbents for heavy metal decontamination: growing approach to wastewater treatment. Materials 14, 4702. doi:10.3390/ma14164702
Gusmini, G., Adrinal, A., Putri, E., Panji, R., and Husna, F. (2021). Phytoremediation agents of rice biochar and cage fertilizer in ex-gold mining and the sunflower growth. IOP Conf. Ser. Earth Environ. Sci. 741, 012034. doi:10.1088/1755-1315/741/1/012034
Haider, F. U., Virk, A. L., Rehmani, M. I. A., Skalicky, M., Ata-Ul-Karim, S. T., Ahmad, N., et al. (2022). Integrated application of thiourea and biochar improves maize growth, antioxidant activity and reduces cadmium bioavailability in cadmium-contaminated soil. Front. Plant Sci. 12, 809322. doi:10.3389/fpls.2021.809322
Han, Z., Guo, Z., Zhang, Y., Xiao, X., Xu, Z., and Sun, Y. (2018). Adsorption-pyrolysis technology for recovering heavy metals in solution using contaminated biomass phytoremediation. Resour. Conservation Recycl. 129, 20–26. doi:10.1016/j.resconrec.2017.10.003
Hartley, W., Dickinson, N. M., Riby, P., and Lepp, N. W. (2009). Arsenic mobility in brownfield soils amended with green waste compost or biochar and planted with Miscanthus. Environ. Pollut. 157, 2654–2662. doi:10.1016/j.envpol.2009.05.011
Hartley, W., and Lepp, N. W. (2008). Remediation of arsenic contaminated soils by iron-oxide application, evaluated in terms of plant productivity, arsenic and phytotoxic metal uptake. Sci. Total Environ. 390, 35–44. doi:10.1016/j.scitotenv.2007.09.021
Herman, W., and Resigia, E. (2018). Pemanfaatan biochar sekam dan kompos jerami padi terhadap pertumbuhan dan produksi padi (Oryza sativa) pada tanah ordo Ultisol. J. Ilm. Pertan. 15, 42–50. doi:10.31849/jip.v15i1.1487
Hossain, M. K., Strezov, V., Chan, K. Y., and Nelson, P. F. (2010). Agronomic properties of wastewater sludge biochar and bioavailability of metals in production of cherry tomato (Lycopersicon esculentum). Chemosphere 78, 1167–1171. doi:10.1016/j.chemosphere.2010.01.009
Houben, D., Couder, E., and Sonnet, P. (2013). Leachability of cadmium, lead, and zinc in a long-term spontaneously revegetated slag heap: implications for phytostabilization. J. Soils Sediments 13, 543–554. doi:10.1007/s11368-012-0546-5
Huang, D.-L., Wang, R.-Z., Liu, Y.-G., Zeng, G.-M., Lai, C., Xu, P., et al. (2015). Application of molecularly imprinted polymers in wastewater treatment: a review. Environ. Sci. Pollut. Res. 22, 963–977. doi:10.1007/s11356-014-3599-8
Huang, D., Hu, Z., Peng, Z., Zeng, G., Chen, G., Zhang, C., et al. (2018). Cadmium immobilization in river sediment using stabilized nanoscale zero-valent iron with enhanced transport by polysaccharide coating. J. Environ. Manag. 210, 191–200. doi:10.1016/j.jenvman.2018.01.001
Huang, L., Gao, X., Liu, M., Du, G., Guo, J., and Ntakirutimana, T. (2012). Correlation among soil microorganisms, soil enzyme activities, and removal rates of pollutants in three constructed wetlands purifying micro-polluted river water. Ecol. Eng. 46, 98–106. doi:10.1016/j.ecoleng.2012.06.004
Isitekhale, H., Osemwota, I., and Amhakhian, S. (2013). Poultry manure and NPK fertilizer application and their residual effects on the yield and yield components of tomato (Lycopersiconesculentum. Mill) in two distinct ecological zones of central southern Nigeria. J. Agric. Veterinary Sci. 3, 40–47. doi:10.9790/2380-0324047
Jha, A. B., Misra, A. N., and Sharma, P. (2017). Phytoremediation of heavy metal-contaminated soil using bioenergy crops. Phytoremediation potential bioenergy plants 17, 63–96. doi:10.1007/978-981-10-3084-0_3
Jones, S., Bardos, R. P., Kidd, P. S., Mench, M., De Leij, F., Hutchings, T., et al. (2016). Biochar and compost amendments enhance copper immobilisation and support plant growth in contaminated soils. J. Environ. Manag. 171, 101–112. doi:10.1016/j.jenvman.2016.01.024
Kanwar, V. S., Sharma, A., Srivastav, A. L., and Rani, L. (2020). Phytoremediation of toxic metals present in soil and water environment: a critical review. Environ. Sci. Pollut. Res. 27, 44835–44860. doi:10.1007/s11356-020-10713-3
Karppinen, E. M., Stewart, K. J., Farrell, R. E., and Siciliano, S. D. (2017). Petroleum hydrocarbon remediation in frozen soil using a meat and bonemeal biochar plus fertilizer. Chemosphere 173, 330–339. doi:10.1016/j.chemosphere.2017.01.016
Kayser, A., Wenger, K., Keller, A., Attinger, W., Felix, H., Gupta, S., et al. (2000). Enhancement of phytoextraction of Zn, Cd, and Cu from calcareous soil: the use of NTA and sulfur amendments. Environ. Sci. Technol. 34, 1778–1783. doi:10.1021/es990697s
Khan, M. A. I., Biswas, B., Smith, E., Naidu, R., and Megharaj, M. (2018). Toxicity assessment of fresh and weathered petroleum hydrocarbons in contaminated soil-a review. Chemosphere 212, 755–767. doi:10.1016/j.chemosphere.2018.08.094
Khan, S., Chao, C., Waqas, M., Arp, H. P. H., and Zhu, Y.-G. (2013). Sewage sludge biochar influence upon rice (Oryza sativa L) yield, metal bioaccumulation and greenhouse gas emissions from acidic paddy soil. Environ. Sci. Technol. 47, 8624–8632. doi:10.1021/es400554x
Khiari, B., Jeguirim, M., Limousy, L., and Bennici, S. (2019). Biomass derived chars for energy applications. Renew. Sustain. Energy Rev. 108, 253–273. doi:10.1016/j.rser.2019.03.057
Kim, E., Jung, C., Han, J., Her, N., Park, C. M., Jang, M., et al. (2016). Sorptive removal of selected emerging contaminants using biochar in aqueous solution. J. Industrial Eng. Chem. 36, 364–371. doi:10.1016/j.jiec.2016.03.004
Kiran, B. R., and Prasad, M. (2019). Biochar and rice husk ash assisted phytoremediation potentials of Ricinus communis L. for lead-spiked soils. Ecotoxicol. Environ. Saf. 183, 109574. doi:10.1016/j.ecoenv.2019.109574
Kolton, M., Graber, E. R., Tsehansky, L., Elad, Y., and Cytryn, E. (2017). Biochar-stimulated plant performance is strongly linked to microbial diversity and metabolic potential in the rhizosphere. New Phytol. 213, 1393–1404. doi:10.1111/nph.14253
Koul, B., and Taak, P. (2018). Biotechnological strategies for effective remediation of polluted soils. Berlin, Germany: Springer.
Kumar, N. S., Shaikh, H. M., Asif, M., and Al-Ghurabi, E. H. (2021). Engineered biochar from wood apple shell waste for high-efficient removal of toxic phenolic compounds in wastewater. Sci. Rep. 11, 2586. doi:10.1038/s41598-021-82277-2
Kumpiene, J., Lagerkvist, A., and Maurice, C. (2008). Stabilization of As, Cr, Cu, Pb and Zn in soil using amendments–a review. Waste Manag. 28, 215–225. doi:10.1016/j.wasman.2006.12.012
Kupryianchyk, D., Hale, S. E., Breedveld, G. D., and Cornelissen, G. (2016). Treatment of sites contaminated with perfluorinated compounds using biochar amendment. Chemosphere 142, 35–40. doi:10.1016/j.chemosphere.2015.04.085
Laghlimi, M., Baghdad, B., El Hadi, H., and Bouabdli, A. (2015). Phytoremediation mechanisms of heavy metal contaminated soils: a review. Open J. Ecol. 5, 375–388. doi:10.4236/oje.2015.58031
Lebrun, M., Bourgerie, S., and Morabito, D. (2021a). The potential of clover green amendment, associated with biochar, activated carbon or ochre, for the phytoremediation, using Populus x. canescens, of a former mine technosol. Plants 10, 1374. doi:10.3390/plants10071374
Lebrun, M., De Zio, E., Miard, F., Scippa, G. S., Renzone, G., Scaloni, A., et al. (2020). Amending an As/Pb contaminated soil with biochar, compost and iron grit: effect on Salix viminalis growth, root proteome profiles and metal (loid) accumulation indexes. Chemosphere 244, 125397. doi:10.1016/j.chemosphere.2019.125397
Lebrun, M., Macri, C., Miard, F., Hattab-Hambli, N., Motelica-Heino, M., Morabito, D., et al. (2017). Effect of biochar amendments on as and Pb mobility and phytoavailability in contaminated mine technosols phytoremediated by Salix. J. Geochem. Explor. 182, 149–156. doi:10.1016/j.gexplo.2016.11.016
Lebrun, M., Miard, F., Hattab-Hambli, N., Bourgerie, S., and Morabito, D. (2018). Assisted phytoremediation of a multi-contaminated industrial soil using biochar and garden soil amendments associated with Salix alba or Salix viminalis: abilities to stabilize As, Pb, and Cu. Water, Air, & Soil Pollut. 229, 163. doi:10.1007/s11270-018-3816-z
Lebrun, M., Miard, F., Nandillon, R., Hattab-Hambli, N., Léger, J., Scippa, G. S., et al. (2021b). Influence of biochar particle size and concentration on Pb and as availability in contaminated mining soil and phytoremediation potential of poplar assessed in a Mesocosm Experiment. Water, Air, & Soil Pollut. 232, 3–21. doi:10.1007/s11270-020-04942-y
Lehmann, J., and Joseph, S. (2015). Biochar for environmental management: science, technology and implementation. United Kingdom: Routledge.
Li, H., Zhang, J., Cao, Y., Liu, C., Li, F., Song, Y., et al. (2020a). Role of acid gases in Hg0 removal from flue gas over a novel cobalt-containing biochar prepared from harvested cobalt-enriched phytoremediation plant. Fuel Process. Technol. 207, 106478. doi:10.1016/j.fuproc.2020.106478
Li, J., Li, Q., Qian, C., Wang, X., Lan, Y., Wang, B., et al. (2019a). Volatile organic compounds analysis and characterization on activated biochar prepared from rice husk. Int. J. Environ. Sci. Technol. 16, 7653–7662. doi:10.1007/s13762-019-02219-4
Li, R., Deng, H., Zhang, X., Wang, J. J., Awasthi, M. K., Wang, Q., et al. (2019b). High-efficiency removal of Pb (II) and humate by a CeO2–MoS2 hybrid magnetic biochar. Bioresour. Technol. 273, 335–340. doi:10.1016/j.biortech.2018.10.053
Li, X., Xiao, J., Ma, C., Salam, M. M. A., Shi, J., and Chen, G. (2021a). The effect of particle size of bamboo biochar on the phytoremediation of Salix psammophila C. to multi-metal polluted soil. Int. J. Phytoremediation 23, 658–668. doi:10.1080/15226514.2020.1849012
Li, X., Xiao, J., Salam, M. M. A., Ma, C., and Chen, G. (2021b). Impacts of bamboo biochar on the phytoremediation potential of Salix psammophila grown in multi-metals contaminated soil. Int. J. Phytoremediation 23, 387–399. doi:10.1080/15226514.2020.1816893
Li, X., Yao, S., Bian, Y., Jiang, X., and Song, Y. (2020b). The combination of biochar and plant roots improves soil bacterial adaptation to PAH stress: insights from soil enzymes, microbiome, and metabolome. J. Hazard. Mater. 400, 123227. doi:10.1016/j.jhazmat.2020.123227
Li, Z., Jia, M., Christie, P., Ali, S., and Wu, L. (2018). Use of a hyperaccumulator and biochar to remediate an acid soil highly contaminated with trace metals and/or oxytetracycline. Chemosphere 204, 390–397. doi:10.1016/j.chemosphere.2018.04.061
Lian, F., and Xing, B. (2017). Black carbon (biochar) in water/soil environments: molecular structure, sorption, stability, and potential risk. Environ. Sci. Technol. 51, 13517–13532. doi:10.1021/acs.est.7b02528
Liang, G., Gong, W., Li, B., Zuo, J., Pan, L., and Liu, X. (2019). Analysis of heavy metals in foodstuffs and an assessment of the health risks to the general public via consumption in Beijing, China. Int. J. Environ. Res. Public Health 16, 909. doi:10.3390/ijerph16060909
Liang, S.-X., Jin, Y., Liu, W., Li, X., Shen, S.-G., and Ding, L. (2017). Feasibility of Pb phytoextraction using nano-materials assisted ryegrass: results of a one-year field-scale experiment. J. Environ. Manag. 190, 170–175. doi:10.1016/j.jenvman.2016.12.064
Lievens, C., Yperman, J., Vangronsveld, J., and Carleer, R. (2008). Study of the potential valorisation of heavy metal contaminated biomass via phytoremediation by fast pyrolysis: Part I. Influence of temperature, biomass species and solid heat carrier on the behaviour of heavy metals. Fuel 87, 1894–1905. doi:10.1016/j.fuel.2007.10.021
Lin, J., Sun, M., Su, B., Owens, G., and Chen, Z. (2019). Immobilization of cadmium in polluted soils by phytogenic iron oxide nanoparticles. Sci. Total Environ. 659, 491–498. doi:10.1016/j.scitotenv.2018.12.391
Liu, J., Ding, Y., Ma, L., Gao, G., and Wang, Y. (2017a). Combination of biochar and immobilized bacteria in cypermethrin-contaminated soil remediation. Int. Biodeterior. Biodegrad. 120, 15–20. doi:10.1016/j.ibiod.2017.01.039
Liu, L., Li, W., Song, W., and Guo, M. (2018). Remediation techniques for heavy metal-contaminated soils: principles and applicability. Sci. total Environ. 633, 206–219. doi:10.1016/j.scitotenv.2018.03.161
Liu, M., Lin, Z., Ke, X., Fan, X., Joseph, S., Taherymoosavi, S., et al. (2021). Rice seedling growth promotion by biochar varies with genotypes and application dosages. Front. Plant Sci. 12, 580462. doi:10.3389/fpls.2021.580462
Liu, W.-J., Jiang, H., and Yu, H.-Q. (2015a). Development of biochar-based functional materials: toward a sustainable platform carbon material. Chem. Rev. 115, 12251–12285. doi:10.1021/acs.chemrev.5b00195
Liu, W., Hou, J., Wang, Q., Yang, H., Luo, Y., and Christie, P. (2015b). Collection and analysis of root exudates of Festuca arundinacea L. and their role in facilitating the phytoremediation of petroleum-contaminated soil. Plant Soil 389, 109–119. doi:10.1007/s11104-014-2345-9
Liu, W., Huo, R., Xu, J., Liang, S., Li, J., Zhao, T., et al. (2017b). Effects of biochar on nitrogen transformation and heavy metals in sludge composting. Bioresour. Technol. 235, 43–49. doi:10.1016/j.biortech.2017.03.052
Logeshwaran, P., Megharaj, M., Chadalavada, S., Bowman, M., and Naidu, R. (2018). Petroleum hydrocarbons (PH) in groundwater aquifers: an overview of environmental fate, toxicity, microbial degradation and risk-based remediation approaches. Environ. Technol. innovation 10, 175–193. doi:10.1016/j.eti.2018.02.001
Lou, Y., Joseph, S., Li, L., Graber, E. R., Liu, X., and Pan, G. (2016). Water extract from straw biochar used for plant growth promotion: an initial test. BioResources 11, 249–266. doi:10.15376/biores.11.1.249-266
Lu, H., Li, Z., Fu, S., Méndez, A., Gascó, G., and Paz-Ferreiro, J. (2015). Combining phytoextraction and biochar addition improves soil biochemical properties in a soil contaminated with Cd. Chemosphere 119, 209–216. doi:10.1016/j.chemosphere.2014.06.024
Lu, K., Yang, X., Gielen, G., Bolan, N., Ok, Y. S., Niazi, N. K., et al. (2017). Effect of bamboo and rice straw biochars on the mobility and redistribution of heavy metals (Cd, Cu, Pb and Zn) in contaminated soil. J. Environ. Manag. 186, 285–292. doi:10.1016/j.jenvman.2016.05.068
Lu, K., Yang, X., Shen, J., Robinson, B., Huang, H., Liu, D., et al. (2014). Effect of bamboo and rice straw biochars on the bioavailability of Cd, Cu, Pb and Zn to Sedum plumbizincicola. Agric. Ecosyst. Environ. 191, 124–132. doi:10.1016/j.agee.2014.04.010
Lucchini, P., Quilliam, R., Deluca, T. H., Vamerali, T., and Jones, D. L. (2014). Does biochar application alter heavy metal dynamics in agricultural soil? Agric. Ecosyst. Environ. 184, 149–157. doi:10.1016/j.agee.2013.11.018
Lyu, J., Xiong, F., Sun, N., Li, Y., Liu, C., and Yin, S. (2021). Photosynthesis and related physiological parameters differences affected the isoprene emission rate among 10 typical tree species in subtropical metropolises. Int. J. Environ. Res. Public Health 18, 954. doi:10.3390/ijerph18030954
Ma, T., Zhou, L., Chen, L. K., Li, Z., Wu, L., Christie, P., et al. (2016). Oxytetracycline toxicity and its effect on phytoremediation by Sedum plumbizincicola and Medicago sativa in metal-contaminated soil. J. Agric. food Chem. 64, 8045–8053. doi:10.1021/acs.jafc.6b02140
Ma, Z., Chen, D., Gu, J., Bao, B., and Zhang, Q. (2015). Determination of pyrolysis characteristics and kinetics of palm kernel shell using TGA–FTIR and model-free integral methods. Energy Convers. Manag. 89, 251–259. doi:10.1016/j.enconman.2014.09.074
Mackie, K., Marhan, S., Ditterich, F., Schmidt, H., and Kandeler, E. 2015. The effects of biochar and compost amendments on copper immobilization and soil microorganisms in a temperate vineyard. Agric. Ecosys. Environ. 201, 58-69. doi:10.1016/j.agee.2014.12.001
Mahar, A., Wang, P., Ali, A., Awasthi, M. K., Lahori, A. H., Wang, Q., et al. (2016). Challenges and opportunities in the phytoremediation of heavy metals contaminated soils: a review. Ecotoxicol. Environ. Saf. 126, 111–121. doi:10.1016/j.ecoenv.2015.12.023
Manori, S., Shah, V., Soni, V., Dutta, K., and Daverey, A. (2021). Phytoremediation of cadmium-contaminated soil by Bidens pilosa L.: impact of pine needle biochar amendment. Environ. Sci. Pollut. Res. 28, 58872–58884. doi:10.1007/s11356-021-12953-3
Maroušek, J., Strunecký, O., and Stehel, V. (2019). Biochar farming: defining economically perspective applications. Clean Technol. Environ. Policy 21, 1389–1395. doi:10.1007/s10098-019-01728-7
Mehmood, S., Rizwan, M., Bashir, S., Ditta, A., Aziz, O., Yong, L. Z., et al. (2018). Comparative effects of biochar, slag and ferrous–Mn ore on lead and cadmium immobilization in soil. Bull. Environ. Contam. Toxicol. 100, 286–292. doi:10.1007/s00128-017-2222-3
Naja, G. M., and Volesky, B. (2017). “Toxicity and sources of Pb, Cd, Hg, Cr, As, and radionuclides in the environment,” in Handbook of advanced industrial and hazardous wastes management (United States: Crc Press).
Narayanan, M., and Ma, Y. (2022). Influences of biochar on bioremediation/phytoremediation potential of metal-contaminated soils. Front. Microbiol. 13, 929730. doi:10.3389/fmicb.2022.929730
Nguyen, T. T. N., Xu, C.-Y., Tahmasbian, I., Che, R., Xu, Z., Zhou, X., et al. (2017). Effects of biochar on soil available inorganic nitrogen: a review and meta-analysis. Geoderma 288, 79–96. doi:10.1016/j.geoderma.2016.11.004
Nie, C., Yang, X., Niazi, N. K., Xu, X., Wen, Y., Rinklebe, J., et al. (2018). Impact of sugarcane bagasse-derived biochar on heavy metal availability and microbial activity: a field study. Chemosphere 200, 274–282. doi:10.1016/j.chemosphere.2018.02.134
Niu, B., Chen, Z., and Xu, Z. (2017). Application of pyrolysis to recycling organics from waste tantalum capacitors. J. Hazard. Mater. 335, 39–46. doi:10.1016/j.jhazmat.2017.04.024
Özkan, A., Günkaya, Z., and Banar, M. (2016). Pyrolysis of plants after phytoremediation of contaminated soil with lead, cadmium and zinc. Bull. Environ. Contam. Toxicol. 96, 415–419. doi:10.1007/s00128-016-1746-2
Palansooriya, K. N., Yang, Y., Tsang, Y. F., Sarkar, B., Hou, D., Cao, X., et al. (2020). Occurrence of contaminants in drinking water sources and the potential of biochar for water quality improvement: a review. Crit. Rev. Environ. Sci. Technol. 50, 549–611. doi:10.1080/10643389.2019.1629803
Pandey, V. C., Abhilash, P., and Singh, N. (2009). The Indian perspective of utilizing fly ash in phytoremediation, phytomanagement and biomass production. J. Environ. Manag. 90, 2943–2958. doi:10.1016/j.jenvman.2009.05.001
Park, J. H., Choppala, G. K., Bolan, N. S., Chung, J. W., and Chuasavathi, T. (2011). Biochar reduces the bioavailability and phytotoxicity of heavy metals. Plant soil 348, 439–451. doi:10.1007/s11104-011-0948-y
Paz-Alberto, A. M., and Sigua, G. C. (2013). Phytoremediation: a green technology to remove environmental pollutants. Am. J. Clim. Change 2, 71–86. doi:10.4236/ajcc.2013.21008
Paz-Ferreiro, J., Lu, H., Fu, S., Mendez, A., and Gasco, G. (2014). Use of phytoremediation and biochar to remediate heavy metal polluted soils: a review. Solid Earth 5, 65–75. doi:10.5194/se-5-65-2014
Peng, Z., Liu, X., Chen, H., Liu, Q., and Tang, J. (2019). Characterization of ultraviolet-modified biochar from different feedstocks for enhanced removal of hexavalent chromium from water. Water Sci. Technol. 79, 1705–1716. doi:10.2166/wst.2019.170
Puga, A., Abreu, C., Melo, L., and Beesley, L. (2015). Biochar application to a contaminated soil reduces the availability and plant uptake of zinc, lead and cadmium. J. Environ. Manag. 159, 86–93. doi:10.1016/j.jenvman.2015.05.036
Rajkovich, S., Enders, A., Hanley, K., Hyland, C., Zimmerman, A. R., and Lehmann, J. (2012). Corn growth and nitrogen nutrition after additions of biochars with varying properties to a temperate soil. Biol. Fertil. Soils 48, 271–284. doi:10.1007/s00374-011-0624-7
Rathika, R., Srinivasan, P., Alkahtani, J., Al-Humaid, L., Alwahibi, M. S., Mythili, R., et al. (2021). Influence of biochar and EDTA on enhanced phytoremediation of lead contaminated soil by Brassica juncea. Chemosphere 271, 129513. doi:10.1016/j.chemosphere.2020.129513
Rathnayake, D., Rego, F., Van Poucke, R., Bridgwater, A. V., Mašek, O., Meers, E., et al. (2021). Chemical stabilization of Cd-contaminated soil using fresh and aged wheat straw biochar. Environ. Sci. Pollut. Res. 28, 10155–10166. doi:10.1007/s11356-020-11574-6
Ratnasari, I. F. D., Hadi, S. N., Suparto, S. R., Herliana, O., and Ahadiyat, Y. R. (2020). Phytoremediation of cadmium-contaminated soil using terrestrial kale (Ipomoea reptans Poir) and corncob biochar. J. Degraded Min. Lands Manag. 7, 2313–2318. doi:10.15243/jdmlm.2020.074.2313
Reddy, K. R., and Chirakkara, R. A. (2013). Green and sustainable remedial strategy for contaminated site: case study. Geotechnical Geol. Eng. 31, 1653–1661. doi:10.1007/s10706-013-9688-5
Redovniković, I. R., De Marco, A., Proietti, C., Hanousek, K., Sedak, M., Bilandžić, N., et al. (2017). Poplar response to cadmium and lead soil contamination. Ecotoxicol. Environ. Saf. 144, 482–489. doi:10.1016/j.ecoenv.2017.06.011
Rees, F., Germain, C., Sterckeman, T., and Morel, J.-L. (2015). Plant growth and metal uptake by a non-hyperaccumulating species (Lolium perenne) and a Cd-Zn hyperaccumulator (Noccaea caerulescens) in contaminated soils amended with biochar. Plant Soil 395, 57–73. doi:10.1007/s11104-015-2384-x
Rees, F., Simonnot, M.-O., and Morel, J.-L. (2014). Short-term effects of biochar on soil heavy metal mobility are controlled by intra-particle diffusion and soil pH increase. Eur. J. Soil Sci. 65, 149–161. doi:10.1111/ejss.12107
Rees, F., Sterckeman, T., and Morel, J. L. (2016). Root development of non-accumulating and hyperaccumulating plants in metal-contaminated soils amended with biochar. Chemosphere 142, 48–55. doi:10.1016/j.chemosphere.2015.03.068
Regkouzas, P., and Diamadopoulos, E. (2019). Adsorption of selected organic micro-pollutants on sewage sludge biochar. Chemosphere 224, 840–851. doi:10.1016/j.chemosphere.2019.02.165
Rizwan, M., Ali, S., Rizvi, H., Rinklebe, J., Tsang, D. C., Meers, E., et al. (2016). Phytomanagement of heavy metals in contaminated soils using sunflower: a review. Crit. Rev. Environ. Sci. Technol. 46, 1498–1528. doi:10.1080/10643389.2016.1248199
Rodríguez-Vila, A., Covelo, E. F., Forján, R., and Asensio, V. (2014). Phytoremediating a copper mine soil with Brassica juncea L., compost and biochar. Environ. Sci. Pollut. Res. 21, 11293–11304. doi:10.1007/s11356-014-2993-6
Roohi, R., Jafari, M., Jahantab, E., Aman, M. S., Moameri, M., and Zare, S. (2020). Application of artificial neural network model for the identification the effect of municipal waste compost and biochar on phytoremediation of contaminated soils. J. Geochem. Explor. 208, 106399. doi:10.1016/j.gexplo.2019.106399
Salam, M. M. A., Mohsin, M., Kaipiainen, E., Villa, A., Kuittinen, S., Pulkkinen, P., et al. (2019). Biomass growth variation and phytoextraction potential of four Salix varieties grown in contaminated soil amended with lime and wood ash. Int. J. phytoremediation 21, 1329–1340. doi:10.1080/15226514.2019.1633257
Shah, V., and Daverey, A. (2020). Phytoremediation: a multidisciplinary approach to clean up heavy metal contaminated soil. Environ. Technol. Innovation 18, 100774. doi:10.1016/j.eti.2020.100774
Shaheen, S. M., Mosa, A., Natasha, N., Soundari, P., Hassan, N. E., Yang, X., et al. (2023). Pros and cons of biochar to soil potentially toxic element mobilization and phytoavailability: environmental implications. Earth Syst. Environ. 7, 321–345. doi:10.1007/s41748-022-00336-8
Simiele, M., Lebrun, M., Miard, F., Trupiano, D., Poupart, P., Forestier, O., et al. (2020). Assisted phytoremediation of a former mine soil using biochar and iron sulphate: effects on as soil immobilization and accumulation in three Salicaceae species. Sci. Total Environ. 710, 136203. doi:10.1016/j.scitotenv.2019.136203
Singh, J., and Lee, B.-K. (2016). Influence of nano-TiO2 particles on the bioaccumulation of Cd in soybean plants (Glycine max): a possible mechanism for the removal of Cd from the contaminated soil. J. Environ. Manag. 170, 88–96. doi:10.1016/j.jenvman.2016.01.015
Song, Y., Li, Y., Zhang, W., Wang, F., Bian, Y., Boughner, L. A., et al. (2016). Novel biochar-plant tandem approach for remediating hexachlorobenzene contaminated soils: proof-of-concept and new insight into the rhizosphere. J. Agric. food Chem. 64, 5464–5471. doi:10.1021/acs.jafc.6b01035
Sun, W., Zhang, S., and Su, C. (2018). “Impact of biochar on the bioremediation and phytoremediation of heavy metal (loid) s in soil,” in Advances in bioremediation and phytoremediation (United States: IntechOpen), 149. doi:10.5772/intechopen.70349
Taskin, E., De Castro Bueno, C., Allegretta, I., Terzano, R., Rosa, A. H., and Loffredo, E. (2019). Multianalytical characterization of biochar and hydrochar produced from waste biomasses for environmental and agricultural applications. Chemosphere 233, 422–430. doi:10.1016/j.chemosphere.2019.05.204
Tomczyk, A., Sokołowska, Z., and Boguta, P. (2020). Biochar physicochemical properties: pyrolysis temperature and feedstock kind effects. Rev. Environ. Sci. Bio/Technology 19, 191–215. doi:10.1007/s11157-020-09523-3
Tu, C., Wei, J., Guan, F., Liu, Y., Sun, Y., and Luo, Y. (2020). Biochar and bacteria inoculated biochar enhanced Cd and Cu immobilization and enzymatic activity in a polluted soil. Environ. Int. 137, 105576. doi:10.1016/j.envint.2020.105576
Ur Rehman, M. Z., Rizwan, M., Ali, S., Ok, Y. S., Ishaque, W., Nawaz, M. F., et al. (2017). Remediation of heavy metal contaminated soils by using Solanum nigrum: a review. Ecotoxicol. Environ. Saf. 143, 236–248. doi:10.1016/j.ecoenv.2017.05.038
Wang, L., Gao, B., Li, Y., Ok, Y. S., Shen, C., and Xue, S. (2017b). Biochar provides a safe and value-added solution for hyperaccumulating plant disposal: a case study of Phytolacca acinosa Roxb (Phytolaccaceae). Chemosphere 178, 59–64. doi:10.1016/j.chemosphere.2017.02.121
Wang, L., Ji, B., Hu, Y., Liu, R., and Sun, W. (2017a). A review on in situ phytoremediation of mine tailings. Chemosphere 184, 594–600. doi:10.1016/j.chemosphere.2017.06.025
Wang, X., Li, L., Yan, X., Meng, X., and Chen, Y. (2020). Processes of chromium (VI) migration and transformation in chromate production site: a case study from the middle of China. Chemosphere 257, 127282. doi:10.1016/j.chemosphere.2020.127282
Wei, L., Liang, S., Guho, N. M., Hanson, A. J., Smith, M. W., Garcia-Perez, M., et al. (2015). Production and characterization of bio-oil and biochar from the pyrolysis of residual bacterial biomass from a polyhydroxyalkanoate production process. J. Anal. Appl. Pyrolysis 115, 268–278. doi:10.1016/j.jaap.2015.08.005
Williams, E. K., Jones, D. L., Sanders, H. R., Benitez, G. V., and Plante, A. F. (2019). Effects of 7 years of field weathering on biochar recalcitrance and solubility. Biochar 1, 237–248. doi:10.1007/s42773-019-00026-1
Wu, F., Yang, W., Zhang, J., and Zhou, L. (2010). Cadmium accumulation and growth responses of a poplar (Populus deltoids× Populus nigra) in cadmium contaminated purple soil and alluvial soil. J. Hazard. Mater. 177, 268–273. doi:10.1016/j.jhazmat.2009.12.028
Xiao, R., Shen, F., Du, J., Li, R., Lahori, A. H., and Zhang, Z. (2018). Screening of native plants from wasteland surrounding a Zn smelter in Feng County China, for phytoremediation. Ecotoxicol. Environ. Saf. 162, 178–183. doi:10.1016/j.ecoenv.2018.06.095
Xiao, R., Sun, X., Wang, J., Feng, J., Li, R., Zhang, Z., et al. (2015). Characteristics and phytotoxicity assay of biochars derived from a Zn-rich antibiotic residue. J. Anal. Appl. pyrolysis 113, 575–583. doi:10.1016/j.jaap.2015.04.006
Xie, T., Reddy, K. R., Wang, C., Yargicoglu, E., and Spokas, K. (2015). Characteristics and applications of biochar for environmental remediation: a review. Crit. Rev. Environ. Sci. Technol. 45, 939–969. doi:10.1080/10643389.2014.924180
Xu, P., Sun, C.-X., Ye, X.-Z., Xiao, W.-D., Zhang, Q., and Wang, Q. (2016). The effect of biochar and crop straws on heavy metal bioavailability and plant accumulation in a Cd and Pb polluted soil. Ecotoxicol. Environ. Saf. 132, 94–100. doi:10.1016/j.ecoenv.2016.05.031
Xu, W., Adewuyi, Y. G., Liu, Y., and Wang, Y. (2018a). Removal of elemental mercury from flue gas using CuOx and CeO2 modified rice straw chars enhanced by ultrasound. Fuel Process. Technol. 170, 21–31. doi:10.1016/j.fuproc.2017.10.017
Xu, Y., Seshadri, B., Sarkar, B., Wang, H., Rumpel, C., Sparks, D., et al. (2018b). Biochar modulates heavy metal toxicity and improves microbial carbon use efficiency in soil. Sci. total Environ. 621, 148–159. doi:10.1016/j.scitotenv.2017.11.214
Yang, W., Liu, Y., Wang, Q., and Pan, J. (2017a). Removal of elemental mercury from flue gas using wheat straw chars modified by Mn-Ce mixed oxides with ultrasonic-assisted impregnation. Chem. Eng. J. 326, 169–181. doi:10.1016/j.cej.2017.05.106
Yang, W., Zhou, X., Tie, B., Peng, L., Li, H., Wang, K., et al. (2017b). Comparison of three types of oil crop rotation systems for effective use and remediation of heavy metal contaminated agricultural soil. Chemosphere 188, 148–156. doi:10.1016/j.chemosphere.2017.08.140
Yao, Q., Liu, J., Yu, Z., Li, Y., Jin, J., Liu, X., et al. (2017). Three years of biochar amendment alters soil physiochemical properties and fungal community composition in a black soil of northeast China. Soil Biol. Biochem. 110, 56–67. doi:10.1016/j.soilbio.2017.03.005
Yavari, S., Malakahmad, A., and Sapari, N. B. (2015). Biochar efficiency in pesticides sorption as a function of production variables—a review. Environ. Sci. Pollut. Res. 22, 13824–13841. doi:10.1007/s11356-015-5114-2
Yrjälä, K., and Lopez-Echartea, E. (2021). “Structure and function of biochar in remediation and as carrier of microbes,” in Advances in chemical pollution, environmental management and protection (Netherlands: Elsevier).
Zanganeh, F., Heidari, A., Sepehr, A., and Rohani, A. (2022). Bioaugmentation and bioaugmentation–assisted phytoremediation of heavy metal contaminated soil by a synergistic effect of cyanobacteria inoculation, biochar, and purslane (Portulaca oleracea L). Environ. Sci. Pollut. Res. 29, 6040–6059. doi:10.1007/s11356-021-16061-0
Zhang, C., Wang, J., Bai, S. H., Zhang, Y., Teng, Y., and Xu, Z. (2019b). Assisted phytoremediation of a co-contaminated soil with biochar amendment: contaminant removals and bacterial community properties. Geoderma 348, 115–123. doi:10.1016/j.geoderma.2019.04.031
Zhang, C., Wang, W., Duan, A., Zeng, G., Huang, D., Lai, C., et al. (2019a). Adsorption behavior of engineered carbons and carbon nanomaterials for metal endocrine disruptors: experiments and theoretical calculation. Chemosphere 222, 184–194. doi:10.1016/j.chemosphere.2019.01.128
Zhang, M., Wang, J., Bai, S. H., Teng, Y., and Xu, Z. (2018). Evaluating the effects of phytoremediation with biochar additions on soil nitrogen mineralization enzymes and fungi. Environ. Sci. Pollut. Res. 25, 23106–23116. doi:10.1007/s11356-018-2425-0
Zhang, M., Wang, W., Wang, J., Teng, Y., and Xu, Z. (2017). Dynamics of biochemical properties associated with soil nitrogen mineralization following nitrification inhibitor and fungicide applications. Environ. Sci. Pollut. Res. 24, 11340–11348. doi:10.1007/s11356-017-8762-6
Zhang, X., Sarmah, A. K., Bolan, N. S., He, L., Lin, X., Che, L., et al. (2016). Effect of aging process on adsorption of diethyl phthalate in soils amended with bamboo biochar. Chemosphere 142, 28–34. doi:10.1016/j.chemosphere.2015.05.037
Zhang, Y., Chen, Z., Chen, C., Li, F., and Shen, K. (2021). Effects of UV-modified biochar derived from phytoremediation residue on Cd bioavailability and uptake in Coriandrum sativum L. in a Cd-contaminated soil. Environ. Sci. Pollut. Res. 28, 17395–17404. doi:10.1007/s11356-020-11931-5
Zhang, Y., Chen, Z., Xu, W., Liao, Q., Zhang, H., Hao, S., et al. (2020). Pyrolysis of various phytoremediation residues for biochars: chemical forms and environmental risk of Cd in biochar. Bioresour. Technol. 299, 122581. doi:10.1016/j.biortech.2019.122581
Zhao, T., Zhang, K., Chen, J., Shi, X., Li, X., Ma, Y., et al. (2019). Changes in heavy metal mobility and availability in contaminated wet-land soil remediated using lignin-based poly (acrylic acid). J. Hazard. Mater. 368, 459–467. doi:10.1016/j.jhazmat.2019.01.061
Zhen, M., Chen, H., Liu, Q., Song, B., Wang, Y., and Tang, J. (2019). Combination of rhamnolipid and biochar in assisting phytoremediation of petroleum hydrocarbon contaminated soil using Spartina anglica. J. Environ. Sci. 85, 107–118. doi:10.1016/j.jes.2019.05.013
Zheng, R.-L., Cai, C., Liang, J.-H., Huang, Q., Chen, Z., Huang, Y.-Z., et al. (2012). The effects of biochars from rice residue on the formation of iron plaque and the accumulation of Cd, Zn, Pb, as in rice (Oryza sativa L.) seedlings. Chemosphere 89, 856–862. doi:10.1016/j.chemosphere.2012.05.008
Keywords: pollutants, biochar, phytoremediation, environment, stabilization, extraction
Citation: Boorboori MR and Lackóová L (2023) Biochar; an effective factor in improving phytoremediation of metal(iod)s in polluted sites. Front. Environ. Sci. 11:1253144. doi: 10.3389/fenvs.2023.1253144
Received: 05 July 2023; Accepted: 11 October 2023;
Published: 19 October 2023.
Edited by:
Paula Pérez-Rodríguez, University of Vigo, SpainReviewed by:
Milan Skalicky, Czech University of Life Sciences Prague, CzechiaLuis Lopez, Universidad Mayor de San Andres, Bolivia
Copyright © 2023 Boorboori and Lackóová. This is an open-access article distributed under the terms of the Creative Commons Attribution License (CC BY). The use, distribution or reproduction in other forums is permitted, provided the original author(s) and the copyright owner(s) are credited and that the original publication in this journal is cited, in accordance with accepted academic practice. No use, distribution or reproduction is permitted which does not comply with these terms.
*Correspondence: Mohammad Reza Boorboori, Ym9vcmJvb3JpQGFoc3p1LmVkdS5jbg==; Lenka Lackóová, bGVua2EubGFja29vdmFAdW5pYWcuc2s=